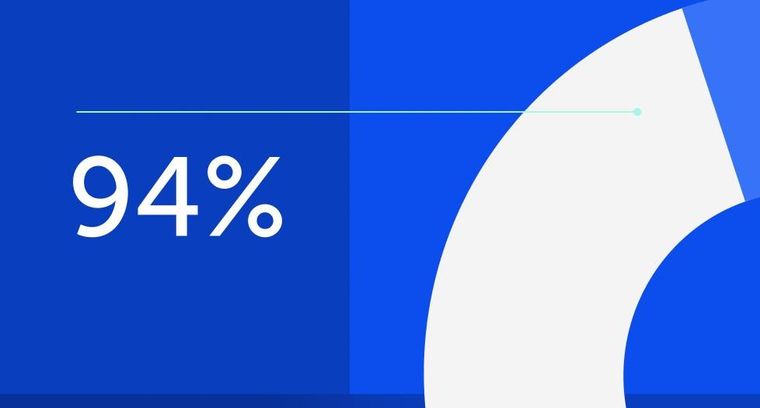
94% of researchers rate our articles as excellent or good
Learn more about the work of our research integrity team to safeguard the quality of each article we publish.
Find out more
REVIEW article
Front. Immunol., 03 October 2022
Sec. Vaccines and Molecular Therapeutics
Volume 13 - 2022 | https://doi.org/10.3389/fimmu.2022.961198
In December 2019, an outbreak emerged of severe acute respiratory syndrome coronavirus 2 (SARS-CoV-2) which leads to coronavirus disease 2019 (COVID-19). The World Health Organisation announced the outbreak a global health emergency on 30 January 2020 and by 11 March 2020 it was declared a pandemic. The spread and severity of the outbreak took a heavy toll and overburdening of the global health system, particularly since there were no available drugs against SARS-CoV-2. With an immediate worldwide effort, communication, and sharing of data, large amounts of funding, researchers and pharmaceutical companies immediately fast-tracked vaccine development in order to prevent severe disease, hospitalizations and death. A number of vaccines were quickly approved for emergency use, and worldwide vaccination rollouts were immediately put in place. However, due to several individuals being hesitant to vaccinations and many poorer countries not having access to vaccines, multiple SARS-CoV-2 variants quickly emerged that were distinct from the original variant. Uncertainties related to the effectiveness of the various vaccines against the new variants as well as vaccine specific-side effects have remained a concern. Despite these uncertainties, fast-track vaccine approval, manufacturing at large scale, and the effective distribution of COVID-19 vaccines remain the topmost priorities around the world. Unprecedented efforts made by vaccine developers/researchers as well as healthcare staff, played a major role in distributing vaccine shots that provided protection and/or reduced disease severity, and deaths, even with the delta and omicron variants. Fortunately, even for those who become infected, vaccination appears to protect against major disease, hospitalisation, and fatality from COVID-19. Herein, we analyse ongoing vaccination studies and vaccine platforms that have saved many deaths from the pandemic.
The COVID-19 pandemic had spread to 228 countries as of September 15, 2022 (plus outbreaks in 2 cruise ships), with over 615.5 million laboratory-confirmed cases and 6.52 million deaths causing considerable social and economic devastation. The main strategy used against COVID-19 is to alleviate symptoms, hospitalisations and deaths. As such, several therapeutic approaches are being evaluated for their effectiveness in reducing/eliminating SARS-CoV-2 viral load and reducing symptoms (1–4). Initially, the strategy was to repurpose existing therapeutics to allow for faster drug development (2, 5, 6). Several clinical trials on existing drugs have been completed, and others are in progress (4, 7–9). Despite the numerous approaches, there have been repeated cases where recovered patients are reinfected, highlighting the need for herd immunity against SARS-CoV-2 (10). However, drugs such as molnupiravir, bamlanivimab, bebtelovimab, and tixagevimab with cilgavimab work well and are predominantly used in elderly and high-risk patients (2, 3, 11). Patients with comorbidities such as cardiovascular disease, chronic respiratory disease, hypertension, type-2 diabetes, and cancer may be affected with unfavorable outcomes (12). Furthermore, the emergence of monkey pox, Langya virus and tomato flu a manifestation of hand, foot and mouth diseases complicates the COVID-19 management process (13–16). SARS-CoV-2 manifestations are more serious in the elderly and those with chronic diseases, owing to the overactive and persistent innate inflammatory processes and anatomical and functional alterations in the physiological systems (17). This will impede not only the capability to defend against respiratory infections but also the ability to build efficient vaccination defense. According to Elizabeth C Stahl (18), “The function of lymphoid and nonlymphoid tissues involved in the host immune response declines with age. The production of naive T and B-lymphocytes is decreased when primary lymphoid organs degenerate, resulting in diminished transition to secondary lymphoid organs and antigen encounter sites. In addition, proinflammatory cells and mediators may accumulate in the lungs and extrapulmonary organs. Protective immunity to vaccination is low or faulty in elderly individuals, while autoimmunity rises. As a result, while developing SARS-CoV-2 vaccines, it will be crucial to keep in mind that older individuals may not react to vaccinations as well as younger people (19).”
The viral structural proteins and genomic organization is summarized in Figure 1. The immune system plays a critical role in the pathogenesis of COVID-19 as well as vaccine effectiveness. To design a safe and effective vaccine, preclinical and clinical trials are conducted with caution to minimise adverse reactions (20). Herein, we discuss different targets and platforms that are currently being used as COVID-19 vaccine candidates, the effects of variants on vaccine efficacy, as well as the issues connected with fast-tracking of vaccines and vaccination ethics (21).
Viral mutations arise when the genetic sequences of the genome that make up a virus change. This virus survival instinct of generating thousands of copies of themselves over time leads to errors in the genetic fingerprint as a part of the natural process. These random genetic drifts may lead to no change or may increase or decrease the virulent capability to spread or cause diseases (22, 23). While maximum viral mutations in the viral genome are mostly point mutations with a deleted arm or neutral mutations, a small percentage will alter functional viral parts and may modify contagiousness or infection severity. The viral genomic evolution or SARS-CoV-2 remained largely unchanged for approximately 11 months after its emergence in late 2019. Later, in 2020 and thereafter, genomic evolution was pigeonholed by the advent mutation series, and what emerged was what is commonly known as ‘variants of concern (VOC)’ (Figure 2). These mutations affect some virus attributes, as well as antigenicity and transmissibility. There is unfavorable evidence of the lowered neutralization capability of some coronavirus variants against the vaccine, which may lead to many uncertainties in effective vaccine development. A possible solution to this is by updating the vaccine sequences considering major mutations of the emerging variants and their interrelationships (24).
Figure 2 SARS-CoV-2 mutations and viral variants. (A) Radial graph of emerging SARS-CoV-2 variants from December 2021 to August 2022 (built with nextstrain/ncov). (B) Frequencies of clades from March 2022 to August 2022 (from nextstrain.org), and (C) classification of SARS-CoV-2 omicron variant based on a clade tree (created with Biorender.com).
The World Health Organization (WHO) demonstrated three types of SARS-CoV-2 variants in collaboration with a SARS-CoV-2 interagency group as (i) variant of interest (VOI), (ii) variant of concern (VOC), and (iii) variant of high consequence (VOHC). VOIs include zeta (P.2), theta (P.3), epsilon (B.1.427, B.1.429), kappa (B.1.617.1), iota (B.1.526), eta (B.1.525), lambda (C.37) and the mu variant (B.1621) (25). The WHO changed the designation of the following VOCs to, ‘previously circulating VOCs’ - the alpha variant (B.1.1.7), beta (B.1.351), gamma variant (P.1), and delta variant (B.1.617.2) (26). The omicron is the current VOC and some sub-variants are classified as, ‘under monitoring’ (B.1.1.529, BA.1, BA.1.1, BA.2, BA.2.12.1, BA.2.75, BA.3, BA.4, and BA.5) (Table 1). No SARS-CoV-2 variants have been classified as VOHC (22, 39).
Most variations in the structure of viruses occurs due to mutation in the genetic material, but recently, due to recombination of two different variants or sub variants, the generation of recombinant variants have been detected. Recombinant variants, also known as hybrid variants, surfaced due to the transfer of genetic material between two viral variants. Recently, in some countries, such as France and Europe, very few cases of recombinant variants have been observed. The WHO designated these variants as XE and XF (Figure 3). Early 2022, a new recombinant variant referred to as “deltacron”, also known as XD was identified which is the combination of omicron and delta variants. An infection of both variants in a single patient was observed in France in January 2022 (40). The delta variant is the most dangerous variant among all variants of COVID-19. The delta variant is responsible for most deaths across the world, and omicron is the most infectious variant among all variants. Deltacron is known as BA.1 x AY.4, where BA.1 is an omicron variant and AY.4 is a delta variant (41). In the United Kingdom, a combination of two subvariants named BA.1 and BA.2 (also known as stealth omicron) of the omicron variant was noted in one patient (XE variant). In the case of the XE variant, most of its structure, including the spike protein, is of the BA.2 sub variant, but the 5′ part of its structure is made up of the BA.1 sub variant (42). In addition, XF, is the result of the combination of BA.1 subvariant of omicron and delta variants (43). Currently, five main sublineages of omicron variants have been detected (BA.1 to BA.5). The BA.4 and BA.5 lineages managed to dominate the planet between April-July 2022 since their emergence, whereas BA.2 began to dwindle at almost the same period (44).
Figure 3 Hybrid variants of SARS-CoV-2 (Created with BioRender.com).
Compared to other RNA viruses, the activation and variability of the SARS-CoV-2 3’-5’ exonuclease during viral genome transcription can lead to changes in non-structural protein (nsp)14 (45). Even a single point mutation may impact viral proteins and their physicochemical nature, which could consequently modify the binding affinity of the virus with host cells (46, 47). Importantly, the emergence of these amino acid mutations may result in an antigenic drift that reduces the neutralization ability of the immune serum from vaccines, causing a new wave of mutations of the SARS-CoV-2 spike protein. Despite the fact that the cumulative mutation rates of the SARS-CoV-2 genome are lower than those of influenza and HIV-1 viruses, the current advent of a spreading mutant strain has raised concerns regarding the efficacy of COVID-19 vaccines (48).
The priority for vaccine development over anti-SARS-CoV-2 drug development is due to the intention to generate herd protection (49). With a worldwide effort, sharing of data, availability of large funding schemes and collaboration with industry, vaccines quickly became available and rolled out for emergency use. Both classic and novel platforms have been utilized (50, 51). Classic platforms used include virus-based vaccines (dead virus or live-attenuated virus) and protein-based vaccines; novel platforms include peptide, DNA, mRNA, viral vector and virus-like particle-based vaccines (52). Except for live-attenuated viral vaccines, all others require the presence of an adjuvant to enhance the antigenicity of the antigen (49, 53). The fact that most of the initial approved vaccines against SARS-CoV-2 that were rolled out were developed using novel platforms because these platforms are highly flexible and require less time for production than traditional methods (49, 54). Despite the novelty of the platforms deployed, the basic goal remains the same, i.e., the development of a safe and efficacious vaccine (Figure 4).
Figure 4 COVID-19 vaccine platforms used. (Some elements are created with BioRender.com).
Messenger ribonucleic acid (mRNA) vaccines contain strands of desirable “transcripts of interest” inside a lipid nanoparticle (55). This coating protects mRNA against enzymatic degradation and aids entry into antigen-presenting cells in the lymph node near the site of injection. Once they enter the cell, mRNA is translated into protein and expressed as antigenic fragments on the surface to induce humoral (antibody) and cellular immunity (55–57).
