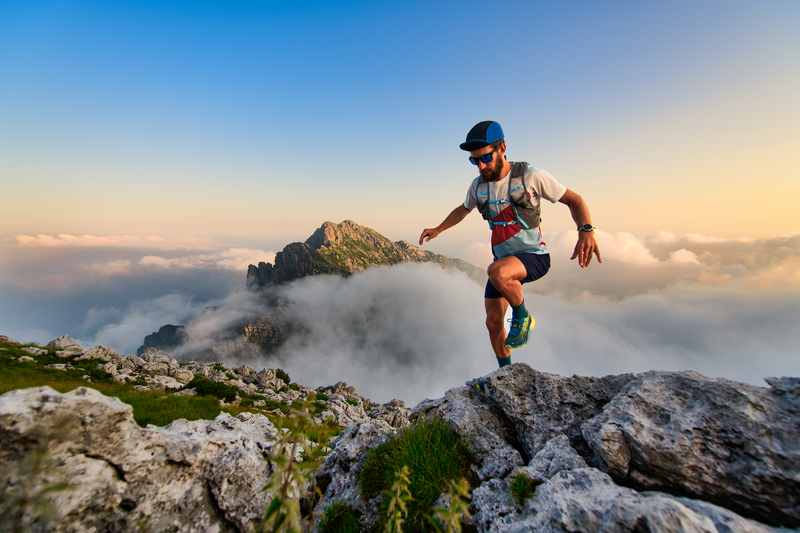
95% of researchers rate our articles as excellent or good
Learn more about the work of our research integrity team to safeguard the quality of each article we publish.
Find out more
REVIEW article
Front. Immunol. , 19 August 2022
Sec. Cancer Immunity and Immunotherapy
Volume 13 - 2022 | https://doi.org/10.3389/fimmu.2022.937565
This article is part of the Research Topic Extracellular Traps in Cancer Immunity and Immunotherapy View all 7 articles
NETs are chromatin-derived webs extruded from neutrophils as a result of either infection or sterile stimulation using chemicals, cytokines, or microbes. In addition to the classical role that NETs play in innate immunity against infection and injuries, NETs have been implicated extensively in cancer progression, metastatic dissemination, and therapy resistance. The purpose of this review is to describe recent investigations into NETs and the roles they play in tumor biology and to explore their potential as therapeutic targets in cancer treatment.
An inflammatory response to tumors is a contributing factor to tumor initiation and progression, allowing cancer cells to escape detection by the immune system. Cancer progression depends on the interaction between tumor-infiltrating immune cells and tumor-derived factors in the tumor microenvironment (TME). Initially, infiltrating and resident immune cells in the TME contribute to tumor growth, metastasis, and responses to immunotherapy. According to conventional wisdom, macrophages are central players in the TME and are activated to respond in a wide variety way to local and circulating stimuli. The multiple roles played by neutrophils in circulating and infiltrating tumors have become increasingly clear in recent years.
Neutrophils represent 50–70% of all leukocytes in the body. As cells in the innate immune system, neutrophils play a prominent role in fighting against bacterial pathogenic infection and have been considered essential players in remodeling the TME via neutrophil extracellular trap (NET) generation or NETosis. NETs are extracellular DNA-integrated chromatin decorated with granular proteins, such as neutrophil-derived neutrophil elastase (NE) and myeloperoxidase (MPO) (1). In addition to the roles they play in host defense, NETs have been widely implicated in noninfectious diseases, such as autoimmune diseases (2–4), rheumatic diseases (5, 6), thrombosis (7–9), diabetes (10), and atherosclerosis (11–13). Increasing evidence indicates that NETs play a critical role in the TME, including in tumor progression, metastasis and therapy resistance (14–28). The effects of NET-related components seem to be intertwined and complicated.
In this review, we illustrate the mechanism and potential role played by NETs within the TME, highlighting their potential as cancer therapy targets.
Among circulating leukocytes in humans, neutrophils are the most abundant cell type. Neutrophils are often the first cells to reach sites of tissue injury or infection. Increasing evidence indicates that inflammation is closely linked to cancer development, and biomarkers of inflammatory reactions have been found to be useful as prognostics in several types of cancer. A highly heterogeneous TME affects tumor malignancy and treatment responses. Inflammatory cells and cytokines create an inflammatory TME, promoting tumor cell proliferation, survival, immune evasion, and migration (29, 30). The various innate immune cells in the TME include tumor-associated macrophages (TAMs), polymorphonuclear neutrophils (PMNs, also known as TANs) and myeloid-derived suppressor cells (MDSCs) (31). In the past two decades, the role played by TAMs in tumor biology has become relatively well established (32–35), but the role played by TANs in these processes is still largely unknown (36). Neutrophils are becoming increasingly recognized as important and contributors to cancer progression, not merely as bystanders in the TME.
Similar to macrophages, TANs undergo polarization state switching, which seems to be determined by the TME. The concept of an N1 and N2 neutrophil phenotype was first introduced by Fridlender in 2009 (37). Previous studies have demonstrated that G-CSF or transforming growth factor-β (TGF-β) are critical for the transformation of neutrophils toward the N2 phenotype (37, 38). Despite neutrophil polarization being reported in some diseases, no clinically relevant biomarker has been identified to distinguish N1 and N2 neutrophils in tumors, in contrast to reports describing M1 and M2 macrophage biomarkers.
In 2004, Brinkmann and colleagues demonstrated that activated neutrophils secrete NETs, which are web-like structures made of decondensed chromatin and various granular proteins (39). Initially, NETosis was thought to be the manifestation of a novel type of cell death that differs from necrosis and apoptosis. NETosis is triggered by a wide range of factors, including pathogens (40), activated platelets, and phorbol myristate acetate (PMA) (41). Peptidyl arginine-deiminase 4 (PAD4) changes the chromatin charge in neutrophils, leading to chromatin recondensation. In the presence of various stimuli, cytoplasmic granules and the nuclear membrane dissolve, and nuclear lobes are lost. Granule enzymes migrate to the nucleus, where they promote chromatin condensation and histone hypercitrullination (42, 43). To date, two forms of NETosis have been established. In one form, the lytic suicide mechanism, the rupture of cytoplasmic membranes is needed, whereas in the other form, vital NETosis, cytoplasmic membrane rupture is not required (44–47).
It was first described that NETs formed followed by cell death or release of lytic NETs.
Lytic NETosis can be caused by several factors, including PMA, autoantibodies, and cholesterol crystals. The activation of NADPH oxidase produces ROS, which activate PAD4 and decondense chromatin. Further chromatin unraveling occurs as a result of neutrophil elastase (NE) and myeloperoxidase (MPO) translocation. Chromosomes are decorated with granular and cytosolic proteins when they enter the cytosol. The neutrophil dies when its plasma membrane has been disrupted, releasing NETs.
The time required for lytic NET formation has been reported to occur primarily at 3 to 4 hours. In contrast, neutrophils can also release NETs in a very rapid (5-60 min) and cell death-independent manner. When S. aureus and E. coli enter the body, their complement receptors, as well as TLR2 and TLR4 receptors, are activated within minutes. Activating PAD4 causes chromatin decondensation, possibly without oxidizing agents. Suicidal NETosis occurs when NE translocates into the nucleus, causing chromatin to unfold. Neutrophils retain their ability to carry out further functions, such as phagocytosis, because of expulsion of protein-decorated chromatin through vesicles.
The functional complexity of neutrophils is closely related to the diversity of their components (42, 43). Histones and DNA are the major components of NETs in various studies (39, 48, 49). Furthermore, many neutrophil granule components, such as NE, MPO, cathepsin G, and proteinase 3 (PR3), are essential components of NETs (42, 43, 50). Recent research has suggested that NETs may participate in various disorders, including diabetes (10), autoimmune diseases (3, 51, 52), atherosclerosis (11–13), thrombosis (7, 53), and cancer (14–18, 26, 54, 55). In the past five years, the study of NETs has provided new insights into the TME.
Increasing recognition has been made of the importance of NETs in the TME. Some explorations into the potential biological role played by NETs in tumors, including tumor growth, metastasis and therapy resistance, have been initiated. Recent studies describing the relationships between NETs and cancer are shown in Table 1 and Figure 1. The following paragraphs will describe the role of NETs in cancer biology in further detail (Table 2).
In the first decade after NET discovery, research on NETs was primarily focused on infectious diseases and circulatory system-related diseases because neutrophils are the most abundant circulating immune cells and exhibit antibacterial activity. NETs have been reported in human solid tumors, with the first observation reported in 2013. This early NET study focused on a small number of Ewing sarcoma samples and suggested that patients with intertumoral NETs had a significantly shorter life expectancy than patients without intertumoral NETs (155). In recent decades, NETs have been considered to be putative biomarkers in patients with various types of cancers. Evidence has suggested that plasma NETs are potential biomarkers for predicting early-stage cancer and tumor metastasis in 73 patients with head and neck cancer (HNC) (138). According to this HCN study, NETs were less common in advanced cancer stages (T3–4, N3), which correlates with an increase in granulocyte colony-stimulating factor (G-CSF) levels. In another study focused on small cohorts of cancer patients, citrullinated histone H3 (CitH3), the gold standard for NET formation identification, was found to be a prognostic blood signature for patients with advanced cancer, as suggested by Melanie Demers et al. (156). Based on their study, high levels of CitH3 powerfully heralds poor clinical results for cancer patients. NETs have also been observed in the peripheral blood, lung tissue, and sputum of lung cancer patients (127). The levels of NETs generated by neutrophils stimulated with IL-8 and LPS from colorectal cancer patients were obviously higher than those in healthy controls and were associated with a poor clinical outcome (157).
A TME is often a hypoxic complex environment replete with cytokines and growth factors. The necrosis of tumor cells caused by the hypoxic microenvironment often leads to the release of danger-associated molecular patterns (DAMPs), which cause inflammation. Hamza O et al. demonstrated that NETs induced increased mitochondrial function in tumor cells, supplying energy for accelerated tumor growth. NET formation in the hypoxic TME is caused by chemokines and high mobility group box 1 (HMGB1) levels, which have been found to be high in conditioned medium prepared with hypoxic cancer cells. In PAD4-knockout (KO) mice, the progression of tumor growth and hepatic metastases were both extremely slow compared to those in wild-type mice. The expression of a number of mitophagy-associated proteins, including DRP-1, MFN-2, PINK1 and Parkin, was increased in cancer cells exposed to NETs. TLR-4 was activated via NE, and mitochondrial biogenesis and lymphocyte proliferation were found in the tumors of PAD4-KO mice (28).
