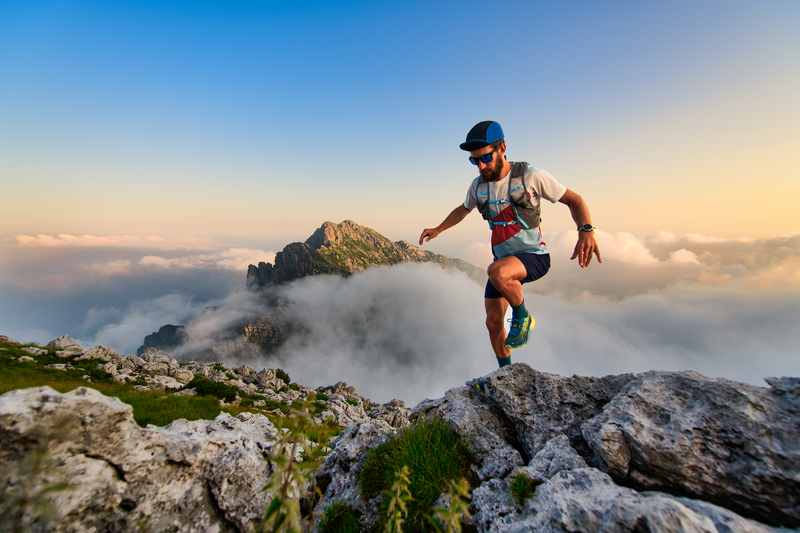
95% of researchers rate our articles as excellent or good
Learn more about the work of our research integrity team to safeguard the quality of each article we publish.
Find out more
REVIEW article
Front. Immunol. , 25 July 2022
Sec. Inflammation
Volume 13 - 2022 | https://doi.org/10.3389/fimmu.2022.941982
Micro ribonucleic acids (miRNAs), as a category of post-transcriptional gene inhibitors, have a wide range of biological functions, are involved in many pathological processes, and are attractive therapeutic targets. Considerable evidence in ophthalmology indicates that miRNAs play an important role in diabetic retinopathy (DR), especially in inflammation, oxidative stress, and neurodegeneration. Targeting specific miRNAs for the treatment of DR has attracted much attention. This is a review focusing on the pathophysiological roles of miRNAs in DR, diabetic macular edema, and proliferative DR complex multifactorial retinal diseases, with particular emphasis on how miRNAs regulate complex molecular pathways and underlying pathomechanisms. Moreover, the future development potential and application limitations of therapy that targets specific miRNAs for DR are discussed.
Micro ribonucleic acids (miRNAs), a family of post-transcriptional gene inhibitors commonly existing in animals, are widely involved in regulating gene expression in various environments (1). The advent of multicellular life was accompanied by new gene categories, including the most prevalent one: genes that encode miRNAs. Multiple articles on miRNAs have been published (about 136,000). These studies have determined that the genes encoding miRNAs can be involved in cell growth, apoptosis, fibrosis, hypertrophy, and senescence (2). Following the first demonstration of the role of miRNAs in chronic lymphocytic leukemia by Calin et al. in 2002 (3), several disease-related dysregulated miRNAs have been reported, such as those in cancer (4), diabetes (5), and cardiovascular diseases (6). To date, deciphering the exact nature of the molecular mechanisms associated with miRNAs in healthy and disease conditions remains challenging in related research. Recently, the efforts of some researchers on the role of miRNAs in diabetic retinopathy (DR) have achieved certain results (7). The main focus of this review is on the roles of miRNAs in DR, diabetic macular edema (DME), and proliferative DR (PDR) as well as their cellular and molecular mechanisms.
MicroRNAs are a category of short non-coding RNAs with a length of about 19 to 25 nucleotides. MicroRNA genes are transcribed by RNA polymerase II into single-stranded primary miRNA (pri-miRNA) with a length of more than 1 kb in the cell nucleus; then, they are processed and matured by a microprocessor complex composed of nuclear RNase III Drosha and cofactor DGCR8 (8). RNase III Drosha cleaves pri-miRNA and releases hairpin-like precursor miRNA (pre-miRNA) containing 65 bases. Pre-miRNA is transported to the cytoplasm, where the Dicer endonuclease cleaves it into 21 nucleotides of small double-stranded RNA near both ends of the ring (9). One strand of the obtained double-stranded RNA forms into a complex with a protein of GW182 (from the Argonaute [AGO] family) (10). This complex is called the miRNA-induced silencing complex (miRISC). In most cases, the nucleotides located at positions 2–7 in miRNA are in the “seed” region, which binds to the 3’ untranslated region nucleotides in messenger RNA (mRNA). The miRISC proteins take effect by recruiting factors that inhibit mRNA translation and promote mRNA deadenylation (11). Some miRNAs completely bind in the gene coding region, inducing the AGO protein to cleave mRNA (12).
Thanks to the development of miRNA sequencing technology, it has been found that each species in the animal kingdom has hundreds of annotated miRNA genes, and the number of miRNA genes recorded by humans is currently 556 (13). MicroRNAs can be divided into different families according to their mature sequences. MicroRNAs in a family can share the same sequence, but most miRNAs only share their seed sequences (14). About 300 miRNAs in humans belong to 177 different miRNA families (14). After finding evidence of the widespread existence of miRNAs in the entire animal kingdom, it was discovered that they guide many important processes related to cell growth, apoptosis, differentiation, metabolism, and immune response (15). During the occurrence and development of DR, miRNAs are involved in DR-related microvascularization, reverse dyslipidemia, and slowing DR progression (16). Moreover, they may be used in DR treatment strategies.
The traditional belief that miRNAs are cell-autonomous was overturned in 2007 by discovering that miRNAs can be secreted into the circulatory system (17). The application of circulating miRNA mapping in diagnosing human disease has become a field of interest and a candidate biomarker in obesity, type 2 diabetes, atherosclerosis, and other diseases (18). Table 1 summarizes the literature review on the expression of miRNAs in DR.
In the small-sample cohort study by Li et al., five miRNAs were found to be differentially expressed in the serum samples of the DR and non-DR (NDR) groups: the expression of miR-190a-5p was upregulated, and the expressions of miR-4448, miR-338-3p, miR-485-5p, and miR-9-5p were downregulated (19). According to another study on the expression of miR-20b and miR-17-3p in serum of the miR-17 family, the level in patients with DR was significantly lower than in healthy people (20). In a recent study involving 47 patients with type 2 diabetes, Tamir et al. found that 16 candidate miRNAs (miR-423, miR-486-3p, miR-320a-3p, miR-320p, miR-200b-3p, miR-221-3p, miR-146a-5p, miR-183-5p, miR-122-5p, miR-126-5p, miR-30p, miR-93-5p, miR-21, miR-27b-3p, let7f-5p, and miR-16-2-3p) changed in the serum of patients with diabetes (37).Other studies showed that the expression of miR-126 (21), miR-210 (22) and miR-27b (23) in the serum of DR patients was up-regulated.
Qing et al. validated the elevated expression levels of three miRNAs (miR-21, miR-181c, and miR-1179) in plasma and predicted their role in PDR (38). In a study by Jiang, it was found that the level of miR-21 increased in the plasma of patients with PDR (24). Some studies have shown that the level of miR-29b in the plasma of patients with DR was significantly lower than in patients with no DR (25), and the level of miR-93 was significantly higher in the DR group than in the NDR group (26).
Garcia et al. obtained early growth endothelial progenitor cells by culturing peripheral blood monocytes in vitro. They detected the expression of miR-221 in the peripheral blood mononuclear cells of 41 patients with type 1 diabetes mellitus (T1 DM) and DR and 35 patients with T1 DM without DR by reverse transcription-polymerase chain reaction and found that the expression of miR-221 in the DR group was significantly higher than in the NDR group (39).The study of Liu et al. showed that the expression of miR-216a in human retinal microvascular endothelial cells was down-regulated (27), while Han et al. found that the expression of miR-203a-3p (40) and miR-200b-3p (41) in retinal vascular endothelial cells of rats was also down-regulated.
