- 1Pediatric Research Institute “Città della Speranza”, Corso Stati Uniti, Padova, Italy
- 2Department of Biomedical Sciences, University of Padova, Padova, Italy
T cells are master regulators of the immune response tuning, among others, B cells, macrophages and NK cells. To exert their functions requiring high sensibility and specificity, T cells need to integrate different stimuli from the surrounding microenvironment. A finely tuned signalling compartmentalization orchestrated in dynamic platforms is an essential requirement for the proper and efficient response of these cells to distinct triggers. During years, several studies have depicted the pivotal role of the cytoskeleton and lipid microdomains in controlling signalling compartmentalization during T cell activation and functions. Here, we discuss mechanisms responsible for signalling amplification and compartmentalization in T cell activation, focusing on the role of CD28, chemokine receptors and the actin cytoskeleton. We also take into account the detrimental effect of mutations carried by distinct signalling proteins giving rise to syndromes characterized by defects in T cell functionality.
Signalling compartmentalization: surrounding molecules to integrate and amplify signals
Cells must be able to sense, decode and integrate a plethora of environmental stimuli. For many years, an outstanding question for cell biologists was how different signalling cascades, exploiting common intracellular effectors, could trigger distinct cellular responses. It is now clear that to allow the proper progress of specific cellular responses, signalling effectors must be locally confined in space and time, a concept referred as signalling compartmentalization. The tight control of the location, duration and frequency of signalling molecules indeed contributed to the relevant functional specificity that enables receptors to encode distinct cellular responses.
Protein compartmentalization is integral to achieving effective and controlled T cell responses, which are drivers of the adaptive branch of the immune system (1, 2). Over the last years, multiple studies have shed light on both the mechanisms by which signals are compartmentalized in T cells and the physiological role played by such compartmentalization (3). Both lymphocyte migration and activation indeed rely on the selective and transient segregation of signalling molecules and membrane receptors that localized in specific cell locations with different kinetics.
The dynamic molecular compartmentation of signalling players in T cells is ensured by the interplay between the plasma membrane (PM), cytoskeleton networks, and intracellular organelles.
Collectively, such events lead to the establishment of a morphological and molecular asymmetry know as lymphocyte polarization which is crucial for T cell migration and activation.
In resting conditions, T lymphocytes present a spherical shape retained by the cytoskeleton tension and, in particular, by the intermediate filaments and the cortical actin (4, 5). T lymphocyte surface is “decorated” with microvilli sustained by parallel bundles of highly dynamic actin filaments (6, 7) (Figure 1). These structures allow T cells to sense the surrounding microenvironment and, importantly, they promote signalling compartmentalization at their tips, leading to the coalescence of proteins and receptors involved in T cell adhesion and activation, as integrin α4β7-Very Late Antigen 4 (VLA-4), L-selectin, chemokines receptors as CXCR4 (8, 9) and T cell receptor (TCR) complexes (6, 10–14). At the tip of microvilli, proteins are found in close proximity thus prompting an easier and more efficient “scanning” of the APC surface in search of the peptide-major histocompatibility complex (pMHC) and, at the same time, increasing the avidity for subsequent interaction of the two cells. Indeed, following T cells adhesion and activation, microvilli are resorbed and integrin avidity is upregulated in a process mediated by ERMs proteins (ezrin, radixin and moesin), acting as a bridge between the PM and the actin cytoskeleton (15–17). Notably, it has been recently proposed that membrane curvature could also promote signalling compartmentalization within microvilli tip (18). This topic has been extensively discussed elsewhere (19).
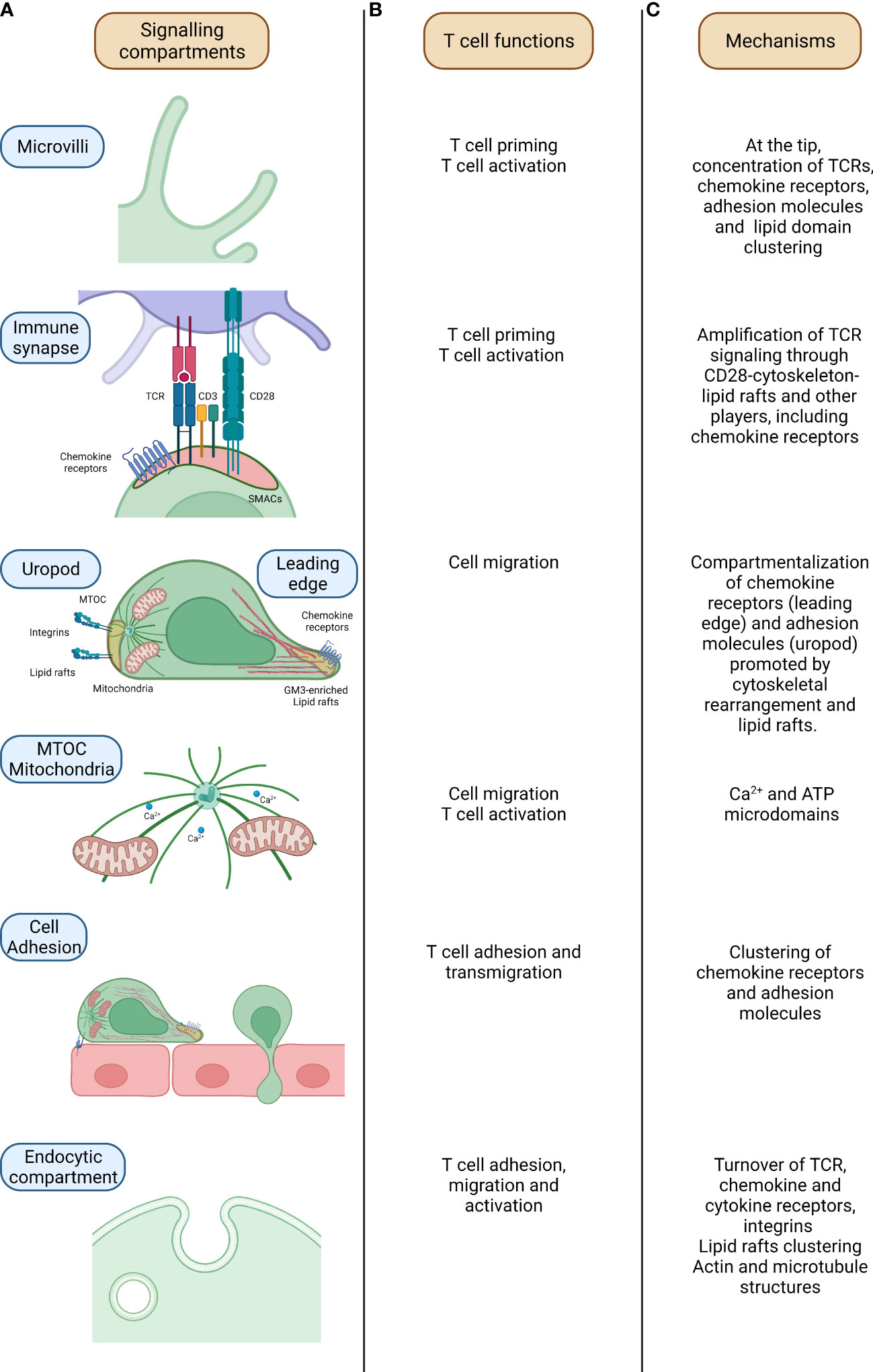
Figure 1 Mechanisms of signalling compartmentalization in T cells. From the left, relevant compartments which regulate signalling compartmentalization in T cells (A), related T cell activities (B) and mechanisms underpinning signalling compartmentalization at these sites are outlined (C). In microvilli, parallel actin filaments allow the sustainment of the structure which assure the concentration of proteins and molecules and signalling compartmentalization in naïve T cells. In the immune synapse (IS), where the formation of the couple between the T cell and the APC is assured by the specific recognition of the Ag recognized on the MHCII molecules by the TCR, the binding of the two cells is further sustained by the CD3 and co-stimulatory molecules (CD28/B7-1/CD80-B7-2/CD86). Here, the compartmentalization of the signalling is mediated by the concerted action of cytoskeletal components, lipid rafts and endocytic compartment. During T cell migration, the T cell acquires an intrinsic polarity mandatory for the definition of a leading edge and a rear pole (uropod). The differential segregation of proteins at these two poles (cytokines and chemokines receptors at the front side while mitochondria and integrins of the rear one) assures the functional motility of the T cell. Mitochondria relocation within the T cell is mediated by microtubules in a Ca2+ dependent fashion. This process is orchestrated by the MTOC (microtubules organizing center) which controls microtubules polymerization and then mitochondria localization in a Ca2+ -dependent fashion. The definition of T cell polarity is mandatory for a proficient T cell migration with, on one side, chemokine receptors guiding the movement at the leading edge while, on the other side, adhesion molecules controlling T cell adhesion hence providing an antithetic force. Lastly, the endocytic compartment, apart from the recycling of molecules, promotes the fine compartmentalization and the amplification of the signal with the juxtapositioning of molecules and proteins. TCR-T cell receptor; MTOC-MicroTubule Organizing Center; ATP-Adenosine TriPhosphate.
