- 1Department of Pharmacology, Okayama University Graduate School of Medicine, Dentistry, and Pharmaceutical Sciences, Okayama, Japan
- 2Translational Research and Drug Development, Okayama University Graduate School of Medicine, Dentistry, and Pharmaceutical Sciences, Okayama, Japan
Introduction
As of February 2022, the COVID-19 pandemic, which began at the end of 2019, has now killed 5.6 million people and infected hundreds of millions of others. One of the biggest concerns since the early days of the pandemic has been the development of drugs to control the severity of the disease, and several drugs have been proposed and are already in clinical use. However, in light of the current pandemic situation, as well as possible future pandemics, it is still important to develop novel drugs with a more diverse range of therapeutic targets. In this opinion, we focus our attention on hemolysis as a candidate target. We consider possible mechanisms underlying the relation between COVID-19 severity and hemolysis, and the feasibility of using drug candidates (or repositioning) targeting hemolysis as a protective strategy against severe COVID-19.
Hemolysis in sepsis, including severe COVID-19-related sepsis
Many efforts have been made to treat hemolytic diseases, including congenital (e.g., sickle cell disease: SCD) and acquired (e.g., autoimmune disease, heterozygous transfusion) hemolytic diseases, which are characterized by increased destruction of red blood cells (RBC). The adverse effects of hemolysis include not only a decrease in oxygen-carrying capacity of the blood due to RBC depletion, but also the damaging activities of free hemoglobin, heme, and Fe2+ as damage associated molecular patterns (DAMPs). These three hemolytic products produce signals and amplify inflammation via toll-like receptors (TLRs) and oxidative stress. Hemoglobin causes vasoconstriction via nitric oxide inactivation (1). Heme has been reported to be highly toxic to many types of cells through its promotion of free radical formation and oxidative stress. On the other hand, Fe2+ produces hydroxyl radicals through the Fenton reaction (2), which is also harmful to the cells. In addition to the damage caused by these products, the nephrotoxicity caused by severe hemolysis can be lethal (3, 4). Recently, hemolysis has also been recognized as an aggravating factor in sepsis (5), but there is still controversy in regard to suitable targets for the treatment of hemolysis.
In the context of COVID-19, several reports have considered the potential of hemolysis as both a biomarker and therapeutic target, but to our best knowledge, no clinical trials targeting hemolysis are undergoing. Reports regarding the possibility of using hemolysis in COVID-19 as a biomarker have considered various indices of hemolysis for this purpose, including decreased hemoglobin (6, 7), elevated free heme (8), increased RBC distribution width (RDW) (9), high ferritin, high lactate dehydrogenase (10, 11), and high bilirubin (12). Heme oxygenase-1 (HO-1) induction (13), and supplementation of haptoglobin and hemopexin (14) have anti-inflammatory effects via scavenging and clearance of hemolysis-related degradation products. In addition, it has been reported that protecting RBCs against hemolysis is important for the proper functioning of the central nervous system (15). Moreover, the increasing risk is reported in the case of COVID-19 infection in patient with glucose-6-phosphtase dehydrogenase (G6PD) deficiency, who usually have structurally vulnerable RBCs which are easy to hemolysis, has been suggested (16). There are also reports on changes in structural proteins and membrane lipids remodeling in RBCs in COVID-19 patients (17). From these data, both targeting hemolysis-related products and protection of RBCs are considered to be reasonable therapeutic strategies to protect against the development of severe COVID-19.
Many of the mechanisms in terms of COVID-19 related hemolysis are thought to be similar to those reported in the pathogenesis of sepsis (e.g., mechanisms involving inflammation, complement activation, autoantibodies) (18). However, mechanisms specific for COVID-19 have also been proposed, for example, viral invasion of the SARS-CoV-2 virus into RBCs (through ACE2, CD147, CD26), which then undergo hemolysis (19–21). Regarding viral infection to RBCs, binding of virus and heme may lead to resistance against the anti-viral effect of heme. In other words, a component protein of SARS-CoV2, such as open-reading frame 8, may bind to heme, resulting in decreased anti-viral activity of heme-induced miRNA processing (22). In fact, a study using a computational-experimental approach reported a direct binding between SARS-CoV2 and heme (23). Interestingly, there are many viruses that have been reported to have an affinity for RBCs (e.g., hepatitis C and B, Ebola, human immunodeficiency virus (HIV), dengue, and Zika) (24–28). On the other hand, there is a report of 6 patients with paroxysmal nocturnal hematuria (PNH) who developed severe hemolysis after the vaccine for COVID-19 (29), which implies that some autoantibodies against SARS-CoV2 component proteins may cause hemolysis (30).
