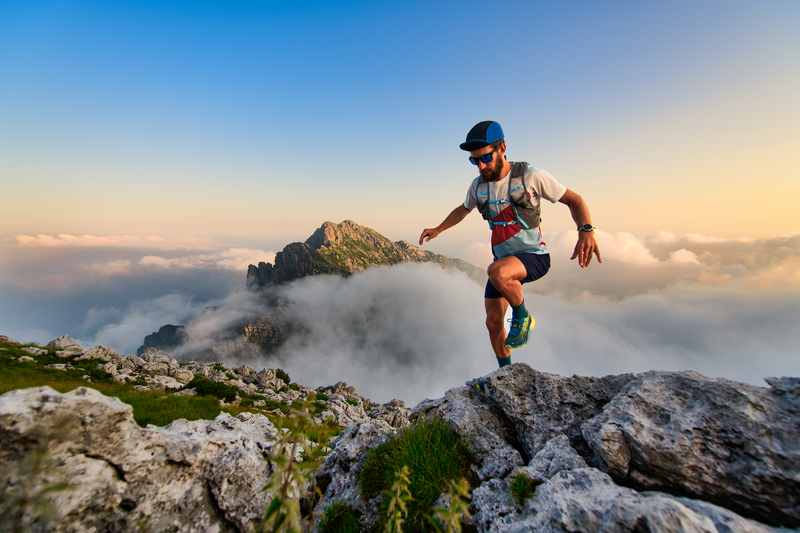
94% of researchers rate our articles as excellent or good
Learn more about the work of our research integrity team to safeguard the quality of each article we publish.
Find out more
REVIEW article
Front. Immunol. , 22 August 2022
Sec. Cancer Immunity and Immunotherapy
Volume 13 - 2022 | https://doi.org/10.3389/fimmu.2022.947885
This article is part of the Research Topic Novel Approaches in Immunotherapy to Overcoming Tumor Cell Intrinsic and/or Extrinsic Metabolic Limitations View all 7 articles
ATP and other nucleoside phosphates have specific receptors named purinergic receptors. Purinergic receptors and ectonucleotidases regulate various signaling pathways that play a role in physiological and pathological processes. Extracellular ATP in the tumor microenvironment (TME) has a higher level than in normal tissues and plays a role in cancer cell growth, survival, angiogenesis, metastasis, and drug resistance. In this review, we investigated the role of purinergic receptors in the development of resistance to therapy through changes in tumor cell metabolism. When a cell transforms to neoplasia, its metabolic processes change. The metabolic reprogramming modified metabolic feature of the TME, that can cause impeding immune surveillance and promote cancer growth. The purinergic receptors contribute to therapy resistance by modifying cancer cells’ glucose, lipid, and amino acid metabolism. Limiting the energy supply of cancer cells is one approach to overcoming resistance. Glycolysis inhibitors which reduce intracellular ATP levels may make cancer cells more susceptible to anti-cancer therapies. The loss of the P2X7R through glucose intolerance and decreased fatty acid metabolism reduces therapeutic resistance. Potential metabolic blockers that can be employed in combination with other therapies will aid in the discovery of new anti-cancer immunotherapy to overcome therapy resistance. Therefore, therapeutic interventions that are considered to inhibit cancer cell metabolism and purinergic receptors simultaneously can potentially reduce resistance to treatment.
Cancer is the second leading cause of mortality worldwide. In 2020, an estimated 19.3 million new cancer cases and 10.0 million cancer deaths are expected to be reported worldwide (1). Surgery, cytotoxic chemotherapy, radiation therapy, endocrine therapy, targeted therapy, and immunotherapy are the most common approaches in cancer treatments (2, 3). The immune system’s ability to recognize and, in some cases, successfully eliminate malignant cells has been demonstrated over the last century, leading to the development of various cancer immunotherapy strategies. One of these strategies is based on inhibiting immune checkpoints (ICPs). ICPs such as cytotoxic T-lymphocyte associated protein 4 (CTLA-4), programmed cell death 1 (PD-1), and programmed cell death ligand 1 (PD-L1) are immune system regulators that prevent the immune system from attacking cells. Some tumor cells can use these ICPs to escape immune response (4).
However, advancements in cancer treatment during the last decades had impressive effects; in the beginning, up to 90% of cancer-related deaths were caused by drug resistance and the subsequent inefficacy of treatment (5). Under the selective pressure of an immune response, cancer cells might develop distinct properties that enable them to escape detection by the immune system or even inhibit a functional immune response, resulting in tumor growth and relapse. So, resistance to conventional chemotherapeutic agents or innovative targeted medications remains a major issue in cancer treatment, accounting for most relapses and one of the leading causes of cancer death (6). A deeper knowledge of the mechanisms causing drug resistance is critically needed since it will contribute to establishing innovative therapeutic approaches that could improve clinical outcomes. On the other hand, metabolic reprogramming is required to accommodate the various demands of tumor cells during carcinogenesis, and recent studies have revealed its role in resistance to therapies. This review will describe how the specific receptors on the cell membrane, called purinergic receptors and its downstream signaling pathway, lead to therapy resistance by altering tumor cell metabolism. By reading this paper, readers will find out how purinergic receptors can provide the basis for treatment resistance with changes in tumor cell metabolism.
Each of the keywords purinergic receptors, therapeutic resistance, and cancer metabolism were searched once in pairs and once all in the Scopus, PubMed, and Google Scholar databases. The authors selected and studied the articles with titles that were exactly on these keywords. Any types of studies were included. The exclusion criteria were: articles published before 2010, unavailable articles, were not in English or were retracted. Duplicated articles are thrown out too. Then we summarize and integrate the findings from the papers into the full text as appropriate.
Cells respond to stress and damage (such as hypoxia) by releasing damage-associated molecular patterns (DAMPs), such as adenosine triphosphate (ATP). Extracellular ATP (eATP) can function as a “find me” signal for immune system cells and attract them to the location of the tissue damage (7). ATP can also be converted to adenosine (ADO) in two steps by CD39 and CD73 activity. ATP, uridine triphosphate (UTP), adenosine diphosphate (ADP), uridine diphosphate (UDP), and ADO are cellular mediators that have specific cell membrane ligands named purinergic receptors. Purinergic receptors and ectonucleotidases regulate various signaling pathways that play a role in physiological (transmitter and neurotransmitter) and pathological processes. Purinergic receptors are divided into three groups. Purinergic P0 receptors (P0Rs), purinergic P1 receptors (P1Rs), and purinergic P2 receptors (P2Rs): P0 responds to adenine, P1 (A1R, A2AR, A2BR, and A3R) responds to ADO, and P2 responds to ATP, ADP, UTP, and UDP. P2 is classified into two subfamilies: P2X, which are ion channels created by complexes of subunits (P2X1-P2X7) that stimulate fast depolarization coupled with Ca2+ and Na+ entry, as well as K+ export (Figure 1) (8), and P2Y, that have eight subtypes P2Y1, P2Y2, P2Y4, P2Y6, and P2Y11-14. ATP can activate P2Rs or be hydrolyzed by ectonucleotidases. Ectonucleoside triphosphate diphosphohydrolases (NTPDases, such as CD39), ectonucleoside pyrophosphatase/phosphodiesterase (ENPP), ecto-5’-nucleotidase (CD73), and alkaline phosphatases (AP) are the four groups of these enzymes (9). These enzymes create extra ligands for P2Y receptors in addition to restricting ATP signaling.
Figure 1 The effect of purinergic receptors on tumorigenesis and tumor cell metabolism. After binding to ATP, the purinergic receptor, P2X7R, removes K+ from the cell as Ca2+ and Na+ enter the cell. Also, extracellular ATP, due to the enzymatic function of CD39 and CD73, is converted to AMP and ADO. By binding to P1 receptors, ADO has tumor-promoting effects by inhibiting the immune response. Stimulation of the P1 receptor also causes activation of Akt and HIF-1a. An increase in intracellular Ca2+ activates PKC and PI3K, and PI3K by converting PIP2 to PIP3 activates Akt. As the main regulator, Akt activates HIF-1a, mTOR/VEGF, and NF-kb pathways and inactivates GSK-3, eventually leading to apoptosis inhibition, EMT, invasion, survival, and proliferation. Akt stimulates the glycolysis pathway. Glycolysis and OXPHOS or cell damage can produce ATP, leaving the cell through the PANX receptor. After binding to ATP, another purinergic receptor, P2YR, can increase ICAM-1, VCAM-1, and MMP, leading to adhesion and invasion of tumor cells. ADO, adenosine; AMP, adenosine monophosphate; ATP, adenosine triphosphate; CD39, cluster of differentiation 39; CD73, cluster of differentiation 73; DC, dendritic cell; EMT, epithelial-mesenchymal transition; GSK-3, glycogen synthase kinase-3; HIF-1a, hypoxia-inducible factor 1-alpha; ICAM-1, intercellular Adhesion Molecule 1; MMP, matrix metallopeptidase; mTOR, mammalian target of rapamycin; NF-kb, nuclear factor kappa B; NK cell, natural killer cell; OXPHOS, oxidative phosphorylation; PANX, pannexin; PI3K, phosphoinositide 3-kinases; PIP2, phosphatidylinositol-4, 5-bisphosphate; PIP3, phosphatidylinositol-3, 4, 5-triphosphate; PKC, protein kinase C; TAM, tumor-associated macrophage; Th2, T helper type 2; Treg, regulatory T cell; VCAM-1, vascular cell adhesion molecule 1; VEGF, vascular endothelial growth factor.
Intracellular ATP is released via the pannexin-1 (PANX1) and P2X7 receptor (P2X7R) channels into the extracellular space (10). eATP levels are 103 to 104 times greater in different cancer types than in normal tissues (11, 12). eATP has an important role in cancer cell survival, growth, and resistance. It was demonstrated that eATP internalizes the cell and increases intracellular ATP, leading to cancer cell survival and drug resistance (13, 14).
On the other hand, as mentioned above, ATP as a DAMP can play a role in the activation and maturation of tumor-specific dendritic cells (DCs), in a process called immunogenic cell death (ICD), along with other factors (15). The ICD process leads to the induction of a tumor-specific and long-lasting acquired immune response (16). This issue can indicate the role of ATP as an anti-tumor and treatment resistance reducer. Whether ATP reduces or increases the resistance to treatment can depend on its concentration, tumor stage and the receptor binded to it.
The substantial amount of ATP and ADO in the TME activate purinergic receptors, including P2X7R (17, 18). When P2X7R is activated, the nucleotide-binding and oligomerization domain (NOD)-, leucine-rich repeats (LRR)- and pyrin domain-containing protein 3 (NLRP3) inflammasome is assembled, and pro-inflammatory cytokines including interleukin-1 (IL-1) and IL-18 are released (19). Moreover, the C-terminal domain of the P2X7R is involved in signal transduction and pore formation, while the N-terminal domain could influence immune cell sensitivity to external nicotinamide adenine dinucleotide (NAD+) and ATP (20, 21). When the P2X7R is overstimulated, it causes a large membrane pore (megapore) formation in cooperation with PANX1, resulting in tumor cell apoptosis, while paradoxically, it can enhance tumor growth and is associated with a high tumor grade, proliferation, survival, and chemo-resistance (22–24), which is discussed below.
These contrasting effects of the P2X7R depend on its level of activation and maybe the cell types used as a model study. Also, the human P2X7R gene is highly polymorphic, and various receptor splice variants have been reported. They play different roles and make diverse outcomes in cancer. P2X7B variant has the protumoral effect as well as P2X7A while lacking the pore-forming cytotoxic activity as well as nfP2X7 (25, 26). The P2X7B and nfP2X7 variants appear to be more expressed than P2X7A on tumor cells and promote cell survival (26–28). The P2X7J variant seems to be over-expressed in cervical cancer and acts as a negative regulator of P2X7A by decreasing its cytotoxic activity (29). Finally, the P2X7-V3 variant acts as long non-coding RNA (lncRNA) and increases the proliferation of tumor cells (30).
In the P2X7R-/- mice, tumor growth was increased and infiltrated immune cells in TME had an immunosuppressive phenotype, with decreased helper and cytotoxic T cells and more suppressor or regulatory T cells (Treg) populations (31). Moreover, in P2X7R-/- or nlrp3-/- mice, anti-cancer therapy was unsuccessful; it was revealed that P2X7R was associated with an anti-cancer response and the NLRP3 inflammasome activation (32). But, P2X7R blocking (A740003 as an antagonist) promotes the infiltration of CD4+ T cells and decreases the expression of CD73 and CD39, reducing TME immunosuppression (31). It seems these controversial results depend on TME ATP levels. In P2X7R-/- mice, the ATP level decreased mostly due to a deficiency in immune cell ATP release and an increase in ATP degradation caused by infiltrating Tregs. But in the P2X7R blocking condition, due to decreased ectonucleotidase expression and increased ATP release from cancer cells, ATP levels in the TME remain unchanged.
CD39 and CD73 are expressed in immune, tumor, and stromal cells, and they regulate ATP and ADO levels in the TME by their enzymatic properties on the cell membrane, converting eATP to ADO in two steps (33). ADO is one of the most critical chemicals identified as a tumor-promoting factor that inhibits the function of anti-tumor immune cells and increases Treg numbers, with an immune evasion effect (Figure 1) (34). By increasing vascular endothelial growth factor (VEGF) release and, as a result, more angiogenesis, ADO increases cancer cell survival and proliferation (35, 36). As the essential immunosuppressive receptors, ADO receptors (A2AR and A2BR) are found in various tumor tissues and act as a promoter of cell growth (37). As mentioned above, eATP acts as a “find me” signal; this signal is reversed in the TME, owing to the conversion of eATP into ADO by the CD39 and CD73 (7). As a result, purinergic signaling determines the tumor and immune cell interaction outcome. Increased CD73 and CD39 in immune cells lead to decreased eATP and increased ADO production, resulting in an immunosuppressive environment.
