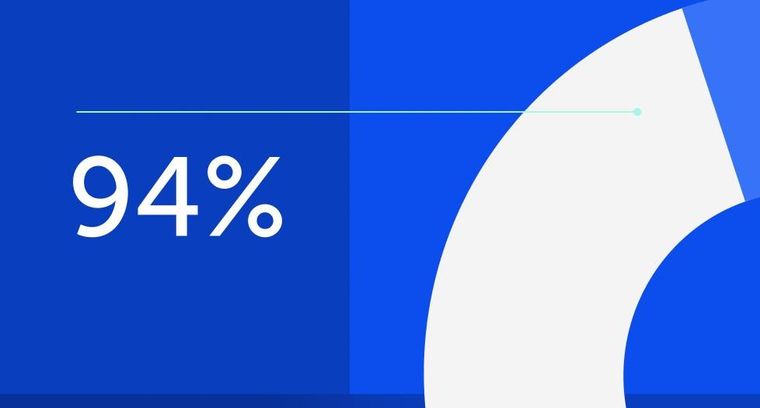
94% of researchers rate our articles as excellent or good
Learn more about the work of our research integrity team to safeguard the quality of each article we publish.
Find out more
REVIEW article
Front. Immunol., 14 September 2022
Sec. Inflammation
Volume 13 - 2022 | https://doi.org/10.3389/fimmu.2022.937120
This article is part of the Research TopicNovel Insights into Inflammatory Roles of Mast Cells and BasophilsView all 31 articles
Mast cells (MCs) are immune cells widely distributed in the body, accompanied by diverse phenotypes and functions. Committed mast cell precursors (MCPs) leave the bone marrow and enter the blood circulation, homing to peripheral sites under the control of various molecules from different microenvironments, where they eventually differentiate and mature. Partly attributable to the unique maturation mechanism, MCs display high functional heterogeneity and potentially plastic phenotypes. High plasticity also means that MCs can exhibit different subtypes to cope with different microenvironments, which we call “the peripheral immune education system”. Under the peripheral immune education system, MCs showed a new character from previous cognition in some cases, namely regulation of allergy and inflammation. In this review, we focus on the mucosal tissues, such as the gastrointestinal tract, to gain insights into the mechanism underlying the migration of MCs to the gut or other organs and their heterogeneity, which is driven by different microenvironments. In particular, the immunosuppressive properties of MCs let us consider that positively utilizing MCs may be a new way to overcome inflammatory and allergic disorders.
MCs predate the emergence of acquired immunity, since they were first named in 1878, a broad understanding of MCs biology has been published (1). Recently, an investigation of the hematopoietic origin of connective tissue MCs using fate-mapping systems has revealed the dual developmental origin of MCs (2). It was found that most connective tissue MCs (CTMCs) are derived from late erythro-myeloid progenitors (EMPs) produced in the yolk sac at E8.5 (the second transient definitive wave of fetal hematopoiesis), while mucosal MCs (MMCs) seem to come from hematopoietic stem cells (HSCs) in the bone marrow in mice (3). Committed progenitor cells enter the bloodstream, where they are found as lineage-negative Lin− c-Kit (CD117)hi FcϵRI+ β7-integrinhi ST2+ CD16/32hi or FcϵRI− cells (4). In human beings, Lin− c-Kitint/hi FcϵRI+ β7-integrin+ CD34hi blood cells are considered the immediate precursor of MCs (5). These MCPs travel in the blood circulation and eventually arrive at the peripheral tissues [e.g., skin, respiratory tract, urogenital tract, gastrointestinal tract, etc. (1)] under the stimulation and induction of various factors such as chemokine receptors and adhesion molecules. For example, in the context of microbiota-influence, MCs migrate to the intestinal tract and mature under the regulation of various pathways [e.g., α4β7-MAdCAM-1/VCAM-1 (6, 7), CXC chemokine receptor 2 (CXCR2) (7) sphingosine 1-phosphate (S1P) (8)].
In the process of migration and maturation, MCs of different origins showed distinct preferences in tissue localization (6). For instance, only the adipose and pleural cavity MCs were derived from early EMPs, most of which were replaced by late EMPs during adulthood in mice (3). Late EMPs generate most of the MCs that home to connective tissues, while mucosal MCs mainly derive from fetal hematopoietic stem cells HSCs (3). Diverse tissue preferences also shape remarkable phenotypical and functional heterogeneity, suggesting that MCs may perform various functions in response to different physiological and pathological states (9).
Besides the developmental origin, differences in the tissue microenvironment are also important causes of MC heterogeneity (10). It is clear that MCs in different organs have noticeable differences (11). However, even in the same organ, the microenvironment under different pathological or physiological conditions can produce utterly distinct MC subtypes. Take some examples, in the lung, only MCs of the proximal lung express MRGPRX2, but not of the distal or medial lung (12); in the gut, both CTMCs and MMCs are present and can be transformed into each other in some cases, such as food allergy (13). All this evidence supports the remarkable microenvironment-dependent heterogeneity in MCs.
Different cellular phenotypes bring different functions—one of the representative features of the heterogeneity of MC is regulatory-like properties against inflammation and allergy. In some occasions, MCs have been shown to release cytokines to inhibit and terminate inflammatory and allergic responses (e.g., IL-2 (14) IL-10 (14–16), and TGF-β1 (17), etc.). In addition, MCs interact with regulatory T cells (Tregs) (14, 18–21) and alternatively activated macrophages (AAMΦs) (22) to inhibit inflammatory and allergic responses.
In addition, various MC-derived cytokines [e.g., IL-4, IL-8, IL-13, IL-22, TNF-α, TGF-β1, vascular endothelial growth factor (VEGF), nerve growth factor (NGF), fibroblast growth factor-2 (FGF-2), and platelet-derived growth factor (PDGF)], proteases (tryptase and chymase/chymotrypsin), histamines, lipid mediators [PGD2 and leukotrienes (LTs), etc.] participate in the process of wound healing [e.g., vascular permeability and immune cells recruitment (monocytes and neutrophils), epithelial proliferation and migration, granular formation and remodeling, and scar formation, etc.] (11, 23).
Collectively, MCs play a diverse role in various physiological and pathological processes due to their highly complex heterogeneity. A full understanding of the phenotypic characteristics and functional heterogeneity of MCs in specific diseases will help us create efficient individualized therapy.
MCs exists in virtually all organs of vertebrates but different widely in their number, phenotype, and function (1). The high heterogeneity is partly attributed to their unique and complex maturation process. Although controversial (11), MCs are believed to have two main origins, bone marrow and yolk sac (2). After leaving the hematopoietic tissue, committed progenitor cells enter the blood, migrate, and colonize the target tissue [e.g., skin, respiratory tract, genitourinary tract, gastrointestinal tract, etc. (1)]. Notably, the exact ontogeny of mice adult MCs has been suggested in recent studies; early EMPs, late EMPs, and fetal HSCs are successively involved in the maturation of fetal MCs (2, 3). Using the inducible runt-related transcription factor1 (Runx1-icre) and colony stimulatory factor 1 receptor (Csf1r-icre) fate-mapping systems, Zhiqing Li et al. separately traced three waves of hematopoiesis (3). They confirmed that late EMP-derived MCs, as long-lived cells, gradually replace early EMPs and become the main contributors to the adult CTMC pool. Early EMP-derived MCs show a short lifespan in connective tissue, only appearing in adipose tissue and the pleural cavity. Fetal HSC-derived MCs, another possible type of short-lived cells, are mainly located in mucosal tissues and constantly renewed by the bone marrow (3). Therefore, there are at least two ways that MCs mature in mice. However, whether these pathways apply to other mammals, including humans, is not fully understood.
After arriving in the peripheral tissues, MCPs finally differentiate into mature MCs with the stimulation of local cytokines and growth factors in humans (e.g., IL-4 (24), IL-9 (25), IL-33 (26, 27), and TGF-β1 (28), etc.) and mice (e.g., IL-3 (29), IL-4 (28), TGF-β1 (28), and NGF (30), etc.). However, unlike other granulocytic leukocytes (e.g., neutrophils, eosinophils, and basophils), MCs mature in peripheral sites rather than bone marrow. Consequently, there are very few MCPs or mature MCs in bone marrow and blood, so it is relatively difficult to evaluate human MCs. Moreover, the molecular expression necessary for MC migration varies not only during maturation, but also according to cellular charcteristics, such CMMCs or MMCs (31, 32). All of these make it relatively difficult to study human MCs in vitro. So far, MCs are studied mainly in rodents (e.g., genetically modified mice) or some malignant MCs cell lines (33, 34). We herein describe some mechanisms by which MCs migrate to the intestinal tract and mature in combination with current findings (Figure 1).
Figure 1 Assembly of MCs in the gut. The MCPs leave the hematopoietic tissue, enter the blood stream, migrate and colonize the target tissue. With c-kit, CXCR2, and integrin α4β7 on the cell surface, MCPs bind to MAdCAM-1 and VCAM1 on the vascular endothelial cells, thereby crossing the vascular endothelium and colonizing the intestinal mucosa and submucosa, where they mature. In addition, transcription factor (dendritic cell-derived T-bet), Lipid mediator (sphingosine 1-phosphate), and some intestinal microbiota affect the homing of MCs to the gut.
The intestinal mucosa, which is exposed to various foreign components (including microbiota and food components), is one of the primary destinations for MCs and the pool for MCPs (35). MCs have been reported to account for approximately 2% of the cells in the lamina propria of the gastrointestinal tract (36). There is no doubt that integrin α4β7 plays a critical role in the migration of MCs to the intestinal mucosa. MCPs is utterly absent in the small intestine of α4β7-deficient mice (6). Evidence suggests that integrin α4β7 interacts with vascular endothelial molecules, including mucosal addressing protein cell adhesion molecule-1 (MAdCAM-1) and vascular cell adhesion molecule-1 (VCAM1), to facilitate directional migration of MCPs across endothelial cells to the small intestine (6, 7).