In addition to hemolysis, a recent study has conjectured that increased erythrophagocytosis may cause the decrease in hemoglobin in COVID-19 patients (31). In this case, the mechanism would involve TLR9 on the surface of RBCs binding to mitochondrial CpG, which would lead to decreased RBC deformability and erythrophagocytosis by macrophages (31). This suggests that RBCs are “immune sentinels” that work at the forefront of the immune system (32). In other words, RBCs block the invasion of viruses, including SARS-CoV2, and thus any entry of the virus into RBCs would be part of a biological defense response rather than a passive invasion.
Lastly, we should consider the relationship between COVID-19 and previously reported severity factors, including hypertension, obesity, diabetes, and thromboembolism caused by neutrophil extracellular traps (NETs) (33–35). Interestingly, hemolytic products such as free heme have been shown to induce NETs as DAMPs (36, 37). Thus, hemolysis could be a marker of disease progression, and a therapeutic target.
Therapeutic mechanisms of dexamethasone in COVID-19
One of the reasons why we focus on the importance of hemolysis in COVID-19 is that dexamethasone is known to have a beneficial effect in the treatment of COVID-19 (38–41). Dexamethasone is one of the earliest therapeutic agents to be applied clinically, as its efficacy was demonstrated in several clinical trials in the early stages of COVID-19. However, its therapeutic mechanism is still not fully understood (42). Surprisingly, although corticosteroids, including dexamethasone, have long been used clinically for sepsis (mainly bacterial sepsis), the results of multiple trials have reported that efficacy of corticosteroids cannot be proven until now (43, 44). Whether viral or bacterial (or fungal), the pathways of the sepsis cascade containing dysregulated innate immune responses are similar in many respects, but the effect of dexamethasone was clearly demonstrated in COVID-19. We hypothesize that an acceleration in the scavenging of hemolytic products by dexamethasone may be one of the therapeutic mechanisms. In fact, corticosteroid therapy has been used for decades in patients with SCD and PNH, which are typical hemolytic diseases (45–47). Corticosteroids have a wide range of pharmacological effects, but the main one is a strong immunosuppressive effect via the glucocorticoid receptor, and one corticosteroid, dexamethasone, is known to promote scavenging of hemolytic products by inducing CD163 receptor expression (48–51). The CD163 receptor is widely known as a scavenger receptor for the hemoglobin-haptoglobin complex, which is abundantly expressed in macrophages (52). In the pathogenesis of COVID-19, the CD163 induction effect of dexamethasone may suppress the deteriorated inflammation by hemolytic products such as free hemoglobin. Since sepsis is a disease condition involving a wide spectrum of pathological processes, the degree of involvement of hemolysis may vary greatly in each individual. In the case of COVID-19-induced sepsis, it is possible that hemolysis might be an exacerbating factor for transition from mild to severe COVID-19 based on the clinical examination data.
Another study reported that dexamethasone was effective in a group of COVID-19 patients who were ventilated or oxygenated, while there was no reduction in mortality in the patients without oxygen (41). A possible explanation for this may be hemolytic toxicity via free radical generation by high oxygen concentration, or ventilator-related hemolysis. On the other hand, in the group of patients whose blood oxygen saturation was so low that they required a ventilator or oxygen, hemolysis at the alveolar level (leakage of RBC into the alveolar space) was prominent, suggesting that the hemolysis-scavenging effect of dexamethasone was of great benefit. In any case, it is clear that dexamethasone is effective for preventing mortality in patients with COVID-19. By setting a new target of scavenging hemolytic products, it would be possible to consider a combination therapy with anti-hemolytic serum proteins, such as haptoglobin and hemopexin, to maximize the efficacy of CD163 upregulation by dexamethasone.
Impaired scavenging hemolytic products under inflammatory conditions
The mechanism of hemolysis in the pathogenesis of severe infections, the amplification of inflammation by hemolytic products and the direct cytotoxicity of those products have already been extensively investigated (2, 18). In this section, we discuss the impaired efficacy of scavenging hemolytic products under the inflammatory condition, which may be a new therapeutic target.