ATP, through purinergic receptors, causes cancer cells to migrate and play a role in the epithelial-mesenchymal transition (EMT) in several cancer types (38). Highly metastatic breast cancer cell lines have been shown to release more ATP into the extracellular medium and lead to P2Y2R activation. As a result, they have more potential to migrate and invade through the mitogen-activated protein kinase (MEK)/extracellular signal-regulated kinases (ERK1/2) or β-catenin pathway (39–41). P2X7R activation causes cell migration, up-regulation of EMT-related genes, and down-regulation of epithelial cadherins (E-cadherins) in prostate, breast, and osteosarcoma cell lines, all of which are mediated through phosphoinositide 3-kinases (PI3K)/Akt phosphorylation and ERK1/2 pathways (Figure 1) (42, 43). Transforming growth factor-β1 (TGF-β1), a well-known EMT inducer, also has been shown to cause lung cancer cells to produce ATP and then activate P2 receptors, which mediated actin remodeling and cell migration (44). In a metastatic breast cancer cell line incubated with endothelial cells, P2Y2R activation enhanced intracellular cell adhesion molecule-1 (ICAM-1) and vascular cell adhesion molecule-1 (VCAM-1) expression, resulting in increased adhesion between cancer cells and endothelial cells as well as cancer cell metastasis (45). P2Y2R expression in breast tumor tissue is higher at the tumor’s invasive edge, and its activation by ATP increases matrix metalloproteinase (MMP) production in prostate cancer cells (Figure 1) (46, 47). In a mouse model, P2X7R blockade significantly reduces neuroblastoma bone marrow metastasis (48). Incubation of gastric or breast cancer cells with ADO increases EMT gene expression, associated with A2AR/A2BR activation and the Akt-mammalian target of rapamycin (mTOR) or adenylyl cyclase (AC)/protein kinase A (PKA)/cyclic adenosine monophosphate (cAMP) pathway (Figure 1) (49). Furthermore, overexpression of CD73 has been demonstrated to stimulate tumor cell migration, invasion, and adhesion (50).
Conventionally tumors were treated with the highest tolerated drug dosage, but it has recently been shown that this therapeutic approach puts persistent pressure on tumors, causing the selection and inducing phenotypes of highly acquired drug-resistant cancer cells. Therapeutic resistance is conferred by anything that limits drug availability near its target or inhibits the cell’s capability to respond to it. Anti-cancer drug resistance is caused by various mechanisms, including genetic mutations, epigenetic alterations, increased repair of DNA damage, alteration of drug target, cancer heterogeneity, senescence escape, EMT, and drug efflux (51).
Metabolic reprogramming is one of the cancer signatures that can be seen as a cause of malignant transformation or a result of that. When a cell transforms to neoplasia and then cancer, its metabolic processes undergo a series of changes due to increased energy requirements with limited availability of nutrients or oxygen. A unique harsh environment is established during cancer development, including low pH, hypoxia, oxidative stress, and nutritional pressure (52). Cancer cell progression and growth require the activation of the cell cycle and metabolic pathways to produce nucleotides, fatty acids (FAs), amino acids, and cell membrane components (53). The modified metabolic environment of the TME can cause immune cells to undergo metabolic reprogramming, impeding immune surveillance and promoting cancer growth (54).
Despite promising advances in immunotherapy (such as immune checkpoint inhibitors, ICPI), the metabolically immunosuppressive TME is still a significant hurdle to tumor immunotherapy effectiveness (55). Tumor cells constantly adapt their nutrient uptake and metabolism to maintain proliferation, putting metabolic stress on infiltrating immune cells, and influencing antigen presentation, leading to immunosuppression and immune escape (56–59). Numerous researches have provided information on the involvement of mitochondria in treatment resistance. On the other hand, ICPI’s drug resistance is thought to be related to altered metabolic reprogramming of the immunosuppressive TME (58, 60).
Also, metabolic reprogramming is crucial for cancer metastasis and therapeutic resistance. Immunological cell infiltration and anti-tumor immune responses can be inhibited by immunosuppressive metabolites produced in the TME. As a result of the association between immune response and cancer metabolism, combination therapies that target ICPs and metabolic pathways could improve anti-cancer therapy effectiveness and overcome immunotherapy resistance. So, comprehensive knowledge of the metabolic variations between normal and cancer cells, as well as their effect on the anti-cancer response, will not only facilitate extending therapeutic alternatives but also combat therapy resistance. Effective therapies that target dysregulated metabolic checkpoints have the potential to remodel the immunological state of the TME, modulate the activation of T cells, improve the immunogenicity of cancer cells, and increase the efficacy of ICPI synergistically.
The purinergic receptors such as P2X7R are essential in metabolic disorders and cancer metabolic reprogramming (61). On the other hand, uncontrolled growth of cancer cells requires highly cellular energy which can be provided by purinergic receptors (62). Non-small cell lung cancer (NSCLC) cell line was found to have the ability to internalize highly concentrated eATP, which promoted intracellular energy supplement and enhanced growth, survival, and EMT (14). P2X1R and P2X7R inhibition reduce mitochondrial activity, calcium level, and cell proliferation in THP-1, Jurkat, HL-60, and U-937 cells. Furthermore, K+ is released through P2XR into the TME by necrotizing tumors, causing effector T cell suppression via autophagy and caloric limitation, which drive epigenetic and metabolic reprogramming (8, 63, 64).
Both cancer and immune cells have similar metabolic characteristics, relying on glycolysis to achieve the energy needed for fast proliferation. So, the TME has an exceptionally high demand for nutrition (glucose, amino acid, FA), and competition between immune and cancer cells can decrease the anti-cancer response (65). The researchers found that intracellular ATP levels were important in multiple drug resistance (MDR); compared to their parental cells, chemo-resistant cancer cell lines had higher intracellular ATP levels. They also demonstrated that delivering liposome-encapsulated ATP to drug-sensitive cells artificially leads to drug resistance while using a glycolysis inhibitor to deplete intracellular ATP sensitized resistant cancer cells (66).
ATP-binding-cassette (ABC) transporters are transmembrane proteins that modulate the biodistribution of endogenous and exogenous products by translocating diverse substrates from intracellular to extracellular media. These transporters are involved in steroid production, immune responses, and reproductive barrier functions (67). ABC transporter subunits include P-gp (P-glycoprotein, or ABCB1), MRP1 (multidrug resistance protein 1, or ABCC1), and BCRP (breast cancer resistance protein, or ABCG2). They can efflux many drugs from the cell, reducing their interaction with intracellular targets and therapeutic efficacy. Overexpression of ABC transporters is one of the key chemo-resistance mechanisms in various malignancies (23, 68). Internalized ATP molecules by micropinocytosis improve ABC transporter expression and then efflux tyrosine kinase inhibitors (TKIs) and chemotherapy drugs, leading to less drug accumulation and enhanced cell survival. Reduced intracellular drug concentrations and increased ATP levels enhance ATP binding and decrease TKI binding on receptor tyrosine kinases (RTKs), resulting in enhanced RTK-dependent signaling and drug resistance. They postulated that ATP serves as an energy molecule that promotes drug efflux as well as a signal-transduction molecule that activates cell survival signaling pathways (13).
eATP may contribute to cancer treatment resistance through purinergic receptor signaling (69). By P2Y-mediated overexpression of MRP2 and drug pumping, researchers revealed that ATP increased chemo-resistance in colorectal cancer cells (70). Non-chemo-resistant patients had higher P2X7R levels and lower A2A levels in CD8+ T cells than chemo-resistant patients (71). Another study found that when ATP stimulated the P2X7R, it had an anti-apoptotic effect in melanoma cells treated with methoxyestradiol (72). eATP increases tumor cell survival and drug resistance by increased glucose transporter 1 (GLUT1) expression through the P2X7R-PI3K-Akt and hypoxia-inducible factor 1α (HIF-1α) (17).
Defects in the autophagy in tumor cells or purinergic receptors in immune cells result in a poor response to the drug (16, 73). Immune cells, dying or stressed cells of TME, can release eATP, potentially resulting in an ATP-rich, tumor-friendly environment (74). eATP plays a role in TME immunomodulation and may impact therapeutic outcomes. NSCLC cell line, through high ATP internalization, promotes intracellular energy supplement and enhances therapy resistance (14). Signaling mediated by ADO derived from eATP would result in the formation of an immunosuppressive environment and therapy resistance (34).
But in a contradictory way, a recent study found that ATP-decorated and doxorubicin-loaded silica had a considerable anti-tumor effect against doxorubicin-resistant murine lymphoma. The nanocomposite increased apoptosis by activating the P2X7R (75). The noteworthy point in this context is that long-term activation of P2X7R by high levels of eATP can result in the formation of a macropore, which causes cell death by the plasma membrane depolarization. Compared to levels found in normal tissues, the TME has higher eATP (76), but these concentrations are probably not obtained due to ectonucleotidases. It seems there will be a concentration window where eATP concentrations are low enough to avoid significant drug resistance but high enough to promote anti-cancer immune responses (51). Hence P2X7R can either promote cell survival or cause cell death depending on its activation state.
In normal conditions, pyruvate enters the tricarboxylic acid cycle (TCA) and undergoes oxidative phosphorylation (OXPHOS) when the oxygen level is sufficient. In the lack of oxygen, glycolysis converts pyruvate to lactate (77). First, Warburg was shown that independent of the access to oxygen or the efficiency of mitochondrial OXPHOS; cancerous cells prefer to receive ATP from glycolysis, which is referred to as the “Warburg effect” or “aerobic glycolysis” (Figure 2) (78). Besides substantial energy, glycolysis provides pivotal intermediates such as nucleotides, amino acids, and lipids required for cancer cell biosynthesis (79).
Figure 2 How purinergic receptor activity induces therapy resistance through metabolic reprogramming in the tumor cell. Due to the activity of the P2X7R and the increase of intracellular Ca2+, Akt and then HIF-1a is activated. HIF-1a stimulates GLUT to allow glucose to enter the cell and trigger the glycolysis pathway. HK, one of the enzymes in the glycolysis pathway, can inhibit tumor cell apoptosis by binding to VDAC on mitochondria and inhibiting it. Pyruvate from the glycolysis pathway is mainly converted to lactate through the Warburg effect. After leaving the cell, lactate can inhibit the anti-tumor immune response by acting on PD-L1. On the other hand, some pyruvate with the effect of PDH, ACC, and FAS eventually convert to fatty acids (palmitic acid). P2X7R activity also stimulates FAS and ACC. Then the fatty acid produced in the cytoplasm enters the mitochondria and the FAO pathway and produces long-chain saturated fatty acids. By reducing membrane fluidity, endocytosis, passive diffusion of drugs, and apoptosis, long-chain saturated fatty acids cause tumor cell therapy resistance. Also, ATP produced through glycolysis and OXPHOS help the drug export via ABC transporter. ABC, ATP-binding cassette; ACC, acetyl-CoA carboxylase; ATP, adenosine triphosphate; DC, dendritic cell; FAO, fatty acid oxidation; FAS, fatty acid synthase; GLUT, glucose transporter; HIF-1a, hypoxia-inducible factor 1-alpha; HK, hexokinase; NK cell, natural killer cell; OXPHOS, oxidative phosphorylation; PDH, pyruvate dehydrogenase; PD-L1, programmed death-ligand 1; TAM, tumor-associated macrophage; Th2, T helper type 2; Treg, regulatory T cell; VDAC, voltage-dependent anion channel.
Glycolytic-promoting factors including c-Myc, HIF-1, GLUT1, hexokinase (HK), pyruvate kinase (PK), phosphofructokinase (PFK), phosphoglycerate kinase 1 (PGK1), lactate dehydrogenase (LDH), and pyruvate dehydrogenase kinase isozyme 1 (PDK-1) that activated in tumor cells are described as tumor markers (80, 81). The higher glycolytic flux increases glucose absorption, glycogen synthesis, and lactate production. Lactic acid accumulation in the TME affects immune cell functions, impairs cell metabolism, proliferation, and activation, and decreases mobility and cytotoxicity (82–84). Furthermore, high lactate levels enhance Treg survival as well as a tolerogenic phenotype in DCs and macrophages, resulting in a more suppressor phenotype in TME while tumor cells are not targeted (Figure 2) (85, 86).
The pentose phosphate pathway (PPP) is another metabolic flux stimulated in malignant cells. The PPP increases the formation of 5-carbon sugars by degrading glucose. 5-carbon sugars are needed for nucleic acid synthesis, as well as the production of the oxidoreductases cofactor nicotinamide adenine dinucleotide phosphate (NADPH). NADPH is required for lipogenesis and keeping the antioxidant glutathione in reduced form (GSH) (87). Since cancer cells prefer aerobic glycolysis, which produces a lot of lactate and H+, the acidic TME facilitates rapid carbon incorporation into nucleotides, lipids, and amino acids and finally promotes cell proliferation (88, 89). As a result, blocking glycolysis as novel anti-tumor therapy can significantly decrease tumor cell proliferation and even play a role in tumor cell death (90).
In the TME, P2X7R stimulation by eATP has induced GLUT1 expression and enhanced aerobic glycolysis and OXPHOS through the PI3K-Akt pathway and HIF-1 signaling, which finally leads to increased ATP production (17, 91). Also, the intracellular HIF-1 was activated by stimulating the A2BR and Warburg effect (Figure 1) (92). Due to increased glucose transport and aerobic glycolysis in tumor cells, they have a higher intracellular ATP level than normal cells in the same tissue (14, 93). The P2X7R activation has downstream consequences similar to the Warburg effect, e.g., overexpression of PDK-1 (94). Amoroso et al. found in P2X7R-transfected neuroblastoma and HEK293 cell line an enhanced lactate production linked with cell proliferation, a characteristic of the Warburg effect (95).