The latest studies and technology breakthroughs (e.g., codon optimization, nucleotide alteration, and comprehending the significance of the 5’-untranslated region; UTR) have permitted mRNA to emerge as a viable therapeutic tool in the field of vaccine production by mitigating downsides such as instability and immunogenicity (55, 58). Currently, there are 45 mRNA vaccines in the clinical development phase, and 3 of these vaccines are approved for emergency use by various countries (59–61).
The use of mRNA has numerous beneficial features over other vaccine platforms. First, mRNA is a safe platform. It is non-infectious, as only part of the antigenic protein is produced. Because it does not penetrate the nucleus, there is no danger of insertional mutagenesis. In addition, it is destroyed using regular cellular activities, and the in vivo half-life may be controlled (58, 62, 63). Furthermore, the inherent antigenicity of mRNA may be reduced to alleviate the safety risk (58, 64, 65). The second distinguishing trait is its great effectiveness. During phase 3 studies, Spikevax (mRNA-1273) and Comirnaty (BNT162b2) showed effectiveness of 94.1% and 94.6%, respectively (66, 67). Extracellular RNases have a strong affinity for naked mRNA (68). As a result, lipid nanoparticles and the creation of modified nucleosides are required to enable mRNA entry into cells (57, 64, 65). Even though mRNA vaccines induce cellular as well as humoral immunity, multidose administration is required for effective protection. Importantly, no anti-vector immunity is generated. The third favorable feature is the scope of rapid, low-cost, and scalable manufacturing (56, 57, 69, 70). Last, it is easy to design and develop a new mRNA vaccine from an existing vaccine. Various antigens comprising the S1 subunit, the N-terminal region of the S protein and the receptor binding domain (RBD) have been used (71, 72). Nevertheless, it remains to be shown whether these antigen candidates are appropriate for inclusion in an mRNA vaccine to prevent COVID-19 in the long term.
mRNA-1273 was shown to stimulate T-helper (Th)-1 immune responses following the first dose, as 0.05% of circulatory CD4+ T cells were shown to secrete tumor necrosis factor (TNF)-alpha and/or interleukin (IL)-2 (73). After just one dose, both mRNA-1273 and BNT162b2 vaccinations generated levels of anti-RBD antibodies that were comparable to or higher than those seen in patients receiving convalescent plasma treatment However, CD8+ T-cell responses were induced only at modest levels. These findings show that protection following a single dose will have a very low neutralizing antibody (nAb) titre to provide sufficient protection against infection (74). Vaccines from Pfizer, Moderna, and AstraZeneca have all been shown to activate type I interferon (IFN), which could lead to pathogen-agnostic immunity (57, 75, 76).
Neutralizing antibody responses following one BNT162b2 immunization, in patients with cancer, was shown to be 67% which was further increased after the second vaccine dose. Spike protein-specific serum antibodies and T cells showed similar patterns in healthy controls, however, the magnitude was lower in cancer patients. Memory B-cell subsets were also noted to be potential predictors of anamnestic reactions to further immunizations in most cancer patients. Following these findings, a phase 1 trial of a third BNT162b2 injection was conducted in 20 participants with cancer (NCT04936997); the primary outcome was induction of immune responses. In sixteen subjects, a threefold increase in nAb responses one week following the third immunization were noted (77). However, T-cell responses remained unchanged following a third dose. In addition, sera of BNT162b2-vaccinated medical workers were found to efficiently neutralize the SARS-CoV-2 variation with the D614G substitution and the B.1.1.7 variant after the second dosage; however, the neutralization of the B.1.351 variant was decreased fivefold (78). Further, human breast milk contains humoral and cellular immune responses following mRNA injections. Antibodies to SARS-CoV-2 spike variants may be neutralized by milk anti-RBD antibodies. Nursed babies are given anti-RBD antibodies, in order to induce passive immunity and protection against SARS-CoV-2 (79). A booster dose with mRNA vaccine demonstrated better protection against the omicron variant (80). Furthermore, SARS-CoV-2-specific memory B-cell responses following the first dose of viral vector-based vaccines (ChAdOx1 nCov-19) may be stimulated by a booster with the mRNA-1273 vaccine (81). In a group of hospital workers who were administered two doses of either mRNA-1273 or BNT162b2 (82), vaccinations showed different effects on Fc-mediated effector functions and epitope-specific antibody responses. Depletion of RBD-specific antibodies emphasized these variations even further. These findings implied that mRNA-1273 and BNT162b2 induce different immune responses despite having identical chemical compositions. The third (booster) dose of BNT162b2 has been noted to reduce the number of confirmed infections, severity of sickness, hospitalisations, and death against several VOCs.
A conventional technique of inactivating the whole pathogen with physical (thermal stimuli, ultraviolet light) and/or chemical treatment followed by a purification process is a proven tool that has been deployed for decades for effective protection against a wide range of diseases, such as, polio, rabies, pertussis, influenza (83, 84) Inactivated viruses cannot produce or replicate and thus, do not produce any pathological complications but are still capable of producing an immunogenic response. Inactivated vaccines show little or no effect on cell-mediated immunity (84, 85). Thus, the effective immune response develops only after the second and/or booster dose. Favorable features of this platform include a high level of safety, reasonable efficacy, and ease of large-scale production (86–88). Additionally, logistical challenges are less stringent for such vaccines. However, inactivated pathogens are always formulated with the addition of immune potentiators (e.g., aluminum preparations) (84, 89). Several precautions in manufacturing (Bio-Safety Level 3) need to be followed. Currently, there are 21 inactive vaccines in the clinical development phase (59–61). CoronaVac and covaxin have shown 50-81% efficacy in several studies (85, 90). Covaxin has also been reported to induce nAbs against the alpha variant (90, 91). A prospective observational study among covaxin recipients was carried out in a tertiary care facility in India between June 28 and September 6, 2021. The study reported at least one adverse event following immunization (AEFI) in 29.8% of individuals. There were no severe adverse events reported, and 1.6% suffered moderate AEFI. The individuals frequently reported myalgia (59%) and pain at the injection site (14.6%, 9.7%). When compared with the second dose (26.4%), the incidence of AEFI after the initial dose was higher (38.1%). Additionally, the study concluded that female sex, history of an allergic reaction, presence of comorbidities, acute infection in the past three months, and use of chronic drugs were the main risk factors for AEFI (92).
Live-attenuated vaccines contain a variety of living pathogens that have been weakened under laboratory conditions (93). Live-attenuated vaccines are developed by techniques such as repeated subculturing and codon pair deoptimisation (94). MMR (mumps, measles, and rubella), polio, and chickenpox vaccines are some examples that have been used for decades. The more resemblance to the original pathogen, the better the immune response generated. Live-attenuated viruses are capable of replicating themselves within cells, but their virulence power is reduced (93, 95). Because of the capability of replication within cells, live-attenuated vaccines have the ability to generate strong cell-mediated immunity in addition to humoral immunity, which is desirable in viral infections (96). Continual antigenic stimuli produced by live attenuated vaccines are sufficient to induce memory cells. They are less safe than inactivated vaccines, as the weakened pathogen can rarely revert to its original wild-type form and can lead to serious adverse events or disease itself (95, 96). Care during cold storage and reconstitution (in the case of lyophilised vaccines) must be taken; otherwise, potential immunization errors can occur (93). A series of live-attenuated vaccine candidates for SARS_CoV-1 (known as SARS) and Middle Eastern respiratory syndrome (MERS, MERS-CoV) were developed and showed good immunogenic responses (97–102). Despite the huge potential of live-attenuated vaccines in terms of generating strong immunogenic responses, only 2 candidates are currently in clinical trials (59). COVI-VAC is one such example.
Protein subunit vaccines contain purified small antigenic proteins instead of the whole pathogen. These fragments are usually proteins but can also be polysaccharides (as in the case of some bacterial vaccines). In order to generate strong immune responses, polysaccharide fractions are usually conjugated with proteins. Protein-based vaccines are considered safe and contain purified fragments that are not capable of producing disease. However, they are less capable of inducing long-lasting immunogenic responses. Their limited ability to generate optimal cellular responses can be enhanced by the addition of immune potentiators and the use of novel delivery systems (103–105). Some protein-based vaccines also require booster doses to produce effective protection against the pathogen. Hepatitis B, pneumococcal, and pertussis vaccines are some examples of protein-based vaccines.
The domains of the S protein (both S1 and S2 subunits) of the SARS-CoV-2 virus are considered important for hACE2 receptor-mediated endocytosis for entry into human cells (104, 106). Therefore, the administration of purified S protein of SARS-CoV-2 and its fractional RBD for the development of nAbs and cellular immunity was thoroughly studied (107). NVX-CoV2373, SCB-2019, and ZF2001 vaccines are some examples being developed using this approach (108, 109). Another potential target, the N protein, is expressed in a stable and abundant amount during SARS-CoV-2 infection and is highly immunogenic (110). Coexpression of the recombinant spike, membrane, and envelope proteins have been explored (111). There are sixty-nine candidate vaccines, of which thirty-two are in phase 3 human clinical trials (59–61). One example is NVX-CoV2373 (known as Novavax; sold under the name of Nuvaxovid and Covovax), which showed 86.3% efficacy against alpha variant and 96.4% against other non-alpha variants (112). In another phase 3 study, NVX-CoV2373 showed some local effects such as tenderness, pain, fatigue with low medically attended adverse events and serious adverse events and no episodes of anaphylaxis. When combined with flu vaccine, the efficacy of the NVX-CoV2373 vaccine decreased from 89.8 in NVX-CoV2373 alone group compared to 87.5% efficacy when combined with flu vaccine (112). The NVX-CoV2373 vaccine, displayed cross-reactive immune responses against omicron (B.1.1.529) and other variants following a two-dose primary regimen. After the third dose, immunological responses were comparable to or beyond levels associated with protection in phase 3 clinical trials, with a 9.3-fold increase in IgG and a 19.9-fold increase in ACE2 inhibition. Immune responses in adolescents were 2- to 4-fold stronger than those of adults against a wide range of variants (113).
Vaccines generally use viral antigenic material either naturally or synthetically to induce an immune response; however, the process varies with different vaccine platforms (114). Viral vector-based vaccines, on the other hand, use a viral vector that encodes the gene for the viral-specific antigen, and once injected, they use host cell machinery to produce such viral antigen (114, 115). The vaccine simulates the pathology of natural infection with some pathogens, specifically viruses, by contaminating cells and imposing them to produce large amounts of the antigen, following activation of an immune response (both T-cell- and B-cell-mediated) (116). To produce effective vaccines quickly against various infectious diseases, the adenoviral vector has been favored. As of 2 June 2022, there were 29 viral vector vaccines under development, and 24 were adenovirus-based vaccines (10 in phase 3, 5 in phase 2, and 9 in phase 1) (117). To date, 6 viral vector vaccines have been approved under the emergency use authorization pathway in different countries and 3 vaccines, Ad26.COV2.S, Vaxzervria, and Covishield™ (Oxford/AstraZeneca formulation) have been granted emergency use listing by the WHO.
Efficacy data of all vaccine candidates currently in phase 3 and 4 are reported to be more than satisfactory. However, the Oxford-AstraZeneca vaccines, sold under the brand names of Vaxzervria and Covishield™ which uses a modified chimpanzee adenovirus vector ChAdOx1 were noted to cause rare but life-threatening thromboembolic adverse events in some patients. These adverse events involve the generation of an antibodies which cross react with platelet factor 4, thus initiating a clotting cascade (118). Presently, the EMA and WHO have clarified that the risk of developing thromboembolic events is not significantly higher than that in the general population (119). One should understand that as of now, the benefits outweigh the risk associated with the use of such vaccines. Nevertheless, long-term evaluation studies are required. A single dose of ChAdOx1 vaccine was demonstrated to evoke polyfunctional antibodies capable of affecting neutralization as well as a variety of additional antibody-dependent effector mechanisms, all of which may aid in disease prevention. Antibodies generated by ChAdOx1 were found to aid phagocytosis and were capable of antibody-depended complement deposition after only one dose which were further boosted after the second dose (120). Furthermore, because of the stimulation of TNF and IFN by CD4+ T cells in response to antigen stimulation in vitro, this vaccine produced robust T-cell responses that peaked 14 days after a single dose. Despite decreased T-cell responses and greater antibody responses after the second dosage, the vaccine’s effectiveness following one and two doses is identical, suggesting that alternative protective mechanisms are active after one versus two doses. Prolonging the interval between doses also increased immunogenicity and efficacy (121).