Extracellular vesicles (EVs) larger than 200 nm are called “microvesicles,” which germinate outward from the plasma membrane. Smaller EVs can germinate outward through the plasma membrane to secrete exosomes, which can also be formed by fusing multivesicular bodies (MVBs) with the plasma membrane (42). The inward germination of late endosomes forms MVBs through the endosomal membrane to form intraluminal vesicles. The fusion of the MVBs with the plasma membrane will release the internal vesicles into the extracellular environment in the form of exosomes. In a recent study, the DR pattern of the miRNA map in circulating EVs was different from the healthy control group. It was found that the expressions of miR-21-3p, miR-17-5p, miR-106a-5p, and miR-21 were upregulated (43), and the expressions of miR-150-5p, miR-342-3p, and miR-155-5p were downregulated (28). MicroRNAs in EVs seem to be involved in the development of DR, and miR-150-5p, miR-21-3p, and miR-30b-5p extracted from circulating EVs are considered biomarkers of DR prognosis (44). Some studies showed that in the circulating EVS, the expression of miR-15a was up-regulated (29), while the expression of miR-20a-5p (30) and miR-222 (31) was down-regulated.
For ethical reasons, healthy people cannot be used as controls. The general practice is to use the vitreous humor obtained from non-diabetic patients undergoing vitrectomy of the idiopathic macular hole as controls, so there is not much information on expression profiling in the vitreous bodies of patients with DR. According to the research results of Friedrich et al., the expression levels of miR-20a-5p, miR-23b-3p, miR-142-3p, miR-185-5p, miR-223-3p, miR-362-5p, and miR-662 in the vitreous humor of patients with PDR were higher than the control group, while those of miR-199a-5p and miR-326 were significantly lower than the control group (32). Some studies have shown that the expression level of miR-21 in the vitreous humor of patients with PDR was increased, and the expression levels of miR-204 and let-7c were decreased (33). The expression levels of miR-15a, miR-320a, miR-320b, miR-93, miR-29a, and miR-423-5p were significantly increased in the vitreous humor of patients with PDR (45). A recent study showed that the expression level of let-7a in the vitreous humor of PDR patients was elevated (34), while the expression level of miR-16 (35) and miR-92 (36) decreased.
The retina is an important photosensitive neural tissue and plays a vital role in the central nervous system. It stems from a part of the central nervous system in the diencephalon, comprising the internal sensory retina and retinal pigment epithelium (46). The process in which oxidative stress, inflammation and metabolic disorder affect the structure and function of the central nervous system could cause irreversible impairment such as retinal degeneration and dysfunction. MiRNAs can be used as a gene regulator to regulate multiple biological processes such as neuronal function, innate immune response, cell proliferation and pluripotency (47). Highly expressed in retinas, it is a biomarker or therapeutic target of retinal diseases.
DR is the most common complication of diabetes, and also the disease affecting vision the most across the world. The abnormal change of retinal vasculature causes the reduction of blood supply to the retina, damage to the retinal microenvironment and retinal metabolic disorder, thus affecting the structure and function of the retina and leading to the loss of vision (48). The pathogenesis of DR is still unclear, so it is imperative to develop new diagnostic and therapeutic techniques (49). The expression of miRNAs in DR is discussed in Section 3, and the mechanism of action of MiRNAs in the pathological pathway of RD will be introduced in detail.
Proliferative vitreoretinopathy (PVR) is a complex disease, and results in severe loss of vision through forming a contractile retinal membrane (50). It is one of the main complications of rhegmatogenous retinal detachment surgery. So far, there has been no drug verified to treat or prevent PVR. Hiroki et al. found that the expression of miR-148a was up-regulated in vitreous humor of patients with retinal detachment, which was significantly associated with the expression level of inflammatory cytokine FGF-2. Besides, they found that miR-199a-5p was involved in the pathogenesis of PVR through inhibiting Caveolin-1 protein in retinal pigment epithelia (51).
Age-related macular degeneration (AMD) is a complex degenerative and progressive disease related to aging and oxidative stress (52), and it is characterized by the degeneration of retinal pigment epithelia between the photoreceptor and choroidal capillaries. A recent study showed that epigenetic mechanisms such as miRNAs regulation of gene expression were associated with the pathophysiology of AMD (53). The study of ElShelmani et al. showed that miR-19a, miR-126 and miR-410 played a role through regulating VEGF signaling, apoptosis and neurodegenerative pathways (54). Shahriari et al. found that hsa-let-7a-5p in retinal pigment epithelia inhibited neural differentiation and promoted the differentiation of retina pigment epithelia by acting on MITF (55).
Glaucoma is a neurodegenerative retinal disease that is characterized by progressive and gradual loss of retinal ganglion cells (RGCs) and the axons, also called glaucoma neurodegeneration. It can continue and progress, thus leading to complete loss of vision (56). In the chronic glaucoma mouse model, investigators found that the expression of miR-149 was up-regulated in RGCs, while the silence of miR-149 promoted RGCs activity by increasing the activation of PI3K/Akt signaling pathway (57). Peng et al. found that the down-regulation of miR-200a in retinal ganglion cells of mice with glaucoma could increase the apoptosis of ganglion cells and the inactivation of Müller cells through enhancing the activation of MAPK mitogen-activated protein kinase signaling pathway (58).
Diabetic retinopathy is characterized by chronic inflammation, oxidative stress, neurodegeneration, vascular leakage, neovascularization, and fibrosis. This section discusses the molecular interactions and signaling pathways during the regulation of miRNAs on the pathological processes of DR, including inflammation, oxidative stress, and neurodegeneration. The cellular and molecular mechanisms of miRNAs involved in these pathological processes come from cell culture and experimental animal models and are valuable for finding potential therapeutic targets for DR. Table 2 summarizes the research progress of miRNAs and their target molecules in the pathogenesis of DR (Figure 1).
Figure 1 Mechanism of action of MiRNAs in DR inflammation, oxidative stress and neurodegeneration. (A) Mechanism of action of MiRNAs in DR inflammation. The targeting of miR-30a to CCL2 in retina enhances the phagocytosis of microglia in the retina. MiR-15a and miR-16 can inhibit the proinflammatory signaling pathway and stasis of retinal white blood cells in DR by inhibiting IL-1β, CD45, TNF-α and NF-κB. MiR-27a involves in the inflammatory response of DR by targeting TLR4 to stimulate the production of TNF-α and IL-1β as well as pro-inflammatory proteins including cyclooxygenase-2 and inducible nitric oxide synthase. The up-regulation of MiR-455-5p significantly attenuated the HG-stimulated inflammatory response by inhibiting the release of inflammatory cytokines, such as IL-6, IL-1β and TNF-α in ARPE-19 cells. (B) Mechanism of action of MiRNAs in the oxidative stress of DR. The overexpression of MiRNA-145 can reduce the levels of ROS and malondialdehyde (MDA) in cells, and increase the activity of superoxide dismutase, thus reducing hyperglycemia-induced oxidative stress and retinal endothelial cell apoptosis. MiRNA-383 can promote the production of ROS and induce apoptosis by increasing the expression of peroxidase redoxin 3 (PRDX3) and Bax/Bcl2. MiR-183 activates PI3K/Akt/VEGF signaling pathway, and increases CD34, endothelial nitric oxide synthase (eNOS) and ROS. MiR-27 inhibits Nox 2 signaling pathway by down-regulating P13K/AKT/mTOR, thus reducing the production of ROS. (C) Mechanism of action of MiRNAs in the neural degeneration of DR. MiR-495 affects the transmission of PTEN/Akt signal by targeting Notch1 and aggravates the damage to ganglion cells. MiR-34a promotes mitochondrial dysfunction and retinal microvascular endothelial cell senescence by inhibiting SIRT1/PGC-1α axis and mitochondrial antioxidants TrxR2 and SOD2. MiR-451a protects mitochondrial function through down-regulation and activation of transcription factor 2 (ATF2) and its downstream target genes CyclinA1, CyclinD1 and MMP2. The up-regulation of miR-486-3P can protect Müller cells in the HG state from oxidative stress, inflammation and apoptosis by inhibiting TLR4/NF-kB axis.
MiRNAs can interact with a variety of inflammatory mediators, which plays an important role in the diversity of signal reactions in the process of DR development. MCP-1, also known as chemokine ligand 2 (CCL2), from the CC family, is a classical chemokine from the CC family. It leads to the progression of many diseases through the main mechanism of the migration and infiltration of inflammatory cells (such as monocytes, macrophages and other cytokines) at the site of inflammation. Murinello et al. showed that the targeting of miR-30a to CCL2 in retina enhanced the phagocytosis of microglia in the retina (69).