In another study, PAD4-deficient mice did not show reduced tumor growth, but the administration of exogenous G-CSF induced intertumoral NET formation in the wild-type (WT) host. These results suggested that the tumor or TME primes neutrophils for NET formation and leads to the accumulation of intertumoral NETs and a growth advantage for tumors (158).
NET levels were high in patients with advanced diffuse large B-cell lymphoma (DLBCL) and were closely related to low survival rates in a previous study. Tumor progression was promoted by the activation of Toll-like receptor 9 (TLR9), which is in a downstream pathway activated by lymphoma-derived IL-8. In preclinical models, blocking the IL-8-CXCR2 axis or TLR9 activation delayed tumor progression (22).
Several cancers are associated with chronic inflammation and infection, as these conditions promote cancer progression. NETs have been proven to be key threads in the transformation from an inflammatory state to cancerogenesis. A study revealed that NETs were induced by sustained inflammation that awakened dormant cancer cells (128). Smoking or inhalation of lipopolysaccharide (LPS) caused chronic lung inflammation that resulted in NET formation, inhibiting lung tumor growth both in vitro and in vivo. Mechanistically, through the MAPK/ERK/MLCK/YAP signaling pathway, NET-mediated proteolytic remodeling of laminin caused an epitope to activate dormant cancer cells, promoting their proliferation (128).
Neutrophils can facilitate angiogenesis in primary tumors by releasing MMP9, S100A8/9, and BV8 to activate VEGF (61, 62, 64, 159, 160). Neutrophil elastase (NE) and MMP9 can promote tumor cell proliferation by releasing growth factors and degrading laminin (67, 128). Furthermore, inflammatory stimuli (IL-1β and TNF-α) can stimulate neutrophil MET expression and HGF binding, leading to NO production and tumor cell death (72). From the perspective of the primary tumor site, neutrophils can promote metastasis by promoting cancer cell escape into blood vessels (161). In the circulation, neutrophils facilitate the progression of circulating tumor cells through their cell cycle (162). It has been shown that neutrophils can direct disseminated cancer cells to specific sites and promote vascular leakiness for easy extravasation (161, 163, 164). Additionally, neutrophils can release protein-coated nucleic acids, called neutrophil extracellular traps (NETs), that catch circulating cancer cells and stimulate cancer growth.
Due to their special structures, NETs have received considerable attention because of their potential role in tumor metastasis (19, 20, 25–27). For example, in orthotopic ovarian cancer models, researchers found that neutrophil influx into the omentum was a precursor to metastatic progression (20). The inflammatory factors derived from ovarian tumors stimulated neutrophils to secrete NETs. Moreover, NETs were observed in the omentum of ovarian tumor-bearing mice before metastasis and in patients with early-stage carcinoma. These NETs bound ovarian cancer cells and may have promoted tumor metastasis, according to the study. Genetic and pharmacological blockade of PAD4 expression notably decreased omental metastasis.
NETs have also been observed in metastatic lung lesions, but they exhibit the highest seroprevalence rate in triple-negative tumors (23). These researchers found that breast cancer cells initiated NETosis to promote metastasis. In mice orthotopically transplanted with murine breast cancer cells, CXCL1 mediated neutrophil recruitment to the TME. Compared to 4T07 (nonmetastatic) tumors, primary 4T1 (metastatic) tumors exhibited a higher number of neutrophils, and blocking CXCL1 expression in 4T1 cells diminished neutrophil infiltration into tumors. Additionally, metastatic cancer cells induced NETosis through the release of G-CSF at sites of NET dissemination, and inhibition of G-CSF release prevented the formation of NETs by 4T1 cells.
In recent years, various reports have discussed the importance of NETs in tumor metastasis, but the definite mechanism for this phenomenon remains unclear. The expression of a5b1, avb3, and avb5 integrins allowed cancer cells to adhere to NETs derived from neutrophil-like cells in vitro. Cancer cells were inhibited from adhering to NETs by the cyclic RGD peptide to a degree comparable to the effect of DNase (21).
In studying the interaction between NETs and metastases, a recent report provided new insight into a NET-DNA receptor on cancer cells (26). According to this novel finding, liver metastases in breast and colon cancer patients were associated with high NET levels in patient serum, and the risk for liver metastases in patients with breast cancer in the early stages was higher than the risk for patients with colon cancer. In cancer cells, the NET-DNA receptor CCDC25 senses extracellular DNA and promotes cell mobility through the ILK–β-parvin pathway. In the clinic, Yang et al. found that CCDC25 expression on primary cancer cells predicted poor outcomes. Based on their original studies, they explained how NETs interact with cancer cells and identified CCDC25 as a DNA sensor involved in the interaction between NETs and cancer cells.
A recent report revealed a novel mechanism by which tumor cells controlled NETosis in metastatic niches. They found that cathepsin C, a tumor-secreted protease, promoted breast-to-lung metastasis by priming the formation of NETs (25). Neutrophil membrane-bound proteinase 3 (PR3) is activated by CTSC to accelerate the progression of interleukin-1β (IL-1β) and nuclear factor κB activation. This activation resulted in increased IL-6 and CCL3 production, leading to NETosis neutralization.
The occurrence of thrombosis is common in most cancers and is closely linked to cancer patient mortality (165–167). Venous thromboembolism (VTE) has been reported to develop in 4% to 20% of children, and arterial thrombosis has been reported in 2% to 5% of children (168–170). Many factors contribute to cancer-associated thrombosis, but the underlying mechanisms remain unclear. Many different biomarkers have been analyzed with the aim of identifying cancer patients at high risk for VTE. Recently, an interest in identifying procoagulant and prothrombotic factors has been generated (53, 171). Furthermore, the first report on NETs in cancer was focused on the potential role played by NETs in cancer-associated thrombosis (129). These early studies showed that malignant neutrophils were likely to form NETs in a chronic myelogenous leukemia mouse model. Cancers cause an expansion in peritoneal NETs through a systemic effect on the host, as shown by the increased likelihood of NET formation in mammary and lung carcinoma models. One study showed that the expression of JAK2V617F was associated with NET formation and thrombosis in patients with Philadelphia chromosome-negative myeloproliferative neoplasms (MPNs) (112).
In addition to neutrophils, other immune cells, such as eosinophils (172), dendritic cells (173), monocytes/macrophages (75, 174), mast cells (175), basophils (176), and lymphocytes (177, 178), may also generate extracellular traps (ETs), indicating possible multiple origins of extracellular DNA in cancer. However, because the different cell types of ETs share the biomarker citH3, there are no accurate methods that can differentiate NETs and other cell types of ETs in tumor microenvironments. Moreover, due to a lack of studies on other cell types of ETs, the mechanism of ET formation in different cell types is still largely understood. It will be necessary to conduct future research to understand the definite mechanism of ET formation and explore the potential clinical translational value of ETs in cancer treatment.
As a result of recent basic and clinical research implicating NETs in resistance to cancer therapy, the status of NETs as a research topic is rapidly changing (14–18, 54, 55, 179). As chemotherapy, radiation therapy, and immunotherapy are essential for cancer treatment, new strategies must be developed to mitigate resistance. NETs are becoming increasingly relevant in cancer therapy resistance, and we review the recent studies that support this relevance (Figure 2).
There is increasing evidence that TANs play an important role in the TME, which is thought to play a central role in resistance to cancer therapy (9, 33–38). As a result of their production of cytokines and chemokines, neutrophils are known to potentiate the survival mechanisms of cancer cells and therefore inhibit the response to therapies (6, 7, 36, 39–45). In recent years, it has been proposed that neutropenia is more than just an indication of sufficient therapeutic dosing but also of resistance mechanisms dependent on TAN (21, 39, 46, 49). It is well known that neutrophils play an important role in cancer treatment resistance, leading to an interest in NETosis as a potential mechanism (127, 138, 156, 157).
A limited number of studies have examined the clinical association between the level of NET formation and response to chemotherapy, with preliminary in vitro and in vivo data indicating that NETosis is a mechanism of chemoresistance. For instance, multiple myeloma (MM) cells were chemoresistant after treatment with doxorubicin (179). In the presence of anthracycline drugs such as doxorubicin, NETs are internalized by neoplastic cells, detoxifying the microenvironment. In animal models, degradation of NETs by DNase restored chemosensitivity, supporting the idea that NETs contribute to chemoresistance. Although, to our knowledge, few studies have been reported describing NETs in chemoresistance, extracellular traps (ETs) in acute promyelocytic leukemia (APL) cells have been observed after treatment with all-trans retinoic acid (ATRA) and arsenic trioxide (ATO) (140, 180–183). These findings are noteworthy since they suggest that NETs or ETs may be potential therapeutic targets for improving chemotherapy responses.
Although radiation therapy is a common treatment for cancer, a significant proportion of patients develop resistance, making it difficult to control tumors locally. In addition to immunological changes in the TME postradiotherapy, neutrophils have been studied for their novel roles in radiotherapy resistance (184, 185). In addition, recent research has indicated that neutrophils play a functional role in radiotherapy resistance through the formation of NETs (54, 55). Studies have also shown that neutrophils play a pivotal role in radiotherapy resistance by forming NETs. After tumor irradiation (IR), NETs form in tumor microenvironments, contributing to radiotherapy resistance. NE inhibitors (NEi) and DNase 1 were effective in inhibiting NETosis and NET degradation, respectively, and they thus increased the effectiveness of radiation therapy, suggesting that these inhibitors may be used to enhance radiation therapy. In addition, researchers have found that NETs were more likely to form in tumors of patients with a poor response to radiation treatment, which correlated with poor outcomes. A combination of NET-based clinical exploration may be a novel and promising treatment for radiotherapy resistance.