There are two main processing systems for hemolysis: a free hemoglobin processing system using haptoglobin (52), and a free heme processing system by hemopexin (53). Both systems function by endocytosis via scavenging receptors (CD163 and CD91, respectively) of macrophages and detoxification of hemolytic products by a degradation and recycling system thorough HO-1. It is clear that these physiological processing systems for hemolysis are depleted or fail to adapt to acute massive hemolysis or chronic persistent hemolysis. Therefore, we focus on the hemolysis in COVID-19 and consider the change in scavenging of hemolysis-related products under severe inflammation and tissue destruction environment. In particular, in the environment of ARDS, which is a major cause of death in COVID-19, increased vascular permeability due to inflammation is evident. ARDS is also associated with the impairment of vascular endothelial cells, which causes RBC leakage, hemolysis, and amplification of inflammation in the extravascular spaces. Furthermore, increased dead cells as well as overloaded macrophages by hemoglobin could cause a change in the microenvironment, including the pH, and could increase the release of some metal ions from platelets and activated mast cells (54, 55). These factors may be responsible for the delayed scavenging of hemolytic products. Thus, the therapeutic targets for hemolysis in sepsis may include preservation of the CD163 scavenger system, such as chelation for excessive metal ions in the microenvironment in addition to the usual therapeutic strategies. Indeed, shedding of the CD163 receptor occurs under sepsis-mimicking conditions (e.g., TLR4 stimulation by lipopolysaccharide) (56). Furthermore, during treatment of acute myelogenous leukemia with gemutuzumab ozogamicin (a CD33-targeted antibody-conjugate), CD163-positive macrophages are impaired and the scavenger ability for hemolysis-related products is significantly reduced (57, 58). In summary, it is necessary to consider the possibility that the impaired scavenging function of CD163-positive macrophages may occur under specific conditions.
Discussion
Typical hemolytic diseases include SCD and thalassemia caused by hereditary β-globin abnormality, and acquired hemolytic anemias such as autoimmune hemolytic disease, PNH, and mechanical hemolysis caused by cardiopulmonary bypass. In recent years, although it has been recognized that hemolysis is also a factor in the severity of sepsis, no anti-hemolysis therapy has been used in clinical settings. In this opinion, we propose hemolysis as a new therapeutic target in COVID-19, because severe COVID-19 is a viral sepsis with a characteristic course of rapid deterioration of ARDS, and thromboembolism with suspected NETs (Figure 1).