The key inflammatory response process is the adhesion and migration of peripheral blood leukocytes to vascular endothelial cells. Leukocyte retention may lead to retinal vascular leakage, enhancing retinal pathogenic effects. Studies have found that the cell adhesion molecule (CAM) increases in extraocular and retinal vessels in DR, resulting in increased leukocyte adhesion, vascular leakage, capillary nonperfusion, and endothelial cell injury (70). The increase of cytokines, chemokines, adhesion molecules, VCAM-1, and VEGF activates NF-κB, causing the leukocytes to adhere directly to vascular endothelial cells, increasing vascular permeability and damaging endothelial cells by releasing free radicals, enzymes, and cytokines (71). Recent studies have shown that the level of the cell signaling regulator pSTAT3 is elevated in circulating bone marrow monocytes from patients with diabetes and streptozotocin mice, resulting in a specific loss of SOCS3, which leads to leukostasis and capillary exfoliation increase (61). According to Ye et al., miR-15a and miR-16 could inhibit the pro-inflammatory signaling pathway and retinal leukostasis in DR, and the lack of these two miRNAs would increase retinal leukostasis, IL-1β, CD45, TNF-α, and NF- κB (59).
MicroRNAs are not only involved in the recruitment of immune cells but also regulate macrophage inflammatory immune signaling pathways at the site of injury. As an important pathogenic factor of DR, retinal hypoxia can activate macrophages, immune cells, and retinal microglia to release TNF-α, IL-8, VEGF, and MCP-1, leading to retinal ischemia and stimulating the production of VEGF and erythropoietin. Experiments have proven that inhibiting miR-27a can stimulate the production of TNF-α and IL-1β as well as pro-inflammatory proteins, including cyclooxygenase-2 and inducible nitric oxide synthase, by targeting toll-like receptor 4 (TLR4), thus participating in the inflammatory response of DR (60). In addition, the upregulation of miR-455-5p significantly attenuated the high glucose (HG)-stimulated inflammatory response by inhibiting the release of inflammatory cytokines, such as IL-6, IL-1β, and TNF-α in arising retinal pigment epithelial (19) cells (61). In conclusion, miRNAs are closely related to the inflammatory response, which plays a vital role in the pathological process of retinal vasculopathy, especially by mediating the interaction between immune and endothelial cells and the extracellular matrix by inducing the inflammatory signaling pathway.
Metabolic abnormalities caused by hyperglycemia can lead to excessive the production of reactive oxygen species (ROS). The accumulation of ROS leads to oxidative stress, which damages the inner and surrounding tissues of retinal vessels. Finally, it leads to DR. There are four classical mechanisms of vascular injury caused by hyperglycemia (72). The association of miRNAs with oxidative stress in DR has been confirmed (73). In the following section, the action mechanism and research progress of miRNAs in DR oxidative stress are discussed in detail from four aspects.
Hyperglycemia induces the activation of the polyol pathway and increases glucose content in cells. Aldose reductase (AR) reduces glucose to sorbitol, and sorbitol dehydrogenase oxidizes it to fructose. In this process, nicotinamide adenine dinucleotide phosphate (NADPH) and glutathione (GSH) are consumed to produce ROS, and the subsequent NADH oxidation is increased during the conversion of sorbitol to fructose. Aldose reductase, as the key rate-limiting enzyme in the polyol pathway, promotes the expressions of inflammatory factors TNF-α and NF-κB. The inhibition of glyceraldehyde-3-phosphate dehydrogenase contributes to the production of dihydroxyacetone phosphate and the increase of protein kinase C (PKC) and advanced glycation end products (AGEs), thereby inducing the increase of NADPH oxidase, the expression of inflammatory factors, and the decrease of endothelial nitric oxide synthase (eNOS) activation (74). The overexpression of miRNA-145 can reduce the levels of ROS and malondialdehyde, and increase the activity of superoxide dismutase, thus reducing hyperglycemia-induced oxidative stress and retinal endothelial cell apoptosis (62).
The AGEs are advanced products of nonenzymatic glycation. Oxidized AGEs activate the receptor of AGEs (RAGE) to stimulate NADPH oxidase-1 and promote the production of ROS in diabetes (75). The AGEs can interact with scavenger receptors involved in AGE capture, removal, and degradation, as well as with pattern recognition receptors (i.e., RAGE) that initiate specific cell signaling (76). The AGE–RAGE interaction can activate a variety of signal transduction pathways, increase the expression of VCAM-1, macrophage inflammatory protein-1 matrix metalloproteinase 9 (MMP9), IL-1β, and TNF-α, and mediate leukocyte adhesion and the vascular inflammatory response, resulting in mitochondrial dysfunction and cell death (77). The RAGE ligands can activate the innate immune system response through TLR4 signaling and increased NF-κB transcription factor activity (78). Methylglyoxal, a precursor of AGE adducts, is elevated during hyperglycemia, which in turn promotes the expression of AGE, RAGE, and RAGE ligands (74). Studies have indicated that increasing the expression of miR-200c promotes the production of ROS (79). Additionally, MiRNA-383 can promote the production of ROS and induce apoptosis by increasing the expression of peroxidase redoxin 3 and Bax/Bcl2 (24).
Protein kinase C is a serine/threonine-related protein kinase associated with diabetes. It has three subtypes: PKC-β, PKC-δ, and PKC-ζ. Protein kinase C-β can increase the expression of VEGF; aldose reductase activates PKC-δ; and the PKC-δ/p38 αMAPK pathway inhibits the apoptosis of pericytes and the formation of acellular capillaries induced by VEGF (80). Protein kinase C-ζ can be detected in endothelial cells and is involved in YYY- and VEGF-mediated cell proliferation and high permeability (81). Mir-183 upregulation inhibits B cell translocation gene 1, activates the PI3K/Akt/VEGF signaling pathway, and increases CD34, eNOS, and ROS (82). MiR-27 inhibits the Nox 2 signaling pathway by downregulating P13K/AKT/mTOR, thus reducing the production of ROS (63).
The increase of hyperglycemia-induced ROS transfers fructose-6-phosphate from the glycolytic pathway to the hexosamine pathway and produces UDP-N-acetylglucosamine by inhibiting the activity of glyceraldehyde-3-phosphate dehydrogenase. The rate-limiting enzyme is fructose-6-phosphate aminotransferase. In retinal neurons, the modification of glucosamine N-acetylglucosamine changes the neuroprotective effect of the insulin/Akt pathway (83). Studies have shown that hyperglycemia promotes N-acetylglucosamine acylation of NF-κB, which leads to retinal ganglion cell death (84). Hyperglycemia also reduces the expression of anti-inflammatory protein A20 through O-glucosamine-N-acetylation-dependent ubiquitination and proteasome degradation in response to inflammatory stimulation in VSMC and endothelial cells (85). Currently, there are no reports that miRNAs play a role in DR by activating the hexokinase pathway; this may be a future research area.
Retinal tissue is composed of vascular tissues, neurons, and glial cells, all of which constitute the neurovascular unit. The occurrence of DR includes two interrelated components: diabetic retinal neurodegeneration (DRN) and diabetic retinal vasculopathy. The occurrence of DRN includes the apoptosis of ganglion and amacrine cells and the activation of Müller and microglia cells (MGs) (86). Previous studies have shown that miRNAs may be involved in the degeneration of Müller cells induced by DR (66). In the following section, the action mechanism of miRNAs in the occurrence and development of DR neurodegeneration and the application of miRNAs antagonists in neurodegeneration animal models will be described in detail.
Many studies have shown that retinal neuronal dysfunction and apoptosis predate the development of vascular abnormalities in DR (87). Studies have also shown that the expressions of c-Fos/c-Jun, JNK, Bax, caspase-9, caspase-3, and Fluoro-Jade B apoptotic cell markers can be identified in the retina of patients with DR, and the activation of JNK/AP-1 signal transduction and the caspase-dependent cell death pathway in mitochondria can cause the degeneration of DR retinal ganglion cells (88). In the HG state, miR-495 may affect PTEN/Akt signal transmission by targeting Notch1 and aggravate the damage to ganglion cells (89). MiR-34a can promote mitochondrial dysfunction and retinal microvascular endothelial cell senescence by inhibiting the SIRT1/PGC-1α axis and mitochondrial antioxidants TrxR2 and SOD2 (64). It was found in experiments that overexpression of miR-451a may protect mitochondrial function by downregulating activating transcription factor 2 and its downstream target genes CyclinA1, CyclinD1, and MMP2, which provides a new perspective for the development of effective treatment for proliferative DR (65).