It is well described that the enzyme ectonucleotide pyrophosphatase/phosphodiesterase 1 (ENPP1) helps regulate soft tissue mineralization as well as skeletal mineralization (186). ENPP1 is well recognized for its roles in purinergic signaling, a form of signaling that is inextricably linked with cancer incidence (187, 188). Breast cancer patients with local recurrence or failure (LRF) after surgery and IR have a dismal prognosis. Notably, Enpp1 caused LRF in a newly developed and refined animal model established in a recent study (54). High expression of Enpp1 in circulating tumor cells (CTCs) results in relapse, requiring PMN-MDSC and NET infiltration within tumors. Enpp1 inhibition or genetic and pharmacological NET blockade might prolong relapse-free survival (54). Enpp1-derived adenosinergic metabolites increased Haptoglobin (Hp) expression, causing myeloid invasion and NETosis. In relapsed human breast cancer tumors, ENPP1 and NET levels were notably elevated. A combination of NET inhibitors may attenuate radiotherapeutic resistance in clinical trials.
Cancer immunotherapy has attracted considerable attention in recent years. The composition of the TME profoundly affects the success of immunotherapy. The molecular mechanisms underlying immune checkpoint blockade resistance are poorly understood and have been the subjects of intense scrutiny in recent years. In several recent studies, NETs have been examined as novel mediators in some tumors that resist checkpoint inhibition (14–18). Using a PDAC model, Zhang et al. demonstrated that IL17 triggered the formation of NETs within tumors, which can lead to the exhaustion of cytotoxic CD8+ T cells in tumors. In addition, IL17 blockade enhanced tumor cell sensitivity to immune checkpoint blockade. Moreover, abrogating NETs through genetic and pharmacological treatment can lead to the same immunotherapy resistance phenotype, indicating that NETs participate in immunotherapy resistance.
Agonists of CXCR1 and CXCR2 have been demonstrated to cause the development of NETs in 4T1-bearing mice, protecting cells from immune cytotoxicity and negatively affecting the efficacy of checkpoint inhibitors (16). NETs coat tumor cells, preventing them from contacting CD8+ T cells and natural killer cells. NETs are necessary for this protection against cytotoxicity because DNase 1 treatment restored effector-target contact and subsequently cancer cell death. Intravital microscopy experiments were performed with lung carcinoma model mice, and the findings confirmed this mechanism of NET protection in vivo.
A novel mechanism in which T-cell exhaustion is regulated by NET formation in liver ischemia/reperfusion (I/R) in a cancer metastasis model was proposed in a recent report (14). In this study, NETs in the tumor microenvironment inhibited the response of T cells by inducing T-cell metabolic and functional exhaustion, thereby enhancing tumor growth. The treatment of mice with DNase inhibited NET formation in vivo, resulting in attenuated tumor growth, a reduced NETosis rate and higher levels of functioning T cells. Immunosuppressive effects of PD-L1 on T-cell exhaustion in the presence of NETs have been reported. Experiments with clinical samples from patients with colorectal liver metastases validated these PD-L1 effects.
NETs have been explored in various types of cancers in recent years. Whether NETs participate in immunotherapy resistance in these different types of cancers has not been fully investigated. In addition, although NETs have been shown to promote checkpoint resistance in previous studies, the underlying mechanism of this process remains unclear. Does a DNA sensor similar to CCDC25 bind NETs on the surface of T cells? If this sensor is present on T cells, could it be a new marker for predicting the effectiveness of immunotherapy?
Considering the present findings, further investigation into NET-targeting therapeutics in combination with immunotherapy is warranted for patients who would otherwise have poor responses to immunotherapy (1, 2).
Figure 1 The potential role of NETs in tumor progression and metastasis. As scaffolds, NETs capture cancer cells and provide a microenvironment in which protumor genic proteins can be delivered to cancer cells. As part of NETs, HMGB1 is released, activating TLR9-dependent pathways in cancer cells. The NE released by NETs triggers the TLR-4 receptor on cancer cells, resulting in the upregulation of PGC-1, increased mitochondrial biogenesis, and accelerated growth. The transmembrane protein CCDC25 on cancer cells senses extracellular DNA and activates the ILK-parvin pathway to enhance cell motility. In turn, certain factors secreted by many primary tumors have been shown to promote NET formation, such as cytokines (HIF-1, IL-8, IL-6), exosomes and proteases (CTSCs).
Figure 2 NETs in cancer therapy resistance. After chemotherapy and radiotherapy, DAMPs (HMGB1) or other signals from cancer cells induce NET generation, contributing to therapy resistance. Neutrophils in tumor microenvironments expelled NETs, which protected tumor cells from cytotoxicity through CD8+ T cells and NK In addition, NETs decorated with PD-L1 neutralized the function of CD8+ T cells, resulting in checkpoint blockade in immunotherapy.
Crosstalk between NET formation and the TME indicates the ways NETs contribute to cancer progression and metastasis. NETs can promote cancer growth, metastasis and treatment resistance to chemotherapy, checkpoint inhibitors and radiotherapy. However, despite the increasing interest in NETs in potential cancer therapies, more studies are required to explore the possibility of pharmacologically interfering with NET formation.
Conceptualization: JZ. Writing original draft preparation: JJ. Writing editing: JJ. Figures: JZ and JJ. All authors contributed to the article and approved the submitted version.
This work was supported by the Natural Science Foundation of Changsha City (kq2202026).
We thank Ge Mang for technical assistance in creating the figures in this manuscript.
The authors declare that the research was conducted in the absence of any commercial or financial relationships that could be construed as a potential conflict of interest.
All claims expressed in this article are solely those of the authors and do not necessarily represent those of their affiliated organizations, or those of the publisher, the editors and the reviewers. Any product that may be evaluated in this article, or claim that may be made by its manufacturer, is not guaranteed or endorsed by the publisher.
APL, acute promyelocytic leukemia; ATO, arsenic trioxide; ATRA, all trans retinoic acid; CitH3, citrullinated histone H3; CTCs, circulating tumor cells (CTCs); DAMPs, danger-associated molecular patterns; DLBLC, diffuse large B-cell lymphoma; HMGB1, high mobility group box 1; HNC, head and neck cancer; IL-1β, interleukin-1β;LRF, local recurrence or failure; MDSC: myeloid-derived suppressor cells; MM, multiple myeloma; MPN, myeloproliferative neoplasms; MPO, myeloperoxidase; NE, neutrophil elastase; NETs, neutrophil extracellular traps; PAD4, Peptidyl arginine-deiminase 4; PMA, phorbol myristate acetate; PMN, polymorphonuclear neutrophil; PR3, proteinase 3; TAMs, tumor-associated macrophages; TAN, Tumor-associated neutrophil; TME, tumor microenvironment; TNF, tumor necrosis factor; VTE, Venous thromboembolism; WT, wild type.
1. Jorch SK, Kubes P. An emerging role for neutrophil extracellular traps in noninfectious disease. Nat Med (2017) 23(3):279–87. doi: 10.1038/nm.4294
2. Lorenz G, Anders HJ. Neutrophils, dendritic cells, toll-like receptors, and interferon-alpha in lupus nephritis. Semin Nephrol (2015) 35(5):410–26. doi: 10.1016/j.semnephrol.2015.08.003
3. Leffler J, Martin M, Gullstrand B, Tyden H, Lood C, Truedsson L, et al. Neutrophil extracellular traps that are not degraded in systemic lupus erythematosus activate complement exacerbating the disease. J Immunol (2012) 188(7):3522–31. doi: 10.4049/jimmunol.1102404
4. Sahebari M, Roshandel G, Saadati N, Saghafi M, Abdolahi N, Rezaieyazdi Z. Cathelicidin (LL-37) and its correlation with pro-oxidant, antioxidant balance and disease activity in systemic lupus erythematosus: a cross-sectional human study. Lupus (2017) 26(9):975–82. doi: 10.1177/0961203317691368
5. Marcos V, Zhou Z, Yildirim AO, Bohla A, Hector A, Vitkov L, et al. CXCR2 mediates NADPH oxidase-independent neutrophil extracellular trap formation in cystic fibrosis airway inflammation. Nat Med (2010) 16(9):1018–23. doi: 10.1038/nm.2209
6. Gray RD, Hardisty G, Regan KH, Smith M, Robb CT, Duffin R, et al. Delayed neutrophil apoptosis enhances NET formation in cystic fibrosis. Thorax (2018) 73(2):134–44. doi: 10.1136/thoraxjnl-2017-210134
7. Zhou P, Li T, Jin J, Liu Y, Li B, Sun Q, et al. Interactions between neutrophil extracellular traps and activated platelets enhance procoagulant activity in acute stroke patients with ICA occlusion. EBioMedicine (2020) 53:102671. doi: 10.1016/j.ebiom.2020.102671
8. Middleton EA, He XY, Denorme F, Campbell RA, Ng D, Salvatore SP, et al. Neutrophil extracellular traps contribute to immunothrombosis in COVID-19 acute respiratory distress syndrome. Blood (2020) 136(10):1169–79. doi: 10.1182/blood.2020007008
9. Hisada Y, Grover SP, Maqsood A, Houston R, Ay C, Noubouossie DF, et al. Neutrophils and neutrophil extracellular traps enhance venous thrombosis in mice bearing human pancreatic tumors. Haematologica (2020) 105(1):218–25. doi: 10.3324/haematol.2019.217083
10. Wong SL, Demers M, Martinod K, Gallant M, Wang Y, Goldfine AB, et al. Diabetes primes neutrophils to undergo NETosis, which impairs wound healing. Nat Med (2015) 21(7):815–9. doi: 10.1038/nm.3887
11. Doring Y, Soehnlein O, Weber C. Neutrophil extracellular traps in atherosclerosis and atherothrombosis. Circ Res (2017) 120(4):736–43. doi: 10.1161/CIRCRESAHA.116.309692
