- Division of Transplant Surgery, Department of Surgery, Indiana University School of Medicine, Indianapolis, IN, United States
Eliminating major xenoantigens in pig cells has drastically reduced human antibody-mediated hyperacute xenograft rejection (HXR). Despite these advancements, acute xenograft rejection (AXR) remains one of the major obstacles to clinical xenotransplantation, mediated by innate immune cells, including macrophages, neutrophils, and natural killer (NK) cells. NK cells play an ‘effector’ role by releasing cytotoxicity granules against xenogeneic cells and an ‘affecter’ role on other immune cells through cytokine secretion. We highlight the key receptor-ligand interactions that determine the NK cell response to target cells, focusing on the regulation of NK cell activating receptor (NKG2D, DNAM1) and inhibitory receptor (KIR2DL1-4, NKG2A, and LIR-1) signaling pathways. Inhibition of NK cell activity may protect xenografts from cytotoxicity. Recent successful approaches to reducing NK cell-mediated HXR and AXR are reviewed, including genetic modifications of porcine xenografts aimed at improving pig-to-human compatibility. Future directions to promote xenograft acceptance are discussed, including NK cell tolerance in pregnancy and NK cell evasion in viral infection.
Introduction
The persistent lack of transplantable organs has resulted in the death of thousands of patients every year. There have been several proposed solutions to address this issue, including manufacturing bioartificial organs (1), 3D printing human organs (2), and transplanting organs from different species into humans, a practice known as xenotransplantation. Xenotransplantation represents one of the most promising approaches. Elimination of major xenoantigens on xenografts by gene-editing tools has proven to be an effective approach to preventing hyperacute xenograft rejection (HXR) (3–6). Earlier this year, the first pig-to-human heart transplantation was performed and supported the patient’s life for two months. In this xenotransplant, HXR was successfully prevented with 10-gene modification, particularly with three major xenoantigens (αGal, Neu5Gc, and Sda) removal in the xenograft (7). Despite this exciting success, xenotransplantation must overcome other barriers before becoming a widespread clinically viable solution. As a result of current advances, the field has shifted towards addressing the next major immunologic barrier: acute and chronic xenograft rejection.
Natural killer (NK) cells are a subset of lymphocytes that not only constitute the innate immune system’s first line of defense but also play a significant role in regulating adaptive immunity (8, 9). NK cells can destroy target cells either directly or via antibody-dependent cellular cytotoxicity (ADCC) in the absence of antigen priming (10). NK cell-mediated cytotoxicity may initiate robust adaptive immune responses via CD8+ T cell priming, antigen-specific CD4+ T cell response, and humoral responses (11). NK cells also secrete cytokines and chemokines, which regulate dendritic cells, macrophages, and neutrophils, as well as antigen-specific T cell and B cell function (9, 12, 13).
NK cells express various activating and inhibitory receptors that interact with the ligands on target cells (9). The balance between activating and inhibitory signals of NK cells determines NK cell activation or tolerance (14). In classical education (also known as NK licensing), naive hyporesponsive NK cells learn to recognize MHC class I molecules as “self” (15). This knowledge of “self” allows NK cells to activate when target cells are missing MHC ligands. Killer cell immunoglobulin-like receptors (KIR) are a major group of human NK inhibitory receptors for HLA class I molecules. Interaction of NK inhibitory cell receptors KIR2DL4 and CD94 (NKG2A) with non-classical class I molecules HLA-G and HLA-E at the fetomaternal interface results in maternal immune tolerance during pregnancy (16) (Figure 1). Activating human NK cell receptors include members of KIR family, NKG2D, natural cytotoxicity receptors such as NKp30, NKP44, NKp46, and the nectin/nectin-like binding receptors DNAM-1 and CRTAM, which are responsible for initiating activating signals (17, 18) (Figure 1).
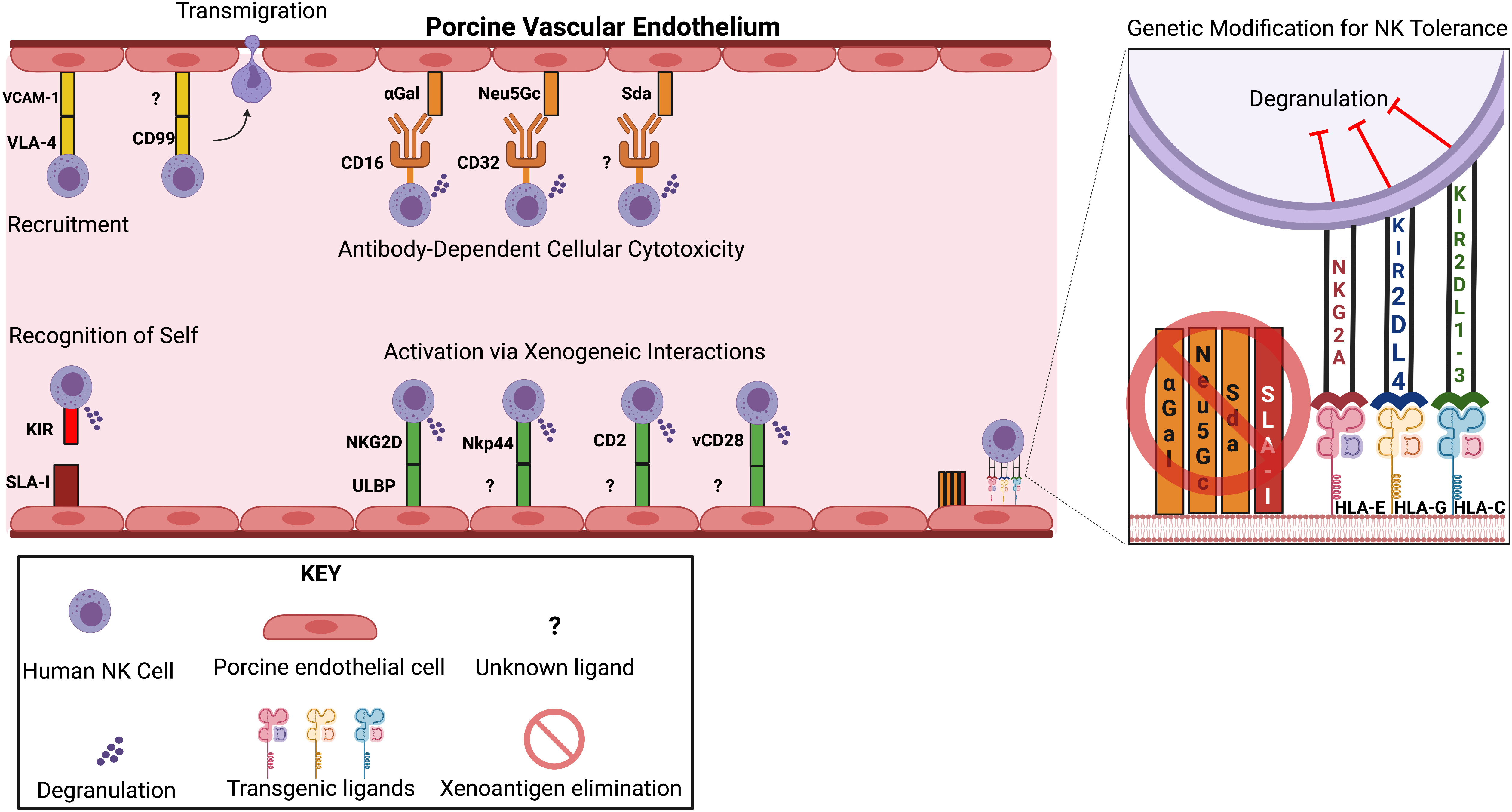
Figure 1 Genetic Modifications that Reduce NK Cell-directed Cytotoxicity. Top left: Recruitment occurs due to adhesive interactions between endothelial ligands and NK cell receptors. Transmigration is mediated by interactions between CD99 and unknown ligands on porcine endothelial cells. Top middle: Antibody-dependent cellular cytotoxicity (ADCC) present upon NK cell recognition of preformed IgG antibodies directed against the xenoantigens αGal, Neu5Gc, and Sda. Bottom left: Failed self-recognition due to non-homology between SLA I and HLA I molecules. Bottom middle: NK cell receptor activation results from interactions with unknown porcine ligands. Right: Summary of current genetic modification proposed to reduce NK cell-mediated cytotoxicity.