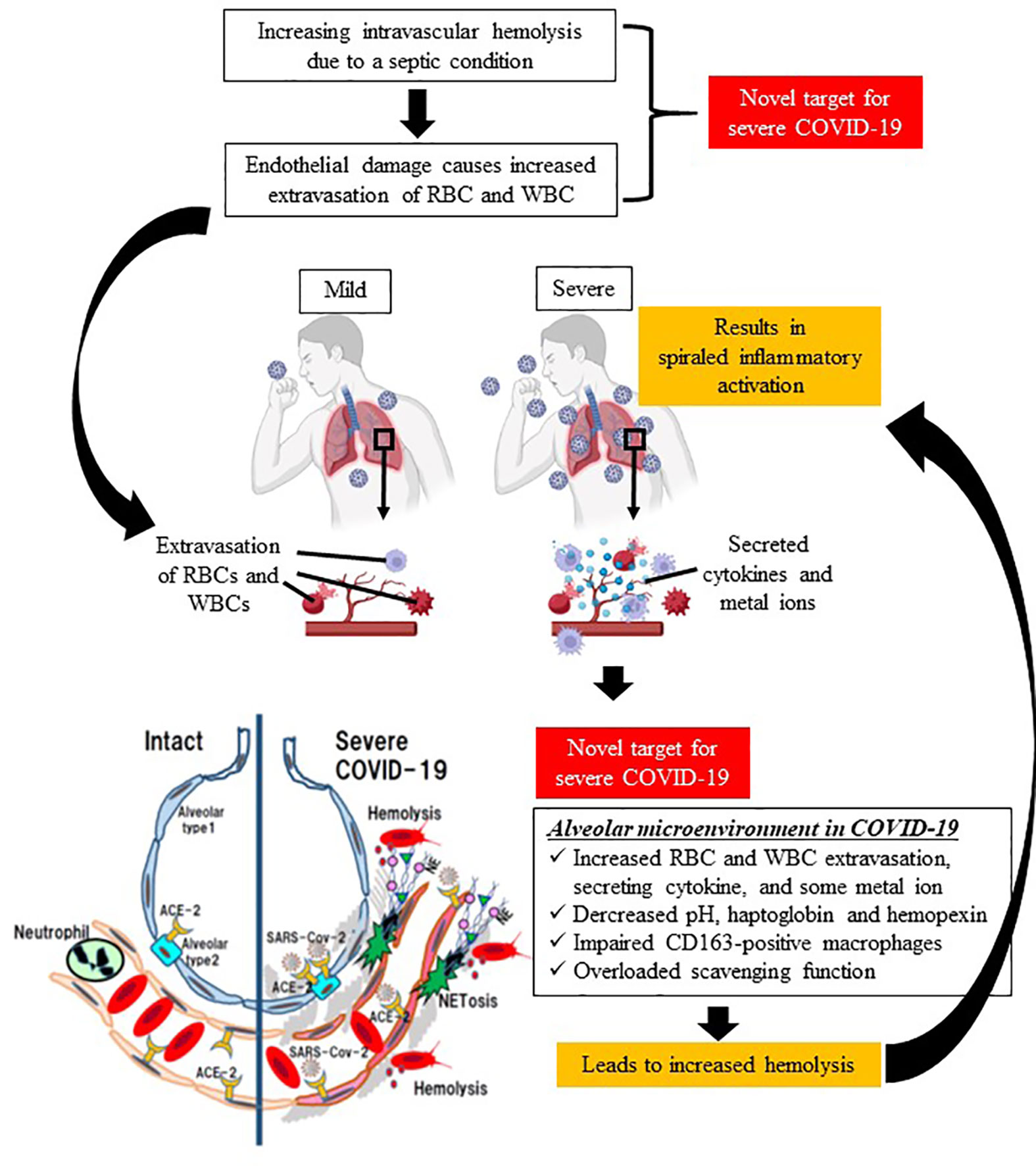
Figure 1 Schematic diagram of spiraled inflammatory activation and tissue damage by hemolysis in severe COVID-19. Sepsis causes intravascular hemolysis by many mechanisms (e.g., microvasculitis, complement activation, glucose metabolism, and eryptosis), leading to increased vascular permeability, and thereby extravasation of both red blood cells and immune system cells. The first target in the proposed treatment strategy is a decrease in intravascular hemolysis. In terms of the alveolar microenvironment, increases in extravasated cells, cytokines, and metal ions deteriorate hemolysis in the extracellular space, which is associated with spiraled inflammatory activation in lung issue. Therefore, the second therapeutic target is a decrease in extravascular hemolysis. (This figure was created at BioRender.com.).
The mechanism of hemolysis in COVID-19 is thought to be similar to that in sepsis (microangiitis, vascular occlusion, eryptosis, membrane deformability, complement activation, hypoglycemia, etc.) (5), but some unique factors have been identified. These factors include direct RBC destruction by SARS-CoV2 (17, 20, 21) and autoantibody-induced hemolysis against viral proteins (30). The accumulation of hemolytic products due to increased hemolysis and delayed scavenging of hemolytic products may amplify the inflammatory response as DAMPs or aggravate the condition through direct tissue damage, which may be the mechanism leading to the severity of COVID-19.
Finally, in this opinion, we addressed the relevance of hemolysis as a novel therapeutic target to reduce the severity of COVID-19. It is necessary to verify hemolysis as a new target and accumulate knowledge in order to alleviate the current situation and prepare for future pandemics.
Author contributions
All authors listed have made a substantial, direct, and intellectual contribution to the work and approved it for publication.
Funding
This research was supported by AMED under Grant Number JP19im0210109h0003.
Conflict of interest
Author MN been an advisor of the Japan Blood Products Organization and obtains the research support from the company.
The remaining author declares that the research was conducted in the absence of any commercial or financial relationships that could be construed as a potential conflict of interest.
Publisher’s note
All claims expressed in this article are solely those of the authors and do not necessarily represent those of their affiliated organizations, or those of the publisher, the editors and the reviewers. Any product that may be evaluated in this article, or claim that may be made by its manufacturer, is not guaranteed or endorsed by the publisher.