The Janssen vaccine sold under the brand name Jcovden is produced by Janssen vaccines in Netherlands and Belgium and by its subsidiary USA company Johnson & Johnson. This vaccine is a viral vector based human adenovirus vaccine (Ad26.COV2.S) which contains the spike protein. The vaccine is currently approved for emergency use in 111 countries, while the global phase 3 clinical trial is ongoing (NCT04505722 and NCT04614948). In a research study conducted during the delta variant pandemic in hospitalized individuals, the vaccine was found to be 81% effective (122). This cohort study in US clinical practice demonstrated consistent vaccine efficacy of Ad26.COV2.S for at least six months prior to and throughout the emergence and dominance of the delta variant. Similarly, the Sisonke single-arm, open-label, phase 3B study conducted in South Africa, demonstrated efficiency of a single-dose of Ad26.COV2.S vaccine against severe COVID-19 illness and COVID-19-related mortality; as well as against both beta and delta variants, and provided empirical support for its widespread usage (123). A single dose of Ad26.COV2.S offered 83% protection against COVID-19-related deaths, 75% against hospitalisations requiring critical or intensive care, and 67% protection against COVID-19-related hospitalisations (123). The effectiveness was also shown in older health-care workers and participants with comorbid HIV infection (123). Similar efficacy was noted against COVID-related hospitalisations and deaths during the beta and delta waves. Protection persisted for at least 6 months (NCT04505722). Despite the efficacy of inducing immunity with only one injection and efficacy in preventing hospitalisations and deaths, cases of thrombocytopenia and Guillain Barre syndrome have been reported. In February 2022, Johnson& Johnson announced that it halted production of Ad26.COV2.S and would resume at some point in the future.
Virus-like particles are adaptable, safe, and are able to stimulate strong humoral and cellular immune responses. They consist of repeated viral surface proteins, are small (20-200 nm), do not replicate and are alternatives to attenuated virus-based vaccines. The currently approved hepatitis-B and human papillomavirus vaccines use this technology. The CoVLP+AS03 (brand name Covifenz) vaccine developed by Medicago in Canada and by GalxoSmithKline comprises virus-like particles grown in Nicotiana benthamiana weed. These virus-like particles are made using a molecular farming technology and as such, it is low-cost, safe and easy and quick to produce. CoVLP+AS03 adjuvant was shown to be effective in preventing COVID-19 caused by a spectrum of variants, with vaccine efficacy ranging from 69.5% against symptomatic infection to 78.8% against moderate-to-severe disease (124). In addition, CoVLP vaccine exhibited 100% efficacy against the alpha variant, 75.3% against delta, and 88.6% against the gamma variant.
“SARS-CoV-2-spike-specific immune responses to Moderna mRNA-1273, Pfizer/BioNTech BNT162b2, Janssen Ad26.COV2. S, and Novavax NVX-CoV2373 were studied longitudinally for 6 months” by Zeli Zhang and colleagues. After mRNA or NVX-CoV2373 immunization, 100% of people developed memory CD4+ T cells, and CD4-CD8 was overrepresented. Although memory CD8+ T cells were identifiable in only 60-67% of subjects at 6 months, mRNA vaccinations and Ad26.COV2. S generated equivalent CD8+ T-cell frequencies. As per the study, although Ad26.COV2. S T cell, B cell, and antibody responses were relatively constant over 6 months, it was not the strongest immunogen by any metric. A high frequency of CXCR3+ memory B cells was a distinguishing feature of Ad26.COV2 vaccination. Over 6 months, neutralizing antibodies in mRNA vaccines decreased significantly, whereas memory T cells and B cells remained rather steady (125).
In healthy adults who received the full dosage of inactivated vaccine (CoronaVac, also known as Sinovac), the booster effect of multiple vaccination platforms, including inactivated vaccine (BBIBP), viral vector vaccine (AZD122), and mRNA vaccine (BNT162b2) were assessed. The outcome demonstrated that the booster dose was safe and had no negative side effects. Additionally, the immunogenicity demonstrated that the booster dose of the viral vector and mRNA vaccine resulted in a significant quantity of Ig anti-RBD, IgG anti-RBD, and IgA anti-S1 booster responses. In contrast, the booster response for inactivated vaccination was lower than for other vaccines. Therefore, against wild-type SARS-CoV-2 and its variants (B.1.1.7-alpha, B.1.351-beta, and B.1.617.2-delta), the neutralizing activity of vaccine serum had a strong inhibition of over 90%. Additionally, IgG anti-nucleocapsid was only detected in the BBIBP booster group. After receiving the additional viral vector or mRNA booster vaccine, the study showed a marked rise in the levels of the IFN-secreting T-cell response (126). Between January 1 and August 3, 2021, another retrospective cohort study involving healthcare workers (HCWs) was carried out in Brazil. A total of 13,813 HCWs were included in the study: 46.2% of them received the CoronaVac vaccine, 42.8% received the ChAdOx1 vaccine, and 11.0% were not immunized. In all, COVID-19 infection cases occurred in 6% of HCWs who were not vaccinated, 3% of HCWs who received two doses of the CoronaVac vaccine, and 0.7% of HCWs who received two doses of the ChAdOx1 vaccine. The estimated vaccination efficacy for ChAdOx1 and CoronaVac was 88.1 and 51.3%, respectively, in the adjusted analyzes. The need for mechanical breathing, hospitalisations, and length of stay were all decreased by both immunizations. For mutations of interest, SARS-CoV-2 samples from 19 HCWs were evaluated. Eighteen of the samples contained the gamma SARS-CoV-2 mutation (127).
Considering the current emerging variants of SARS-CoV-2 and the efficacy of the currently available vaccines, it is clear that one needs variant-specific vaccines rather than repeated booster dosing with the existing vaccine, as each vaccine under an emergency use approval license has some degree of adverse events reported, and none provide complete protection against the emerging variants. Figure 5 summarizes the immune responses post vaccination.
Figure 5 Immune reaction after vaccination. After intramuscular (IM), intradermal (ID) or subcutaneous (SC) vaccine delivery, dermal dendritic cells (DCs) take up antigens and migrate to draining lymph nodes to stimulate T cells (CD8+ T cells and CD4+ T cells). Plasma cells secrete antibodies and memory B cells. CD8+ T cells can be stimulated by Th1 cytokines and in turn acquire the ability to attack the infected cells. However, imbalanced immune responses have the potential to cause pulmonary immunopathology, partially due to an aberrant Th2 response or antibody-dependent enhancement (ADE). Created with BioRender.com.
The twist created by the spread of mutant strains has put a question mark on the efficacy of the current COVID-19 vaccines, although some of these vaccine candidates have also proven their efficacy against some of these mutant strains. The emergence of postvaccine reinfections with SARS-CoV-2 lineages can have a substantial influence on public health and the economy. The possible use of regular vaccination shots at 6 months or 1 year for the control of variants cannot be ruled out. As of now, this disease has been very unpredictable, and it is still too early for such assumptions. Logistic challenges associated with nucleic acid-based and vector-based vaccines, especially in poor and developing countries, are still a concern. The SARS-CoV-2 pandemic has shown many unpredictable outcomes, but the scientific community is optimistic in the fight against all challenges.
Researchers are competing against time to build immunity against this intriguing virus, whose capacity to change and evolve appears to be exceeding our capability to attain herd immunity. The long-term COVID-19 pandemic has been dependent on balance by a dispersal of approved vaccines and emerging viral variants (22, 128). Due to new variants, the race to the finish line may be a sprint. The variants are worrisome for several reasons. First, the VOCs transmit at least 20-50% faster from person to person. This encourages them to infect more individuals and spread more quickly and widely, eventually becoming the prevailing paradigm. Second, there is a concern that SARS-CoV-2 mutations can result in them being more contagious, induce more severe sickness, as well as an increase in hospitalisations and mortality (Table 1) (129). Although vaccine effectiveness is necessary for vaccine licensure, it may not always represent the impact of immunization in the actual world, particularly when clinical trials for vaccines involve primarily younger, healthy individuals rather than those most at risk of extreme infection, and these studies took place before some of the more recently reported SARS-CoV-2 variants were discovered (130). As a consequence, it is crucial to determine the degree and duration of defense against infection or illness across all age groups and populations; in the case of COVID-19, this is especially critical given the greater risk of severe disease in older adults (131). The delta variant has the same high resistance as other omicron sub-variants and no increased sensitivity to serum acquired during the delta wave (132). Even though the impact of delta-derived spike mutations in the N-terminal region on viral proliferation and pathogenicity is unknown, they do not seem to decrease neutralization resistance. Recombination of viral variants and the possibility of the formation of a far more virulent variety with significant immune evasion is a major issue that must be closely monitored.
The waning of protection has been seen with time since vaccination, particularly with the delta variant, which is able to at least to some extent evade natural and vaccine-induced immunity. However, booster doses provide a rapid and significant boost in protection against both mild and severe diseases (34, 133). The epidemiology of viral VOCs along with the efficacy of EUA vaccines is vital for creating immunization strategies and the advancement of novel vaccines (Table 2). In contrast to similar BNT162b2 vaccination, heterologous BNT162b2 boost following ChAdOx1 nCoV-19 reliably results in greater neutralizing titres against the alpha, beta and gamma variants. Surprisingly, homologous BNT162b2 prime boost appears to be more effective in producing delta variant-neutralizing antibodies (150, 151). Furthermore, there is presently a scarcity of data on the duration of protection provided by mRNA vaccines. There are currently no effective vaccination measures or conclusive empirical estimates of vaccine longevity. Due to decreased nAbs, reinfection with different variants of SARS-CoV-2 has been observed. The COVID-19 vaccination booster dosage is critical for the production of neutralizing immune reactions against omicron, according to new findings, and improved therapeutic antibodies are required for this and future versions (152). A recent study revealed that natural infection significantly increases the number, quality, and diversity of humoral immunity, irrespective of whether it happens before or after vaccination (153). Hybrid immunity, which is the result of prior COVID-19 and a subsequent vaccine, seems to provide the best protection against SARS-CoV-2 infections; however, there are still significant information limitations in this area.
There is no doubt that mass vaccinations have revolutionized global health. The single most innovative medical outcome was the eradication of smallpox following worldwide mass vaccinations, and more recently, on 28 August 2020, polio was declared eradicated in Africa following a mass vaccination campaign that started in 1980. Likewise, a huge decrease in the number of incidences and mortalities against measles, mumps, rubella, chickenpox, whooping cough, tuberculosis, etc., have been recorded in the last few decades with childhood vaccination programs. Within the first year of COVID-19 vaccine rollouts, it is estimated to have saved 19.8 million lives, and thus far, over 12 billion doses of COVID-19 vaccines have been administered in most countries around the world. The aim of the current vaccines was to reduce severe symptoms, illness, hospitalisations and death (154). Indeed, COVID-19 vaccines have saved millions of lives thus far, even though breakthroughs are common. COVID-19 continues to be a pandemic, with thousands of daily cases and hundreds of daily deaths, and as the virus continues to circulate, new variants emerge, some of which are more transmissive and more dangerous, including some variants that reduce the efficacy of vaccines (154, 155). Further investments and the development of new improved vaccines against the variants are required and should be administered annually, similar to influenza virus vaccines (155).