Like all blood-borne leukocytes, adhesion interactions are not the only requirement for MCPs recruitment to peripheral tissues, but also the migration to target tissues. Stem cell factor (SCF) has chemotactic effects on both mouse (37) and human (38) MCs in vitro. In vivo, SCF activates the c-kit expressed by MCs (or MCPs) through PI3K, then mediates migration, survival, proliferation, and activation with the help of α4 integrin (39–42). No intestinal MCPs was detected in SCF-deficient mice (6).
CXCR2 also contributes to the migration of MCs. It was experimentally demonstrated that MCs lacking CXCR2 are less able to migrate to the small intestine (7). Although the research of MC migration by CXCR2 is not detailed, the role of CXCR2 has been extensively studied in other cells. For example, in rat cardiogenic endothelial cells, the binding of CXCR2 and its ligand CXCL5 leads to downstream PI3K activation and further cytokine production (43). Another study in which human CXCR2 was stably transfected into lymphoblastic JY cell lines showed that the activation of IL-8 (CXCR2 ligand) induced transient adhesion of α4β7 dependent on VCAM-1 (44). Consequently, it is reasonable to speculate that in the circulatory system, c-kit and CXCR2 expressed by MCPs can interact with their respective ligands to upregulate the affinity of α4β7 integrin with MAdCAM-1 and VCAM-1, and also enhance the expression of VCAM-1 on epithelial cells via PI3K pathway (45), which further promotes the migration of MCs (7).
Previous studies have indicated that gut microbiota can mediate the constitutive migration of MCs into the intestine (46). Germ-free (GF) mice showed a lower intestinal MC density and higher blood MCs density compared to SPF (specific pathogen free) mice, followed by an impaired intestinal MCs function and maturity (reduced edema was observed after injecting the degranulation-provoking compound 48/80) (47). In detail, the expression of CXCR2 ligands, e.g., CXCL2, CXCL2, and CXCL5, were significantly reduced in the intestinal epithelial cells of GF mice and MyD88-/- mice compared to the control group (46). Results revealed that the expression of CXCR2 ligands in intestinal epithelial cells induced by intestinal microbiota was at least partially dependent on the Toll-like receptor (TLR) -MyD88 pathway, which promotes MC migration to the intestine. It remains to be seen whether intestinal bacteria directly or indirectly affect MC recruitment via their components and metabolites.
Interestingly, intestinal migration of MCs appears to be induced by a combination of gut bacteria, rather than a single bacterial strain. A study based on the Gram-positive Lactobacillus plantarum provided partial evidence that a single strain did not seem to mediate MC migration (47). Martin Schwarzer et al. found GF mice showed lower levels of CXCL1 and CXCL2 in the jejunal with fewer and less mature intestinal MCs in comparison to conventional mice after the induction of food allergy. In detail, the susceptibility to allergy symptoms in GF mice was fully restored after they were cohoused with conventional mice. However, similar results were not observed in mice mono-colonized with L. plantarum (47). L. plantarum was previously shown to aggravate allergic reactions associated with degranulation of MCs (48). Thus, it remains to be determined whether other single strains (e.g., Gram-negative bacteria) or specific bacterial products affect MC migration and maturation.
In addition, T-bet, a Th1-specific T-box transcription factor expressed by leukocytes [e.g., natural killer (NK) cells, dendritic cells (DCs), and CD8+ T cells], seems to mediate the migration of MCPs to the intestinal tract indirectly. Pilar Alcaide et al. found that T-bet-/- mice showed lower number of MCPs homing to the small intestine or lungs (49). It is worth mentioning that T-bet expressing DCs somehow contribute to the migration of MCs since MC itself does not seem to express T-bet during development (49).
Moreover, our previous studies showed that S1P mediates the migration of MCs to the large intestine (8). S1P is a sphingolipid metabolite from platelets and MCs, which is thought to play a role in mediating the migration of lymphocytes from secondary lymphoid organs and the thymus (50). It has been know that MCs express various types of S1P receptors, including type I S1P receptor (S1P1) (8), associated with Gi protein and small GTPases (e.g., Rho and Rac) (51, 52). By administration of FTY720 to mice, a blocker of S1P1-mediated signaling, we found that FTY720 can directly inhibit the migration of MCs, demonstrating the requirement of S1P-S1P1 signaling for MC migration (8).
The unique maturation mechanism of MCs also contributes to their astonishing plasticity and heterogeneity, which fully reflects the complex interaction between MCs and microenvironmental signals transmitted by different tissues (10). Traditionally, MCs are classified according to the production of serine proteases, such as trypsin and chymase (11, 53). In mice, MCs are divided into MMCs and CTMCs (11, 53). MMCs, as the name implies, mainly exist in the mucosa (e.g., the intestinal mucosa) and express chymase mMCP-1 and mMCP-2. MMCs are the dominant phenotype in the mucous layer, such as the gut (53). CTMCs can be found in connective tissue (e.g., intestinal submucosa, peritoneum, and skin) (1, 11, 54). CTMCs express chymase mMCP-4, trypsin (mMCP-6 and mMCP7), elastase (mMCP-5), and carboxypeptidase A3, etc. (1, 11, 54). Besides, the cytoplasm of CTMCs contains higher concentrations of heparin proteoglycan, histamine, and prostaglandin D2, while MMCs granules contain almost no heparin proteoglycan and lower levels of histamine (53, 55). Aside from the differences between the anatomic location and protease composition, MMCs and CTMCs vary in the requirement of T cells-derived cytokines, IL-3, and IL-4 (56) (Figure 2). Similar to mouse MCs, human MCs can be divided into two types: one subtype secretes both tryptase, chymase and carboxypeptidase A3 (MCTC); the other secretes tryptase alone (MCT) (1). The former is similar to mouse CTMCs, while the latter is equivalent to MMCs. MCTC is mainly distributed in the skin and small intestinal submucosa, and participates in pathological processes, such as urticaria (57). MCT is mostly expressed in the lung and small intestinal mucosa and is related to asthma and food allergy (1). In the intestine, it is reported that MCT accounts for approximately 98% of the total MC population in the mucous layer but only 13% of the total MC population in the intestinal submucosa (58), while MCTC accounts for approximately 77% of the MC population in the submucosa (59). Another phenotype, MCC, has also been mentioned in the intestinal mucosa (60). MCC seems rich in chymase but deficient in tryptase (60). MCC accounts for about 7% of the MCs in the intestinal mucosa and 17% in the intestinal submucosa (60); however, MCC does not seem to be a unique population, given the small number of reports.
Figure 2 Heterogeneity of MCs. MCs mature uniquely, developing them more susceptible to the tissue homeostasis of the peripheral tissue microenvironment, and thus exhibit different subtypes and secrete different mediators to achieve different functions.
With the progression of research, it is currently considered that the existing MC classification system cannot distinguish the highly microenvironment-dependent MC heterogeneity (10, 54). For example, it has been revealed that CTMCs can express different protease phenotypes in different microenvironments within tissue locations in mice. CTMCs in trachea are mMCP-1+, -2+, -4 to 7+, and CPA3+, while those in skin and gut are only mMCP-4 to 7+, and CPA3+ (61). The ImmGen-project-derived microarray data also indicated that CTMCs subpopulations present more or less tissue-specific genetic programming differences in distinct anatomical locations, such as the skin, tongue, esophagus, trachea, and peritoneal cavity in mice (9). It has been reported that only 110 genes differentially expressed in tongue relative to trachea MCs and 122 in tongue relative to esophagus MCs. However, the difference in gene expression transcripts in peritoneal MCs relative tongue and skin MCs was 612 and 957, respectively (9). As a result, there is a growing call for a new classification method.
The plasticity of MCs in different environments has been well described. The most obvious evidence is that human MCs cultured from progenitor cells that obtained from different sites (e.g., peripheral blood, bone marrow, and cord blood) show significantly different in the expressions of receptors and granular components, and also differ in the response to the cytokines (33, 62, 63). In addition, according to the culture conditions by incubation with IL-1β, IL-4, IL-6, TGF-β1, and lipopolysaccharide in SCF-containing medium, human MCs change the protease expressions from trypsin to chymase (64, 65). In mouse MCs, co-culture with fibroblasts and stimulation with SCF induces the ability to synthesize and store heparin (66). Our previous study found that MCs co-cultured with intestinal-derived fibroblasts produce more heparin and MCPT1 and MCPT2 (10). Skin-derived fibroblasts, on the other hand, had quite different results (i.e., the increased expression of chondroitin sulfate and MCPT4 in MCs) (10, 67). According to our previous studies, there are significant differences between MCs in different organs or tissues. For example, intestinal MCs expressed high levels of the extracellular ATP receptor P2X7, which mediates the activation of MCs and worsens intestinal inflammation. In contrast, skin MCs expressed lower levels of P2X7; importantly, once the expression of P2X7 is highly induced in skin MCs, chronic inflammation occurs (10, 68). With engaging, different tissue microenvironments can produce distinct MC subtypes, which seem to be required to maintain tissue homeostasis (11). Thus, it is worth mentioning that these series of terminal maturation of MCs may receive the effect of a “peripheral immune education system” dependent on the tissue microenvironment.
The gut serves as a vast repository of agranular Lin− c-Kit+ FcϵRIα− α4β7+ IL-33Rα+ FcγRIII/II+ MCPs (69, 70). The gut has almost 10 times more MCPs than the bone narrow in mice (71). With the constantly-changing microenvironment, the intestinal tract is likely to create a range of diverse MC phenotypes. For example, MCs differentiate in different pathological environments and obtain a more tissue- and disease- specific phenotype relative to health conditions, which shows their extreme plasticity. Nematodes, as one of the main drivers of intestinal MCs, not only mediate the migration and expansion of MCs (71), but also induce the change of MC phenotypes. Several studies based on the Trichinella spiralis helminth infection model have revealed that MCs have different phenotypes at different stages of the immune response. During nematode infection, MCs in the jejunum move from the submucosa to the epithelium and sequentially expressed mMCP-2, transiently expressed mMCP-9, stopped expressing mMCP-5 to -7, and finally expressed mMCP-1 (72–74). However, in the convalescent stage of infection, MCs returned to their original site and stopped expressing mMCP-1, mMCP-2, and mMCP-5 successively, accompanied by different expression combinations of mMCP-6, -7, and -9 (72–74). The parasite repelling effect of mMCP-1 has been proven (75). In conclusion, the plasticity of MCs has been fully demonstrated by their ability to migrate at different depths in intestinal tissue for the important host defense system.