In this landscape, a particular focus should be made on the actin cytoskeleton bearing a ubiquitous but fundamental role in multiple cellular processes. As for T cells, the actin cytoskeleton has a key importance in their activation, mainly during the formation and maintenance of a specialized junction named the Immunological Synapse (IS). In accordance to this, recently, it has become clear that mechanical and biochemical signals at the IS are integrated by actin dynamics (20).
Besides cytoskeleton, signalling compartmentalization in T cells is orchestrated also by “small (10–200 nm), heterogeneous, highly dynamic, sterol- and sphingolipid-enriched domains that compartmentalize cellular processes” (21, 22), defined as lipid rafts. Even if, due to technical issues, their description and existence has been debated for years, it has been clear from the beginning that their main feature is the ability to promote signalling via proteins juxtaposition (controlling the inclusion/exclusion of proteins) thus generating “signalling hubs”. Giving their limited size, lipid rafts can welcome only a limited number of proteins which probably stand among 10-30 proteins (22, 23). The partitioning of molecules within their structure can be regulated by multiple factors including the intrinsic molecule state, the signalling state of the cell and post-translational modifications (PTMs). Interestingly, lipid rafts are not stand alone structure by they are connected to the cytoskeleton via actin-binding proteins as ezrin and filamin acquiring then the definition of “floating island” or “flying kites” (21, 24).
The advancements in imaging techniques, with the introduction of single molecule and scanning confocal imaging, have overcome this primordial separation of these compartments. For instance, the “Picket and Fence Model” postulated by Kusumi and colleagues defined confinements area within the membrane (between 30 and 700nm) where transmembrane proteins are anchored and lined up along a fence of cytoskeletal proteins (25). This model arises from the evidence that transmembrane proteins can also move within confined areas and they have to “hop” when changing it. This concept was expanded by the definition of the “proteins island model” in the PM. Protein islands (both rafts and non-rafts islands) are actin-rich areas where membrane-associated proteins are clustered (26, 27) surrounded by a “sea” on protein-free regions. It has been observed that, in activated membranes, rafts and non-rafts regions presented more frequent contacts, a feature that probably shapes and influences their functionality and morphology (26). Interestingly, actin cytoskeleton is mandatory for their establishment, while cholesterol depletion does not impair proteins distribution between rafts and non-rafts regions, implying a superior organization order (26). Even if reports in this direction are still missing, it could not be excluded that protein islands may also have a role in cell-cell communication, membrane trafficking and membrane fusion.
Compelling evidence also indicates that the endocytic compartment works as a signalling hub within the cell (Figure 1). As elegantly revised by Scita and Di Fiore (18), endosomes sustain the signal originated by the PM and, at the same time, allow the generation of unique signals. This is possible thanks to their small volume which favors the coincidence of detectors, the specific enrichment of some lipids, the rapid microtubule-mediated transport of molecules; moreover, the endosome acidic pH might trigger and regulate specific enzymatic functions. Even if additional studies are required to model and experimentally validate endosome dynamics, it is clear how endocytosis provides a controlled spatial and temporal dimension for distinct signaling pathways.
Overall, the spatial ordering of molecular players in distinct cellular compartment enables the complexity of multiple signaling events, a feature which is mandatory for a proficient T cell migration and activation (28).
Signalling compartmentalization in T cells
Even if in resting conditions T cells present a round-shaped morphology, they acquire functional polarity upon stimulation by a variety of ligands. This is particularly evident during T cell migration and priming, when the definition of a cellular polarity allows the maintenance of active and distinct signalling compartments. In the past, our laboratory has analyzed the mechanisms responsible for signaling amplification and compartmentalization during these two processes, focusing on the role of CD28 and chemokine receptors governed by the actin cytoskeleton. In this manuscript, we will focus on the contribution of the aforementioned players in T cell migration and activation.
CD28 at the crossroad of cytoskeleton and lipid rafts
T cell priming starts in lymph nodes (LNs) with the formation of a stable interaction between naive T cells and antigen presenting dendritic cells (DCs). This represents a very sensitive process ultimately leading to multiple cellular responses, including T cell proliferation and the secretion of a wide range of cytokines, chemokines and cytotoxic mediators. By the use of two-photon microscopy, seminal studies unveiled the kinetics of this event in vivo by proposing the 3-stage paradigm: upon antigen encounter, T cells engaged first transient serial interactions (phase 1) and next stable contacts (phase 2) with antigen-loaded DCs; finally they increased their motility, detached and proliferate (phase 3) (29). In particular, the interactions between T cells and APCs are transient between 2 and 8h following the first encounters, stable between 8 and 24h, and again transient by 24–36h (30, 31).
The stability of T cell-DC interaction is determined by multiple interconnected signals, from TCRs as well as stimulatory and inhibitory receptors that are integrated in a specialized membrane junction named the “immunological synapse”-IS (32, 33) (Figure 1). In T lymphocytes, signalling events occurring at this platform cause multiple downstream effects ranging from the dynamic rearrangement of the actin cytoskeleton, and the initiation of a gene expression cascade ultimately leading to the generation of effector and memory T cells (34–36). At the IS, the duration of distinct molecular signals including the amplitude and kinetics of intracellular Ca2+ waves ranges between few minutes to hours (37).
The formation of the IS is initiated with the extension of filopodia and lamellipodia from the T cell toward the APC. The interaction of the two cells leads to the establishment of a F-actin rich interface. Then, TCR and co-stimulatory molecules, including CD28, trigger the reorganization of the cytoskeleton with the recruitment of the actin polymerization machinery and its regulatory proteins at the IS where, in a positive-feedback loop, they promote the maintenance of the TCR signalling (38, 39). Actin segregates into radial asymmetric zones defined as the supramolecular activation clusters (SMACs) (34, 40–42). We can distinguish the cSMAC (central SMAC), comprising the TCR and co-stimulation molecules; an outer ring named as pSMAC (peripheral SMAC) containing the LFA-1 (41, 43) and a distal SMAC (dSMAC) including the CD43 and CD45 (44, 45).
Mechanistically, several protein tyrosine kinases (PTKs), including Src family PTKs such as Lck and Fyn and the Syk family PTK zeta chain of TCR-associated protein 70 (ZAP-70) are brought into proximity of the CD3 complex upon TCR engagement (46). There, Lck or Fyn causes the phosphorylation of the immunoreceptor tyrosine-based activation motifs (ITAM) in the CD3 subunits. Tyrosine phosphorylation of CD3 provides the binding site for ZAP-70 via its SH2 domain, and then Lck or Fyn activates ZAP-70 by phosphorylation (47, 48). ZAP-70 activation in turn favored the phosphorylation of downstream adaptors, including the linker for activation of T cells (LAT) and SH2 domain-containing leukocyte phosphoprotein of 76 kDa (SLP-76) acting as scaffolds to recruit additional signalling molecules. As a consequence, multiple signalling pathways are activated at the IS eventually leading to T-cell activation, proliferation, and differentiation (49).
Importantly, in naïve T cells, the outcome of TCR stimulation is regulated by costimulatory signals. Among them, the CD28-mediated signalling strongly influences T cell priming. At the IS, CD28 signals lower T cell activation threshold by enabling an effective priming by few antigenic complexes (40, 50, 51). When CD28 is recruited at the IS, it promotes the recruitment of multiple downstream interactors at its cytoplasmic tail. Among them, the phosphoinositide 3-kinase (PI3K) (52), Lck (53, 54), growth factor receptor-bound protein 2 (Grb2) (55), Grb2-related adaptor protein (Gads) (56), IL2-inducible T cell kinase (Itk), the guaninenucleotide exchange factor Vav (57), Akt (58), protein phosphatase 2A (PP2A) (59, 60), and protein kinase C theta (PKCθ) (57). With respect to PKCθ, it has been reported that CD28-mediated signals are required for the specific localization of this kinase to the center region of the IS through its V3 motif (61).
As well, CD28 attends the selective sorting of molecular interactors in lipid membrane domains, acting as privileged sites in which signals are protected and amplified. Indeed, we showed that the CD28 co-stimulation of the TCR signaling cascade is based on lipid rafts (62). Next, we found that the kinase Lck is recruited into CD28-signaling rafts and directed to the IS upon CD28 engagement by a process requiring the CD28 COOH-terminal PxxPP motif and Vav-1, key regulator of the actin cytoskeleton rearrangements (63). Of note, IS lipid microdomains are also enriched in TCR signalling proteins, including the Src-family kinase Fyn, the adapter protein LAT, phosphoprotein associated with glycosphingolipid-enriched domains (PAG) or Csk-activating protein (Cbp) and Lck-interacting molecule (LIME) (33, 64). Interestingly, the partitioning of Lck and LAT at the IS lipid microdomains is dictated by the post-translational modifications (PTM, including protein S-acylation) (65–67). In this regard, a recent report showed that S-acylation of the plasma membrane channel ORAI1 is crucial for the selective trapping of this channel in cholesterol-rich lipid microdomains at the IS where it controls the local Ca2+ fluxes leading to T cell activation (68).
