- Food College, Northeast Agricultural University, Harbin, China
The intestine is the largest digestive and immune organ in the human body, with an intact intestinal mucosal barrier. Bifidobacterium longum is the specific gut commensals colonized in the human gut for boosting intestinal immunity to defend against intestinal mucosal immune injury. In the LPS-induced intestinal injury model, the Bifidobacterium longum BL-10 was suggested to boost the intestinal immune. Detailly, compared with the LPS-induced mice, the BL10 group significantly reduced intestine (jejunum, ileum, and colon) tissue injury, pro-inflammatory cytokines (TNF-α, IFN-γ, IL-2, IL-6, IL-17, IL-22, and IL-12) levels and myeloperoxidase activities. Moreover, the B. longum BL-10 significantly increased the number of immunocytes (CD4+ T cells, IgA plasma cells) and the expression of tight junction protein (Claudin1 and Occludin). B. longum BL-10 regulated the body’s immune function by regulating the Th1/Th2 and Th17/Treg balance, which showed a greater impact on the Th1/Th2 balance. Moreover, the results also showed that B. longum BL-10 significantly down-regulated the intestinal protein expression of TLR4, p-IκB, and NF-κB p65. The B. longum BL-10 increased the relative abundance of the genera, including Lachnospiraceae_NK4A136_group and Clostridia_UCG-014, which were related to declining the levels of intestinal injury. Overall, these results indicated that the B. longum BL-10 had great functionality in reducing LPS-induced intestinal mucosal immune injury.
Introduction
Bifidobacterium is the specific gut commensals colonized in the human gut, especially in the colon. Numerous studies have demonstrated that Bifidobacterium is crucial for maintaining the intestinal micro-ecological balance necessary for anti-tumor, immunological modulation, and the deconstruction and transformation of nutrition (1). It also interacts with human immunocytes and modulates specific pathways, involving innate and adaptive immune processes (2). Recent evidence proposed that newborns were born with a sterile gut and their physical immunity increased with age, positively correlated with the number of bifidobacteria (3). Thus, Bifidobacterium is critical in the maturation of the human immune system from gestation to childhood. This became crucial to understanding the processes by which certain strains might modify the gut microbiota and control immune responses directly or indirectly.
The intestine, directly exposed to disease-causing germs, viruses, and allergens (4), is a critical immunological organ of the body, and its structural and functional characteristics contribute to the formation of the most extensive mucosal immune system (5). Gut-associated lymphoid tissue (GALT) is an essential part of the intestinal mucosal immune system (6), and it is also the leading site of mucosal immunity, which consists mainly of mesenteric lymph nodes (MIN), lamina propria (LP), Peyer’s patch (PP), and intestinal epithelial lymphocytes (IEL) (7). In detail, the initial line of intestinal defense is based on intestinal epithelial cells secreting antimicrobial peptides (AMP) termed defensins (8). The second line of defense is the creation of immunoglobulin A (IgA) that may pass the epithelial barrier between the host and the microbiota (9), completely covering each bacterium and blocking bacterial translocation. The secretory immunoglobulin A (sIgA) is an essential antibody on the mucosal surface exerting immune clearance without inflammatory immune response (10). The sIgA prevents intestinal infection by conditionally harmful bacteria by binding to antigens and blocking adhesion and invasion (11). SJOGREN et al. demonstrated that Bifidobacterium bifidum increased sIgA secretion in intestinal mucosa by measuring sIgA levels in stool and saliva specimens from infants (12). The third line of defense consists mainly of immune cells associated with it, such as dendritic cells (DC), B lymphocytes, T lymphocytes, and innate lymphocytes (13). Previous studies revealed that Bifidobacterium might directly modulate the immune system by interacting with GALT.
Bifidobacterium has a protective effect on the intestinal mucosa in two ways (14), involving maintaining the balance of the intestinal microflora (14) and directly acting on the immune system to induce intestinal immunity. Yan et al. confirmed that Bifidobacterium bifidum YS108R fermented milk partially reversed the imbalance of gut microbiota in DSS-induced mice and prevented the increase of pathogenic bacteria (15). Further, Bifidobacterium can stimulate intestinal mucosal immune cells to secrete cytokines, with an important role in inducing proliferation and differentiation of immune cells and enhancing the immune response. The growth of T cells in the thymus was affected by Bifidobacterium via enhancing the maturation of regional dendritic cells and IL-12 expression in the gut, according to the research (16).
Bifidobacterium can suppress the expression of toll-like receptor 4 (TLR4), regulate T cell differentiation, and inhibit the NF-κB pathway activity by improving the intestinal immune response to pathogenic bacteria (17). Previous researches on the relationship between it and immune cells pointed to Bifidobacterium treatment mice stimulating DCs proliferation and immunological activation by increasing DCs numbers and anti-tumor CD8+ T cell proliferation (18). Moreover, Bifidobacterium can improve the mucosal immune system by indirectly controlling the gut microenvironment (19). For instance, DONG et al. demonstrated that Bifidobacterium could enhance intestinal immune function, as it promoted IL-12 secretion from intestinal dendritic cells, up-regulated IL-10 and IFN-γ secretion in plasma, increased the IFN-γ/IL-4 ratio in the intestinal mucosa, and promoted thymic T cells differentiation toT helper cells 1 (Th1) (16).
Bifidobacterium longum BL-10, a newly identified probiotic, was obtained from the feces of healthy breast-fed infants in the key lab of dairy science (KLDS). In a recent study, B. longum BL-10 possessing beneficial intestinal colonization properties was the most effective promoter of proliferation on normal colon epithelial CCD 841 CoN cells (20). In vitro experiment, we evaluated its effect on an LPS-induced cell injury model and showed that B. longum BL-10 could promote intestinal development by facilitating human fetal colon epithelial cell proliferation and accelerating the maturation of the internal barrier (20). However, the immunoregulatory properties of B. longum BL-10 remain unknown in vivo, as is its immunoregulatory mechanism. In this study, we selected the LPS-induced BALB/c mice model to explore the mechanism of B. longum BL-10 to regulate intestinal immunity. We also investigated the cytokines and immune cell regulatory responses of B. longum BL-10 treatment on pro-inflammatory cytokine levels (TNF-α, IL-4, IL-6, and IL-12) and key regulatory protein expressions. This study provides a theoretical basis for B. longum practical applications, aiming to develop probiotic preparations for enhancing immunity.
Materials and methods
Bacterial isolates and culture conditions
B. longrum BL-10 was a novel strain of bacteria from the intestines of healthy infants and Bifidobacterium animalis subsp. lactis BB-12 was purchased from Chr. Hansen Inc (Hoersholm, Denmark). All the experimental strains were stored at the Key Laboratory of Dairy Science (KLDS) of Northeast Agricultural University (NEAU, Harbin, China). For three generations, they were incubated in the MRS (de MAN, ROGOSA, and SHARPE) Broth with 0.05% L-cysteine (AOBOX, Beijing, China) at 37°C for 24 h in an anaerobic condition. After cryogenic centrifugation at 8000 rpm for 10 min, cell pellets were extracted and washed twice with physiological saline (0.9% NaCl). As to being administered intragastrically, the experimental strains were adjusted to 1.0×109 CFU/mL and stored at 4°C.
Animals and experimental design
40 BALB/c mice (SPF, female, six weeks old, 18–20 g) were obtained from the Beijing Vital River Laboratory Animal Technology Co. Ltd (Beijing, China). For experimental mice, they were acclimated to the laboratory environment at Northeast Agricultural University (Harbin, China) for at least one week. The environment maintained pathogen-free at constant temperature (20°C ± 2°C) and humidity (50% ± 5%) with 12 h/12 h light/dark cycle, access to water, and standard food. The experiment was authorized by the Animal Ethics Committee of NEAU (ethic approval code: NEAUEC20210475). Figure 1 depicts the detailed animal experiment. Briefly, all the mice were randomly divided into four groups (n=10), including the Control group, LPS group, BL10 group, and BB12 group. The mice in the control and LPS groups were intragastric with 0.2 mL saline daily, and the other two groups received 0.2mL bacteria suspension of B. longrum BL-10 and Bifidobacterium animalis subsp.lactis BB-12 (1.0×109 CFU/mL), respectively. After 14 days, laboratory mice except the control group were injected intraperitoneally at 5 mg/kg body weight and carried out euthanasia after 6 hours.
Hematoxylin-eosin stain
The intestinal tissues were treated for histological observation as the method described previously (21). Briefly, the jejunum, ileum, and colon samples were collected, fixed by immersion in 4% formaldehyde, and embedded in paraffin. Subsequently, the paraffin slices were placed on slides and stained with hematoxylin and eosin (H&E). Histological examination was performed using Microscopy BX53 (OLUMPUS, Japan) at × 200 magnification. Each mouse’s disease activity index (DAI) and histological score (HS) were evaluated based on the standards (Supplementary Tables 1, 2) to assess the degree of intestinal injury.
Alcian blue-periodic acid sthiff stain
The AB-PAS stain revealed the presence of goblet cells and mucin in the colon and ileum. The staining solution was dripped over the samples’ paraffin sections, and the sections were subsequently washed in water. Afterward, the slices were rinsed with tap water and re-stained with hematoxylin. All samples were observed and photographed using the Microscopy BX53 (OLUMPUS, Japan) at × 200 magnification. The results of Chiu’s score were assessed in light of the factor (Supplementary Table 3).