In addition to parasitic infections, increased MCs in the intestinal mucosa have also been detected in IBD (15, 76), IBS (77, 78), and food allergy (13, 79, 80). In food allergy, inoculation of food antigens leads to a dramatic increase in MMCs (due partly to infiltrating MCPs and CTMCs). Induced by TGF-β1 (81) and the Notch signaling (13) in the inflamed intestine, precursor cells mature and even undergo a reciprocal transformation of CTMCs in the submucosa and/or MMCs in the mucosa, further demonstrating the environment-dependent heterogeneity of MCs (82). Additionally, Chen et al. identified a specific group of MMCs in food allergy named MMC9 (80). MMC9 cells are a group of MMCs of Lin− c-Kit+ FcϵRIα+ β7-Integrinlow ST2+, which can secrete large amounts of IL-9 and IL-13. This population of cells develops from MCPs that migrate to the intestinal mucosa with the help of IL-4. Under the action of IL-3 and SCF, MMC9 has the potential to rapidly develops into granular MCs and drive IgE-mediated food allergy (80, 83).
Crosstalk between MCs and surrounding cells has been widely discussed, which significantly increased the complexity of the terminal differentiation of MCs. For example, studies showed intestinal neurons could be activated by the increased histamine and trypsin secreted from MCs enriched in IBS (77) and IBD gut (76). Correspondingly, intestinal neurons mediated MCs activation by secreting neuronal factors (e.g., substance P, somatostatin, ATP, neuropeptide corticotropin-releasing factor, etc.) that stimulate MC surface receptors (e.g., MRGPRX2, P2X7, etc.) (78). The activation of MCs and their pro-inflammatory and pro-allergic mechanisms were described in more detail elsewhere (11). In addition to intestinal neurons, MCs also crosstalk with other cell types such as Tregs and intestinal epithelial cells to show the identity transformation from pathogenic or allergenic to regulatory, which plays a pivotal role in the fight against allergic responses (14) and inflammation (84). The transformation of MCs from pathogenic to regulatory roles will be discussed in more detail in the next section.
Notably, with the continuous development of technology, more comprehensive methods have been used to detect the phenotypes of different MC populations, such as single-cell RNA sequencing (scRNA-seq). In mice, a study on hematopoietic stem and progenitor cells (HSPCs) in mouse bone marrow showed that E-cadherin could be expressed by early precursors of basophils and MCs, representing the commitment to the lineages (85). Notably, scRNA-seq based on the peritoneal cavity of mice may establish an entirely new developmental trajectory for MCs, given that a discrete group of cells called P1 was found under suitable in vitro culture conditions, which have the potential to differentiate into MCs and basophils (86). In human, a study based on scRNA-seq revealed MCs are existed in the human yolk sac. In these human yolk sac-derived MCs, hairy and enhancer of split 1 (HES1), nuclear receptor subfamily 4 group A member 2 (NR4A2), and Kruppel-like factor 1 (KLF1) were detected, which may have non-redundant effects on the differentiation and activation of MCs (87). Another origin of human MCs was also confirmed by scRNA-seq. The analysis of human cord blood identified a group of basophil/eosinophil/MCs progenitors, revealing a close link between MCs and erythroid commitment cells downstream from HSCs (88). In addition, an analysis of nasal polyps in the patients of chronic rhinosinusitis with nasal polyposis detected an intermediate CD38highCD117high MC phenotype distinct from circulating MCPs (12). The same study also confirmed that MRGPRX2 was expressed only in MCs of the proximal lung, but not in the distal or medial lung (12). A high ratio of tumor-suppressive TNF+/vascular endothelial growth factor A (VEGFA)+ MC phenotype is found in nasopharyngeal cancer in the patients. It seems associated with a better prognosis (89), while another ground glass nodule adenocarcinoma analysis detected pro-inflammatory MCs (90). These results clearly indicated the origin- and microenvironment-dependent heterogeneity of MCs.
Deeper analysis of innovative techniques provide sufficient resolution to fully reveal the heterogeneity of MCs and show the differentiation trajectory and transcriptional heterogeneity of specific cell types in different tissues or disease settings, which helps identify novel putative immune cell subtypes (91).
In pathological reactions, such as allergy and inflammation, MCs and their precursors can recruit and expand in the disease site. For example, Lin− c-Kitint/hi FcϵRI+ CD34hi MCPs are recruited to peripheral tissues mediated by molecules [e.g., α4β7 integrins, VCAM-1 (92, 93)] and cells [e.g., CD11c+ cells (94), CD4+ cells (95)] in acute allergy. IgE (96) and its immune complexes (97) seem to be involved in the survival of MCs and the recruitment of MCPs. Consequently, MCs are closely involved in most allergy and inflammation processes.
Despite having outstanding achievements in innate and adaptive immunity, MCs have a well-deserved reputation as promoters of pathological actions, such as inflammation and allergy. However, as discussed previously, MCs’ distinctive migration and maturation patterns contribute to their remarkable plasticity and tissue-microenvironment-dependent heterogeneity. On this basis, we have reason to assume that MCs, as one of the most critical and primary immune cells in damaged tissues, may have positive regulatory functions in inflammation, allergy, tissue regeneration, and tissue repair. It is gratifying to note that there is now accumulating evidence to support the immunoregulatory part of MCs (Table 1).
In the early years, MCs were demonstrated to have immunosuppressive effects. For instance, Hart et al. found that in mice, dermal MCs play an indispensable role in inhibiting systemic contact hypersensitivity (CHS) response to ultraviolet radiation [wavelength 280-320 nm: (UVB)], and that histamine derived by MCs may be a critical factor for the suppression of inflammation (109). Both dermal MC-derived and exogenous histamines inhibited the immune response of UVB-exposed mice (109), through the production of PGE2. PGE2 inhibits the production of IL-12, an inducer of pathogenic Th1 immune responses (110).
In IBD, IL-33 is one of the earliest cytokines released from the necrotic cells of injured tissues (84). IL-33 has been shown to polarize AAMΦs in vivo, which requires the participation of MCs (22). AAMΦs have been demonstrated to have the potential to suppress inflammation and promote wound healing (111). Arginase-1 expressed by AAMΦs depletes the extracellular arginine necessary for T cell activation (112). Braune et al. and Fernando et al. found that IL-33 can induce IL-6 and IL-13 secretion by MCs through ST2, which plays a crucial role in AAMΦ polarization (111, 113). Consequently, IL-33 exerts immunosuppressive and anti-inflammatory effects by indirectly promoting AAMΦ polarization, thereby inhibiting the production of IL-17 and IFN-γ by T cells. Moreover, upon IL-33 stimulation, MCs secrete IL-10 and histamine to suppress LPS-mediated monocyte activation, which might be helpful against rheumatoid arthritis (114). Hence, it is plausible to believe that the IL-33/ST2-MC axis plays a positive role in limiting inflammation (115).
CD4+CD25+Foxp3+ Treg plays an essential role in immune tolerance. Several studies have shown intricate reciprocal crosstalk between MCs and Tregs. Experiments demonstrated that OX40 on the Treg surface could bind to OX40L expressed on the surface of MCs, subsequently inhibiting IgE-mediated MC degranulation (18). Moreover, TGF-β1 and IL-10 secreted by Tregs were thought to inhibit the expression of FcϵRI on the MC surface, which also inhibit the MC degranulation (17, 78, 116). Notably, TGF-β1 reduced the expression of FcϵRI subunit proteins α, β, and γ, while IL-10 inhibited only β proteins. TGF-β1 has also been reported to induce the apoptosis of MCs (108). Research on allotransplantation in experimental mice showed that Treg-derived IL-9 could maintain the tolerance of allografts by affecting MCs (19). IL-9 is a critical factor in promoting the SCF-dependent growth, proliferation, recruitment, and activation of MCs (25), thus Treg-derived IL-9 and SCF synergistically activate hepatic MCs and promote the release of histamine, IL-2, and TGF-β1 in tolerogenic liver allografts. TGF-β1 promoted the generation of γδT cells, and IL-17 released by γδT cells further attracted Tregs and enhanced their immunosuppressive properties (117).
MCs also have inverse impacts on Tregs. MCs secrete TGF-β1, which contribute to Tregs production and mediate autoantigen tolerance (20, 107). Epidermal growth factor (EGF)-like growth factor amphiregulin, which MCs also secrete, directly boosted the Treg function in colitis and tumor vaccination models by activating the EGF receptor (EGFR) on them (21). EGFR is reported to mediate intrinsic immunosuppression in tumors, and EGFR-targeted therapies are widely used for tumors such as colorectal cancer (118) and non-small cell lung cancer (119). Therefore, the immunoregulatory mechanism of MCs may be further applied in tumor therapy.
MCs also seem to ensure the sustained and stable expression of Foxp3 in Treg cells by secreting IL-2, thus maintaining the development, proliferation, activity, and survival of Tregs at sites (98) to sustain their inhibitory function (120). Studies have shown that MC-derived IL-2 in the lung promotes Tregs proliferation and limits allergic airway inflammation (115). In addition, the feasibility of regulating food allergy by inducing Treg cells with a continuous exposure of low concentration of IL-2 has been demonstrated (79).