There is a race to develop a vaccine that is both safe and effective against COVID-19. With a global effort and collaboration and funding, one could be in the market within 12–18 months. One fully approved vaccine and 38 emergency use approved (EUA) vaccines for COVID-19 in the market in less than two years define the speed of the vaccine development race. Because of the expedited pace of innovation, global health researchers are warning that vaccines will be authorized with insufficient data and analysis. For instance, at least one candidate did not undergo animal testing. In the meantime, CanSino Biologics’ exploratory COVID-19 vaccine was authorized for use in the Chinese military before the completion of phase 3 trials (156). One of the deepest concerns is the possibility that a fast-tracked vaccine will have unexpected effects. No vaccine is completely safe, but if a billion people are vaccinated, one in 10,000 major negative incidents will impact 100,000 of those people. In May 2021, it was demonstrated that four of the 45 participants in Moderna’s phase 1 vaccine trial had medically significant adverse events (157). In identifying the severity of the existing public health crisis and the idea of making a vaccine accessible as quickly as possible, one assumes that a median 2-month follow-up after completion of the vaccine regimen will provide the necessary safety and efficacy data to endorse the allocation of an experimental vaccine under emergency use authorization (158).
Phase 3 randomized trials that investigate the incidence of COVID-19 in large groups of immunised and nonimmunised patients are the actual test of the vaccine’s efficacy. This type of study will determine whether one, many, or none of the new COVID-19 vaccines offer effective or limited immunity and whether their usage is associated with serious side effects. Science-based initiatives and significant funding have supported the development of 38 vaccine candidates that will be licensed for public use and contend head-to-head with each other (159).
The most important consideration is that vaccines against SARS-CoV-2 need to be dosed multiple times in the world population in a timely manner, at a level far beyond other types of vaccinations in history. The global vaccine supply chain has played the most crucial role in delivering each vaccine shot to end users (160). In the last two years, the government and healthcare organizations worldwide have faced the complex task of obtaining and distributing supplies to their populations and administering the vaccine (Figure 6). While a handful of countries and companies managed to receive approval for vaccines with the required efficacy, acquiring sufficient doses for all the countries is just the start. Vaccines must then be transported securely to multiple locations and preserved at the right temperature as well as be protected from tampering to assure product integrity. While all types of vaccines must be transported in cold conditions, creating secure cold chain management of the global vaccine chain supply is vital (161). This global pandemic shows how complex global supply chains developed to deliver billions of vaccine doses. Global COVID-19 vaccine supply chains facilitated sustained production and access. Even though obtaining enough vaccines remained difficult for many countries last year, the worldwide trade of vaccines was 26% higher during the first six months of 2021 compared with the full 12 months of 2020. The vaccine business was also supplemented by increasing trade in associated or intermediate inputs, strengthening their wider manufacturing and distribution. Even through nationalistic policies from the United States and European Union, international interdependence still emerged. This highlights the capacity of global supply chains to ramp up and boost the distribution and production of essential vaccines in record time. A global supply chain is still important in immunizing all parts of the world to lower the risk of new variants emerging.
Ethical considerations to develop a vaccine and be tested in humans must be met, and approvals must be in place. There are standard procedures regarding clinical trials and testing of vaccines in humans (Figure 7). From preclinical in vitro and in vivo studies to larger animal immunological and toxicology studies to human phase 1, 2, 4 clinical trials, strict ethical guidelines and procedures must be met, and the obligation of reporting human clinical outcomes follows a clear path (164). However, several other ethical issues regarding vaccination against SARS-CoV-2 attracted much controversy at every stage of its development and use. Vaccines were developed in record time and as such raised many issues with the population, and it was difficult to persuade some reluctant populations to receive the vaccine. In addition, government mandates were an issue with some that raised much debate regarding their moral rights vs a duty. However, the ethical debate was not only to those who refused to be vaccinated but also to those who rushed or jumped queues to be first. Several ethical issues have had to be overcome regarding which population group should be the first to receive the vaccine and what could be done with those who were reluctant. In addition, there were issues regarding which countries and groups should benefit before others. As such, some countries, i.e., the US, Europe and Australia mandated the vaccine to reach over an 80-90% vaccination rate, while leaving out other nations generally low-income nations. Vaccine passports also raised issues to an unequally vaccinated world, where those who were vaccinated were able to go to work, go to shops and restaurants, engage in leisure activities and travel (165). Overall, the inadequate immunization coverage, even though the vaccines were shown to be safe and effective, at least in the time frame tested, the uncontrolled number of COVID-19 cases and hospitalisations, and the rapid upsurge of more new variants tipped the scales towards some form of mandatory vaccination policy (39, 166). Voluntary vaccination compared to compulsory vaccination policies is at the center of debate in the last 2 years.
COVID-19 shows that rising infectious diseases present a substantial and growing danger to global health, the human and economic costs are vast, and many other viruses could result in another pandemic. To minimize pathogenicity in humans, entire pathogens were initially utilized as vaccine agents, either inactivated or attenuated. Various mRNA- and DNA-based vaccines were authorized for the first time in human history. The development of vaccine formulations against COVID-19 within record time in this pandemic by following the same protocols for previously emerging SARS-CoV and MERS-CoV and as an emergency vaccination discovery approach to skip over some preliminary preclinical steps of vaccine development has allowed for the creation of the life-saving shots for the eight billion global population in two years. Unexpected correlations between parameters were discovered during the deconvolution of patient features inside groups. By combining large datasets using uniform manifold approximation and projection-assisted clustering, patient groupings containing precise estimates may be identified, as well as unanticipated relationships among clinical factors. This use of machine learning to delineate illness pathophysiology and possible treatment strategies is a strong strategy (167).
Vaccine effectiveness, in contrast to vaccine efficacy, refers to the reduced risk of illness or sickness among vaccinated people. According to Zhang and colleagues (125), “head-to-head comparisons of T cell, B cell, and antibody responses to diverse vaccines in humans are likely to be informative for understanding protective immunity against COVID-19, with particular interest in immune memory. Here, SARS-CoV-2-spike-specific immune responses to Moderna mRNA-1273, Pfizer/BioNTech BNT162b2, Janssen Ad26.COV2. S, and Novavax NVX-CoV2373 were examined longitudinally for 6 months. One hundred percent of individuals made memory CD4+ T cells, with SARS-CoV-2 spike-specific circulating follicular helper T cells (cTfhs) and CD4-CTLs highly represented after mRNA or NVX-CoV2373 vaccination. mRNA vaccines and Ad26.COV2. S induced comparable CD8+ T-cell frequencies, although only detectable in 60-67% of subjects at 6 months. A differentiating feature of Ad26.COV2. S immunization resulted in a high frequency of CXCR3+ memory B cells. mRNA vaccines had substantial declines in antibodies, while memory T and B cells were comparatively stable. These results may also be relevant for insights against other pathogens.” The vaccine’s population-dependent effects, along with immunization schedules and vaccine processing, can have an impact on this. Various large-scale research studies have established why it is essential to have vaccinations, as they offer a considerably greater level of protection against COVID-19, including against new variants of concern, whether you have been previously infected or not. However, the analysis also evidently reveals that this protection from just two primary doses vanishes significantly within months, which is why the rollout of booster shots has been effective to avoid infection and illness, particularly against new COVID-19 variants. Socioeconomic COVID-19 risks of the pandemic and sociomedical safety measures as a means of flattening the pandemic curve and preserving vaccine supplies as well as integrated vaccination therapeutic tactics to tackle the COVID-19 pandemic. Spike amino acid replacements and subtractions that affect neutralizing antibodies are common in the worldwide virus demography, and research suggests that the variants exhibit resistance to antibody-mediated immunity induced by vaccines. Attempts are ongoing to stop the spread of the virus from the local site by IgA-mediated protection with the help of intranasal route of administration and DC-based vaccines (168–170).
In the middle of the high death rate and the alarming fall of the global healthcare system in the initial two years of the COVID-19 pandemic, SARS-CoV-2 vaccines were approved for the first time based on novel vaccine technologies (either RNA or DNA). Fast-track vaccine approval by regulators, manufacturing of vaccines at a large scale, and the effective distribution of COVID-19 vaccines have played a significant role in distributing vaccine shots that provide robust protection against infectious SARS-CoV-2 and its evolving variants. Fortunately, even for those who become infected, vaccination protects against major disease, hospitalisation, and fatality from COVID-19. The key vaccine development processes and vaccination programs are still ongoing because of the devastating effects of the pandemic. Even the most industrially developed and economically prosperous nations can succumb to the pandemic. Therefore, new manufacturing facilities, steadfast cold-chain supply chain networks, and continuous research should be in place to streamline development and maintain the safety and efficacy of future vaccines. Unparalleled as the COVID-19 pandemic might have been, more variants of SARS-CoV-2 will enter the circulation, and other zoonotic diseases will occur. Overall, the fast-track rollout of vaccines has become the “New Normal.”
VC prepared the backbone of the manuscript. VC, QY, LV, and AP wrote the original draft of the manuscript. CP, LV, VA, RB, Z-SC, QY, and VC refined the first draft. VC critically revised the manuscript for intellectually correct content. All authors contributed to the article and approved the submitted version.
VC would like to dedicate this work to L M College of pharmacy as a part of the 75th year celebration of the college. VA would like to thank the support from the Immunology and Translational Research Group, within the Institute for Health and Sport, Victoria University Australia. VA was supported by Victoria University Research, VIC Australia.
The authors declare that the research was conducted in the absence of any commercial or financial relationships that could be construed as a potential conflict of interest.
All claims expressed in this article are solely those of the authors and do not necessarily represent those of their affiliated organizations, or those of the publisher, the editors and the reviewers. Any product that may be evaluated in this article, or claim that may be made by its manufacturer, is not guaranteed or endorsed by the publisher.
1. Shetty R, Ghosh A, Honavar SG, Khamar P, Sethu S. Therapeutic opportunities to manage COVID-19/SARS-CoV-2 infection: Present and future. Indian J Ophthalmol (2020) 68(5):693–702. doi: 10.4103/ijo.IJO_639_20
2. Chavda VP, Prajapati R, Lathigara D, Nagar B, Kukadiya J, Redwan EM, et al. Therapeutic monoclonal antibodies for COVID-19 management: an update. Expert Opin Biol Ther (2022) 22(6):1–18. doi: 10.1080/14712598.2022.2078160
3. Chavda VP, Hanuma Kumar Ghali EN, Yallapu MM, Apostolopoulos V. Therapeutics to tackle omicron outbreak. Immunotherapy (2022) 14(11):833–8. doi: 10.2217/imt-2022-0064
4. Basu D, Chavda VP, Mehta AA. Therapeutics for COVID-19 and post COVID-19 complications: An update. Curr Res Pharmacol Drug Discov (2022) 3:100086. doi: 10.1016/j.crphar.2022.100086
5. Chavda VP, Gajjar N, Shah N, Dave DJ. Darunavir ethanolate: Repurposing an anti-HIV drug in COVID-19 treatment. Eur J Med Chem Rep (2021) 3:100013. doi: 10.1016/j.ejmcr.2021.100013
6. Huang Z, Chavda VP, Vora LK, Gajjar N, Apostolopoulos V, Shah N, et al. 2-Deoxy-D-Glucose and its derivatives for the COVID-19 treatment: An update. Front Pharmacol (2022) 13:899633. doi: 10.3389/fphar.2022.899633
7. Saha RP, Sharma AR, Singh MK, Samanta S, Bhakta S, Mandal S, et al. Repurposing drugs, ongoing vaccine, and new therapeutic development initiatives against COVID-19. Front Pharmacol (2020) 11:1258. doi: 10.3389/fphar.2020.01258
8. Chavda VP, Kapadia C, Soni S, Prajapati R, Chauhan SC, Yallapu MM, et al. A global picture: therapeutic perspectives for COVID-19. Immunotherapy (2022) 14(5):351–71. doi: 10.2217/imt-2021–0168
9. USFDA. Clinical trial for SARS-CoV-2 vaccine (COVID-19). NIH Public Acces (2022) Available at: https://covid19.nih.gov/clinical-trials, Accessed on 15/07/2022.