Furthermore, according to the protein islands theory, LAT clusters appear to aggregate with CD3/CD28 complexes in the activating surface of T cells (26). LAT acts a central mediator for T cell activation dictating, once phosphorylated, the co-clustering of CD2 and Lck in membrane discrete microdomains via protein-protein interactions in a process initiated by F-actin and actin-associated proteins. Beside this, LAT also regulate calcium dynamics at the IS and Ras signalling (28). As mentioned before, CD28 acts as a master regulator of actin cytoskeleton rearrangements during T cell activation by tuning the actin polymerization machinery. This process is under the control of several interactors: upon TCR-engagement, the kynase ZAP-70 phosphorylates the adaptors SLP-76 that then binds Nck and the guanine nucleotide exchange factor Vav-1. More, Nck constitutively associated with WASp (69, 70) thus acting as a bridge to recruit WASp itself to the SLP-76 signaling complex. In association with SLP-76, Vav-1 mediates the exchange of GDP- to GTP-bound Cdc42, Rho family GTPases that interacts with the conserved VCA domain of WASp allowing its binding to the Arp2/3 complex. Once bound to the VCA domain, Arp2/3 promotes the branching of the actin polymerization and rearrangement at the T cell-APC contact site (71). Arp2/3 cooperates with filamins that are actin crosslinking proteins. In this landscape of interactors, we pointed out the actin-binding protein Filamin-A (FLNa) as the molecular partner of CD28 both in the reshaping of the actin cytoskeleton and in the lipid rafts recruitment at the IS (72). In this study, we showed that the COOH-terminal PxxPP motif of CD28 is required for CD28–FLNa association, and that FLNa has a direct role in CD28 signalling by recruiting Cdc42 at the site of Vav-1 activation. Vav-1 plays a crucial role in the regulation of the CD28 costimulation. Indeed, it has been shown that the adaptor molecule Cbl-b controls the CD28 dependence of T-cell activation by selectively suppressing TCR-mediated Vav activation (73). Cytoskeletal actin dynamics are also regulated by the phosphatidylinositol bisphosphate (PIP2) produced by the activity of the PIP5K enzymes. In this regard, we and other showed that, in collaboration with PIP5Kα and Vav1, PIP5Kβ promotes actin polymerization and CD28 signaling in human T cells (74, 75). Other reports further support the relevance of the dynamic regulation of actin in CD28-mediated costimulation by linking the actin-uncapping proteins Rltpr (76) and CapZIP (77) to the CD28 costimulatory signalling.
More recently, a phenomenological agent-based model has been developed for assessing the contribution of actin-driven forces to IS formation and CD28 localization. By applying this model, authors proposed that although CD28 can reach the IS center by passively following TCR clusters, the ring-like pattern of CD28 at the synapse is determined by the coupling to the actin cytoskeleton (78).
Taken together this evidence endorses the outstanding role of CD28 as a signalling hub in T cells finely tuning cytoskeletal dynamics and lipid rafts reorganization.
Beside CD28, which positively regulates T cell activation, other inhibitory molecules are present on the T cell surface. Among these, the most characterized are CTLA4 and PD1, whose importance rapidly increased in recent years as targets for immune-mediated therapies. These are recruited within the cSMAC together with their downstream mediators and here they compete with CD28 ligands (B7-1/CD80 and B7-2/CD86) for binding, thus promoting the establishment of T cell anergy (5, 34, 79). Interestingly, most of CTLA4 seems to reside within endocytic vesicles, a mechanism facilitating its signalling with a fine compartmentalization (5, 79). Similarly to CTLA4, also PD-1 presents a minimal expression in resting conditions, further increased after T cell activation (79). Thanks to the binding to PD-L1 and PD-L2, it abolishes IL-2 production in T cells and, albeit only in some settings, it also induce T cells apoptosis (79). As was recently revised, both these molecules affect T cell motility reducing its ability to “pause” when encountering the cognate APC thus raising the threshold for IS formation in the “reverse-stop signal model” (80, 81). This effect seems to be mediated by phosphatidylinositol 3-kinase, Vav-1, Cdc42, and myosin light chain MLC kinase (82) which also affect T cell motility to inflamed sites (80). In addition, in was reported that PD-1 mediates the inhibition of T cell function acting mostly on CD28 rather than on TCR (83).
Chemokine receptors
In T cells, the activation of chemokine receptor signalling contributes to the spatial and temporal repositioning ofwfi 2 intracellular and membrane-bound players, ultimately defining T cell polarity. During migration, polarity refers to the ability of cells to change their morphology in response to chemoattractants, and to maintain a stable asymmetric shape with two poles: the leading edge, which protrudes at the cell front, and the rear edge (termed uropod in leukocytes), at the back (84). This process, which is initiated by chemokine receptor signalling and adhesive interactions with the extracellular matrix (ECM), increases the sensitivity toward chemokine gradients, by the selective recruitment of chemokine receptors at the T cell front (85) (Figure 1). Compartmentalization of the PM into distinct lipid microdomains is pivotal in establishing and maintaining leukocyte polarity and perturbation of lipid microdomains inhibits both cell polarization and migration (85, 86).
The spatial organization of chemokine receptors into dimers and higher-ordered oligomers further adds to the complexity of possible GPCR arrangements, and consequently modulation of signaling (87). Recent studies shed light on how cholesterol dictates the spatial organization of GPCRs within the PM. in particular, it has been proposed that cholesterol promotes the oligomerization of chemokine receptors at the PM that ultimately enabling the integration of distinct signaling pathways at the receptor-membrane interface (88). Previously, it has been shown that the CXCR4 and CCR5 receptors associate to GM3-enriched lipid rafts and are consequently redistributed to the leading edge of moving cells. Interestingly, both CXCR4 and CCR5 directly interact with FLNa, that actively modulates their signalling pathways. Indeed, the specific blockade of CXCR4–FLNa interaction inhibited CXCL12-induced chemotaxis in T cells. As for CXCR4, filamin-A expression did not affect CCR5-mediated Ca2+ flux, but regulated F-actin remodelling (89).
Chemokine receptors play a pivotal role during T cell activation, too. Long-lasting interactions between T cells and APCs are dependent on antigens (90, 91), but antigen-specific interactions are preceded by antigen-independent, chemokine-promoted adhesive contacts in the T cell-APC pair, enabling T cells to scan the surface of their cellular partners (92–94). Although the induction of cell polarity at the IS was thought to be dependent on TCR triggering, we have shown that CXCR4-induced activation of LFA-1 at the contact site with APCs starts MTOC and mitochondria relocation towards the upcoming IS (95). Importantly, we found that, by recruiting mitochondria to the IS, LFA-1 sustains and amplifies the upcoming TCR-induced Ca2+ signalling, indicating that establishment of T-cell polarity is pivotal to a prompt and sustained T cell activation (95) (Figure 1). Interestingly, by bringing mitochondria and ORAI channels into close proximity and by re-organizing plasma membrane calcium ATPases (PMCAs) into discrete regions co-localizing with mitochondria, the IS prevents Ca2+ -dependent channel inactivation and reduce local Ca2+-dependent PMCA modulation (96).
The tight spatial and temporal regulation of cytosolic calcium (Ca2+) is of paramount importance for multiple T cell effector functions as differentiation, proliferation, metabolism, cytokine release and cytotoxicity. The IS indeed controls Ca2+ microdomains by bringing mitochondria and ORAI channels into close proximity and favoring the segregation of PMCA into distinct PM domains. The proteins and organelles re-distribution allows mitochondria to rapidly take up the inflowing Ca2+, thereby avoiding high Ca2+ microdomains close to ORAI channels, which prevents Ca2+-dependent channel inactivation and reduce local Ca2+-dependent PMCA modulation. This optimizes net Ca2+ influx at the IS (96).
The mechanisms responsible for Ca2+ signal compartmentalization in T cells have been extensively explored and described (97). Early recruitment of mitochondria at the T-cell IS occurs independently of TCR stimulation and through a mechanism requiring chemokine receptor signalling (95). Interestingly, we had also shown that chemokine receptor signaling induces accumulation of mitochondria at the uropode of migrating cells, where they are required to sustain phosphorylation of the MLC, a key step in high-speed moving cells (98).
In addition to shaping T cells for effective signaling, chemokine receptors directly support the IS stabilization and indeed and T cell activation. We had demonstrated that CXCR4 and CCR5 are stably recruited into the IS by APC-secreted chemokines (70). In this context, chemokine receptors contribute to the amplification of the TCR signalling acting as powerful costimulatory molecules (99). Indeed, their recruitment at the IS prolong the duration of the T cell–APC interaction and strengthen T cell–APC pair attraction ultimately avoiding premature splitting due to chemoattractant sources (99).
Of note, TCR engagement significantly impacts on chemokine receptor signaling properties by favoring the selective triggering of distinct downstream players (79). Canonically, chemokine signaling, initiated following ligand binding, causes the dissociation of the Gai and Gbg subunits of the heterotrimeric G proteins, leading to calcium flux, PI3K triggering and the activation of the small Rho GTPases signaling. However, alternative signalling pathways resulting from the coupling with other G proteins have also been reported for these receptors (100). Importantly, we showed that at the IS chemokines promote the preferential association of the receptor CCR5 with the Gq/11 subunit instead of Gi one (99).