12. Franck G, Mawson TL, Folco EJ, Molinaro R, Ruvkun V, Engelbertsen D, et al. Roles of PAD4 and NETosis in experimental atherosclerosis and arterial injury: Implications for superficial erosion. Circ Res (2018) 123(1):33–42. doi: 10.1161/CIRCRESAHA.117.312494
13. An Z, Li J, Yu J, Wang X, Gao H, Zhang W, et al. Neutrophil extracellular traps induced by IL-8 aggravate atherosclerosis via activation NF-kappaB signaling in macrophages. Cell Cycle (2019) 18(21):2928–38. doi: 10.1080/15384101.2019.1662678
14. Kaltenmeier C, Yazdani HO, Morder K, Geller DA, Simmons RL, Tohme S. Neutrophil extracellular traps promote T cell exhaustion in the tumor microenvironment. Front Immunol (2021) 12:785222. doi: 10.3389/fimmu.2021.785222
15. Shahzad MH, Feng L, Su X, Brassard A, Dhoparee-Doomah I, Ferri LE, et al. Neutrophil extracellular traps in cancer therapy resistance. Cancers (Basel) 14(5) (2022) 14(5):1359. doi: 10.3390/cancers14051359
16. Teijeira A, Garasa S, Gato M, Alfaro C, Migueliz I, Cirella A, et al. CXCR1 and CXCR2 chemokine receptor agonists produced by tumors induce neutrophil extracellular traps that interfere with immune cytotoxicity. Immunity (2020) 52(5):856–871 e8. doi: 10.1016/j.immuni.2020.03.001
17. Zhang H, Wang Y, Onuma A, He J, Wang H, Xia Y, et al. Neutrophils extracellular traps inhibition improves PD-1 blockade immunotherapy in colorectal cancer. Cancers (Basel) (2021) 13(21):5333. doi: 10.3390/cancers13215333
18. Zhang Y, Chandra V, Riquelme Sanchez E, Dutta P, Quesada PR, Rakoski A, et al. Interleukin-17-induced neutrophil extracellular traps mediate resistance to checkpoint blockade in pancreatic cancer. J Exp Med (2020) 217(12):e20190354. doi: 10.1084/jem.20190354
19. Jiang ZZ, Peng ZP, Liu XC, Guo HF, Zhou MM, Jiang D, et al. Neutrophil extracellular traps induce tumor metastasis through dual effects on cancer and endothelial cells. Oncoimmunology (2022) 11(1):2052418. doi: 10.1080/2162402X.2022.2052418
20. Lee W, Ko SY, Mohamed MS, Kenny HA, Lengyel E, Naora H. Neutrophils facilitate ovarian cancer premetastatic niche formation in the omentum. J Exp Med (2019) 216(1):176–94. doi: 10.1084/jem.20181170
21. Monti M, De Rosa V, Iommelli F, Carriero MV, Terlizzi C, Camerlingo R, et al. Neutrophil extracellular traps as an adhesion substrate for different tumor cells expressing RGD-binding integrins. Int J Mol Sci (2018) 19(8):2350. doi: 10.3390/ijms19082350
22. Nie M, Yang L, Bi X, Wang Y, Sun P, Yang H, et al. Neutrophil extracellular traps induced by IL8 promote diffuse Large b-cell lymphoma progression via the TLR9 signaling. Clin Cancer Res (2019) 25(6):1867–79. doi: 10.1158/1078-0432.CCR-18-1226
23. Park J, Wysocki RW, Amoozgar Z, Maiorino L, Fein MR, Jorns J, et al. Cancer cells induce metastasis-supporting neutrophil extracellular DNA traps. Sci Transl Med (2016) 8(361):361ra138. doi: 10.1126/scitranslmed.aag1711
24. Tohme S, Yazdani HO, Al-Khafaji AB, Chidi AP, Loughran P, Mowen K, et al. Neutrophil extracellular traps promote the development and progression of liver metastases after surgical stress. Cancer Res (2016) 76(6):1367–80. doi: 10.1158/0008-5472.CAN-15-1591
25. Xiao Y, Cong M, Li J, He D, Wu Q, Tian P, et al. Cathepsin c promotes breast cancer lung metastasis by modulating neutrophil infiltration and neutrophil extracellular trap formation. Cancer Cell (2021) 39(3):423–437 e7. doi: 10.1016/j.ccell.2020.12.012
26. Yang L, Liu Q, Zhang X, Liu X, Zhou B, Chen J, et al. DNA Of neutrophil extracellular traps promotes cancer metastasis via CCDC25. Nature (2020) 583(7814):133–8. doi: 10.1038/s41586-020-2394-6
27. Yang LY, Luo Q, Lu L, Zhu WW, Sun HT, Wei R, et al. Increased neutrophil extracellular traps promote metastasis potential of hepatocellular carcinoma via provoking tumorous inflammatory response. J Hematol Oncol (2020) 13(1):3. doi: 10.1186/s13045-019-0836-0
28. Yazdani HO, Roy E, Comerci AJ, van der Windt DJ, Zhang H, Huang H, et al. Neutrophil extracellular traps drive mitochondrial homeostasis in tumors to augment growth. Cancer Res (2019) 79(21):5626–39. doi: 10.1158/0008-5472.CAN-19-0800
29. Aggarwal BB, Shishodia S, Sandur SK, Pandey MK, Sethi G. Inflammation and cancer: how hot is the link? Biochem Pharmacol (2006) 72(11):1605–21. doi: 10.1016/j.bcp.2006.06.029
30. Whiteside TL. The tumor microenvironment and its role in promoting tumor growth. Oncogene (2008) 27(45):5904–12. doi: 10.1038/onc.2008.271
31. Engblom C, Pfirschke C, Pittet MJ. The role of myeloid cells in cancer therapies. Nat Rev Cancer (2016) 16(7):447–62. doi: 10.1038/nrc.2016.54
32. Allavena P, Anfray C, Ummarino A, Andon FT. Therapeutic manipulation of tumor-associated macrophages: Facts and hopes from a clinical and translational perspective. Clin Cancer Res (2021) 27(12):3291–7. doi: 10.1158/1078-0432.CCR-20-1679
33. Noy R, Pollard JW. Tumor-associated macrophages: from mechanisms to therapy. Immunity (2014) 41(1):49–61. doi: 10.1016/j.immuni.2014.06.010
34. Pittet MJ, Michielin O, Migliorini D. Clinical relevance of tumour-associated macrophages. Nat Rev Clin Oncol (2022) 19(6):402–21. doi: 10.1038/s41571-022-00620-6
35. Vitale I, Manic G, Coussens LM, Kroemer G, Galluzzi L. Macrophages and metabolism in the tumor microenvironment. Cell Metab (2019) 30(1):36–50. doi: 10.1016/j.cmet.2019.06.001
36. Yang L, Zhang Y. Tumor-associated macrophages: from basic research to clinical application. J Hematol Oncol (2017) 10(1):58. doi: 10.1186/s13045-017-0430-2
37. Fridlender ZG, Sun J, Kim S, Kapoor V, Cheng G, Ling L, et al. Polarization of tumor-associated neutrophil phenotype by TGF-beta: "N1" versus "N2" TAN. Cancer Cell (2009) 16(3):183–94. doi: 10.1016/j.ccr.2009.06.017
38. Casbon AJ, Reynaud D, Park C, Khuc E, Gan DD, Schepers K, et al. Invasive breast cancer reprograms early myeloid differentiation in the bone marrow to generate immunosuppressive neutrophils. Proc Natl Acad Sci USA (2015) 112(6):E566–75. doi: 10.1073/pnas.1424927112
39. Brinkmann V, Reichard U, Goosmann C, Fauler B, Uhlemann Y, Weiss DS, et al. Neutrophil extracellular traps kill bacteria. Science (2004) 303(5663):1532–5. doi: 10.1126/science.1092385
40. Raftery MJ, Lalwani P, Krautkrmer E, Peters T, Scharffetter-Kochanek K, Kruger R, et al. beta2 integrin mediates hantavirus-induced release of neutrophil extracellular traps. J Exp Med (2014) 211(7):1485–97. doi: 10.1084/jem.20131092
41. Fuchs TA, Abed U, Goosmann C, Hurwitz R, Schulze I, Wahn V, et al. Novel cell death program leads to neutrophil extracellular traps. J Cell Biol (2007) 176(2):231–41. doi: 10.1083/jcb.200606027
42. Sollberger G, Tilley DO, Zychlinsky A. Neutrophil extracellular traps: The biology of chromatin externalization. Dev Cell (2018) 44(5):542–53. doi: 10.1016/j.devcel.2018.01.019
43. Papayannopoulos V. Neutrophil extracellular traps in immunity and disease. Nat Rev Immunol (2018) 18(2):134–47. doi: 10.1038/nri.2017.105
44. Pilsczek FH, Salina D, Poon KK, Fahey C, Yipp BG, Sibley CD, et al. A novel mechanism of rapid nuclear neutrophil extracellular trap formation in response to staphylococcus aureus. J Immunol (2010) 185(12):7413–25. doi: 10.4049/jimmunol.1000675
45. Yousefi S, Mihalache C, Kozlowski E, Schmid I, Simon HU. Viable neutrophils release mitochondrial DNA to form neutrophil extracellular traps. Cell Death Differ (2009) 16(11):1438–44. doi: 10.1038/cdd.2009.96
46. Yipp BG, Kubes P. NETosis: how vital is it? Blood (2013) 122(16):2784–94. doi: 10.1182/blood-2013-04-457671
47. Yipp BG, Petri B, Salina D, Jenne CN, Scott BN, Zbytnuik LD, et al. Infection-induced NETosis is a dynamic process involving neutrophil multitasking in vivo. Nat Med (2012) 18(9):1386–93. doi: 10.1038/nm.2847
48. Rahman S, Gadjeva M. Does NETosis contribute to the bacterial pathoadaptation in cystic fibrosis? Front Immunol (2014) 5:378. doi: 10.3389/fimmu.2014.00378
49. Noubouossie DF, Whelihan MF, Yu YB, Sparkenbaugh E, Pawlinski R, Monroe DM, et al. In vitro activation of coagulation by human neutrophil DNA and histone proteins but not neutrophil extracellular traps. Blood (2017) 129(8):1021–9. doi: 10.1182/blood-2016-06-722298
50. Urban CF, Ermert D, Schmid M, Abu-Abed U, Goosmann C, Nacken W, et al. Neutrophil extracellular traps contain calprotectin, a cytosolic protein complex involved in host defense against candida albicans. PLoS Pathog (2009) 5(10):e1000639. doi: 10.1371/journal.ppat.1000639
51. Negreros M, Flores-Suarez LF. A proposed role of neutrophil extracellular traps and their interplay with fibroblasts in ANCA-associated vasculitis lung fibrosis. Autoimmun. Rev (2021) 20(4):102781. doi: 10.1016/j.autrev.2021.102781
52. Laridan E, Denorme F, Desender L, Francois O, Andersson T, Deckmyn H, et al. Neutrophil extracellular traps in ischemic stroke thrombi. Ann Neurol (2017) 82(2):223–32. doi: 10.1002/ana.24993
53. Martinod K, Wagner DD. Thrombosis: tangled up in NETs. Blood (2014) 123(18):2768–76. doi: 10.1182/blood-2013-10-463646
54. Ruiz-Fernandez de Cordoba B, Moreno H, Valencia K, Perurena N, Ruedas P, Walle T, et al. Tumor ENPP1(CD203a)/Haptoglobin axis exploits myeloid-derived suppressor cells to promote post-radiotherapy local recurrence in breast cancer. Cancer Discov (2022) 12(5):1356–77. doi: 10.1158/2159-8290.CD-21-0932
55. Shinde-Jadhav S, Mansure JJ, Rayes RF, Marcq G, Ayoub M, Skowronski R, et al. Role of neutrophil extracellular traps in radiation resistance of invasive bladder cancer. Nat Commun (2021) 12(1):2776. doi: 10.1038/s41467-021-23086-z
56. Rotondo R, Barisione G, Mastracci L, Grossi F, Orengo AM, Costa R, et al. IL-8 induces exocytosis of arginase 1 by neutrophil polymorphonuclears in nonsmall cell lung cancer. Int J Cancer (2009) 125(4):887–93. doi: 10.1002/ijc.24448
57. Yang TH, St John LS, Garber HR, Kerros C, Ruisaard KE, Clise-Dwyer K, et al. Membrane-associated proteinase 3 on granulocytes and acute myeloid leukemia inhibits T cell proliferation. J Immunol (2018) 201(5):1389–99. doi: 10.4049/jimmunol.1800324
58. el-Hag A, Clark RA. Immunosuppression by activated human neutrophils. dependence on the myeloperoxidase system. J Immunol (1987) 139(7):2406–13.