In leukemia cells, increased ATP release was associated with increased mitochondrial quantity and activity, resulting in cancer cell proliferation (96). P2X7R can stimulate mitochondria by elevating the resting mitochondrial potential (ΔΨm) and mitochondrial calcium level, supporting the Warburg effect and proliferation (97). Furthermore, P2X7R enhanced O2 consumption and NADPH oxidase 2 and decreased respiratory rate, while its overstimulation leads to a decline in ΔΨm, as well as mitochondrial fragmentation and cell death (91, 95, 98, 99). P2X7R also reduced pyruvate dehydrogenase (PDH) activity, enhanced ERK phosphorylation, the PI3K/Akt/glycogen synthase kinase 3 (GSK3)/β-catenin, and mTOR/HIF1/VEGF signaling pathway (Figure 1) and increased the glycogen storage due to a decrease in GSK3 (100, 101). These metabolic changes are made to prevent aerobic responses (94, 95).
Cancer stem cells (CSCs) are the subpopulation of cancer cells with the ability to self-renewal and differentiation into any cell type. They have a role in recurrence, metastasis, heterogeneity, and drug resistance. With mitochondrial energy metabolism, CSCs absorb low-level FAs to produce ATP, NADPH, TCA intermediates, and nucleotide bases, which drive cancer cell survival and proliferation (102). The activation of the Akt pathway in CSCs causes the production of glycolysis enzymes such as PDK-1 and HK-1 (103). CSCs had lower glucose consumption, reactive oxygen species (ROS), intracellular ATP levels, and a preference for OXPHOS for energy supply (104). Many studies have shown that the P2X7R promotes CSC maintenance and asymmetric cell division (23, 105).
Because cancer cells have a high rate of glucose uptake and glycolysis, the competition for glucose suppresses calcium signaling, mTOR activity, glycolysis, and interferon (IFN)-production in T cells, leading to T cell exhaustion, reducing anti-cancer immunity, leading to immune evasion (106). An immunomodulatory receptor, PD-L1 overexpression suppresses the cytotoxic function of T-cells and enhances tumor cell resistance to lysis. According to Chang et al., increased PD-L1 on cancer cells increases mTOR function and glycolysis (Figure 2), leading to cancer-driven glucose limitation, changes CD8+ T-cell metabolism, and reduces T cell’s ability to produce IFN-γ (107). Antibodies that inhibit PD-1/PD-L1 or CTLA-4 impair tumor cell glycolysis and increase TME glucose levels and T cells glycolysis (106). By restricting the Ca2+-nuclear factor of activated T cells 1 (NFAT1) signaling pathway in CD4+ T cells, glucose deprivation additionally reduces anti-cancer activities of intratumoral T helper 1 (Th1) CD4+ T cells (108). A chemo-resistant stem-like side population in malignancies has increased glycolytic activity. This modulation is dependent on Akt pathway stimulation because of increased intracellular ATP concentration suppressing AMP-activated protein kinase (AMPK) (103). Overall, the findings suggest that tumor-derived glycolysis metabolites like lactate suppresses immune cell activity and leads to immunological evasion or therapy resistance (60, 109, 110). One of the mechanisms by which lactate reduces the effectiveness of immunotherapy is to increase the expression of PD-L1 (Figure 2) (111). Therefore, targeting LDH, which converts pyruvate to lactate, is one of the potential targets for overcoming treatment resistance (112).
P2Y1 receptors on vascular endothelial cells are activated by eATP and ADP, which transactivates VEGFR2 to induce angiogenesis (113, 114). In a recent study, Palinski et al. showed that extracellular vesicles from sarcoma patients enhance neo-angiogenesis via a P2X4R-dependent pathway. They also found that intracellular Ca2+ mobilization, mitochondrial activation, increased eATP, and lysosomal P2X4R trafficking to the cell membrane, all of which are essential for cell migration and vessel formation (115). In another study, Lapel et al. showed that glycolysis and OXPHOS are required for vasa vasorum endothelial cell (VVEC) angiogenic responses. The increased glycolytic activity was accompanied by increased PFKB3, HK, and GLUT 1 in VVEC after P2R agonists and ATP treatment. P2R agonists reduced PDH phosphorylation while increasing the expression of succinate dehydrogenase (SDH), cytochrome oxidase IV (COX IV), and F1F0-ATP synthase subunit. Furthermore, P2R stimulation caused an increase in mitochondrial Ca2+, showing that mitochondria are involved in VVEC angiogenic activation (116).
FAs uptake by tumor cells is very high, and their metabolism is crucial to the synthesis and maintenance of the cellular membrane, cell division, energy supply, and signaling pathway intermediates (117). In other words, tumor cell survival and metastasis are dependent on FAs absorption, consumption, and catabolism via the FA oxidation (FAO) pathway (118). Lipid accumulation leads to cancer cell survival and contributes to chemotherapy resistance, which is also significantly linked to CSCs in the tumor cell population (119). Citrate comes from glycolysis or glutaminolysis, exits mitochondria, and enters the cytoplasm, where ATP-citrate lyase converts it to the lipogenic substrate acetyl-CoA. Acetyl-CoA serves as a source of FAs, converted into phospholipids and triacylglycerols (120). FA synthase (FAS) is a central regulator of lipid metabolism that creates palmitate from the condensation of acetyl-CoA and malonyl-CoA (Figure 2). Overexpression of FAS has been associated with cancer growth, poor prognosis, and invasion in various cancers (121, 122), so it could be a potential candidate for anti-cancer drugs (123). One of the major components of membrane lipids is cholesterol, which plays a role in T-cell receptor (TCR) clustering and immunological synapses development (124). Because of an increase in the cell membrane cholesterol content of CD8+ T cells, suppressing cholesterol esterification resulted in potentiated effector activity and improved proliferation of CD8+ but not CD4+ T cells (125).
A group of studies links P2X7R activity to increased FA catabolic pathway and tumor cell plasticity (126). Furthermore, the loss of the P2X7R favored FAO and decreased ΔΨm, FA metabolism enzymes like acetyl-CoA carboxylase (ACC) and FAS. Also, loss of the P2X7R leads to higher serum cholesterol, triglyceride, lipid accumulation, adipocyte hyperplasia, obesity, and insulin resistance, all of which reduce proliferation, survival, and metastasis of tumor cells (127–129).
Lipid raft enrichment has been seen in prostate and breast cancer cell lines (130). The P2X7R isoforms cannot open the pore due to cholesterol in cell membranes (131). So, cholesterol-rich lipid raft regions are favored sites for P2X7R to preserve its ion channel activities just while avoiding apoptosis. In this way, lowering cholesterol or destroying lipid rafts causes cancer cell death (132).
On the other hand, high FAs can block the killing activity of T cells against tumor cells, allowing the tumor to escape the immune system, and lowering the efficacy of anti-cancer therapies that require a competent immune system (133). Lipid accumulation in tumor cells was associated with CSCs function and therapeutic resistance (119). FAS overexpression has been related to chemotherapy resistance in various tumors (121). Reducing low-density lipoprotein uptake decreases pancreatic adenocarcinoma’s oncogenic characteristics and makes cancer cells more susceptible to treatment (134). As a result, manipulating the lipid metabolism of tumor cells to increase immune function could be novel immunotherapy in the future.
Critical amino acids such as glutamine are necessary for growth and proliferation because they provide both carbon and nitrogen for the biosynthesis of nucleotides (135). Enhanced glutamine metabolism is involved in different metabolic pathways like the Warburg effect in cancer cells. Glutaminase converts glutamine to glutamate, and glutamate is converted to α-ketoglutarate in the mitochondria by the glutamate dehydrogenase enzyme. α-ketoglutarate is a TCA cycle intermediate that serves as a substrate for the synthesis of NADH and oxaloacetate (136).
Because tumor cells require glutamine metabolism for survival, glutamine levels are associated with tumor sensitivity to anti-cancer treatments (137). Tumor cells consume glutamine and make it out of the T cell’s reach, preventing the T cells from proliferation and anti-tumor activity (138). According to a recent study, glutamine metabolism in tumor cells reduces the effectiveness of anti-tumor immune responses (139). Myeloid−derived suppressor cells (MDSCs) are immunosuppressive cells that are activated and increased in response to several growth factors and cytokines released by cancer cells (140). MDSC apoptosis is induced by glutaminase inhibitors, making ICPI-resistant cancers susceptible to immunotherapy (141). JHU083, a novel glutamine antagonist, could overcome cancer immune escape and boost anti-cancer responses (139). These findings demonstrate that amino acid deprivation can cause immunosuppressive TME, decreasing anti-tumor immune responses.
Tryptophan, as another key amino acid, is required for T cell function, but indoleamine 2,3-dioxygenase (IDO), the enzyme that catalyzes tryptophan to kynurenine, is extensively expressed in human tumors. Kynurenine, a ligand for the aryl hydrocarbon receptor (AHR), suppresses T cells’ anti-tumor response (142). IDO expression has been associated with poor prognosis by consuming tryptophan (143).
Immune suppression occurs within the TME when tumor cells, DCs, macrophages, cancer-associated fibroblast (CAF), tumor-associated macrophages (TAMs), and MDSCs express IDO (60, 144, 145). IDO prevents T cell survival, proliferation, and function by tryptophan consumption (146–148). The AHR binds to kynurenine produced by IDO, promoting immunosuppression by increasing Treg development, which inhibits anti-tumor immune response (149, 150). Furthermore, tumor cell arginine metabolism increases tumor development and immune evasion. So, targeting the IDO-kynurenine-tryptophan axis and depleting arginine in combination with anti-PD-1 could be a promising approach for eliminating immunotherapy resistance (65, 151).
Rapid tumor cell proliferation causes low oxygen concentration and hypoxic stress in the TME. At the center of the TME cellular mass, HIF-1α/β is activated in response to low oxygen levels, allowing cells to adapt to hypoxic environments (152). Proliferation, differentiation, angiogenesis, metastasis, and metabolic reprogramming of cancer cells can control by HIF (153–155). HIF is one of the pathways that can induce CD39 and CD73 expression and promotes ADO formation (Figure 1) (156, 157).
Hypoxia appears to be a significant metabolic regulator in TME, leading to immunosuppression or therapy resistance by inhibiting CD4+ T cell effector function and increasing Treg activity (158–160). Hypoxia also causes immunosuppression by increasing the expression of PD-L1 on tumor cells and immuno-modulatory metabolites such as lactate and ADO (161, 162). Nitroglycerin (also known as GTN), a nitric oxide (NO) signaling activator, and TH-302, a hypoxia-activated prodrug, in hypoxic cancer cells inhibits PD-L1 expression, decreases MDSCs and hypoxia-mediated cytotoxic T-cell death, overall, making cancer cells more susceptible to T cell-mediated cytotoxicity (162, 163).
Hypoxia can decrease anti-tumor immunity by affecting functional effector cells in TME. For example, it inhibits the killing capability of NK cells by reducing their activation receptors, CD16 and NKG2D (164). HIF-1 induced the expression of CD47, the “don’t eat me” signal, on tumor cells, leading to decreased phagocytosis and promoting cancer growth and immune evasion (165).
Moreover, HIF-1 reduces the surface expression of the major histocompatibility complex (MHC) class I chain-related (MIC) immune cell activator, allowing the tumor cell to escape immune response (166). Hypoxia-induced C-C motif chemokine ligand 28 (CCL28) promotes tumor immune evasion by attracting C-C motif chemokine receptor 10 (CCR10)-positive Tregs to the tumor site (167). Overall, hypoxia enhances the VEGF expression, which stimulates M2 macrophage polarization and MDSC infiltration and suppresses antigen presentation, DC maturation, T cell anti-tumor function, contributing to tumor progression (168–171). In addition to the hypoxic environment, tumor cells can escape immune surveillance in an acidic TME through cytokine denaturation (172, 173). As mentioned before, eATP stimulates P2X7R and P2X7R enhanced mTOR/HIF1/VEGF signaling pathway. Also, the intracellular HIF-1 was activated by stimulating the A2BR and Warburg effect. Then HIF-1α increases cancer drug resistance by increasing GLUT1 (Figure 2) (17, 92, 100, 101). As a result, a therapy targeting HIF-1 or reducing acidity like blocking proton export could be a fascinating strategy for increasing immunotherapy efficacy.
FAO and OXPHOS are the primary energy sources for naive T lymphocytes and are essential for Treg cells, while glycolysis, glutaminolysis, OXPHOS, and lipid synthesis are enhanced in effector T cells (174–177). The metabolic differences between CD4+ T and CD8+ cells are significant too. Compared to CD4+ T cells, CD8+ T cells are less reliant on oxygen, OXPHOS, and GLUT1 levels in the TME and have more metabolic flexibility, causing CD8+ T cells to proliferate faster (178–180). Tregs in the TME are highly apoptotic because of oxidative stress, and these apoptotic Tregs promote immunosuppression by high conversion of ATP to ADO by CD39 and CD73 (181).
