- Lydia Becker Institute of Immunology and Inflammation, School of Biological Sciences, Faculty of Biology, Medicine and Health, University of Manchester, Manchester Academic Health Science Centre, Manchester, United Kingdom
Mast cells occupy a unique niche within tissues as long lived perpetrators of IgE mediated hypersensitivity and anaphylaxis, as well as other immune responses. However, mast cells are not identical in different tissues and the impact of this tissue heterogeneity on the interaction with other immune cells and on defined immune responses is still unclear. In this review, we synthesize the characteristics of mast cell heterogeneity in the gut and the skin. Furthermore, we attempt to connect mast cell heterogeneity with functional diversity by exploring differences in mast cell-induced immune cell recruitment in these two model organs. The differential expression of certain receptors on mast cells of different tissues, notably tissue-specific expression patterns of integrins, complement receptors and MRGPRX2, could indicate that tissue environment-dependent factors skew mast cell-immune cell interactions, for example by regulating the expression of these receptors.
Introduction
Beyond their classical role in IgE-mediated hypersensitivity reactions, mast cells (MCs) are now recognised to have diverse immunological functions (1). Their longevity as tissue-resident cells, particularly at barrier sites in the skin, intestine, lung and around blood vessels makes them uniquely situated to initiate, shape and resolve responses to insults, injury and infections (2). Additionally, we now appreciate MCs exhibit remarkable plasticity and specialisation such that, not only are their phenotype and responses shaped by their specific tissue environment in a steady-state, but also, the particular repertoire of preformed and synthesised mediators they release is context and stimulus-dependent (3). Here, we highlight current understanding of the differing nature of MC-induced immune cells recruitment, drawing on the examples of skin and intestinal MCs as archetypes of the traditional distinction between connective tissue and mucosal MCs.
Mast cell mediator release
MCs of differing type and species produce a vast array of biologically active molecules including proteases, biogenic amines, cytokines, chemokines, growth factors and eicosanoids (4). While some of these products are stored in secretory granules, for near immediate release upon activation, others are newly synthesised and secreted within hours. Secretory granules consist of a dense gel matrix core formed of negatively charged proteoglycans such as heparin and chondroitin sulphate and amines such are histamine and serotonin as well as proteases, which typically, but variably include tryptases, chymases, carboxypeptidase A3, beta-hexosaminidase and cathepsins among others (5). Some cytokines including most notably TNFα, but also IL-4, IL-5, IL-13, IL-16, TGFβ, SCF, and growth factors, VEGF and NGF have been reported to be stored in MC granules in some circumstances (6–10). The release of granules, or their contents is tightly regulated and is an active process requiring calcium signalling, nucleoside catabolism and actin reorganisation (6).
Little is known about the cellular mechanisms of preformed mediator-storage in MCs apart from that of TNFα, which differs even between mice and human MCs, but involves transient exocytosis and re-endocytosis in humans (11). However, multiple different secretory vesicles and degranulation pathways exist suggesting a great deal of complexity in the control of secretion (12, 13). Apart from multigranular exocytosis, MCs also release smaller amounts of granule contents through piecemeal degranulation, kiss-and-run exocytosis, extracellular vesicle release and immune synapse formation. The release of de novo synthesised chemokines/cytokines likely differs from secretory granule mechanisms (6).
Whilst MC degranulation induced by FcϵR1α crosslinking, C3a, or substance P, results in immediate release, and later de-novo synthesis of chemokines/cytokines (14–16), activation of other receptors [reviewed elsewhere (1)] leads to more finely-tuned responses. For example IgE binding, in the absence of antigen-crosslinking, induces the release of IL-6 (17) and increases MCP-1 and CXCL8 release from IL-4-conditioned MCs (18). Weak, low receptor occupancy, FcϵR1α-crosslinking favours the production of chemokines, CCL2, CCL3 and CCL4 over cytokine production (19). Toll-Like Receptor (TLR) engagement results in the release of cytokines (eg IL-6) and chemokines in the absence of degranulation (1). MC responses are individualised to specific bacteria, where pathogenic L.monocytogenes induces degranulation and high levels of CXCL8 and MCP-1 release, while gut commensal E.coli induces lower levels of CXCL8 and MCP-1 without degranulation. Skin commensal S.aureus on the other hand does not induce CXCL8 or MCP-1 release and induces PGD2 at much lower levels (3). MC responses to viral infection include TNFα, type I interferon (20, 21), IL-4, IL-13, and chemokine production (22, 23). Purinergic receptor activation of MCs also releases cytokines (IL-6, TNFα, IL-33), leukotrienes, and chemokines (CCL2, CCL7, CXCL2) (24, 25).
The full plethora or MC mediators, cytokines, chemokines and their various roles are extensively reviewed elsewhere (1, 26). Those that induce immune cell recruitment and their cellular targets are listed in Table 1. MC-mediated activities that recruit leukocytes across tissues involves increasing vascular permeability (tryptase, histamine, VEGF, IL-6, CXCL8, LTC4, PGD2) (65, 66), upregulating adhesion molecule expression (TNFα) (26), recruitment of neutrophils (TNFα, IL-6, CXCL8, CXCL2) (51) monocyte/macrophages (TNFα, IL-6, CCL2) (67), ILCs (PGD2) (25), DCs (TNFα, GM-CSF, IL-1β) (68, 69), and CD8+ T-cells, (CCL5, LTB4) (4, 70). For example TNFα is stored and released by MCs from both skin and gut (71, 72), CCL2 is widely secreted and belongs to the core MC-transcriptional signature (44, 73, 74), and MCs have been shown to be essential for cell recruitment in many murine models (50, 51, 75). Mediator release is influenced by the inflammatory environment, for instance by IL-4, IL-5, IL-9, IL-33 and IFNγ (4, 76–79) which further regulate the expression of MC IL-2, IL-13, IL-9 and IL-25 (25, 29, 80–82). However there are tissue-specific aspects such as intestinal MCs lacking IFNγ (27) and skin MCs not producing LTC4 (57), which are yet to be fully elucidated.
Mast cell heterogeneity between and within tissues
The traditional categorisation of MCs based on histological findings into connective tissue (CTMC) and mucosal MCs (MMCs) in mice, and the human correlates of tryptase+ (MCT), tryptase+ and chymase+ (MCTC) and chymase+ (MCC) is well established (83–86). However, at transcriptional level, protease content exhibits greater tissue-specific heterogeneity both between and within tissues (73, 87). Additionally, while evolutionarily conserved, there are also differences between human and mouse mast cells (88).
One source of tissue heterogeneity is the MC embryonic origin. In both humans and mice, tissue MCs are seeded from MC progenitors originating from both embryonic yolk sac and adult bone marrow (89–91). During embryogenesis in mice, three waves of MCs are seeded successively from early erythromyeloid progenitors (EMPs), late EMPs and foetal hematopoietic stem cells. At birth, early EMPs-derived MCs constitute over 15% of MCs in pleural cavity, adipose tissue and skin, but less than 3% in gut and spleen. In contrast HSC-derived MCs were much higher in gut (>30%) than in skin (~8%) (89). In adult mice early EMP-derived MCs are maintained in the adipose tissue, a likely stem cell niche for MCs (92), but give way to other MC progenitors in skin (89, 90). Although sharing a core MC signature, these cells are transcriptionally distinct. Both embryonic and bone marrow-derived MCs, complete their maturation in peripheral tissues (89, 92). They are therefore subject to tissue-specific cues during that maturation process making them, to some degree, site specific. Additionally, in mice skin MCs are known to undergo in situ self-renewal by clonal expansion in the steady-state (93), whereas mucosal MCs are dependent on recruitment of MCp from the circulation (94–96). Nevertheless, both MMCs and CTMCs can be augmented by recruitment of MCp from the circulation during inflammation (93, 97, 98). Given the longevity of MCs this implies that environmental interactions during a lifespan can alter MC set points in the tissue which adds further complexity to MC heterogeneity.