NK cells play a crucial role in influencing immune responses to solid organ allografts. Activated NK cells can kill allogeneic target cells and secrete immunomodulatory chemokines and cytokines, contributing to either rejection or tolerance (19).
In this review, we focus on (i) the dual function of NK cells in rejection and tolerance in allotransplantation, (ii) the state of current research regarding genetic modifications to promote NK cell tolerance in xenotransplantation, and (iii) promising future directions to advance xenotransplantation to the clinical reality.
NK cells in allotransplantation
Within days of solid organ transplantation, NK cell infiltration has been observed in allografts (20). Historically, acute rejection episodes have been characterized by an increased number of circulating cytotoxic NK cells (21). NK cells are primarily responsible for augmenting the immune response by secreting key pro-inflammatory cytokines, such as TNF-α and INF-γ (22) and recruiting activated lymphocytes (23). Although T cells are the dominant cell type in allograft rejection, fully activated NK cells have been implicated in allograft rejection in the absence of T cells and B cells in mice (24). The early recruitment of immune cells to the graft via NK cell cytokine secretion can propagate the acute rejection process, linking the innate and adaptive immune responses (25). NK cell facilitation of rejection is evidenced by simultaneous and significantly elevated expression of NKG2D and its ligands during acute cardiac allograft rejection in mice (26).
Beyond acute rejection, the role of NK cells in chronic rejection has been uncovered in multiple studies. For example, depletion of mouse NK cells by anti-NK1.1 monoclonal antibodies prevented early phase cardiac allograft vasculopathy (CAV) (27). In a RAG1 -/- murine model (deficient in T and B cells), NK-induced CAV lesions were restored when wild-type helper T (Th) cells were transferred to the recipient, suggesting that NK cells may play a role in chronic rejection in a Th cell-dependent manner (19, 28). A similar role in chronic rejection was noted in a murine lung transplant model as the use of an anti-NK1.1 monoclonal antibody attenuated bronchiolitis obliterans, a form of chronic lung transplant rejection (29). In a retrospective immunogenetic study of human cadaveric kidney allografts, lacking inhibitory combinations of functional recipient KIR and donor HLA ligand led to a higher risk of chronic rejection (30). Collectively these findings support the conclusion that the presence of NK cells promotes chronic allograft rejection. Thus, inhibiting NK cell activation could be beneficial in improving allograft survival.
Additionally, NK cells promote kidney graft rejection independently of immunosuppressant cyclosporine A therapy, which blocks T cell activation through IL-2 inhibition (31). Due to the varying mechanisms of action of immunosuppressants, uniform influence on NK cell cytotoxicity is not seen. Immunosuppressants limit NK cell functions, such as IFN‐γ production, expression of activation/inhibitory and adhesion markers, antibody-dependent cell-mediated cytotoxicity (ADCC), and NK cell proliferation in different manners to different degrees (32). However, identifying potent inhibitors of NK cell functions (methylprednisone and intravenous immunoglobulin) has important implications for their use in promoting xenotransplant acceptance (32).
The role of NK cells in allograft tolerance is more complex, especially given that both recipient and donor NK cells contribute to allograft rejection and tolerance (33–35). Depletion of recipient NK cells has been reported to prolong allograft survival in a murine liver transplantation model (23). Conversely, in a mouse skin allograft model, recipient NK cells were shown to target and destroy donor antigen-presenting cells, ultimately leading to improved skin allograft tolerance (36). A human lung allotransplantation study also showed these opposing actions of rejection and tolerance (37). Given the array of NK cell functions in acute and chronic allograft rejection, a thorough understanding of their precise roles will be essential to designing an immunologically optimized porcine xenograft.
NK cells in xenotransplantation
Direct contact of human blood with porcine vascular endothelial cells leads to pig endothelial cell injury and secretion of cytokines and chemokines (6, 38, 39). Adhesion molecules, including platelet endothelial cell adhesion molecule, E-selectin (CD62E), P-selectin (CD62P), and vascular cell adhesion molecule-1 (VCAM-1), are upregulated on activated porcine endothelial cells (pEC), and function in recruiting human NK cells (6, 40). Studies showed that interactions of porcine VCAM-1 with human very late antigen-4 (VLA-4) facilitated human NK cell adhesion to pEC (41, 42), and later transendothelial migration was mediated CD99 (43). Manipulating the VCAM-1/integrin adhesion pathway is impractical as the deletion of VCAM-1 was lethal in mice (44).
Preformed human anti-pig antibodies binding to xenoantigens on porcine vascular endothelium initiates the hyperacute xenograft rejection cascade (45, 46). ADCC is a major mechanism for NK cell-mediated cytotoxicity through Fc-receptors (CD16a) binding to IgG1 and IgG3 on pEC and releasing lytic granules (47–49). The αGal-knockout (KO) in pEC line has been shown to significantly suppress ADCC (50, 51). Additional elimination of N-glycolylneuraminic acid (Neu5Gc) (52) and Sda (product of β-1,4 N-acetylgalactosaminyl transferase) (53, 54) carbohydrates and SLA-I xenoantigen in pECs may further inhibit NK cell-mediated ADCC (51). The pivotal work in reducing NK cell hyperacute xenograft rejection is well summarized by Puga Yung et al. (6).
Several studies have been conducted to evaluate the role of NK cells in acute xenograft rejection. In a pig-to-nonhuman primate (NHP) preclinical cardiac xenotransplantation with antibody depletion, Itescu et al. observed greater levels of NK cell and macrophage infiltration in xenografts than in allografts when antibodies were depleted (55), suggesting xenografts experience a more robust cellular immune response even in the absence of antibody-mediated rejection. In a guinea pig-to-rat cardiac xenotransplantation study, continuous depletion of NK cells can prolong xenograft survival (56), further indicating that the absence of NK cells may promote long-term xenograft acceptance. An early and transiently elevated IFN-γ was recognized in pig-to-NHP heart and kidney xenotransplantation when retrospective analyses of NHP sera were performed. Further study indicated that NHP NK cells are the source of early IFN-γ upon the stimulation by both wild-type and αGal-KO pEC in vitro, which could amplify inflammation (57). Taken together, these findings suggest that xenografts experience profound cellular rejection, and NK cells play a pivotal role in both executing and amplifying this response.
Given the importance of NK cells in acute xenograft rejection, the interspecies receptor-ligand interaction has been investigated with the goal of manipulating porcine ligands to prevent human NK cell cytotoxicity. Three HLA class I molecules were expressed in an immortalized porcine bone marrow-derived endothelial cell line. HLA-Cw3 provided substantial protection against cytotoxicity of several NK clones, but HLA-A2 and HLA-B27 provided no protection (58). The co-expression of HLA-Cw3 and HLA-Cw4 did not offer additional benefit than when each was expressed alone (59).
The inhibitory role of swine leukocyte antigen-I (SLA-I) towards human NK cells has been investigated in the past with mixed findings. Sullivan et al. reported that SLA-I is unable to efficiently transmit inhibitory signals to human NK cells because amino acid residues critical for the binding to human NK cell inhibitory receptors are altered in SLA-I when compared to HLA class I (60). In contrast, Kwiatkowski et al. found that induction of SLA-I expression on porcine endothelium by TNF-α reduced human NK cell-mediated cytotoxicity (41). Hein et al. reported that decreased SLA-I expression (SLA-I low) did not decrease NK cell activation (61). A recent study demonstrated that SLA-I is not an inhibitory ligand for human NK cells using an immortalized pEC with SLA-I gene disruption (5). The absence of inhibitory ligands on pEC triggers human NK cell destruction.