As the primary macroglial cells in the retina, MGs are surrounded by neurons, extending from the ganglion cell layer to the photoreceptor inner ganglion region. In the early stage of DR, MG abnormalities, including increased expression of the glial fibrillary acidic protein, MG swelling, and activation of pro-inflammatory receptors in the microglia via P2X7 purines, can be observed (90). Microglia, including two activated phenotypes of pro-inflammatory (M1) state and anti-inflammatory (M2) state, is one of the components of neurovascular units. Pro-inflammatory microglia secrete IL-1β, IL-6, IL-8, and TNF-α cytokines, and anti-inflammatory microglia secrete IL-4, IL-10, IL-13, and transforming growth factor-β cytokines (91). It can be observed that microglia change from an anti-inflammatory state to a pro-inflammatory state before DR retinal neuron cell apoptosis. The increase of hyperglycemia-induced microglia AGEs stimulates the expression of TNF-α through ERK and NF-κB and induces VEGF expression in microglia through the ERK1/2-NF-κB signaling pathway (92). It has been shown in previous studies that upregulation of miR-486-3p protects MGs in the HG state from oxidative stress, inflammation, and apoptosis by inhibiting the TLR4/NF-kB axis (66). MiR-320a can promote the internalization of aquaporin-4 (AQP4), thus reducing the edema of MG under hypoxic stress (67). Under HG, miR-365 can promote retinal oxidative stress and microglial proliferation by regulating the expression of TIMP3, aggravating DR disease (93). MiR-30a activates retinal microglia in an NLRP3-dependent manner, thus promoting the progress of DR (68).
Streptozotocin-induced type 1 diabetic rats and type db/db2 diabetic mice are often used in research to simulate diabetic animal models. MiR-30a-3p synthesized in streptozotocin-induced type 1 diabetic rats can effectively alleviate retinal vascular dysfunction in rats (94). According to Chen et al., intravitreal injection of miR-21-5p inhibitors into the vitreous humor of db/db2 mice targets the peroxisome proliferator-activated receptor α and can reduce retinal inflammation (26).
Diabetic retinopathy is a common microvascular complication of diabetes and the leading cause of blindness in adults. Diabetic retinopathy can be divided into non-PDR and PDR. Non-proliferative DR is clinically characterized by microaneurysm, intraretinal microvascular abnormality, and altered vascular permeability. Advanced PDR is characterized by hypoxia and angiogenesis. Due to the instability of these vessels, vitreous hemorrhage caused by vascular leakage may occur. Diabetic macular edema results from fluid accumulation in the inner and outer layers of the reticular retinal layer caused by changes in retinal blood flow. Diabetic retinopathy has been regarded as a vascular disease for many years, but it is now widely accepted that pathological processes such as oxidative stress, neurodegeneration, chronic inflammation, gliosis, and fibrosis play a vital role in the pathogenesis of early and advanced DR (95). The current treatments for DME include retinal laser photocoagulation, intravitreal VEGF inhibitor, intravitreal steroid, and vitrectomy. Unfortunately, each of these treatments has certain side effects. Retinal laser photocoagulation usually reduces the risk of moderate vision loss in patients with foveal DME but generally does not bring any visual gain (96). Frequent intravitreal injections of drug therapy (VEGF inhibitors and steroids) inconvenience most patients. Repeated use of VEGF inhibitors will increase the risk of fibrosis complications and tractive retinal detachment and may even cause neurodegeneration (97). Corticosteroids are associated with a high risk of cataract formation and elevated intraocular pressure (98). Therefore, efforts are being made to find new alternative drugs with long-term safety and effectiveness. Targeting specific miRNAs are viable treatment options for both DR and DME. The following section will focus on two promising methods to provide miRNA treatment.
Nuclease has a poor affinity and easy degradation, but chemically-modified nucleic acids can overcome these shortcomings. Studies have shown that locked nucleic acid (LNA)-modified oligonucleotides have strong stability, nuclease resistance, high affinity, and specificity for complementary RNA or DNA oligonucleotides and have become a promising candidate for nucleic acid-based therapeutic strategy in vitro and in vivo (99).
Studies have shown that LNA anti-miRNAs can inhibit target molecules for up to 15 weeks in living animals, suitable for targeting DR candidate markers (100). The downregulation of miRNA activity can be achieved by adding nucleotide-based inhibitors bound to STA-B. A study by Huang et al. showed that the downregulation of miRNA activity could be achieved by adding nucleotide inhibitors that bind to STA-Bb to reduce the activity of miRNAs (101).
Extracellular vesicles in organisms function to exchange nucleic acids, lipids, and proteins between cells. Such EVs represent a promising delivery vector for new drugs and gene therapy, with great potential for clinical applications, by manipulating EVs to load miRNAs in vitro, delivering them to target cells as drugs or for bioengineering purposes, or regulating the specificity of EVs of recipient cells as high-precision carriers. In practice, several miRNA delivery methods such as AAV vector, plasmid, piggyback expression vector, nanoparticles such as CC9 with tumor targeting and penetrating bifunctional peptides, and peptide nucleic acid-artificial peptide polymer combined with target nucleotide sequence are also used (102). Clinically, adding N-acetylgalactosamine to siRNA-based drugs can target miRNAs in specific tissues (103).
In conclusion, miRNAs play an essential role in the key disease symptoms of DR, such as chronic inflammation, oxidative stress, and neurodegeneration. In addition, miRNAs are present in the circulating blood and vitreous humor, and they can regulate the above disease processes at multiple levels. They may play a role by interacting with various inflammatory factors (such as IL-1β, IL-6, TNF-α, IL-8, and MCP-1) and transcription factor NF-κB. Although the specific roles of miRNAs in the retina are not fully understood, they can be considered an essential part of the pathological signal transduction, and their role may have a therapeutic effect on DR. Nuclease-protected miRNAs and anti-miRNAs can be used to target DR candidate markers with the potential to exert broader biological effects. In clinical studies, gene therapy delivery vehicles, such as EVs, AAV vectors, plasmids, piggyback expression vectors, nanoparticles (including CC9), and artificial peptide polymers have shown favorable prospects. Despite the possibility of miRNAs in the treatment of multifactorial and complex ophthalmic diseases, including DR, given the fact that miRNAs have a broad impact on many biological functions and basic cell signal cascades, it is necessary to take into account the possible traps based on miRNA therapeutic targets, monitor the possible adverse reactions of miRNA therapy, and determine a safe therapy window.
Conception and design of the research: XZ and FL; Acquisition of data: GZ and NY; Analysis and interpretation of the data: FL; Statistical analysis: GZ and NY; Obtaining financing: XZ; Writing of the manuscript: XZ and FL; Critical revision of the manuscript for intellectual content: JY and XX. All authors contributed to the article and approved the submitted version.
Health Science and Technology Plan of Inner Mongolia Autonomous Region Health Commission (No.202201522).
The authors declare that the research was conducted in the absence of any commercial or financial relationships that could be construed as a potential conflict of interest.
All claims expressed in this article are solely those of the authors and do not necessarily represent those of their affiliated organizations, or those of the publisher, the editors and the reviewers. Any product that may be evaluated in this article, or claim that may be made by its manufacturer, is not guaranteed or endorsed by the publisher.
MiRNAs, MicroRNAs; DR, Diabetic Retinopathy; DME, Diabetic Macular Edema; PDR, Proliferative Diabetic Retinopathy; miRNA, pre-miRNA; AGO, Argonaute; miRISC, miRNA-Induced Silencing Complex; UTR, three prime untranslated region; RT-PCR, Reverse Transcription-Polymerase Chain Reaction; MVB, Multivesicular Body; EV, Circulating extracellular vesicles; TF, Transcription Factor; PVR, Proliferative Vitreoretinopathy; AMD, Age-related Macular Degeneration; RGCs, Retinal Ganglion Cells; IL-1β, Interleukin-1β; IL-6, Interleukin-6; TNF-a, Tumor Necrosis Factor- a; IL-8, Interleukin-8; MCP-1, Monocyte Chemoattractant Protein-1; NF-κB, Nuclear Factor-κB; CAM, Cell Adhesion Molecule; EPO, erythropoietin; TLR4, Toll like receptor 4; ROS, reactive oxygen species; AR, Aldose Reductase; GAPDH, glyceraldehyde-3-phosphate dehydrogenase; DHAP, dihydroxyacetone phosphate; eNOS, Nitric oxide synthase; MDA, malondialdehyde; RAGE, Receptor for Advanced Glycation End products; MIP-1, Macrophage Inflammatory Protein-1; MMP 9, Matrix metalloproteinase 9; PRDX 3, Peroxidase redoxin 3; ox-LDL, Oxidized low density lipoprotein; BTG1, B cell translocation gene 1; GFAT, Glutamine:fructose-6-phosphate aminotransferase; GlcNAc, Glucosamine N-acetylglucosamine; DRN, Diabetic Retina Neuropathy; DRV, Diabetic RetinaVessel; ATF2, activating transcription factor 2; MG, Müller glia cells; GCL, Ganglion Cell Layer; GFAP, Glial Fibrillary Acidic Protein; AQP4, Aquaporin-4; PPAR-a, Peroxisome Proliferator-Activated Receptor-α; NPDR, Non-proliferative Diabetic Retinopathy; IRMA, Intraretinal Microvascular Abnormalities; LNA, Locked Nucleic Acid; Gal-NAC, N-Acetyl-galactosamine.