A growing body of transcriptomic work demonstrates that MCs are distinct and highly variable between and within tissues. Indeed, MCs form a distinct transcriptional cluster differing greatly from other granulocytes. Furthermore, comparing between different types of connective tissue MCs, nearly 1000 genes can be differentially expressed (73).
Mouse MCs also display a marked tissue heterogeneity in their receptor profile (87). In both humans and mice TLR4 expression is low in skin, peritoneal and duodenal MCs but higher in colon and lung (99). Similarly, in mice the ATP receptor P2X7 is expressed more greatly in MCs from colon or lung rather than skin (24). C5aR1 expression also differs in some circumstances, in humans (100, 101). MRGPR receptor gene expression is a core component of murine foetal derived MCs (89) and tissue-resident MCs (73). However, intriguingly MRGPRX2, a receptor with wide agonist range and a key driver of pseudo-allergic reactions, shows large variation between tissues in humans (102). It is expressed at a high level in human skin, fat and synovial MCs, i.e. CTMCs. It is only expressed at low levels in lung and colonic MCs, which are refractory to substance P stimulation (57). Thus, this would suggest a distinct MRGPRX2-mediated response between mucosal and connective tissue.
Single cell RNA sequencing (scRNA-seq) across disease states in eosinophilic oesophagitis revealed a predominant tryptase and amphiregulin positive population of MCTC in the lamina propria during homeostasis with the development of persistent epithelial localised chymase and cathepsin G high expressing MCs, and transient CD117high, ST2high population during active disease. In remission the transient locally proliferative population disappears, while the chymase population remains in the epithelium and the resident lamina propria MCs become CSF1high. These changes exemplify the plastic nature of human MCs which show both acute and chronic phenotypic alterations in response to tissue environment and spatial compartmentalisation. For example, MCs may switch from homeostatic to an inflammatory IL-33-responsive, and end with an IL-13-producing, macrophages supportive phenotype (103). Similar results have been obtained from scRNAseq studies in chronic rhinosinusitis in MCT cells (CD117low, FcϵR1αlow), and MCTC (CD117high, chymase+, cathepsin G+) from the epithelial and sub-epithelial compartments respectively. MCTC expressed ST2, CSF1 and IL-13 in addition to CCL2, CCL3 and CCL4, whereas MCT expressed TRAIL, FGL2 and IL-17RB. In this tissue, the authors discovered polarised states of a core set of MC genes that distinguished them from proliferative and unpolarised MCs and concluded that the polarised cells are primed to respond either according to a pro-inflammatory (MCTC) or a Th2-skewed pattern (MCT). Although similarities existed between MCTC in this study and skin MCTC, the authors showed key differences in C5aR1 and MRGPRX2 expression, not only between the two populations but also within the lung (104). The continuous, rather than discrete, nature of human MC heterogeneity in the respiratory tract is supported by evidence from a large number of expressed cell surface proteins which show a continuous distribution in their expression (105).
Despite the marked transcriptomic heterogeneity observed by several authors, proteomic comparisons between two types of human connective tissue MCs, skin and fat, showed remarkably low levels of differentially expressed markers, at least in a quiescent state (102). There was also a remarkable interspecies correlation between mouse and human CTMCs and a common mast cell protein signature (102). Additionally, although mouse peritoneal MCs are reported to express the transcript for TLR4 (89), it was not found among 4620 MC expressed proteins during proteomic analysis (102).
In summary, discussions about the role of MCs in a given tissue drawn from general aspects of MC biology obtained across multiple tissues, cell lines or models are worthwhile and useful commentaries. However, one should challenge those views in light of the well-established tissue-specific heterogeneity of MCs and undertake a bigger effort in investigating MC-driven tissue-specific aspects of immunity.
Mast cell-mediated immune cell recruitment in the gut
Situated throughout the gastro-intestinal tract, MCs are regarded as key in controlling organ homeostasis (106). Contributing to the maintenance of the epithelial barrier, the initiation and modulation of both innate and adaptive immune responses to pathogens, and the cross-talk with the enteric nervous system, MCs regulate tissue homeostasis and disease (107).
Intestine constitutive homing of MCp in mice, via transendothelial migration, is controlled by MC α4β7 integrin binding to adhesion molecules MAdCAM-1 and VCAM-1 (96) while chemoattraction is dependent on MC CXCR2 (108). Since germ-free mice have fewer intestinal MCs and express lower levels of CXCR2 ligands, this process is thought to be driven by commensal bacterial interactions with intestinal epithelial cells (109). In humans, intestinal MCs express mainly α2β1 integrin, which is not expressed in other mucosal or connective tissue MCs (110).
MCs play a key role in the acute inflammatory response of the gut (see Figure 1). MC-derived TNFα recruits neutrophils, eosinophils and macrophages (94). Furthermore, human intestinal MCs produce an array of chemokines de novo when primed with SCF or IL-4 and activated by FcεRI-receptor crosslinking. These include CCL1, CCL2, CCL3, CCL4, CCL5, CCL18, CCL20, CXCL2, CXCL3, CXCL8 and XCL1 (44). Interestingly, CCL2, but not CXCL8, release by intestinal MCs in response to FcϵRIα activation exhibits a diurnal pattern indicating a role for MCs in intestine circadian biology (111). Moreover, in a gut injury mouse model, MC activation and Mcpt5, Mcpt6 and CPA3 protease release, is associated with neutrophil influx and alterations in epithelial barrier integrity. MC stabiliser cromolyn sodium results in preservation of barrier integrity, reduced neutrophil influx associated with significant reductions in TNFα, CCL2, CCL5, IL-1β and CXCL1 (112).