References
1. Reiter CD, Wang X, Tanus-Santos JE, Hogg N, Cannon RO 3rd, Schechter AN, et al. Cell-free hemoglobin limits nitric oxide bioavailability in sickle-cell disease. Nat Med (2002) 8(12):1383–9. doi: 10.1038/nm1202-799
2. Bozza MT, Jeney V. Pro-inflammatory actions of heme and other hemoglobin-derived DAMPs. Front Immunol (2020) 11:1323. doi: 10.3389/fimmu.2020.01323
3. Camus SM, Gausserès B, Bonnin P, Loufrani L, Grimaud L, Charue D, et al. Erythrocyte microparticles can induce kidney vaso-occlusions in a murine model of sickle cell disease. Blood (2012) 120(25):5050–8. doi: 10.1182/blood-2012-02-413138
4. Van Avondt K, Nur E, Zeerleder S. Mechanisms of haemolysis-induced kidney injury. Nat Rev Nephrol (2019) 15(11):671–92. doi: 10.1038/s41581-019-0181-0
5. Larsen R, Gozzelino R, Jeney V, Tokaji L, Bozza FA, Japiassú AM, et al. A central role for free heme in the pathogenesis of severe sepsis. Sci Transl Med (2010) 2(51):51ra71. doi: 10.1126/scitranslmed.3001118
6. Lippi G, Mattiuzzi C. Hemoglobin value may be decreased in patients with severe coronavirus disease 2019. Hematol Transfus Cell Ther (2020) 42(2):116–7. doi: 10.1016/j.htct.2020.03.001
7. Algassim AA, Elghazaly AA, Alnahdi AS, Mohammed-Rahim OM, Alanazi AG, Aldhuwayhi NA, et al. Prognostic significance of hemoglobin level and autoimmune hemolytic anemia in SARS-CoV-2 infection. Ann Hematol (2021) 100(1):37–43. doi: 10.1007/s00277-020-04256-3
8. Su WL, Lin CP, Hang HC, Wu PS, Cheng CF, Chao YC. Desaturation and heme elevation during COVID-19 infection: A potential prognostic factor of heme oxygenase-1. J Microbiol Immunol Infect (2021) 54(1):113–6. doi: 10.1016/j.jmii.2020.10.001
9. Foy BH, Carlson JCT, Reinertsen E, Padros IVR, Pallares Lopez R, Palanques-Tost E, et al. Association of red blood cell distribution width with mortality risk in hospitalized adults with SARS-CoV-2 infection. JAMA Netw Open (2020) 3(9):e2022058. doi: 10.1001/jamanetworkopen.2020.22058
10. Wang D, Hu B, Hu C, Zhu F, Liu X, Zhang J, et al. Clinical characteristics of 138 hospitalized patients with 2019 novel coronavirus-infected pneumonia in wuhan, China. JAMA (2020) 323(11):1061–9. doi: 10.1001/jama.2020.1585
11. Zhou F, Yu T, Du R, Fan G, Liu Y, Liu Z, et al. Clinical course and risk factors for mortality of adult inpatients with COVID-19 in wuhan, China: a retrospective cohort study. Lancet (2020) 395(10229):1054–62. doi: 10.1016/S0140-6736(20)30566-3
12. Paliogiannis P, Zinellu A. Bilirubin levels in patients with mild and severe covid-19: A pooled analysis. Liver Int (2020) 40(7):1787–8. doi: 10.1111/liv.14477
13. Maiti BK. Heme/Hemeoxygenase-1 system is a potential therapeutic intervention for COVID-19 patients with severe complications. ACS Pharmacol Transl Sci (2020) 3(5):1032–4. doi: 10.1021/acsptsci.0c00136
14. Wagener F, Pickkers P, Peterson SJ, Immenschuh S, Abraham NG. Targeting the heme-heme oxygenase system to prevent severe complications following COVID-19 infections. Antioxid (Basel) (2020) 9(6):540. doi: 10.3390/antiox9060540
15. Akhter N, Ahmad S, Alzahrani FA, Dar SA, Wahid M, Haque S, et al. Impact of COVID-19 on the cerebrovascular system and the prevention of RBC lysis. Eur Rev Med Pharmacol Sci (2020) 24(19):10267–78. doi: 10.26355/eurrev_202010_23251
16. Aydemir D, Dağlıoğlu G, Candevir A, Kurtaran B, Bozdogan ST, Inal TC, et al. COVID-19 may enhance risk of thrombosis and hemolysis in the G6PD deficient patients. Nucleosides Nucleotides Nucleic Acids (2021) 40(5):505–17. doi: 10.1080/15257770.2021.1897457