The functional versatility of chemokine receptors in the context of T cell activation may depend on their ability to heterodimerize with other GPCRs. For example, we showed that CXCR4/CCR5-mediated costimulation grounded on their ability to form heterodimers at the IS (101).
In addition, inhibitory molecules (as CTLA4) have been demonstrated to alter the motility both via the up-regulation of chemokine receptors (CCR5 and CCR7) and by the increase in the sensitivity to their respective chemokines (CCL4 (MIP-1β), CXCL12 (SDF1α) and CCL19). This evidence leads to the proposal of a model for chemotaxis integrating CD28 and CTLA-4 signals via the G protein-coupled receptor kinase GRK. CD28 triggers CCR5 phosphorylation via GRK, while CTLA-4 engagement inactivates GRK2 counteracting this mechanism (80).
More recently, an additional mechanism elucidating CXCL12-induced T cell co-stimulation has been proposed. Smith and colleagues showed that the chemokine enhances the number, stability, and phosphorylation of SLP-76 microclusters formed in response to stimulation of the TCR. This results in proximity of SLP-76 and ZAP-70 clusters and in enhanced TCR-dependent gene expression (102).
Multiple studies worked to clarify whether other chemokines preferentially act as co-stimulatory partners for the TCR ultimately promoting T-cell activation. Recently, it has been proposed that CCR7, which drives T cell and DC migration and trafficking in LNs, colocalizes with the TCR at the IS, within sub-synaptic vesicles. There, CCR7 promotes and prolongs ZAP70 activity, resulting in T cell costimulation (103).
All these data, together with many more that we could not include in our discussion, suggest that T cell priming results from a timely and spatially regulated interplay between adhesive and chemoattractant forces mainly occurring in LNs, enabling T cell scanning for the cognate antigen and the formation of long-lasting interaction upon recognition (104).
Congenital defects in cytoskeletal proteins lead to impairment of T cell activation
Perturbations in the equilibrium between adhesive and chemotactic forces leads to defects in the formation of a productive IS, due to the instability of the T cell-APC mating (105). Of note, different inborn errors in genes encoding for proteins controlling these functions, lead to syndromes linked to defects in T cell motility and/or activation (106).
The Warts, Hypogammaglobulinemia, Infections, and Myelokathexis (WHIM) syndrome is a primary immunodeficiency disorder in which a genetic mutation impairs CXCR4 internalization and enhances its responsiveness to CXCL12. WHIM patients experience a wide range of symptoms, including recurring infections, human papillomavirus (HPV)-induced warts, reduced long-term immunoglobulin G (IgG) titers, myelokathexis, and leukopenia (107). The dominant mutations in the chemokine receptor CXCR4 lead to the truncation of its carboxy-terminal domain, ultimately resulting in a defective ability of the receptor to internalize after binding its ligand. As a consequence, immune cells bearing the WHIM-mutant receptor display increased signalling and enhanced migration in response to chemokine stimulation (108). We observed that, in contrast to the wild-type CXCR4, the WHIM-mutant CXCR4 failed to be recruited into the IS and impaired the formation of long-lasting T-APC interactions, thus limiting T cell priming and immune responses to antigens (109). Thus, the hyperfunctional WHIM-mutant CXCR4 favors motility over formation of stable IS, resulting in aberrant T cell activation (109).
The Wiskott-Aldrich syndrome (WAS) is a primary immunodeficiency determined by mutations in the WAS-protein (WASp), a member of a larger family of proteins (WASP family) that functions as nucleation-promoting factors for the Arp2/3 complex, which drives the generation of branched actin filaments (110). WASp is exclusively expressed in cells of the haematopoietic lineage and its loss-of-function mutations cause a syndrome characterized by a broad range of clinical signs, with patients showing an increased susceptibility to infections, haemorrhages, eczema and different autoimmune disorders (111). Upon TCR engagement, WASP is recruited to the IS where it interacts with VAV, RAC and Cdc42 and is activated by VAV effectors (6, 112, 113). WAS patients present alterations in T cell actin cytoskeleton dynamics (114, 115). WASp-/- T cells fail to polymerize actin in response to anti-CD3 stimulation, and show defective IS. The disorganized signaling platforms of WASp-/- T cells do not allow complete and efficient cellular activation and, consequently, T cells from WAS patients show decreased cell proliferation and cell survival (111). Interestingly, this is linked with a severe impairment in CD28 internalization possibly caused by the formation of the functional complex WASp/SNX9/p85/CD28 (116).
Mutations in the WASp-interacting protein (WIP) can also determine a syndrome with clinical signs similar to WAS. WIP is involved in the regulation of WASp activity by promoting its stability, activation and localization to sites of active actin polymerization. Moreover, independently from WASp, WIP regulates actin cytoskeleton in lymphocytes affecting the homing of T cells to infected tissues (117).
Additional immunodeficiencies caused by defects in actin-binding proteins and leading to T cell synapse instability have been described. Among them, the deficiency of the ARPC1B protein, part of the Arp2/3 complex, caused the emission of aberrant actin-rich structures, including spikes and long filopodia-like structures, both in the context of 2D IS and contact with APC (118). Thus, patients suffering of ARPC1B deficiency show defects in T cell proliferation and cytotoxic activity. Interestingly, ARPC1B also contributes to the recycling of the TCR, CD8 and GLUT1 (119), thus causing reduced expression of these molecules in ARPC1B-deficient CD8+ T cells. In addition, as a result of an impaired endosome-to-membrane recycling processes caused by a deficient actin remodeling, T cells lacking the Arp2/3 activator WASH also fail to maintain surface levels of the TCR, CD28, LFA-1 and GLUT1 molecules (120).
Although relevant for T cell activity, other defects, including HEM1 and WDR1 deficiencies, might not be solely explained by defective IS and have been reviewed elsewhere (121). Further investigations are needed to mechanistically explore the role of CD28 and other costimulatory molecules in these disorders.
Future directions
Although here we focused our discussion on chemical signaling, it must be noted that mechanical signals control T cell functions and are required for cell polarization, migration and activation. In particular, membrane curvature seems to initiate signaling events resulting in the organization of larger signaling platforms (122, 123). In both neutrophils and CD8+ T cells, cell polarization was shown to be dependent on local increase of plasma membrane curvature induced by initial adhesion (122). The curved membrane can orchestrate the formation of signaling platforms through the Bin-Amphiphysin-Rvs (BAR) superfamily. BAR proteins induce, regulate and detect membrane curvature (124) and recruit to the curved membrane other proteins, including regulators of actin dynamics.
While the N-BAR and the F-BAR proteins are generally associated with membrane invaginations, the I-BAR are present in various membrane protrusions (125) and involved in microvilli formation (126). Little is known about the role of BAR proteins in T cell functions. The I-BAR IRSp53 is expressed in T cells and essential for the release of HIV particles through a pathway involving Rac1, Wave2 and Arp2/3 (127, 128), but its role in microvilli formation and TCR signaling is unknown. On the other hand, sorting nexin 9 (SNX9), which belongs to the N-BAR subfamily but regulates filopodia formation (129), forms a signaling complex on endocytic vesicles with CD28, WASp and p85 in T cells triggered by CD3/CD28 antibodies (116). In a feed-forward fashion, SNX9 itself was recently shown, once recruited to the IS, to generate membrane tubulation out of CD28 clusters with these dynamic structures regulating both CD28 phosphorylation status and IL-2 production (130).
Further studies will be required to shed light on the role of BAR domain proteins and the membrane curvature in signaling compartmentalization and T cell functions. However, it seems conceivable that cells employ a combination of physical and biochemical forces to tune the formation of structures and domains on the plasma membrane (131). How the integration of the different forces occurred in T cells will be an interesting subject for future investigations.
In addition, accumulating evidence suggests that mechanical forces are key determinants in initiating signaling through the TCR that clearly acts as a membrane mechanoreceptor. In this regard, very recently a new model for TCR triggering has been proposed (132). Indeed, the TCR Bending Mechanosignal (TBM) model predicts that mechanical forces might cause membrane curvature around engaged pMHC/TCR complexes; such mechanical cue is necessary to reach the energy threshold required for the triggering of the signalling cascade ultimately activating T responses (132).
Of note, the investigation of whether and how mechanical signals control costimulatory molecules, as CD28 and chemokine receptors, would be an interesting advancement in this field.
Signalling compartmentalization is essential for immune cells to respond with high specificity and sensitivity. Thus, achieving a deeper understanding of the mechanisms regulating the generation of signalling compartments during T cell migration and activation will be important to modulate immune responses with future therapeutics and will be vital to design effective CAR-T cells.
Author contributions
BM, CL, AV conceived and wrote the manuscript. CL drew the figure. All authors contributed to the article and approved the submitted version.