Our recent research revealed the role of MCs in the regulation of food allergies (14). To delve into the mechanism of a more effective treatment for food allergy-oral allergen desensitization-oral immunotherapy (OIT) from a mucosal immune system perspective, we established a clinically relevant murine model of OIT with an escalating oral dose of ovalbumin (OVA). The OIT protocol induced mucosal desensitized MCs in the intestinal compartment with a low degranulation capacity and IL-4 production but high IFN-γ production, which plays an indispensable role in allergy (14). IL-4 plays a key role in the initiation of food allergy to mediate the isotype switching of IgE, the food-specific-IgE generation from B cells, and the release of MC mediators (e.g., histamine) (121, 122). Moreover, Tomar et al. found that IL-4 promotes the development of MMC9 cells, partly through a basic leucine zipper ATF-like transcription factor-dependent pathway (83). Returning to our study, we detected a significant increase in Tregs from the mucosa, peripheral blood, and spleen in OIT treated mice group. In addition, we discovered that the dual synthesis of IL-2 and IL-10 by mucosal desensitized MCs induces Tregs and inhibits allergic symptom (14). In addition, OIT treatment induce desensitization to MCs and reduced their allergenicity by acquiring the regulatory function (Figure 3). It can be expected that elucidation of switch pathway of MCs from allergenic to regulatory properties can lead to the utilization of MCs in a positive manner to overcome the allergy.
Figure 3 Regulatory properties of MCs in the immunotherapy. OIT treatment for food allergy induces desensitization of MCs with the low expression of Th2 cytokines and induces expression of IFN-γ. Desensitized MCs also synthesize IL-2 and IL-10. MC-derived IL-2 and amphiregulin promote proliferation and enhancement of the function of Tregs. Activated Tregs release IL-10 and TGF-β1, and the OX40 on their surface binds to OX40L from MCs, which inhibits the expression of FcϵRI on MCs and the release of TNF-α and IL-13, thereby inhibiting the Th2 responses.
IL-10 is a well-known anti-inflammatory cytokine that has been demonstrated to diminish the degree or duration of innate immune or acquired responses (123) by inhibiting the production of pro-inflammatory cytokines [TNF-α, IFN-γ, IL-1, and IL-6 (99)] and chemokines, and also the proliferation of T cells [e.g., CD4+ T cells (123) and CD8+ T cells (123, 124)]. It has been reported that MCs can reduce inflammation, tissue damage, leukocyte infiltration and restore the epithelial barrier function in an IL-10-dependent manner in multiple organs or tissues [e.g., gut (15) bladder (106) and skin (16)] and the antigen-specific T cell immune response caused by Anopheles mosquito saliva (125). MC deficiency can increase intestinal permeability in IL10-/- mice and cause spontaneous colitis (15). Artificial IL-10 supplementation prevented IFNγ-induced epithelial barrier dysfunction (105). In addition to the aforementioned Treg, MCs also secrete IL-10. Evidence of the secretion of IL-10 by MCs has been revealed both in vivo (14, 16) and in vitro (126). In Treg-independent graft-versus-host disease (GVHD), MCs have been found to show an immunosuppressive function by inhibiting T cell proliferation in an IL-10-dependent manner to inhibit inflammation (104). Although the mechanism through which MCs are triggered to produce IL-10 in the disease remains to be determined, it is speculated that endotoxin (LPS) from intestinal bacteria activates TLR4 in MCs, given that LPS has been shown to promote the production of Th2 cytokines by MCs (127). UVB promotes the production of MC-derived IL-10 by increasing the synthesis of vitamin D3 (128) to reduce allergic skin inflammation. Additionally, IL-10 derived from MCs, rather than Tregs, inhibits the migration of mature DCs to the lymph nodes in the bladder, which helps building the “immune privilege” (106).
In addition to their anti-inflammatory and allergic effects, MCs promote tissue repair and wound healing. Studies have shown that depletion of MCs partly inhibits wound healing (129). Furthermore, it has been revealed that IL-33/ST2 promoted the release of IL-13 and IL-22 from MCs (84). IL-13 signaling activates STAT6, which contributes to the survival and migration of epithelial cells and IL-22 can directly promote the production of mucus and the proliferation of epithelial cells (130, 131). Moreover, available evidence suggests that MCs are involved in almost all events of wound healing process (fibroblast migration/proliferation, and remodeling). In the early stage of wound formation, MCs are recruited to the injured site under the action of keratinocyte-derived IL-33, CCL2, and SCF; then they secrete various substances (23, 132). For example, MC produces TNF-α and stimulates DCs to express factor XIIIa, which promotes coagulation (23). Also, MC-derived histamine, lipid mediators [e.g., PGD2 and leukotrienes (LTs), etc.], and VEGF increase the vascular permeability to recruit monocytes and neutrophils (11, 23). Moreover, MCs are essential for the fibrotic process of wound healing. Proteases (e.g., tryptase and chymase), VEGF, IL-4, IL-8, NGF, FGF-2, PDGF, and TGF-β1, which are secreted by MCs, can contribute to angiogenesis, fibrin production, or re-epithelialization (23). At the end of wound healing, the proliferated blood vessels in the tissue degenerate, and the active granulation tissue is remodeled into fiber-rich scar tissue (133). Most studies agree that MCs are involved in this process (134), which might be mediated by gap-junctional intercellular communication between MCs and fibroblasts or myofibroblasts (135). In addition, MCs and zinc have been determined to induce the IL-6 production via Zn receptor GPR39 expressed on the cells in the inflammatory tissues such as fibroblasts, macrophages, and DCs (136). IL-6 is essential for wound healing (137).
In summary, although MCs show more inflammatory and allergic properties in the acute phase, they may exhibit more prominent functions during periods of inflammation or chronic inflammation subside to prevent excessive tissue damage and the development of chronic inflammation.
In general, MCs have high plasticity and tissue microenvironment-dependent heterogeneity owing to the distinctive migration and maturation modes. MCs interact with various cells at different physiological and pathological stages to exhibit vastly different functions. Comprehensively, understanding the heterogeneity of human MCs and determining their physiological functions under specific circumstances is a great challenge. Additionally, human MCs are difficult to obtain and isolate, and have poor ability to expand in vitro (63). Therefore, rodent MCs are widely used for the time being, which also gives rise to a new question: whether the results observed in these alternative models can be wholly inferred to human MCs. For example, human MCs and mouse MCs sometimes show different responses to the same cytokines or anti-allergic drugs (33, 138). Clinically, due to the profound negative impression that MCs promote inflammation and allergy, until recently, simply inhibiting MCs is still the mainstream way for clinical treatment of allergy and inflammation. Moreover, it is undeniable that MC neutralizing therapies do not seem to have satisfactory therapeutic effects due to the imperfect research at the present stage. According to this review, based on the strong microenvironment-dependent plasticity and heterogeneity of MCs, it appears promising to induce MCs to behave in a manner that regulates and inhibits chronic inflammatory and allergic responses. Accumulating research evidence might have revealed the great potential of MCs in treating disease by proper education in the peripheral tissues to terminate inflammation and allergy.
ZZ and YK conceived the idea for the review. ZZ and YK wrote the manuscript with constructive input from PE and HK. All authors read and approved the final version of the manuscript.
This work was supported by grants from The Ministry of Education, Culture, Sports, Science, and Technology (MEXT) for LEADER; Japan Agency for Medical Research and Development (AMED) PRIME (20gm6010012h0004/20gm6210024h0001) and Project Focused on Developing Key Technology for Discovering and Manufacturing Drugs for Next-Generation Treatment and Diagnosis, The next-generation drug discovery and development technology on regulating intestinal microbiome (NeDDTrim) (JP21ae0121040); Japan Society for the Promotion of Science (JSPS) for Grant-in-Aid for Scientific Research S (18H05280) and Scientific Research B (19H03450), Challenging Research (Exploratory) 21K19494], Funds for the Promotion of Joint International Research (18KK0432), Future Medicine Funds at Chiba University, Danone Institute of Japan Foundation, The Naito Foundation, Hoyu Science Foundation, Waksman foundation of Japan, Yamada Science Foundation, The Institute of Medical Science, University of Tokyo (IMSUT) International Joint Research Project K003, and the Chiba University-UC San Diego Center for Mucosal Immunology, Allergy, and Vaccines (cMAV).
We thank Mr. Izumi Tanaka for the discussion. Figures were produced using Servier Medical Art (http://smart.servier.com/), licensed under a Creative Common Attribution 3.0 Generic License (https://creativecommons.org/licenses/by/3.0/).
HK is director and founder of HanaVax Inc.
The remaining authors declare that the research was conducted in the absence of any commercial or financial relationships that could be construed as a potential conflict of interest.
All claims expressed in this article are solely those of the authors and do not necessarily represent those of their affiliated organizations, or those of the publisher, the editors and the reviewers. Any product that may be evaluated in this article, or claim that may be made by its manufacturer, is not guaranteed or endorsed by the publisher.