TAMs play an essential role in tumor cell proliferation, angiogenesis, and the development of an immunosuppressive TME. TAM’s metabolic profiles are frequently marked by increased glycolysis, FA synthesis, and glutamine metabolism. These profiles cause TAM to contribute to angiogenesis and metastasis (182–184). TAMs can release arachidonic acid, tumor necrosis factor-α (TNF-α), TGF-β, and IL-6 in melanoma, causing cancer cells to produce VEGF-A, which accelerates angiogenesis (185). Also, IL-6 through PGK1 phosphorylation in cancer cells and lncRNAs released from TAM increases tumor cell glycolysis and thereby boosts malignant progression (186, 187). TAM-derived metabolites play a significant function in chemotherapy resistance in tumors. TAM abundance is directly associated with a lower response to a chemotherapy drug, gemcitabine, in pancreatic ductal adenocarcinoma (PDAC) (188). Immunosuppressive cells like TAMs express P2X7R at high levels, and the lack of P2X7R disrupted the polarization of TAMs (189).
Exogenous lipid uptake by lipid transport receptors shifts metabolic reprogramming of cancer-infiltrating MDSC from glycolysis to FAO (190). Increased exogenous FA intake by MDSCs promotes tumor growth by increasing their immunosuppressive effect on T-cells (151, 191). By increasing NO and ROS production, depletion of L-cysteine and L-arginine, or secretion of inhibitory cytokines, MDSCs suppress T cell activation and proliferation and drive the development of macrophage M2 and Tregs (192). Furthermore, MDSCs could contribute to establishing an immunosuppressive TME, which boosts cancer development and immune escape (140).
DCs are effective stimulators of T cell activation because they absorb, process, and present antigens in the TME. Tumor-associated DCs generate ROS, which causes endoplasmic reticulum (ER) stress and lipid production. The accumulation of lipids in DCs can suppress the anti-tumor response by decreasing antigen presentation capacity (193). The ability of tumor-infiltrating DCs to present tumor antigens modifies due to lipid accumulation and reduced arginine and tryptophan, affecting T cell anti-tumor immunity and resulting in tumor immune escape (194, 195).
Tumors can evade immune surveillance due to ADO-AR signals, which decrease the anti-cancer function of immune cells like CD8+ T cells, DCs, NK cells, and M1 macrophages while boosting the immunosuppressive function of cells like MDSCs and Tregs, increasing the expression of IL-10, TGF-β, arginase-2, and IDO-1, lead to Th2 or M2 differentiation (196). In a mouse model, treatment with the CD39 inhibitor increased CD8+ T cells and NK cell-mediated killing capacity, indicating improved anti-tumor immunity (197). Furthermore, some nucleotides, e.g., ADO, and cancer-derived amino acids, e.g., kynurenine, affect immunotherapy efficacy. To overcome treatment resistance, multiple blockers targeting these compounds can be combined with PD-1 or PD-L1 blockers (151). In tumor models, the combination of anti-CD73 and anti-PD-1 antibodies exhibited synergistic effectiveness against ADO-driven immunosuppression, prompting a phase I trial of anti-CD73 in cancer patients (NCT02503774) (198).
Because ADO in the TME impairs the immune response, the CD39/CD73 and ADO receptors are considered promising anti-cancer therapeutic targets. Chemotherapy and radiotherapy cause tissue damage and cell death, resulting in ATP release, CD73 overexpression, and increased ADO in the TME, so CD73 inhibition combined with radiotherapy and ICPI improved DC infiltration and T cell responses (199, 200).
Single-drug treatment approaches kill sensitive tumor cells while allowing resistant cancer cells to survive and proliferate, so these approaches are most likely to fail due to drug resistance. But, combination therapy, which targets more driver genes simultaneously or with energy blocking, suppresses more clones in a tumor and improves the efficacy of the drug. Novel therapeutic approaches, “on and off” and “high dose followed by low dose”, lead to extended survival and inhibit drug resistance since this intermittent or flexible dosing permits the competition of sensitive and resistant cells and prevents the formation of drug-resistant cells (201). These findings prompted clinical trials of this intermittent dosage strategy (NCT02196181).
Cancer cells could circumvent any strategy, but they couldn’t escape the energy demand for their growth, proliferation, metastasis, or drug resistance. Tumor cells tend to have greater ATP levels for survival and therapy resistance. Limiting the energy supply of cancer cells is one approach to overcoming resistance. Prescreening tumors based on their ability to internalize ATP and the expression of a certain set of ABC transporters will give meaningful insights for selecting appropriate anti-cancer drugs and predicting patient response to therapies, restricting drug resistance, and improving therapeutic effectiveness. Drug efficacy may be improved by lowering eATP concentrations, ATP synthesis-inhibitor, or preventing ATP internalization via diminishing purinergic receptor activation, causing tumor cells to stop growing and cell death (51). When injected into a rat glioma model, an ATPase called apyrase was found to inhibit the growth of glioblastoma (70).
The signaling of purinergic receptors appears to contribute to cancer progression and resistance to treatment by altering the metabolism of cancer cells. Therefore, therapeutic interventions that are considered to inhibit cancer cell metabolism and purinergic receptors simultaneously can potentially reduce resistance to treatment. Given that, molecules such as the P2X7R have emerged as an attractive target for anti-tumor therapy in various tumors. Also, P2X7R is an aerobic glycolysis potent activator (18). The loss of the P2X7R through glucose intolerance, insulin resistance, and decreased FA metabolism reduce therapeutic resistance (127).
Humans express functional P2X7R splice isoforms lacking the C terminal domain, like P2X7R variant B, a variant incapable of forming the macropore and lacking cytotoxic activity but yet possessing ion channel features (25, 202). Interestingly, full-length P2X7R variant A facilitates doxorubicin and daunorubicin cellular absorption and cytotoxicity. In contrast, the P2X7RB has tumor-promoting properties and is correlated with a poor prognosis in a variety of cancers (17, 203–205). The P2X7RB protects cells from daunorubicin toxicity, most likely because of a daunorubicin-dependent rise in ATP concentration in the TME. As a result, treatment with daunorubicin increased P2X7RB expression while decreasing P2X7RA expression in AML patients, leading to P2X7RB overexpression. So, the P2X7RB is a promising therapeutic target for this leukemia (26).
Although the normal cells are more flexible in consuming different energy sources, tumor cells tend to be inflexible. For instance, aggressive tumors with a poor prognosis are glucose-dependent, according to positron emission tomography (PET) imaging (206). This difference between tumor and non-tumor cells can be investigated to tackle cancer growth. These tumor cells are addicted to glucose and are more sensitive to environment glucose concentration than non-tumor cells, so dying faster in glucose-depleted conditions (207). Glycolysis enzymes (GAPDH and LDH) inhibitors such as 3-bromopyruvate, FX11, and oxamate, which reduce intracellular ATP levels, may make tumor cells more susceptible to anti-cancer therapies (208, 209). Using a glucose transport inhibitor, a glycolysis inhibitor, eATP degrader, or inhibition of eATP internalization in combination with other therapies may be especially effective in triggering tumor cell death.
Stemness, tumor metastasis, and therapeutic resistance all require metabolic reprogramming. However, the TME has a complicated composition, and the metabolic landscape inside this microenvironment is poorly known; the TME metabolite’s signature or pattern could be a potential cancer biomarker. On the other hand, developing metabolic inhibitors to maintain T cell activity is critical. As a result, focusing on the cancer cell, metabolic pathways will not only increase our understanding of the cellular interplay in the TME but will also let us overcome immunotherapy resistance by broadening our therapeutic options. Potential metabolic blockers that can be employed in combination with ICPIs will aid in the discovery of new anti-cancer immunotherapy.
Fighting therapeutic resistance appears to be an endless battle because tumor cells can always explore novel strategies to combat present treatment. Identifying novel targets for developing anti-cancer therapies are becoming increasingly important to combat chemotherapeutic resistance. Also, the induction of a specific type of cell death called ICD can help to suppress resistance to treatment in tumor cells (perhaps by altering tumor cell metabolism). Last but not least, the well-explained function of ATP in ICD gives room for a different treatment approach that aims to trigger a controlled release of ATP in the TME to increase DC responses. A combination therapy involving ICD inducers and immunotherapy (e.g., anti-PD-L1), which induces a long-lasting immune response, paints a promising prospect in reducing therapy resistance.
Recent research attempts to determine how the P2X7R affects cancer cell metabolic reprogramming, e.g., intracellular ATP synthesis, allowing cell division and cytoskeletal alterations required for tumor development and metastasis. To improve current anti-tumor therapeutic strategies, it is crucial to comprehend the significance of the complex metabolic changes related to cancer and a deep understanding of P2X7R expression, regulation, and involvement in metabolic disorders, cancer metabolism, and metabolic reprogramming.
In this review, we investigated the role of purinergic receptors in the development of resistance to therapy through changes in tumor cell metabolism. For cancer progression and therapeutic resistance, metabolic reprogramming is necessary. Increased energy demands of tumor cells, or a lack of nutrients or oxygen, might cause metabolic changes that affect cell fate. Cancer cells are exposed to a combination of receptors and extracellular molecules, and their combination determines the cell fate. To develop and win, cancer hijacks the whole body’s energy and function. Cancer cells increase nutrient uptake, deplete oxygen, elevate TME acidity, and increase the pro-tumor metabolic pathway to generate an immunosuppressive TME that supports cancer proliferation and immune escape.
The purinergic receptors are crucial in both normal and malignant cells. They contribute to therapy resistance by modifying cancer cells’ glucose, lipid, and amino acid metabolism. Extensive in vitro and in vivo preclinical findings showed that extracellular nucleotides and their receptors affect tumor progression. In recent years, mechanisms for ATP release into the extracellular milieu and plasma membrane receptors responsible for these various effects have been defined. An important issue that exists is the dual roles of ATP and signaling caused by purinergic receptors, which can lead to an increase or decrease in resistance to treatment. Although factors such as ATP concentration, type of binding receptor, or tumor stage can affect the final result, there is a strong need for more extensive studies on why these dual behaviors of ATP and purinergic receptors exist. Therefore, to improve current anti-tumor medicines, a thorough understanding of purinergic receptor expression, regulation, and role in cancer metabolic reprogramming is crucial.
All authors contributed to the study conception and design. Review and editing were performed by MR, AD,GN, BM, MB, and AT. Visualization was performed by SN, and the project was supervised by MV and YM. The first draft of the manuscript was written by HA, and all authors commented on previous versions of the manuscript. All authors read and approved the final manuscript.
The authors declare that the research was conducted in the absence of any commercial or financial relationships that could be construed as a potential conflict of interest.
All claims expressed in this article are solely those of the authors and do not necessarily represent those of their affiliated organizations, or those of the publisher, the editors and the reviewers. Any product that may be evaluated in this article, or claim that may be made by its manufacturer, is not guaranteed or endorsed by the publisher.