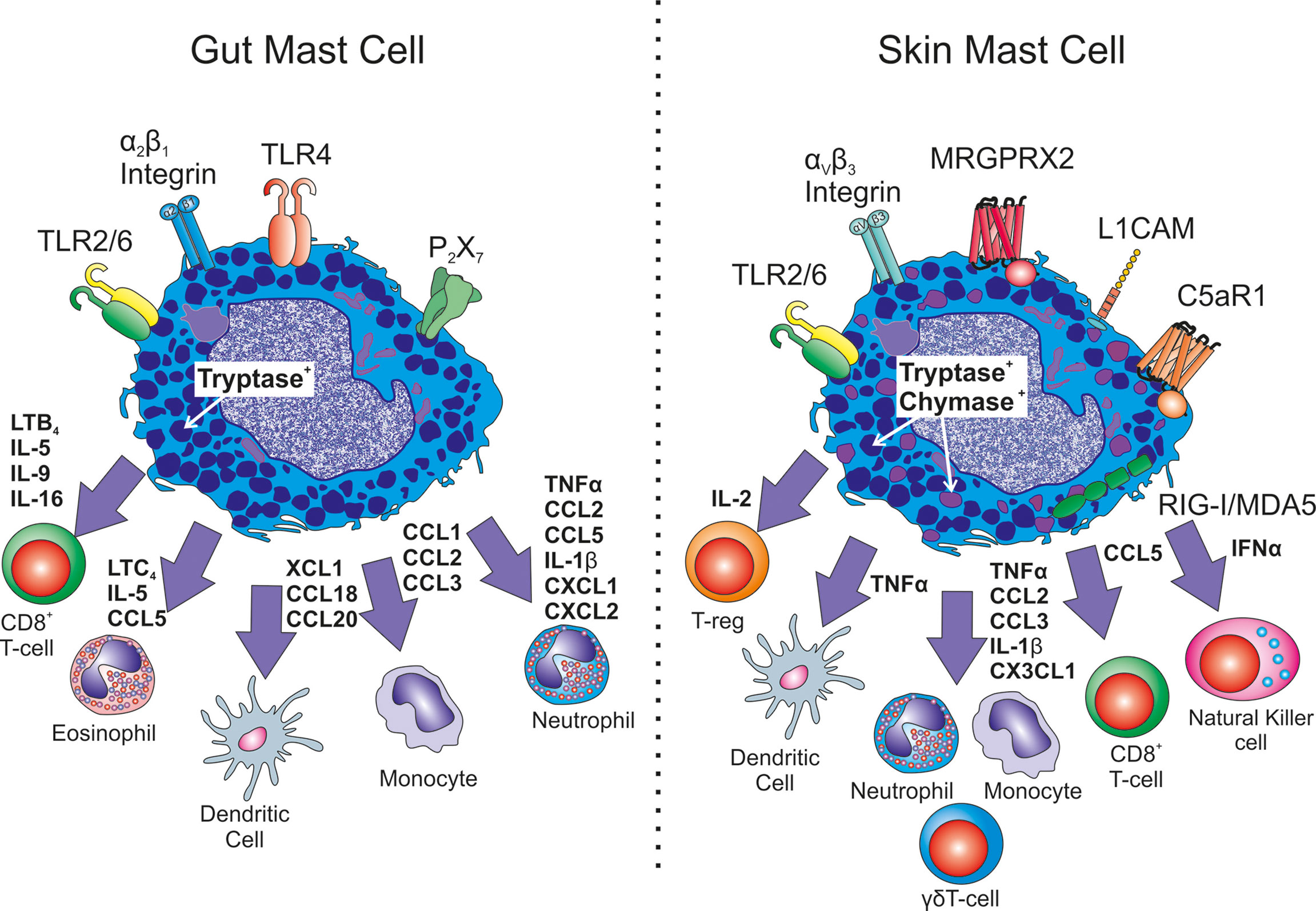
Figure 1 Heterogeneity in immune cell recrutiment. Exemplary differences between mucosal gut mast cells and connective tissue skin mast cells are shown. Differences in receptor expression include TLR4, P2X7, L1CAM, C5aR1, MRGPRX2 as well as integrin expression. Different mast cell mediators have been shown to be released with altered down stream consequences for immune cell recruitment in infection or inflammation.
Direct evidence of MC involvement in anti-parasitic immunity of the gut is provided by a number of rodent models. Trichinella spiralis infection induces an influx of MC to the gut which transiently alters their protease expression. This is associated with a strong Th2 immune response, eosinophilia and marked increase in barrier permeability. The MC influx and the transiently-expressed MC-derived Mcpt1 is critical to control worm burden (113). Additionally, Mcpt6 is required for eosinophil recruitment (114). Mice lacking the proteoglycan serglycin, important in granule storage, also exhibit a deficit in worm clearance with lower circulating TNFα, IL-1β, IL-10, IL-13 compared to wild type infected controls (115). Human skin MCs also express these cytokines, and IL-13 downregulates Th1 responses in mice (116, 117), which raises the prospect that intestinal MCs could be a critical source of these cytokines in anti-parasitic immunity. Additionally Th2/Th9 cytokine IL-9, released early during parasitic infections and important for murine worm expulsion (118, 119) is also secreted by MC activated synergistically with LPS (120, 121), although the importance of MC-derived IL-9 in the intestine has not been proven definitively. In this regard, MCs in human gut are also immunoreactive for IL-5 and IL-16 both of which enhance T-cell recruitment and activation (26, 99, 122).
MCs have also been described to limit human colorectal cancers. In murine models MC-derived LTB4 was found to be critical for CD8+ T-cell recruitment and anti-tumor immunity (123).
Mast cell-mediated immune cell recruitment in the skin
Like the gut, the skin is a barrier exposed to a wide array of environmental insults on a nearly continual basis. However, the skin is generally a less permeable barrier than the gut and as such MCs localise to the upper dermis around eccrine glands and blood vessels and not to the epidermis (124). Therefore, they regularly encounter fewer pathogens unless barrier integrity is disturbed through mechanical (e.g. wound or bite) or inflammatory means. This is thought to be a reason why TLR and P2X7 expression in skin MCs appears low, and maintains the cells in an unresponsive state (99). Low TLR4 expression might imply a diminished role for skin MCs in human skin Th9 biology (125). To our knowledge IL-9 has not been demonstrated in skin MCs. Nevertheless, skin MCs do respond to lipoteichoic acid, a TLR2 agonist component of commensal gram-positive bacterial cell walls, leading to enhanced responses to pox causing vaccinia virus in mice (126). Similar to recruitment in the gut, the skin microbiome is responsible for MC maturation. In this case through TLR2-dependent SCF production from keratinocytes (127) rather than direct interaction. In humans their adhesion is probably dependent on a different integrin αvβ3 (128). Large amounts of retinoic acid can also overcome the P2X7 down regulation in mouse skin MCs (129), emphasising the plasticity of tissue-resident cells.
Like other MCs, skin MCs have been identified to store and release TNFα in response to SP, FcϵRI, UVB and calcium ionohpore activation (130–132). There is good evidence from the murine passive cutaneous anaphylaxis model and delayed hypersensitivity reactions in skin, that leukocyte recruitment is dependent on MC-derived TNFα (51, 133), and that CD8+ dendritic cell migration to draining lymph nodes of the skin also relies on MC TNFα (68, 134) (Figure 1). Skin MCs can also exocytose TNFα containing granules which can be transported to the draining lymph nodes to enhance antigen presentation and antigen specific responses (135). This isn’t a phenomenon so far identified in other tissues.
MCs have recently been shown to have antiviral immune activities to dengue virus, a mosquito borne pathogen via activation of innate response pathway RIG-I/MDA5 resulting in TNFα, IFNα, CCL5, CXCL12 and CX3CL1 production. These MC-derived cytokines appear to be critical to limiting viral spread to lymph nodes through recruitment of NK and NKT-cells to the infected skin in a mouse model (53). Additionally, MCs form an immune synapse in infected skin with γδT-cells which induced activation through the T-cell receptor (136). There is also evidence that MCs are important in limiting other intradermal viral infections through DC activation (137). MC-derived TNFα and IL-6 have also been shown to be important for protection from cutaneous herpes simplex virus infection (138). In contrast, intestinal MCs are thought to have deficiencies in IFNγ production, and whilst RIG-I might be present in lung mucosal MCs it has not yet been identified in gut MCs (139).