Intestinal immunohistochemistry
The immunohistochemistry (IHC) method was performed as previously described by Liu (22). The paraffin-embedded ileac sections were sliced to a thickness of 4 μm and placed on a slide. After being deparaffinized in xylene and rehydrated through ethanol to water, the slides were washed in PBS-0.1% (v/v) Tween 20 and dried. Endogenous peroxidase activity was suppressed for 15 min with 3% hydrogen peroxide in distilled water diluted with PBST. Following antigen extraction, the standard goat serum working solution was used to inhibit the non-specific antibody binding sites for 1 hour. The sections were respectively incubated with one of the following primary antibodies: rabbit anti-CD4+ (1:200, Abcam, Cambridge, UK), rabbit anti-CD8 alpha (1:200, Abcam), and rabbit anti-CD209 mAb (1:200, ABclonal, Wuhan, China). The slices were incubated for 30 min at 25°C with goat anti-rabbit IgG (1:400) from Millipore (Billerica, MA, USA) and observed by fluorescence microscopy at × 200 magnification. The outcomes were measured according to the Frenchy’s method (23), detailly, the mean gray value (staining intensity) and positive area percentage (staining area) of positive cells were used as IHC measurement indexes with high positive (3+), positive (2+), low Positive (1+) and negative (0).
Intestinal Immunol fluorescence
The detection of IgA-producing plasma cells on the ileum was assayed by direct immunofluorescence (IF) with the method of de Moreno et al. (24). The tissue sections were blocked with normal goat serum working solution for 10 minutes and incubated with Goat Anti-Mouse IgA alpha chain (Biotin) (1:200, Abcam) for 1 hour at 25°C. Each sample was treated with donkey anti-goat IgG (H+L) (1:150, Abcam) and photographed under a fluorescence microscope. The result was expressed as the photograph’s mean gray value (integrated density/area).
Enzyme-linked immunosorbent assay
The 2% tissue samples homogenized in saline (w/v=1:49) were prepared to determine IFN-γ, TNF-α, TGF-β, IL-2, IL-4, IL-6, IL-10, and IL-22 levels. The serum samples were left to stand at room temperature for 30 min and isolated by centrifugation at 4°C (3500 r/min, 10 min) to measure MPO activity and D-lactate (D-Lac) activities. All the samples were detected using commercial ELISA kits (Conodi creatures, Fujian, China) by following the kit instructions.
Quantitative RT-PCR
The mRNA expression levels of the major tight junction (TJ) protein ZO-1, Claudin1, Occludin, and mucoprotein mucin (Muc) 2 were investigated by RT-qPCR. Total RNA was obtained from ileac tissues by the RNAiso Plus kit, and cDNA was generated by the Transcriptor First Strand cDNA Synthesis kit (Vazyme, Nanjing, China). The PCR reactions were performed by Stormstar SybrGreen qPCR Master Mix (Promega, Madison, USA) on the Go Taq® SYBR-Green qPCR Master Mix (Promega, Madison, USA). Especially, in this study, the β-actin was used as the internal reference gene (Supplementary Table 2.3).
Western blot
The total protein was extracted from the appropriate ileac tissue regarding the kit method and was determined via the BCA kit. The Western blot assay was referenced from Xie et al. (25). Briefly, 30 μg issue was placed on a 10% SDS-PAGE gel to separate the proteins in each well. Western blots were blocked in 5% bovine serum albumin (BSA) and produced in Tris-buffered saline containing PBS-0.1% (v/v) Tween 20 for 2 hours at room temperature (TBST). Subsequently, all the samples were treated overnight at 4°C with rabbit polyclonal antibodies: anti-T-bet, anti-GATA-3, and anti-β-actin. After incubation with secondary HRP-conjugated goat antirabbit IgG, the result was analyzed by Gel-Pro-Analyzer software and normalized to the β-actin and Histone H3.
16S rRNA gene of gut microbiota
The gut microbiota total DNA was extracted according to the instructions of the fecal DNA extraction kit, a mix of the samples was based on the amount of data produced and the size of the fragments. Then samples were sequenced in the third generation on the PacBio sequencing platform. The effective-CCS sequence was 30 clustered according to the similarity of 97.0% to obtain OTUs. Finally, the diversity, difference, relevance, and species classification of the gut microbiota were analyzed based on the composition of the OTU sequence.
Statistical analysis
The result was analyzed using SPSS 25.0 software (SPSS Inc., Chicago, IL, USA), and assessed via independent sample T-test (Independent t-test) and the One-way ANOVA (Tukey’s tests). The significance level was set at p < 0.05, and the graphs were generated with GraphPad Prism 9.3 software (GraphPad Inc., La Jolla, CA, USA).
Results
B. longum BL-10 treatment improves the pathological changes in mice
Bifidobacterium treatment had no palpable toxic side effects on mice as the bodyweight of mice gradually increased and the difference among all groups was significant after intraperitoneal injection. The disease activity index (DAI) score and MPO activity suggested intestinal injury levels were significantly (p<0.05) increased in the LPS group, while those were significantly (p<0.05) decreased in the BL10 group. Figure 2D was the result of H&E staining to reflect the tissue damage in the jejunum, ileum, and colon, which reflected the pathological changes to some extent. In the control group, the intestinal villi were neatly arranged, epithelial cells were intact, and the tissue structure was clear, without edema and inflammation. In contrast, the tissue in the LPS group had obvious edema, intestinal wall congestion, shortened intestinal villi, loss of glands, and infiltration of inflammatory cells. Treatment with Bifidobacterium recovered the intestinal injury compared to the LPS group, including improving the morphological structure of the intestine and reducing the inflammation level in mice, with B. longum BL-10 being the most significant. Meanwhile, the histological score of mice was investigated, and the significant increase was in accord with the H&E staining (Figure 2D).
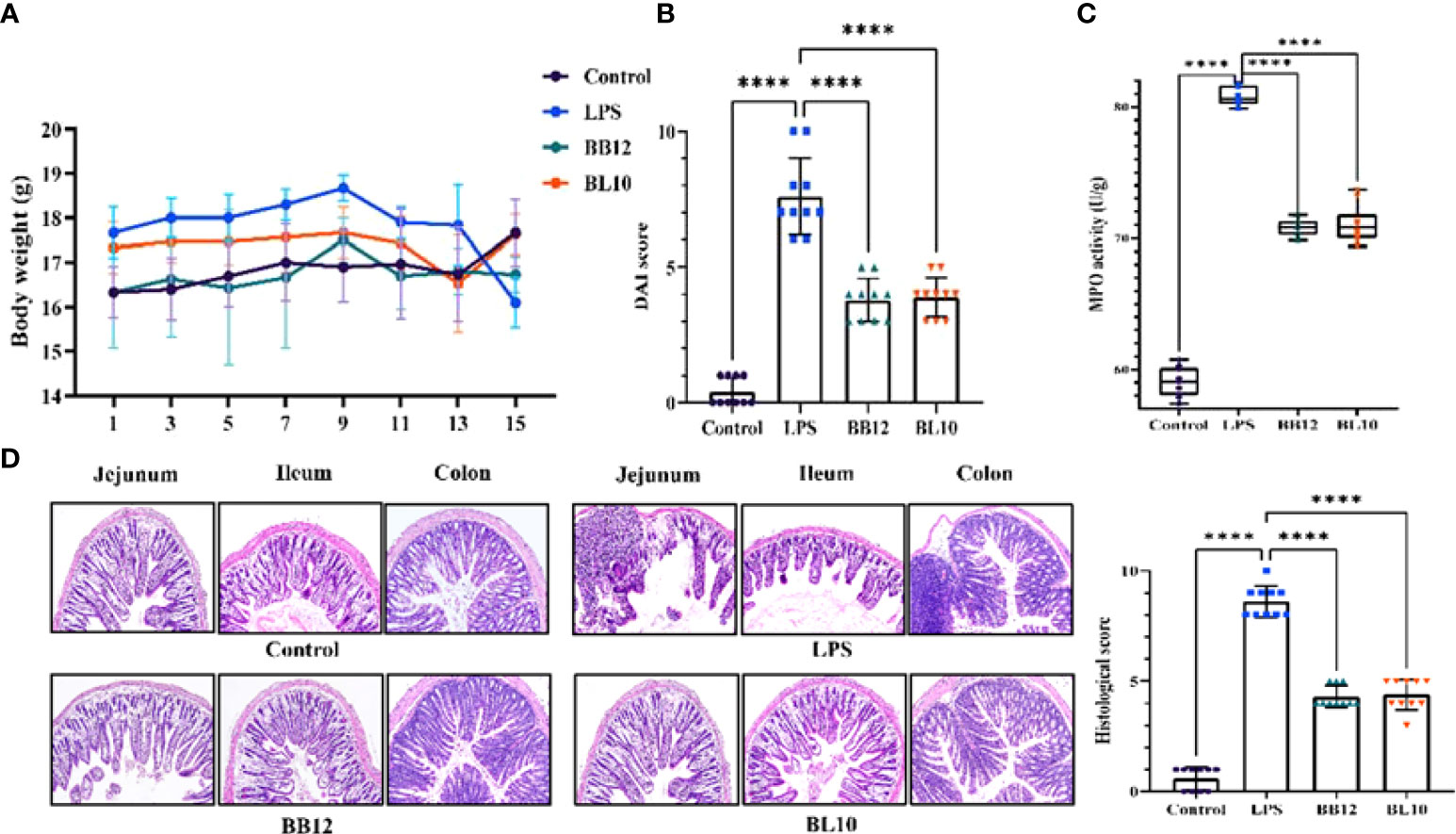
Figure 2 Effect of B longum BL-10 on LPS-induced intestinal immune injury. (A) Body weight; (B) DAI score; (C) MPO activity; (D) H&E staining (magnification × 200) and colon histological score. All data are expressed as mean ± SD (n=10). Values with a different superscript letter (****) were significantly different at p< 0.0001.
B. longum BL-10 treatment recovers the barrier function recover in mice
The AB-PAS staining was done to clearly identify the intestinal goblet cells and mucin production, and the intestinal Chiu’s score showed how badly the gut barrier had been damaged. In Figure 3A, there is a clear trend of increase (p<0.05) in Chiu’s score and a sharp decrease in mucin secretion of the LPS group compared with the control group. Further indicators of intestinal barrier function, including TJ protein (Claudin1, Occludin, and ZO-1) and mucin 2 (Muc 2), were substantially reduced (p<0.05) in the LPS group, hinting barrier function injury (Figure 3B). In contrast, the Bifidobacterium groups increased the expression of mRNA in each indicator, and B. longum BL-10 was preferable to increase expression levels of Claudin1 and Occludin (p<0.05). Overall, these results indicated that B. longum BL-10 effectively alleviated the reduced intestinal barrier function caused by LPS.