1. Sung H, Ferlay J, Siegel RL, Laversanne M, Soerjomataram I, Jemal A, et al. Global cancer statistics 2020: GLOBOCAN estimates of incidence and mortality worldwide for 36 cancers in 185 countries. CA Cancer J Clin (2021) 71:209–49. doi: 10.3322/caac.21660
2. Khalil DN, Smith EL, Brentjens RJ, Wolchok JD. The future of cancer treatment: immunomodulation, CARs and combination immunotherapy. Nat Rev Clin Oncol (2016) 13:273–90. doi: 10.1038/nrclinonc.2016.25
3. Damin DC, Lazzaron AR. Evolving treatment strategies for colorectal cancer: a critical review of current therapeutic options. World J Gastroenterol WJG (2014) 20:877. doi: 10.3748/wjg.v20.i4.877
4. Pardoll DM. The blockade of immune checkpoints in cancer immunotherapy. Nat Rev Cancer (2012) 12:252–64. doi: 10.1038/nrc3239
5. Rascio F, Spadaccino F, Rocchetti MT, Castellano G, Stallone G, Netti GS, et al. The pathogenic role of PI3K/AKT pathway in cancer onset and drug resistance: An updated review. Cancers (Basel) (2021) 13:3949. doi: 10.3390/cancers13163949
6. Rueff J, Rodrigues AS. Cancer drug resistance: A brief overview from a genetic viewpoint. Cancer Drug Resist (2016) 1395:1–18. doi: 10.1007/978-1-4939-3347-1_1
7. de Andrade Mello P, Coutinho-Silva R, Savio LEB. Multifaceted effects of extracellular adenosine triphosphate and adenosine in the tumor–host interaction and therapeutic perspectives. Front Immunol (2017) 8:1526. doi: 10.3389/fimmu.2017.01526
8. Coddou C, Yan Z, Obsil T, Huidobro-Toro JP, Stojilkovic SS. Activation and regulation of purinergic P2X receptor channels. Pharmacol Rev (2011) 63:641–83. doi: 10.1124/pr.110.003129
9. Yegutkin GG. Enzymes involved in metabolism of extracellular nucleotides and nucleosides: functional implications and measurement of activities. Crit Rev Biochem Mol Biol (2014) 49:473–97. doi: 10.3109/10409238.2014.953627
10. Furlow PW, Zhang S, Soong TD, Halberg N, Goodarzi H, Mangrum C, et al. Mechanosensitive pannexin-1 channels mediate microvascular metastatic cell survival. Nat Cell Biol (2015) 17:943–52. doi: 10.1038/ncb3194
11. Pellegatti P, Raffaghello L, Bianchi G, Piccardi F, Pistoia V, Di Virgilio F. Increased level of extracellular ATP at tumor sites: in vivo imaging with plasma membrane luciferase. PloS One (2008) 3:e2599. doi: 10.1371/journal.pone.0002599
12. Falzoni S, Donvito G, Di Virgilio F. Detecting adenosine triphosphate in the pericellular space. Interface Focus (2013) 3:20120101. doi: 10.1098/rsfs.2012.0101
13. Wang X, Li Y, Qian Y, Cao Y, Shriwas P, Zhang H, et al. Extracellular ATP, as an energy and phosphorylating molecule, induces different types of drug resistances in cancer cells through ATP internalization and intracellular ATP level increase. Oncotarget (2017) 8:87860. doi: 10.18632/oncotarget.21231
14. Qian Y, Wang X, Liu Y, Li Y, Colvin RA, Tong L, et al. Extracellular ATP is internalized by macropinocytosis and induces intracellular ATP increase and drug resistance in cancer cells. Cancer Lett (2014) 351:242–51. doi: 10.1016/j.canlet.2014.06.008
15. Locy H, De Mey S, De Mey W, De Ridder M, Thielemans K, Maenhout SK. Immunomodulation of the tumor microenvironment: turn foe into friend. Front Immunol (2018) 9:2909. doi: 10.3389/fimmu.2018.02909
16. Ghiringhelli F, Apetoh L, Tesniere A, Aymeric L, Ma Y, Ortiz C, et al. Activation of the NLRP3 inflammasome in dendritic cells induces IL-1β–dependent adaptive immunity against tumors. Nat Med (2009) 15:1170–8. doi: 10.1038/nm.2028
17. Di Virgilio F, Sarti AC, Falzoni S, De Marchi E, Adinolfi E. Extracellular ATP and P2 purinergic signalling in the tumour microenvironment. Nat Rev Cancer (2018) 18:601–18. doi: 10.1038/s41568-018-0037-0
18. Di Virgilio F, Ferrari D, Adinolfi E. P2X 7: a growth-promoting receptor–implications for cancer. Purinergic Signal (2009) 5:251–6. doi: 10.1007/s11302-009-9145-3
19. Gombault A, Baron L, Couillin I. ATP release and purinergic signaling in NLRP3 inflammasome activation. Front Immunol (2013) 3:414. doi: 10.3389/fimmu.2012.00414
20. Sun C, Heid ME, Keyel PA, Salter RD. The second transmembrane domain of P2X7 contributes to dilated pore formation. PloS One (2013) 8:e61886. doi: 10.1371/journal.pone.0061886
21. Schwarz N, Drouot L, Nicke A, Fliegert R, Boyer O, Guse AH, et al. Alternative splicing of the n-terminal cytosolic and transmembrane domains of P2X7 controls gating of the ion channel by ADP-ribosylation. PLoS One(2012) 7(7):e41269. doi: 10.1371/journal.pone.0041269
22. Di Virgilio F, Schmalzing G, Markwardt F. The elusive P2X7 macropore. Trends Cell Biol (2018) 28:392–404. doi: 10.1016/j.tcb.2018.01.005
23. Arnaud-Sampaio VF, Rabelo ILA, Ulrich H, Lameu C. The P2X7 receptor in the maintenance of cancer stem cells, chemoresistance and metastasis. Stem Cell Rev Rep (2020) 16:288–300. doi: 10.1007/s12015-019-09936-w
24. Pegoraro A, De Marchi E, Adinolfi E. P2X7 variants in oncogenesis. Cells (2021) 10:189. doi: 10.3390/cells10010189
25. Adinolfi E, Cirillo M, Woltersdorf R, Falzoni S, Chiozzi P, Pellegatti P, et al. Trophic activity of a naturally occurring truncated isoform of the P2X7 receptor. FASEB J (2010) 24:3393–404. doi: 10.1096/fj.09-153601
26. Pegoraro A, Orioli E, De Marchi E, Salvestrini V, Milani A, Di Virgilio F, et al. Differential sensitivity of acute myeloid leukemia cells to daunorubicin depends on P2X7A versus P2X7B receptor expression. Cell Death Dis (2020) 11:1–12. doi: 10.1038/s41419-020-03058-9
27. Giuliani AL, Colognesi D, Ricco T, Roncato C, Capece M, Amoroso F, et al. Trophic activity of human P2X7 receptor isoforms a and b in osteosarcoma. PloS One (2014) 9:e107224. doi: 10.1371/journal.pone.0107224
28. Gilbert SM, Oliphant CJ, Hassan S, Peille AL, Bronsert P, Falzoni S, et al. ATP in the tumour microenvironment drives expression of nfP2X7, a key mediator of cancer cell survival. Oncogene (2019) 38:194–208. doi: 10.1038/s41388-018-0426-6
29. Feng YH, Li X, Zeng R, Gorodeski GI. Endogenously expressed truncated P2X7 receptor lacking the c-terminus is preferentially upregulated in epithelial cancer cells and fails to mediate ligand-induced pore formation and apoptosis. Nucleosides Nucleotides Nucleic Acids (2006) 25:1271–6. doi: 10.1080/15257770600890921
30. Adinolfi E, Raffaghello L, Giuliani AL, Cavazzini L, Capece M, Chiozzi P, et al. Expression of P2X7 receptor increases In vivo tumor GrowthP2X7 and cancer. Cancer Res (2012) 72:2957–69. doi: 10.1158/0008-5472.CAN-11-1947
31. De Marchi E, Orioli E, Pegoraro A, Sangaletti S, Portararo P, Curti A, et al. The P2X7 receptor modulates immune cells infiltration, ectonucleotidases expression and extracellular ATP levels in the tumor microenvironment. Oncogene (2019) 38:3636–50. doi: 10.1038/s41388-019-0684-y
32. Aymeric L, Apetoh L, Ghiringhelli F, Tesniere A, Martins I, Kroemer G, et al. Tumor cell death and ATP release prime dendritic cells and efficient anticancer immunity. Cancer Res (2010) 70:855–8. doi: 10.1158/0008-5472.CAN-09-3566
33. Allard B, Beavis PA, Darcy PK, Stagg J. Immunosuppressive activities of adenosine in cancer. Curr Opin Pharmacol (2016) 29:7–16. doi: 10.1016/j.coph.2016.04.001
34. Ohta A. A metabolic immune checkpoint: adenosine in tumor microenvironment. Front Immunol (2016) 109. doi: 10.3389/fimmu.2016.00109
35. Mittal D, Sinha D, Barkauskas D, Young A, Kalimutho M, Stannard K, et al. Adenosine 2B receptor expression on cancer cells promotes metastasis. Cancer Res (2016) 76:4372–82. doi: 10.1158/0008-5472.CAN-16-0544
36. Gessi S, Bencivenni S, Battistello E, Vincenzi F, Colotta V, Catarzi D, et al. Inhibition of A2A adenosine receptor signaling in cancer cells proliferation by the novel antagonist TP455. Front Pharmacol (2017) 8:888. doi: 10.3389/fphar.2017.00888
37. Campos-Contreras A del R, Díaz-Muñoz M, Vázquez-Cuevas FG. Purinergic signaling in the hallmarks of cancer. Cells (2020) 9:1612. doi: 10.3390/cells9071612
38. Martínez-Ramírez AS, Díaz-Muñoz M, Butanda-Ochoa A, Vázquez-Cuevas FG. Nucleotides and nucleoside signaling in the regulation of the epithelium to mesenchymal transition (EMT). Purinergic Signal (2017) 13:1–12. doi: 10.1007/s11302-016-9550-3
39. Eun SY, Ko YS, Park SW, Chang KC, Kim HJ. P2Y2 nucleotide receptor-mediated extracellular signal-regulated kinases and protein kinase c activation induces the invasion of highly metastatic breast cancer cells. Oncol Rep (2015) 34:195–202. doi: 10.3892/or.2015.3972
40. Li W-H, Qiu Y, Zhang H-Q, Tian X-X, Fang W-G. P2Y2 receptor and EGFR cooperate to promote prostate cancer cell invasion via ERK1/2 pathway. PloS One (2015) 10:e0133165. doi: 10.1371/journal.pone.0133165
41. Zhang J, Liu Y, Yang H, Zhang H, Tian X, Fang W. ATP-P2Y2-β-catenin axis promotes cell invasion in breast cancer cells. Cancer Sci (2017) 108:1318–27. doi: 10.1111/cas.13273
42. Cao Y, Wang X, Li Y, Evers M, Zhang H, Chen X. Extracellular and macropinocytosis internalized ATP work together to induce epithelial–mesenchymal transition and other early metastatic activities in lung cancer. Cancer Cell Int (2019) 19:1–16. doi: 10.1186/s12935-019-0973-0
43. Xia J, Yu X, Tang L, Li G, He T. P2X7 receptor stimulates breast cancer cell invasion and migration via the AKT pathway. Oncol Rep (2015) 34:103–10. doi: 10.3892/or.2015.3979
44. Takai E, Tsukimoto M, Harada H, Sawada K, Moriyama Y, Kojima S. Autocrine regulation of TGF-β1-induced cell migration by exocytosis of ATP and activation of P2 receptors in human lung cancer cells. J Cell Sci (2012) 125:5051–60. doi: 10.1242/jcs.104976
45. Jin H, Eun SY, Lee JS, Park SW, Lee JH, Chang KC, et al. P2Y 2 receptor activation by nucleotides released from highly metastatic breast cancer cells increases tumor growth and invasion via crosstalk with endothelial cells. Breast Cancer Res (2014) 16:1–14. doi: 10.1186/bcr3694
46. Qiu Y, Liu Y, Li W-H, Zhang H-Q, Tian X-X, Fang W-G. P2Y2 receptor promotes the migration and invasion of breast cancer cells via EMT-related genes snail and e-cadherin. Oncol Rep (2018) 39:138–50. doi: 10.3892/or.2017.6081
47. Zhang Y, Gong L, Zhang H, Du Q, You J, Tian X, et al. Extracellular ATP enhances in vitro invasion of prostate cancer cells by activating rho GTPase and upregulating MMPs expression. Cancer Lett (2010) 293:189–97. doi: 10.1016/j.canlet.2010.01.010
48. Ulrich H, Ratajczak MZ, Schneider G, Adinolfi E, Orioli E, Ferrazoli EG, et al. Kinin and purine signaling contributes to neuroblastoma metastasis. Front Pharmacol (2018) 9:500. doi: 10.3389/fphar.2018.00500
49. Shi L, Wu Z, Miao J, Du S, Ai S, Xu E, et al. Adenosine interaction with adenosine receptor A2a promotes gastric cancer metastasis by enhancing PI3K–AKT–mTOR signaling. Mol Biol Cell (2019) 30:2527–34. doi: 10.1091/mbc.E19-03-0136
50. Wang L, Zhou X, Zhou T, Ma D, Chen S, Zhi X, et al. Ecto-5′-nucleotidase promotes invasion, migration and adhesion of human breast cancer cells. J Cancer Res Clin Oncol (2008) 134:365–72. doi: 10.1007/s00432-007-0292-z
51. Wang X, Zhang H, Chen X. Drug resistance and combating drug resistance in cancer. Cancer Drug Resist (Alhambra Calif) (2019) 2:141–60. doi: 10.20517/cdr.2019.10