The recently identified MC receptor for substance P MRGPRX2 and its murine homologue mrgprb2 (140, 141) has revealed a surprising dependence of a large degree of skin inflammation and pain on MCs. Green et al. (142) observed that Mrgprb2-/- mice had significantly reduced hypersensitivity to inflammatory pain with not only ablated MC recruitment, but also reduced neutrophil and monocyte recruitment to the affected skin with a reduction in both CCL2 and CCL3 in response to injury or SP treatment of skin tissue. There has been much speculation about the involvement of gut MCs in painful symptoms of disease, however such compelling evidence is still lacking in other tissues and mucosal MCs have low MRGPRX2 expression.
In different murine models and human tissue samples, it has been demonstrated that skin MCs are sources of other cytokines with a likely impact on inflammation and immune activation. These include; IL-1β, which drives inflammasome activation and neutrophil recruitment in the skin (143, 144), an association also present in the gut (112); IL-2, not yet identified in gut MCs, which supresses inflammation in contact-hypersensivity through MC-dependent T-regulatory cell recruitment (30); IL-4 which drives type 2 inflammation in atopic dermatitis (32); IL-10 which as one of the first products of MCs, limit contact hypersensitivity reactions (37, 145) and also has a role in MC intestinal IBD pathology (146).
Conclusions
Despite their heterogeneous origins, functionally, MCs from diverse tissues share a common set of responses including not least to IgE/allergen but also the release of key cytokines and chemokines involved in the initiation of inflammation i.e. TNFα, IL-1β, CCL2. MC plasticity then allows further functional differentiation based on the tissue context and lifetime environmental interactions such as pathogen or allergen exposure, or type I/type II inflammation. This plasticity will be shaped by existing heterogeneity in receptor expression such as C5aR1, MRGPRX2 and P2X7 which will limit the form of response that the cells can mount to a particular stimulus. For instance skin MCs might be better equipped to release IFNγ than intestinal MCs (27) whereas skin MCs will be more susceptible to neurogenic inflammation (57). Ultimately the application of new multi-omics, single cell and imaging mass cytometry technologies to isolated cells and tissues will reveal an as yet unforeseen level of detail about tissue-specific heterogeneity.
Author Contributions
PW and SB-P reviewed literature and wrote the article. All authors contributed to the article and approved the submitted version.
Funding
SBP is the recipient of a grant from MRC DPFS: MR/S036954/1. PW is supported by a GSK research grant. The funder was not involved in the collection of data, analysis, interpretation of data, the writing of this article or the decision to submit it for publication.
Conflict of Interest
The authors declare that the review was conducted in the absence of any commercial or financial relationships that could be construed as a potential conflict of interest.
Publisher’s Note
All claims expressed in this article are solely those of the authors and do not necessarily represent those of their affiliated organizations, or those of the publisher, the editors and the reviewers. Any product that may be evaluated in this article, or claim that may be made by its manufacturer, is not guaranteed or endorsed by the publisher.
Abbreviations
FcϵRI, high-affinity IgE receptor; IL, interleukin; MCs, mast cells; MRGPRX2, Mas-related G-protein coupled receptor member X2; TNF, Tumor necrosis factor; TGF, transforming growth factor; SCF, Stem cell factor; VEGF, Vascular endothelial growth factor; NGF, Nerve growth factor; MCP, Monocyte chemoattractant protein; TLR, Toll-like receptor; PGD, Prostaglandin D; LTC, leukotriene C; GM-CSF, Granulocyte-macrophage colony stimulating factor; IFN interferon; CTMC, Connective tissue mast cell; MMC, Mucosal mast cell; EMP, Erythromyeloid progenitor; HSC, hematopoietic stem cell; CSF, Colony stimulating factor; TRAIL, Tumor necrosis factor-related apoptosis-inducing ligand; FGL, Fibrinogen-like protein; MadCAM, mucosal addressin cell adhesion molecule-1; VCAM, vascular cell adhesion molecule; RIG-I, retinoic acid-inducible gene I; MDA-5, melanoma differentiation-associated protein 5; NK, Natural killer; SP, Substance P.
References
1. Elieh Ali Komi D, Wöhrl S, Bielory L. Mast cell biology at molecular level: A comprehensive review. Clin Rev Allergy Immunol (2020) 58:342–65. doi: 10.1007/S12016-019-08769-2
2. Chan CY, St John AL, Abraham SN. Plasticity in mast cell responses during bacterial infections. Curr Opin Microbiol (2012) 15:78–84. doi: 10.1016/j.mib.2011.10.007
3. Garcia-Rodriguez KM, Bahri R, Sattentau C, Roberts IS, Goenka A, Bulfone-Paus S. Human mast cells exhibit an individualized pattern of antimicrobial responses. Immunity Inflammation Dis (2020) 8:198–210. doi: 10.1002/IID3.295
4. Bulfone-Paus S, Bahri R. Mast cells as regulators of T cell responses. Front Immunol (2015) 6:394. doi: 10.3389/fimmu.2015.00394
5. Vukman KV, Försönits A, Oszvald Á, Tóth E, Buzás EI. Mast cell secretome: Soluble and vesicular components. Semin Cell Dev Biol (2017) 67:65–73. doi: 10.1016/j.semcdb.2017.02.002
6. Blank U, Madera-Salcedo IK, Danelli L, Claver J, Tiwari N, Sánchez-Miranda E, et al. Vesicular trafficking and signaling for cytokine and chemokine secretion in mast cells. Front Immunol (2014) 5:453. doi: 10.3389/FIMMU.2014.00453
7. de Paulis A, Minopoli G, Arbustini E, de Crescenzo G, Dal Piaz F, Pucci P, et al. Stem cell factor is localized in, released from, and cleaved by human mast cells. J Immunol (1999) 163:2799–808. doi: 10.4049/issn.1550-6606
8. Lindstedt KA, Wang Y, Shiota N, Saarinen J, Hyytiäinen M, Hyytiäinen H, et al. Activation of paracrine TGF-β1 signaling upon stimulation and degranulation of rat serosal mast cells: A novel function for chymase. FASEB J (2001) 15:1377–88. doi: 10.1096/FJ.00-0273COM
9. Leon A, Buriani A, Dal Toso R, Fabris M, Romanello S, Aloe L, et al. Mast cells synthesize, store, and release nerve growth factor. Proc Natl Acad Sci U S A (1994) 91:3739–43. doi: 10.1073/PNAS.91.9.3739
10. Laberge S, Pinsonneault S, Ernst P, Olivenstein R, Ghaffar O, Center DM, et al. Phenotype of IL-16-Producing cells in bronchial mucosa: Evidence for the human eosinophil and mast cell as cellular sources of IL-16 in asthma. Int Arch Allergy Immunol (1999) 119:120–5. doi: 10.1159/000024186
11. Olszewski MB, Groot AJ, Dastych J, Knol EF. TNF trafficking to human mast cell granules: Mature chain-dependent endocytosis. J Immunol (2007) 178:5701–9. doi: 10.4049/JIMMUNOL.178.9.5701