59. el-Hag A, Lipsky PE, Bennett M, Clark RA. : Immunomodulation by neutrophil myeloperoxidase and hydrogen peroxide: differential susceptibility of human lymphocyte functions. J Immunol (1986) 136(9):3420–6.
60. Zhang Z, Yang C, Li L, Zhu Y, Su K, Zhai L, et al. "gammadeltaT cell-IL17A-Neutrophil" axis drives immunosuppression and confers breast cancer resistance to high-dose anti-VEGFR2 therapy. Front Immunol (2021) 12:699478. doi: 10.3389/fimmu.2021.699478
61. Shojaei F, Singh M, Thompson JD, Ferrara N. Role of Bv8 in neutrophil-dependent angiogenesis in a transgenic model of cancer progression. Proc Natl Acad Sci USA (2008) 105(7):2640–5. doi: 10.1073/pnas.0712185105
62. Shojaei F, Wu X, Zhong C, Yu L, Liang XH, Yao J, et al. Bv8 regulates myeloid-cell-dependent tumour angiogenesis. Nature (2007) 450(7171):825–31. doi: 10.1038/nature06348
63. McCourt M, Wang JH, Sookhai S, Redmond HP. Proinflammatory mediators stimulate neutrophil-directed angiogenesis. Arch Surg (1999) 134(12):1325–31; discussion 1331-2. doi: 10.1001/archsurg.134.12.1325
64. Bekes EM, Schweighofer B, Kupriyanova TA, Zajac E, Ardi VC, Quigley JP, et al. Tumor-recruited neutrophils and neutrophil TIMP-free MMP-9 regulate coordinately the levels of tumor angiogenesis and efficiency of malignant cell intravasation. Am J Pathol (2011) 179(3):1455–70. doi: 10.1016/j.ajpath.2011.05.031
65. Pylaeva E, Harati MD, Spyra I, Bordbari S, Strachan S, Thakur BK, et al. NAMPT signaling is critical for the proangiogenic activity of tumor-associated neutrophils. Int J Cancer (2019) 144(1):136–49. doi: 10.1002/ijc.31808
66. Liang J, Piao Y, Holmes L, Fuller GN, Henry V, Tiao N, et al. Neutrophils promote the malignant glioma phenotype through S100A4. Clin Cancer Res (2014) 20(1):187–98. doi: 10.1158/1078-0432.CCR-13-1279
67. Houghton AM, Rzymkiewicz DM, Ji H, Gregory AD, Egea EE, Metz HE, et al. Neutrophil elastase-mediated degradation of IRS-1 accelerates lung tumor growth. Nat Med (2010) 16(2):219–23. doi: 10.1038/nm.2084
68. Queen MM, Ryan RE, Holzer RG, Keller-Peck CR, Jorcyk CL. Breast cancer cells stimulate neutrophils to produce oncostatin m: potential implications for tumor progression. Cancer Res (2005) 65(19):8896–904. doi: 10.1158/0008-5472.CAN-05-1734
69. Kerfoot SM, Raharjo E, Ho M, Kaur J, Serirom S, McCafferty DM, et al. Exclusive neutrophil recruitment with oncostatin m in a human system. Am J Pathol (2001) 159(4):1531–9. doi: 10.1016/S0002-9440(10)62538-2
70. Lichtenstein A, Seelig M, Berek J, Zighelboim J. Human neutrophil-mediated lysis of ovarian cancer cells. Blood (1989) 74(2):805–9. doi: 10.1182/blood.V74.2.805.805
71. Martin A, Seignez C, Racoeur C, Isambert N, Mabrouk N, Scagliarini A, et al. Tumor-derived granzyme b-expressing neutrophils acquire antitumor potential after lipid a treatment. Oncotarget (2018) 9(47):28364–78. doi: 10.18632/oncotarget.25342
72. Finisguerra V, Di Conza G, Di Matteo M, Serneels J, Costa S, Thompson AA, et al. MET is required for the recruitment of anti-tumoural neutrophils. Nature (2015) 522(7556):349–53. doi: 10.1038/nature14407
73. Yee PP, Wei Y, Kim SY, Lu T, Chih SY, Lawson C, et al. Neutrophil-induced ferroptosis promotes tumor necrosis in glioblastoma progression. Nat Commun (2020) 11(1):5424. doi: 10.1038/s41467-020-19193-y
74. Cui C, Chakraborty K, Tang XA, Zhou G, Schoenfelt KQ, Becker KM, et al. Neutrophil elastase selectively kills cancer cells and attenuates tumorigenesis. Cell (2021) 184(12):3163–3177 e21. doi: 10.1016/j.cell.2021.04.016
75. Xu SS, Li H, Li TJ, Li S, Xia HY, Long J, et al. Neutrophil extracellular traps and macrophage extracellular traps predict postoperative recurrence in resectable nonfunctional pancreatic neuroendocrine tumors. Front Immunol (2021) 12:577517. doi: 10.3389/fimmu.2021.577517
76. Abdol Razak N, Elaskalani O, Metharom P. Pancreatic cancer-induced neutrophil extracellular traps: A potential contributor to cancer-associated thrombosis. Int J Mol Sci (2017) 18(3):487. doi: 10.3390/ijms18030487
77. Boone BA, Murthy P, Miller-Ocuin J, Doerfler WR, Ellis JT, Liang X, et al. 3rd: Chloroquine reduces hypercoagulability in pancreatic cancer through inhibition of neutrophil extracellular traps. BMC Cancer (2018) 18(1):678. doi: 10.1186/s12885-018-4584-2
78. Campello E, Ilich A, Simioni P, Key NS. The relationship between pancreatic cancer and hypercoagulability: a comprehensive review on epidemiological and biological issues. Br J Cancer (2019) 121(5):359–71. doi: 10.1038/s41416-019-0510-x
79. Deng J, Kang Y, Cheng CC, Li X, Dai B, Katz MH, et al. DDR1-induced neutrophil extracellular traps drive pancreatic cancer metastasis. JCI Insight (2021) 6(17):e146133. doi: 10.1172/jci.insight.146133
80. Jin W, Xu HX, Zhang SR, Li H, Wang WQ, Gao HL, et al. Tumor-infiltrating NETs predict postsurgical survival in patients with pancreatic ductal adenocarcinoma. Ann Surg Oncol (2019) 26(2):635–43. doi: 10.1245/s10434-018-6941-4
81. Jin W, Yin H, Li H, Yu XJ, Xu HX, Liu L. Neutrophil extracellular DNA traps promote pancreatic cancer cells migration and invasion by activating EGFR/ERK pathway. J Cell Mol Med (2021) 25(12):5443–56. doi: 10.1111/jcmm.16555
82. Kajioka H, Kagawa S, Ito A, Yoshimoto M, Sakamoto S, Kikuchi S, et al. Targeting neutrophil extracellular traps with thrombomodulin prevents pancreatic cancer metastasis. Cancer Lett (2021) 497:1–13. doi: 10.1016/j.canlet.2020.10.015
83. Miller-Ocuin JL, Liang X, Boone BA, Doerfler WR, Singhi AD, Tang D, et al. 3rd: DNA released from neutrophil extracellular traps (NETs) activates pancreatic stellate cells and enhances pancreatic tumor growth. Oncoimmunology (2019) 8(9):e1605822. doi: 10.1080/2162402X.2019.1605822
84. Schoeps B, Eckfeld C, Prokopchuk O, Bottcher J, Haussler D, Steiger K, et al. TIMP1 triggers neutrophil extracellular trap formation in pancreatic cancer. Cancer Res (2021) 81(13):3568–79. doi: 10.1158/0008-5472.CAN-20-4125
85. Yu M, Li T, Li B, Liu Y, Wang L, Zhang J, et al. Phosphatidylserine-exposing blood cells, microparticles and neutrophil extracellular traps increase procoagulant activity in patients with pancreatic cancer. Thromb Res (2020) 188:5–16. doi: 10.1016/j.thromres.2020.01.025
86. van der Windt DJ, Sud V, Zhang H, Varley PR, Goswami J, Yazdani HO, et al. Neutrophil extracellular traps promote inflammation and development of hepatocellular carcinoma in nonalcoholic steatohepatitis. Hepatology (2018) 68(4):1347–60. doi: 10.1002/hep.29914
87. Wang H, Zhang H, Wang Y, Brown ZJ, Xia Y, Huang Z, et al. Regulatory T-cell and neutrophil extracellular trap interaction contributes to carcinogenesis in non-alcoholic steatohepatitis. J Hepatol (2021) 75(6):1271–83. doi: 10.1016/j.jhep.2021.07.032
88. Yang LY, Shen XT, Sun HT, Zhu WW, Zhang JB, Lu L. Neutrophil extracellular traps in hepatocellular carcinoma are enriched in oxidized mitochondrial DNA which is highly pro-inflammatory and pro-metastatic. J Cancer (2022) 13(4):1261–71. doi: 10.7150/jca.64170
89. Zenlander R, Havervall S, Magnusson M, Engstrand J, Agren A, Thalin C, et al. Neutrophil extracellular traps in patients with liver cirrhosis and hepatocellular carcinoma. Sci Rep (2021) 11(1):18025. doi: 10.1038/s41598-021-97233-3