10. Sharma O, Sultan AA, Ding H, Triggle CR. A review of the progress and challenges of developing a vaccine for COVID-19. Front Immunol (2020) 11(1):1–17. doi: 10.3389/fimmu.2020.585354
11. Jayk Bernal A, Gomes da Silva MM, Musungaie DB, Kovalchuk E, Gonzalez A, Delos Reyes V, et al. Molnupiravir for oral treatment of covid-19 in nonhospitalized patients. N Engl J Med (2021) 386(6):509–20. doi: 10.1056/NEJMoa2116044
12. Team CDCC-19 R. Severe outcomes among patients with coronavirus disease 2019 (COVID-19) - united states, February 12-march 16, 2020. MMWR Morb Mortal Wkly Rep (2020) 69(12):343–6. doi: 10.15585/mmwr.mm6912e2
13. Chavda VP, Apostolopoulos V. Rare monkeypox: Is it really a threat to the elderly? Maturitas (2022) 163:90–1. doi: 10.1016/j.maturitas.2022.05.014
14. Chavda VP, Vora LK, Apostolopoulos V. Monkeypox: a new face of outbreak. Expert Rev Vaccines (2022) Online ahead of print:1–4. doi: 10.1080/14760584.2022.2113515
15. Chavda VP, Patel K, Apostolopoulos V. Tomato flu outbreak in India. Lancet Respir Med (2022) S2213-2600(22)00300-9. doi: 10.1016/S2213-2600(22)00300-9
16. Zhang X-A, Li H, Jiang F-C, Zhu F, Zhang Y-F, Chen J-J, et al. A zoonotic henipavirus in febrile patients in China. N Engl J Med (2022) 387(5):470–2. doi: 10.1056/NEJMc2202705
17. Bajaj V, Gadi N, Spihlman AP, Wu SC, Choi CH, Moulton VR. Aging, immunity, and COVID-19: How age influences the host immune response to coronavirus infections? Front Physiol (2021) 11:1793. doi: 10.3389/fphys.2020.571416
18. Stahl EC, Brown BN. Cell therapy strategies to combat immunosenescence. Organogenesis (2015) 11(4):159–72. doi: 10.1080/15476278.2015.1120046
19. Mueller AL, McNamara MS, Sinclair DA. Why does COVID-19 disproportionately affect older people? Aging (Albany NY) (2020) 12(10):9959–81. doi: 10.18632/aging.103344
20. Sempowski GD, Saunders KO, Acharya P, Wiehe KJ, Haynes BF. Pandemic preparedness: Developing vaccines and therapeutic antibodies for COVID-19. Cell (2020) 181(7):1458–63. doi: 10.1016/j.cell.2020.05.041
21. Annavajhala MK, Mohri H, Zucker JE, Sheng Z, Wang P, Gomez-Simmonds A, et al. A novel SARS-CoV-2 variant of concern, B.1.526, identified in new York. medRxiv : preprint server Health Sci (2021) Version 1. medRxiv. Preprint. doi: 10.1101/2021.02.23.21252259
22. Chavda VP, Patel AB, Vaghasiya DD. SARS-CoV-2 variants and vulnerability at the global level. J Med Virol . (2022) 94:2986–3005. doi: 10.1002/jmv.27717
23. Chavda VP, Apostolopoulos V. Omicron variant (B.1.1.529) of SARS-CoV-2: Threat for the elderly? Maturitas (2022) 158:78–81. doi: 10.1016/j.maturitas.2022.01.011
24. Harvey WT, Carabelli AM, Jackson B, Gupta RK, Thomson EC, Harrison EM, et al. SARS-CoV-2 variants, spike mutations and immune escape. Nat Rev Microbiol (2021) 614:409–24. doi: 10.1038/s41579-021-00573-0
25. Rayati Damavandi A, Dowran R, Al Sharif S, Kashanchi F, Jafari R. Molecular variants of SARS-CoV-2: antigenic properties and current vaccine efficacy. Med Microbiol Immunol (2022) 211(2-3)1–25. doi: 10.1007/s00430-022-00729-6
26. Chakraborty C, Bhattacharya M, Sharma AR. Present variants of concern and variants of interest of severe acute respiratory syndrome coronavirus 2: Their significant mutations in s-glycoprotein, infectivity, re-infectivity, immune escape and vaccines activity. Rev Med Virol (2021) 32(2):e2270. doi: 10.1002/rmv.2270
27. Mohammadi M, Shayestehpour M, Mirzaei H. The impact of spike mutated variants of SARS-CoV2 [Alpha, beta, gamma, delta, and lambda] on the efficacy of subunit recombinant vaccines. Braz J Infect Dis an Off Publ Braz Soc Infect Dis (2021) 25(4):101606. doi: 10.1016/j.bjid.2021.101606
28. Yadav PD, Sarkale P, Razdan A, Gupta N, Nyayanit DA, Sahay RR, et al. Isolation and characterization of SARS-CoV-2 beta variant from UAE travelers. J Infect Public Health (2022) 15(2):182–6. doi: 10.1016/j.jiph.2021.12.011
29. Huang H-C, Liao C-C, Wang S-H, Lee I-J, Lee T-A, Hsu J-M, et al. Hyperglycosylated spike of SARS-CoV-2 gamma variant induces breast cancer metastasis. Am J Cancer Res (2021) 11(10):4994–5005.
30. Jaspe RC, Loureiro CL, Sulbaran Y, Moros ZC, D’Angelo P, Rodríguez L, et al. Introduction and rapid dissemination of SARS-CoV-2 gamma variant of concern in Venezuela. Infect Genet Evol J Mol Epidemiol Evol Genet Infect Dis (2021) 96:105147. doi: 10.1016/j.meegid.2021.105147
31. Dhawan M, Sharma A, Priyanka, Thakur N, Rajkhowa TK, Choudhary OP. Delta variant (B.1.617.2) of SARS-CoV-2: Mutations, impact, challenges and possible solutions. Hum Vaccin Immunother (2022) 18(5):2068883. doi: 10.1080/21645515.2022.2068883
32. Chavda VP, Apostolopoulos V. Global impact of delta plus variant and vaccination. Expert Rev Vaccines (2022) 21(5):597–600. doi: 10.1080/14760584.2022.2044800
33. Ren S-Y, Wang W-B, Gao R-D, Zhou A-M. Omicron variant (B.1.1.529) of SARS-CoV-2: Mutation, infectivity, transmission, and vaccine resistance. World J Clin cases (2022) 10(1):1–11. doi: 10.12998/wjcc.v10.i1.1
34. Chavda VP, Apostolopoulos V. Is booster dose strategy sufficient for omicron variant of SARS-CoV-2? Vaccines (2022) 10:367. doi: 10.3390/vaccines10030367
35. Chavda VP. Omicron variant (B.1.1.529) of SARS-CoV-2: Threat for the elderly? Maturitas (2022) 158:78–81. doi: 10.1016/j.maturitas.2022.01.011
36. Kumar S, Karuppanan K, Subramaniam G. Omicron (BA.1) and sub-variants (BA.1.1, BA.2, and BA.3) of SARS-CoV-2 spike infectivity and pathogenicity: A comparative sequence and structural-based computational assessment. J Med Virol (2022) 94(10):4780–91. doi: 10.1002/jmv.27927
37. Desingu PA, Nagarajan K, Dhama K. Emergence of omicron third lineage BA.3 and its importance. J Med Virol (2022) 94(5):1808–10. doi: 10.1002/jmv.27601
38. Chen J, Wei G-W. Omicron BA.2 (B.1.1.529.2): high potential to becoming the next dominating variant. J Phys Chem Lett (2022) 13(17):3840–9. doi: 10.21203/rs.3.rs-1362445/v1
39. Chavda VP, Sonak SS, Munshi NK, Dhamade PN. Pseudoscience and fraudulent products for COVID-19 management. Environ Sci pollut Res Int (2022) 29(42):62887–912. doi: 10.1007/s11356-022-21967-4
40. Chakraborty C, Bhattacharya M, Sharma AR, Dhama K. Recombinant SARS-CoV-2XE, and XF: The emergence of recombinant variants requires an urgent call for research - correspondence Int J Surg (London England) (2022) 102:106670. doi: 10.1016/j.ijsu.2022.106670
41. Mohapatra RK, Kandi V, Tuli HS, Chakraborty C, Dhama K. The recombinant variants of SARS-CoV-2: Concerns continues amid COVID-19 pandemic. J Med Virol (2022) 94:3506–8. doi: 10.1002/jmv.27780
42. VanInsberghe D, Neish AS, Lowen AC, Koelle K. Recombinant SARS-CoV-2 genomes are currently circulating at low levels. bioRxiv: preprint server Biol (2021) 2020.08.05.238386. doi: 10.1101/2020.08.05.238386
43. Rahimi F, Talebi Bezmin Abadi A. Hybrid SARS-CoV-2 variants. Int J Surg (2022) 102:106656. doi: 10.1016/j.ijsu.2022.106656
44. Dhawan M, Saied AA, Emran TB, Choudhary OP. Emergence of omicron variant’s sublineages BA.4 and BA.5: risks assessment and possible countermeasures. New Microbes New Infect (2022) 48:100997. doi: 10.1016/j.nmni.2022.100997
45. Ding C, He J, Zhang X, Jiang C, Sun Y, Zhang Y, et al. Crucial mutations of spike protein on SARS-CoV-2 evolved to variant strains escaping neutralization of convalescent plasmas and RBD-specific monoclonal antibodies. Front Immunol (2021) 12:693775. doi: 10.3389/fimmu.2021.693775
46. Luan B, Wang H, Huynh T. Enhanced binding of the N501Y-mutated SARS-CoV-2 spike protein to the human ACE2 receptor: insights from molecular dynamics simulations. FEBS Lett (2021) 595(10):1454–61. doi: 10.1002/1873-3468.14076
47. Kemenesi G, Tóth GE, Bajusz D, Keserű GM, Terhes G, Burián K, et al. Effect of an 84-bp deletion of the receptor-binding domain on the ACE2 binding affinity of the SARS-CoV-2 spike protein: An in silico analysis. Genes (Basel) (2021) 12(2):194. doi: 10.3390/genes12020194
48. Khairnar P, Soni M, Handa M, Riadi Y, Kesharwani P, Shukla R. Recent highlights on omicron as a new SARS-COVID-19 variant: evolution, genetic mutation, and future perspectives. J Drug Target (2022) 30(6):1–11. doi: 10.1080/1061186X.2022.2056187
49. Pollard AJ, Bijker EM. A guide to vaccinology: from basic principles to new developments. Nat Rev Immunol (2021) 21(2):83–100. doi: 10.1038/s41577-020-00479-7
50. van Riel D, de Wit E. Next-generation vaccine platforms for COVID-19. Nat Mater (2020) 19(8):810–2. doi: 10.1038/s41563-020-0746-0
51. Wu S-C. Progress and concept for COVID-19 vaccine development. Biotechnol J (2020) 15(6):e2000147. doi: 10.1002/biot.202000147
52. Hossain MK, Hassanzadeganroudsari M, Feehan J, Apostolopoulos V. The race for a COVID-19 vaccine: where are we up to? Expert Rev Vaccines (2022) 21(3):355–76. doi: 10.1080/14760584.2022.2021074
53. Vasou A, Sultanoglu N, Goodbourn S, Randall RE, Kostrikis LG. Targeting pattern recognition receptors (PRR) for vaccine adjuvantation: From synthetic PRR agonists to the potential of defective interfering particles of viruses. Viruses (2017) 9(7):186. doi: 10.3390/v9070186