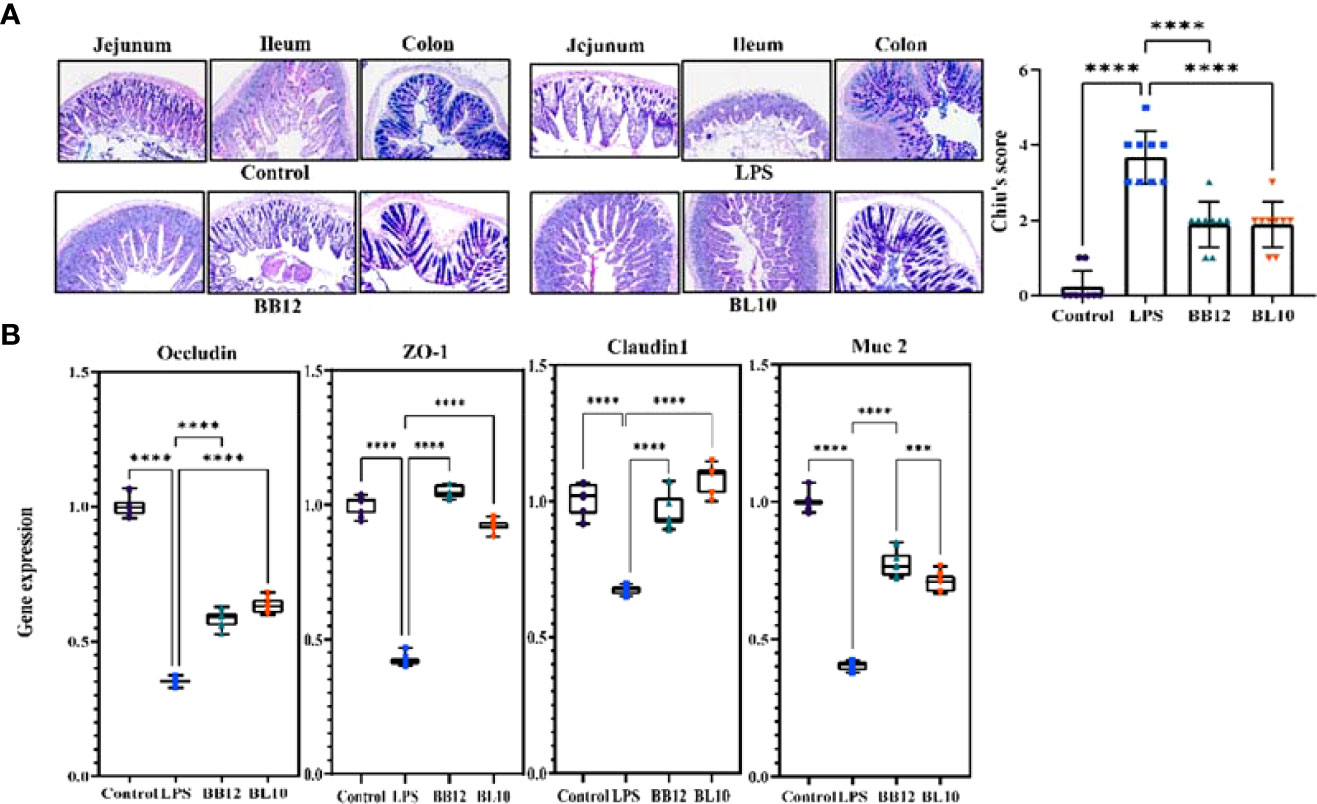
Figure 3 Effect of B longum BL-10 on LPS-induced intestinal barrier protection. (A) AB-PAS staining (magnification × 200); (B) Tight junction protein mRNA expression. All data are expressed as mean ± SD (n=10). Values with a different superscript letter (*** and ****) were significantly different at p< 0.001 and p< 0.0001.
B. longum BL-10 treatment increases the IgA plasma cells score in the ileum
Activated B cells in the lamina propria develop into IgA plasma cells, which release sIgA to boost the antibody response. After intraperitoneal injection of LPS, IgA plasma cells were substantially reduced (p<0.05) in the LPS group, indicating that LPS inhibited the cell differentiation of B cells to IgA plasma cells (Figure 4A). The scores of IgA plasma cells in the Bifidobacterium groups were significantly increased (p<0.05) compared with the LPS group, and B. longum BL-10 preferably increased it. Moreover, as sIgA was an important secretory immunoglobulin secreted by IgA plasma cells, its level further reflected the intestinal mucosal immunity (Figure 4B). Compared with the LPS group, Bifidobacterium, including B. animalis subsp. lactis BB-12 and B. longum BL-10 markedly increased (p<0.05) the sIgA level, especially in the BL10 group. Our findings showed that B. longum BL-10 might enhance gut immunity by promoting IgA plasma cell proliferation and differentiation.
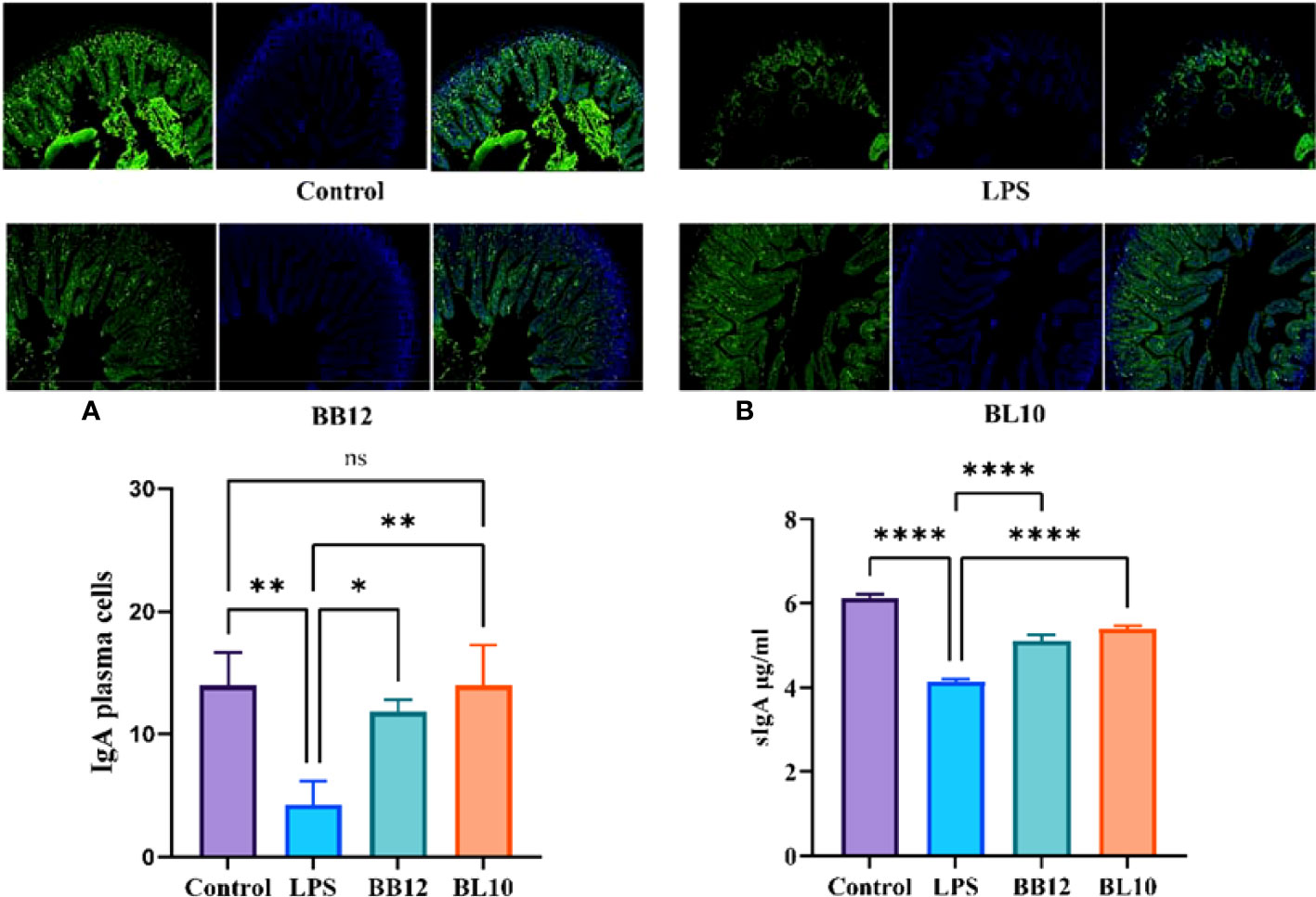
Figure 4 Effect of B longum BL-10 on ileal IgA plasma cells. (A) IgA plasma cells; (B) sIgA level. All data are expressed as mean ± SD (n=3). Values with a different superscript letter (*, **, **** and ns) were significantly different at p<0.05, p<0.01, p<0.0001 and p>0.05.
B. longum BL-10 treatment increases immune cells score in the ileum
T cells divided into CD4+ and CD8+ T cells were the main immunocyte of the immune response and immune regulation. In this experiment, immunosuppression did not influence the CD8+ T cell population since B. longum BL-10 treatment had comparable CD8+ T cell counts to the control and LPS groups (Figure 5B), and there were no significant changes (p>0.05) in any of the groups. Nevertheless, compared with the Control group, the number of CD4+ T cells in the LPS group was significantly decreased (p<0.05). After intragastric injection of Bifidobacterium, the CD4+ T cells count increased significantly (p<0.05) in the BB12 and BL10 groups, with the BL10 group having the greatest potential to boost CD4+ T cells (Figure 5A). Moreover, the intensity of immunity of the intestinal mucosa can also be reflected in the number of DC cells. As shown in Figure 5C, compared with the Control group, the number of DC cells in the LPS group was slightly reduced. However, compared to the LPS group, the Bifidobacterium treatment groups significantly increased (p<0.05) the number of DC cells, with the best results for the BL10 group (p<0.05). These results showed that B. longum BL-10 improved intestinal immunity by increasing the number of immunocytes, including CD4+ T cells and DC cells.