90. Guan X, Lu Y, Zhu H, Yu S, Zhao W, Chi X, et al. The crosstalk between cancer cells and neutrophils enhances hepatocellular carcinoma metastasis via neutrophil extracellular traps-associated cathepsin G component: A potential therapeutic target. J Hepatocell Carcinoma (2021) 8:451–65. doi: 10.2147/JHC.S303588
91. Seo JD, Gu JY, Jung HS, Kim YJ, Kim HK. Contact system activation and neutrophil extracellular trap markers: Risk factors for portal vein thrombosis in patients with hepatocellular carcinoma. Clin Appl Thromb Hemost (2019) 25:1076029618825310. doi: 10.1177/1076029618825310
92. Arelaki S, Arampatzioglou A, Kambas K, Papagoras C, Miltiades P, Angelidou I, et al. Gradient infiltration of neutrophil extracellular traps in colon cancer and evidence for their involvement in tumour growth. PLoS One (2016) 11(5):e0154484. doi: 10.1371/journal.pone.0154484
93. Rayes RF, Mouhanna JG, Nicolau I, Bourdeau F, Giannias B, Rousseau S, et al. Primary tumors induce neutrophil extracellular traps with targetable metastasis promoting effects. JCI Insight (2019) 5. doi: 10.1172/jci.insight.128008
94. Rayes RF, Vourtzoumis P, Bou Rjeily M, Seth R, Bourdeau F, Giannias B, et al. Neutrophil extracellular trap-associated CEACAM1 as a putative therapeutic target to prevent metastatic progression of colon carcinoma. J Immunol (2020) 204(8):2285–94. doi: 10.4049/jimmunol.1900240
95. Ren J, He J, Zhang H, Xia Y, Hu Z, Loughran P, et al. Platelet TLR4-ERK5 axis facilitates NET-mediated capturing of circulating tumor cells and distant metastasis after surgical stress. Cancer Res (2021) 81(9):2373–85. doi: 10.1158/0008-5472.CAN-20-3222
96. Shang A, Gu C, Zhou C, Yang Y, Chen C, Zeng B, et al. Exosomal KRAS mutation promotes the formation of tumor-associated neutrophil extracellular traps and causes deterioration of colorectal cancer by inducing IL-8 expression. Cell Commun Signal (2020) 18(1):52. doi: 10.1186/s12964-020-0517-1
97. Stehr AM, Wang G, Demmler R, Stemmler MP, Krug J, Tripal P, et al. Neutrophil extracellular traps drive epithelial-mesenchymal transition of human colon cancer. J Pathol (2022) 256(4):455–67. doi: 10.1002/path.5860
98. Yang L, Liu L, Zhang R, Hong J, Wang Y, Wang J, et al. IL-8 mediates a positive loop connecting increased neutrophil extracellular traps (NETs) and colorectal cancer liver metastasis. J Cancer (2020) 11(15):4384–96. doi: 10.7150/jca.44215
99. Zhang Y, Wang C, Yu M, Zhao X, Du J, Li Y, et al. Neutrophil extracellular traps induced by activated platelets contribute to procoagulant activity in patients with colorectal cancer. Thromb Res (2019) 180:87–97. doi: 10.1016/j.thromres.2019.06.005
100. Chu ZQ, Zhang KC, Chen L. Neutrophil extracellular traps in gastrointestinal cancer. World J Gastroenterol (2021) 27(33):5474–87. doi: 10.3748/wjg.v27.i33.5474
101. Li R, Zou X, Zhu T, Xu H, Li X, Zhu L. Destruction of neutrophil extracellular traps promotes the apoptosis and inhibits the invasion of gastric cancer cells by regulating the expression of bcl-2, bax and NF-kappaB. Onco Targets Ther (2020) 13:5271–81. doi: 10.2147/OTT.S227331
102. Xia X, Zhang Z, Zhu C, Ni B, Wang S, Yang S, et al. Neutrophil extracellular traps promote metastasis in gastric cancer patients with postoperative abdominal infectious complications. Nat Commun (2022) 13(1):1017. doi: 10.1038/s41467-022-28492-5
103. Yang C, Sun W, Cui W, Li X, Yao J, Jia X, et al. Procoagulant role of neutrophil extracellular traps in patients with gastric cancer. Int J Clin Exp Pathol (2015) 8(11):14075–86.
104. Zhang Y, Hu Y, Ma C, Sun H, Wei X, Li M, et al. Diagnostic, therapeutic predictive, and prognostic value of neutrophil extracellular traps in patients with gastric adenocarcinoma. Front Oncol (2020) 10:1036. doi: 10.3389/fonc.2020.01036
105. Zhu T, Zou X, Yang C, Li L, Wang B, Li R, et al. Neutrophil extracellular traps promote gastric cancer metastasis by inducing epithelialmesenchymal transition. Int J Mol Med (2021) 48(1):127. doi: 10.3892/ijmm.2021.4960
106. Zhang H, Lv H, Weng M, Wang H, Cata JP, Chen W, et al. Preoperative leukocytosis is associated with increased tumor-infiltrating neutrophil extracellular traps and worse outcomes in esophageal cancer. Ann Transl Med (2020) 8(7):441. doi: 10.21037/atm.2020.03.190
107. Guglietta S, Chiavelli A, Zagato E, Krieg C, Gandini S, Ravenda PS, et al. Coagulation induced by C3aR-dependent NETosis drives protumorigenic neutrophils during small intestinal tumorigenesis. Nat Commun (2016) 7:11037. doi: 10.1038/ncomms11037
108. Li Y, Yuan R, Ren T, Yang B, Miao H, Liu L, et al. Role of sciellin in gallbladder cancer proliferation and formation of neutrophil extracellular traps. Cell Death Dis (2021) 12(1):30. doi: 10.1038/s41419-020-03286-z
109. Cristinziano L, Modestino L, Loffredo S, Varricchi G, Braile M, Ferrara AL, et al. Anaplastic thyroid cancer cells induce the release of mitochondrial extracellular DNA traps by viable neutrophils. J Immunol (2020) 204(5):1362–72. doi: 10.4049/jimmunol.1900543
110. Cai Z, Zhang M, Boafo Kwantwi L, Bi X, Zhang C, Cheng Z, et al. Breast cancer cells promote self-migration by secreting interleukin 8 to induce NET formation. Gene (2020) 754:144902. doi: 10.1016/j.gene.2020.144902
111. Cao W, Zhu MY, Lee SH, Lee SB, Kim HJ, Park BO, et al. Modulation of cellular NAD(+) attenuates cancer-associated hypercoagulability and thrombosis via the inhibition of tissue factor and formation of neutrophil extracellular traps. Int J Mol Sci (2021) 22(21):12085. doi: 10.3390/ijms222112085
112. Wolach O, Sellar RS, Martinod K, Cherpokova D, McConkey M, Chappell RJ, et al. Increased neutrophil extracellular trap formation promotes thrombosis in myeloproliferative neoplasms. Sci Transl Med (2018) 10(436). doi: 10.1126/scitranslmed.aan8292
113. Gomes T, Varady CBS, Lourenco AL, Mizurini DM, Rondon AMR, Leal AC, et al. IL-1beta blockade attenuates thrombosis in a neutrophil extracellular trap-dependent breast cancer model. Front Immunol (2019) 10:2088. doi: 10.3389/fimmu.2019.02088
114. Martins-Cardoso K, Almeida VH, Bagri KM, Rossi MID, Mermelstein CS, Konig S, et al. Neutrophil extracellular traps (NETs) promote pro-metastatic phenotype in human breast cancer cells through epithelial-mesenchymal transition. Cancers (Basel) (2020) 12(6):1542. doi: 10.3390/cancers12061542
115. Peng Z, Liu C, Victor AR, Cao DY, Veiras LC, Bernstein EA, et al. Tumors exploit CXCR4(hi)CD62L(lo) aged neutrophils to facilitate metastatic spread. Oncoimmunology (2021) 10(1):1870811. doi: 10.1080/2162402X.2020.1870811
116. Safarulla S, Madan A, Xing F, Chandrasekaran A. CXCR2 mediates distinct neutrophil behavior in brain metastatic breast tumor. Cancers (Basel) (2022) 14(3):515. doi: 10.3390/cancers14030515
117. Todorova VK, Hsu PC, Wei JY, Lopez-Candales A, Chen JZ, Su LJ, et al. Biomarkers of inflammation, hypercoagulability and endothelial injury predict early asymptomatic doxorubicin-induced cardiotoxicity in breast cancer patients. Am J Cancer Res (2020) 10(9):2933–45.