1. Zhang S, Zhou Y, Wang Y, Wang Z, Xiao Q, Zhang Y, et al. The Mechanistic, Diagnostic and Therapeutic Novel Nucleic Acids for Hepatocellular Carcinoma Emerging in Past Score Years. Briefings Bioinf (2020) 22(2):1860–83. doi: 10.1093/bib/bbaa023
2. Greco S, Salgado Somoza A, Devaux Y, Martelli F. Long Noncoding RNAs and Cardiac Disease. Antioxid Redox Signal (2018) 29(9):880–901. doi: 10.1089/ars.2017.7126
3. Calin GA, Dumitru CD, Shimizu M, Bichi R, Zupo S, Noch E, et al. Frequent Deletions and Down-Regulation of Micro- RNA Genes Mir15 and Mir16 at 13q14 in Chronic Lymphocytic Leukemia. Proc Natl Acad Sci USA (2002) 99(24):15524–9. doi: 10.1073/pnas.242606799
4. Peng Y, Croce CM. The Role of MicroRNAs in Human Cancer. Signal Transduct Targeted Ther (2016) 1:15004. doi: 10.1038/sigtrans.2015.4
5. Satake E, Pezzolesi MG, Md Dom ZI, Smiles AM, Niewczas MA, Krolewski AS. Circulating miRNA Profiles Associated With Hyperglycemia in Patients With Type 1 Diabetes Mellitus. Diabetes (2018) 67(5):1013–23. doi: 10.2337/db17-1207
6. Ultimo S, Zauli G, Martelli AM, Vitale M, McCubrey JA, Capitani S, et al. Cardiovascular Disease-Related miRNAs Expression: Potential Role as Biomarkers and Effects of Training Exercise. Oncotarget (2018) 9(24):17238–54. doi: 10.18632/oncotarget.24428
7. He X, Kuang G, Wu Y, Ou C. Emerging Roles of Exosomal miRNAs in Diabetes Mellitus. Clin Trans Med (2021) 11(6):e468. doi: 10.1002/ctm2.468
8. Ha M, Kim VN. Regulation of microRNA Biogenesis. Nat Rev Mol Cell Biol (2014) 15(8):509–24. doi: 10.1038/nrm3838
9. Hutvágner G, McLachlan J, Pasquinelli AE, Bálint E, Tuschl T, Zamore PD. A Cellular Function for the RNA-Interference Enzyme Dicer in the Maturation of the Let-7 Small Temporal RNA. Science (2001) 293(5531):834–8. doi: 10.1126/science.1062961
10. Schwarzenbach H, Nishida N, Calin GA, Pantel K. Clinical Relevance of Circulating Cell-Free microRNAs in Cancer. Nat Rev Clin Oncol (2014) 11(3):145–56. doi: 10.1038/nrclinonc.2014.5
11. Bartel DP. MicroRNAs: Genomics, Biogenesis, Mechanism, and Function. Cell (2004) 116(2):281–97. doi: 10.1016/S0092-8674(04)00045-5
12. Höck J, Meister G. The Argonaute Protein Family. Genome Biol (2008) 9(2):210. doi: 10.1186/gb-2008-9-2-210
13. Fromm B, Domanska D, Høye E, Ovchinnikov V, Kang W, Aparicio-Puerta E, et al. MirGeneDB 2.0: The Metazoan microRNA Complement. Nucleic Acids Res (2020) 48:1172. doi: 10.1093/nar/gkz885
15. Rodrigues DVS, Monteiro VVS, Navegantes-Lima KC, Oliveira AL, Gaspar , et al. MicroRNAs in Cell Cycle Progression and Proliferation: Molecular Mechanisms and Pathways. Non Coding RNA Invest (2018) 2:28–8. doi: 10.21037/ncri.2018.04.06
16. Gong Q, Su G. Roles of miRNAs and Long Noncoding RNAs in the Progression of Diabetic Retinopathy. Biosci Rep (2017) 37(6):BSR20171157. doi: 10.1042/BSR20171157
17. Valadi H, Ekstrom K, Bossios A, Sjöstrand M, Lee JJ, Lötvall JO. Exosome-Mediated Transfer of mRNAs and microRNAs is a Novel Mechanism of Genetic Exchange Between Cells. Nat Cell Biol (2007) 9(6):654–9. doi: 10.1038/ncb1596
18. Mori MA, Ludwig RG, Garcia-Martin R, Brandão BB, Kahn CR. Extracellular miRNAs: From Biomarkers to Mediators of Physiology and Disease. Cell Metab (2019) 30(4):656–73. doi: 10.1016/j.cmet.2019.07.011
19. Li Z, Dong Y, He C, Pan X, Liu D, Yang J, et al. RNA-Seq Revealed Novel Non-Proliferative Retinopathy Specific Circulating MiRNAs in T2DM Patients. Front Genet (2019) 10:531. doi: 10.3389/fgene.2019.00531
20. Shaker OG, Abdelaleem OO, Mahmoud RH, Abdelghaffar NK, Ahmed TI, Said OM, et al. Diagnostic and Prognostic Role of Serum miR-20b, miR-17-3p, HOTAIR, and MALAT1 in Diabetic Retinopathy. IUBMB Life (2019) 71(3):310–20. doi: 10.1002/iub.1970
21. Rezk NA, Sabbah NA, Saad MS. Role of MicroRNA 126 in Screening, Diagnosis, and Prognosis of Diabetic Patients in Egypt. IUBMB Life (2016) 68(6):452–8. doi: 10.1002/iub.1502
22. Yin C, Lin X, Sun Y, Ji X. Dysregulation of miR-210 is Involved in the Development of Diabetic Retinopathy and Serves a Regulatory Role in Retinal Vascular Endothelial Cell Proliferation. Eur J Med Res (2020) 25(1):20. doi: 10.1186/s40001-020-00416-3
23. Zampetaki A, Willeit P, Burr S, Yin X, Langley SR, Kiechl S, et al. Angiogenic microRNAs Linked to Incidence and Progression of Diabetic Retinopathy in Type 1 Diabetes. Diabetes (2016) 65(1):216–27. doi: 10.2337/db15-0389
24. Jiang Y, Sang Y, Qiu Q. microRNA-383 Mediates High Glucose-Induced Oxidative Stress and Apoptosis in Retinal Pigment Epithelial Cells by Repressing Peroxiredoxin 3. Am J Trans Res (2017) 9(5):2374–83.