54. Kanekiyo M, Ellis D, King NP. New vaccine design and delivery technologies. J Infect Dis (2019) 219(Supplement_1):S88–96. doi: 10.1093/infdis/jiy745
55. Jackson NAC, Kester KE, Casimiro D, Gurunathan S, DeRosa F. The promise of mRNA vaccines: a biotech and industrial perspective. NPJ Vaccines (2020) 5(1):11. doi: 10.1038/s41541-020-0159-8
56. Maruggi G, Zhang C, Li J, Ulmer JB, Yu D. mRNA as a transformative technology for vaccine development to control infectious diseases. Mol Ther (2019) 27(4):757–72. doi: 10.1016/j.ymthe.2019.01.020
57. Pardi N, Hogan MJ, Porter FW, Weissman D. mRNA vaccines — a new era in vaccinology. Nat Rev Drug Discov (2018) 17(4):261–79. doi: 10.1038/nrd.2017.243
58. Guan S, Rosenecker J. Nanotechnologies in delivery of mRNA therapeutics using nonviral vector-based delivery systems. Gene Ther (2017) 24(3):133–43. doi: 10.1038/gt.2017.5
59. Mehta M, Shyh GI. A review of remdesivir for COVID-19: Data to date. Cardiol Rev (2020) 28(6):332–4. doi: 10.1097/CRD.0000000000000337
60. Singh AK, Singh A, Singh R, Misra A. Remdesivir in COVID-19: A critical review of pharmacology, pre-clinical and clinical studies. Diabetes Metab Syndr (2020) 14(4):641–8. doi: 10.1016/j.dsx.2020.05.018
61. Frediansyah A, Nainu F, Dhama K, Mudatsir M, Harapan H. Remdesivir and its antiviral activity against COVID-19: A systematic review. Clin Epidemiol Glob Heal (2021) 9:123–7. doi: 10.1016/j.cegh.2020.07.011
62. Kauffman KJ, Webber MJ, Anderson DG. Materials for non-viral intracellular delivery of messenger RNA therapeutics. J Control Release. (2016) 240:227–34. doi: 10.1016/j.jconrel.2015.12.032
63. Karikó K, Muramatsu H, Welsh FA, Ludwig J, Kato H, Akira S, et al. Incorporation of pseudouridine into mRNA yields superior nonimmunogenic vector with increased translational capacity and biological stability. Mol Ther (2008) 16(11):1833–40. doi: 10.1038/mt.2008.200
64. Karikó K, Muramatsu H, Ludwig J, Weissman D. Generating the optimal mRNA for therapy: HPLC purification eliminates immune activation and improves translation of nucleoside-modified, protein-encoding mRNA. Nucleic Acids Res (2011) 39(21):e142. doi: 10.1093/nar/gkr695
65. Thess A, Grund S, Mui BL, Hope MJ, Baumhof P, Fotin-Mleczek M, et al. Sequence-engineered mRNA without chemical nucleoside modifications enables an effective protein therapy in Large animals. Mol Ther (2015) 23(9):1456–64. doi: 10.1038/mt.2015.103
66. Polack FP, Thomas SJ, Kitchin N, Absalon J, Gurtman A, Lockhart S, et al. Safety and efficacy of the BNT162b2 mRNA covid-19 vaccine. N Engl J Med (2020) 383(27):2603–15. doi: 10.1056/NEJMoa2034577
67. Baden LR, El Sahly HM, Essink B, Kotloff K, Frey S, Novak R, et al. Efficacy and safety of the mRNA-1273 SARS-CoV-2 vaccine. N Engl J Med (2021) 384(5):403–16. doi: 10.1056/NEJMoa2035389
68. Tsui NBY, Ng EKO, Lo YMD. Stability of endogenous and added RNA in blood specimens, serum, and plasma. Clin Chem (2002) 48(10):1647–53. doi: 10.1093/clinchem/48.10.1647
69. Kallen K-J, Theß A. A development that may evolve into a revolution in medicine: mRNA as the basis for novel, nucleotide-based vaccines and drugs. Ther Adv Vaccines (2014) 2(1):10–31. doi: 10.1177/2051013613508729
70. Kis Z, Shattock R, Shah N, Kontoravdi C. Emerging technologies for low-cost, rapid vaccine manufacture. Biotechnol J (2019) 14(1):e1800376. doi: 10.1002/biot.201970055
71. Arashkia A, Jalilvand S, Mohajel N, Afchangi A, Azadmanesh K, Salehi-Vaziri M, et al. Severe acute respiratory syndrome-coronavirus-2 spike (S) protein based vaccine candidates: State of the art and future prospects. Rev Med Virol (2021) 31(3):e2183. doi: 10.1002/rmv.2183
72. Yi C, Sun X, Lin Y, Gu C, Ding L, Lu X, et al. Comprehensive mapping of binding hot spots of SARS-CoV-2 RBD-specific neutralizing antibodies for tracking immune escape variants. Genome Med (2021) 13(1):164. doi: 10.1186/s13073-021-00985-w
73. Anderson EJ, Rouphael NG, Widge AT, Jackson LA, Roberts PC, Makhene M, et al. Safety and immunogenicity of SARS-CoV-2 mRNA-1273 vaccine in older adults. N Engl J Med (2020) 383(25):2427–38. doi: 10.1056/NEJMoa2028436
74. Sadarangani M, Marchant A, Kollmann TR. Immunological mechanisms of vaccine-induced protection against COVID-19 in humans. Nat Rev Immunol (2021) 21(8):475–84. doi: 10.1038/s41577-021-00578-z
75. Hensley S, Cun A, Giles-Davis W, Li Y, Xiang Z, Lasaro MO. Type I interferon inhibits antibody responses induced by a chimpanzee adenovirus vector. Therapy (2007) 15(2):393–403. doi: 10.1038/sj.mt.6300024
76. Collignon C, Bol V, Chalon A, Surendran N, Morel S, van den Berg RA, et al. Innate immune responses to chimpanzee adenovirus vector 155 vaccination in mice and monkeys. Front Immunol (2020) 11. doi: 10.3389/fimmu.2020.579872
77. Shroff RT, Chalasani P, Wei R, Pennington D, Quirk G, Schoenle MV, et al. Immune responses to two and three doses of the BNT162b2 mRNA vaccine in adults with solid tumors. Nat Med (2021) 27(11):2002–11. doi: 10.1038/s41591-021-01542-z
78. Jalkanen P, Kolehmainen P, Häkkinen HK, Huttunen M, Tähtinen PA, Lundberg R, et al. COVID-19 mRNA vaccine induced antibody responses against three SARS-CoV-2 variants. Nat Commun (2021) 12(1):1–11. doi: 10.21203/rs.3.rs-343388/v1
79. Narayanaswamy V, Pentecost BT, Schoen CN, Alfandari D, Schneider SS, Baker R, et al. Neutralizing antibodies and cytokines in breast milk after coronavirus disease 2019 (COVID-19) mRNA vaccination. Obstet Gynecol (2022) 139(2):181–91. doi: 10.1097/AOG.0000000000004661
80. Zeng C, Evans JP, Chakravarthy K, Qu P, Reisinger S, Song N-J, et al. COVID-19 mRNA booster vaccines elicit strong protection against SARS-CoV-2 omicron variant in patients with cancer. Cancer Cell (2022) 40:117–9. doi: 10.1016/j.ccell.2021.12.014
81. Normark J, Vikström L, Gwon Y-D, Persson I-L, Edin A, Björsell T, et al. Heterologous ChAdOx1 nCoV-19 and mRNA-1273 vaccination. N Engl J Med . (2021) 385(11):1049–51. doi: 10.1056/NEJMc2110716
82. Paulina K, Deniz C, Stephanie F, Ai-ris C, Todd S, Caitlyn L, et al. mRNA-1273 and BNT162b2 COVID-19 vaccines elicit antibodies with differences in fc-mediated effector functions. Sci Transl Med (2022) 14(645):eabm2311. doi: 10.1126/scitranslmed.abm2311
83. Delrue I, Verzele D, Madder A, Nauwynck HJ. Inactivated virus vaccines from chemistry to prophylaxis: merits, risks and challenges. Expert Rev Vaccines (2012) 11(6):695–719. doi: 10.1586/erv.12.38
84. Sanders B, Koldijk M, Schuitemaker H. Inactivated viral vaccines. In: Nunnally B, Turula V, Sitrin R Vaccine Analysis: Strategies, Principles, and Control. Springer, Berlin, Heidelberg (2015). 127 (Online ahead of print). doi: 10.1007/978-3-662-45024-6_2
85. Majid S, Khan MS, Rashid S, Niyaz A, Farooq R, Bhat SA, et al. COVID-19: Diagnostics, therapeutic advances, and vaccine development. Curr Clin Microbiol Rep (2021), 1–15. doi: 10.1007/s40588-021-00157-9
86. Furuya Y. Return of inactivated whole-virus vaccine for superior efficacy. Immunol Cell Biol (2012) 90(6):571–8. doi: 10.1038/icb.2011.70
87. Spruth M, Kistner O, Savidis-Dacho H, Hitter E, Crowe B, Gerencer M, et al. A double-inactivated whole virus candidate SARS coronavirus vaccine stimulates neutralising and protective antibody responses. Vaccine (2006) 24(5):652–61. doi: 10.1016/j.vaccine.2005.08.055
88. Huang AT, Garcia-Carreras B, Hitchings MDT, Yang B, Katzelnick LC, Rattigan SM, et al. A systematic review of antibody mediated immunity to coronaviruses: kinetics, correlates of protection, and association with severity. Nat Commun (2020) 11(1):4704. doi: 10.1038/s41467-020-18450-4
89. Wang H, Zhang Y, Huang B, Deng W, Quan Y, Wang W, et al. Development of an inactivated vaccine candidate, BBIBP-CorV, with potent protection against SARS-CoV-2. Cell (2020) 182(3):713–21.e9. doi: 10.1016/j.cell.2020.06.008
90. Sapkal GN, Yadav PD, Ella R, Deshpande GR, Sahay RR, Gupta N, et al. Inactivated COVID-19 vaccine BBV152/COVAXIN effectively neutralizes recently emerged b 1.1.7 variant of SARS-CoV-2. J Travel Med (2021) 28(4):taab051. doi: 10.1093/jtm/taab051
91. Rawat K, Kumari P, Saha L. COVID-19 vaccine: A recent update in pipeline vaccines, their design and development strategies. Eur J Pharmacol (2021) 892:173751. doi: 10.1016/j.ejphar.2020.173751
92. Parida SP, Sahu DP, Singh AK, Alekhya G, Subba SH, Mishra A, et al. Adverse events following immunization of COVID-19 (Covaxin) vaccine at a tertiary care center of India. J Med Virol (2022) 94(6):2453–9. doi: 10.1002/jmv.27655
93. Lauring AS, Jones JO, Andino R. Rationalizing the development of live attenuated virus vaccines. Nat Biotechnol (2010) 28(6):573–9. doi: 10.1038/nbt.1635
94. Kames J, Holcomb DD, Kimchi O, DiCuccio M, Hamasaki-Katagiri N, Wang T, et al. Sequence analysis of SARS-CoV-2 genome reveals features important for vaccine design. Sci Rep (2020) 10(1):15643. doi: 10.1038/s41598-020-72533-2
95. Arvin AM, Greenberg HB. New viral vaccines. Virology (2006) 344(1):240–9. doi: 10.1016/j.virol.2005.09.057