118. Wang Z, Yang C, Li L, Jin X, Zhang Z, Zheng H, et al. Tumor-derived HMGB1 induces CD62L(dim) neutrophil polarization and promotes lung metastasis in triple-negative breast cancer. Oncogenesis (2020) 9(9):82. doi: 10.1038/s41389-020-00267-x
119. Yang C, Wang Z, Li L, Zhang Z, Jin X, Wu P, et al. Aged neutrophils form mitochondria-dependent vital NETs to promote breast cancer lung metastasis. J Immunother Cancer (2021) 9(10). doi: 10.1136/jitc-2021-002875
120. Zhu B, Zhang X, Sun S, Fu Y, Xie L, Ai P. NF-kappaB and neutrophil extracellular traps cooperate to promote breast cancer progression and metastasis. Exp Cell Res (2021) 405(2):112707. doi: 10.1016/j.yexcr.2021.112707
121. Varady CBS, Oliveira AC, Monteiro RQ, Gomes T. Recombinant human DNase I for the treatment of cancer-associated thrombosis: A pre-clinical study. Thromb Res (2021) 203:131–7. doi: 10.1016/j.thromres.2021.04.028
122. Zeng J, Xu H, Fan PZ, Xie J, He J, Yu J, et al. Kaempferol blocks neutrophil extracellular traps formation and reduces tumour metastasis by inhibiting ROS-PAD4 pathway. J Cell Mol Med (2020) 24(13):7590–9. doi: 10.1111/jcmm.15394
123. Zhang Y, Wang C, Li W, Tian W, Tang C, Xue L, et al. Neutrophil cyto-pharmaceuticals suppressing tumor metastasis via inhibiting hypoxia-inducible factor-1alpha in circulating breast cancer cells. Adv Healthc Mater (2022) 11(3):e2101761. doi: 10.1002/adhm.202101761
124. Zheng Z, Li YN, Jia S, Zhu M, Cao L, Tao M, et al. Lung mesenchymal stromal cells influenced by Th2 cytokines mobilize neutrophils and facilitate metastasis by producing complement C3. Nat Commun (2021) 12(1):6202. doi: 10.1038/s41467-021-26460-z
125. Zha C, Meng X, Li L, Mi S, Qian D, Li Z, et al. Neutrophil extracellular traps mediate the crosstalk between glioma progression and the tumor microenvironment via the HMGB1/RAGE/IL-8 axis. Cancer Biol Med (2020) 17(1):154–68. doi: 10.20892/j.issn.2095-3941.2019.0353
126. Zhang S, Guo M, Liu Q, Liu J, Cui Y. Neutrophil extracellular traps induce a hypercoagulable state in glioma. Immun Inflamm Dis (2021) 9(4):1383–93. doi: 10.1002/iid3.488
127. Li Y, Yang Y, Gan T, Zhou J, Hu F, Hao N, et al. Extracellular RNAs from lung cancer cells activate epithelial cells and induce neutrophil extracellular traps. Int J Oncol (2019) 55(1):69–80. doi: 10.3892/ijo.2019.4808
128. Albrengues J, Shields MA, Ng D, Park CG, Ambrico A, Poindexter ME, et al. Neutrophil extracellular traps produced during inflammation awaken dormant cancer cells in mice. Science (2018) 361(6409). doi: 10.1126/science.aao4227
129. Demers M, Krause D, Schatzberg D, Martinod K, Voorhees J, Fuchs T, et al. Cancers predispose neutrophils to release extracellular DNA traps that contribute to cancer-associated thrombosis. Proc Natl Acad Sci USA (2012) 109(32):13076–81. doi: 10.1073/pnas.1200419109
130. Cools-Lartigue J, Spicer J, McDonald B, Gowing S, Chow S, Giannias B, et al. Neutrophil extracellular traps sequester circulating tumor cells and promote metastasis. J Clin Invest (2013) 123(8):3456–8. doi: 10.1172/JCI67484
131. Inoue M, Nakashima R, Enomoto M, Koike Y, Zhao X, Yip K, et al. Plasma redox imbalance caused by albumin oxidation promotes lung-predominant NETosis and pulmonary cancer metastasis. Nat Commun (2018) 9(1):5116. doi: 10.1038/s41467-018-07550-x
132. Lin CF, Chien SY, Chen CL, Hsieh CY, Tseng PC, Wang YC. IFN-gamma induces mimic extracellular trap cell death in lung epithelial cells through autophagy-regulated DNA damage. J Interferon Cytokine Res (2016) 36(2):100–12. doi: 10.1089/jir.2015.0011
133. Ortiz-Espinosa S, Morales X, Senent Y, Alignani D, Tavira B, Macaya I, et al. Complement C5a induces the formation of neutrophil extracellular traps by myeloid-derived suppressor cells to promote metastasis. Cancer Lett (2022) 529:70–84. doi: 10.1016/j.canlet.2021.12.027
134. Zhang L, Yi H, Chen J, Li H, Luo Y, Cheng T, et al. Neutrophil extracellular traps facilitate A549 cell invasion and migration in a macrophage-maintained inflammatory microenvironment. BioMed Res Int (2022) 2022:8316525. doi: 10.1155/2022/8316525
135. Zhou J, Yang Y, Gan T, Li Y, Hu F, Hao N, et al. Lung cancer cells release high mobility group box 1 and promote the formation of neutrophil extracellular traps. Oncol Lett (2019) 18(1):181–8. doi: 10.3892/ol.2019.10290
136. de Andrea CE, Ochoa MC, Villalba-Esparza M, Teijeira A, Schalper KA, Abengozar-Muela M, et al. Heterogenous presence of neutrophil extracellular traps in human solid tumours is partially dependent on IL-8. J Pathol (2021) 255(2):190–201. doi: 10.1002/path.5753
137. Lee J, Lee D, Lawler S, Kim Y. Role of neutrophil extracellular traps in regulation of lung cancer invasion and metastasis: Structural insights from a computational model. PLoS Comput Biol (2021) 17(2):e1008257. doi: 10.1371/journal.pcbi.1008257
138. Decker AS, Pylaeva E, Brenzel A, Spyra I, Droege F, Hussain T, et al. Prognostic role of blood NETosis in the progression of head and neck cancer. Cells (2019) 8(9):946. doi: 10.3390/cells8090946
139. Millrud CR, Kagedal A, Kumlien Georen S, Winqvist O, Uddman R, Razavi R, et al. NET-producing CD16(high) CD62L(dim) neutrophils migrate to tumor sites and predict improved survival in patients with HNSCC. Int J Cancer (2017) 140(11):2557–67. doi: 10.1002/ijc.30671
140. Wang Y, Wang C, Zuo N, Yang H, Fang S, Shi J. Extracellular traps increase burden of bleeding by damaging endothelial cell in acute promyelocytic leukaemia. Front Immunol (2022) 13:841445. doi: 10.3389/fimmu.2022.841445
141. Berger-Achituv S, Elhasid R. Reduced neutrophil elastase activity and neutrophil extracellular traps in pediatric acute myeloid leukemia may increase the rate of infections. J Pediatr Hematol Oncol (2018) 40(4):e248–52. doi: 10.1097/MPH.0000000000001015
142. Marin Oyarzun CP, Carestia A, Lev PR, Glembotsky AC, Castro Rios MA, Moiraghi B, et al. Neutrophil extracellular trap formation and circulating nucleosomes in patients with chronic myeloproliferative neoplasms. Sci Rep (2016) 6:38738. doi: 10.1038/srep38738
143. Li M, Lin C, Deng H, Strnad J, Bernabei L, Vogl DT, et al. A novel peptidylarginine deiminase 4 (PAD4) inhibitor BMS-P5 blocks formation of neutrophil extracellular traps and delays progression of multiple myeloma. Mol Cancer Ther (2020) 19(7):1530–8. doi: 10.1158/1535-7163.MCT-19-1020
144. Schedel F, Mayer-Hain S, Pappelbaum KI, Metze D, Stock M, Goerge T, et al. Evidence and impact of neutrophil extracellular traps in malignant melanoma. Pigment Cell Melanoma Res (2020) 33(1):63–73. doi: 10.1111/pcmr.12818
145. Liu K, Sun E, Lei M, Li L, Gao J, Nian X, et al. BCG-Induced formation of neutrophil extracellular traps play an important role in bladder cancer treatment. Clin Immunol (2019) 201:4–14. doi: 10.1016/j.clim.2019.02.005
146. Garley M, Dziemianczyk-Pakiela D, Grubczak K, Surazynski A, Dabrowska D, Ratajczak-Wrona W, et al. Differences and similarities in the phenomenon of NETs formation in oral inflammation and in oral squamous cell carcinoma. J Cancer (2018) 9(11):1958–65. doi: 10.7150/jca.24238
147. Garley M, Dziemianczyk-Pakiela D, Ratajczak-Wrona W, Pryczynicz A, Nowak K, Lazarczyk B, et al. NETs biomarkers in saliva and serum OSCC patients: One hypothesis, two conclusions. Adv Med Sci (2022) 67(1):45–54. doi: 10.1016/j.advms.2021.12.004
148. Garley M, Jablonska E, Miltyk W, Grubczak K, Surazynski A, Ratajczak-Wrona W, et al. Cancers cells in traps? the pathways of NETs formation in response to OSCC in humans-a pilot study. Cancer Control (2020) 27(1):1073274820960473. doi: 10.1177/1073274820960473
149. Jablonska E, Garley M, Surazynski A, Grubczak K, Iwaniuk A, Borys J, et al. Neutrophil extracellular traps (NETs) formation induced by TGF-beta in oral lichen planus - possible implications for the development of oral cancer. Immunobiology (2020) 225(2):151901. doi: 10.1016/j.imbio.2019.151901
150. Li B, Liu Y, Hu T, Zhang Y, Zhang C, Li T, et al. Neutrophil extracellular traps enhance procoagulant activity in patients with oral squamous cell carcinoma. J Cancer Res Clin Oncol (2019) 145(7):1695–707. doi: 10.1007/s00432-019-02922-2
151. Ronchetti L, Terrenato I, Ferretti M, Corrado G, Goeman F, Donzelli S, et al. Circulating cell free DNA and citrullinated histone H3 as useful biomarkers of NETosis in endometrial cancer. J Exp Clin Cancer Res (2022) 41(1):151. doi: 10.1186/s13046-022-02359-5
152. Muqaku B, Pils D, Mader JC, Aust S, Mangold A, Muqaku L, et al. Neutrophil extracellular trap formation correlates with favorable overall survival in high grade ovarian cancer. Cancers (Basel) (2020) 12(2):505. doi: 10.3390/cancers12020505
153. Singel KL, Grzankowski KS, Khan A, Grimm MJ, D'Auria AC, Morrell K, et al. Mitochondrial DNA in the tumour microenvironment activates neutrophils and is associated with worse outcomes in patients with advanced epithelial ovarian cancer. Br J Cancer (2019) 120(2):207–17. doi: 10.1038/s41416-018-0339-8
154. Yan B, Dai X, Ma Q, Wu X. Stromal neutrophil extracellular trap density is an independent prognostic factor for cervical cancer recurrence. Front Oncol (2021) 11:659445. doi: 10.3389/fonc.2021.659445