25. Dantas da Costa E Silva ME, Polina ER, Crispim D, Sbruzzi RC, Lavinsky D, Mallmann F, et al. Plasma Levels of miR-29b and miR-200b in Type 2 Diabetic Retinopathy. J Cell Mol Med (2019) 23(2):1280–7. doi: 10.1111/jcmm.14030
26. Zou HL, Wang Y, Gang Q, Zhang Y, Sun Y. Plasma Level of miR-93 is Associated With Higher Risk to Develop Type 2 Diabetic Retinopathy. Graefe\"s Arch Clin Exp Ophthalmol (2017) 255(6):1159–66. doi: 10.1007/s00417-017-3638-5
27. Liu Y, Xiao J, Zhao Y, Zhao C, Yang Q, Du X, et al. microRNA-216a Protects Against Human Retinal Microvascular Endothelial Cell Injury in Diabetic Retinopathy by Suppressing the NOS2/JAK/STAT Axis. Exp Mol Pathol (2020) 115:104445. doi: 10.1016/j.yexmp.2020.104445
28. Estrella S, Garcia-Diaz DF, Codner E, Camacho-Guillén P, Pérez-Bravo F. Expression of miR-22 and miR-150 in Type 1 Diabetes Mellitus: Possible Relationship With Autoimmunity and Clinical Characteristics. Med Clin (2016) 147:245–7, S238702061630612X. doi: 10.1016/j.medcle.2016.10.014
29. Kamalden TA, Macgregor-Das AM, Kannan SM, Dunkerly-Eyring B, Khaliddin N, Xu Z, et al. Exosomal MicroRNA-15a Transfer From the Pancreas Augments Diabetic Complications by Inducing Oxidative Stress. Antioxid Redox Signal (2017) 27(13):913–30. doi: 10.1089/ars.2016.6844
30. Maisto R, Trotta MC, Petrillo F, Izzo S, Cuomo G, Alfano R, et al. Resolvin D1 Modulates the Intracellular VEGF-Related miRNAs of Retinal Photoreceptors Challenged With High Glucose. Front Pharmacol (2020) 11:235. doi: 10.3389/fphar.2020.00235
31. Safwat A, Sabry D, Ragiae A, Amer E, Mahmoud RH, Shamardan RM. Adipose Mesenchymal Stem Cells-Derived Exosomes Attenuate Retina Degeneration of Streptozotocin-Induced Diabetes in Rabbits. J Circ biomark (2018) 7:1849454418807827. doi: 10.1177/1849454418807827
32. Friedrich J, Steel DHW, Schlingemann RO, Koss MJ, Hammes HP, Krenning G, et al. microRNA Expression Profile in the Vitreous of Proliferative Diabetic Retinopathy Patients and Differences From Patients Treated With Anti-VEGF Therapy. Trans Vision Sci Technol (2020) 9(6):16. doi: 10.1167/tvst.9.6.16
33. Usui-Ouchi A, Ouchi Y, Kiyokawa M, Sakuma T, Ito R, Ebihara N. Upregulation of Mir-21 Levels in the Vitreous Humor Is Associated With Development of Proliferative Vitreoretinal Disease. PloS One (2016) 11(6):e0158043. doi: 10.1371/journal.pone.0158043
34. Kubatka P, Kello M, Kajo K, Samec M, Liskova A, Jasek K, et al. (Sumac) Demonstrates Oncostatic Activity in the Therapeutic and Preventive Model of Breast Carcinoma. Int J Mol Sci (2020) 22(1):183. doi: 10.3390/ijms22010183
35. Smit-McBride Z, Nguyen AT, Yu AK, Modjtahedi SP, Hunter AA, Rashid S, et al. Unique Molecular Signatures of microRNAs in Ocular Fluids and Plasma in Diabetic Retinopathy. PloS One (2020) 15(7):e0235541. doi: 10.1371/journal.pone.0235541
36. Liu HN, Li X, Wu N, Tong MM, Chen S, Zhu SS, et al. Serum microRNA-221 as a Biomarker for Diabetic Retinopathy in Patients Associated With Type 2 Diabetes. Int J Ophthalmol (2018) 11(12):1889–94. doi: 10.18240/ijo.2018.12.02
37. Jiménez-Lucena R, Rangel-Zúñiga OA, Alcalá-Díaz JF, López-Moreno J, Roncero-Ramos I, Molina-Abril H, et al. Circulating miRNAs as Predictive Biomarkers of Type 2 Diabetes Mellitus Development in Coronary Heart Disease Patients From the CORDIOPREV Study. Mol Ther Nucleic Acids (2018) 12:146–57. doi: 10.1016/j.omtn.2018.05.002
38. Qing S, Yuan S, Yun C, Hui H, Mao P, Wen F, et al. Serum MiRNA Biomarkers Serve as a Fingerprint for Proliferative Diabetic Retinopathy. Cell Physiol Biochem Int J Exp Cell Physiol Biochem Pharmacol (2014) 34(5):1733–40. doi: 10.1159/000366374
39. García de la Torre N, Fernández-Durango R, Gómez R, Fuentes M, Roldán-Pallarés M, Donate J, et al. Expression of Angiogenic MicroRNAs in Endothelial Progenitor Cells From Type 1 Diabetic Patients With and Without Diabetic Retinopathy. Invest Opthalmol Visual Sci (2015) 56(6):4090–8. doi: 10.1167/iovs.15-16498
40. Han N, Xu H, Yu N, Wu Y, Yu L. MiR-203a-3p Inhibits Retinal Angiogenesis and Alleviates Proliferative Diabetic Retinopathy in Oxygen-Induced Retinopathy (OIR) Rat Model via Targeting VEGFA and HIF-1α. Clin Exp Pharmacol Physiol (2020) 47(1):85–94. doi: 10.1111/1440-1681.13163
41. Han N, Tian W, Yu N, Yu L. YAP1 is Required for the Angiogenesis in Retinal Microvascular Endothelial Cells via the Inhibition of MALAT1-Mediated miR-200b-3p in High Glucose-Induced Diabetic Retinopathy. J Cell Physiol (2020) 235(2):1309–20. doi: 10.1002/jcp.29047
42. Niel GV, D'Angelo G, Raposo G. Shedding Light on the Cell Biology of Extracellular Vesicles. Nat Rev Mol Cell Biol (2018) 19(4):213–28. doi: 10.1038/nrm.2017.125
43. Chen Q, Qiu F, Zhou K, Matlock HG, Takahashi Y, Rajala RVS, et al. Pathogenic Role of microRNA-21 in Diabetic Retinopathy Through Downregulation of Pparα. Diabetes (2017) 66(6):1671–82. doi: 10.2337/db16-1246
44. Mazzeo A, Lopatina T, Gai C, Trento M, Porta M, Beltramo E. Functional Analysis of miR-21-3p, miR-30b-5p and miR-150-5p Shuttled by Extracellular Vesicles From Diabetic Subjects Reveals Their Association With Diabetic Retinopathy. Exp Eye Res (2019) 184:56–63. doi: 10.1016/j.exer.2019.04.015
45. Hirota K, Keino H, Inoue M, Ishida H, Hirakata A. Comparisons of microRNA Expression Profiles in Vitreous Humor Between Eyes With Macular Hole and Eyes With Proliferative Diabetic Retinopathy. Graefe\"s Arch Clin Exp Ophthalmol (2015) 253(3):335–42. doi: 10.1007/s00417-014-2692-5
46. Salehi H, Amirpour N, Razavi S, Esfandiari E, Zavar R. Overview of Retinal Differentiation Potential of Mesenchymal Stem Cells: A Promising Approach for Retinal Cell Therapy. Ann Anat (2017) 210:52–63. doi: 10.1016/j.aanat.2016.11.010
47. Yang L, Han B, Zhang Z, Wang S, Bai Y, Zhang Y, et al. Extracellular Vesicle-Mediated Delivery of Circular RNA SCMH1 Promotes Functional Recovery in Rodent and Nonhuman Primate Ischemic Stroke Models. Circulation (2020) 142(6):556–74. doi: 10.1161/CIRCULATIONAHA.120.045765
48. McLaughlin T, Siddiqi M, Wang JJ, Zhang SX. Loss of XBP1 Leads to Early-Onset Retinal Neurodegeneration in a Mouse Model of Type I Diabetes. J Clin Med (2019) 8(6):906. doi: 10.3390/jcm8060906