52. Phan LM, Yeung S-CJ, Lee M-H. Cancer metabolic reprogramming: importance, main features, and potentials for precise targeted anti-cancer therapies. Cancer Biol Med (2014) 11:1. doi: 10.7497/j.issn.2095-3941.2014.01.001
53. Israël M, Schwartz L. The metabolic advantage of tumor cells. Mol Cancer (2011) 10:70. doi: 10.1186/1476-4598-10-70
54. Rinaldi G, Rossi M, Fendt S. Metabolic interactions in cancer: cellular metabolism at the interface between the microenvironment, the cancer cell phenotype and the epigenetic landscape. Wiley Interdiscip Rev Syst Biol Med (2018) 10:e1397. doi: 10.1002/wsbm.1397
55. Hegde PS, Chen DS. Top 10 challenges in cancer immunotherapy. Immunity (2020) 52:17–35. doi: 10.1016/j.immuni.2019.12.011
56. Deberardinis RJ, Chandel NS. Fundamentals of cancer metabolism. Sci Adv (2016) 2:e1600200. doi: 10.1126/sciadv.1600200
57. Sidaway P. Cancer risks persist after Hodgkin lymphoma. Nat Rev Clin Oncol (2016) 13:134. doi: 10.1038/nrclinonc.2016.6
58. Ramapriyan R, Caetano MS, Barsoumian HB, Mafra ACP, Zambalde EP, Menon H, et al. Altered cancer metabolism in mechanisms of immunotherapy resistance. Pharmacol Ther (2019) 195:162–71. doi: 10.1016/j.pharmthera.2018.11.004
59. Jiang X, Wang J, Deng X, Xiong F, Ge J, Xiang B, et al. Role of the tumor microenvironment in PD-L1/PD-1-mediated tumor immune escape. Mol Cancer (2019) 18(1):10. doi: 10.1186/s12943-018-0928-4
60. Li X, Wenes M, Romero P, Huang SC-C, Fendt S-M, Ho P-C. Navigating metabolic pathways to enhance antitumour immunity and immunotherapy. Nat Rev Clin Oncol (2019) 16:425–41. doi: 10.1038/s41571-019-0203-7
61. Tang Z, Ye W, Chen H, Kuang X, Guo J, Xiang M, et al. Role of purines in regulation of metabolic reprogramming. Purinergic Signal (2019) 15:423–38. doi: 10.1007/s11302-019-09676-z
62. Ledderose C, Woehrle T, Ledderose S, Strasser K, Seist R, Bao Y, et al. Cutting off the power: inhibition of leukemia cell growth by pausing basal ATP release and P2X receptor signaling? Purinergic Signal (2016) 12:439–51. doi: 10.1007/s11302-016-9510-y
63. Eil R, Vodnala SK, Clever D, Klebanoff CA, Sukumar M, Pan JH, et al. Ionic immune suppression within the tumour microenvironment limits T cell effector function. Nature (2016) 537:539–43. doi: 10.1038/nature19364
64. Kumar VS, Robert E JKR, Madhusudhanan S NYT, Ngoc-Han H, Ping-Hsien L, MinHwa S, et al. T Cell stemness and dysfunction in tumors are triggered by a common mechanism. Sci (80- ) (2019) 363:eaau0135. doi: 10.1126/science.aau0135
65. Jiang Z, Hsu JL, Li Y, Hortobagyi GN, Hung M-C. Cancer cell metabolism bolsters immunotherapy resistance by promoting an immunosuppressive tumor microenvironment. Front Oncol (2020) 10:1197. doi: 10.3389/fonc.2020.01197
66. Zhou Y, Tozzi F, Chen J, Fan F, Xia L, Wang J, et al. Intracellular ATP levels are a pivotal determinant of chemoresistance in colon cancer cells. Cancer Res (2012) 72:304–14. doi: 10.1158/0008-5472.CAN-11-1674
67. Bloise E, Ortiga-Carvalho TM, Reis FM, Lye SJ, Gibb W, Matthews SG. ATP-binding cassette transporters in reproduction: a new frontier. Hum Reprod Update (2016) 22:164–81. doi: 10.1093/humupd/dmv049
68. Begicevic R-R, Falasca M. ABC Transporters in cancer stem cells: beyond chemoresistance. Int J Mol Sci (2017) 18:2362. doi: 10.3390/ijms18112362
69. Vinette V, Placet M, Arguin G, Gendron F-P. Multidrug resistance-associated protein 2 expression is upregulated by adenosine 5’-triphosphate in colorectal cancer cells and enhances their survival to chemotherapeutic drugs. PloS One (2015) 10:e0136080. doi: 10.1371/journal.pone.0136080
70. Morrone FB, Oliveira DL, Gamermann P, Stella J, Wofchuk S, Wink MR, et al. In vivo glioblastoma growth is reduced by apyrase activity in a rat glioma model. BMC Cancer (2006) 6:1–10. doi: 10.1186/1471-2407-6-226
71. Ruiz-Rodríguez VM, Turiján-Espinoza E, Guel-Pañola JA, García-Hernández MH, Zermeño-Nava J de J, López-López N, et al. Chemoresistance in breast cancer patients associated with changes in P2X7 and A2A purinergic receptors in CD8+ T lymphocytes. Front Pharmacol (2020) 11:576955. doi: 10.3389/fphar.2020.576955
72. Deli T, Varga N, Ádám A, Kenessey I, Rásó E, Puskás LG, et al. Functional genomics of calcium channels in human melanoma cells. Int J Cancer (2007) 121:55–65. doi: 10.1002/ijc.22621
73. Galluzzi L, Buqué A, Kepp O, Zitvogel L, Kroemer G. Immunogenic cell death in cancer and infectious disease. Nat Rev Immunol (2017) 17:97. doi: 10.1038/nri.2016.107
74. Piccini A, Carta S, Tassi S, Lasiglié D, Fossati G, Rubartelli A. ATP is released by monocytes stimulated with pathogen-sensing receptor ligands and induces IL-1β and IL-18 secretion in an autocrine way. Proc Natl Acad Sci (2008) 105:8067–72. doi: 10.1073/pnas.0709684105
75. Gilbert SM, Gidley Baird A, Glazer S, Barden JA, Glazer A, Teh LC, et al. A phase I clinical trial demonstrates that nfP2X7-targeted antibodies provide a novel, safe and tolerable topical therapy for basal cell carcinoma. Br J Dermatol (2017) 177:117–24. doi: 10.1111/bjd.15364
76. Lara R, Adinolfi E, Harwood CA, Philpott M, Barden JA, Di Virgilio F, et al. P2X7 in cancer: from molecular mechanisms to therapeutics. Front Pharmacol (2020) 11:793. doi: 10.3389/fphar.2020.00793
77. Greiner EF, Guppy M, Brand K. Glucose is essential for proliferation and the glycolytic enzyme induction that provokes a transition to glycolytic energy production. J Biol Chem (1994) 269:31484–90. doi: 10.1016/S0021-9258(18)31720-4
78. Warburg O. The metabolism of carcinoma cells. J Cancer Res (1925) 9:148–63. doi: 10.1158/jcr.1925.148
79. Lunt SY, Vander Heiden MG. Aerobic glycolysis: meeting the metabolic requirements of cell proliferation. Annu Rev Cell Dev Biol (2011) 27:441–64. doi: 10.1146/annurev-cellbio-092910-154237
80. Sawayama H, Ishimoto T, Sugihara H, Miyanari N, Miyamoto Y, Baba Y, et al. Clinical impact of the warburg effect in gastrointestinal cancer. Int J Oncol (2014) 45:1345–54. doi: 10.3892/ijo.2014.2563
81. Ancey P, Contat C, Meylan E. Glucose transporters in cancer–from tumor cells to the tumor microenvironment. FEBS J (2018) 285:2926–43. doi: 10.1111/febs.14577
82. Fischer K, Hoffmann P, Voelkl S, Meidenbauer N, Ammer J, Edinger M, et al. Inhibitory effect of tumor cell–derived lactic acid on human T cells. Blood (2007) 109:3812–9. doi: 10.1182/blood-2006-07-035972
83. Dietl K, Renner K, Dettmer K, Timischl B, Eberhart K, Dorn C, et al. Lactic acid and acidification inhibit TNF secretion and glycolysis of human monocytes. J Immunol (2010) 184:1200–9. doi: 10.4049/jimmunol.0902584
84. Haas R, Smith J, Rocher-Ros V, Nadkarni S, Montero-Melendez T, D’Acquisto F, et al. Lactate regulates metabolic and pro-inflammatory circuits in control of T cell migration and effector functions. PloS Biol (2015) 13(7):e1002202. doi: 10.1371/journal.pbio.1002202
85. Ippolito L, Morandi A, Giannoni E, Chiarugi P. Lactate: a metabolic driver in the tumour landscape. Trends Biochem Sci (2019) 44:153–66. doi: 10.1016/j.tibs.2018.10.011
86. Liu N, Luo J, Kuang D, Xu S, Duan Y, Xia Y, et al. Lactate inhibits ATP6V0d2 expression in tumor-associated macrophages to promote HIF-2α–mediated tumor progression. J Clin Invest (2019) 129:631–46. doi: 10.1172/JCI123027
87. Méndez I, Díaz-Muñoz M. Circadian and metabolic perspectives in the role played by NADPH in cancer. Front Endocrinol (Lausanne) (2018) 9:93. doi: 10.3389/fendo.2018.00093
88. Panaghie G, Abbott GW. The role of S4 charges in voltage-dependent and voltage-independent KCNQ1 potassium channel complexes. J Gen Physiol (2007) 129:121–33. doi: 10.1085/jgp.200609612
89. Koppenol WH, Bounds PL. The warburg effect and metabolic efficiency: re-crunching the numbers. Sci (80- ) (2009) 324:1029–33. doi: 10.1126/science.1160809
90. Pelicano H, Martin DS, Xu and RH, Huang P. Glycolysis inhibition for anticancer treatment. Oncogene (2006) 25:4633–46. doi: 10.1038/sj.onc.1209597
91. Di Virgilio F, Adinolfi E. Extracellular purines, purinergic receptors and tumor growth. Oncogene (2017) 36:293–303. doi: 10.1038/onc.2016.206
92. Magnifico MC, Macone A, Marani M, Bouzidi A, Giardina G, Rinaldo S, et al. Linking infection and prostate cancer progression: Toll-like Receptor3 stimulation rewires glucose metabolism in prostate cells. Anticancer Res (2019) 39:5541–9. doi: 10.21873/anticanres.13747
93. Koppenol WH, Bounds PL, Dang CV. Otto Warburg’s contributions to current concepts of cancer metabolism. Nat Rev Cancer (2011) 11:325–37. doi: 10.1038/nrc3038
94. Amoroso F, Falzoni S, Adinolfi E, Ferrari D, Di Virgilio F. The P2X7 receptor is a key modulator of aerobic glycolysis. Cell Death Dis (2012) 3:e370–0. doi: 10.1038/cddis.2012.105
95. Amoroso F, Capece M, Rotondo A, Cangelosi D, Ferracin M, Franceschini A, et al. The P2X7 receptor is a key modulator of the PI3K/GSK3β/VEGF signaling network: evidence in experimental neuroblastoma. Oncogene (2015) 34:5240–51. doi: 10.1038/onc.2014.444
96. Choi HY, Siddique HR, Zheng M, Kou Y, Yeh D-W, Machida T, et al. p53 destabilizing protein skews asymmetric division and enhances NOTCH activation to direct self-renewal of TICs. Nat Commun (2020) 11:1–16. doi: 10.1038/s41467-020-16616-8
97. Adinolfi E, Pizzirani C, Idzko M, Panther E, Norgauer J, Di Virgilio F, et al. P2X 7 receptor: Death or life? Purinergic Signal (2005) 1:219. doi: 10.1007/s11302-005-6322-x
98. Sarti AC, Vultaggio-Poma V, Falzoni S, Missiroli S, Giuliani AL, Boldrini P, et al. Mitochondrial P2X7 receptor localization modulates energy metabolism enhancing physical performance. Function (2021) 2:zqab005. doi: 10.1093/function/zqab005
99. Giacovazzo G, Fabbrizio P, Apolloni S, Coccurello R, Volonté C. Stimulation of P2X7 enhances whole body energy metabolism in mice. Front Cell Neurosci (2019) 13:390. doi: 10.3389/fncel.2019.00390
100. Stefano L, Rössler OG, Griesemer D, Hoth M, Thiel G. P2X7 receptor stimulation upregulates egr-1 biosynthesis involving a cytosolic Ca2+ rise, transactivation of the EGF receptor and phosphorylation of ERK and elk-1. J Cell Physiol (2007) 213:36–44. doi: 10.1002/jcp.21085
101. Choi JH, Ji YG, Ko JJ, Cho HJ, Lee DH. Activating P2X7 receptors increases proliferation of human pancreatic cancer cells via ERK1/2 and JNK. Pancreas (2018) 47:643–51. doi: 10.1097/MPA.0000000000001055
102. Shin M-K, Cheong J-H. Mitochondria-centric bioenergetic characteristics in cancer stem-like cells. Arch Pharm Res (2019) 42:113–27. doi: 10.1007/s12272-019-01127-y
103. Liu PP, Liao J, Tang ZJ, Wu WJ, Yang J, Zeng ZL, et al. Metabolic regulation of cancer cell side population by glucose through activation of the akt pathway. Cell Death Differ (2014) 21:124–35. doi: 10.1038/cdd.2013.131
104. Gao C, Shen Y, Jin F, Miao Y, Qiu X. Cancer stem cells in small cell lung cancer cell line H446: higher dependency on oxidative phosphorylation and mitochondrial substrate-level phosphorylation than non-stem cancer cells. PloS One (2016) 11:e0154576. doi: 10.1371/journal.pone.0154576
105. Ulrich H. “Purinergic receptors in stem cell biology.,”. In: Stem cells and cancer stem cells, vol. Volume 8. Dordrecht:Springer (2012). p. 267–74.