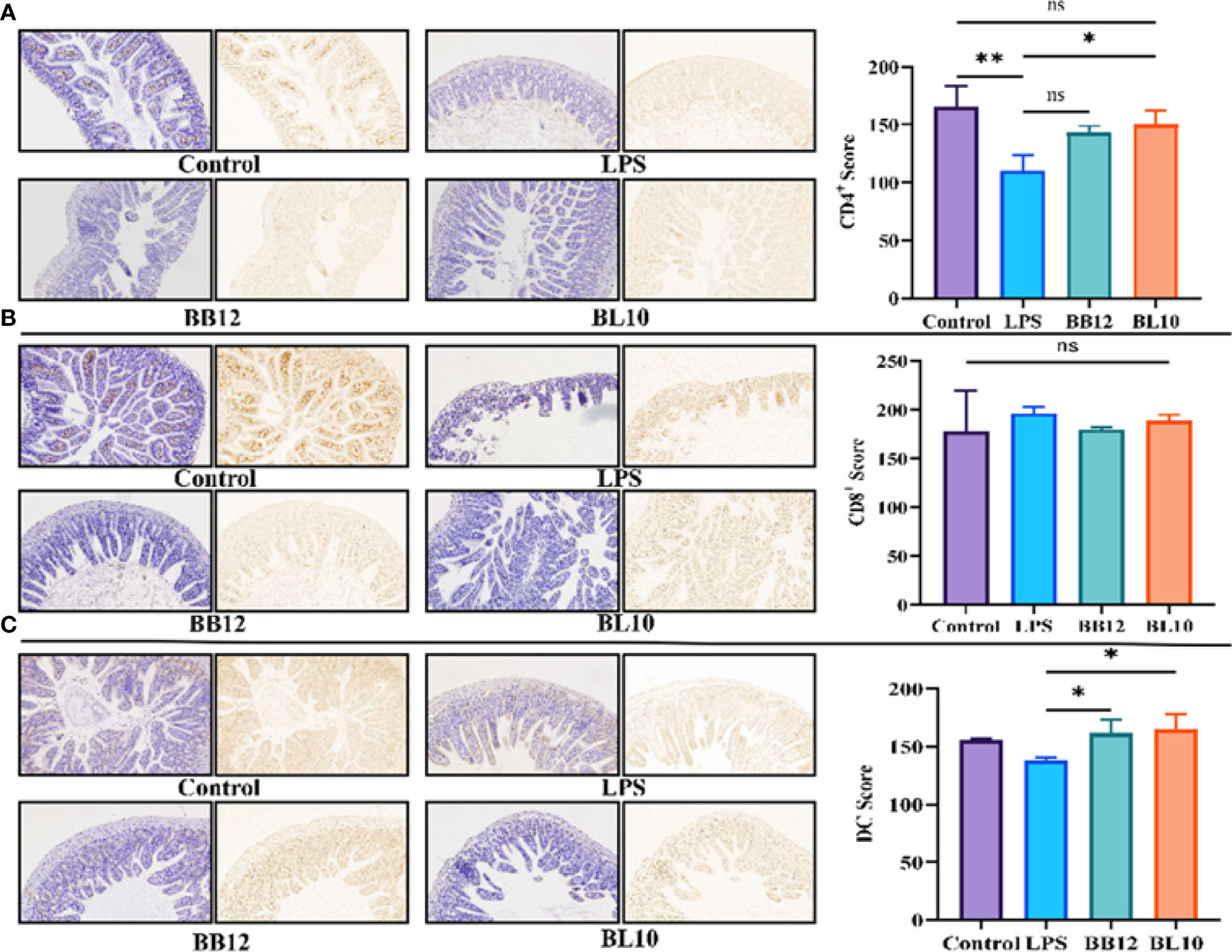
Figure 5 Effect of B longum BL-10 on ileal DCs, CD4+ and CD8+ T cells. (A) CD4+ T cells; (B) CD8+ T cells; (C) DC cells. All data are expressed as mean ± SD (n=3). Values with a different superscript letter (*, ** and ns) were significantly different at p<0.05, p<0.01 and p>0.05.
B. longum BL-10 treatment adjusts cytokines secretion in the ileum
The cytokines, transmitting biological information between cells, were used to indicate intestinal mucosal immunity (Figure 6). B. longum BL-10 treatment significantly reduced (p<0.05) the levels of TNF-α, IFN-γ, and IL-2, which belonged to Th1 type dominant cytokines. Th2 type dominant cytokines, including IL-4, IL-6, and IL-10, were also altered in the experimental groups, where the proinflammatory cytokine IL-6 was significantly increased (p<0.05) and anti-inflammatory cytokines (IL-4 and IL-10) were significantly reduced (p<0.05) in the LPS group. B. longum BL-10 treatment substantially reversed adverse circumstances, especially in increasing IL-4 levels. Moreover, treatment with B. longum BL-10 significantly altered the cytokine profile in the ileum, namely the cytokines IL-17, IL-22, and IL-23, which were associated with Th17 and Treg cells’ immunological responses, respectively. After giving a gavage of B. longum BL-10, the levels of IL-17, IL-22, and IL-23 were evidently reduced (p<0.05) compared to the LPS group. These results suggested that B. longum BL-10 affected cytokine secretion on the injury of intestinal mucosal immuno-barrier function.
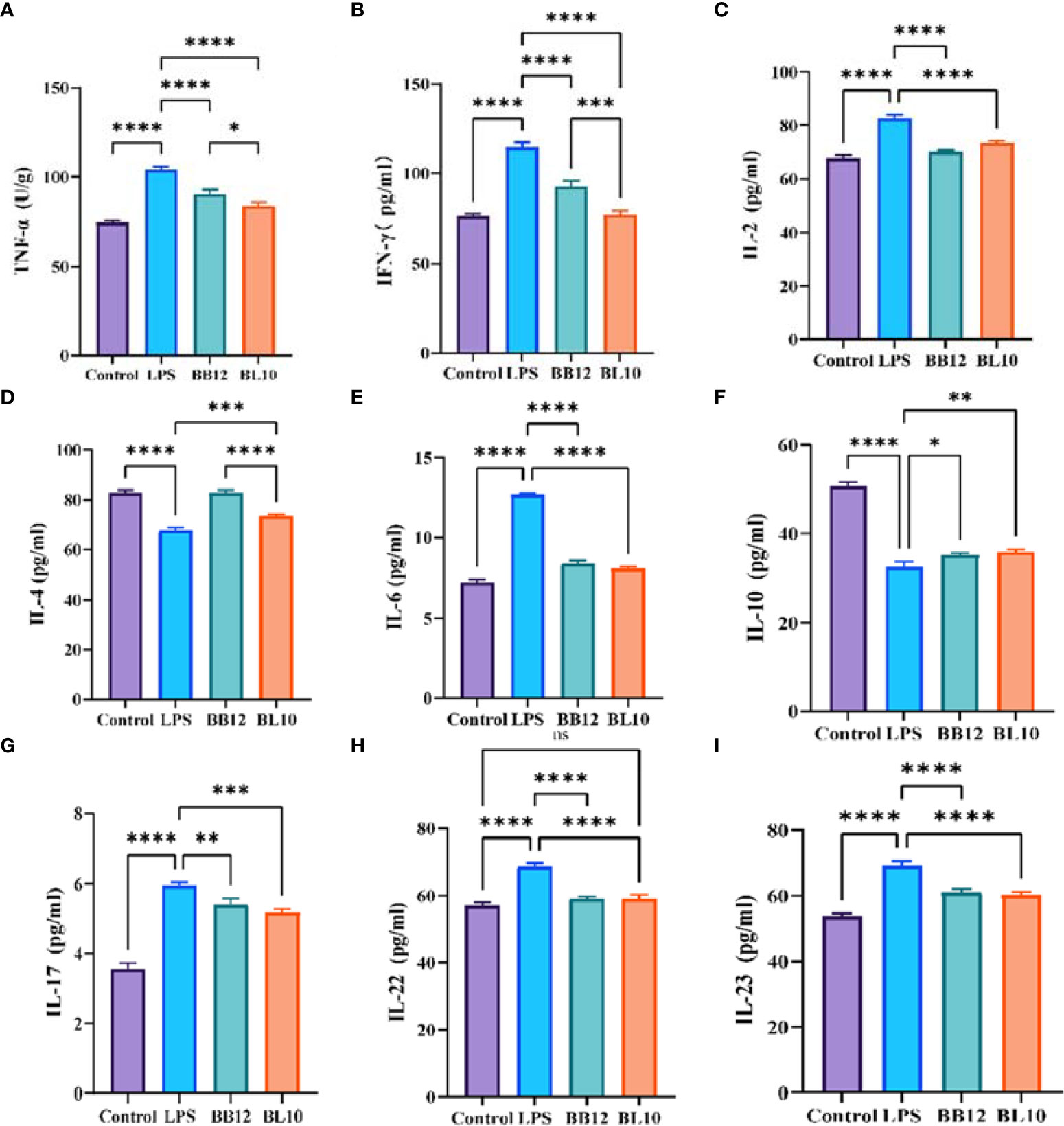
Figure 6 Effect of B longum BL-10 on ileal inflammatory factor. (A) TNF-α; (B) IFN-γ; (C) IL-2; (D) IL-4; (E) IL-6; (F) IL-10; (G) IL-17; (H) IL-22; (I) IL-23. All data are expressed as mean ± SD (n=3). Values with a different superscript letter (*, **, ***, **** and ns) were significantly different at p<0.05, p<0.01, p<0.001, p<0.0001 and p>0.05.