1. Galli SJ, Gaudenzio N, Tsai M. Mast cells in inflammation and disease: Recent progress and ongoing concerns. Annu Rev Immunol (2020) 38:49–77. doi: 10.1146/annurev-immunol-071719-094903
2. Gentek R, Ghigo C, Hoeffel G, Bulle MJ, Msallam R, Gautier G, et al. Hemogenic endothelial fate mapping reveals dual developmental origin of mast cells. Immunity (2018) 48(6):1160–71.e5. doi: 10.1016/j.immuni.2018.04.025
3. Li Z, Liu S, Xu J, Zhang X, Han D, Liu J, et al. Adult connective tissue-resident mast cells originate from late erythro-myeloid progenitors. Immunity (2018) 49(4):640–53.e5. doi: 10.1016/j.immuni.2018.09.023
4. Dahlin JS, Heyman B, Hallgren J. Committed mast cell progenitors in mouse blood differ in maturity between Th1 and Th2 strains. Allergy (2013) 68(10):1333–7. doi: 10.1111/all.12223
5. Dahlin JS, Malinovschi A, Ohrvik H, Sandelin M, Janson C, Alving K, et al. Lin- CD34hi CD117int/hi FcepsilonRI+ cells in human blood constitute a rare population of mast cell progenitors. Blood (2016) 127(4):383–91. doi: 10.1182/blood-2015-06-650648
6. Gurish MF, Tao H, Abonia JP, Arya A, Friend DS, Parker CM, et al. Intestinal mast cell progenitors require CD49dbeta7 (alpha4beta7 integrin) for tissue-specific homing. J Exp Med (2001) 194(9):1243–52. doi: 10.1084/jem.194.9.1243
7. Abonia JP, Austen KF, Rollins BJ, Joshi SK, Flavell RA, Kuziel WA, et al. Constitutive homing of mast cell progenitors to the intestine depends on autologous expression of the chemokine receptor CXCR2. Blood (2005) 105(11):4308–13. doi: 10.1182/blood-2004-09-3578
8. Kurashima Y, Kunisawa J, Higuchi M, Gohda M, Ishikawa I, Takayama N, et al. Sphingosine 1-phosphate-mediated trafficking of pathogenic Th2 and mast cells for the control of food allergy. J Immunol (2007) 179(3):1577–85. doi: 10.4049/jimmunol.179.3.1577
9. Dwyer DF, Barrett NA, Austen KF, Immunological Genome Project C. Expression profiling of constitutive mast cells reveals a unique identity within the immune system. Nat Immunol (2016) 17(7):878–87. doi: 10.1038/ni.3445
10. Kurashima Y, Amiya T, Fujisawa K, Shibata N, Suzuki Y, Kogure Y, et al. The enzyme Cyp26b1 mediates inhibition of mast cell activation by fibroblasts to maintain skin-barrier homeostasis. Immunity (2014) 40(4):530–41. doi: 10.1016/j.immuni.2014.01.014
11. Zhang Z, Kurashima Y. Two sides of the coin: Mast cells as a key regulator of allergy and Acute/Chronic inflammation. Cells (2021) 10(7):1615. doi: 10.3390/cells10071615
12. Dwyer DF, Ordovas-Montanes J, Allon SJ, Buchheit KM, Vukovic M, Derakhshan T, et al. Human airway mast cells proliferate and acquire distinct inflammation-driven phenotypes during type 2 inflammation. Sci Immunol (2021) 6(56):eabb7221. doi: 10.1126/sciimmunol.abb7221
13. Honjo A, Nakano N, Yamazaki S, Hara M, Uchida K, Kitaura J, et al. Pharmacologic inhibition of notch signaling suppresses food antigen-induced mucosal mast cell hyperplasia. J Allergy Clin Immunol (2017) 139(3):987–96.e10. doi: 10.1016/j.jaci.2016.05.046
14. Takasato Y, Kurashima Y, Kiuchi M, Hirahara K, Murasaki S, Arai F, et al. Orally desensitized mast cells form a regulatory network with treg cells for the control of food allergy. Mucosal Immunol (2021) 14(3):640–51. doi: 10.1038/s41385-020-00358-3
15. Chichlowski M, Westwood GS, Abraham SN, Hale LP. Role of mast cells in inflammatory bowel disease and inflammation-associated colorectal neoplasia in IL-10-deficient mice. PLoS One (2010) 5(8):e12220. doi: 10.1371/journal.pone.0012220
16. Grimbaldeston MA, Nakae S, Kalesnikoff J, Tsai M, Galli SJ. Mast cell-derived interleukin 10 limits skin pathology in contact dermatitis and chronic irradiation with ultraviolet b. Nat Immunol (2007) 8(10):1095–104. doi: 10.1038/ni1503
17. Gomez G, Ramirez CD, Rivera J, Patel M, Norozian F, Wright HV, et al. TGF-beta 1 inhibits mast cell fc epsilon RI expression. J Immunol (2005) 174(10):5987–93. doi: 10.4049/jimmunol.174.10.5987
18. Gri G, Piconese S, Frossi B, Manfroi V, Merluzzi S, Tripodo C, et al. CD4+CD25+ regulatory T cells suppress mast cell degranulation and allergic responses through OX40-OX40L interaction. Immunity (2008) 29(5):771–81. doi: 10.1016/j.immuni.2008.08.018
19. Lu LF, Lind EF, Gondek DC, Bennett KA, Gleeson MW, Pino-Lagos K, et al. Mast cells are essential intermediaries in regulatory T-cell tolerance. Nature (2006) 442(7106):997–1002. doi: 10.1038/nature05010
20. Belghith M, Bluestone JA, Barriot S, Megret J, Bach JF, Chatenoud L. TGF-beta-dependent mechanisms mediate restoration of self-tolerance induced by antibodies to CD3 in overt autoimmune diabetes. Nat Med (2003) 9(9):1202–8. doi: 10.1038/nm924
21. Zaiss DM, van Loosdregt J, Gorlani A, Bekker CP, Grone A, Sibilia M, et al. Amphiregulin enhances regulatory T cell-suppressive function via the epidermal growth factor receptor. Immunity (2013) 38(2):275–84. doi: 10.1016/j.immuni.2012.09.023
22. Finlay CM, Cunningham KT, Doyle B, Mills KHG. IL-33-Stimulated murine mast cells polarize alternatively activated macrophages, which suppress T cells that mediate experimental autoimmune encephalomyelitis. J Immunol (2020) 205(7):1909–19. doi: 10.4049/jimmunol.1901321
23. Komi DEA, Khomtchouk K, Santa Maria PL. A review of the contribution of mast cells in wound healing: Involved molecular and cellular mechanisms. Clin Rev Allergy Immunol (2020) 58(3):298–312. doi: 10.1007/s12016-019-08729-w
24. Toru H, Eguchi M, Matsumoto R, Yanagida M, Yata J, Nakahata T. Interleukin-4 promotes the development of tryptase and chymase double-positive human mast cells accompanied by cell maturation. Blood (1998) 91(1):187–95. doi: 10.1182/blood.v91.1.187.187_187_195
25. Matsuzawa S, Sakashita K, Kinoshita T, Ito S, Yamashita T, Koike K. IL-9 enhances the growth of human mast cell progenitors under stimulation with stem cell factor. J Immunol (2003) 170(7):3461–7. doi: 10.4049/jimmunol.170.7.3461
26. Iikura M, Suto H, Kajiwara N, Oboki K, Ohno T, Okayama Y, et al. IL-33 can promote survival, adhesion and cytokine production in human mast cells. Lab Invest (2007) 87(10):971–8. doi: 10.1038/labinvest.3700663
27. Allakhverdi Z, Smith DE, Comeau MR, Delespesse G. Cutting edge: The ST2 ligand IL-33 potently activates and drives maturation of human mast cells. J Immunol (2007) 179(4):2051–4. doi: 10.4049/jimmunol.179.4.2051
28. Macey MR, Sturgill JL, Morales JK, Falanga YT, Morales J, Norton SK, et al. IL-4 and TGF-beta 1 counterbalance one another while regulating mast cell homeostasis. J Immunol (2010) 184(9):4688–95. doi: 10.4049/jimmunol.0903477
29. Razin E, Ihle JN, Seldin D, Mencia-Huerta JM, Katz HR, LeBlanc PA, et al. Interleukin 3: A differentiation and growth factor for the mouse mast cell that contains chondroitin sulfate e proteoglycan. J Immunol (1984) 132(3):1479–86.
30. Matsuda H, Kannan Y, Ushio H, Kiso Y, Kanemoto T, Suzuki H, et al. Nerve growth factor induces development of connective tissue-type mast cells in vitro from murine bone marrow cells. J Exp Med (1991) 174(1):7–14. doi: 10.1084/jem.174.1.7
31. Weller CL, Collington SJ, Brown JK, Miller HR, Al-Kashi A, Clark P, et al. Leukotriene B4, an activation product of mast cells, is a chemoattractant for their progenitors. J Exp Med (2005) 201(12):1961–71. doi: 10.1084/jem.20042407
32. Ochi H, Hirani WM, Yuan Q, Friend DS, Austen KF, Boyce JA. T Helper cell type 2 cytokine-mediated comitogenic responses and CCR3 expression during differentiation of human mast cells in vitro. J Exp Med (1999) 190(2):267–80. doi: 10.1084/jem.190.2.267
33. Saito H, Kato A, Matsumoto K, Okayama Y. Culture of human mast cells from peripheral blood progenitors. Nat Protoc (2006) 1(4):2178–83. doi: 10.1038/nprot.2006.344
34. McNeil BD, Pundir P, Meeker S, Han L, Undem BJ, Kulka M, et al. Identification of a mast-cell-specific receptor crucial for pseudo-allergic drug reactions. Nature (2015) 519(7542):237–41. doi: 10.1038/nature14022
35. Gurish MF, Boyce JA. Mast cells: ontogeny, homing, and recruitment of a unique innate effector cell. J Allergy Clin Immunol (2006) 117(6):1285–91. doi: 10.1016/j.jaci.2006.04.017
36. Bischoff SC, Wedemeyer J, Herrmann A, Meier PN, Trautwein C, Cetin Y, et al. Quantitative assessment of intestinal eosinophils and mast cells in inflammatory bowel disease. Histopathology (1996) 28(1):1–13. doi: 10.1046/j.1365-2559.1996.262309.x
37. Meininger CJ, Yano H, Rottapel R, Bernstein A, Zsebo KM, Zetter BR. The c-kit receptor ligand functions as a mast cell chemoattractant. Blood (1992) 79(4):958–63. doi: 10.1182/blood.v79.4.958.958
38. Nilsson G, Butterfield JH, Nilsson K, Siegbahn A. Stem cell factor is a chemotactic factor for human mast cells. J Immunol (1994) 153(8):3717–23.