12. Woska JR, Gillespie ME. SNARE complex-mediated degranulation in mast cells. J Cell Mol Med (2012) 16:649. doi: 10.1111/J.1582-4934.2011.01443.X
13. Puri N, Roche PA. Mast cells possess distinct secretory granule subsets whose exocytosis is regulated by different SNARE isoforms. Proc Natl Acad Sci USA (2008) 105:2580–5. doi: 10.1073/PNAS.0707854105
14. Kulka M, Sheen CH, Tancowny BP, Grammer LC, Schleimer RP. Neuropeptides activate human mast cell degranulation and chemokine production. Immunology (2008) 123:398–410. doi: 10.1111/j.1365-2567.2007.02705.x
15. West PW, Bahri R, Garcia-Rodriguez KM, Sweetland G, Wileman G, Shah R, et al. Interleukin-33 amplifies human mast cell activities induced by complement anaphylatoxins. Front Immunol (2021) 11:615236. doi: 10.3389/FIMMU.2020.615236
16. Oliveira SHP, Lukacs NW. Stem cell factor and IgE-stimulated murine mast cells produce chemokines (CCL2, CCL17, CCL22) and express chemokine receptors. Inflammation Res (2001) 50:168–74. doi: 10.1007/s000110050741
17. Kalesnikoff J, Huber M, Lam V, Damen JE, Zhang J, Siraganian RP, et al. Monomeric IgE stimulates signaling pathways in mast cells that lead to cytokine production and cell survival. Immunity (2001) 14:801–11. doi: 10.1016/S1074-7613(01)00159-5
18. Matsuda K, Piliponsky AM, Iikura M, Nakae S, Wang EW, Dutta SM, et al. Monomeric IgE enhances human mast cell chemokine production: IL-4 augments and dexamethasone suppresses the response. J Allergy Clin Immunol (2005) 116:1357–63. doi: 10.1016/J.JACI.2005.08.042
19. Gonzalez-Espinosa C, Odom S, Olivera A, Hobson JP, Cid Martinez ME, Oliveira-dos-Santos A, et al. Preferential signaling and induction of allergy-promoting lymphokines upon weak stimulation of the high affinity IgE receptor on mast cells. J Exp Med (2003) 197:1453–65. doi: 10.1084/JEM.20021806
20. Kulka M, Alexopoulou L, Flavell RA, Metcalfe DD. Activation of mast cells by double-stranded RNA: Evidence for activation through toll-like receptor 3. J Allergy Clin Immunol (2004) 114:174–82. doi: 10.1016/j.jaci.2004.03.049
21. Lappalainen J, Rintahaka J, Kovanen PT, Matikainen S, Eklund KK. Intracellular RNA recognition pathway activates strong anti-viral response in human mast cells. Clin Exp Immunol (2013) 172:121–8. doi: 10.1111/CEI.12042
22. Brown MG, McAlpine SM, Huang YY, Haidl ID, Al-Afif A, Marshall JS, et al. RNA Sensors enable human mast cell anti-viral chemokine production and IFN-mediated protection in response to antibody-enhanced dengue virus infection. PloS One (2012) 7:e34055. doi: 10.1371/JOURNAL.PONE.0034055
23. Saluja R, Metz M, Maurer M. Role and relevance of mast cells in fungal infections. Front Immunol (2012) 3:146. doi: 10.3389/FIMMU.2012.00146
24. Kurashima Y, Amiya T, Nochi T, Fujisawa K, Haraguchi T, Iba H, et al. Extracellular ATP mediates mast cell-dependent intestinal inflammation through P2X7 purinoceptors. Nat Commun (2012) 3:1034. doi: 10.1038/ncomms2023
25. Symowski C, Voehringer D. Interactions between innate lymphoid cells and cells of the innate and adaptive immune system. Front Immunol (2017) 8:1422. doi: 10.3389/FIMMU.2017.01422
26. Mukai K, Tsai M, Saito H, Galli SJ. Mast cells as sources of cytokines, chemokines and growth factors. Immunol Rev (2018) 282:121. doi: 10.1111/IMR.12634
27. Lorentz A, Schwengberg S, Sellge G, Manns MP, Bischoff SC. Human intestinal mast cells are capable of producing different cytokine profiles: Role of IgE receptor cross-linking and IL-4. J Immunol (2000) 164:43–8. doi: 10.4049/jimmunol.164.1.43
28. Summers SA, Chan J, Gan PY, Dewage L, Nozaki Y, Steinmetz OM, et al. Mast cells mediate acute kidney injury through the production of TNF. J Am Soc Nephrol (2011) 22:2226–36. doi: 10.1681/ASN.2011020182
29. Burd PR, Thompson WC, Max EE, Mills FC. Activated mast cells produce interleukin 13. J Exp Med (1995) 181:1373–80. doi: 10.1084/jem.181.4.1373
30. Hershko AY, Suzuki R, Charles N, Alvarez-Errico D, Sargent JL, Laurence A, et al. Mast cell interleukin-2 production contributes to suppression of chronic allergic dermatitis. Immunity (2011) 35:562–71. doi: 10.1016/J.IMMUNI.2011.07.013
31. Roediger B, Kyle R, Yip KH, Sumaria N, Guy TV, Kim BS, et al. Cutaneous immuno-surveillance and regulation of inflammation by group 2 innate lymphoid cells. Nat Immunol (2013) 14:564. doi: 10.1038/NI.2584
32. Horsmanheimo L, Harvima IT, Järvikallio A, Harvima RJ, Naukkarinen A, Horsmanheimo M. Mast cells are one major source of interleukin-4 in atopic dermatitis. Br J Dermatol (1994) 131:348–53. doi: 10.1111/j.1365-2133.1994.tb08522.x
33. Dubois GR, Bruijnzeel-Koomen CAFM, Bruijnzeel PLB. IL-4-Induced migration of eosinophils in allergic inflammation. Agents Actions (1994) 41:C256–7. doi: 10.1007/BF01987660
34. Bacon K, Gearing A, Camp R. Induction of In vitro human lymphocyte migration by interleukin 3, interleukin 4, and interleukin 6. Cytokine (1990) 2:100–5. doi: 10.1016/1043-4666(90)90003-C
35. Lorentz A, Schwengberg S, Mierke C, Manns MP, Bischoff SC. Human intestinal mast cells produce IL-5 in vitro upon IgE receptor cross-linking and In vivo in the course of intestinal inflammatory disease. Eur J Immunol (1999) 29:1496–503. doi: 10.1002/(SICI)1521-4141(199905)29:05<1496::AID-IMMU1496>3.0.CO;2-5
36. Li J, Chen S, Xiao X, Zhao Y, Ding W, Li XC. IL-9 and Th9 cells in health and diseases–from tolerance to immunopathology. Cytokine Growth Factor Rev (2017) 37:47–55. doi: 10.1016/J.CYTOGFR.2017.07.004
37. Grimbaldeston MA, Nakae S, Kalesnikoff J, Tsai M, Galli SJ. Mast cell-derived interleukin 10 limits skin pathology in contact dermatitis and chronic irradiation with ultraviolet b. Nat Immunol (2007) 8:1095–104. doi: 10.1038/ni1503
38. Abrams J, Figdor CG, De Waal Malefyt R, Bennett B, De Vries JE. Interleukin 10(IL-10) inhibits cytokine synthesis by human monocytes: An autoregulatory role of IL-10 produced by monocytes. J Exp Med (1991) 174:1209–20. doi: 10.1084/JEM.174.5.1209
39. Jinquan T, Larsen CG, Gesser B, Matsushima K, Thestrup-Pedersen K. Human IL-10 is a chemoattractant for CD8+ T lymphocytes and an inhibitor of IL-8-Induced CD4+ T lymphocyte migration. J Immunol (1993) 151:4545–51. doi: 10.1016/0923-1811(92)90162-5
40. Zhang X, Niessner A, Nakajima T, Ma-Krupa W, Kopecky SL, Frye RL, et al. Interleukin 12 induces T-cell recruitment into the atherosclerotic plaque. Circ Res (2006) 98:524–31. doi: 10.1161/01.RES.0000204452.46568.57
41. Rumsaeng V, Cruikshank WW, Foster B, Prussin C, Kirshenbaum AS, Davis TA, et al. Human mast cells produce the CD4+ T lymphocyte chemoattractant factor, IL-16. J Immunol (1997) 159:1904–2910.