B. longum BL-10 treatment adjusts the key protein expression levels in the ileum
LPS activated intracellular NF-κB to produce large amounts of pro-inflammatory factors, triggering an inflammatory cascade in intestinal mucosal immunity. The protein expression levels of ileac TLR4, IκB, and NF-κB p65 in the LPS, KLDS BL-10, and BB12 groups were detected and the outcomes were shown in Figure 7. LPS resulted in a significant rise (p<0.05) of key proteins (TLR4, p-IκB, and NF-κB p65) in the NF-κB signaling pathway, while the IκB expression was significantly reduced (p<0.05) compared with the control group. Supplementation of B. longum BL-10 dramatically decreased (p<0.05) the expression of cytoplasmic TLR4, p-IκB in and nuclear NF-κB p65, and the cytoplasmic IκB and NF-κB p65 were significantly increased (p<0.05). Furthermore, the B. longum BL-10 had a more significant effect compared to the BB12 group. Moreover, the protein levels of specific transcription factors T-bet, GATA-3, RORγt, and Foxp3 derived from Th1 cells, Th2 cells, Th17 cells, and Treg cells were examined by Western blotting. The result showed that the levels of GATA-3 and Foxp3 in the Control and BL10 groups were significantly higher (p<0.05) than that of the LPS group, while the LPS group was significantly increased (p<0.05) the T-bet and RORγt levels compared with the control group. Additionally, the T-bet/GATA-3 ratio in the BL10 group was declined by upregulated transcriptional activity of Th2 cells and downregulated the transcriptional activity of Th1 cells. The outcomes also manifested that B. longum BL-10 downregulated the ratio of RORγt/Foxp3 to recover the balance of Th17 and Treg cells. To summarize, B. longum BL-10 could prevent the injury of intestinal mucosal immunity via regulating the balance of immunocytes and inhibiting inflammation.
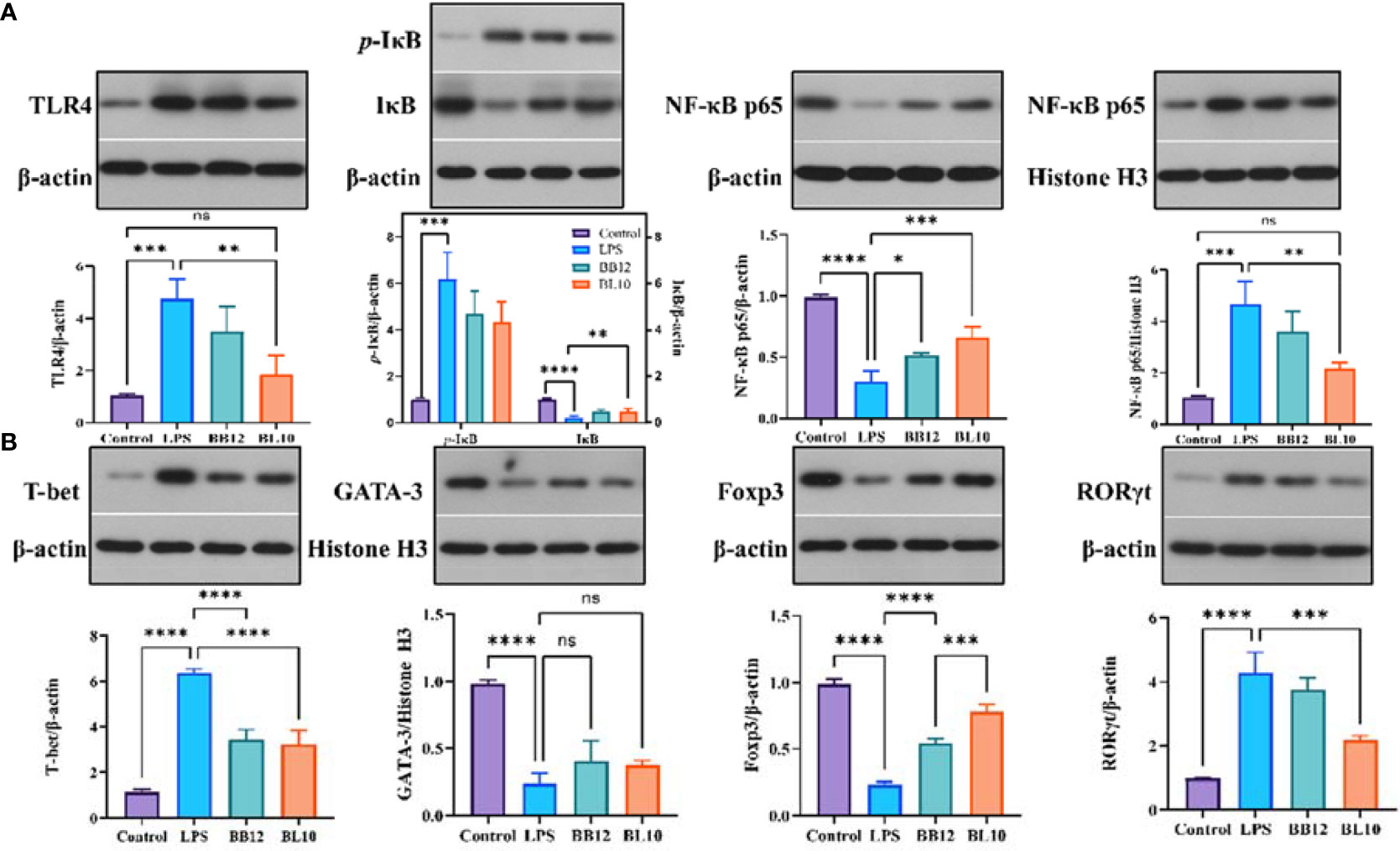
Figure 7 Effect of B. longum BL-10 on gut microbiota composition. (A) PCA; (B) Gut microbial changes at the phylum level; (C) Gut microbial changes at the genus level; (D) Prediction of the gut microbiota function; (E) Correlation analysis about gut microbial alteration. All data are expressed as mean ± SD (n=3). Values with a different superscript letter (*, **, ***, **** and ns) were significantly different at p<0.05, p<0.01, p<0.001, p<0.0001 and p>0.05.
B. longum BL-10 treatment recovers gut microbiota imbalance
PCA denoted the discrepancy in gut microbial composition between all the groups (Figure 8A), and the composition in the BL10 group was similar to the Control group. Three experimental groups were divided into different clusters by PC1 (53.2%) and PC2 (26.2%) influence factors, suggesting the differences in gut microbiota. Firmicutes, Bacteroidota, Proteobacteria, and Actinobacteriota were prime bacteria in the three groups (Figure 8B). Firmicutes (51.35%) and Bacteroidota (9.90%) declined but Proteobacteria (28.41%) increased in the LPS group versus the Control group (Firmicutes, Bacteroidota, and Proteobacteria were 72.09%, 16.26%, and 0.82%, respectively). The BL10 group increased Firmicutes (17.70%) and Bacteroidota (1.35%) and reduced Proteobacteria (22.32%), compared to the LPS group. As shown in Figure 8C, the histogram explained the relative abundance of microorganisms at the genus level. Compared with the Control group, Lachnospiraceae_NK4A136_group, Clostridia_UCG-014 and Muribaculaceae were reduced to 15.06%, 7.81%, and 6.22%, respectively, but Escherichia-Shigella was increased to 28.02%. B. longum BL-10 treatment recovered gut microbiota imbalance by reversing these changes in the gut. To infer the distinct gene functions between the LPS and BL10 groups, Figure 8D performed that carbohydrate metabolism Unclassified, cyanoamino acid metabolism, D-arginine and D-ornithine metabolism, transporters, and ABC transporters were higher in the BL10 group than these in the LPS group, but lipopolysaccharide biosynthesis was lower. Based on the correlation analysis (Figure 8E), the intestinal injury was positively correlated with Lachnospiraceae_NK4A136_group, Clostridia_UCG-014, and Muribaculaceae, but it was negatively correlated with Escherichia-Shigella.
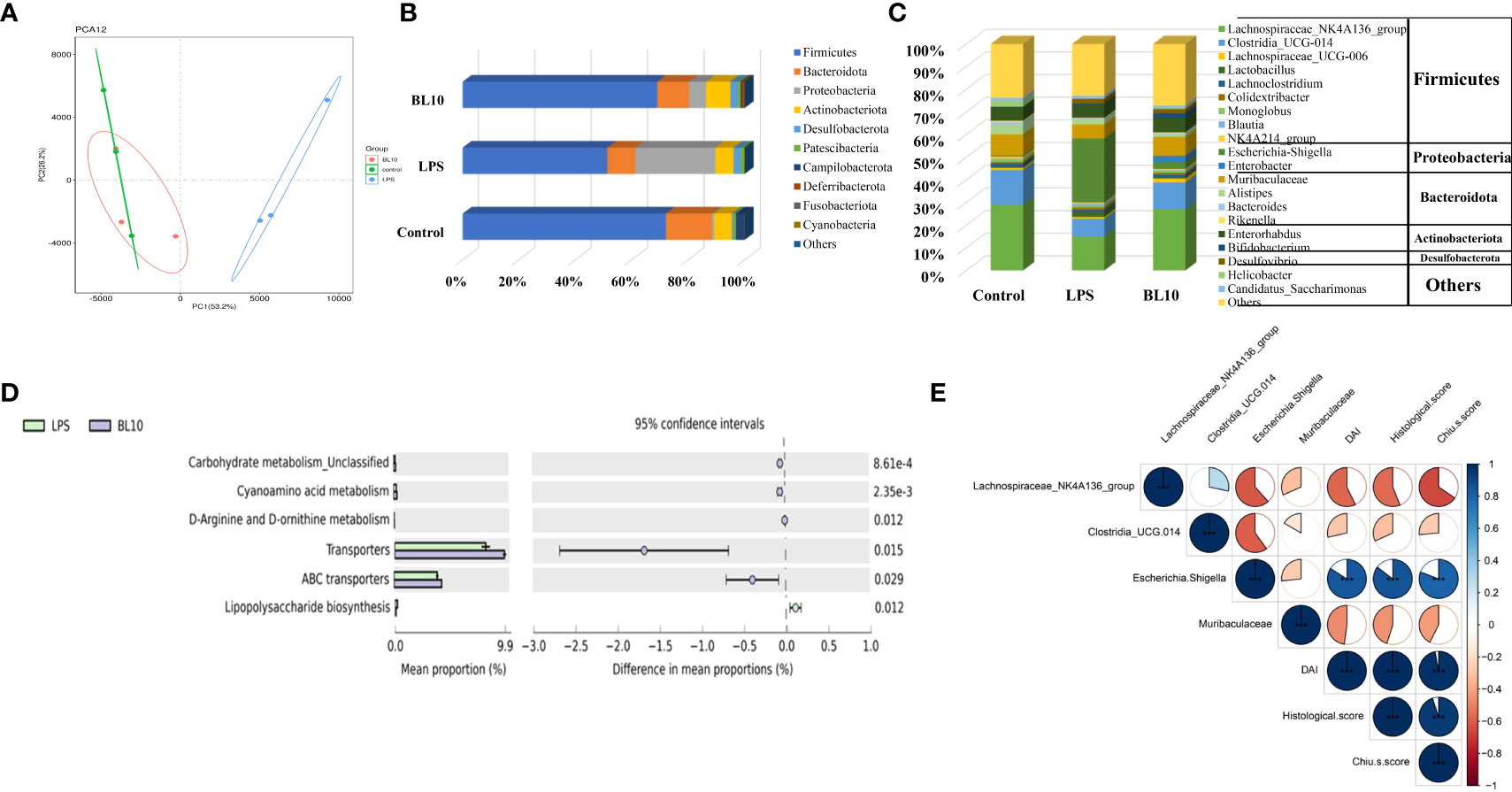
Figure 8 Effect of B longum BL-10 on NF-κB signaling pathway and immune key protein. (A) NF-κB signaling pathway; (B) Immune key protein. All data are expressed as mean ± SD (n=3). Values with a different superscript letter (*, **, ***, **** and ns) were significantly different at p<0.05, p<0.01, p<0.001, p<0.0001 and p>0.05.