39. Lorentz A, Bischoff SC. Regulation of human intestinal mast cells by stem cell factor and IL-4. Immunol Rev (2001) 179:57–60. doi: 10.1034/j.1600-065x.2001.790106.x
40. Wang Z, Mascarenhas N, Eckmann L, Miyamoto Y, Sun X, Kawakami T, et al. Skin microbiome promotes mast cell maturation by triggering stem cell factor production in keratinocytes. J Allergy Clin Immunol (2017) 139(4):1205–16.e6. doi: 10.1016/j.jaci.2016.09.019
41. Huang B, Lei Z, Zhang GM, Li D, Song C, Li B, et al. SCF-mediated mast cell infiltration and activation exacerbate the inflammation and immunosuppression in tumor microenvironment. Blood (2008) 112(4):1269–79. doi: 10.1182/blood-2008-03-147033
42. Tan BL, Yazicioglu MN, Ingram D, McCarthy J, Borneo J, Williams DA, et al. Genetic evidence for convergence of c-kit- and alpha4 integrin-mediated signals on class IA PI-3kinase and the rac pathway in regulating integrin-directed migration in mast cells. Blood (2003) 101(12):4725–32. doi: 10.1182/blood-2002-08-2521
43. Chandrasekar B, Melby PC, Sarau HM, Raveendran M, Perla RP, Marelli-Berg FM, et al. (CXCL5) amplifies a proinflammatory cytokine response via a phosphatidylinositol 3-kinase-NF-kappa b pathway. J Biol Chem (2003) 278(7):4675–86. doi: 10.1074/jbc.M207006200
44. Sadhu C, Masinovsky B, Staunton DE. Differential regulation of chemoattractant-stimulated beta 2, beta 3, and beta 7 integrin activity. J Immunol (1998) 160(11):5622–8.
45. Hallgren J, Jones TG, Abonia JP, Xing W, Humbles A, Austen KF, et al. Pulmonary CXCR2 regulates VCAM-1 and antigen-induced recruitment of mast cell progenitors. Proc Natl Acad Sci U.S.A. (2007) 104(51):20478–83. doi: 10.1073/pnas.0709651104
46. Kunii J, Takahashi K, Kasakura K, Tsuda M, Nakano K, Hosono A, et al. Commensal bacteria promote migration of mast cells into the intestine. Immunobiology (2011) 216(6):692–7. doi: 10.1016/j.imbio.2010.10.007
47. Schwarzer M, Hermanova P, Srutkova D, Golias J, Hudcovic T, Zwicker C, et al. Germ-free mice exhibit mast cells with impaired functionality and gut homing and do not develop food allergy. Front Immunol (2019) 10:205. doi: 10.3389/fimmu.2019.00205
48. Meijerink M, Wells JM, Taverne N, de Zeeuw Brouwer ML, Hilhorst B, Venema K, et al. Immunomodulatory effects of potential probiotics in a mouse peanut sensitization model. FEMS Immunol Med Microbiol (2012) 65(3):488–96. doi: 10.1111/j.1574-695X.2012.00981.x
49. Alcaide P, Jones TG, Lord GM, Glimcher LH, Hallgren J, Arinobu Y, et al. Dendritic cell expression of the transcription factor T-bet regulates mast cell progenitor homing to mucosal tissue. J Exp Med (2007) 204(2):431–9. doi: 10.1084/jem.20060626
50. Cyster JG. Chemokines, sphingosine-1-phosphate, and cell migration in secondary lymphoid organs. Annu Rev Immunol (2005) 23:127–59. doi: 10.1146/annurev.immunol.23.021704.115628
51. Lee MJ, Evans M, Hla T. The inducible G protein-coupled receptor edg-1 signals via the g(i)/mitogen-activated protein kinase pathway. J Biol Chem (1996) 271(19):11272–9. doi: 10.1074/jbc.271.19.11272
52. Lee MJ, Thangada S, Claffey KP, Ancellin N, Liu CH, Kluk M, et al. Vascular endothelial cell adherens junction assembly and morphogenesis induced by sphingosine-1-phosphate. Cell (1999) 99(3):301–12. doi: 10.1016/s0092-8674(00)81661-x
53. Gurish MF, Austen KF. Developmental origin and functional specialization of mast cell subsets. Immunity (2012) 37(1):25–33. doi: 10.1016/j.immuni.2012.07.003
54. Frossi B, Mion F, Sibilano R, Danelli L, Pucillo CEM. Is it time for a new classification of mast cells? what do we know about mast cell heterogeneity? Immunol Rev (2018) 282(1):35–46. doi: 10.1111/imr.12636
55. Heavey DJ, Ernst PB, Stevens RL, Befus AD, Bienenstock J, Austen KF. Generation of leukotriene C4, leukotriene B4, and prostaglandin D2 by immunologically activated rat intestinal mucosa mast cells. J Immunol (1988) 140(6):1953–7.
56. Madden KB, Urban JF Jr., Ziltener HJ, Schrader JW, Finkelman FD, Katona IM. Antibodies to IL-3 and IL-4 suppress helminth-induced intestinal mastocytosis. J Immunol (1991) 147(4):1387–91.
57. Smith CH, Kepley C, Schwartz LB, Lee TH. Mast cell number and phenotype in chronic idiopathic urticaria. J Allergy Clin Immunol (1995) 96(3):360–4. doi: 10.1016/s0091-6749(95)70055-2
58. Irani AA, Schechter NM, Craig SS, DeBlois G, Schwartz LB. Two types of human mast cells that have distinct neutral protease compositions. Proc Natl Acad Sci U S A (1986) 83(12):4464–8. doi: 10.1073/pnas.83.12.4464
59. Irani AM, Bradford TR, Kepley CL, Schechter NM, Schwartz LB. Detection of MCT and MCTC types of human mast cells by immunohistochemistry using new monoclonal anti-tryptase and anti-chymase antibodies. J Histochem Cytochem (1989) 37(10):1509–15. doi: 10.1177/37.10.2674273
60. Weidner N, Austen KF. Heterogeneity of mast cells at multiple body sites. fluorescent determination of avidin binding and immunofluorescent determination of chymase, tryptase, and carboxypeptidase content. Pathol Res Pract (1993) 189(2):156–62. doi: 10.1016/S0344-0338(11)80086-5
61. Xing W, Austen KF, Gurish MF, Jones TG. Protease phenotype of constitutive connective tissue and of induced mucosal mast cells in mice is regulated by the tissue. Proc Natl Acad Sci U S A (2011) 108(34):14210–5. doi: 10.1073/pnas.1111048108
62. Schmetzer O, Valentin P, Smorodchenko A, Domenis R, Gri G, Siebenhaar F, et al. A novel method to generate and culture human mast cells: Peripheral CD34+ stem cell-derived mast cells (PSCMCs). J Immunol Methods (2014) 413:62–8. doi: 10.1016/j.jim.2014.07.003
63. Elst J, Sabato V, van der Poorten MM, Faber M, Van Gasse AL, De Puysseleyr LP, et al. Peripheral blood cultured mast cells: Phenotypic and functional outcomes of different culture protocols. J Immunol Methods (2021) 492:113003. doi: 10.1016/j.jim.2021.113003
64. Kirshenbaum AS, Swindle E, Kulka M, Wu Y, Metcalfe DD. Effect of lipopolysaccharide (LPS) and peptidoglycan (PGN) on human mast cell numbers, cytokine production, and protease composition. BMC Immunol (2008) 9:45. doi: 10.1186/1471-2172-9-45
65. Gebhardt T, Lorentz A, Detmer F, Trautwein C, Bektas H, Manns MP, et al. Growth, phenotype, and function of human intestinal mast cells are tightly regulated by transforming growth factor beta1. Gut (2005) 54(7):928–34. doi: 10.1136/gut.2004.054650
66. Levi-Schaffer F, Austen KF, Gravallese PM, Stevens RL. Coculture of interleukin 3-dependent mouse mast cells with fibroblasts results in a phenotypic change of the mast cells. Proc Natl Acad Sci U S A (1986) 83(17):6485–8. doi: 10.1073/pnas.83.17.6485
67. Yamada K, Sato H, Sakamaki K, Kamada M, Okuno Y, Fukuishi N, et al. Suppression of IgE-independent degranulation of murine connective tissue-type mast cells by dexamethasone. Cells (2019) 8(2):112. doi: 10.3390/cells8020112
68. Kurashima Y, Amiya T, Nochi T, Fujisawa K, Haraguchi T, Iba H, et al. Extracellular ATP mediates mast cell-dependent intestinal inflammation through P2X7 purinoceptors. Nat Commun (2012) 3:1034. doi: 10.1038/ncomms2023
69. Arinobu Y, Iwasaki H, Gurish MF, Mizuno S, Shigematsu H, Ozawa H, et al. Developmental checkpoints of the basophil/mast cell lineages in adult murine hematopoiesis. Proc Natl Acad Sci U S A (2005) 102(50):18105–10. doi: 10.1073/pnas.0509148102
70. Chen CC, Grimbaldeston MA, Tsai M, Weissman IL, Galli SJ. Identification of mast cell progenitors in adult mice. Proc Natl Acad Sci U S A (2005) 102(32):11408–13. doi: 10.1073/pnas.0504197102
71. Ernst PB, Petit A, Befus AD, Clark DA, Rosenthal KL, Ishizaka T, et al. Murine intestinal intraepithelial lymphocytes II. Comparison of freshly isolated and cultured intraepithelial lymphocytes. Eur J Immunol (1985) 15(3):216–21. doi: 10.1002/eji.1830150303
72. Friend DS, Ghildyal N, Austen KF, Gurish MF, Matsumoto R, Stevens RL. Mast cells that reside at different locations in the jejunum of mice infected with trichinella spiralis exhibit sequential changes in their granule ultrastructure and chymase phenotype. J Cell Biol (1996) 135(1):279–90. doi: 10.1083/jcb.135.1.279
73. Friend DS, Ghildyal N, Gurish MF, Hunt J, Hu X, Austen KF, et al. Reversible expression of tryptases and chymases in the jejunal mast cells of mice infected with trichinella spiralis. J Immunol (1998) 160(11):5537–45.