Future directions
Xenotransplantation differs from allotransplantation in that preventing rejection can be achieved through genetic modification of the donor pig, improving donor-recipient compatibility. A thorough understanding of the NK cell tolerance mechanisms is necessary for developing novel strategies to mitigate NK cell activation in xenotransplantation. To overcome NK cell-mediated acute xenograft rejection, future directions may require emulating the tolerance strategies used at the maternal-fetal interface and the evasion mechanisms employed by viruses (Table 1).
Introducing inhibitory ligands in pig organs
An example of natural human tolerance is maternal acceptance of the fetus during pregnancy. NK cells constitute the largest proportion of immune cells present in the post-conception endometrium and play a vital role in establishing maternal-fetal immune tolerance. Unique co-expression of fetal HLA-C, HLA-E, and HLA-G in extravillous trophoblasts (EVT) provides inhibitory signals to NK cells (Figure 2) (22). Previous studies demonstrated that reduced expression of inhibitory receptors (i.e., KIR2DL1, KIR2DL2, and KIR2DL3) was associated with recurrent spontaneous abortion (62) and that disruption of the NKG2A/HLA-E axis can lead to adverse fetal outcomes in a murine model (63). This specific in-utero environment provides a model for xenotransplantation as the presence of MHC Class I molecules induce an immunotolerant phenotype of maternal NK cells.
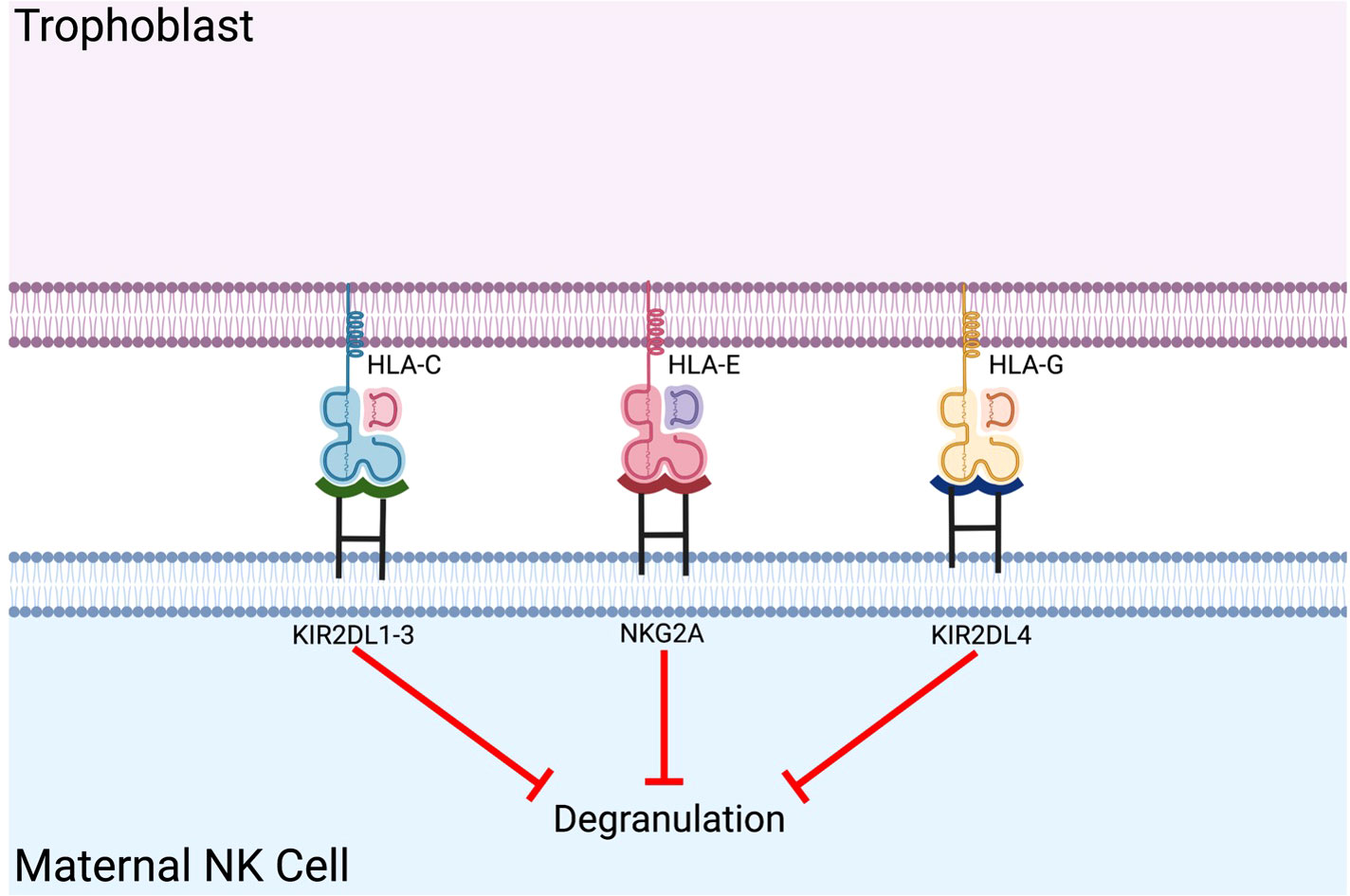
Figure 2 Co-expression of fetal HLA-C, HLA-E, and HLA-G in extravillous trophoblasts provides inhibitory signals to maternal NK cells. Maternal NK cell tolerance is established via expression of fetal classical and non-classical HLA class I molecules on the placental extravillous trophoblast.
Genetically-modified pECs expressing HLA-E demonstrated partial protection from human NK cytotoxicity, and this effect was most pronounced in NK cells with high NKG2A expression (64). This protection was later reproduced in pECs isolated from a double HLA-E β2M transgenic pig (65), confirming the utility of this genetic modification. In an ex vivo porcine limb perfusion study with human blood using transgenic pigs co-expressing HLA-E and human CD46 (HLA-E/hCD46), decreased NK cell tissue infiltration was observed (66). Moreover, in vitro NK cell-mediated lysis of pECs from HLA-E/hCD46 transgenic pig was significantly reduced compared to pECs from both wild-type and hCD46 alone (66). As a result of transgenic HLA-E expression, ex vivo perfusion models have also demonstrated increased survival of porcine lungs (67) and improved function of porcine hearts (68). The ability of HLA-E expression to improve graft compatibility across different organs suggests a generalizable mechanism of immune protection, making it a particularly useful genetic modification for optimizing organ donor pigs.
Expressing HLA-G in porcine aortic endothelial cells inhibits xenogeneic human NK cell-mediated cytotoxicity (69). Dose-dependent soluble HLA-G1 has also been shown to reduce human NK cell-mediated cytotoxicity towards pEC (70). In another study, co-transfection with human β2M enhanced the level of HLA-G1, which led to a significant reduction of NK cell-mediated pEC lysis compared to the HLA-G3 isoform (71)
Given the substantial evidence that both HLA-E and HLA-G expression reduce human NK cell-mediated cytotoxicity, co-expression of HLA-E and HLA-G in xenografts may provide immunoprotection and should be further studied (72). Matsunami et al. discussed the effects of co-transfection of HLA-E and HLA-G into pECs while evaluating NK cell response. Though differences were noted across leader peptides and expression rate, substantial NK cell inhibition was noted in HLA-E and HLA-G co-expression compared to either alone. This synergistic relationship between HLA-E and HLA-G demonstrates the potential use in xenografts (73).