Discussion
In the medical area, Bifidobacterium is frequently utilized as a micro-ecological agent to control micro-ecological illnesses and preserve micro-ecological equilibrium. Meanwhile, as a physiological probiotic, it has various functions including biological barrier, anti-tumor, immune enhancement, and improvement of the gastrointestinal tract. Recent evidence showed that Bifidobacterium could be in intensive communication with the epithelial cells of the intestinal mucosa, forming a spatial barrier that protects the cells from colonization by pathogenic bacteria (26). Therefore, in this research by establishing an LPS-induced model of impaired intestinal mucosal immunity in mice, we investigated the enhancement of intestinal mucosal immunity by B. longum and its immunomodulatory mechanism.
In terms of the basic structural morphology of the intestine, we used H&E staining to clearly view the histomorphology, and it was observed that LPS extremely influenced the morphological integrity and growth of intestinal mucosa, which was consistent with the previous study (27). In the LPS group, the intestinal epithelium appeared anomalous, with an abnormal inflammatory cell infiltration and edema pattern. On the contrary, the abnormal pattern that disappeared in the Bifidobacterium treatment group was no significant congestion of the intestinal epithelium and occasionally congested, which was consistent with Cui et al. (28). We also quantified intestinal damage by DAI and histological scores. According to the aforementioned findings, Bifidobacterium may significantly lessen intestinal damage brought on by LPS, in particular in the BL-10 group. A survey by Xiang et al. discovered that Bifidobacterium could reduce the LPS-induced increased intestinal permeability and damage to intestinal cells (29), which was identical to our findings.
The intestinal goblet cells on the surface of the mucosa can generate mucus, which represents the main barrier limiting the contact between the host and the commensal flora and preventing microbial translocation (30). Hence, genes associated with mucus production and TJ protein expression play a critical role in gut-associated infections (31). Simultaneously, Chiu’s score and AB-PAS staining indicated alterations in the intestine (32). Elevated intestinal mucosal permeability was manifested by elevated serum D-Lac levels (33), which was also expressed in our results. The serum D-Lac content was substantially increased after 6 hours of intraperitoneal LPS injection, while it was significantly decreased in the Bifidobacterium treatment groups, particularly in the BL10 group. In the result of Chiu’s score, the B. longum BL-10 decreased with the greatest difference compared to the LPS group. Moreover, the formation of functional TJ proteins, including Claudin1, Occludin, and ZO-1, is critical for the maintenance of gut permeability and intestinal barrier function (34). In Figure 2, there was a clear trend of an increase in all the TJ proteins mRNA expression of the BL10 group compared with the LPS group. The research of Xiang et al. also indicated that Bifidobacterium pretreatment up-regulated occludin and ZO-1 expression to reduce intestinal epithelial damage, thereby enhancing intestinal barrier function. The mucus layer, which comprises mucin and trefoil factor that obstructs the pathogen colonization in the gut, is an imperative line of defense for the intestinal barrier (29, 35). The goblet-cell-specific mucin Muc 2 expression can also alter epithelial protection (36). Figure 2 showed the Muc 2 of Bifidobacterium was significantly increased, which corresponded with Shashank et al. discovering higher Muc-2 mRNA expression to prevent mucosal damage (34). This study has shown that the B.longum BL-10 can prevent LPS damage to tight junction proteins, mucins, and goblet cells to protect the intestine’s immune function.
The immune system of the intestine consists mainly of CD4+ T cells, CD8+ T cells, DC cells, IgA plasma cells, etc., including the products they synthesize and secrete, such as cytokines (37). Bifidobacterium can modulate specific immune cells to enhance intestinal mucosal immunity (38). The findings indicated that LPS reduced the number of CD4+ T cells and CD8+ T cells in the intestine, with a more pronounced reduction in the number of CD4+ T cells. The analogical conclusions were obtained from the experiments of Zhu et al. (39). The number of CD4+ T cells was significantly increased in the B.longum BL-10 treatment group compared to the LPS group, and increased immune cells indicated enhanced immunity in mice. A previous study by Jiang et al. demonstrated that a decrease in the CD4+/CD8+ T cells ratio led to intestinal dysfunction (40). Our research indicated that B. longum BL-10 substantially raised the cell ratio, which demonstrated that it enhanced intestinal mucosal immunity. Additionally, in the number of IgA plasma cells study results, there was a clear trend of increase in the BL10 group compared with the BB12 group, and it is consistent with the discovery of Xie et al. (25). DCs are specialized antigen-presenting cells essential for the regulation of T cell-associated immune responses (41). As with other immune cells, the number of DC cells was significantly higher in the bifidobacteria intervention than in the LPS group. Therefore, B.longum BL-10 increased the number of immune cells in the intestine, including CD4+T cells, CD8+T cells, IgA plasma cells, and DC cells, to enhance intestinal mucosal immunity.
It is well known that Th1 and Th2 are the two subpopulations of activated CD4+ T cells’ differentiation (42). According to cytokine secretion and immune function differences, Th1 cells release the cytokines IL-2, IFN-γ, and TNF-α, which are mostly engaged in cell-mediated immunity, whereas Th2 cells secrete the cytokines IL-4, IL-6, and IL-10, which are primarily involved in humoral immunity (43). To maintain normal immune function, Th1 and Th2 should function in a dynamic balance in the body (44). Noritoshi et al. showed that B. longum improved the Th1/Th2 balance to enhance immunity in mice (45). The present experiment results showed that Th1-mediated cytokines were significantly adjusted compared with the LPS group after the interaction of B. longum BL10, especially in TNF-α and IFN-γ. In contrast, Th2-mediated cytokines including IL-4, IL-6, and IL-10 were increased, which is similar to Xie et al’s results (25). Moreover, in the reports on reducing allergic inflammation of β-lactoglobulin, B. longum increased Th1 cytokines and decreased Th2 cytokine production in mice. We deduced the results might be associated with the mice models and different immunoreactions. Th17 is a novel subpopulation of T-cell differentiation that has a reciprocal relationship with Th1, Th2, and Treg cells (46). Therefore, it has been suggested that there may be a Th1/Th2/Th17/Treg balance in the immune-inflammatory response (46). IL-6 and IL-17 are critical cytokines that induce differentiation of CD4+ T cells to Th17 cells, and IL-23 maintains stable Th17 differentiation and maturation in the late stages of differentiation (47). The results of the experiment showed an increased level of IL-17, IL-22, and IL-23 after treatment with B.longum BL-10 (48). These results indicated that B.longum BL-10 maintained the normal intestinal immune function by stimulating the secretion of cytokines and regulating the Th1/Th2 and Th17/Treg balance. T-bet, GATA-3, RORγt, and Foxp3 are key regulatory proteins for Thl, Th2, Thl7, and Treg cell differentiation respectively. The ratio of T-bet/GATA-3 and RORγt/Foxp3 is also used to reflect the Th1/Th2 and Th17/Treg balance (49). Western blot results showed that the T-bet/GATA-3 ratio of the B. longum BL-10 group was significantly lower than that of the LPS group. The ratio of RORγt/Foxp3 was also significantly reduced in the BL10 group compared to the LPS group. Our study clearly demonstrated that B. longum BL-10 regulated the body’s immune function by regulating the Th1/Th2 and Th17/Treg balance, which showed a greater impact on the Th1/Th2 balance.
An important factor in the damage caused to the intestinal mucosa by LPS is TLR4 activation (50). It has been shown that LPS damages intestinal epithelial cells directly in the intestinal mucosa and influences the expression of intestinal tight junction proteins via the TLR4-mediated signaling pathway (51). Thus, activating intracellular NF-κB produces large amounts of pro-inflammatory factors, triggers an inflammatory cascade in vivo (52), and contcontinuesexacerbate intestinal damage (53). In Figure 7, there was a significant trend of increase in the protein expression of TLR4 and NF-κB p65 in the LPS group, which was consistent with Li et al. (54). However, the expressions in the B.longum BL-10 group were significantly reduced, indicating reduced levels of inflammation in mice. This result was also verified by the conclusions of Li et al. (54). All of the analyses indicated that the B.longum BL-10 group was effective at inhibiting the activation of the NF-κB signaling pathway.