96. Todorov G, Uversky VN. A possible path towards rapid development of live-attenuated SARS-CoV-2 vaccines: Plunging into the natural pool. Biomolecules (2020) 10:1438. doi: 10.3390/biom10101438
97. Zumla A, Chan JFW, Azhar EI, Hui DSC, Yuen K-Y. Coronaviruses - drug discovery and therapeutic options. Nat Rev Drug Discov (2016) 15(5):327–47. doi: 10.1038/nrd.2015.37
98. Graham RL, Becker MM, Eckerle LD, Bolles M, Denison MR, Baric RS. A live, impaired-fidelity coronavirus vaccine protects in an aged, immunocompromised mouse model of lethal disease. Nat Med (2012) 18(12):1820–6. doi: 10.1038/nm.2972
99. Menachery VD, Gralinski LE, Mitchell HD, Dinnon KH 3rd, Leist SR, Yount BLJ, et al. Combination attenuation offers strategy for live attenuated coronavirus vaccines. J Virol (2018) 92(17):e00710–18. doi: 10.1128/JVI.00710-18
100. Chafekar A, Fielding BC. MERS-CoV: Understanding the latest human coronavirus threat. Viruses (2018) 10(2):93. doi: 10.3390/v10020093
101. Bolles M, Deming D, Long K, Agnihothram S, Whitmore A, Ferris M, et al. A double-inactivated severe acute respiratory syndrome coronavirus vaccine provides incomplete protection in mice and induces increased eosinophilic proinflammatory pulmonary response upon challenge. J Virol (2011) 85(23):12201–15. doi: 10.1128/JVI.06048-11
102. Gadanec LK, McSweeney KR, Qaradakhi T, Ali B, Zulli A, Apostolopoulos V. Can SARS-CoV-2 virus use multiple receptors to enter host cells? Int J Mol Sci (2021) 22(3):992. doi: 10.3390/ijms22030992
103. McClean S. Prospects for subunit vaccines: Technology advances resulting in efficacious antigens requires matching advances in early clinical trial investment. Hum Vaccin Immunother (2016) 12(12):3103–6. doi: 10.1080/21645515.2016.1216287
104. Wang N, Shang J, Jiang S, Du L. Subunit vaccines against emerging pathogenic human coronaviruses. Front Microbiol (2020) 11:298. doi: 10.3389/fmicb.2020.00298
105. Kate Gadanec L, Qaradakhi T, Renee McSweeney K, Ashiana Ali B, Zulli A, Apostolopoulos V. Dual targeting of toll-like receptor 4 and angiotensin-converting enzyme 2: a proposed approach to SARS-CoV-2 treatment. Future Microbiol (2021) 16:205–9. doi: 10.2217/fmb-2021-0018
106. Ou X, Liu Y, Lei X, Li P, Mi D, Ren L, et al. Characterization of spike glycoprotein of SARS-CoV-2 on virus entry and its immune cross-reactivity with SARS-CoV. Nat Commun (2020) 11(1):1620. doi: 10.1038/s41467-020-15562-9
107. Liang JG, Su D, Song T-Z, Zeng Y, Huang W, Wu J, et al. S-trimer, a COVID-19 subunit vaccine candidate, induces protective immunity in nonhuman primates. Nat Commun (2021) 12(1):1346. doi: 10.1038/s41467-021-21634-1
108. Richmond P, Hatchuel L, Dong M, Ma B, Hu B, Smolenov I, et al. Safety and immunogenicity of s-trimer (SCB-2019), a protein subunit vaccine candidate for COVID-19 in healthy adults: a phase 1, randomised, double-blind, placebo-controlled trial. Lancet (London England) (2021) 397(10275):682–94. doi: 10.1016/S0140-6736(21)00241-5
109. Yang S, Li Y, Dai L, Wang J, He P, Li C, et al. Safety and immunogenicity of a recombinant tandem-repeat dimeric RBD-based protein subunit vaccine (ZF2001) against COVID-19 in adults: two randomised, double-blind, placebo-controlled, phase 1 and 2 trials. Lancet Infect Dis (2021) 21(8):1107–19. doi: 10.1016/S1473-3099(21)00127-4
110. Dutta NK, Mazumdar K, Gordy JT. The nucleocapsid protein of SARS–CoV-2: a target for vaccine development. J Virol (2020) 94(13):e00647–20. doi: 10.1128/JVI.00647-20
111. Li T, Zhang T, Gu Y, Li S, Xia N. Current progress and challenges in the design and development of a successful COVID-19 vaccine. Fundam Res (2021) 1(2):139–50. doi: 10.1016/j.fmre.2021.01.011
112. Heath PT, Galiza EP, Baxter DN, Boffito M, Browne D, Burns F, et al. Safety and efficacy of NVX-CoV2373 covid-19 vaccine. N Engl J Med (2021) 385(13):1172–83. doi: 10.1056/NEJMoa2107659
113. Mallory RM, Formica N, Pfeiffer S, Wilkinson B, Marcheschi B, Albert G, et al. Safety and immunogenicity following a homologous booster dose of a SARS-CoV-2 recombinant spike protein vaccine (NVX-CoV2373): A secondary analysis of a randomised, placebo-controlled, phase 2 trial. Lancet Infect Dis. (2022) 10:S1473–3099(22)00420-0. doi: 10.1016/S1473-3099(22)00420-0
114. Chavda VP, Bezbaruah R, Athalye M, Parikh PK, Chhipa AS, Patel S, et al. Replicating viral vector-based vaccines for COVID-19: Potential avenue in vaccination arena. Viruses (2022) 14:759. doi: 10.3390/v14040759
115. Dai L, Gao GF. Viral targets for vaccines against COVID-19. Nat Rev Immunol (2021) 21(2):73–82. doi: 10.1038/s41577-020-00480-0
116. Kyriakidis NC, López-Cortés A, González EV, Grimaldos AB, Prado EO. SARS-CoV-2 vaccines strategies: a comprehensive review of phase 3 candidates. NPJ Vaccines (2021) 6(1):28. doi: 10.1038/s41541-021-00292-w
117. WHO. COVID-19 vaccine tracker and landscape (2022). Available at: https://www.who.int/publications/m/item/draft-landscape-of-covid-19-candidate-vaccines.
118. Scully M, Singh D, Lown R, Poles A, Solomon T, Levi M, et al. Pathologic antibodies to platelet factor 4 after ChAdOx1 nCoV-19 vaccination. N Engl J Med (2021) 384(23):2202–11. doi: 10.1056/NEJMoa2105385
119. Cines DB, Bussel JB. SARS-CoV-2 vaccine–induced immune thrombotic thrombocytopenia. N Engl J Med (2021) 384(23):2254–6. doi: 10.1056/NEJMe2106315
120. Barrett J, Belij-Rammerstorfer S, Dold C. Phase 1/2 trial of SARS-CoV-2 vaccine ChAdOx1 nCoV-19 with a booster dose induces multifunctional antibody responses. Med KE-N Nat (2021) 27(2):279–88. doi: 10.1038/s41591-020-01179-4
121. Voysey M, Clemens S, Madhi S, Weckx LY. Single-dose administration and the influence of the timing of the booster dose on immunogenicity and efficacy of ChAdOx1 nCoV-19 (AZD1222) vaccine. Lancet (2021) 397(10277):881–91. doi: 10.1016/S0140-6736(21)00432-3
122. Polinski JM, Weckstein AR, Batech M, Kabelac C, Kamath T, Harvey R, et al. Durability of the single-dose Ad26.COV2.S vaccine in the prevention of COVID-19 infections and hospitalizations in the US before and during the delta variant surge. JAMA Netw Open (2022) 5(3):e222959–e222959. doi: 10.1001/jamanetworkopen.2022.2959
123. Bekker L-G, Garrett N, Goga A, Fairall L, Reddy T, Yende-Zuma N, et al. Effectiveness of the Ad26.COV2.S vaccine in health-care workers in south Africa (the sisonke study): results from a single-arm, open-label, phase 3B, implementation study. Lancet (2022) 399(10330):1141–53. doi: 10.1016/S0140-6736(22)00007-1
124. Hager KJ, Pérez Marc G, Gobeil P, Diaz RS, Heizer G, Llapur C, et al. Efficacy and safety of a recombinant plant-based adjuvanted covid-19 vaccine. N Engl J Med (2022) 386(22):2084–96. doi: 10.1056/NEJMoa2201300
125. Zhang Z, Mateus J, Coelho CH, Dan JM, Moderbacher CR, Galvez RI, et al. Humoral and cellular immune memory to four COVID-19 vaccines. Cell (2022) 185(14):2434–2451.e17. doi: 10.1016/j.cell.2022.05.022
126. Kanokudom S, Assawakosri S, Suntronwong N, Auphimai C, Nilyanimit P, Vichaiwattana P, et al. Safety and immunogenicity of the third booster dose with inactivated, viral vector, and mRNA COVID-19 vaccines in fully immunized healthy adults with inactivated vaccine. Vaccines (2022) 10(1):86. doi: 10.3390/vaccines10010086
127. Marra AR, Miraglia JL, Malheiros DT, Guozhang Y, Teich VD, Da Silva Victor E, et al. Effectiveness of two COVID-19 vaccines (viral vector and inactivated viral vaccine) against SARS-CoV-2 infection in a cohort of healthcare workers. Infect Control Hosp Epidemiol (2022), 1–20. doi: 10.1017/ice.2022.50
128. Lauring AS, Hodcroft EB. Genetic variants of SARS-CoV-2–what do they mean? JAMA (2021) 325(6):529–31. doi: 10.1001/jama.2020.27124
129. Bian L, Gao F, Zhang J, He Q, Mao Q, Xu M, et al. Effects of SARS-CoV-2 variants on vaccine efficacy and response strategies. Expert Rev Vaccines (2021) 20(4):365–73. doi: 10.1080/14760584.2021.1903879
130. Al Kaabi N, Zhang Y, Xia S, Yang Y, Al Qahtani MM, Abdulrazzaq N, et al. Effect of 2 inactivated SARS-CoV-2 vaccines on symptomatic COVID-19 infection in adults: A randomized clinical trial. JAMA - J Am Med Assoc (2021) 326(1):35–45. doi: 10.1001/jama.2021.8565
131. Emary KRW, Golubchik T, Aley PK, Ariani CV, Angus B, Bibi S, et al. Efficacy of ChAdOx1 nCoV-19 (AZD1222) vaccine against SARS-CoV-2 variant of concern 202012/01 (B.1.1.7): an exploratory analysis of a randomised controlled trial. Lancet (London England) (2021) 397(10282):1351–62. doi: 10.1016/S0140-6736(21)00628-0
132. Evans JP, Qu P, Zeng C, Zheng Y-M, Carlin C, Bednash JS, et al. Neutralization of the SARS-CoV-2 deltacron and BA.3 variants. N Engl J Med (2022) 386(24):2340–2. doi: 10.1056/NEJMc2205019
133. Andrews N, Stowe J, Kirsebom F, Toffa S, Rickeard T, Gallagher E, et al. Covid-19 vaccine effectiveness against the omicron (B.1.1.529) variant. N Engl J Med (2022) 386(16):1532–46. doi: 10.1056/NEJMoa2119451
134. Raps org. COVID-19 vaccne tracker RAPS, Vol. 20. (2020) Available at:https://www.raps.org/news-and-articles/news-articles/2020/3/covid-19-vaccine-tracker, Accessed on 15/07/2022