Funding
BM and AV received research grant from Istituto di Ricerca pediatrica Fondazione Città della Speranza
Conflict of interest
The authors declare that the research was conducted in the absence of any commercial or financial relationships that could be construed as a potential conflict of interest.
Publisher’s note
All claims expressed in this article are solely those of the authors and do not necessarily represent those of their affiliated organizations, or those of the publisher, the editors and the reviewers. Any product that may be evaluated in this article, or claim that may be made by its manufacturer, is not guaranteedor endorsed by the publisher.
References
1. Jun JE, Goodnow CC. Scaffolding of antigen receptors for immunogenic versus tolerogenic signaling. Nat Immunol (2003) 4(11):1057–64. doi: 10.1038/ni1001.
2. Abraham RT, Weiss A. Jurkat T cells and development of the T-cell receptor signalling paradigm. Nat Rev Immunol (2004) 4(4):301–8. doi: 10.1038/nri1330
3. Russell S, Oliaro J. Compartmentalization in T-cell signalling: Membrane microdomains and polarity orchestrate signalling and morphology. Immunol Cell Biol (2006) 84(1):107–13. doi: 10.1111/j.1440-1711.2005.01415.x
4. Etienne-Manneville S. Cytoplasmic intermediate filaments in cell biology. Annu Rev Cell Dev Biol (2018) 34(1):1–28. doi: 10.1146/annurev-cellbio-100617-062534
5. Mastrogiovanni M, Juzans M, Alcover A, Di Bartolo V. Coordinating cytoskeleton and molecular traffic in T cell migration, activation, and effector functions. Front Cell Dev Biol (2020) 8:1138. doi: 10.3389/fcell.2020.591348
6. Burkhardt JK, Carrizosa E, Shaffer MH. The actin cytoskeleton in T cell activation. Annu Rev Immunol (2008) 26:233–59. doi: 10.1146/annurev.immunol.26.021607.090347
7. Majstoravich S, Zhang J, Nicholson-Dykstra S, Linder S, Friedrich W, Siminovitch KA, et al. Lymphocyte microvilli are dynamic, actin-dependent structures that do not require wiskott-Aldrich syndrome protein (WASp) for their morphology. Blood (2004) 104(5):1396–403. doi: 10.1182/blood-2004-02-0437
8. Berlin C, Bargatze RF, Campbell JJ, von Andrian UH, Szabo MC, Hasslen SR, et al. Alpha 4 integrins mediate lymphocyte attachment and rolling under physiologic flow. Cell (1995) 80(3):413–22. doi: 10.1016/0092-8674(95)90491-3
9. Singer II, Scott S, Kawka DW, Chin J, Daugherty BL, DeMartino JA, et al. CCR5, CXCR4, and CD4 are clustered and closely apposed on microvilli of human macrophages and T cells. J Virol (2001) 75(8):3779–90. doi: 10.1128/JVI.75.8.3779-3790.2001
10. Bretscher MS, Aguado-Velasco C. Membrane traffic during cell locomotion. Curr Opin Cell Biol (1998) 10(4):537–41. doi: 10.1016/S0955-0674(98)80070-7
11. Cai E, Marchuk K, Beemiller P, Beppler C, Rubashkin MG, Weaver VM, et al. Visualizing dynamic microvillar search and stabilization during ligand detection by T cells. Science (2017) 356(6338):eaal3118. doi: 10.1126/science.aal3118
12. Griffiths GM, Tsun A, Stinchcombe JC. The immunological synapse: a focal point for endocytosis and exocytosis. J Cell Biol (2010) 189(3):399–406. doi: 10.1083/jcb.201002027
13. Niedergang F, Di Bartolo V, Alcover A. Comparative anatomy of phagocytic and immunological synapses. Front Immunol (2016) 7:18. doi: 10.3389/fimmu.2016.00018
14. Stein JV, Cheng G, Stockton BM, Fors BP, Butcher EC, von Andrian UH. L-selectin-mediated leukocyte adhesion in vivo: microvillous distribution determines tethering efficiency, but not rolling velocity. J Exp Med (1999) 189(1):37–50. doi: 10.1084/jem.189.1.37
15. Alon R, Feigelson S. From rolling to arrest on blood vessels: leukocyte tap dancing on endothelial integrin ligands and chemokines at sub-second contacts. Semin Immunol (2002) 14(2):93–104. doi: 10.1006/smim.2001.0346
16. Brown MJ, Nijhara R, Hallam JA, Gignac M, Yamada KM, Erlandsen SL, et al. Chemokine stimulation of human peripheral blood T lymphocytes induces rapid dephosphorylation of ERM proteins, which facilitates loss of microvilli and polarization. Blood (2003) 102(12):3890–9. doi: 10.1182/blood-2002-12-3807
17. Nijhara R, van Hennik PB, Gignac ML, Kruhlak MJ, Hordijk PL, Delon J, et al. Rac1 mediates collapse of microvilli on chemokine-activated T lymphocytes. J Immunol (2004) 173(8):4985–93. doi: 10.4049/jimmunol.173.8.4985
18. Scita G, Di Fiore PP. The endocytic matrix. Nature (2010) 463(7280):464–73. doi: 10.1038/nature08910
19. McMahon HT, Gallop JL. Membrane curvature and mechanisms of dynamic cell membrane remodelling. Nature (2005) 438(7068):590–6. doi: 10.1038/nature04396
20. Roy NH, Burkhardt JK. The actin cytoskeleton: A mechanical intermediate for signal integration at the immunological synapse. Front Cell Dev Biol (2018) 6:116. doi: 10.3389/fcell.2018.00116
21. Mayor S, Viola A, Stan RV, del Pozo MA. Flying kites on slippery slopes at keystone. symposium on lipid rafts and cell function. EMBO Rep (2006) 7(11):1089–93. doi: 10.1038/sj.embor.7400836
22. Pike LJ. Lipid rafts: bringing order to chaos. J Lipid Res (2003) 44(4):655–67. doi: 10.1194/jlr.R200021-JLR200
23. Subczynski WK, Kusumi A. Dynamics of raft molecules in the cell and artificial membranes: approaches by pulse EPR spin labeling and single molecule optical microscopy. Biochim Biophys Acta (2003) 1610(2):231–43. doi: 10.1016/S0005-2736(03)00021-X
24. Viola A, Gupta N. Tether and trap: regulation of membrane-raft dynamics by actin-binding proteins. Nat Rev Immunol (2007) 7(11):889–96. doi: 10.1038/nri2193
25. Ritchie K, Iino R, Fujiwara T, Murase K, Kusumi A. The fence and picket structure of the plasma membrane of live cells as revealed by single molecule techniques (Review). Mol Membr Biol (2003) 20(1):13–8. doi: 10.1080/0968768021000055698
26. Lillemeier BF, Pfeiffer JR, Surviladze Z, Wilson BS, Davis MM. Plasma membrane-associated proteins are clustered into islands attached to the cytoskeleton. Proc Natl Acad Sci USA (2006) 103(50):18992–7. doi: 10.1073/pnas.0609009103
27. Goyette J, Gaus K. Mechanisms of protein nanoscale clustering. Curr Opin Cell Biol (2017) 44:86–92. doi: 10.1016/j.ceb.2016.09.004
28. Douglass AD, Vale RD. Single-molecule microscopy reveals plasma membrane microdomains created by protein-protein networks that exclude or trap signaling molecules in T cells. Cell (2005) 121(6):937–50. doi: 10.1016/j.cell.2005.04.009
29. Mempel TR, Henrickson SE, von Andrian UH. T-Cell priming by dendritic cells in lymph nodes occurs in three distinct phases. Nature (2004) 427(6970):154–9. doi: 10.1038/nature02238
30. Gérard A, Khan O, Beemiller P, Oswald E, Hu J, Matloubian M, et al. Secondary T cell-T cell synaptic interactions drive the differentiation of protective CD8+ T cells. Nat Immunol (2013) 14(4):356–63. doi: 10.1038/ni.2547
31. Ueda H, Morphew MK, McIntosh JR, Davis MM. CD4+ T-cell synapses involve multiple distinct stages. Proc Natl Acad Sci USA (2011) 108(41):17099–104. doi: 10.1073/pnas.1113703108
32. Davis MM, Boniface JJ, Reich Z, Lyons D, Hampl J, Arden B, et al. Ligand recognition by alpha beta T cell receptors. Annu Rev Immunol (1998) 16:523–44. doi: 10.1146/annurev.immunol.16.1.523
33. Mazzon C, Viola A. From tango to quadrilla. Cell Adhes Migr (2007) 1(1):7–12. doi: 10.4161/cam.3982
34. Dustin ML, Choudhuri K. Signaling and polarized communication across the T cell immunological synapse. Annu Rev Cell Dev Biol (2016) 32:303–25. doi: 10.1146/annurev-cellbio-100814-125330