74. Shin K, Watts GF, Oettgen HC, Friend DS, Pemberton AD, Gurish MF, et al. Mouse mast cell tryptase mMCP-6 is a critical link between adaptive and innate immunity in the chronic phase of trichinella spiralis infection. J Immunol (2008) 180(7):4885–91. doi: 10.4049/jimmunol.180.7.4885
75. Knight PA, Wright SH, Lawrence CE, Paterson YY, Miller HR. Delayed expulsion of the nematode trichinella spiralis in mice lacking the mucosal mast cell-specific granule chymase, mouse mast cell protease-1. J Exp Med (2000) 192(12):1849–56. doi: 10.1084/jem.192.12.1849
76. Bischoff SC. Physiological and pathophysiological functions of intestinal mast cells. Semin Immunopathol (2009) 31(2):185–205. doi: 10.1007/s00281-009-0165-4
77. Bischoff SC. Mast cells in gastrointestinal disorders. Eur J Pharmacol (2016) 778:139–45. doi: 10.1016/j.ejphar.2016.02.018
78. Wouters MM, Vicario M, Santos J. The role of mast cells in functional GI disorders. Gut (2016) 65(1):155–68. doi: 10.1136/gutjnl-2015-309151
79. Bonnet B, Vigneron J, Levacher B, Vazquez T, Pitoiset F, Brimaud F, et al. Low-dose IL-2 induces regulatory T cell-mediated control of experimental food allergy. J Immunol (2016) 197(1):188–98. doi: 10.4049/jimmunol.1501271
80. Chen CY, Lee JB, Liu B, Ohta S, Wang PY, Kartashov AV, et al. Induction of interleukin-9-Producing mucosal mast cells promotes susceptibility to IgE-mediated experimental food allergy. Immunity (2015) 43(4):788–802. doi: 10.1016/j.immuni.2015.08.020
81. Wright SH, Brown J, Knight PA, Thornton EM, Kilshaw PJ, Miller HR. Transforming growth factor-beta1 mediates coexpression of the integrin subunit alphaE and the chymase mouse mast cell protease-1 during the early differentiation of bone marrow-derived mucosal mast cell homologues. Clin Exp Allergy (2002) 32(2):315–24. doi: 10.1046/j.1365-2222.2002.01233.x
82. Nakano N, Saida K, Hara M, Izawa K, Ando T, Kaitani A, et al. Mucosal mast cell-specific gene expression is promoted by interdependent action of notch and TGF-beta signaling. J Immunol (2021) 207(12):3098–106. doi: 10.4049/jimmunol.2100112
83. Tomar S, Ganesan V, Sharma A, Zeng C, Waggoner L, Smith A, et al. IL-4-BATF signaling directly modulates IL-9 producing mucosal mast cell (MMC9) function in experimental food allergy. J Allergy Clin Immunol (2021) 147(1):280–95. doi: 10.1016/j.jaci.2020.08.043
84. He Z, Song J, Hua J, Yang M, Ma Y, Yu T, et al. Mast cells are essential intermediaries in regulating IL-33/ST2 signaling for an immune network favorable to mucosal healing in experimentally inflamed colons. Cell Death Dis (2018) 9(12):1173. doi: 10.1038/s41419-018-1223-4
85. Wanet A, Bassal MA, Patel SB, Marchi F, Mariani SA, Ahmed N, et al. E-cadherin is regulated by GATA-2 and marks the early commitment of mouse hematopoietic progenitors to the basophil and mast cell fates. Sci Immunol (2021) 6(56):eaba0178. doi: 10.1126/sciimmunol.aba0178
86. Hamey FK, Lau WWY, Kucinski I, Wang X, Diamanti E, Wilson NK, et al. Single-cell molecular profiling provides a high-resolution map of basophil and mast cell development. Allergy (2021) 76(6):1731–42. doi: 10.1111/all.14633
87. Popescu DM, Botting RA, Stephenson E, Green K, Webb S, Jardine L, et al. Decoding human fetal liver haematopoiesis. Nature (2019) 574(7778):365–71. doi: 10.1038/s41586-019-1652-y
88. Zheng S, Papalexi E, Butler A, Stephenson W, Satija R. Molecular transitions in early progenitors during human cord blood hematopoiesis. Mol Syst Biol (2018) 14(3):e8041. doi: 10.15252/msb.20178041
89. Cheng S, Li Z, Gao R, Xing B, Gao Y, Yang Y, et al. A pan-cancer single-cell transcriptional atlas of tumor infiltrating myeloid cells. Cell (2021) 184(3):792–809.e23. doi: 10.1016/j.cell.2021.01.010
90. Lu T, Yang X, Shi Y, Zhao M, Bi G, Liang J, et al. Single-cell transcriptome atlas of lung adenocarcinoma featured with ground glass nodules. Cell Discovery (2020) 6:69. doi: 10.1038/s41421-020-00200-x
91. Watcham S, Kucinski I, Gottgens B. New insights into hematopoietic differentiation landscapes from single-cell RNA sequencing. Blood (2019) 133(13):1415–26. doi: 10.1182/blood-2018-08-835355
92. Abonia JP, Hallgren J, Jones T, Shi T, Xu Y, Koni P, et al. Alpha-4 integrins and VCAM-1, but not MAdCAM-1, are essential for recruitment of mast cell progenitors to the inflamed lung. Blood (2006) 108(5):1588–94. doi: 10.1182/blood-2005-12-012781
93. Mendez-Enriquez E, Alvarado-Vazquez PA, Abma W, Simonson OE, Rodin S, Feyerabend TB, et al. Mast cell-derived serotonin enhances methacholine-induced airway hyperresponsiveness in house dust mite-induced experimental asthma. Allergy (2021) 76(7):2057–69. doi: 10.1111/all.14748
94. Dahlin JS, Feinstein R, Cui Y, Heyman B, Hallgren J. CD11c+ cells are required for antigen-induced increase of mast cells in the lung. J Immunol (2012) 189(8):3869–77. doi: 10.4049/jimmunol.1201200
95. Jones TG, Hallgren J, Humbles A, Burwell T, Finkelman FD, Alcaide P, et al. Antigen-induced increases in pulmonary mast cell progenitor numbers depend on IL-9 and CD1d-restricted NKT cells. J Immunol (2009) 183(8):5251–60. doi: 10.4049/jimmunol.0901471
96. Mathias CB, Freyschmidt EJ, Caplan B, Jones T, Poddighe D, Xing W, et al. IgE influences the number and function of mature mast cells, but not progenitor recruitment in allergic pulmonary inflammation. J Immunol (2009) 182(4):2416–24. doi: 10.4049/jimmunol.0801569
97. Dahlin JS, Ivarsson MA, Heyman B, Hallgren J. IgE immune complexes stimulate an increase in lung mast cell progenitors in a mouse model of allergic airway inflammation. PLoS One (2011) 6(5):e20261. doi: 10.1371/journal.pone.0020261
98. Hershko AY, Suzuki R, Charles N, Alvarez-Errico D, Sargent JL, Laurence A, et al. Mast cell interleukin-2 production contributes to suppression of chronic allergic dermatitis. Immunity (2011) 35(4):562–71. doi: 10.1016/j.immuni.2011.07.013
99. Fiorentino DF, Zlotnik A, Vieira P, Mosmann TR, Howard M, Moore KW, et al. IL-10 acts on the antigen-presenting cell to inhibit cytokine production by Th1 cells. J Immunol (1991) 146(10):3444–51.
100. Mirmonsef P, Shelburne CP, Fitzhugh Yeatman C. 2nd, h. j. chong and j. j. Ryan: Inhibition of kit expression by IL-4 and IL-10 in murine mast cells: role of STAT6 and phosphatidylinositol 3'-kinase. J Immunol (1999) 163(5):2530–9.