49. Zhang SJ, Chen X, Li CP, Li XM, Liu C, Liu BH, et al. Identification and Characterization of Circular RNAs as a New Class of Putative Biomarkers in Diabetes Retinopathy. Invest Ophthalmol Vis Sci (2017) 58(14):6500–9. doi: 10.1167/iovs.17-22698
50. Zhou RM, Wang XQ, Yao J, Shen Y, Chen SN, Yang H, et al. Identification and Characterization of Proliferative Retinopathy-Related Long Noncoding RNAs. Biochem Biophys Res Commun (2015) 465(3):324–30. doi: 10.1016/j.bbrc.2015.07.120
51. Kaneko H, Terasaki H. Biological Involvement of MicroRNAs in Proliferative Vitreoretinopathy. Transl Vis Sci Technol (2017) 6(4):5. doi: 10.1167/tvst.6.4.5
52. Lefevere E, Toft-Kehler AK, Vohra R, Kolko M, Moons L, Van Hove I. Mitochondrial Dysfunction Underlying Outer Retinal Diseases. Mitochondrion (2017) :36:66–76. doi: 10.1016/j.mito.2017.03.006
53. Berber P, Grassmann F, Kiel C, Weber BH. An Eye on Age-Related Macular Degeneration: The Role of MicroRNAs in Disease Pathology. Mol Diagn Ther (2017) 21(1):31–43. doi: 10.1007/s40291-016-0234-z
54. ElShelmani H, Wride MA, Saad T, Rani S, Kelly DJ, Keegan D. The Role of Deregulated MicroRNAs in Age-Related Macular Degeneration Pathology. Transl Vis Sci Technol (2021) 10(2):12. doi: 10.1167/tvst.10.2.12
55. Shahriari F, Satarian L, Moradi S, Zarchi AS, Günther S, Kamal A, et al. MicroRNA Profiling Reveals Important Functions of miR-125b and Let-7a During Human Retinal Pigment Epithelial Cell Differentiation. Exp Eye Res (2020) 190:107883. doi: 10.1016/j.exer.2019.107883
56. Syc-Mazurek SB, Libby RT. Axon Injury Signaling and Compartmentalized Injury Response in Glaucoma. Prog Retin Eye Res (2019) 73:100769. doi: 10.1016/j.preteyeres.2019.07.002
57. Nie XG, Fan DS, Huang YX, He YY, Dong BL, Gao F. Downregulation of microRNA-149 in Retinal Ganglion Cells Suppresses Apoptosis Through Activation of the PI3K/Akt Signaling Pathway in Mice With Glaucoma. Am J Physiol Cell Physiol (2018) 315(6):C839–49. doi: 10.1152/ajpcell.00324.2017
58. Peng H, Sun YB, Hao JL, Lu CW, Bi MC, Song E. Neuroprotective Effects of Overexpressed microRNA-200a on Activation of Glaucoma-Related Retinal Glial Cells and Apoptosis of Ganglion Cells via Downregulating FGF7-Mediated MAPK Signaling Pathway. Cell Signal (2019) 54:179–90. doi: 10.1016/j.cellsig.2018.11.006
59. Ye EA, Liu L, Jiang Y, Jan J, Gaddipati S, Suvas S, et al. miR-15a/16 Reduces Retinal Leukostasis Through Decreased Pro-Inflammatory Signaling. J Neuroinflamm (2016) 13(1):305. doi: 10.1186/s12974-016-0771-8
60. Lv YN, Ou-Yang AJ, Fu LS. MicroRNA-27a Negatively Modulates the Inflammatory Response in Lipopolysaccharide-Stimulated Microglia by Targeting TLR4 and IRAK4. Cell Mol Neurobiol (2017) 37(2):195–210. doi: 10.1007/s10571-016-0361-4
61. Chen M, Obasanmi G, Armstrong D, Lavery NJ, Kissenpfennig A, Lois N, et al. STAT3 Activation in Circulating Myeloid-Derived Cells Contributes to Retinal Microvascular Dysfunction in Diabetes. J Neuroinflamm (2019) 16(1):138. doi: 10.1186/s12974-019-1533-1
62. Hui Y, Yin Y. MicroRNA-145 Attenuates High Glucose-Induced Oxidative Stress and Inflammation in Retinal Endothelial Cells Through Regulating TLR4/NF-κb Signaling. Life Sci (2018) 207:212–8. doi: 10.1016/j.lfs.2018.06.005
63. Li J, Hui L, Kang Q, Li R. Down-Regulation of microRNA-27b Promotes Retinal Pigment Epithelial Cell Proliferation and Migration by Targeting Nox2. Pathol Res Pract (2018) 214(7):925–33. doi: 10.1016/j.prp.2018.05.025
64. Thounaojam MC, Jadeja RN, Warren M, Powell FL, Raju R, Gutsaeva D, et al. MicroRNA-34a (miR-34a) Mediates Retinal Endothelial Cell Premature Senescence Through Mitochondrial Dysfunction and Loss of Antioxidant Activities. Antioxid (Basel) (2019) 8(9):328. doi: 10.3390/antiox8090328
65. Shao Y, Dong LJ, Takahashi Y, Chen J, Liu X, Chen Q, et al. miRNA-451a Regulates RPE Function Through Promoting Mitochondrial Function in Proliferative Diabetic Retinopathy. Am J Physiol Endocrinol Metab (2019) 316(3):443–52. doi: 10.1152/ajpendo.00360.2018
66. Li W, Jin L, Cui Y, Nie A, Xie N, Liang G. Bone Marrow Mesenchymal Stem Cells-Induced Exosomal microRNA-486-3p Protects Against Diabetic Retinopathy Through TLR4/NF-κb Axis Repression. J Endocrinol Invest (2021) 44(6):1193–207. doi: 10.1007/s40618-020-01405-3
67. Chen Z, Yang Z, Li X, Wang H, Wang Y, Ding C, et al. microRNA-320a Prevent Müller Cells From Hypoxia Injury by Targeting Aquaporin-4. J Cell Biochem (2020) 121:4711–23. doi: 10.1002/jcb.29524
68. Dong N, Wang Y. MiR-30a Regulates S100A12-Induced Retinal Microglial Activation and Inflammation by Targeting Nlrp3. Curr Eye Res (2019) 44(11):1236–43. doi: 10.1080/02713683.2019.1632350
69. Murinello S, Usui Y, Sakimoto S, Kitano M, Aguilar E, Friedlander HM, et al. miR-30a-5p Inhibition Promotes Interaction of Fas+ Endothelial Cells and FasL+ Microglia to Decrease Pathological Neovascularization and Promote Physiological Angiogenesis. Glia (2019) 67(2):332–44. doi: 10.1002/glia.23543
70. Simó R, Hernández C. Novel Approaches for Treating Diabetic Retinopathy Based on Recent Pathogenic Evidence. Prog Retinal Eye Res (2015) 48:160–80. doi: 10.1016/j.preteyeres.2015.04.003
71. Capito M, Soares R. Angiogenesis and Inflammation Crosstalk in Diabetic Retinopathy. J Cell Biochem (2016) 117(11):2443–53. doi: 10.1002/jcb.25575
72. Kowluru RA, Chan PS. Oxidative Stress and Diabetic Retinopathy. Exp Diabetes Res (2007) 43603. doi: 10.1155/2007/43603
73. Satari M, Aghadavod E, Mobini M, Asemi Z. Association Between miRNAs Expression and Signaling Pathways of Oxidative Stress in Diabetic Retinopathy. J Cell Physiol (2019) 234(6):8522–32. doi: 10.1002/jcp.27801
74. Yuan T, Yang T, Chen H, Fu D, Hu Y, Wang J, et al. New Insights Into Oxidative Stress and Inflammation During Diabetes Mellitus-Accelerated Atherosclerosis. Redox Biol (2019) 20:247–60. doi: 10.1016/j.redox.2018.09.025
75. Chen J, Jing J, Yu S, Song M, Tan H, Cui B, et al. Advanced Glycation Endproducts Induce Apoptosis of Endothelial Progenitor Cells by Activating Receptor RAGE and NADPH Oxidase/JNK Signaling Axis. Am J Trans Res (2016) 8(5):2169–78.