17. Thomas T, Stefanoni D, Dzieciatkowska M, Issaian A, Nemkov T, Hill RC, et al. Evidence of structural protein damage and membrane lipid remodeling in red blood cells from COVID-19 patients. J Proteome Res (2020) 19(11):4455–69. doi: 10.1021/acs.jproteome.0c00606
18. Effenberger-Neidnicht K, Hartmann M. Mechanisms of hemolysis during sepsis. Inflammation (2018) 41(5):1569–81. doi: 10.1007/s10753-018-0810-y
19. Khakwani M, Horgan C, Ewing J. COVID-19-associated oxidative damage to red blood cells. Br J Haematol (2021) 193(3):481. doi: 10.1111/bjh.17317
20. Radzikowska U, Ding M, Tan G, Zhakparov D, Peng Y, Wawrzyniak P, et al. Distribution of ACE2, CD147, CD26, and other SARS-CoV-2 associated molecules in tissues and immune cells in health and in asthma, COPD, obesity, hypertension, and COVID-19 risk factors. Allergy (2020) 75(11):2829–45. doi: 10.1111/all.14429
21. Khawaja UA, Shamsoddin E, Desideri LF, Tovani-Palone MR. Infection of red blood cells by SARS-CoV-2: new evidence. Einstein (Sao Paulo) (2021) 19:eCE6285. doi: 10.31744/einstein_journal/2021CE6285
22. Rapozzi V, Juarranz A, Habib A, Ihan A, Strgar R. Is haem the real target of COVID-19? Photodiagnosis Photodyn Ther (2021) 35:102381. doi: 10.1016/j.pdpdt.2021.102381
23. Hopp MT, Domingo-Fernández D, Gadiya Y, Detzel MS, Graf R, Schmalohr BF, et al. Linking COVID-19 and heme-driven pathophysiologies: A combined computational-experimental approach. Biomolecules (2021) 11(5):644. doi: 10.3390/biom11050644
24. Drakesmith H, Prentice A. Viral infection and iron metabolism. Nat Rev Microbiol (2008) 6(7):541–52. doi: 10.1038/nrmicro1930
25. Lecerf M, Scheel T, Pashov AD, Jarossay A, Ohayon D, Planchais C, et al. Prevalence and gene characteristics of antibodies with cofactor-induced HIV-1 specificity. J Biol Chem (2015) 290(8):5203–13. doi: 10.1074/jbc.M114.618124
26. Gupta N, de Wispelaere M, Lecerf M, Kalia M, Scheel T, Vrati S, et al. Neutralization of Japanese encephalitis virus by heme-induced broadly reactive human monoclonal antibody. Sci Rep (2015) 5:16248. doi: 10.1038/srep16248
27. Assunção-Miranda I, Cruz-Oliveira C, Neris RL, Figueiredo CM, Pereira LP, Rodrigues D, et al. Inactivation of dengue and yellow fever viruses by heme, cobalt-protoporphyrin IX and tin-protoporphyrin IX. J Appl Microbiol (2016) 120(3):790–804. doi: 10.1111/jam.13038
28. Neris RLS, Figueiredo CM, Higa LM, Araujo DF, Carvalho CAM, Verçoza BRF, et al. Co-Protoporphyrin IX and Sn-protoporphyrin IX inactivate zika, chikungunya and other arboviruses by targeting the viral envelope. Sci Rep (2018) 8(1):9805. doi: 10.1038/s41598-018-27855-7
29. Gerber GF, Yuan X, Yu J, Cher BAY, Braunstein EM, Chaturvedi S, et al. COVID-19 vaccines induce severe hemolysis in paroxysmal nocturnal hemoglobinuria. Blood (2021) 137(26):3670–3. doi: 10.1182/blood.2021011548
30. Sahu KK, Borogovac A, Cerny J. COVID-19 related immune hemolysis and thrombocytopenia. J Med Virol (2021) 93(2):1164–70. doi: 10.1002/jmv.26402
31. Lam LKM, Murphy S, Kokkinaki D, Venosa A, Sherrill-Mix S, Casu C, et al. DNA Binding to TLR9 expressed by red blood cells promotes innate immune activation and anemia. Sci Transl Med (2021) 13(616):eabj1008. doi: 10.1126/scitranslmed.abj1008
32. Minton K. Red blood cells join the ranks as immune sentinels. Nat Rev Immunol (2021) 21(12):760–1. doi: 10.1038/s41577-021-00648-2