35. Shah K, Al-Haidari A, Sun J, Kazi JU. T Cell receptor (TCR) signaling in health and disease. Signal Transduct Target Ther (2021) 6(1):1–26. doi: 10.1038/s41392-021-00823-w
36. Werlen G, Palmer E. The T-cell receptor signalosome: a dynamic structure with expanding complexity. Curr Opin Immunol (2002) 14(3):299–305. doi: 10.1016/S0952-7915(02)00339-4
37. Friedmann KS, Bozem M, Hoth M. Calcium signal dynamics in T lymphocytes: Comparing in vivo and in vitro measurements. Semin Cell Dev Biol (2019) 94:84–93. doi: 10.1016/j.semcdb.2019.01.004
38. Lasserre R, Charrin S, Cuche C, Danckaert A, Thoulouze MI, de Chaumont F, et al. Ezrin tunes T-cell activation by controlling Dlg1 and microtubule positioning at the immunological synapse. EMBO J (2010) 29(14):2301–14. doi: 10.1038/emboj.2010.127
39. Nguyen K, Sylvain NR, Bunnell SC. T Cell costimulation via the integrin VLA-4 inhibits the actin-dependent centralization of signaling microclusters containing the adaptor SLP-76. Immunity (2008) 28(6):810–21. doi: 10.1016/j.immuni.2008.04.019
40. Grakoui A, Bromley SK, Sumen C, Davis MM, Shaw AS, Allen PM, et al. The immunological synapse: a molecular machine controlling T cell activation. Science (1999) 285(5425):221–7. doi: 10.1126/science.285.5425.221
41. Monks CRF, Freiberg BA, Kupfer H, Sciaky N, Kupfer A. Three-dimensional segregation of supramolecular activation clusters in T cells. Nature (1998) 395(6697):82–6. doi: 10.1038/25764
42. Shaw AS, Dustin ML. Making the T cell receptor go the distance: a topological view of T cell activation. Immunity (1997) 6(4):361–9. doi: 10.1016/S1074-7613(00)80279-4
43. Lee KH, Holdorf AD, Dustin ML, Chan AC, Allen PM, Shaw AS. T Cell receptor signaling precedes immunological synapse formation. Science (2002) 295(5559):1539–42. doi: 10.1126/science.1067710
44. Delon J, Kaibuchi K, Germain RN. Exclusion of CD43 from the immunological synapse is mediated by phosphorylation-regulated relocation of the cytoskeletal adaptor moesin. Immunity (2001) 15(5):691–701. doi: 10.1016/S1074-7613(01)00231-X
45. Freiberg BA, Kupfer H, Maslanik W, Delli J, Kappler J, Zaller DM, et al. Staging and resetting T cell activation in SMACs. Nat Immunol (2002) 3(10):911–7. doi: 10.1038/ni836
46. June CH, Fletcher MC, Ledbetter JA, Schieven GL, Siegel JN, Phillips AF, et al. Inhibition of tyrosine phosphorylation prevents T-cell receptor-mediated signal transduction. Proc Natl Acad Sci USA (1990) 87(19):7722–6. doi: 10.1073/pnas.87.19.7722
47. Zhang W, Sloan-Lancaster J, Kitchen J, Trible RP, Samelson LE. LAT: the ZAP-70 tyrosine kinase substrate that links T cell receptor to cellular activation. Cell (1998) 92(1):83–92. doi: 10.1016/S0092-8674(00)80901-0
48. Chan AC, Iwashima M, Turck CW, Weiss A. ZAP-70: a 70 kd protein-tyrosine kinase that associates with the TCR zeta chain. Cell (1992) 71(4):649–62. doi: 10.1016/0092-8674(92)90598-7
49. Wang H, Kadlecek TA, Au-Yeung BB, Goodfellow HES, Hsu LY, Freedman TS, et al. ZAP-70: An essential kinase in T-cell signaling. Cold Spring Harb Perspect Biol (2010) 2(5):a002279. doi: 10.1101/cshperspect.a002279
50. Viola A, Lanzavecchia A. T Cell activation determined by T cell receptor number and tunable thresholds. Science (1996) 273(5271):104–6. doi: 10.1126/science.273.5271.104
51. Andres PG, Howland KC, Dresnek D, Edmondson S, Abbas AK, Krummel MF. CD28 signals in the immature immunological synapse. J Immunol (2004) 172(10):5880–6. doi: 10.4049/jimmunol.172.10.5880
52. Harada Y, Ohgai D, Watanabe R, Okano K, Koiwai O, Tanabe K, et al. A single amino acid alteration in cytoplasmic domain determines IL-2 promoter activation by ligation of CD28 but not inducible costimulator (ICOS). J Exp Med (2003) 197(2):257–62. doi: 10.1084/jem.20021305
53. Holdorf AD, Green JM, Levin SD, Denny MF, Straus DB, Link V, et al. Proline residues in Cd28 and the src homology (Sh)3 domain of lck are required for T cell costimulation. J Exp Med (1999) 190(3):375–84. doi: 10.1084/jem.190.3.375
54. Liu P, Aitken K, Kong YY, Opavsky MA, Martino T, Dawood F, et al. The tyrosine kinase p56 lck is essential in coxsackievirus B3-mediated heart disease. Nat Med (2000) 6(4):429–34. doi: 10.1038/74689
55. Raab M, Cai YC, Bunnell SC, Heyeck SD, Berg LJ, Rudd CE. p56Lck and p59Fyn regulate CD28 binding to phosphatidylinositol 3-kinase, growth factor receptor-bound protein GRB-2, and T cell-specific protein-tyrosine kinase ITK: implications for T-cell costimulation. Proc Natl Acad Sci USA (1995) 92(19):8891–5. doi: 10.1073/pnas.92.19.8891
56. Watanabe R, Harada Y, Takeda K, Takahashi J, Ohnuki K, Ogawa S, et al. Grb2 and gads exhibit different interactions with CD28 and play distinct roles in CD28-mediated costimulation. J Immunol (2006) 177(2):1085–91. doi: 10.4049/jimmunol.177.2.1085
57. Villalba M, Coudronniere N, Deckert M, Teixeiro E, Mas P, Altman A. A novel functional interaction between vav and PKCtheta is required for TCR-induced T cell activation. Immunity (2000) 12(2):151–60. doi: 10.1016/S1074-7613(00)80168-5
58. Kane LP, Andres PG, Howland KC, Abbas AK, Weiss A. Akt provides the CD28 costimulatory signal for up-regulation of IL-2 and IFN-gamma but not TH2 cytokines. Nat Immunol (2001) 2(1):37–44. doi: 10.1038/83144
59. Chuang E, Fisher TS, Morgan RW, Robbins MD, Duerr JM, Vander Heiden MG, et al. The CD28 and CTLA-4 receptors associate with the serine/threonine phosphatase PP2A. Immunity (2000) 13(3):313–22. doi: 10.1016/S1074-7613(00)00031-5
60. Alegre ML, Frauwirth KA. Thompson CB. T-cell regulation by CD28 and CTLA-4. Nat Rev Immunol (2001) 1(3):220–8. doi: 10.1038/35105024
61. Brzostek J, Gascoigne NRJ, Rybakin V. Cell type-specific regulation of immunological synapse dynamics by B7 ligand recognition. Front Immunol (2016) 7:24. doi: 10.3389/fimmu.2016.00024
62. Viola A, Schroeder S, Sakakibara Y, Lanzavecchia A. T Lymphocyte costimulation mediated by reorganization of membrane microdomains. Science (1999) 283(5402):680–2. doi: 10.1126/science.283.5402.680
63. Tavano R, Gri G, Molon B, Marinari B, Rudd CE, Tuosto L, et al. CD28 and lipid rafts coordinate recruitment of lck to the immunological synapse of human T lymphocytes. J Immunol Baltim Md (2004) 173(9):5392–7. doi: 10.4049/jimmunol.173.9.5392
64. Mañes S, Viola A. Lipid rafts in lymphocyte activation and migration. Mol Membr Biol (2006) 23(1):59–69. doi: 10.1080/09687860500430069
65. Harder T, Kuhn M. Selective accumulation of raft-associated membrane protein LAT in T cell receptor signaling assemblies. J Cell Biol (2000) 151(2):199–208. doi: 10.1083/jcb.151.2.199
66. Zhang W, Trible RP, Samelson LE. LAT palmitoylation: its essential role in membrane microdomain targeting and tyrosine phosphorylation during T cell activation. Immunity (1998) 9(2):239–46. doi: 10.1016/S1074-7613(00)80606-8
67. Resh MD. Myristylation and palmitylation of src family members: the fats of the matter. Cell (1994) 76(3):411–3. doi: 10.1016/0092-8674(94)90104-X
68. Carreras-Sureda A, Abrami L, Ji-Hee K, Wang WA, Henry C, Frieden M, et al. Acylation by ZDHHC20 targets ORAI1 channels to lipid rafts for efficient Ca2+ signaling by jurkat T cell receptors at the immune synapse. Lewis RS, Swartz KJ, editors. eLife (2021) 10:e72051. doi: 10.7554/eLife.72051