The dysbiosis in gut microbiota has been recently found to be associated with intestinal injury pathogenesis (55). The changes in the gut microbiota and increase of harmful metabolites (LPS) promote intestinal injury exacerbation (56). In this study, the relative abundance of Escherichia-Shigella was significantly increased in the LPS-induced intestinal injury mice, and pretreatment with B. longum BL-10 alleviated the liver injury in mice, as indicated by reducing the Escherichia-Shigella level. Moreover, B. longum BL-10 had preferable to increase Lachnospiraceae_NK4A136_group and Clostridia_UCG-014, suggesting probiotics mitigated intestinal injury by inhibiting pernicious bacteria. Therefore, the dysbiosis in gut microbiota was prospective related to the pathogenesis of LPS-induced intestinal injury, and B. longum BL-10 treatment relieved the gut dysbiosis and shifted it to a beneficial profile.
Conclusion
The results of the current study showed that B. longum BL-10 might reduce the gut microbial imbalances and immunological damage caused by LPS in mice. Evidently, pretreatment with B. longum BL-10 increased the relative proportions of the Clostridia UCG-014 and Lachnospiraceae NK4A136 group while decreasing the proportions of Escherichia-Shigella. As seen by lower levels of the intestinal pro-inflammatory cytokines TNF-, IFN-γ, and IL-2 and increased production of the anti-inflammatory cytokines IL-4 and IL-10, it also modulated the TLR4/NF-B signaling pathways to alleviate the intestinal mucosal immune damage. In the end, intestinal immunity was improved by decreasing intestinal mucosal damage, increasing the number of immune cells, and modifying the immunogenic cell ratio. Therefore, the current study offered a functionally thorough evaluation of B. longum BL-10 as a preventative for intestinal mucosal immune damage.
Data availability statement
The original contributions presented in the study are included in the article/Supplementary Material. Further inquiries can be directed to the corresponding authors.
Ethics statement
The animal study was reviewed and approved by the Animal Ethics Committee of NEAU (ethic approval code: NEAUEC20210475).
Author contributions
JD: Conceptualization, Methodology, Software, Writing- Reviewing and Editing. LP: Data curation, Writing- Original draft preparation. TC: Data curation, Writing- Original draft preparation. LS: Visualization, Investigation. DL: Visualization, Investigation. BL: Supervision. SW (Corresponding Author): Supervision. GH (Corresponding Author): Supervision. All authors contributed to the article and approved the submitted version.
Funding
Present research work was financially supported by the Natural Science Foundation of Heilongjiang Province (YQ2020C013), Young Elite Scientist Sponsorship Program by CAST (YESS20200271), the National Natural Science Foundation of China (32101919), Chinese nutrition society - Feihe physique nutrition and health research fund (CNS-Feihe2020A37) and the Open Project Program of China-Canada Joint Lab of Food Nutrition and Health, Beijing Technology and Business University (BTBU), Beijing 100048, China (KFKT-ZJ-2104).
Conflict of interest
The authors declare that the research was conducted in the absence of any commercial or financial relationships that could be construed as a potential conflict of interest.
Publisher’s note
All claims expressed in this article are solely those of the authors and do not necessarily represent those of their affiliated organizations, or those of the publisher, the editors and the reviewers. Any product that may be evaluated in this article, or claim that may be made by its manufacturer, is not guaranteed or endorsed by the publisher.
Supplementary material
The Supplementary Material for this article can be found online at: https://www.frontiersin.org/articles/10.3389/fimmu.2022.947755/full#supplementary-material
References
2. Ruiz L, Delgado S, Ruas-Madiedo P, Sánchez B, Margolles A. Bifidobacteria and their molecular communication with the immune system. Front Microbiol (2017) 8:2345. doi: 10.3389/fmicb.2017.02345
3. Hill CJ, Lynch DB, Murphy K, Ulaszewska M, Jeffery IB, O’Shea CA, et al. Evolution of gut microbiota composition from birth to 24 weeks in the INFANTMET cohort. Microbiome (2017) 5:1–18. doi: 10.1186/s40168-016-0213-y
5. Buettner M, Lochner M. Development and function of secondary and tertiary lymphoid organs in the small intestine and the colon. Front Immunol (2016) 7:342. doi: 10.3389/fimmu.2016.00342
6. Mörbe UM, Jørgensen PB, Fenton TM, von Burg N, Riis LB, Spencer J, et al. Human gut-associated lymphoid tissues (GALT); diversity, structure, and function. Mucosal Immunol (2021) 14:793–802. doi: 10.1038/s41385-021-00389-4
7. Nagashima K, Sawa S, Nitta T, Tsutsumi M, Okamura T, Penninger JM, et al. Identification of subepithelial mesenchymal cells that induce IgA and diversify gut microbiota. Nat Immunol (2017) 18:675–82. doi: 10.1038/ni.3732
8. Liévin-Le Moal V, Servin AL. The front line of enteric host defense against unwelcome intrusion of harmful microorganisms: mucins, antimicrobial peptides, and microbiota. Clin Microbiol Rev (2006) 19:315–37. doi: 10.1128/CMR.19.2.315-337.2006
9. McCracken VJ, Lorenz RG. The gastrointestinal ecosystem: A precarious alliance among epithelium, immunity, and microbiota: Microreview. Cell Microbiol (2001) 3:1–11. doi: 10.1046/j.1462-5822.2001.00090.x
10. Mantis NJ, Rol N, Corthésy B. Secretory IgA's complex roles in immunity and mucosal homeostasis in the gut. Mucosal Immunol (2011) 4:603–11. doi: 10.1038/mi.2011.41
11. Vaerman J-P, Langendries A, Pabst R, Rothkötter H-J. Contribution of serum IgA to intestinal lymph IgA, and vice versa, in minipigs. Veterinary Immunol immunopathol (1997) 58:301–8. doi: 10.1016/S0165-2427(97)00041-X
12. Sjögren YM, Tomicic S, Lundberg A, Böttcher MF, Björkstén B, Sverremark‐Ekström E, et al. Influence of early gut microbiota on the maturation of childhood mucosal and systemic immune responses: gut microbiota and immune responses. Clin Exp Allergy (2009) 39:1842–51. doi: 10.1111/j.1365-2222.2009.03326.x
13. Burcelin R. Gut microbiota and immune crosstalk in metabolic disease. Mol Metab (2016) 5:771–81. doi: 10.1016/j.molmet.2016.05.016
14. Kawahara T, Makizaki Y, Oikawa Y, Tanaka Y, Maeda A, Shimakawa M, et al. Oral administration of bifidobacterium bifidum G9-1 alleviates rotavirus gastroenteritis through regulation of intestinal homeostasis by inducing mucosal protective factors. PloS One (2017) 12:e0173979. doi: 10.1371/journal.pone.0173979
15. Yan S, Yang B, Ross RP, Stanton C, Zhang H, Zhao J, et al. Bifidobacterium longum subsp. longum YS108R fermented milk alleviates DSS induced colitis via anti-inflammation, mucosal barrier maintenance and gut microbiota modulation. J Funct Foods (2020) 73:104153. doi: 10.1016/j.jff.2020.104153
16. Dong P, Yang Y. & Wang, w.-p. the role of intestinal bifidobacteria on immune system development in young rats. Early Hum Dev (2010) 86:51–8. doi: 10.1016/j.earlhumdev.2010.01.002
17. O'Mahony C, Scully P, O'Mahony D, Murphy S, O'Brien F, Lyons A, et al. Commensal-induced regulatory T cells mediate protection against pathogen-stimulated NF-κB activation. PloS Pathog (2008) 4:e1000112. doi: 10.1371/journal.ppat.1000112
18. Sivan A, Corrales L, Hubert N, Williams JB, Aquino-Michaels K, Earley ZM, et al. Commensal bifidobacterium promotes antitumor immunity and facilitates anti–PD-L1 efficacy. Science (2015) 350:1084–9. doi: 10.1126/science.aac4255
19. Boirivant M, Strober W. The mechanism of action of probiotics. Curr Opin Gastroenterol (2007) 23:679–92. doi: 10.1097/MOG.0b013e3282f0cffc
20. Guan J, Liu F, Zhao S, Evivie SE, Shi J, Li N, et al. Effect of bifidobacterium longum subsp. longum on the proliferative and tight-junction activities of human fetal colon epithelial cells. J Funct Foods (2021) 86:104715. doi: 10.1016/j.jff.2021.104715
21. Muhammad I, Sun X, Wang H, Li W, Wang X, Cheng P, et al. Curcumin successfully inhibited the computationally identified CYP2A6 enzyme-mediated bioactivation of aflatoxin B1 in arbor acres broiler. Front Pharmacol (2017) 8:143. doi: 10.3389/fphar.2017.00143
22. Liu L, Lu Y, Kong H, Li L, Marshall C, Xiao M, et al. Aquaporin-4 deficiency exacerbates brain oxidative damage and memory deficits induced by long-term ovarian hormone deprivation and d-galactose injection. Int J Neuropsychopharmacol (2012) 15:55–68. doi: 10.1017/S1461145711000022
23. Varghese F, Bukhari AB, Malhotra R, De A. IHC profiler: an open source plugin for the quantitative evaluation and automated scoring of immunohistochemistry images of human tissue samples. PloS One (2014) 9:e96801. doi: 10.1371/journal.pone.0096801