An additional model to reduce NK cell cytotoxicity is noted in viral proteins that increase inhibitory signaling in efforts to circumvent immune destruction. Herpesviridae, a family which includes herpes simplex virus (HSV) and human cytomegalovirus (HCMV), have adapted several unique mechanisms centered around decreasing activating and increasing inhibitory receptor-ligand interactions to evade immune detection by NK cells (74). HCMV evades NK cell destruction by amplifying inhibitory signaling via the expression of UL40 and gpUL18 in the endoplasmic reticulum of infected cells (75). UL40-bound HLA-E binds to the NK cell inhibitory receptor NKG2A with greater affinity (6-fold more tightly) than HLA-E alone (Figure 3) (76). Similarly, gpUL18, an MHC I decoy ligand, binds to the inhibitory NK cell receptor LIR-1 1000-fold more tightly than native MHC I molecules, resulting in a significant reduction in NK cell cytotoxicity (Figure 3) (77–79). The combination of enhanced cell-surface expression of HLA-E and tighter binding to NKG2A results in a marked decrease in NK cell cytotoxicity (80, 81). Repurposing the success of HCMV by expressing these unique viral peptides in xenograft cell lines may induce NK cell tolerance via amplification of inhibitory signals (Table 1).
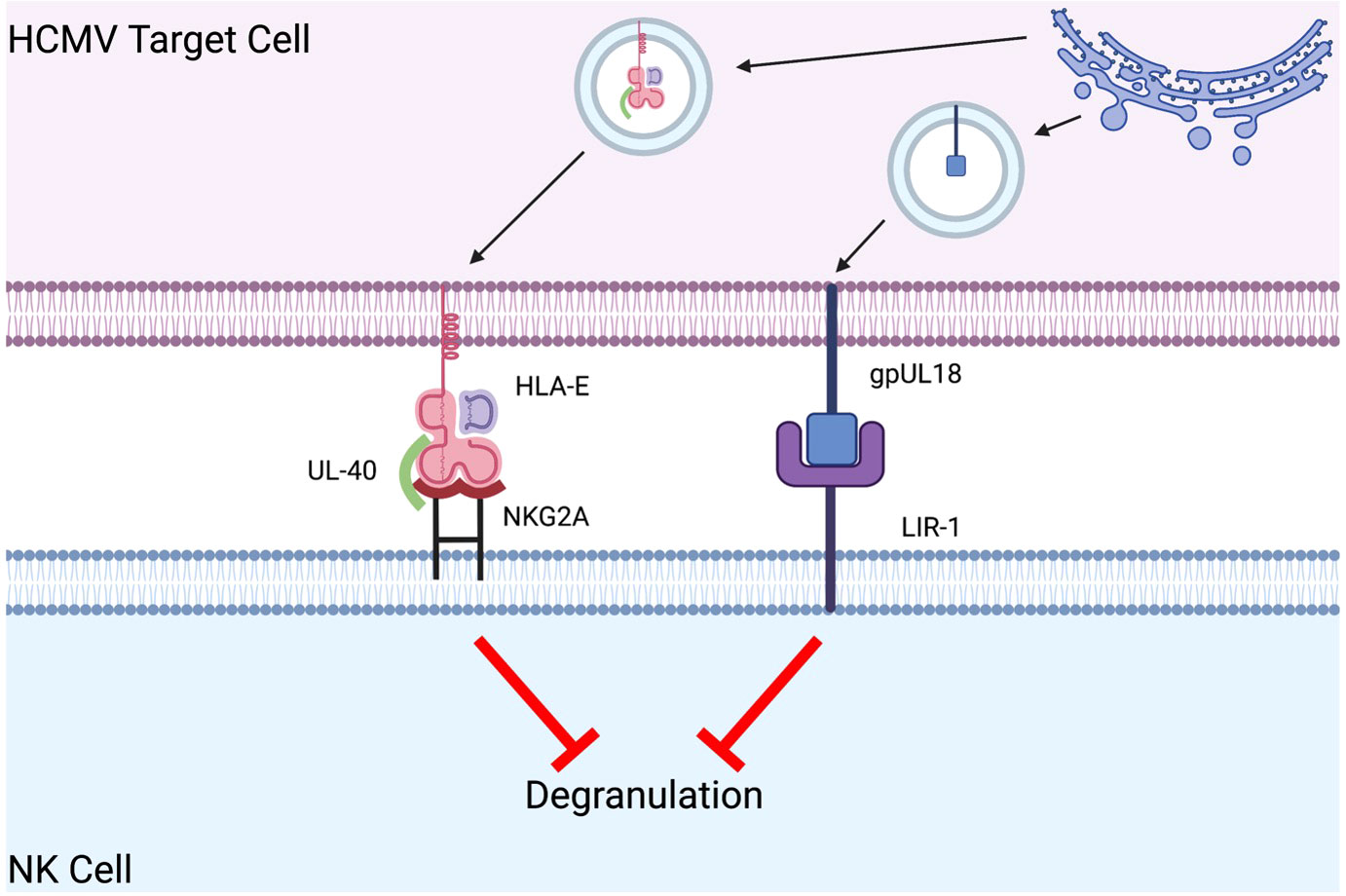
Figure 3 Human CMV evades NK cell destruction by amplifying inhibitory signaling. In human CMV (HCMV) infected target cells, UL40-bound HLA-E bind six-fold greater affinity to the NK cell inhibitory receptor NKG2A than HLA-E alone. HCMV gpUL18 is an MHC I decoy ligand that binds to the inhibitory NK cell receptor LIR-1 with a 1000-fold greater affinity than native MHC I molecules. These interactions result in a significant reduction in NK cell cytotoxicity.
Eliminating activating ligands in pig organs
Another strategy to reduce human NK cell cytotoxicity is eliminating ligands that bind to human NK cell activating receptors. Blocking of activating ligands is a strategy used by HSV-infected cells to downregulate the expression of several ligands of the activating NK cell receptor NKG2D, including MICB (major histocompatibility complex class I polypeptide-related sequence B) and UL-16 binding proteins (ULBP1,2,3) (82, 83). HSV-infected cells transcribe miR-H8, a microRNA that prevents anchoring of NKG2D-activating ligands such as ULBP-2 and ULBP-3 to the cell surface (74, 84–86). HCMV achieves a similar result by expressing the virally encoded UL-16 protein that binds to and sequesters MICB, ULBP1, and ULBP2 (87). Efforts to mimic these mechanisms may provide an approach to promote xenograft acceptance. Nevertheless, findings surrounding these ligand-receptor interactions are still unclear, and further experimentation is necessary.
Lilienfeld et al. confirmed porcine ULBP1 as a ligand for human NKG2D through anti-porcine ULBP1 polyclonal antibody studies (88). Tran et al. subsequently demonstrated additional unknown porcine ligands to NKG2D (89). After generating a ULBP1-KO on a 5GKO pEC line (ULBP1KO/5GKO), our laboratory concluded that porcine ULBP1 is not the dominant ligand for NKG2D as its deletion did not offer a statistically significant decrease in NK cell activation (90). Additional studies are needed to identify functional porcine ligands to human NKG2D in pig cells.
Glycoprotein D of HSV and pseudorabies virus downregulates CD112 expression, a ligand for the activating NK cell receptor DNAM-1, which results in decreased NK cell activation and reduced cytotoxicity (18, 91). Two porcine isoforms of CD112 have been reported (92), but further study is needed to evaluate the role of porcine CD112 in human NK cell activation. Discovery and deletion of porcine activating ligands could promote human NK cell tolerance (Table 1). Expressing viral proteins in pigs to reduce activating ligands may represent a complementary approach to inhibiting NK cell-mediated cytotoxicity.
When evaluating an array of activating NK cell receptors (NKp46, 2B4, CD49d, CD48, CD2, and NKG2D), Kim et al. discovered that only CD2 and NKG2D were involved in both cytotoxicity and cytokine-secreting functions against porcine cells (93). It is important to note that complete protection against NK cell-mediated cytotoxicity was only obtained when combinations of activating receptors (NKp44 and NKG2D or CD2 and NKG2D) were blocked (93). Structural protein modeling of human CD2 and porcine CD58 demonstrated a highly conserved interface (94). In fact, blocking with an anti-porcine-CD58 antibody inhibited lysis of porcine cells (95), indicating porcine CD58 is a potential activating ligand for modification. Genetic modifications aimed at elimination of activating ligands will require extensive research to identify the most potent receptor-ligand interactions. Simultaneously maximizing inhibitory signaling will be important to induce NK cell tolerance in xenotransplantation.