69. Rivero-Lezcano OM, Marcilla A, Sameshima JH, Robbins KC. Wiskott-Aldrich syndrome protein physically associates with nck through src homology 3 domains. Mol Cell Biol (1995) 15(10):5725–31. doi: 10.1128/MCB.15.10.5725
70. Paensuwan P, Ngoenkam J, Khamsri B, Preechanukul K, Sanguansermsri D, Pongcharoen S. Evidence for inducible recruitment of wiskott-Aldrich syndrome protein to T cell receptor-CD3 complex in jurkat T cells. Asian Pac J Allergy Immunol (2015) 33(3):189–95. doi: 10.12932/AP0544.33.3.2015
71. Matalon O, Reicher B, Barda-Saad M. Wiskott-Aldrich syndrome protein–dynamic regulation of actin homeostasis: from activation through function and signal termination in T lymphocytes. Immunol Rev (2013) 256(1):10–29. doi: 10.1111/imr.12112
72. Tavano R, Contento RL, Baranda SJ, Soligo M, Tuosto L, Manes S, et al. CD28 interaction with filamin-a controls lipid raft accumulation at the T-cell immunological synapse. Nat Cell Biol (2006) 8(11):1270–6. doi: 10.1038/ncb1492
73. Chiang YJ, Kole HK, Brown K, Naramura M, Fukuhara S, Hu RJ, et al. Cbl-b regulates the CD28 dependence of T-cell activation. Nature (2000) 403(6766):216–20. doi: 10.1038/35003235
74. Kallikourdis M, Trovato AE, Roselli G, Muscolini M, Porciello N, Tuosto L, et al. Phosphatidylinositol 4-phosphate 5-kinase β controls recruitment of lipid rafts into the immunological synapse. J Immunol Baltim Md (2016) 196(4):1955–63. doi: 10.4049/jimmunol.1501788
75. Porciello N, Kunkl M, Viola A, Tuosto L. Phosphatidylinositol 4-phosphate 5-kinases in the regulation of T cell activation. Front Immunol (2016) 7:186. doi: 10.3389/fimmu.2016.00186
76. Liang Y, Cucchetti M, Roncagalli R, Yokosuka T, Malzac A, Bertosio E, et al. The lymphoid lineage-specific actin-uncapping protein rltpr is essential for costimulation via CD28 and the development of regulatory T cells. Nat Immunol (2013) 14(8):858–66. doi: 10.1038/ni.2634
77. Tian R, Wang H, Gish GD, Petsalaki E, Pasculescu A, Shi Y, et al. Combinatorial proteomic analysis of intercellular signaling applied to the CD28 T-cell costimulatory receptor. Proc Natl Acad Sci USA (2015) 112(13):E1594–1603. doi: 10.1073/pnas.1503286112
78. Siokis A, Robert PA, Demetriou P, Dustin ML, Meyer-Hermann M. F-Actin-Driven CD28-CD80 localization in the immune synapse. Cell Rep (2018) 24(5):1151–62. doi: 10.1016/j.celrep.2018.06.114
79. Intlekofer AM, Thompson CB. At The bench: Preclinical rationale for CTLA-4 and PD-1 blockade as cancer immunotherapy. J Leukoc Biol (2013) 94(1):25–39. doi: 10.1189/jlb.1212621
80. Brunner-Weinzierl MC, Rudd CE. CTLA-4 and PD-1 control of T-cell motility and migration: Implications for tumor immunotherapy. Front Immunol (2018) 9:2737. doi: 10.3389/fimmu.2018.02737
81. Rudd CE. The reverse stop-signal model for CTLA4 function. Nat Rev Immunol (2008) 8(2):153–60. doi: 10.1038/nri2253
82. Wei B, Dias S da R, Wang H, Rudd CE. CTL-associated antigen-4 ligation induces rapid T cell polarization that depends on phosphatidylinositol 3-kinase, vav-1, Cdc42, and myosin light chain kinase. J Immunol (2007) 179(1):400–8. doi: 10.4049/jimmunol.179.1.400
83. Hui E, Cheung J, Zhu J, Su X, Taylor MJ, Wallweber HA, et al. T Cell costimulatory receptor CD28 is a primary target for PD-1-mediated inhibition. Science (2017) 355(6332):1428–33. doi: 10.1126/science.aaf1292
84. Sánchez-Madrid F, del Pozo MA. Leukocyte polarization in cell migration and immune interactions. EMBO J (1999) 18(3):501–11. doi: 10.1093/emboj/18.3.501
85. Gómez-Móuton C, Abad JL, Mira E, Lacalle RA, Gallardo E, Jiménez-Baranda S, et al. Segregation of leading-edge and uropod components into specific lipid rafts during T cell polarization. Proc Natl Acad Sci USA (2001) 98(17):9642–7. doi: 10.1073/pnas.171160298
86. Mañes S, Ana Lacalle R, Gómez-Moutón C, Martínez-A C. From rafts to crafts: membrane asymmetry in moving cells. Trends Immunol (2003) 24(6):320–6. doi: 10.7554/eLife.72051
87. Mellado M, Martínez-A C, Rodríguez-Frade JM. Analysis of G-protein-coupled receptor dimerization following chemokine signaling. Methods (2002) 27(4):111–24. doi: 10.1016/s1046-2023(02)00093-2
88. Legler DF, Matti C, Laufer JM, Jakobs BD, Purvanov V, Uetz-von Allmen E, et al. Modulation of chemokine receptor function by cholesterol: New prospects for pharmacological intervention. Mol Pharmacol (2017) 91(4):331–8. doi: 10.1124/mol.116.107151
89. Jiménez-Baranda S, Gómez-Moutón C, Rojas A, Martínez-Prats L, Mira E, Ana Lacalle R, et al. Filamin-a regulates actin-dependent clustering of HIV receptors. Nat Cell Biol (2007) 9(7):838–46. doi: 10.1038/ncb1610
90. Skokos D, Shakhar G, Varma R, Waite JC, Cameron TO, Lindquist RL, et al. Peptide-MHC potency governs dynamic interactions between T cells and dendritic cells in lymph nodes. Nat Immunol (2007) 8(8):835–44. doi: 10.1038/ni1490
91. Henrickson SE, Mempel TR, Mazo IB, Liu B, Artyomov MN, Zheng H, et al. T Cell sensing of antigen dose governs interactive behavior with dendritic cells and sets a threshold for T cell activation. Nat Immunol (2008) 9(3):282–91. doi: 10.1038/ni1559
92. Revy P, Sospedra M, Barbour B, Trautmann A. Functional antigen-independent synapses formed between T cells and dendritic cells. Nat Immunol (2001) 2(10):925–31. doi: 10.1038/ni713
93. Montoya MC, Sancho D, Vicente-Manzanares M, Sánchez-Madrid F. Cell adhesion and polarity during immune interactions. Immunol Rev (2002) 186:68–82. doi: 10.1034/j.1600-065X.2002.18607.x
94. Friedman RS, Jacobelli J, Krummel MF. Mechanisms of T cell motility and arrest: deciphering the relationship between intra- and extracellular determinants. Semin Immunol (2005) 17(6):387–99. doi: 10.1016/j.smim.2005.09.006
95. Contento RL, Campello S, Trovato AE, Magrini E, Anselmi F, Viola A. Adhesion shapes T cells for prompt and sustained T-cell receptor signalling. EMBO J (2010) 29(23):4035–47. doi: 10.1038/emboj.2010.258
96. Quintana A, Pasche M, Junker C, Al-Ansary D, Rieger H, Kummerow C, et al. Calcium microdomains at the immunological synapse: how ORAI channels, mitochondria and calcium pumps generate local calcium signals for efficient T-cell activation. EMBO J (2011) 30(19):3895–912. doi: 10.1038/emboj.2011.289
97. Trebak M, Kinet JP. Calcium signalling in T cells. Nat Rev Immunol (2019) 19(3):154–69. doi: 10.1038/s41577-018-0110-7
98. Campello S, Lacalle RA, Bettella M, Mañes S, Scorrano L, Viola A. Orchestration of lymphocyte chemotaxis by mitochondrial dynamics. J Exp Med (2006) 203(13):2879–86. doi: 10.1084/jem.20061877
99. Molon B, Gri G, Bettella M, Gómez-Moutón C, Lanzavecchia A, Martínez-A C, et al. T Cell costimulation by chemokine receptors. Nat Immunol (2005) 6(5):465–71. doi: 10.1038/ni1191
100. Thelen M, Stein JV. How chemokines invite leukocytes to dance. Nat Immunol (2008) 9(9):953–9. doi: 10.1038/ni.f.207
101. Contento RL, Molon B, Boularan C, Pozzan T, Manes S, Marullo S, et al. CXCR4–CCR5: A couple modulating T cell functions. Proc Natl Acad Sci (2008) 105(29):10101–6. doi: 10.1073/pnas.0804286105
102. Smith X, Schneider H, Köhler K, Liu H, Lu Y, Rudd CE. The chemokine CXCL12 generates costimulatory signals in T cells to enhance phosphorylation and clustering of the adaptor protein SLP-76. Sci Signal (2013) 6(286):ra65. doi: 10.1126/scisignal.2004018
103. Laufer JM, Kindinger I, Artinger M, Pauli A, Legler DF. CCR7 is recruited to the immunological synapse, acts as Co-stimulatory molecule and drives LFA-1 clustering for efficient T cell adhesion through ZAP70. Front Immunol (2019) 9:3115. doi: 10.3389/fimmu.2018.03115