76. Byun K, Yoo YC, Son M, Lee J, Jeong GB, Park YM, et al. Advanced Glycation End-Products Produced Systemically and by Macrophages: A Common Contributor to Inflammation and Degenerative Diseases. Pharmacol Ther (2017) 177:44–55. doi: 10.1016/j.pharmthera.2017.02.030
77. Bongarzone S, Savickas V, Luzi F, Gee AD. Targeting the Receptor for Advanced Glycation Endproducts (RAGE): A Medicinal Chemistry Perspective[J]. J Med Chem (2017) 60(17):7213–32. doi: 10.1021/acs.jmedchem.7b00058
78. Nielsen TB, Pantapalangkoor P, Yan J, Luna BM, Dekitani K, Bruhn K, et al. Diabetes Exacerbates Infection via Hyperinflammation by Signaling Through TLR4 and RAGE. Mbio (2017) 8(4):e00818–17. doi: 10.1128/mBio.00818-17
79. Magenta A, Ciarapica R, Capogrossi MC. The Emerging Role of miR-200 Family in Cardiovascular Diseases. Circ Res (2017) 120(9):1399–402. doi: 10.1161/CIRCRESAHA.116.310274
80. Geraldes P, Hiraoka-Yamamoto J, Matsumoto M, Clermont A, Leitges M, Marette A, et al. Activation of PKC-δ and SHP-1 by Hyperglycemia Causes Vascular Cell Apoptosis and Diabetic Retinopathy. Nat Med (2009) 15(11):1298–306. doi: 10.1038/nm.2052
81. Aveleira C, Lin C-M, Abcouwer SF, Ambrósio AF, Antonetti DA. TNF-[Alpha] Signals Through PKC [Zeta]/NF-[Kappa]B to Alter the Tight Junction Complex and Increase Retinal Endothelial Cell Permeability. Diabetes (2010) 59(11):2872–82. doi: 10.2337/db09-1606
82. Zhang ZZ, Qin XH, Zhang J. MicroRNA-183 Inhibition Exerts Suppressive Effects on Diabetic Retinopathy by Inactivating BTG1-Mediated PI3K/Akt/VEGF Signaling Pathway. Am J Physiol Endocrinol Metab (2019) 316(6):1050–60. doi: 10.1152/ajpendo.00444.2018
83. Gurel Z, Sheibani N. O-Linked β–Acetylglucosamine (O-GlcNAc) Modification a New Pathway to Decode Pathogenesis of Diabetic Retinopathy. Clin Sci (2018) 132(2):185–98. doi: 10.1042/CS20171454
84. Kim SJ, Yoo WS, Choi M, Chung I, Yoo JM, Choi WS. Increased O-GlcNAcylation of NF-κb Enhances Retinal Ganglion Cell Death in Streptozotocin-Induced Diabetic Retinopathy. Curr Eye Res (2016) 41(2):249–57. doi: 10.3109/02713683.2015.1006372
85. Li H, Horke S, Frstermann U. Oxidative Stress in Vascular Disease and Itspharmacological Prevention. Trends Pharmacol Sci (2013) 34(6):313–9. doi: 10.1016/j.tips.2013.03.007
86. Barber AJ. A New View of Diabetic Retinopathy: A Neurodegenerative Disease of the Eye. Prog Neuropsychopharmacol Biol Psychiatry (2003) 27(2):283–90. doi: 10.1016/S0278-5846(03)00023-X
87. Montesano G, Ometto G, Higgins BE, Das R, Graham KW, Chakravarthy U, et al. Evidence for Structural and Functional Damage of the Inner Retina in Diabetes With No Diabetic Retinopathy. Invest Ophthalmol Vis Sci (2021) 62(3):35. doi: 10.1167/iovs.62.3.35
88. Oshitari T, Yamamoto S, Roy S. Increased Expression of C-Fos, C-Jun and C-Jun N-Terminal Kinase Associated With Neuronal Cell Death in Retinas of Diabetic Patients. Curr Eye Res (2014) 39(5):527–31. doi: 10.3109/02713683.2013.833248
89. Zhang X, Yang Y, Feng Z. Suppression of microRNA-495 Alleviates High-Glucose-Induced Retinal Ganglion Cell Apoptosis by Regulating Notch/PTEN/Akt Signaling. Biomed Pharmacother = Biomed Pharmacother (2018) 106:923–9. doi: 10.1016/j.biopha.2018.07.018
90. Portillo JC, Lopez Corcino Y, Miao Y, Tang J, Sheibani N, Kern TS, et al. CD40 in Retinal Müller Cells Induces P2X7-Dependent Cytokine Expression in Macrophages/Microglia in Diabetic Mice and Development of Early Experimental Diabetic Retinopathy. Diabetes (2017) 66(2):483–93. doi: 10.2337/db16-0051
91. Arroba AI, Alcalde-Estevez E, García-Ramírez M, Cazzoni D, de la Villa P, Sánchez-Fernández EM, et al. Modulation of Microglia Polarization Dynamics During Diabetic Retinopathy in Db/Db Mice. Biochim Et Biophys Acta (2016) 1862(9):1663–74. doi: 10.1016/j.bbadis.2016.05.024
92. Zhang T, Ouyang H, Mei X, Lu B, Yu Z, Chen K, et al. Erianin Alleviates Diabetic Retinopathy by Reducing Retinal Inflammation Initiated by Microglial Cells via Inhibiting Hyperglycemia-Mediated ERK1/2-NF-κb Signaling Pathway. FASEB J (2019) 33(11):11776–90. doi: 10.1096/fj.201802614RRR
93. Wang J, Zhang J, Chen X, Yang Y, Wang F, Li W, et al. miR-365 Promotes Diabetic Retinopathy Through Inhibiting Timp3 and Increasing Oxidative Stress. Exp Eye Res (2018) 168:89–99. doi: 10.1016/j.exer.2017.11.006
94. Shan K, Liu C, Liu BH, Chen X, Dong R, Liu X, et al. Circular Noncoding RNA HIPK3 Mediates Retinal Vascular Dysfunction in Diabetes Mellitus. Circulation (2017) 136(17):1629–42. doi: 10.1161/CIRCULATIONAHA.117.029004
95. Stitt AW, Curtis TM, Chen M, Medina RJ, McKay GJ, Jenkins A, et al. The Progress in Understanding and Treatment of Diabetic Retinopathy. Prog Retinal Eye Res (2016) 51:156–86. doi: 10.1016/j.preteyeres.2015.08.001
96. Bucolo C, Gozzo L, Longo L, Mansueto S, Vitale DC, Drago F. Long-Term Efficacy and Safety Profile of Multiple Injections of Intravitreal Dexamethasone Implant to Manage Diabetic Macular Edema: A Systematic Review of Real-World Studies. J Pharmacol Sci (2018) 138(4):219–32. doi: 10.1016/j.jphs.2018.11.001
97. Beck M, Munk MR, Ebneter A, Wolf S, Zinkernagel MS. Retinal Ganglion Cell Layer Change in Patients Treated With Anti-Vascular Endothelial Growth Factor for Neovascular Age-Related Macular Degeneration. Am J Ophthalmol (2016) 167:10–7. doi: 10.1016/j.ajo.2016.04.003
98. Nurözler Tabakcı B, Ünlü N. Corticosteroid Treatment in Diabetic Macular Edema. Turk J Ophthalmol (2017) 47(3):156–60. doi: 10.4274/tjo.56338
99. Veedu R, Wengel J. Locked Nucleic Acids: Promising Nucleic Acid Analogs for Therapeutic Applications. Chem Biodivers (2010) 7(3):536–42. doi: 10.1002/cbdv.200900343
100. Leucci E, Vendramin R, Spinazzi M, Laurette P, Fiers M, Wouters J, et al. Melanoma Addiction to the Long non-Coding RNA SAMMSON. Nature (2016) 531(7595):518–22. doi: 10.1038/nature17161
101. Huang X, Leroux JC, Castagner B. Well-Defined Multivalent Ligands for Hepatocytes Targeting via Asialoglycoprotein Receptor. Bioconjug Chem (2017) 28(2):283–95. doi: 10.1021/acs.bioconjchem.6b00651
102. Christopher AF, Kaur RP, Kaur G, Kaur A, Gupta V, Bansal P. MicroRNA Therapeutics: Discovering Novel Targets and Developing Specific Therapy. Perspect Clin Res (2016) 7(2):68–74. doi: 10.4103/2229-3485.179431
Keywords: micro ribonucleic acids (miRNAs), diabetic retinopathy (DR), inflammation, oxidative stress, neurodegeneration
Citation: Zhao X, Ling F, Zhang Gw, Yu N, Yang J and Xin Xy (2022) The Correlation Between MicroRNAs and Diabetic Retinopathy. Front. Immunol. 13:941982. doi: 10.3389/fimmu.2022.941982
Received: 12 May 2022; Accepted: 23 June 2022;
Published: 25 July 2022.
Edited by:
Christophe Chevillard, TAGC Theories and Approaches of Genomic Complexity, FranceReviewed by:
Saumya Patel, Gujarat University, IndiaCopyright © 2022 Zhao, Ling, Zhang, Yu, Yang and Xin. This is an open-access article distributed under the terms of the Creative Commons Attribution License (CC BY). The use, distribution or reproduction in other forums is permitted, provided the original author(s) and the copyright owner(s) are credited and that the original publication in this journal is cited, in accordance with accepted academic practice. No use, distribution or reproduction is permitted which does not comply with these terms.
*Correspondence: Jing Yang, eWFuZ2ppbmdpbXVAMjFjbi5jb20=; Xiang yang Xin, eGlueGlhbmd5YW5naW11QG91dGxvb2suY29t
†These authors have contributed equally to this work
Disclaimer: All claims expressed in this article are solely those of the authors and do not necessarily represent those of their affiliated organizations, or those of the publisher, the editors and the reviewers. Any product that may be evaluated in this article or claim that may be made by its manufacturer is not guaranteed or endorsed by the publisher.
Research integrity at Frontiers
Learn more about the work of our research integrity team to safeguard the quality of each article we publish.