42. Halwani R, Al-Kufaidy R, Vazquez-Tello A, Pureza MA, BaHammam AS, Al-Jahdali H, et al. IL-17 enhances chemotaxis of primary human b cells during asthma. PLoS One (2014) 9:e114604. doi: 10.1371/JOURNAL.PONE.0114604
43. Yoshimoto T, Nakanishi K. Roles of IL-18 in basophils and mast cells. Allergol Int (2006) 55:105–13. doi: 10.2332/ALLERGOLINT.55.105
44. Feuser K, Thon KP, Bischoff SC, Lorentz A. Human intestinal mast cells are a potent source of multiple chemokines. Cytokine (2012) 58:178–85. doi: 10.1016/J.CYTO.2012.01.001
45. Wang X, Chen H, Zhang M, Liu Z. Roles of mast cells and monocyte chemoattractant protein-1 in the renal injury of obesity-related glomerulopathy. Am J Med Sci (2013) 346:295–301. doi: 10.1097/MAJ.0B013E31827559F8
46. Wakahara S, Fujii Y, Nakao T, Tsuritani K, Hara T, Saito H, et al. Gene expression profiles for fc epsilon RI, cytokines and chemokines upon fc epsilon RI activation in human cultured mast cells derived from peripheral blood. Cytokine (2001) 16:143–52. doi: 10.1006/CYTO.2001.0958
47. Cheng JW, Sadeghi Z, Levine AD, Penn MS, von Recum HA, Caplan AI, et al. The role of CXCL12 and CCL7 chemokines in immune regulation, embryonic development, and tissue regeneration. Cytokine (2014) 69:277–83. doi: 10.1016/J.CYTO.2014.06.007
48. Tsicopoulos A, Chang Y, Ait Yahia S, De Nadai P, Chenivesse C. Role of CCL18 in asthma and lung immunity. Clin Exp Allergy (2013) 43:716–22. doi: 10.1111/CEA.12065
50. De Filippo K, Dudeck A, Hasenberg M, Nye E, Van Rooijen N, Hartmann K, et al. Mast cell and macrophage chemokines CXCL1/CXCL2 control the early stage of neutrophil recruitment during tissue inflammation. Blood (2013) 121:4930–7. doi: 10.1182/BLOOD-2013-02-486217
51. Biedermann T, Kneilling M, Mailhammer R, Maier K, Sander CA, Kollias G, et al. Mast cells control neutrophil recruitment during T cell-mediated delayed-type hypersensitivity reactions through tumor necrosis factor and macrophage inflammatory protein 2. J Exp Med (2000) 192:1441–52. doi: 10.1084/jem.192.10.1441
52. Ohta T, Sugiyama M, Hemmi H, Yamazaki C, Okura S, Sasaki I, et al. Crucial roles of XCR1-expressing dendritic cells and the XCR1-XCL1 chemokine axis in intestinal immune homeostasis. Sci Rep (2016) 6:1–11. doi: 10.1038/srep23505
53. St. John AL, Rathore APS, Yap H, Ng ML, Metcalfe DD, Vasudevan SG, et al. Immune surveillance by mast cells during dengue infection promotes natural killer (NK) and NKT-cell recruitment and viral clearance. Proc Natl Acad Sci USA (2011) 108:9190–5. doi: 10.1073/PNAS.1105079108
54. Liu WM, Jiang L, Bian C, Liang Y, Xing R, Yishakea M, et al. Role of CX3CL1 in diseases. Arch Immunol Ther Exp (2016) 64:371–83. doi: 10.1007/S00005-016-0395-9
55. Subramanian BC, Majumdar R, Parent CA. The role of the LTB4-BLT1 axis in chemotactic gradient sensing and directed leukocyte migration. Semin Immunol (2017) 33:16–29. doi: 10.1016/J.SMIM.2017.07.002
56. Taube C, Miyahara N, Ott V, Swanson B, Takeda K, Loader J, et al. The leukotriene B4 receptor (BLT1) is required for effector CD8+ T cell-mediated, mast cell-dependent airway hyperresponsiveness. J Immunol (2006) 176:3157–64. doi: 10.4049/JIMMUNOL.176.5.3157
57. Varricchi G, Pecoraro A, Loffredo S, Poto R, Rivellese F, Genovese A, et al. Heterogeneity of human mast cells with respect to MRGPRX2 receptor expression and function. Front Cell Neurosci (2019) 13:299/BIBTEX. doi: 10.3389/FNCEL.2019.00299/BIBTEX
58. Kopicky-Burd JA, Kagey-Sobotka A, Peters SP, Dvorak AM, Lennox DW, Lichtenstein LM, et al. Characterization of human synovial mast cells. J Rheumatol (1988) 15:1326–33.