106. Chang C-H, Qiu J, O’Sullivan D, Buck MD, Noguchi T, Curtis JD, et al. Metabolic competition in the tumor microenvironment is a driver of cancer progression. Cell (2015) 162:1229–41. doi: 10.1016/j.cell.2015.08.016
107. Lupiáñez DG, Kraft K, Heinrich V, Krawitz P, Brancati F, Klopocki E, et al. Disruptions of topological chromatin domains cause pathogenic rewiring of gene-enhancer interactions. Cell (2015) 161:1012–25. doi: 10.1016/j.cell.2015.04.004
108. Ho P-C, Bihuniak JD, Macintyre AN, Staron M, Liu X, Amezquita R, et al. Phosphoenolpyruvate is a metabolic checkpoint of anti-tumor T cell responses. Cell (2015) 162:1217–28. doi: 10.1016/j.cell.2015.08.012
109. Goossens P, Rodriguez-Vita J, Etzerodt A, Masse M, Rastoin O, Gouirand V, et al. Membrane cholesterol efflux drives tumor-associated macrophage reprogramming and tumor progression. Cell Metab (2019) 29:1376–1389.e4. doi: 10.1016/j.cmet.2019.02.016
110. Cascone T, McKenzie JA, Mbofung RM, Punt S, Wang Z, Xu C, et al. Increased tumor glycolysis characterizes immune resistance to adoptive T cell therapy. Cell Metab (2018) 27:977–987.e4. doi: 10.1016/j.cmet.2018.02.024
111. Feng J, Yang H, Zhang Y, Wei H, Zhu Z, Zhu B, et al. Tumor cell-derived lactate induces TAZ-dependent upregulation of PD-L1 through GPR81 in human lung cancer cells. Oncogene (2017) 36:5829–39. doi: 10.1038/onc.2017.188
112. Feichtinger RG, Lang R. Targeting l-lactate metabolism to overcome resistance to immune therapy of melanoma and other tumor entities. J Oncol (2019) 2019:2084195. doi: 10.1155/2019/2084195
113. Buxton ILO, Yokdang N, Matz RM. Purinergic mechanisms in breast cancer support intravasation, extravasation and angiogenesis. Cancer Lett (2010) 291:131–41. doi: 10.1016/j.canlet.2009.09.021
114. Rumjahn SM, Yokdang N, Baldwin KA, Thai J, Buxton ILO. Purinergic regulation of vascular endothelial growth factor signaling in angiogenesis. Br J Cancer (2009) 100:1465–70. doi: 10.1038/sj.bjc.6604998
115. Palinski W, Monti M, Camerlingo R, Iacobucci I, Bocella S, Pinto F, et al. Lysosome purinergic receptor P2X4 regulates neoangiogenesis induced by microvesicles from sarcoma patients. Cell Death Dis (2021) 12:797. doi: 10.1038/s41419-021-04069-w
116. Lapel M, Weston P, Strassheim D, Karoor V, Burns N, Lyubchenko T, et al. Glycolysis and oxidative phosphorylation are essential for purinergic receptor-mediated angiogenic responses in vasa vasorum endothelial cells. Am J Physiol Physiol (2017) 312:C56–70. doi: 10.1152/ajpcell.00250.2016
117. Yi M, Li J, Chen S, Cai J, Ban Y, Peng Q, et al. Emerging role of lipid metabolism alterations in cancer stem cells. J Exp Clin Cancer Res (2018) 37:1–18. doi: 10.1186/s13046-018-0784-5
118. Kamphorst JJ, Cross JR, Fan J, De Stanchina E, Mathew R, White EP, et al. Hypoxic and ras-transformed cells support growth by scavenging unsaturated fatty acids from lysophospholipids. Proc Natl Acad Sci (2013) 110:8882–7. doi: 10.1073/pnas.1307237110
119. Kuo C-Y, Ann DK. When fats commit crimes: fatty acid metabolism, cancer stemness and therapeutic resistance. Cancer Commun (2018) 38:1–12. doi: 10.1186/s40880-018-0317-9
120. Schug ZT, Peck B, Jones DT, Zhang Q, Grosskurth S, Alam IS, et al. Acetyl-CoA synthetase 2 promotes acetate utilization and maintains cancer cell growth under metabolic stress. Cancer Cell (2015) 27:57–71. doi: 10.1016/j.ccell.2014.12.002
121. Xu S, Chen T, Dong L, Li T, Xue H, Gao B, et al. Fatty acid synthase promotes breast cancer metastasis by mediating changes in fatty acid metabolism. Oncol Lett (2021) 21:1. doi: 10.3892/ol.2020.12288
122. Yasumoto Y, Miyazaki H, Vaidyan LK, Kagawa Y, Ebrahimi M, Yamamoto Y, et al. Inhibition of fatty acid synthase decreases expression of stemness markers in glioma stem cells. PloS One (2016) 11:e0147717. doi: 10.1371/journal.pone.0147717
123. Fhu CW, Ali A. Fatty acid synthase: An emerging target in cancer. Molecules (2020) 25:3935. doi: 10.3390/molecules25173935
124. Wu W, Shi X, Xu C. Regulation of T cell signalling by membrane lipids. Nat Rev Immunol (2016) 16:690–701. doi: 10.1038/nri.2016.103
125. Yang W, Bai Y, Xiong Y, Zhang J, Chen S, Zheng X, et al. Potentiating the antitumour response of CD8+ T cells by modulating cholesterol metabolism. Nature (2016) 531:651–5. doi: 10.1038/nature17412
126. Rabelo ILA, Arnaud-Sampaio VF, Adinolfi E, Ulrich H, Lameu C. Cancer metabostemness and metabolic reprogramming via P2X7 receptor. Cells (2021) 10:1782. doi: 10.3390/cells10071782
127. Arguin G, Bourzac J-F, Placet M, Molle CM, Paquette M, Beaudoin J-F, et al. The loss of P2X7 receptor expression leads to increase intestinal glucose transit and hepatic steatosis. Sci Rep (2017) 7:1–16. doi: 10.1038/s41598-017-13300-8
128. Adinolfi E, Callegari MG, Ferrari D, Bolognesi C, Minelli M, Wieckowski MR, et al. Basal activation of the P2X7 ATP receptor elevates mitochondrial calcium and potential, increases cellular ATP levels, and promotes serum-independent growth. Mol Biol Cell (2005) 16:3260–72. doi: 10.1091/mbc.e04-11-1025
129. Giacovazzo G, Apolloni S, Coccurello R. Loss of P2X7 receptor function dampens whole body energy expenditure and fatty acid oxidation. Purinergic Signal (2018) 14:299–305. doi: 10.1007/s11302-018-9610-y
130. Li YC, Park MJ, Ye S-K, Kim C-W, Kim Y-N. Elevated levels of cholesterol-rich lipid rafts in cancer cells are correlated with apoptosis sensitivity induced by cholesterol-depleting agents. Am J Pathol (2006) 168:1107–18. doi: 10.2353/ajpath.2006.050959
131. Karasawa A, Michalski K, Mikhelzon P, Kawate T. The P2X7 receptor forms a dye-permeable pore independent of its intracellular domain but dependent on membrane lipid composition. Elife (2017) 6:e31186. doi: 10.7554/eLife.31186.018
132. Resnik N, Repnik U, Kreft ME, Sepčić K, Maček P, Turk B, et al. Highly selective anti-cancer activity of cholesterol-interacting agents methyl-β-cyclodextrin and ostreolysin a/pleurotolysin b protein complex on urothelial cancer cells. PloS One (2015) 10:e0137878. doi: 10.1371/journal.pone.0137878
133. Kleinfeld AM, Okada C. Free fatty acid release from human breast cancer tissue inhibits cytotoxic T-lymphocyte-mediated killing. J Lipid Res (2005) 46:1983–90. doi: 10.1194/jlr.m500151-jlr200
134. Guillaumond F, Bidaut G, Ouaissi M, Servais S, Gouirand V, Olivares O, et al. Cholesterol uptake disruption, in association with chemotherapy, is a promising combined metabolic therapy for pancreatic adenocarcinoma. Proc Natl Acad Sci (2015) 112:2473–8. doi: 10.1073/pnas.1421601112
135. Fu S, Li Z, Xiao L, Hu W, Zhang L, Xie B, et al. Glutamine synthetase promotes radiation resistance via facilitating nucleotide metabolism and subsequent DNA damage repair. Cell Rep (2019) 28:1136–43. doi: 10.1016/j.celrep.2019.07.002
136. Scalise M, Pochini L, Galluccio M, Console L, Indiveri C. Glutamine transport and mitochondrial metabolism in cancer cell growth. Front Oncol (2017) 7:306. doi: 10.3389/fonc.2017.00306
137. Jeon YJ, Khelifa S, Ratnikov B, Scott DA, Feng Y, Parisi F, et al. Regulation of glutamine carrier proteins by RNF5 determines breast cancer response to ER stress-inducing chemotherapies. Cancer Cell (2015) 27:354–69. doi: 10.1016/j.ccell.2015.02.006
138. Altman BJ, Stine ZE, Dang CV. From Krebs to clinic: glutamine metabolism to cancer therapy. Nat Rev Cancer (2016) 16:619–34. doi: 10.1038/nrc.2016.71
139. Leone RD, Zhao L, Englert JM, Sun I-M, Oh M-H, Sun I-H, et al. Glutamine blockade induces divergent metabolic programs to overcome tumor immune evasion. Sci (80- ) (2019) 366:1013–21. doi: 10.1126/science.aav2588
140. Parker KH, Beury DW, Ostrand-Rosenberg S. Myeloid-derived suppressor cells: Critical cells driving immune suppression in the tumor microenvironment. Adv Cancer Res (2015) 12895–139. doi: 10.1016/bs.acr.2015.04.002.
141. Oh M-H, Sun I-H, Zhao L, Leone RD, Sun I-M, Xu W, et al. Targeting glutamine metabolism enhances tumor-specific immunity by modulating suppressive myeloid cells. J Clin Invest (2020) 130:3865–84. doi: 10.1172/JCI131859
142. Uyttenhove C, Pilotte L, Théate I, Stroobant V, Colau D, Parmentier N, et al. Evidence for a tumoral immune resistance mechanism based on tryptophan degradation by indoleamine 2, 3-dioxygenase. Nat Med (2003) 9:1269–74. doi: 10.1038/nm934
143. Munn DH. Blocking IDO activity to enhance anti-tumor immunity. Front Biosci (Elite Ed) (2012) 4:734–45. doi: 10.2741/e414
144. Balachandran VP, Cavnar MJ, Zeng S, Bamboat ZM, Ocuin LM, Obaid H, et al. Imatinib potentiates antitumor T cell responses in gastrointestinal stromal tumor through the inhibition of ido. Nat Med (2011) 17:1094–100. doi: 10.1038/nm.2438
145. Opitz CA, Litzenburger UM, Sahm F, Ott M, Tritschler I, Trump S, et al. An endogenous tumour-promoting ligand of the human aryl hydrocarbon receptor. Nature (2011) 478:197–203. doi: 10.1038/nature10491
146. Munn DH, Shafizadeh E, Attwood JT, Bondarev I, Pashine A, Mellor AL. Inhibition of T cell proliferation by macrophage tryptophan catabolism. J Exp Med (1999) 189:1363–72. doi: 10.1084/jem.189.9.1363
147. Munn DH, Mellor AL. IDO in the tumor microenvironment: Inflammation, counter-regulation, and tolerance. Trends Immunol (2016) 37:193–207. doi: 10.1016/j.it.2016.01.002
148. Mbongue JC, Nicholas DA, Torrez TW, Kim N-S, Firek AF, Langridge WHR. The role of indoleamine 2, 3-dioxygenase in immune suppression and autoimmunity. Vaccines (2015) 3:703–29. doi: 10.3390/vaccines3030703
149. Nguyen NT, Kimura A, Nakahama T, Chinen I, Masuda K, Nohara K, et al. Aryl hydrocarbon receptor negatively regulates dendritic cell immunogenicity via a kynurenine-dependent mechanism. Proc Natl Acad Sci (2010) 107:19961–6. doi: 10.1073/pnas.1014465107
150. Wang W-L, Chang W-L, Yang H-B, Chang I-W, Lee C-T, Chang C-Y, et al. Quantification of tumor infiltrating Foxp3+ regulatory T cells enables the identification of high-risk patients for developing synchronous cancers over upper aerodigestive tract. Oral Oncol (2015) 51:698–703. doi: 10.1016/j.oraloncology.2015.04.015
151. Wei F, Wang D, Wei J, Tang N, Tang L, Xiong F, et al. Metabolic crosstalk in the tumor microenvironment regulates antitumor immunosuppression and immunotherapy resisitance. Cell Mol Life Sci (2021) 78:173–93. doi: 10.1007/s00018-020-03581-0
152. Satija S, Kaur H, Tambuwala MM. Hypoxia-inducible factor (hif): fuel for cancer progression. PCurr Mol Pharmacol. (2021) 14(3):321–32. doi: 10.2174/1874467214666210120154929
153. Tang T, Yang L, Cao Y, Wang M, Zhang S, Gong Z, et al. LncRNA AATBC regulates pinin to promote metastasis in nasopharyngeal carcinoma. Mol Oncol (2020) 14:2251–70. doi: 10.1002/1878-0261.12703
154. Wang D, Zeng Z, Zhang S, Xiong F, He B, Wu Y, et al. Epstein-Barr Virus-encoded miR-BART6-3p inhibits cancer cell proliferation through the LOC553103-STMN1 axis. FASEB J (2020) 34:8012–27. doi: 10.1096/fj.202000039RR
155. Wang D, Tang L, Wu Y, Fan C, Zhang S, Xiang B, et al. Abnormal X chromosome inactivation and tumor development. Cell Mol Life Sci (2020) 77:2949–58. doi: 10.1007/s00018-020-03469-z
156. Hatfield SM, Kjaergaard J, Lukashev D, Schreiber TH, Belikoff B, Abbott R, et al. Immunological mechanisms of the antitumor effects of supplemental oxygenation. Sci Transl Med (2015) 7:277ra30–277ra30. doi: 10.1126/scitranslmed.aaa1260
157. Poth JM, Brodsky K, Ehrentraut H, Grenz A, Eltzschig HK. Transcriptional control of adenosine signaling by hypoxia-inducible transcription factors during ischemic or inflammatory disease. J Mol Med (2013) 91:183–93. doi: 10.1007/s00109-012-0988-7
158. Wu Q, Zhou W, Yin S, Zhou Y, Chen T, Qian J, et al. Blocking triggering receptor expressed on myeloid cells-1-Positive tumor-associated macrophages induced by hypoxia reverses immunosuppression and anti-programmed cell death ligand 1 resistance in liver cancer. Hepatology (2019) 70:198–214. doi: 10.1002/hep.30593
159. Ge J, Wang J, Wang H, Jiang X, Liao Q, Gong Q, et al. The BRAF V600E mutation is a predictor of the effect of radioiodine therapy in papillary thyroid cancer. J Cancer (2020) 11:932–9. doi: 10.7150/jca.33105