33. Veras FP, Pontelli MC, Silva CM, Toller-Kawahisa JE, de Lima M, Nascimento DC, et al. SARS-CoV-2-triggered neutrophil extracellular traps mediate COVID-19 pathology. J Exp Med (2020) 217(12):e20201129. doi: 10.1084/jem.20201129
34. Zuo Y, Yalavarthi S, Shi H, Gockman K, Zuo M, Madison JA, et al. Neutrophil extracellular traps in COVID-19. JCI Insight (2020) 5(11):e138999. doi: 10.1172/jci.insight.138999
35. Skendros P, Mitsios A, Chrysanthopoulou A, Mastellos DC, Metallidis S, Rafailidis P, et al. Complement and tissue factor-enriched neutrophil extracellular traps are key drivers in COVID-19 immunothrombosis. J Clin Invest (2020) 130(11):6151–7. doi: 10.1172/JCI141374
36. Kono M, Saigo K, Takagi Y, Takahashi T, Kawauchi S, Wada A, et al. Heme-related molecules induce rapid production of neutrophil extracellular traps. Transfusion (2014) 54(11):2811–9. doi: 10.1111/trf.12700
37. Nishibori M. Novel aspects of sepsis pathophysiology: NETs, endothliopathy, plasma glycoproteins and COVID-19. J Pharmacol Sci (2022) 150:9–20. doi: 10.1016/j.jphs.2022.06.001
38. Dequin PF, Heming N, Meziani F, Plantefève G, Voiriot G, Badié J, et al. Effect of hydrocortisone on 21-day mortality or respiratory support among critically ill patients with COVID-19: A randomized clinical trial. JAMA (2020) 324(13):1298–306. doi: 10.1001/jama.2020.16761
39. Tomazini BM, Maia IS, Cavalcanti AB, Berwanger O, Rosa RG, Veiga VC, et al. Effect of dexamethasone on days alive and ventilator-free in patients with moderate or severe acute respiratory distress syndrome and COVID-19: The CoDEX randomized clinical trial. JAMA (2020) 324(13):1307–16. doi: 10.1001/jama.2020.17021
40. Angus DC, Derde L, Al-Beidh F, Annane D, Arabi Y, Beane A, et al. Effect of hydrocortisone on mortality and organ support in patients with severe COVID-19: The REMAP-CAP COVID-19 corticosteroid domain randomized clinical trial. JAMA (2020) 324(13):1317–29. doi: 10.1001/jama.2020.17022
41. Horby P, Lim WS, Emberson JR, Mafham M, Bell JL, Linsell L, et al. Dexamethasone in hospitalized patients with covid-19. N Engl J Med (2021) 384(8):693–704. doi: 10.1056/NEJMoa2021436
42. Matthay MA, Thompson BT. Dexamethasone in hospitalised patients with COVID-19: addressing uncertainties. Lancet Respir Med (2020) 8(12):1170–2. doi: 10.1016/S2213-2600(20)30503-8
43. Venkatesh B, Finfer S, Cohen J, Rajbhandari D, Arabi Y, Bellomo R, et al. Adjunctive glucocorticoid therapy in patients with septic shock. N Engl J Med (2018) 378(9):797–808. doi: 10.1056/NEJMoa1705835
44. Vandewalle J, Libert C. Glucocorticoids in sepsis: To be or not to be. Front Immunol (2020) 11:1318. doi: 10.3389/fimmu.2020.01318
45. Griffin TC, McIntire D, Buchanan GR. High-dose intravenous methylprednisolone therapy for pain in children and adolescents with sickle cell disease. N Engl J Med (1994) 330(11):733–7. doi: 10.1056/NEJM199403173301101
46. Bernini JC, Rogers ZR, Sandler ES, Reisch JS, Quinn CT, Buchanan GR. Beneficial effect of intravenous dexamethasone in children with mild to moderately severe acute chest syndrome complicating sickle cell disease. Blood (1998) 92(9):3082–9. doi: 10.1182/blood.V92.9.3082
47. Parker C, Omine M, Richards S, Nishimura J, Bessler M, Ware R, et al. Diagnosis and management of paroxysmal nocturnal hemoglobinuria. Blood (2005) 106(12):3699–709. doi: 10.1182/blood-2005-04-1717
48. Morganelli PM, Guyre PM. IFN-gamma plus glucocorticoids stimulate the expression of a newly identified human mononuclear phagocyte-specific antigen. J Immunol (1988) 140(7):2296–304.