104. Viola A, Contento RL, Molon B. T Cells and their partners: The chemokine dating agency. Trends Immunol (2006) 27(9):421–7. doi: 10.1016/j.it.2006.07.004
105. Dustin ML. Stop and go traffic to tune T cell responses. Immunity (2004) 21(3):305–14. doi: 10.1016/j.immuni.2004.08.016
106. Kallikourdis M, Viola A, Benvenuti F. Human immunodeficiencies related to defective APC/T cell interaction. Front Immunol (2015) 6:433. doi: 10.3389/fimmu.2015.00433
107. Hernandez PA, Gorlin RJ, Lukens JN, Taniuchi S, Bohinjec J, Francois F, et al. Mutations in the chemokine receptor gene CXCR4 are associated with WHIM syndrome, a combined immunodeficiency disease. Nat Genet (2003) 34(1):70–4. doi: 10.1038/ng1149
108. Balabanian K, Lagane B, Pablos JL, Laurent L, Planchenault T, Verola O, et al. WHIM syndromes with different genetic anomalies are accounted for by impaired CXCR4 desensitization to CXCL12. Blood (2005) 105(6):2449–57. doi: 10.1182/blood-2004-06-2289
109. Kallikourdis M, Trovato AE, Anselmi F, Sarukhan A, Roselli G, Tassone L, et al. The CXCR4 mutations in WHIM syndrome impair the stability of the T-cell immunologic synapse. Blood (2013) 122(5):666–73. doi: 10.1182/blood-2012-10-461830
110. Derry JM, Ochs HD, Francke U. Isolation of a novel gene mutated in wiskott-Aldrich syndrome. Cell (1994) 78(4):635–44. doi: 10.1016/0092-8674(94)90528-2
111. Albert MH, Notarangelo LD, Ochs HD. Clinical spectrum, pathophysiology and treatment of the wiskott-Aldrich syndrome. Curr Opin Hematol (2011) 18(1):42–8. doi: 10.1097/MOH.0b013e32834114bc
112. Barda-Saad M, Braiman A, Titerence R, Bunnell SC, Barr VA, Samelson LE. Dynamic molecular interactions linking the T cell antigen receptor to the actin cytoskeleton. Nat Immunol (2005) 6(1):80–9. doi: 10.1038/ni1143
113. Zeng R, Cannon JL, Abraham RT, Way M, Billadeau DD, Bubeck-Wardenberg J, et al. SLP-76 coordinates nck-dependent wiskott-Aldrich syndrome protein recruitment with vav-1/Cdc42-dependent wiskott-Aldrich syndrome protein activation at the T cell-APC contact site. J Immunol Baltim Md (2003) 171(3):1360–8. doi: 10.4049/jimmunol.171.3.1360
114. Snapper SB, Meelu P, Nguyen D, Stockton BM, Bozza P, Alt FW, et al. WASP deficiency leads to global defects of directed leukocyte migration in vitro and in vivo. J Leukoc Biol (2005) 77(6):993–8. doi: 10.1189/jlb.0804444
115. Lafouresse F, Cotta-de-Almeida V, Malet-Engra G, Galy A, Valitutti S, Dupré L. Wiskott-Aldrich syndrome protein controls antigen-presenting cell-driven CD4+ T-cell motility by regulating adhesion to intercellular adhesion molecule-1. Immunology (2012) 137(2):183–96. doi: 10.1111/j.1365-2567.2012.03620.x
116. Badour K, McGavin MKH, Zhang J, Freeman S, Vieira C, Filipp D, et al. Interaction of the wiskott–Aldrich syndrome protein with sorting nexin 9 is required for CD28 endocytosis and cosignaling in T cells. Proc Natl Acad Sci (2007) 104(5):1593–8. doi: 10.1073/pnas.0610543104
117. Massaad MJ, Oyoshi MK, Kane J, Koduru S, Alcaide P, Nakamura F, et al. Binding of WIP to actin is essential for T cell actin cytoskeleton integrity and tissue homing. Mol Cell Biol (2014) 34(23):4343–54. doi: 10.1128/MCB.00533-14
118. Brigida I, Zoccolillo M, Cicalese MP, Pfajfer L, Barzaghi F, Scala S, et al. T-Cell defects in patients with ARPC1B germline mutations account for combined immunodeficiency. Blood (2018) 132(22):2362–74. doi: 10.1182/blood-2018-07-863431
119. Randzavola LO, Strege K, Juzans M, Asano Y, Stinchcombe JC, Gawden-Bone CM, et al. Loss of ARPC1B impairs cytotoxic T lymphocyte maintenance and cytolytic activity. J Clin Invest (2019) 129(12):5600–14. doi: 10.1172/JCI129388
120. Piotrowski JT, Gomez TS, Schoon RA, Mangalam AK, Billadeau DD. WASH knockout T cells demonstrate defective receptor trafficking, proliferation, and effector function. Mol Cell Biol (2013) 33(5):958–73. doi: 10.1128/MCB.01288-12
121. Dupré L, Boztug K, Pfajfer L. Actin dynamics at the T cell synapse as revealed by immune-related actinopathies. Front Cell Dev Biol (2021) 9:665519. doi: 10.3389/fcell.2021.665519
122. Ren C, Yuan Q, Braun M, Zhang X, Petri B, Zhang J, et al. Leukocyte cytoskeleton polarization is initiated by plasma membrane curvature from cell attachment. Dev Cell (2019) 49(2):206–219.e7. doi: 10.1016/j.devcel.2019.02.023
123. Orbach R, Su X. Surfing on membrane waves: Microvilli, curved membranes, and immune signaling. Front Immunol (2020) 11:2187. doi: 10.3389/fimmu.2020.02187
124. Kessels MM, Qualmann B. Interplay between membrane curvature and the actin cytoskeleton. Curr Opin Cell Biol (2021) 68:10–9. doi: 10.1016/j.ceb.2020.08.008
125. Saarikangas J, Zhao H, Pykäläinen A, Laurinmäki P, Mattila PK, Kinnunen PKJ, et al. Molecular mechanisms of membrane deformation by I-BAR domain proteins. Curr Biol (2009) 19(2):95–107. doi: 10.1016/j.cub.2008.12.029
126. Postema MM, Grega-Larson NE, Neininger AC, Tyska MJ. IRTKS (BAIAP2L1) elongates epithelial microvilli using EPS8-dependent and independent mechanisms. Curr Biol (2018) 28(18):2876–88.e4. doi: 10.1016/j.cub.2018.07.022
127. Inamdar K, Tsai FC, Dibsy R, de Poret A, Manzi J, Merida P, et al. Full assembly of HIV-1 particles requires assistance of the membrane curvature factor IRSp53. eLife (2021) 10:e67321. doi: 10.7554/eLife.67321
128. Thomas A, Mariani-Floderer C, López-Huertas MR, Gros N, Hamard-Péron E, Favard C, et al. Involvement of the Rac1-IRSp53-Wave2-Arp2/3 signaling pathway in HIV-1 gag particle release in CD4 T cells. J Virol (2015) 89(16):8162–81. doi: 10.1128/JVI.00469-15
129. Jarsch IK, Gadsby JR, Nuccitelli A, Mason J, Shimo H, Pilloux L, et al. A direct role for SNX9 in the biogenesis of filopodia. J Cell Biol (2020) 219(4):e201909178. doi: 10.1083/jcb.201909178
130. Ecker M, Schregle R, Kapoor-Kaushik N, Rossatti P, Betzler VM, Kempe D, et al. SNX9-induced membrane tubulation regulates CD28 cluster stability and signalling. eLife (2022) 11:e67550.
131. Sens P, Plastino J. Membrane tension and cytoskeleton organization in cell motility. J Phys Condens Matter Inst Phys J (2015) 27(27):273103. doi: 10.1088/0953-8984/27/27/273103
Keywords: immune synapse, Chemokine receptors, CD28, costimulation, lipid raft
Citation: Molon B, Liboni C and Viola A (2022) CD28 and chemokine receptors: Signalling amplifiers at the immunological synapse. Front. Immunol. 13:938004. doi: 10.3389/fimmu.2022.938004
Received: 06 May 2022; Accepted: 08 July 2022;
Published: 02 August 2022.
Edited by:
Jerome Delon, INSERM U1016 Institut Cochin, FranceReviewed by:
Susana Minguet, University of Freiburg, GermanyAskar M. Akimzhanov, University of Texas Health Science Center at Houston, United States
Jeremie Rossy, Biotechnology Institute Thurgau, Switzerland
Copyright © 2022 Molon, Liboni and Viola. This is an open-access article distributed under the terms of the Creative Commons Attribution License (CC BY). The use, distribution or reproduction in other forums is permitted, provided the original author(s) and the copyright owner(s) are credited and that the original publication in this journal is cited, in accordance with accepted academic practice. No use, distribution or reproduction is permitted which does not comply with these terms.
*Correspondence: Barbara Molon, YmFyYmFyYS5tb2xvbkB1bmlwZC5pdA==