135. Craven J. COVID-19 therapeutics tracker. (Rockville, Maryland: Regul Aff Prof Soc (RAPS)) (2020) p. 1–3.
136. Covid- RNA vaccine data. In: COVID-19 COVID-19 vaccines work what we know about how well COVID-19 vaccines are working real-world conditions is reassuring. research suggests that for mRNA COVID-19 vaccines , two doses are better than one. COVID-19 vaccine breakthrough cases. p. 19–20. Available at:https://www.hopkinsmedicine.org/health/conditions-and-diseases/coronavirus/coronavirus-vaccines-infographic, Accessed on 15/07/22
137. Fabiani M, Ramigni M, Gobbetto V, Mateo-Urdiales A, Pezzotti P, Piovesan C. Effectiveness of the comirnaty (BNT162b2, BioNTech/Pfizer) vaccine in preventing SARS-CoV-2 infection among healthcare workers, treviso province, veneto region, Italy, 27 December 2020 to 24 march 2021. Eurosurveillance (2021) 26(17):1–7. doi: 10.2807/1560-7917.ES.2021.26.17.2100420
138. Veneti L, Dag Berild J, Watle SV, Starrfelt J, Greve-Isdahl M, Langlete P, et al. Vaccine effectiveness with BNT162b2 (Comirnaty, pfizer-BioNTtech) vaccine against reported SARS-CoV-2 delta and omicron infection among adolescents, Norway, august 2021 to January 2022. SSRN Electron J (2022) 2:1–11. doi: 10.2139/ssrn.4062776
139. Wu K, Werner AP, Moliva JI, Koch M, Choi A, Stewart-Jones GBE, et al. mRNA-1273 vaccine induces neutralizing antibodies against spike mutants from global SARS-CoV-2 variants. bioRxiv : preprint server Biol (2021) Preprint, 01.25.427948. doi: 10.1101/2021.01.25.427948
140. Bruxvoort KJ, Sy LS, Qian L, Ackerson BK, Luo Y, Lee GS, et al. Effectiveness of mRNA-1273 against delta, mu, and other emerging variants of SARS-CoV-2: Test negative case-control study. BMJ (2021) 375:1–10. doi: 10.1136/bmj-2021-068848
141. Menni C, Klaser K, May A, Polidori L, Capdevila J, Louca P, et al. Vaccine side-effects and SARS-CoV-2 infection after vaccination in users of the COVID symptom study app in the UK: a prospective observational study. Lancet Infect Dis (2021) 21(7):939–49. doi: 10.1016/S1473-3099(21)00224-3
142. Thiagarajan K. Covid-19: India is at centre of global vaccine manufacturing, but opacity threatens public trust. BMJ (2021) 372:n196. doi: 10.1136/bmj.n196
143. . Sputnik Vaccine registration , efficacy , price , side effects , dose Gap.0 (2021). pp. 1–64, Registration SV, Effects S, Gap D. Available at:https://www.pacehospital.com/sputnik-v-vaccine-efficacy-doses-side-effects-and-price; Accessed on 15/07/22
144. Green LA. Sputnik Light COVID-19 vaccine: What we know (2021). pp. 1–8, Covid- SL. Available at:https://www.medicalnewstoday.com/articles/sputnik-light-covid-19-vaccine-what-we-know; Accessed on 15/07/22
145. Tukhvatulin AI, Dolzhikova IV, Shcheblyakov DV, Zubkova OV, Dzharullaeva AS, Kovyrshina AV, et al. An open, non-randomised, phase 1/2 trial on the safety, tolerability, and immunogenicity of single-dose vaccine “Sputnik light” for prevention of coronavirus infection in healthy adults. Lancet Reg Heal - Eur (2021) 11:100241. doi: 10.1016/j.lanepe.2021.100241
146. Johnson & Johnson. Positive new data for Johnson & Johnson single-shot COVID-19 vaccine on activity against delta variant and long-lasting durability of response. (2021) Available at:https://www.jnj.com/positive-new-data-for-johnson-johnson-single-shot-covid-19-vaccine-on-activity-against-delta-variant-and-long-lasting-durability-of-response ; Accessed on 15/07/22
147. WHO. The sinovac COVID-19 vaccine: What you need to know (2021). Available at: https://www.who.int/news-room/feature-stories/detail/the-sinovac-covid-19-vaccine-what-you-need-to-know#:~:text.
148. Craven J. COVID-19 vaccine tracker . regulatory FocusTM (2021). Available at: https://www.raps.org/news-and-articles/news-articles/2020/3/covid-19-vaccine-tracker.
149. Biotech B. COVAXIN ® - India ’ s first indigenous COVID-19 vaccine, Vol. 154. (2021). pp. 1–6, Covid- FI. Accessed on 15/07/22
150. Hammerschmidt SI, Bosnjak B, Bernhardt G, Friedrichsen M, Ravens I, Dopfer-Jablonka A, et al. Neutralization of the SARS-CoV-2 delta variant after heterologous and homologous BNT162b2 or ChAdOx1 nCoV-19 vaccination. Cell Mol Immunol (2021) 18(10):2455–6. doi: 10.1038/s41423-021-00755-z
151. Barros-Martins J, Hammerschmidt SI, Cossmann A, Odak I, Stankov MV, Morillas Ramos G, et al. Immune responses against SARS-CoV-2 variants after heterologous and homologous ChAdOx1 nCoV-19/BNT162b2 vaccination. Nat Med (2021) 27(9):1525–9. doi: 10.1038/s41591-021-01449-9
152. Shen X. Boosting immunity to omicron. Nat Med (2022) 28(3):445–6. doi: 10.1038/s41591-022-01727-0
153. Bates TA, McBride SK, Leier HC, Guzman G, Lyski ZL, Schoen D, et al. Vaccination before or after SARS-CoV-2 infection leads to robust humoral response and antibodies that effectively neutralize variants. Sci Immunol (2022) 7(68):eabn8014. doi: 10.1126/sciimmunol.abn8014
154. WHO. WHO releases global COVID-19 vaccination strategy update to reach unprotected. news release (2022). Available at: https://www.who.int/news/item/22-07-2022-who-releases-global-covid-19-vaccination-strategy-update-to-reach-unprotected.
155. Watson OJ, Barnsley G, Toor J, Hogan AB, Winskill P, Ghani AC. Global impact of the first year of COVID-19 vaccination: A mathematical modelling study. Lancet Infect Dis (2022) 22(9):1293–302. doi: 10.1016/S1473-3099(22)00320-6
156. Megget K. What are the risks of fast-tracking a covid-19 vaccine? In: Chemistry world (2021). Available at: https://www.chemistryworld.com/news/what-are-the-risks-of-fast-tracking-a-covid-19-vaccine/4012130.article. Accessed on 15/07/22
157. Klein NP, Lewis N, Goddard K, Fireman B, Zerbo O, Hanson KE, et al. Surveillance for adverse events after COVID-19 mRNA vaccination. JAMA (2021) 326(14):1390–9. doi: 10.1001/jama.2021.15072
158. Krause PR, Gruber MF. Emergency use authorization of covid vaccines - safety and efficacy follow-up considerations. N Engl J Med (2020) 383(19):e107. doi: 10.1056/NEJMp2031373
159. Forni G, Mantovani A, Forni G, Mantovani A, Moretta L, Rappuoli R, et al. COVID-19 vaccines: Where we stand and challenges ahead. Cell Death Differ (2021) 28(2):626–39. doi: 10.1038/s41418-020-00720-9
160. Kazancoglu Y, Sezer MD, Ozbiltekin-Pala M, Kucukvar M. Investigating the role of stakeholder engagement for more resilient vaccine supply chains during COVID-19. Oper Manag Res (2022) 15(1-2):428–39. doi: 10.1007/s12063-021-00223-x
161. Fahrni ML, Ismail IA-N, Refi DM, Almeman A, Yaakob NC, Saman KM, et al. Management of COVID-19 vaccines cold chain logistics: a scoping review. J Pharm Policy Pract (2022) 15(1):16. doi: 10.1186/s40545-022-00411-5
162. Gamad N, Shafiq N, Mohindra R, Bhalla A, Malhotra S. Some reflections on vaccine research ethics during COVID-19 pandemic. Postgrad Med J (2021) 98(e2):e84–5. doi: 10.1136/postgradmedj-2020-139145
164. Van Norman GA. Limitations of animal studies for predicting toxicity in clinical trials: Part 2: Potential alternatives to the use of animals in preclinical trials. JACC Basic to Transl Sci . 2020/04/27. (2020) 5(4):387–97. doi: 10.1016/j.jacbts.2020.03.010
165. Porat T, Burnell R, Calvo RA, Ford E, Paudyal P, Baxter WL, et al. “Vaccine passports” may backfire: Findings from a cross-sectional study in the UK and Israel on willingness to get vaccinated against COVID-19. Vaccines (2021) 9:902. doi: 10.3390/vaccines9080902
166. Chavda VP, Chen Y, Dave J, Chen Z-S, Chauhan SC, Yallapu MM, et al. COVID-19 and vaccination: myths vs science. Expert Rev Vaccines (2022) Online ahead of print, 1–18. doi: 10.1080/14760584.2022.2114900
167. Greenwood D, Taverner T, Adderley NJ, Price M, Gokhale K, Sainsbury C, et al. Machine learning of COVID-19 clinical data identifies population structures with therapeutic potential. iScience (2022) 25(7):104480. doi: 10.1016/j.isci.2022.104480
168. Chavda VP, Vora LK, Pandya AK, Patravale VB. Intranasal vaccines for SARS-CoV-2: From challenges to potential in COVID-19 management. Drug Discov Today (2021) 26(11):2619–36. doi: 10.1016/j.drudis.2021.07.021
169. Chavda VP, Vora LK, Apostolopoulos V. Inhalable vaccines: Can they help control pandemics? Vaccines (2022) 10(8):1309. doi: 10.3390/vaccines10081309
Keywords: COVID-19 outbreak, SARS-CoV-2 variants, vaccine, variant of concern, omicron variant, delta variant
Citation: Chavda VP, Yao Q, Vora LK, Apostolopoulos V, Patel CA, Bezbaruah R, Patel AB and Chen Z-S (2022) Fast-track development of vaccines for SARS-CoV-2: The shots that saved the world. Front. Immunol. 13:961198. doi: 10.3389/fimmu.2022.961198
Received: 04 June 2022; Accepted: 08 September 2022;
Published: 03 October 2022.
Edited by:
Giorgio Fedele, National Institute of Health (ISS), ItalyReviewed by:
Faisal Raza, Shanghai Jiao Tong University, ChinaCopyright © 2022 Chavda, Yao, Vora, Apostolopoulos, Patel, Bezbaruah, Patel and Chen. This is an open-access article distributed under the terms of the Creative Commons Attribution License (CC BY). The use, distribution or reproduction in other forums is permitted, provided the original author(s) and the copyright owner(s) are credited and that the original publication in this journal is cited, in accordance with accepted academic practice. No use, distribution or reproduction is permitted which does not comply with these terms.
*Correspondence: Vivek P. Chavda, vivek.chavda@lmcp.ac.in ; Zhe-Sheng Chen, Chenz@stjohns.edu; Vasso Apostolopoulos, vasso.apostolopoulos@vu.edu.au
†These authors have contributed equally to this work and share first authorship
‡ORCID: Vivek P. Chavda, 0000-0002-7701-8597
Vasso Apostolopoulos, 0000-0001-6788-2771
Zhe-Sheng Chen, 0000-0002-8289-097X
Disclaimer: All claims expressed in this article are solely those of the authors and do not necessarily represent those of their affiliated organizations, or those of the publisher, the editors and the reviewers. Any product that may be evaluated in this article or claim that may be made by its manufacturer is not guaranteed or endorsed by the publisher.
Research integrity at Frontiers
Learn more about the work of our research integrity team to safeguard the quality of each article we publish.