Conclusions
NK cells play a pivotal role in acute xenograft rejection, yet relatively few attempts have been made to overcome this barrier. Inducing human NK cell tolerance by expressing inhibitory ligands and eliminating activating ligands in porcine xenografts via genetic engineering approach may provide a novel way to protect xenografts from NK cell-mediated destruction. Understanding the mechanism of cross-species immune recognition and response, developing a novel approach to induce local immune tolerance/acceptance, and guiding the engineering of pigs to meet clinical needs will be our future focus in xenotransplantation.
Author contributions
BE and PL provided critical guidance in the concept and design of the review paper. KL and AC-N wrote the initial draft of the manuscript. KL and AC-N designed the figures and tables. All authors participated in a critical review of the manuscript. All authors approved the final manuscript.
Funding
Work on xenotransplantation at Indiana University has been supported by internal funds of the Department of Surgery, in part, with support by the Board of Directors of the Indiana University Health Values Fund for Research Award (VFR-457-Ekser), the Indiana Clinical and Translational Sciences Institute, funded in part by Grant # UL1TR001108 from the National Institutes of Health (NIH), National Center for Advancing Translational Sciences, Clinical and Translational Sciences Award, and NIH NIAID R21AI164002 (PL).
Conflict of interest
The authors declare that the research was conducted in the absence of any commercial or financial relationships that could be construed as a potential conflict of interest.
Publisher’s note
All claims expressed in this article are solely those of the authors and do not necessarily represent those of their affiliated organizations, or those of the publisher, the editors and the reviewers. Any product that may be evaluated in this article, or claim that may be made by its manufacturer, is not guaranteed or endorsed by the publisher.
Abbreviations
αGal, galactose-α(1,3)galactose; AXR, Acute xenograft rejection; β4galNT2, Beta-1,4-N-Acetyl-Galactosaminyltransferase 2; CMAH, Cytidine monophospho-N-acetylneuraminic acid hydroxylase; GGTA1, Alpha-1,3-galactosyltransferase; HLA, Human leukocyte antigen; HXR, Hyperacute xenograft rejection; MHC, Major histocompatibility complex; Neu5Gc, N-glycolylneuraminic acid; NK Cells, Natural Killer Cells; PBMC, Peripheral blood mononuclear cells; pEC, Porcine endothelial cells; SLA, Swine leukocyte antigen; 5GKO, GGTA1/CMAH/β4galNT2/SLA-Iα/β2-microglobulin KO.
References
1. Wang X. Bioartificial organ manufacturing technologies. Cell Transplant (2019) 28(1):5–17. doi: 10.1177/0963689718809918
2. Murphy SV, Atala A. 3d bioprinting of tissues and organs. Nat Biotechnol (2014) 32(8):773–85. doi: 10.1038/nbt.2958
3. Lutz AJ, Li P, Estrada JL, Sidner RA, Chihara RK, Downey SM, et al. Double knockout pigs deficient in n-glycolylneuraminic acid and galactose α-1,3-Galactose reduce the humoral barrier to xenotransplantation. Xenotransplantation (2013) 20(1):27–35. doi: 10.1111/xen.12019
4. Estrada JL, Martens G, Li P, Adams A, Newell KA, Ford ML, et al. Evaluation of human and non-human primate antibody binding to pig cells lacking Ggta1/Cmah/β4galnt2 genes. Xenotransplantation (2015) 22(3):194–202. doi: 10.1111/xen.12161
5. Li P, Walsh JR, Lopez K, Isidan A, Zhang W, Chen AM, et al. Genetic engineering of porcine endothelial cell lines for evaluation of human-to-Pig xenoreactive immune responses. Sci Rep (2021) 11(1):13131. doi: 10.1038/s41598-021-92543-y
6. Puga Yung G, Schneider MKJ, Seebach JD. The role of nk cells in pig-to-Human xenotransplantation. J Immunol Res (2017) 2017:4627384. doi: 10.1155/2017/4627384
7. Griffith B. P., Goerlich C. E., Singh A. K., Rothblatt M., Lau C.L., Shah A, et al. Genetically Modified Porcine-to-Human Cardiac Xenotransplantation. N Engl J Med (2022) 387(1):35–44. doi: 10.1056/NEJMoa2201422
8. Moretta L, Bottino C, Cantoni C, Mingari MC, Moretta A. Human natural killer cell function and receptors. Curr Opin Pharmacol (2001) 1(4):387–91. doi: 10.1016/s1471-4892(01)00067-4
9. Vivier E, Raulet DH, Moretta A, Caligiuri MA, Zitvogel L, Lanier LL, et al. Innate or adaptive immunity? the example of natural killer cells. Science (2011) 331(6013):44–9. doi: 10.1126/science.1198687
10. Lanier LL. Nk cell recognition. Annu Rev Immunol (2005) 23:225–74. doi: 10.1146/annurev.immunol.23.021704.115526
11. Krebs P, Barnes MJ, Lampe K, Whitley K, Bahjat KS, Beutler B, et al. Nk-Cell-Mediated killing of target cells triggers robust antigen-specific T-Cell-Mediated and humoral responses. Blood (2009) 113(26):6593–602. doi: 10.1182/blood-2009-01-201467
12. Walzer T, Dalod M, Robbins SH, Zitvogel L, Vivier E. Natural-killer cells and dendritic cells: "L'union fait la force". Blood (2005) 106(7):2252–8. doi: 10.1182/blood-2005-03-1154
13. Moretta A, Marcenaro E, Sivori S, Della Chiesa M, Vitale M, Moretta L. Early liaisons between cells of the innate immune system in inflamed peripheral tissues. Trends Immunol (2005) 26(12):668–75. doi: 10.1016/j.it.2005.09.008
14. Orr MT, Lanier LL. Natural killer cell education and tolerance. Cell (2010) 142(6):847–56. doi: 10.1016/j.cell.2010.08.031
15. Anfossi N, André P, Guia S, Falk CS, Roetynck S, Stewart CA, et al. Human nk cell education by inhibitory receptors for mhc class I. Immunity (2006) 25(2):331–42. doi: 10.1016/j.immuni.2006.06.013
16. Bai Y, Liang J, Liu W, Wang F, Li C. Possible roles of hla-G regulating immune cells in pregnancy and endometrial diseases Via Kir2dl4. J Reprod Immunol (2020) 142:103176. doi: 10.1016/j.jri.2020.103176
17. Moretta L, Moretta A. Unravelling natural killer cell function: Triggering and inhibitory human nk receptors. EMBO J (2004) 23(2):255–9. doi: 10.1038/sj.emboj.7600019
18. Grauwet K, Cantoni C, Parodi M, De Maria A, Devriendt B, Pende D, et al. Modulation of Cd112 by the alphaherpesvirus gd protein suppresses dnam-1-Dependent nk cell-mediated lysis of infected cells. Proc Natl Acad Sci U.S.A. (2014) 111(45):16118–23. doi: 10.1073/pnas.1409485111
19. Benichou G, Yamada Y, Aoyama A, Madsen JC. Natural killer cells in rejection and tolerance of solid organ allografts. Curr Opin Organ Transplant (2011) 16(1):47–53. doi: 10.1097/MOT.0b013e32834254cf