160. Westendorf A, Skibbe K, Adamczyk A, Buer J, Geffers R, Hansen W, et al. Hypoxia enhances immunosuppression by inhibiting CD4+ effector T cell function and promoting treg activity. Cell Physiol Biochem (2017) 41:1271–84. doi: 10.1159/000464429
161. Li Y, Patel SP, Roszik J, Qin Y. Hypoxia-driven immunosuppressive metabolites in the tumor microenvironment: New approaches for combinational immunotherapy. Front Immunol (2018) 9:1591. doi: 10.3389/fimmu.2018.01591
162. Jayaprakash P, Ai M, Liu A, Budhani P, Bartkowiak T, Sheng J, et al. Targeted hypoxia reduction restores T cell infiltration and sensitizes prostate cancer to immunotherapy. J Clin Invest (2018) 128:5137–49. doi: 10.1172/JCI96268
163. Barsoum IB, Smallwood CA, Siemens DR, Graham CH. A mechanism of hypoxia-mediated escape from adaptive immunity in cancer cells. Cancer Res (2014) 74:665–74. doi: 10.1158/0008-5472.CAN-13-0992
164. Sarkar S, Germeraad WTV, Rouschop KMA, Steeghs EMP, van Gelder M, GMJ B, et al. Hypoxia induced impairment of NK cell cytotoxicity against multiple myeloma can be overcome by IL-2 activation of the NK cells. PloS One (2013) 8:e64835. doi: 10.1371/journal.pone.0064835
165. Zhang H, Lu H, Xiang L, Bullen JW, Zhang C, Samanta D, et al. HIF-1 regulates CD47 expression in breast cancer cells to promote evasion of phagocytosis and maintenance of cancer stem cells. Proc Natl Acad Sci (2015) 112:E6215–23. doi: 10.1073/pnas.1520032112
166. Barsoum IB, Hamilton TK, Li X, Cotechini T, Miles EA, Siemens DR, et al. Hypoxia induces escape from innate immunity in cancer cells via increased expression of ADAM10: role of nitric oxide. Cancer Res (2011) 71:7433–41. doi: 10.1158/0008-5472.CAN-11-2104
167. Facciabene A, Peng X, Hagemann IS, Balint K, Barchetti A, Wang L-P, et al. Tumour hypoxia promotes tolerance and angiogenesis via CCL28 and T reg cells. Nature (2011) 475:226–30. doi: 10.1038/nature10169
168. Al-Anazi A, Parhar R, Saleh S, Al-Hijailan R, Inglis A, Al-Jufan M, et al. Intracellular calcium and NF-kB regulate hypoxia-induced leptin, VEGF, IL-6 and adiponectin secretion in human adipocytes. Life Sci (2018) 212:275–84. doi: 10.1016/j.lfs.2018.10.014
169. Gabrilovich D. Mechanisms and functional significance of tumour-induced dendritic-cell defects. Nat Rev Immunol (2004) 4:941–52. doi: 10.1038/nri1498
170. Khaled YS, Ammori BJ, Elkord E. Myeloid-derived suppressor cells in cancer: recent progress and prospects. Immunol Cell Biol (2013) 91:493–502. doi: 10.1038/icb.2013.29
171. Wheeler KC, Jena MK, Pradhan BS, Nayak N, Das S, Hsu C-D, et al. VEGF may contribute to macrophage recruitment and M2 polarization in the decidua. PloS One (2018) 13:e0191040. doi: 10.1371/journal.pone.0191040
172. Lardner A. The effects of extracellular pH on immune function. J Leukoc Biol (2001) 69:522–30. doi: 10.1189/jlb.69.4.522
173. Nathan I, Groopman JE, Quan SG, Bersch N, Golde DW. Immune (γ) interferon produced by a human T-lymphoblast cell line. Nature (1981) 292:842–4. doi: 10.1038/292842a0
174. Pearce EL, Poffenberger MC, Chang C-H, Jones RG. Fueling immunity: insights into metabolism and lymphocyte function. Sci (80- ) (2013) 342:1242454. doi: 10.1126/science.1242454
175. Palmer CS, Ostrowski M, Balderson B, Christian N, Crowe SM. Glucose metabolism regulates T cell activation, differentiation, and functions. Front Immunol (2015) 6:1. doi: 10.3389/fimmu.2015.00001
176. Aria H, Ghaedrahmati F, Ganjalikhani-Hakemi M. Cutting edge: Metabolic immune reprogramming, reactive oxygen species, and cancer. J Cell Physiol (2021) 236:6168–89. doi: 10.1002/jcp.30303
177. Le Bourgeois T, Strauss L, Aksoylar H-I, Daneshmandi S, Seth P, Patsoukis N, et al. Targeting T cell metabolism for improvement of cancer immunotherapy. Front Oncol (2018) 8:237. doi: 10.3389/fonc.2018.00237
178. Xiao Z, Dai Z, Locasale JW. Metabolic landscape of the tumor microenvironment at single cell resolution. Nat Commun (2019) 10:1–12. doi: 10.1038/s41467-019-11738-0
179. Macintyre AN, Gerriets VA, Nichols AG, Michalek RD, Rudolph MC, Deoliveira D, et al. The glucose transporter Glut1 is selectively essential for CD4 T cell activation and effector function. Cell Metab (2014) 20:61–72. doi: 10.1016/j.cmet.2014.05.004
180. Cao Y, Rathmell JC, Macintyre AN. Metabolic reprogramming towards aerobic glycolysis correlates with greater proliferative ability and resistance to metabolic inhibition in CD8 versus CD4 T cells. PloS One (2014) 9:e104104. doi: 10.1371/journal.pone.0104104
181. Maj T, Wang W, Crespo J, Zhang H, Wang W, Wei S, et al. Oxidative stress controls regulatory T cell apoptosis and suppressor activity and PD-L1-blockade resistance in tumor. Nat Immunol (2017) 18:1332. doi: 10.1038/ni.3868
182. Rath M, Müller I, Kropf P, Closs EI, Munder M. Metabolism via arginase or nitric oxide synthase: Two competing arginine pathways in macrophages. Front Immunol (2014) 5:532. doi: 10.3389/fimmu.2014.00532
183. Liu D, Chang C, Lu N, Wang X, Lu Q, Ren X, et al. Comprehensive proteomics analysis reveals metabolic reprogramming of tumor-associated macrophages stimulated by the tumor microenvironment. J Proteome Res (2017) 16:288–97. doi: 10.1021/acs.jproteome.6b00604
184. Netea-Maier RT, Smit JWA, Netea MG. Metabolic changes in tumor cells and tumor-associated macrophages: a mutual relationship. Cancer Lett (2018) 413:102–9. doi: 10.1016/j.canlet.2017.10.037
185. Torisu H, Ono M, Kiryu H, Furue M, Ohmoto Y, Nakayama J, et al. Macrophage infiltration correlates with tumor stage and angiogenesis in human malignant melanoma: possible involvement of TNFα and IL-1α. Int J Cancer (2000) 85:182–8. doi: 10.1002/(SICI)1097-0215(20000115)85:2%3C182::AID-IJC6%3E3.0.CO;2-M
186. Zhang Y, Yu G, Chu H, Wang X, Xiong L, Cai G, et al. Macrophage-associated PGK1 phosphorylation promotes aerobic glycolysis and tumorigenesis. Mol Cell (2018) 71:201–15. doi: 10.1016/j.molcel.2018.06.023
187. Chen F, Chen J, Yang L, Liu J, Zhang X, Zhang Y, et al. Extracellular vesicle-packaged HIF-1α-stabilizing lncRNA from tumour-associated macrophages regulates aerobic glycolysis of breast cancer cells. Nat Cell Biol (2019) 21:498–510. doi: 10.1038/s41556-019-0299-0
188. Binenbaum Y, Fridman E, Yaari Z, Milman N, Schroeder A, Ben David G, et al. Transfer of miRNA in macrophage-derived exosomes induces drug resistance in pancreatic adenocarcinoma. Cancer Res (2018) 78:5287 LP – 5299. doi: 10.1158/0008-5472.CAN-18-0124
189. Qin J, Zhang X, Tan B, Zhang S, Yin C, Xue Q, et al. Blocking P2X7-mediated macrophage polarization overcomes treatment resistance in lung cancer. Cancer Immunol Res (2020) 8:1426–39. doi: 10.1158/2326-6066.CIR-20-0123
190. Ostrand-Rosenberg S. Myeloid derived-suppressor cells: their role in cancer and obesity. Curr Opin Immunol (2018) 51:68–75. doi: 10.1016/j.coi.2018.03.007
191. Yan D, Adeshakin AO, Xu M, Afolabi LO, Zhang G, Chen YH, et al. Lipid metabolic pathways confer the immunosuppressive function of myeloid-derived suppressor cells in tumor. Front Immunol (2019) 10:1399. doi: 10.3389/fimmu.2019.01399
192. Kumar V, Patel S, Tcyganov E, Gabrilovich DI. The nature of myeloid-derived suppressor cells in the tumor microenvironment. Trends Immunol (2016) 37:208–20. doi: 10.1016/j.it.2016.01.004
193. Cubillos-Ruiz JR, Silberman PC, Rutkowski MR, Chopra S, Perales-Puchalt A, Song M, et al. ER stress sensor XBP1 controls anti-tumor immunity by disrupting dendritic cell homeostasis. Cell (2015) 161:1527–38. doi: 10.1016/j.cell.2015.05.025
194. Merad M, Salmon H. A dendritic-cell brake on antitumour immunity. Nature (2015) 523:294–5. doi: 10.1038/523294a
195. Giovanelli P, Sandoval TA, Cubillos-Ruiz JR. Dendritic cell metabolism and function in tumors. Trends Immunol (2019) 40:699–718. doi: 10.1016/j.it.2019.06.004
196. Jacob F, Novo CP, Bachert C, Van Crombruggen K. Purinergic signaling in inflammatory cells: P2 receptor expression, functional effects, and modulation of inflammatory responses. Purinergic Signal (2013) 9:285–306. doi: 10.1007/s11302-013-9357-4
197. Bastid J, Regairaz A, Bonnefoy N, Déjou C, Giustiniani J, Laheurte C, et al. Inhibition of CD39 enzymatic function at the surface of tumor cells alleviates their immunosuppressive activity. Cancer Immunol Res (2015) 3:254–65. doi: 10.1158/2326-6066.CIR-14-0018
198. Hay CM, Sult E, Huang Q, Mulgrew K, Fuhrmann SR, McGlinchey KA, et al. Targeting CD73 in the tumor microenvironment with MEDI9447. Oncoimmunology (2016) 5:e1208875. doi: 10.1080/2162402X.2016.1208875
199. de Leve S, Wirsdörfer F, Jendrossek V. Targeting the immunomodulatory CD73/adenosine system to improve the therapeutic gain of radiotherapy. Front Immunol (2019) 10:698. doi: 10.3389/fimmu.2019.00698
200. Wennerberg E, Spada S, Rudqvist N-P, Lhuillier C, Gruber S, Chen Q, et al. CD73 blockade promotes dendritic cell infiltration of irradiated tumors and tumor rejection. Cancer Immunol Res (2020) 8:465–78. doi: 10.1158/2326-6066.CIR-19-0449
201. Kaiser J. When less is more. Science (2017) 355(6330):1144–46. doi: 10.1126/science.355.6330.1144
202. Cheewatrakoolpong B, Gilchrest H, Anthes JC, Greenfeder S. Identification and characterization of splice variants of the human P2X7 ATP channel. Biochem Biophys Res Commun (2005) 332:17–27. doi: 10.1016/j.bbrc.2005.04.087
203. Adinolfi E, Capece M, Amoroso F, De Marchi E, Franceschini A. Emerging roles of P2X receptors in cancer. Curr Med Chem (2015) 22:878–90. doi: 10.2174/0929867321666141012172913
204. Di Virgilio F, Falzoni S, Giuliani AL, Adinolfi E. P2 receptors in cancer progression and metastatic spreading. Curr Opin Pharmacol (2016) 29:17–25. doi: 10.1016/j.coph.2016.05.001
205. Adinolfi E, Amoroso F, Giuliani AL. P2X7 receptor function in bone-related cancer. J Osteoporos (2012) 2012:637863. doi: 10.1155/2012/637863
206. Zhu A, Lee D, Shim H. Metabolic positron emission tomography imaging in cancer detection and therapy response. Semin Oncol (2011) 38(1):55–69. doi: 10.1053/j.seminoncol.2010.11.012
207. Chen X, Qian Y, Wu S. The warburg effect: evolving interpretations of an established concept. Free Radic Biol Med (2015) 79:253–63. doi: 10.1016/j.freeradbiomed.2014.08.027
208. Qian Y, Wang X, Chen X. Inhibitors of glucose transport and glycolysis as novel anticancer therapeutics. World (2014) 3(2):37–57. doi: 10.5528/wjtm.v3.i2.37
Keywords: therapy resistance, tumor microenvironment, metabolic reprogramming, purinergic receptor, cancer metabolism, immunometabolism
Citation: Aria H, Rezaei M, Nazem S, Daraei A, Nikfar G, Mansoori B, Bahmanyar M, Tavassoli A, Vakil MK and Mansoori Y (2022) Purinergic receptors are a key bottleneck in tumor metabolic reprogramming: The prime suspect in cancer therapeutic resistance. Front. Immunol. 13:947885. doi: 10.3389/fimmu.2022.947885
Received: 19 May 2022; Accepted: 04 August 2022;
Published: 22 August 2022.
Edited by:
Wei-Hua Yan, Wenzhou Medical University, ChinaReviewed by:
Claudiana Lameu, University of São Paulo, BrazilCopyright © 2022 Aria, Rezaei, Nazem, Daraei, Nikfar, Mansoori, Bahmanyar, Tavassoli, Vakil and Mansoori. This is an open-access article distributed under the terms of the Creative Commons Attribution License (CC BY). The use, distribution or reproduction in other forums is permitted, provided the original author(s) and the copyright owner(s) are credited and that the original publication in this journal is cited, in accordance with accepted academic practice. No use, distribution or reproduction is permitted which does not comply with these terms.
*Correspondence: Yaser Mansoori, ZnVtcy5tYW5zb29yaUBnbWFpbC5jb20=; Mohammad Kazem Vakil, bW9oYW1tYWRrYXplbXZha2lsLmZ1bXNAZ21haWwuY29t
†These authors have contributed equally to this work and share correspondence
Disclaimer: All claims expressed in this article are solely those of the authors and do not necessarily represent those of their affiliated organizations, or those of the publisher, the editors and the reviewers. Any product that may be evaluated in this article or claim that may be made by its manufacturer is not guaranteed or endorsed by the publisher.
Research integrity at Frontiers
Learn more about the work of our research integrity team to safeguard the quality of each article we publish.