59. Busse WW. Leukotrienes and inflammation. Am J Respir Crit Care Med (1998) 157:S210–3. doi: 10.1164/ajrccm.157.6.mar-1
60. Komi DEA, Mortaz E, Amani S, Tiotiu A, Folkerts G, Adcock IM. The role of mast cells in IgE-independent lung diseases. Clin Rev Allergy Immunol (2020) 58:377–87. doi: 10.1007/s12016-020-08779-5
61. Jin J, Sunusi S, Lu H. Group 2 innate lymphoid cells (ILC2s) are important in typical type 2 immune-mediated diseases and an essential therapeutic target. J Int Med Res (2022) 50:1–14. doi: 10.1177/03000605211053156
62. Kastl BC, Pohlman LM. “Basophils, Mast Cells, and Their Disorders,” in Schalm’s Veterinary Hematology, eds. M. B Brooks, K. E Harr, D. M Seelig, K. J Wardrop, D. J Weiss, (John Wiley & Sons, Ltd), 373–380. doi: 10.1002/9781119500537.CH47
63. Arima M, Fukuda T. Prostaglandin D2 and TH2 inflammation in the pathogenesis of bronchial asthma. Korean J Intern Med (2011) 26:8. doi: 10.3904/KJIM.2011.26.1.8
64. Galli SJ, Nakae S, Tsai M. Mast cells in the development of adaptive immune responses. Nat Immunol (2005) 6:135–42. doi: 10.1038/ni1158
65. Kennelly R, B.Conneely J, Bouchier-Hayes D, C. Winter D. Mast cells in tissue healing: From skin to the gastrointestinal tract. Curr Pharm Des (2011) 17:3772–5. doi: 10.2174/138161211798357854
66. Ashina K, Tsubosaka Y, Nakamura T, Omori K, Kobayashi K, Hori M, et al. Histamine induces vascular hyperpermeability by increasing blood flow and endothelial barrier disruption In vivo. PLoS One (2015) 10(7):e0132367. doi: 10.1371/JOURNAL.PONE.0132367
67. Chu Y-T, Wan S-W, Anderson R, Lin Y-S. Mast cell-macrophage dynamics in modulation of dengue virus infection in skin. Immunology (2015) 146:163–72. doi: 10.1111/imm.12492
68. Suto H, Nakae S, Kakurai M, Sedgwick JD, Tsai M, Galli SJ. Mast cell-associated TNF promotes dendritic cell migration. J Immunol (2006) 176:4102–12. doi: 10.4049/jimmunol.176.7.4102
69. de Vries VC, Pino-Lagos K, Nowak EC, Bennett KA, Oliva C, Noelle RJ. Mast cells condition dendritic cells to mediate allograft tolerance. Immunity (2011) 35:550–61. doi: 10.1016/J.IMMUNI.2011.09.012
70. Ott VL, Cambier JC, Kappler J, Marrack P, Swanson BJ. Mast cell–dependent migration of effector CD8+ T cells through production of leukotriene B4. Nat Immunol 2003 410 (2003) 4:974–81. doi: 10.1038/ni971
71. Bischoff SC, Lorentz A, Schwengberg S, Weier G, Raab R, Manns MP. Mast cells are an important cellular source of tumour necrosis factor α in human intestinal tissue. Gut (1999) 44:643–52. doi: 10.1136/GUT.44.5.643
72. Walsh LJ, Trinchieri G, Waldorf HA, Whitaker D, Murphy GF. Human dermal mast cells contain and release tumor necrosis factor α, which induces endothelial leukocyte adhesion molecule 1. Proc Natl Acad Sci USA (1991) 88:4220–4. doi: 10.1073/PNAS.88.10.4220
73. Dwyer DF, Barrett NA, Austen KF, Kim EY, Brenner MB, Shaw L, et al. Expression profiling of constitutive mast cells reveals a unique identity within the immune system. Nat Immunol (2016) 17:878–87. doi: 10.1038/ni.3445
74. Gordon JR. Monocyte chemoattractant peptide-1 expression during cutaneous allergic reactions in mice is mast cell dependent and largely mediates the monocyte recruitment response. J Allergy Clin Immunol (2000) 106:110–6. doi: 10.1067/MAI.2000.107036
75. Dudeck J, Kotrba J, Immler R, Hoffmann A, Voss M, Alexaki VI, et al. Directional mast cell degranulation of tumor necrosis factor into blood vessels primes neutrophil extravasation. Immunity (2021) 54:468–83.e5. doi: 10.1016/J.IMMUNI.2020.12.017
76. Bischoff SC, Sellge G, Lorentz A, Sebald W, Raab R, Manns MP. IL-4 enhances proliferation and mediator release in mature human mast cells. Proc Natl Acad Sci (1999) 96:8080–5. doi: 10.1073/pnas.96.14.8080
77. Ochi H, De Jesus NH, Hsieh FH, Austen KF, Boyce JA. IL-4 and -5 prime human mast cells for different profiles of IgE-dependent cytokine production. Proc Natl Acad Sci (2000) 97:10509–13. doi: 10.1073/pnas.180318697
78. Kearley J, Erjefalt JS, Andersson C, Benjamin E, Jones CP, Robichaud A, et al. IL-9 governs allergen-induced mast cell numbers in the lung and chronic remodeling of the airways. Am J Respir Crit Care Med (2011) 183:865–75. doi: 10.1164/rccm.200909-1462OC
79. Bischoff SC, Sellge G, Manns MP, Lorentz A. Interleukin-4 induces a switch of human intestinal mast cells from proinflammatory cells to Th2-type cells. Int Arch Allergy Immunol (2001) 124:151–4. doi: 10.1159/000053695
80. Ho LH, Ohno T, Oboki K, Kajiwara N, Suto H, Iikura M, et al. IL-33 induces IL-13 production by mouse mast cells independently of IgE-fc RI signals. J Leukoc Biol (2007) 82:1481–90. doi: 10.1189/jlb.0407200
81. Moretti S, Renga G, Oikonomou V, Galosi C, Pariano M, Iannitti RG, et al. A mast cell-ILC2-Th9 pathway promotes lung inflammation in cystic fibrosis. Nat Commun (2017) 8:14017. doi: 10.1038/ncomms14017
82. Hepworth MR, Daniłowicz-Luebert E, Rausch S, Metz M, Klotz C, Maurer M, et al. Mast cells orchestrate type 2 immunity to helminths through regulation of tissue-derived cytokines. Proc Natl Acad Sci USA (2012) 109:6644–9. doi: 10.1073/PNAS.1112268109
83. Ribatti D. The staining of mast cells: A historical overview. Int Arch Allergy Immunol (2018) 176:55–60. doi: 10.1159/000487538
84. Enerbäck L. Mast cells in rat gastrointestinal mucosa. Acta Pathol Microbiol Scand (1966) 66:303–12. doi: 10.1111/APM.1966.66.3.303
85. Irani AM, Bradford TR, Kepley CL, Schechter NM, Schwartz LB. Detection of MCT and MCTC types of human mast cells by immunohistochemistry using new monoclonal anti-tryptase and anti-chymase antibodies. J Histochem Cytochem (1989) 37:1509–15. doi: 10.1177/37.10.2674273
86. Pejler G, Åbrink M, Ringvall M, Wernersson S. Mast cell proteases. Adv Immunol (2007) 95:167–255. doi: 10.1016/S0065-2776(07)95006-3
87. Akula S, Paivandy A, Fu Z, Thorpe M, Pejler G, Hellman L. Quantitative in-depth analysis of the mouse mast cell transcriptome reveals organ-specific mast cell heterogeneity. Cells (2020) 9:211. doi: 10.3390/CELLS9010211
88. St. John AL, Rathore APS, Ginhoux F. New perspectives on the origins and heterogeneity of mast cells. Nat Rev Immunol (2022), 1–14. doi: 10.1038/s41577-022-00731-2
89. Li Z, Liu S, Xu J, Zhang X, Han D, Liu J, et al. Adult connective tissue-resident mast cells originate from late erythro-myeloid progenitors. Immunity (2018) 49:640–53.e5. doi: 10.1016/j.immuni.2018.09.023
90. Gentek R, Ghigo C, Hoeffel G, Bulle MJ, Msallam R, Gautier G, et al. Hemogenic endothelial fate mapping reveals dual developmental origin of mast cells. Immunity (2018) 48:1160–71. doi: 10.1016/j.immuni.2018.04.025