20. Baldwin WM 3rd, Larsen CP, Fairchild RL. Innate immune responses to transplants: A significant variable with cadaver donors. Immunity (2001) 14(4):369–76. doi: 10.1016/s1074-7613(01)00117-0
21. Cooksey G, Robins RA, Blamey RW. Natural killer cells in renal allograft rejection. Br J Surg (1984) 71(11):874–7. doi: 10.1002/bjs.1800711124
22. Szekeres-Bartho J. Regulation of nk cell cytotoxicity during pregnancy. Reprod BioMed Online (2008) 16(2):211–7. doi: 10.1016/s1472-6483(10)60576-7
23. Obara H, Nagasaki K, Hsieh CL, Ogura Y, Esquivel CO, Martinez OM, et al. Ifn-gamma, produced by nk cells that infiltrate liver allografts early after transplantation, links the innate and adaptive immune responses. Am J Transplant (2005) 5(9):2094–103. doi: 10.1111/j.1600-6143.2005.00995.x
24. Kroemer A, Xiao X, Degauque N, Edtinger K, Wei H, Demirci G, et al. The innate nk cells, allograft rejection, and a key role for il-15. J Immunol (2008) 180(12):7818–26. doi: 10.4049/jimmunol.180.12.7818
25. Kondo T, Morita K, Watarai Y, Auerbach MB, Taub DD, Novick AC, et al. Early increased chemokine expression and production in murine allogeneic skin grafts is mediated by natural killer cells. Transplantation (2000) 69(5):969–77. doi: 10.1097/00007890-200003150-00051
26. Feng L, Ke N, Ye Z, Guo Y, Li S, Li Q, et al. Expression of Nkg2d and its ligand in mouse heart allografts may have a role in acute rejection. Transplant Proc (2009) 41(10):4332–9. doi: 10.1016/j.transproceed.2009.08.060
27. Hirohashi T, Chase CM, DellaPelle P, Sebastian D, Farkesh E, Colvin RB, et al. Depletion of T regulatory cells promotes natural killer cell-mediated cardiac allograft vasculopathy. Transplantation (2014) 98(8):828–34. doi: 10.1097/tp.0000000000000329
28. Uehara S, Chase CM, Kitchens WH, Rose HS, Colvin RB, Russell PS, et al. Nk cells can trigger allograft vasculopathy: The role of hybrid resistance in solid organ allografts. J Immunol (2005) 175(5):3424–30. doi: 10.4049/jimmunol.175.5.3424
29. Kawakami T, Ito K, Matsuda Y, Noda M, Sakurada A, Hoshikawa Y, et al. Cytotoxicity of natural killer cells activated through Nkg2d contributes to the development of bronchiolitis obliterans in a murine heterotopic tracheal transplant model. Am J Transplant (2017) 17(9):2338–49. doi: 10.1111/ajt.14257
30. Littera R, Piredda G, Argiolas D, Lai S, Congeddu E, Ragatzu P, et al. Kir and their hla class I ligands: Two more pieces towards completing the puzzle of chronic rejection and graft loss in kidney transplantation. PloS One (2017) 12(7):e0180831. doi: 10.1371/journal.pone.0180831
31. Ashraf MI, Sarwar A, Kühl AA, Hunger E, Sattler A, Aigner F, et al. Natural killer cells promote kidney graft rejection independently of cyclosporine a therapy. Front Immunol (2019) 10:2279. doi: 10.3389/fimmu.2019.02279
32. Pradier A, Papaserafeim M, Li N, Rietveld A, Kaestel C, Gruaz L, et al. Small-molecule immunosuppressive drugs and therapeutic immunoglobulins differentially inhibit nk cell effector functions in vitro. Front Immunol (2019) 10:556. doi: 10.3389/fimmu.2019.00556
33. Harmon C, Sanchez-Fueyo A, O'Farrelly C, Houlihan DD. Natural killer cells and liver transplantation: Orchestrators of rejection or tolerance? Am J Transplant (2016) 16(3):751–7. doi: 10.1111/ajt.13565
34. Huang H, Lu Y, Zhou T, Gu G, Xia Q. Innate immune cells in immune tolerance after liver transplantation. Front Immunol (2018) 9:2401. doi: 10.3389/fimmu.2018.02401
35. Hennessy C, Lewik G, Cross A, Hester J, Issa F. Recent advances in our understanding of the allograft response. Fac Rev (2021) 10:21. doi: 10.12703/r/10-21
36. Yu G, Xu X, Vu MD, Kilpatrick ED, Li XC. Nk cells promote transplant tolerance by killing donor antigen-presenting cells. J Exp Med (2006) 203(8):1851–8. doi: 10.1084/jem.20060603
37. Calabrese DR, Lanier LL, Greenland JR. Natural killer cells in lung transplantation. Thorax (2019) 74(4):397–404. doi: 10.1136/thoraxjnl-2018-212345
38. Gilli UO, Schneider MK, Loetscher P, Seebach JD. Human polymorphonuclear neutrophils are recruited by porcine chemokines acting on cxc chemokine receptor 2, and platelet-activating factor. Transplantation (2005) 79(10):1324–31. doi: 10.1097/01.tp.0000155429.44902.44
39. Goodman DJ, Von Albertini M, Willson A, Millan MT, Bach FH. Direct activation of porcine endothelial cells by human natural killer cells. Transplantation (1996) 61(5):763–71. doi: 10.1097/00007890-199603150-00016
40. Schneider MK, Strasser M, Gilli UO, Kocher M, Moser R, Seebach JD. Rolling adhesion of human nk cells to porcine endothelial cells mainly relies on Cd49d-Cd106 interactions. Transplantation (2002) 73(5):789–96. doi: 10.1097/00007890-200203150-00023
41. Kwiatkowski P, Artrip JH, John R, Edwards NM, Wang SF, Michler RE, et al. Induction of swine major histocompatibility complex class I molecules on porcine endothelium by tumor necrosis factor-alpha reduces lysis by human natural killer cells. Transplantation (1999) 67(2):211–8. doi: 10.1097/00007890-199901270-00005
42. Zhang XF, Feng MF. Adherence of human monocytes and nk cells to human tnf-Alpha-Stimulated porcine endothelial cells. Immunol Cell Biol (2000) 78(6):633–40. doi: 10.1046/j.1440-1711.2000.00970.x
43. Schneider MK, Ghielmetti M, Rhyner DM, Antsiferova MA, Seebach JD. Human leukocyte transmigration across Galalpha(1,3)Gal-negative porcine endothelium is regulated by human Cd18 and Cd99. Transplantation (2009) 87(4):491–9. doi: 10.1097/TP.0b013e318195fb8d
44. Kwee L, Baldwin HS, Shen HM, Stewart CL, Buck C, Buck CA, et al. Defective development of the embryonic and extraembryonic circulatory systems in vascular cell adhesion molecule (Vcam-1) deficient mice. Development (1995) 121(2):489–503. doi: 10.1242/dev.121.2.489
45. Watier H, Guillaumin JM, Vallée I, Thibault G, Gruel Y, Lebranchu Y, et al. Human nk cell-mediated direct and igg-dependent cytotoxicity against xenogeneic porcine endothelial cells. Transpl Immunol (1996) 4(4):293–9. doi: 10.1016/s0966-3274(96)80050-5
46. Seebach J, Yamada K, McMorrow I, Sachs D, DerSimonian H. Xenogeneic human anti-pig cytotoxicity mediated by activated natural killer cells. Xenotransplantation (1996) 3(2):188–97.