24. de Moreno de LeBlanc A, Dogi CA, Galdeano CM, Carmuega E, Weill R, Perdigón G. Effect of the administration of a fermented milk containing lactobacillus casei DN-114001 on intestinal microbiota and gut associated immune cells of nursing mice and after weaning until immune maturity. BMC Immunol (2008) 9:1–12. doi: 10.1186/1471-2172-9-27
25. Xie J, Yu Q, Nie S, Fan S, Xiong T, Xie M. Effects of lactobacillus plantarum NCU116 on intestine mucosal immunity in immunosuppressed mice. J Agric Food Chem (2015) 63:10914–20. doi: 10.1021/acs.jafc.5b04757
26. Bron PA, Van Baarlen P, Kleerebezem M. Emerging molecular insights into the interaction between probiotics and the host intestinal mucosa. Nat Rev Microbiol (2012) 10:66–78. doi: 10.1038/nrmicro2690
27. Xie W, Song L, Wang X, Xu Y, Liu Z, Zhao D, et al. A bovine lactoferricin-lactoferrampin-encoding lactobacillus reuteri CO21 regulates the intestinal mucosal immunity and enhances the protection of piglets against enterotoxigenic escherichia coli K88 challenge. Gut Microbes (2021) 13:1956281. doi: 10.1080/19490976.2021.1956281
28. Cui Y, Wang Q, Sun R, Guo L, Wang M, Jia J, et al. Astragalus membranaceus (Fisch.) bunge repairs intestinal mucosal injury induced by LPS in mice. BMC Complementary Altern Med (2018) 18:1–6. doi: 10.1186/s12906-018-2298-2
29. Ling X, Linglong P, Weixia D, Hong W. Protective effects of bifidobacterium on intestinal barrier function in LPS-induced enterocyte barrier injury of caco-2 monolayers and in a rat NEC model. PloS One (2016) 11:e0161635. doi: 10.1371/journal.pone.0161635
30. McGuckin MA, Lindén SK, Sutton P, Florin TH. Mucin dynamics and enteric pathogens. Nat Rev Microbiol (2011) 9:265–78. doi: 10.1038/nrmicro2538
31. Singh S, Bhatia R, Khare P, Sharma S, Rajarammohan S, Bishnoi M, et al. Anti-inflammatory bifidobacterium strains prevent dextran sodium sulfate induced colitis and associated gut microbial dysbiosis in mice. Sci Rep (2020) 10:1–14. doi: 10.1038/s41598-020-75702-5
32. Wang F, Bao YF, Si JJ, Duan Y, Weng ZB, Shen XC, et al. The beneficial effects of a polysaccharide from moringa oleifera leaf on gut microecology in mice. J Medicinal Food (2019) 22:907–18. doi: 10.1089/jmf.2018.4382
33. Zhu R, Li L, Li M, Yu Z, Wang HH, Quan YN, et al. Effects of dietary glycinin on the growth performance, immunity, hepatopancreas and intestinal health of juvenile rhynchocypris lagowskii dybowski. Aquaculture (2021) 544:737030. doi: 10.1016/j.aquaculture.2021.737030
34. Furuse M, Hata M, Furuse K, Yoshida Y, Haratake A, Sugitani Y, et al. Claudin-based tight junctions are crucial for the mammalian epidermal barrier: a lesson from claudin-1–deficient mice. J Cell Biol (2002) 156:1099–111. doi: 10.1083/jcb.200110122
35. Perez-Lopez A, Behnsen J, Nuccio S-P, Raffatellu M. Mucosal immunity to pathogenic intestinal bacteria. Nat Rev Immunol (2016) 16:135–48. doi: 10.1038/nri.2015.17
36. Burger-van Paassen N, Vincent A, Puiman PJ, van der Sluis M, Bouma J, Boehm G, et al. The regulation of intestinal mucin MUC2 expression by short-chain fatty acids: implications for epithelial protection. Biochem J (2009) 420:211–9. doi: 10.1042/BJ20082222
37. Tsai Y-T, Cheng P-C, Pan T-M. The immunomodulatory effects of lactic acid bacteria for improving immune functions and benefits. Appl Microbiol Biotechnol (2012) 96:853–62. doi: 10.1007/s00253-012-4407-3
38. Schiavi E, Gleinser M, Molloy E, Groeger D, Frei R, Ferstl R, et al. The surface-associated exopolysaccharide of bifidobacterium longum 35624 plays an essential role in dampening host proinflammatory responses and repressing local TH17 responses. Appl Environ Microbiol (2016) 82:7185–96. doi: 10.1128/AEM.02238-16
39. Zhu H, Liu Y, Xie X, Huang J, Hou Y. Effect of l-arginine on intestinal mucosal immune barrier function in weaned pigs after escherichia coli LPS challenge. Innate Immun (2013) 19:242–52. doi: 10.1177/1753425912456223
40. Jiang W, Wu N, Wang X, Chi Y, Zhang Y, Qiu X, et al. Dysbiosis gut microbiota associated with inflammation and impaired mucosal immune function in intestine of humans with non-alcoholic fatty liver disease. Sci Rep (2015) 5:1–7. doi: 10.1038/srep08096
41. Liu K, Nussenzweig MC. Origin and development of dendritic cells. Immunol Rev (2010) 234:45–54. doi: 10.1111/j.0105-2896.2009.00879.x
42. McGuirk P, Mills KH. Pathogen-specific regulatory T cells provoke a shift in the Th1/Th2 paradigm in immunity to infectious diseases. Trends Immunol (2002) 23:450–5. doi: 10.1016/S1471-4906(02)02288-3
43. Kidd P. Th1/Th2 balance: the hypothesis, its limitations, and implications for health and disease. Altern Med Rev (2003) 8:223–46. doi: 10.1.1.333.4347&rep=rep1&type=pdf
44. Borchers AT, Keen CL, Gershwin ME. The influence of yogurt/Lactobacillus on the innate and acquired immune response. Clin Rev Allergy Immunol (2002) 22:207–30. doi: 10.1007/s12016-002-0009-7
45. Takahashi N, Kitazawa H, Iwabuchi N, Xiao JZ, Miyaji K, Iwatsuki K, et al. Oral administration of an immunostimulatory DNA sequence from bifidobacterium longum improves Th1/Th2 balance in a murine model. Bioscience biotech Biochem (2006) 70:2013–7. doi: 10.1271/bbb.60260
46. Liu H, Rohowsky-Kochan C. Regulation of IL-17 in human CCR6+ effector memory T cells. J Immunol (2008) 180:7948–57. doi: 10.4049/jimmunol.180.12.7948
47. Liu L-l, Qin Y, Cai JF, Wang HY, Tao JL, Li H, et al. Th17/Treg imbalance in adult patients with minimal change nephrotic syndrome. Clin Immunol (2011) 139:314–20. doi: 10.1016/j.clim.2011.02.018
48. Pandiyan P, Conti HR, Zheng L, Peterson AC, Mathern DR, Hernández-Santos N, et al. CD4+ CD25+ Foxp3+ regulatory T cells promote Th17 cells in vitro and enhance host resistance in mouse candida albicans Th17 cell infection model. Immunity (2011) 34:422–34. doi: 10.1016/j.immuni.2011.03.002
49. Bettelli E, Carrier Y, Gao W, Korn T, Strom TB, Oukka M, et al. Reciprocal developmental pathways for the generation of pathogenic effector TH17 and regulatory T cells. Nature (2006) 441:235–8. doi: 10.1038/nature04753
50. Guo S, Al-Sadi R, Said HM, Ma TY. Lipopolysaccharide causes an increase in intestinal tight junction permeability in vitro and in vivo by inducing enterocyte membrane expression and localization of TLR-4 and CD14. Am J Pathol (2013) 182:375–87. doi: 10.1016/j.ajpath.2012.10.014
51. Guo S, Nighot M, Al-Sadi R, Alhmoud T, Nighot P, Ma TY, et al. Lipopolysaccharide regulation of intestinal tight junction permeability is mediated by TLR4 signal transduction pathway activation of FAK and MyD88. J Immunol (2015) 195:4999–5010. doi: 10.4049/jimmunol.1402598
52. Cho S, Kim K, Kim H. Extracellular signal-regulated kinase induces phosphorylation of IκBα in helicobacter pylori-infected gastric epithelial AGS cells. Inflammopharmacology (2007) 15:26–30. doi: 10.1007/s10787-006-1547-z
53. Sharif O, Bolshakov VN, Raines S, Newham P, Perkins ND. Transcriptional profiling of the LPS induced NF-κB response in macrophages. BMC Immunol (2007) 8:1–17. doi: 10.1186/1471-2172-8-1
54. Li B, Tang M. Research progress of nanoparticle toxicity signaling pathway. Life Sci (2020) 263:118542. doi: 10.1016/j.lfs.2020.118542
55. Meng X, Li S, Li Y, Gan R-Y, Li H-B. Gut microbiota’s relationship with liver disease and role in hepatoprotection by dietary natural products and probiotics. Nutrients (2018) 10:1457. doi: 10.3390/nu10101457
Keywords: bifidobacterium longum, intestinal immunity, immunocyte, NF-κB pathway, Th1/Th2/Th17/Treg, gut microbiota
Citation: Dong J, Ping L, Cao T, Sun L, Liu D, Wang S, Huo G and Li B (2022) Immunomodulatory effects of the Bifidobacterium longum BL-10 on lipopolysaccharide-induced intestinal mucosal immune injury. Front. Immunol. 13:947755. doi: 10.3389/fimmu.2022.947755
Received: 19 May 2022; Accepted: 27 July 2022;
Published: 24 August 2022.
Edited by:
Wentao Yang, Jilin Agriculture University, ChinaReviewed by:
Cuiming Zhu, University of South China, ChinaHuaxi Yi, Ocean University of China, China
Zhenlong Wu, China Agricultural University, China
Copyright © 2022 Dong, Ping, Cao, Sun, Liu, Wang, Huo and Li. This is an open-access article distributed under the terms of the Creative Commons Attribution License (CC BY). The use, distribution or reproduction in other forums is permitted, provided the original author(s) and the copyright owner(s) are credited and that the original publication in this journal is cited, in accordance with accepted academic practice. No use, distribution or reproduction is permitted which does not comply with these terms.
*Correspondence: Song Wang, d3NfMTk4NEAxNjMuY29t; Guicheng Huo, Z3VpY2hlbmdodW9AMTI2LmNvbQ==; Bailiang Li, MTU4NDYwOTIzNjJAMTYzLmNvbQ==