49. Schaer DJ, Boretti FS, Schoedon G, Schaffner A. Induction of the CD163-dependent haemoglobin uptake by macrophages as a novel anti-inflammatory action of glucocorticoids. Br J Haematol (2002) 119(1):239–43. doi: 10.1046/j.1365-2141.2002.03790.x
50. Boretti FS, Buehler PW, D'Agnillo F, Kluge K, Glaus T, Butt OI, et al. Sequestration of extracellular hemoglobin within a haptoglobin complex decreases its hypertensive and oxidative effects in dogs and guinea pigs. J Clin Invest (2009) 119(8):2271–80. doi: 10.1172/JCI39115
51. Vallelian F, Schaer CA, Kaempfer T, Gehrig P, Duerst E, Schoedon G, et al. Glucocorticoid treatment skews human monocyte differentiation into a hemoglobin-clearance phenotype with enhanced heme-iron recycling and antioxidant capacity. Blood (2010) 116(24):5347–56. doi: 10.1182/blood-2010-04-277319
52. Kristiansen M, Graversen JH, Jacobsen C, Sonne O, Hoffman HJ, Law SK, et al. Identification of the haemoglobin scavenger receptor. Nature (2001) 409(6817):198–201. doi: 10.1038/35051594
53. Hvidberg V, Maniecki MB, Jacobsen C, Højrup P, Møller HJ, Moestrup SK. Identification of the receptor scavenging hemopexin-heme complexes. Blood (2005) 106(7):2572–9. doi: 10.1182/blood-2005-03-1185
54. Kabu K, Yamasaki S, Kamimura D, Ito Y, Hasegawa A, Sato E, et al. Zinc is required for fc epsilon RI-mediated mast cell activation. J Immunol (2006) 177(2):1296–305. doi: 10.4049/jimmunol.177.2.1296
55. Mammadova-Bach E, Braun A. Zinc homeostasis in platelet-related diseases. Int J Mol Sci (2019) 20(21):5258. doi: 10.3390/ijms20215258
56. Weaver LK, Hintz-Goldstein KA, Pioli PA, Wardwell K, Qureshi N, Vogel SN, et al. Pivotal advance: activation of cell surface toll-like receptors causes shedding of the hemoglobin scavenger receptor CD163. J Leukoc Biol (2006) 80(1):26–35. doi: 10.1189/jlb.1205756
57. Maniecki MB, Hasle H, Friis-Hansen L, Lausen B, Nielsen OJ, Bendix K, et al. Impaired CD163-mediated hemoglobin-scavenging and severe toxic symptoms in patients treated with gemtuzumab ozogamicin. Blood (2008) 112(4):1510–4. doi: 10.1182/blood-2007-09-114165
Keywords: hemolysis, COVID-19, sepsis, haptoglobin, hemopexin, CD163, dexamethasone, free hemoglobin
Citation: Ousaka D and Nishibori M (2022) Is hemolysis a novel therapeutic target in COVID-19? Front. Immunol. 13:956671. doi: 10.3389/fimmu.2022.956671
Received: 30 May 2022; Accepted: 26 July 2022;
Published: 19 August 2022.
Edited by:
Ralf Kircheis, Syntacoll GmbH, GermanyReviewed by:
Christopher Lotz, University Hospital Würzburg, GermanyCopyright © 2022 Ousaka and Nishibori. This is an open-access article distributed under the terms of the Creative Commons Attribution License (CC BY). The use, distribution or reproduction in other forums is permitted, provided the original author(s) and the copyright owner(s) are credited and that the original publication in this journal is cited, in accordance with accepted academic practice. No use, distribution or reproduction is permitted which does not comply with these terms.
*Correspondence: Masahiro Nishibori, bWJvcmlAbWQub2theWFtYS11LmFjLmpw