47. Sulica A, Morel P, Metes D, Herberman RB. Ig-binding receptors on human nk cells as effector and regulatory surface molecules. Int Rev Immunol (2001) 20(3-4):371–414. doi: 10.3109/08830180109054414
48. Resch T, Fabritius C, Ebner S, Ritschl P, Kotsch K. The role of natural killer cells in humoral rejection. Transplantation (2015) 99(7):1335–40. doi: 10.1097/tp.0000000000000757
49. Kumagai-Braesch M, Satake M, Qian Y, Holgersson J, Möller E. Human nk cell and adcc reactivity against xenogeneic porcine target cells including fetal porcine islet cells. Xenotransplantation (1998) 5(2):132–45. doi: 10.1111/j.1399-3089.1998.tb00019.x
50. Galili U, Macher BA, Buehler J, Shohet SB. Human natural anti-Alpha-Galactosyl igg. ii. the specific recognition of alpha (1–-3)-Linked galactose residues. J Exp Med (1985) 162(2):573–82. doi: 10.1084/jem.162.2.573
51. Maeda A, Kogata S, Toyama C, Lo PC, Okamatsu C, Yamamoto R, et al. The innate cellular immune response in xenotransplantation. Front Immunol (2022) 13:858604. doi: 10.3389/fimmu.2022.858604
52. Park JY, Park MR, Kwon DN, Kang MH, Oh M, Han JW, et al. Alpha 1,3-galactosyltransferase deficiency in pigs increases sialyltransferase activities that potentially raise non-gal xenoantigenicity. J BioMed Biotechnol (2011) 2011:560850. doi: 10.1155/2011/560850
53. Byrne GW, Du Z, Stalboerger P, Kogelberg H, McGregor CG. Cloning and expression of porcine β1,4 n-acetylgalactosaminyl transferase encoding a new xenoreactive antigen. Xenotransplantation (2014) 21(6):543–54. doi: 10.1111/xen.12124
54. Byrne GW, McGregor CGA, Breimer ME. Recent investigations into pig antigen and anti-pig antibody expression. Int J Surg (2015). doi: 10.1016/j.ijsu.2015.07.724
55. Itescu S, Kwiatkowski P, Artrip JH, Wang SF, Ankersmit J, Minanov OP, et al. Role of natural killer cells, macrophages, and accessory molecule interactions in the rejection of pig-to-Primate xenografts beyond the hyperacute period. Hum Immunol (1998) 59(5):275–86. doi: 10.1016/s0198-8859(98)00026-3
56. Xia G, Ji P, Rutgeerts O, Waer M. Natural killer cell- and macrophage mediated discordant Guinea pig–>Rat xenograft rejection in the absence of complement, xenoantibody and T cell immunity. Transplantation (2000) 70(1):86–93.
57. Park EM, Lee H, Kang HJ, Oh KB, Kim JS, Chee HK, et al. Early interferon-gamma response in nonhuman primate recipients of solid-organ xenotransplantation. Transplant Proc (2021) 53(10):3093–100. doi: 10.1016/j.transproceed.2021.09.028
58. Seebach JD, Comrack C, Germana S, LeGuern C, Sachs DH, DerSimonian H. Hla-Cw3 expression on porcine endothelial cells protects against xenogeneic cytotoxicity mediated by a subset of human nk cells. J Immunol (1997) 159(7):3655–61.
59. Forte P, Baumann BC, Schneider MK, Seebach JD. Hla-Cw4 expression on porcine endothelial cells reduces cytotoxicity and adhesion mediated by Cd158a+ human nk cells. Xenotransplantation (2009) 16(1):19–26. doi: 10.1111/j.1399-3089.2009.00510.x
60. Sullivan JA, Oettinger HF, Sachs DH, Edge AS. Analysis of polymorphism in porcine mhc class I genes: Alterations in signals recognized by human cytotoxic lymphocytes. J Immunol (1997) 159(5):2318–26.
61. Hein R, Sake HJ, Pokoyski C, Hundrieser J, Brinkmann A, Baars W, et al. Triple (Ggta1, cmah, B2m) modified pigs expressing an sla class I(Low) phenotype-effects on immune status and susceptibility to human immune responses. Am J Transplant (2020) 20(4):988–98. doi: 10.1111/ajt.15710
62. Varla-Leftherioti M, Spyropoulou-Vlachou M, Keramitsoglou T, Papadimitropoulos M, Tsekoura C, Graphou O, et al. Lack of the appropriate natural killer cell inhibitory receptors in women with spontaneous abortion. Hum Immunol (2005) 66(1):65–71. doi: 10.1016/j.humimm.2004.10.005
63. Shreeve N, Depierreux D, Hawkes D, Traherne JA, Sovio U, Huhn O, et al. The Cd94/Nkg2a inhibitory receptor educates uterine nk cells to optimize pregnancy outcomes in humans and mice. Immunity (2021). doi: 10.1016/j.immuni.2021.03.021
64. Forte P, Baumann BC, Weiss EH, Seebach JD. Hla-e expression on porcine cells: Protection from human nk cytotoxicity depends on peptide loading. Am J Transplant (2005) 5(9):2085–93. doi: 10.1111/j.1600-6143.2005.00987.x
65. Weiss EH, Lilienfeld BG, Müller S, Müller E, Herbach N, Kessler B, et al. Hla-E/Human Beta2-microglobulin transgenic pigs: Protection against xenogeneic human anti-pig natural killer cell cytotoxicity. Transplantation (2009) 87(1):35–43. doi: 10.1097/TP.0b013e318191c784
66. Puga Yung G, Bongoni AK, Pradier A, Madelon N, Papaserafeim M, Sfriso R, et al. Release of pig leukocytes and reduced human nk cell recruitment during ex vivo perfusion of hla-E/Human Cd46 double-transgenic pig limbs with human blood. Xenotransplantation (2018) 25(1):1–13. doi: 10.1111/xen.12357
67. Laird CT, Burdorf L, French BM, Kubicki N, Cheng X, Braileanu G, et al. Transgenic expression of human leukocyte antigen-e attenuates Galko.Hcd46 porcine lung xenograft injury. Xenotransplantation (2017) 24(2):1–8. doi: 10.1111/xen.12294
68. Abicht JM, Sfriso R, Reichart B, Längin M, Gahle K, Puga Yung GL, et al. Multiple genetically modified Gtko/Hcd46/Hla-E/Hβ2-Mg porcine hearts are protected from complement activation and natural killer cell infiltration during ex vivo perfusion with human blood. Xenotransplantation (2018) 25(5):e12390. doi: 10.1111/xen.12390
69. Forte P, Matter-Reissmann UB, Strasser M, Schneider MK, Seebach JD. Porcine aortic endothelial cells transfected with hla-G are partially protected from xenogeneic human nk cytotoxicity. Hum Immunol (2000) 61(11):1066–73. doi: 10.1016/s0198-8859(00)00202-0
70. Zeng MH, Fang CY, Wang SS, Zhu M, Xie L, Li R, et al. A study of soluble hla-G1 protecting porcine endothelial cells against human natural killer cell-mediated cytotoxicity. Transplant Proc (2006) 38(10):3312–4. doi: 10.1016/j.transproceed.2006.10.179
71. Matsunami K, Miyagawa S, Nakai R, Murase A, Shirakura R. The possible use of hla-G1 and G3 in the inhibition of nk cell-mediated swine endothelial cell lysis. Clin Exp Immunol (2001) 126(1):165–72. doi: 10.1046/j.1365-2249.2001.01622.x
72. Crew MD. Play it in e or G: Utilization of hla-e and -G in xenotransplantation. Xenotransplantation (2007) 14(3):198–207. doi: 10.1111/j.1399-3089.2007.00395.x
73. Matsunami K, Miyagawa S, Nakai R, Yamada M, Shirakura R. Modulation of the leader peptide sequence of the hla-e gene up-regulates its expression and down-regulates natural killer cell-mediated swine endothelial cell lysis. Transplantation (2002) 73(10):1582–9. doi: 10.1097/00007890-200205270-00010
74. Koyanagi N, Kawaguchi Y. Evasion of the cell-mediated immune response by alphaherpesviruses. Viruses (2020) 12(12):1–16. doi: 10.3390/v12121354