101. Helmby H, Grencis RK. Contrasting roles for IL-10 in protective immunity to different life cycle stages of intestinal nematode parasites. Eur J Immunol (2003) 33(9):2382–90. doi: 10.1002/eji.200324082
102. Bouton LA, Ramirez CD, Bailey DP, Yeatman CF, Yue J, Wright HV, et al. Costimulation with interleukin-4 and interleukin-10 induces mast cell apoptosis and cell-cycle arrest: the role of p53 and the mitochondrion. Exp Hematol (2004) 32(12):1137–45. doi: 10.1016/j.exphem.2004.09.002
103. Bailey DP, Kashyap M, Bouton LA, Murray PJ, Ryan JJ. Interleukin-10 induces apoptosis in developing mast cells and macrophages. J Leukoc Biol (2006) 80(3):581–9. doi: 10.1189/jlb.0405201
104. Leveson-Gower DB, Sega EI, Kalesnikoff J, Florek M, Pan Y, Pierini A, et al. Mast cells suppress murine GVHD in a mechanism independent of CD4+CD25+ regulatory T cells. Blood (2013) 122(22):3659–65. doi: 10.1182/blood-2013-08-519157
105. Oshima T, Laroux FS, Coe LL, Morise Z, Kawachi S, Bauer P, et al. Interferon-gamma and interleukin-10 reciprocally regulate endothelial junction integrity and barrier function. Microvasc Res (2001) 61(1):130–43. doi: 10.1006/mvre.2000.2288
106. Chan CY, St John AL, Abraham SN. Mast cell interleukin-10 drives localized tolerance in chronic bladder infection. Immunity (2013) 38(2):349–59. doi: 10.1016/j.immuni.2012.10.019
107. Zhao YB, Yang SH, Shen J, Deng K, Li Q, Wang Y, et al. Interaction between regulatory T cells and mast cells via IL-9 and TGF-beta production. Oncol Lett (2020) 20(6):360. doi: 10.3892/ol.2020.12224
108. Norozian F, Kashyap M, Ramirez CD, Patel N, Kepley CL, Barnstein BO, et al. TGFbeta1 induces mast cell apoptosis. Exp Hematol (2006) 34(5):579–87. doi: 10.1016/j.exphem.2006.02.003
109. Hart PH, Grimbaldeston MA, Swift GJ, Jaksic A, Noonan FP, Finlay-Jones JJ. Dermal mast cells determine susceptibility to ultraviolet b-induced systemic suppression of contact hypersensitivity responses in mice. J Exp Med (1998) 187(12):2045–53. doi: 10.1084/jem.187.12.2045
110. van der Pouw Kraan TC, Boeije LC, Smeenk RJ, Wijdenes J, Aarden LA. Prostaglandin-E2 is a potent inhibitor of human interleukin 12 production. J Exp Med (1995) 181(2):775–9. doi: 10.1084/jem.181.2.775
111. Fernando MR, Reyes JL, Iannuzzi J, Leung G, McKay DM. The pro-inflammatory cytokine, interleukin-6, enhances the polarization of alternatively activated macrophages. PLoS One (2014) 9(4):e94188. doi: 10.1371/journal.pone.0094188
112. Pesce JT, Ramalingam TR, Mentink-Kane MM, Wilson MS, El Kasmi KC, Smith AM, et al. Arginase-1-expressing macrophages suppress Th2 cytokine-driven inflammation and fibrosis. PLoS Pathog (2009) 5(4):e1000371. doi: 10.1371/journal.ppat.1000371
113. Braune J, Weyer U, Hobusch C, Mauer J, Bruning JC, Bechmann I, et al. IL-6 regulates M2 polarization and local proliferation of adipose tissue macrophages in obesity. J Immunol (2017) 198(7):2927–34. doi: 10.4049/jimmunol.1600476
114. Rivellese F, Suurmond J, Habets K, Dorjee AL, Ramamoorthi N, Townsend MJ, et al. Ability of interleukin-33- and immune complex-triggered activation of human mast cells to down-regulate monocyte-mediated immune responses. Arthritis Rheumatol (2015) 67(9):2343–53. doi: 10.1002/art.39192
115. Morita H, Arae K, Unno H, Miyauchi K, Toyama S, Nambu A, et al. An interleukin-33-Mast cell-Interleukin-2 axis suppresses papain-induced allergic inflammation by promoting regulatory T cell numbers. Immunity (2015) 43(1):175–86. doi: 10.1016/j.immuni.2015.06.021
116. Gillespie SR, DeMartino RR, Zhu J, Chong HJ, Ramirez C, Shelburne CP, et al. IL-10 inhibits fc epsilon RI expression in mouse mast cells. J Immunol (2004) 172(5):3181–8. doi: 10.4049/jimmunol.172.5.3181
117. Nakano T, Lai CY, Goto S, Hsu LW, Kawamoto S, Ono K, et al. Immunological and regenerative aspects of hepatic mast cells in liver allograft rejection and tolerance. PloS One (2012) 7(5):e37202. doi: 10.1371/journal.pone.0037202
118. Cunningham D, Humblet Y, Siena S, Khayat D, Bleiberg H, Santoro A, et al. Cetuximab monotherapy and cetuximab plus irinotecan in irinotecan-refractory metastatic colorectal cancer. N Engl J Med (2004) 351(4):337–45. doi: 10.1056/NEJMoa033025
119. Bonner JA, Harari PM, Giralt J, Azarnia N, Shin DM, Cohen RB, et al. Radiotherapy plus cetuximab for squamous-cell carcinoma of the head and neck. N Engl J Med (2006) 354(6):567–78. doi: 10.1056/NEJMoa053422
120. Thornton AM, Donovan EE, Piccirillo CA, Shevach EM. Cutting edge: IL-2 is critically required for the in vitro activation of CD4+CD25+ T cell suppressor function. J Immunol (2004) 172(11):6519–23. doi: 10.4049/jimmunol.172.11.6519
121. Swain SL, Weinberg AD, English M, Huston G. IL-4 directs the development of Th2-like helper effectors. J Immunol (1990) 145(11):3796–806.
122. Bischoff SC, Sellge G, Lorentz A, Sebald W, Raab R, Manns MP. IL-4 enhances proliferation and mediator release in mature human mast cells. Proc Natl Acad Sci U S A (1999) 96(14):8080–5. doi: 10.1073/pnas.96.14.8080
123. Moore KW, de Waal Malefyt R, Coffman RL, O'Garra A. Interleukin-10 and the interleukin-10 receptor. Annu Rev Immunol (2001) 19:683–765. doi: 10.1146/annurev.immunol.19.1.683
124. Groux H, Bigler M, de Vries JE, Roncarolo MG. Inhibitory and stimulatory effects of IL-10 on human CD8+ T cells. J Immunol (1998) 160(7):3188–93.
125. Depinay N, Hacini F, Beghdadi W, Peronet R, Mecheri S. Mast cell-dependent down-regulation of antigen-specific immune responses by mosquito bites. J Immunol (2006) 176(7):4141–6. doi: 10.4049/jimmunol.176.7.4141
126. Galli SJ, Kalesnikoff J, Grimbaldeston MA, Piliponsky AM, Williams CM, Tsai M. Mast cells as "tunable" effector and immunoregulatory cells: recent advances. Annu Rev Immunol (2005) 23:749–86. doi: 10.1146/annurev.immunol.21.120601.141025
127. Masuda A, Yoshikai Y, Aiba K, Matsuguchi T. Th2 cytokine production from mast cells is directly induced by lipopolysaccharide and distinctly regulated by c-jun n-terminal kinase and p38 pathways. J Immunol (2002) 169(7):3801–10. doi: 10.4049/jimmunol.169.7.3801
128. Biggs L, Yu C, Fedoric B, Lopez AF, Galli SJ, Grimbaldeston MA. Evidence that vitamin D(3) promotes mast cell-dependent reduction of chronic UVB-induced skin pathology in mice. J Exp Med (2010) 207(3):455–63. doi: 10.1084/jem.20091725
129. Younan GJ, Heit YI, Dastouri P, Kekhia H, Xing W, Gurish MF, et al. Mast cells are required in the proliferation and remodeling phases of microdeformational wound therapy. Plast Reconstr Surg (2011) 128(6):649e–58e. doi: 10.1097/PRS.0b013e318230c55d
130. Brand S, Beigel F, Olszak T, Zitzmann K, Eichhorst ST, Otte JM, et al. IL-22 is increased in active crohn's disease and promotes proinflammatory gene expression and intestinal epithelial cell migration. Am J Physiol Gastrointest Liver Physiol (2006) 290(4):G827–38. doi: 10.1152/ajpgi.00513.2005
131. Sugimoto K, Ogawa A, Mizoguchi E, Shimomura Y, Andoh A, Bhan AK, et al. IL-22 ameliorates intestinal inflammation in a mouse model of ulcerative colitis. J Clin Invest (2008) 118(2):534–44. doi: 10.1172/JCI33194
132. Trautmann A, Toksoy A, Engelhardt E, Brocker EB, Gillitzer R. Mast cell involvement in normal human skin wound healing: expression of monocyte chemoattractant protein-1 is correlated with recruitment of mast cells which synthesize interleukin-4 in vivo. J Pathol (2000) 190(1):100–6. doi: 10.1002/(SICI)1096-9896(200001)190:1<100::AID-PATH496>3.0.CO;2-Q
133. Nishikori Y, Shiota N, Okunishi H. The role of mast cells in cutaneous wound healing in streptozotocin-induced diabetic mice. Arch Dermatol Res (2014) 306(9):823–35. doi: 10.1007/s00403-014-1496-0
134. Ud-Din S, Wilgus TA, Bayat A. Mast cells in skin scarring: A review of animal and human research. Front Immunol (2020) 11:552205. doi: 10.3389/fimmu.2020.552205
135. Moyer KE, Saggers GC, Ehrlich HP. Mast cells promote fibroblast populated collagen lattice contraction through gap junction intercellular communication. Wound Repair Regener (2004) 12(3):269–75. doi: 10.1111/j.1067-1927.2004.012310.x
136. Nishida K, Hasegawa A, Yamasaki S, Uchida R, Ohashi W, Kurashima Y, et al. Mast cells play role in wound healing through the ZnT2/GPR39/IL-6 axis. Sci Rep (2019) 9(1):10842. doi: 10.1038/s41598-019-47132-5
137. Lin ZQ, Kondo T, Ishida Y, Takayasu T, Mukaida N. Essential involvement of IL-6 in the skin wound-healing process as evidenced by delayed wound healing in IL-6-deficient mice. J Leukoc Biol (2003) 73(6):713–21. doi: 10.1189/jlb.0802397
Keywords: mast cells, heterogeneity, inflammation, allergy, tolerance
Citation: Zhang Z, Ernst PB, Kiyono H and Kurashima Y (2022) Utilizing mast cells in a positive manner to overcome inflammatory and allergic diseases. Front. Immunol. 13:937120. doi: 10.3389/fimmu.2022.937120
Received: 05 May 2022; Accepted: 26 July 2022;
Published: 14 September 2022.
Edited by:
Satoshi Tanaka, Kyoto Pharmaceutical University, JapanReviewed by:
Simon Patrick Hogan, Michigan Medicine, University of Michigan, United StatesCopyright © 2022 Zhang, Ernst, Kiyono and Kurashima. This is an open-access article distributed under the terms of the Creative Commons Attribution License (CC BY). The use, distribution or reproduction in other forums is permitted, provided the original author(s) and the copyright owner(s) are credited and that the original publication in this journal is cited, in accordance with accepted academic practice. No use, distribution or reproduction is permitted which does not comply with these terms.
*Correspondence: Yosuke Kurashima, eW9zdWtla0BjaGliYS11Lmpw
Disclaimer: All claims expressed in this article are solely those of the authors and do not necessarily represent those of their affiliated organizations, or those of the publisher, the editors and the reviewers. Any product that may be evaluated in this article or claim that may be made by its manufacturer is not guaranteed or endorsed by the publisher.
Research integrity at Frontiers
Learn more about the work of our research integrity team to safeguard the quality of each article we publish.