155. Berger-Achituv S, Brinkmann V, Abed UA, Kuhn LI, Ben-Ezra J, Elhasid R, et al. A proposed role for neutrophil extracellular traps in cancer immunoediting. Front Immunol (2013) 4:48. doi: 10.3389/fimmu.2013.00048
156. Thalin C, Lundstrom S, Seignez C, Daleskog M, Lundstrom A, Henriksson P, et al. Citrullinated histone H3 as a novel prognostic blood marker in patients with advanced cancer. PLoS One (2018) 13(1):e0191231. doi: 10.1371/journal.pone.0191231
157. Richardson JJR, Hendrickse C, Gao-Smith F, Thickett DR. Neutrophil extracellular trap production in patients with colorectal cancer in vitro. Int J Inflam (2017) 2017:4915062. doi: 10.1155/2017/4915062
158. Demers M, Wong SL, Martinod K, Gallant M, Cabral JE, Wang Y, et al. Priming of neutrophils toward NETosis promotes tumor growth. Oncoimmunology (2016) 5(5):e1134073. doi: 10.1080/2162402X.2015.1134073
159. Deryugina EI, Zajac E, Juncker-Jensen A, Kupriyanova TA, Welter L, Quigley JP. Tissue-infiltrating neutrophils constitute the major in vivo source of angiogenesis-inducing MMP-9 in the tumor microenvironment. Neoplasia (2014) 16(10):771–88. doi: 10.1016/j.neo.2014.08.013
160. Nguyen YTM, Fujisawa M, Nguyen TB, Suehara Y, Sakamoto T, Matsuoka R, et al. Tet2 deficiency in immune cells exacerbates tumor progression by increasing angiogenesis in a lung cancer model. Cancer Sci (2021) 112(12):4931–43. doi: 10.1111/cas.15165
161. Bald T, Quast T, Landsberg J, Rogava M, Glodde N, Lopez-Ramos D, et al. Ultraviolet-radiation-induced inflammation promotes angiotropism and metastasis in melanoma. Nature (2014) 507(7490):109–13. doi: 10.1038/nature13111
162. Szczerba BM, Castro-Giner F, Vetter M, Krol I, Gkountela S, Landin J, et al. Neutrophils escort circulating tumour cells to enable cell cycle progression. Nature (2019) 566(7745):553–7. doi: 10.1038/s41586-019-0915-y
163. Wculek SK, Malanchi I. Neutrophils support lung colonization of metastasis-initiating breast cancer cells. Nature (2015) 528(7582):413–7. doi: 10.1038/nature16140
164. Kowanetz M, Wu X, Lee J, Tan M, Hagenbeek T, Qu X, et al. Granulocyte-colony stimulating factor promotes lung metastasis through mobilization of Ly6G+Ly6C+ granulocytes. Proc Natl Acad Sci USA (2010) 107(50):21248–55. doi: 10.1073/pnas.1015855107
165. Hisada Y, Mackman N. Cancer-associated pathways and biomarkers of venous thrombosis. Blood (2017) 130(13):1499–506. doi: 10.1182/blood-2017-03-743211
166. Lopez-Salazar J, Ramirez-Tirado LA, Gomez-Contreras N, Pacheco-Bravo I, Cortes E, Gallardo D, et al. Cancer-associated prothrombotic pathways: leucocytosis, but not thrombocytosis, correlates with venous thromboembolism in women with ovarian cancer. Intern Med J (2020) 50(3):366–70. doi: 10.1111/imj.14762
167. Sood SL. Cancer-associated thrombosis. Curr Opin Hematol (2009) 16(5):378–85. doi: 10.1097/MOH.0b013e32832ea31b
168. Khorana A, Francis C, Culakova E, Kuderer N, Lyman G. Thromboembolism is a leading cause of death in cancer patients receiving outpatient chemotherapy. J Thromb haemost. JTH (2007) 5(3):632–4. doi: 10.1111/j.1538-7836.2007.02374.x
169. Sevestre MA, Soudet S. Epidemiology and risk factors for cancer-associated thrombosis. J Med Vasc (2020) 45(6S):6S3–7. doi: 10.1016/S2542-4513(20)30513-7
170. Blom JW, Vanderschoot JP, Oostindier MJ, Osanto S, van der Meer FJ, Rosendaal FR. Incidence of venous thrombosis in a large cohort of 66,329 cancer patients: results of a record linkage study. J Thromb Haemost (2006) 4(3):529–35. doi: 10.1111/j.1538-7836.2006.01804.x
171. Panicker S, Mehta-D'souza P, Zhang N, Klopocki A, Shao B, McEver R. Circulating soluble p-selectin must dimerize to promote inflammation and coagulation in mice. Blood (2017) 130(2):181–91. doi: 10.1182/blood-2017-02-770479
172. Francischetti IMB, Alejo JC, Sivanandham R, Davies-Hill T, Fetsch P, Pandrea I, et al. Neutrophil and eosinophil extracellular traps in Hodgkin lymphoma. Hemasphere (2021) 5(9):e633. doi: 10.1097/HS9.0000000000000633
173. Loures FV, Rohm M, Lee CK, Santos E, Wang JP, Specht CA, et al. Recognition of aspergillus fumigatus hyphae by human plasmacytoid dendritic cells is mediated by dectin-2 and results in formation of extracellular traps. PLoS Pathog (2015) 11(2):e1004643. doi: 10.1371/journal.ppat.1004643
174. Chen T, Wang Y, Nan Z, Wu J, Li A, Zhang T, et al. Interaction between macrophage extracellular traps and colon cancer cells promotes colon cancer invasion and correlates with unfavorable prognosis. Front Immunol (2021) 12:779325. doi: 10.3389/fimmu.2021.779325
175. von Kockritz-Blickwede M, Goldmann O, Thulin P, Heinemann K, Norrby-Teglund A, Rohde M, et al. Phagocytosis-independent antimicrobial activity of mast cells by means of extracellular trap formation. Blood (2008) 111(6):3070–80. doi: 10.1182/blood-2007-07-104018
176. Yousefi S, Morshed M, Amini P, Stojkov D, Simon D, von Gunten S, et al. Basophils exhibit antibacterial activity through extracellular trap formation. Allergy (2015) 70(9):1184–8. doi: 10.1111/all.12662
177. Rocha Arrieta YC, Rojas M, Vasquez G, Lopez J. The lymphocytes stimulation induced DNA release, a phenomenon similar to NETosis. Scand J Immunol (2017) 86(4):229–38. doi: 10.1111/sji.12592
178. Agak GW, Mouton A, Teles RM, Weston T, Morselli M, Andrade PR, et al. Extracellular traps released by antimicrobial TH17 cells contribute to host defense. J Clin Invest (2021) 131(2). doi: 10.1172/JCI141594
179. Ramachandran IR, Condamine T, Lin C, Herlihy SE, Garfall A, Vogl DT, et al. Bone marrow PMN-MDSCs and neutrophils are functionally similar in protection of multiple myeloma from chemotherapy. Cancer Lett (2016) 371(1):117–24. doi: 10.1016/j.canlet.2015.10.040
180. Cao M, Li T, He Z, Wang L, Yang X, Kou Y, et al. Promyelocytic extracellular chromatin exacerbates coagulation and fibrinolysis in acute promyelocytic leukemia. Blood (2017) 129(13):1855–64. doi: 10.1182/blood-2016-09-739334
181. Li T, Ma R, Zhang Y, Mo H, Yang X, Hu S, et al. Arsenic trioxide promoting ETosis in acute promyelocytic leukemia through mTOR-regulated autophagy. Cell Death Dis (2018) 9(2):75. doi: 10.1038/s41419-017-0018-3
182. Ma R, Li T, Cao M, Si Y, Wu X, Zhao L, et al. Extracellular DNA traps released by acute promyelocytic leukemia cells through autophagy. Cell Death Dis (2016) 7(6):e2283. doi: 10.1038/cddis.2016.186
183. Yoo HJ, Lee JS, Kim JE, Gu J, Koh Y, Kim I, et al. Extracellular histone released from leukemic cells increases their adhesion to endothelium and protects them from spontaneous and chemotherapy-induced leukemic cell death. PLoS One (2016) 11(10):e0163982. doi: 10.1371/journal.pone.0163982
184. Wisdom AJ, Hong CS, Lin AJ, Xiang Y, Cooper DE, Zhang J, et al. Neutrophils promote tumor resistance to radiation therapy. Proc Natl Acad Sci USA (2019) 116(37):18584–9. doi: 10.1073/pnas.1901562116
185. Boivin G, Ancey PB, Vuillefroy de Silly R, Kalambaden P, Contat C, Petit B, et al. Anti-Ly6G binding and trafficking mediate positive neutrophil selection to unleash the anti-tumor efficacy of radiation therapy. Oncoimmunology (2021) 10(1):1876597. doi: 10.1080/2162402X.2021.1876597
186. Roberts F, Zhu D, Farquharson C, Macrae VE. ENPP1 in the regulation of mineralization and beyond. Trends Biochem Sci (2019) 44(7):616–28. doi: 10.1016/j.tibs.2019.01.010
187. Stefan C, Jansen S, Bollen M. Modulation of purinergic signaling by NPP-type ectophosphodiesterases. Purinergic Signal (2006) 2(2):361–70. doi: 10.1007/s11302-005-5303-4
Keywords: neutrophil extracellular trap, tumor-associated neutrophils, tumor microenvironment, metastatic, therapy resistance
Citation: Zhao J and Jin J (2022) Neutrophil extracellular traps: New players in cancer research. Front. Immunol. 13:937565. doi: 10.3389/fimmu.2022.937565
Received: 06 May 2022; Accepted: 02 August 2022;
Published: 19 August 2022.
Edited by:
Michal Amit Rahat, Technion-Israel Institute of Technology, IsraelReviewed by:
Amblessed Onuma, The Ohio State University, United StatesCopyright © 2022 Zhao and Jin. This is an open-access article distributed under the terms of the Creative Commons Attribution License (CC BY). The use, distribution or reproduction in other forums is permitted, provided the original author(s) and the copyright owner(s) are credited and that the original publication in this journal is cited, in accordance with accepted academic practice. No use, distribution or reproduction is permitted which does not comply with these terms.
*Correspondence: Junjie Zhao, empqODcwNjMwMDcxQDE2My5jb20=; Jiaqi Jin, MTg4NDU4OTM2NDBAMTYzLmNvbQ==
Disclaimer: All claims expressed in this article are solely those of the authors and do not necessarily represent those of their affiliated organizations, or those of the publisher, the editors and the reviewers. Any product that may be evaluated in this article or claim that may be made by its manufacturer is not guaranteed or endorsed by the publisher.
Research integrity at Frontiers
Learn more about the work of our research integrity team to safeguard the quality of each article we publish.