75. Prod'homme V, Tomasec P, Cunningham C, Lemberg MK, Stanton RJ, McSharry BP, et al. Human cytomegalovirus Ul40 signal peptide regulates cell surface expression of the nk cell ligands hla-e and Gpul18. J Immunol (2012) 188(6):2794–804. doi: 10.4049/jimmunol.1102068
76. Llano M, Lee N, Navarro F, García P, Albar JP, Geraghty DE, et al. Hla-E-Bound peptides influence recognition by inhibitory and triggering Cd94/Nkg2 receptors: Preferential response to an hla-G-Derived nonamer. Eur J Immunol (1998) 28(9):2854–63. doi: 10.1002/(sici)1521-4141(199809)28:09<2854::Aid-immu2854>3.0.Co;2-w
77. Reyburn HT, Mandelboim O, Valés-Gómez M, Davis DM, Pazmany L, Strominger JL. The class I mhc homologue of human cytomegalovirus inhibits attack by natural killer cells. Nature (1997) 386(6624):514–7. doi: 10.1038/386514a0
78. Cosman D, Fanger N, Borges L, Kubin M, Chin W, Peterson L, et al. A novel immunoglobulin superfamily receptor for cellular and viral mhc class I molecules. Immunity (1997) 7(2):273–82. doi: 10.1016/s1074-7613(00)80529-4
79. Yang Z, Bjorkman PJ. Structure of Ul18, a peptide-binding viral mhc mimic, bound to a host inhibitory receptor. Proc Natl Acad Sci U.S.A. (2008) 105(29):10095–100. doi: 10.1073/pnas.0804551105
80. De Pelsmaeker S, Romero N, Vitale M, Favoreel HW. Herpesvirus evasion of natural killer cells. J Virol (2018) 92(11):991–1005. doi: 10.1128/jvi.02105-17
81. Ulbrecht M, Martinozzi S, Grzeschik M, Hengel H, Ellwart JW, Pla M, et al. Cutting edge: The human cytomegalovirus Ul40 gene product contains a ligand for hla-e and prevents nk cell-mediated lysis. J Immunol (2000) 164(10):5019–22. doi: 10.4049/jimmunol.164.10.5019
82. Schepis D, D'Amato M, Studahl M, Bergström T, Kärre K, Berg L. Herpes simplex virus infection downmodulates Nkg2d ligand expression. Scand J Immunol (2009) 69(5):429–36. doi: 10.1111/j.1365-3083.2009.02241.x
83. Tognarelli EI, Palomino TF, Corrales N, Bueno SM, Kalergis AM, González PA. Herpes simplex virus evasion of early host antiviral responses. Front Cell Infect Microbiol (2019) 9:127. doi: 10.3389/fcimb.2019.00127
84. Enk J, Levi A, Weisblum Y, Yamin R, Charpak-Amikam Y, Wolf DG, et al. Hsv1 microrna modulation of gpi anchoring and downstream immune evasion. Cell Rep (2016) 17(4):949–56. doi: 10.1016/j.celrep.2016.09.077
85. Kinoshita T. Biosynthesis and deficiencies of glycosylphosphatidylinositol. Proc Jpn Acad Ser B Phys Biol Sci (2014) 90(4):130–43. doi: 10.2183/pjab.90.130
86. Ohishi K, Inoue N, Kinoshita T. Pig-s and pig-T, essential for gpi anchor attachment to proteins, form a complex with Gaa1 and Gpi8. EMBO J (2001) 20(15):4088–98. doi: 10.1093/emboj/20.15.4088
87. Dunn C, Chalupny NJ, Sutherland CL, Dosch S, Sivakumar PV, Johnson DC, et al. Human cytomegalovirus glycoprotein Ul16 causes intracellular sequestration of Nkg2d ligands, protecting against natural killer cell cytotoxicity. J Exp Med (2003) 197(11):1427–39. doi: 10.1084/jem.20022059
88. Lilienfeld BG, Garcia-Borges C, Crew MD, Seebach JD. Porcine Ul16-binding protein 1 expressed on the surface of endothelial cells triggers human nk cytotoxicity through Nkg2d. J Immunol (2006) 177(4):2146–52. doi: 10.4049/jimmunol.177.4.2146
89. Tran PD, Christiansen D, Winterhalter A, Brooks A, Gorrell M, Lilienfeld BG, et al. Porcine cells express more than one functional ligand for the human lymphocyte activating receptor Nkg2d. Xenotransplantation (2008) 15(5):321–32. doi: 10.1111/j.1399-3089.2008.00489.x
90. Lopez K, Isidan A, Cross-Najafi A, Campana G, Zhang W, Ekser B, et al. Disrupting porcine ul-binding protein does not significantly reduce human nk cell activation in an in vitro model. Xenotransplantation (2021) 28(5):21–3. doi: 10.1111/xen.12711
91. Denaeghel S, De Pelsmaeker S, Van Waesberghe C, Favoreel HW. Pseudorabies virus infection causes downregulation of ligands for the activating nk cell receptor Nkg2d. Viruses (2021) 13(2):1–14. doi: 10.3390/v13020266
92. Wang L, Zhang W, Wu DA, Chen C, Xu QZ, Zhao B, et al. Molecular cloning, characterization and three-dimensional modeling of porcine nectin-2/Cd112. Vet Immunol Immunopathol (2009) 132(2-4):257–63. doi: 10.1016/j.vetimm.2009.05.008
93. Kim TJ, Kim N, Kim EO, Choi JR, Bluestone JA, Lee KM. Suppression of human anti-porcine natural killer cell xenogeneic responses by combinations of monoclonal antibodies specific to Cd2 and Nkg2d and extracellular signal-regulated kinase kinase inhibitor. Immunology (2010) 130(4):545–55. doi: 10.1111/j.1365-2567.2010.03253.x
94. Brossay A, Hubé F, Moreau T, Bardos P, Watier H. Porcine Cd58: Cdna cloning and molecular dissection of the porcine Cd58-human Cd2 interface. Biochem Biophys Res Commun (2003) 309(4):992–8. doi: 10.1016/j.bbrc.2003.08.099
Keywords: NK cells, NK cell tolerance, xenotransplant, xenotranplantation, tolerance
Citation: Lopez KJ, Cross-Najafi AA, Farag K, Obando B, Thadasina D, Isidan A, Park Y, Zhang W, Ekser B and Li P (2022) Strategies to induce natural killer cell tolerance in xenotransplantation. Front. Immunol. 13:941880. doi: 10.3389/fimmu.2022.941880
Received: 11 May 2022; Accepted: 28 July 2022;
Published: 22 August 2022.
Edited by:
Aurore Saudemont, GlaxoSmithKline, United KingdomReviewed by:
Jörg Dieter Seebach, Geneva University Hospitals (HUG), SwitzerlandTao Li, Second Affiliated Hospital of Hainan Medical University, China
Copyright © 2022 Lopez, Cross-Najafi, Farag, Obando, Thadasina, Isidan, Park, Zhang, Ekser and Li. This is an open-access article distributed under the terms of the Creative Commons Attribution License (CC BY). The use, distribution or reproduction in other forums is permitted, provided the original author(s) and the copyright owner(s) are credited and that the original publication in this journal is cited, in accordance with accepted academic practice. No use, distribution or reproduction is permitted which does not comply with these terms.
*Correspondence: Ping Li, cGlsaUBpdXB1aS5lZHU=; Burcin Ekser, YmVrc2VyQGl1cHVpLmVkdQ==
†These authors share first authorship
‡These authors share senior authorship
§ORCID and Emails: Kevin J. Lopez, 0000-0002-5807-3136, a2V2bG9wZXpAaXUuZWR1
Arthur A. Cross-Najafi, 0000-0003-1536-0300, YWNyb3NzbmFAaXVwdWkuZWR1
Kristine Farag, 0000-0002-6858-9099, a2ZhcmFnQGl1LmVkdQ==
Benjamin Obando, 0000-0001-6544-6538, Ym9iYW5kb0BpdS5lZHU=
Deepthi Thadasina, 0000-0002-6896-1479, ZHRoYWRhc2lAaW5kaWFuYS5lZHU=
Abdulkadir Isidan, 0000-0001-6655-1268, a2lzaWRhbkBpdS5lZHU=
Yujin Park, 0000-0001-6542-5761, eXAxNEBpdS5lZHU=
Wenjun Zhang, 0000-0001-9801-0634, d2VuemhhbmdAaXUuZWR1
Burcin Ekser, 0000-0003-0741-8007, YmVrc2VyQGl1cHVpLmVkdQ==
Ping Li, 0000-0003-1055-9505, cGlsaUBpdXB1aS5lZHU=