91. Wang X, Yang L, Wang YC, Xu ZR, Feng Y, Zhang J, et al. Comparative analysis of cell lineage differentiation during hepatogenesis in humans and mice at the single-cell transcriptome level. Cell Res (2020) 30:1109–26. doi: 10.1038/s41422-020-0378-6
92. Grootens J, Ungerstedt JS, Nilsson G, Dahlin JS. Deciphering the differentiation trajectory from hematopoietic stem cells to mast cells. Blood Adv (2018) 2:2273–81. doi: 10.1182/bloodadvances.2018019539
93. Weitzmann A, Naumann R, Dudeck A, Zerjatke T, Gerbaulet A, Roers A. Mast cells occupy stable clonal territories in adult steady-state skin. J Invest Dermatol (2020) 140:2433–41. doi: 10.1016/j.jid.2020.03.963
94. Albert-Bayo M, Paracuellos I, González-Castro AM, Rodríguez-Urrutia A, Rodríguez-Lagunas MJ, Alonso-Cotoner C, et al. Intestinal mucosal mast cells: Key modulators of barrier function and homeostasis. Cells (2019) 8:135. doi: 10.3390/CELLS8020135
95. Collington SJ, Hallgren J, Pease JE, Jones TG, Rollins BJ, Westwick J, et al. The role of the CCL2/CCR2 axis in mouse mast cell migration In vitro and In vivo. J Immunol (2010) 184:6114–23. doi: 10.4049/JIMMUNOL.0904177
96. Gurish MF, Tao H, Abonia JP, Arya A, Friend DS, Parker CM, et al. Intestinal mast cell progenitors require CD49dbeta7 (Alpha4beta7 integrin) for tissue-specific homing. J Exp Med (2001) 194:1243–52. doi: 10.1084/JEM.194.9.1243
97. Zarnegar B, Mendez-Enriquez E, Westin A, Söderberg C, Dahlin JS, Grönvik KO, et al. Influenza infection in mice induces accumulation of lung mast cells through the recruitment and maturation of mast cell progenitors. Front Immunol (2017) 8:310. doi: 10.3389/FIMMU.2017.00310
98. Nakano T, Sonoda T, Hayashi C, Yamatodani A, Kanayama Y, Yamamura T, et al. Fate of bone marrow-derived cultured mast cells after intracutaneous, intraperitoneal, and intravenous transfer into genetically mast cell-deficient W/Wv mice. evidence that cultured mast cells can give rise to both connective tissue type and mucosal mast cells. J Exp Med (1985) 162:1025–43. doi: 10.1084/JEM.162.3.1025
99. Frossi B, Mion F, Sibilano R, Danelli L, Pucillo CEM. Is it time for a new classification of mast cells? what do we know about mast cell heterogeneity? Immunol Rev (2018) 282:35–46. doi: 10.1111/IMR.12636
100. Füreder W, Agis H, Willheim M, Bankl HC, Maier U, Kishi K, et al. Differential expression of complement receptors on human basophils and mast cells. evidence for mast cell heterogeneity and CD88/C5aR expression on skin mast cells. J Immunol (1995) 155:3152–60. doi: 10.4049/issn.1550-6606
101. Oskeritzian CA, Zhao W, Min HK, Xia HZ, Pozez A, Kiev J, et al. Surface CD88 functionally distinguishes the MCTC from the MCT type of human lung mast cell. J Allergy Clin Immunol (2005) 115:1162–8. doi: 10.1016/j.jaci.2005.02.022
102. Plum T, Wang X, Rettel M, Krijgsveld J, Feyerabend TB, Rodewald HR. Human mast cell proteome reveals unique lineage, putative functions, and structural basis for cell ablation. Immunity (2020) 52:404–16.e5. doi: 10.1016/j.immuni.2020.01.012
103. Ben-Baruch Morgenstern N, Ballaban AY, Wen T, Shoda T, Caldwell JM, Kliewer K, et al. Single-cell RNA sequencing of mast cells in eosinophilic esophagitis reveals heterogeneity, local proliferation, and activation that persists in remission. J Allergy Clin Immunol (2022) 149:2026–77. doi: 10.1016/J.JACI.2022.02.025
104. Dwyer DF, Ordovas-Montanes J, Allon SJ, Buchheit KM, Vukovic M, Derakhshan T, et al. Human airway mast cells proliferate and acquire distinct inflammation-driven phenotypes during type 2 inflammation. Sci Immunol (2021) 6:eabb7221. doi: 10.1126/sciimmunol.abb7221
105. Rönnberg E, Boey DZH, Ravindran A, Säfholm J, Orre AC, Al-Ameri M, et al. Immunoprofiling reveals novel mast cell receptors and the continuous nature of human lung mast cell heterogeneity. Front Immunol (2022) 12:804812. doi: 10.3389/FIMMU.2021.804812
106. Bischoff SC, Krämer S. Human mast cells, bacteria, and intestinal immunity. Immunol Rev (2007) 217:329–37. doi: 10.1111/J.1600-065X.2007.00523.X
107. Bischoff SC. Mast cells in gastrointestinal disorders. Eur J Pharmacol (2016) 778:139–45. doi: 10.1016/J.EJPHAR.2016.02.018
108. Abonia JP, Austen KF, Rollins BJ, Joshi SK, Flavell RA, Kuziel WA, et al. Constitutive homing of mast cell progenitors to the intestine depends on autologous expression of the chemokine receptor CXCR2. Blood (2005) 105:4308. doi: 10.1182/BLOOD-2004-09-3578
109. Kunii J, Takahashi K, Kasakura K, Tsuda M, Nakano K, Hosono A, et al. Commensal bacteria promote migration of mast cells into the intestine. Immunobiology (2011) 216:692–7. doi: 10.1016/J.IMBIO.2010.10.007
110. Lorentz A, Schuppan D, Gebert A, Manns MP, Bischoff SC. Regulatory effects of stem cell factor and interleukin-4 on adhesion of human mast cells to extracellular matrix proteins. Blood (2002) 99:966–72. doi: 10.1182/BLOOD.V99.3.966
111. Baumann A, Feilhauer K, Bischoff SC, Froy O, Lorentz A. IgE-dependent activation of human mast cells and fMLP-mediated activation of human eosinophils is controlled by the circadian clock. Mol Immunol (2015) 64:76–81. doi: 10.1016/J.MOLIMM.2014.10.026
112. Karhausen J, Qing M, Gibson A, Moeser AJ, Griefingholt H, Hale LP, et al. Intestinal mast cells mediate gut injury and systemic inflammation in a rat model of deep hypothermic circulatory arrest. Crit Care Med (2013) 41:e200. doi: 10.1097/CCM.0B013E31827CAC7A
113. McDermott JR, Bartram RE, Knight PA, Miller HRP, Garrod DR, Grencis RK. Mast cells disrupt epithelial barrier function during enteric nematode infection. Proc Natl Acad Sci USA (2003) 100:7761. doi: 10.1073/PNAS.1231488100
114. Shin K, Watts GFM, Oettgen HC, Friend DS, Pemberton AD, Gurish MF, et al. Mouse mast cell tryptase mMCP-6 is a critical link between adaptive and innate immunity in the chronic phase of trichinella spiralis infection. J Immunol (2008) 180:4885. doi: 10.4049/JIMMUNOL.180.7.4885
115. Roy A, Sawesi O, Pettersson U, Dagälv A, Kjellén L, Lundén A, et al. Serglycin proteoglycans limit enteropathy in trichinella spiralis-infected mice. BMC Immunol (2016) 17:1–13. doi: 10.1186/S12865-016-0155-Y
116. Brink N, Szamel M, Young AR, Wittern KP, Bergemann J. Comparative quantification of IL-1β, IL-10, IL-10r, tnfα and IL-7 mRNA levels in UV-irradiated human skin In vivo. Inflammation Res (2014) 49:290–6. doi: 10.1007/PL00000209
117. Leyva-Castillo JM, Das M, Artru E, Yoon J, Galand C, Geha RS. Mast cell–derived IL-13 downregulates IL-12 production by skin dendritic cells to inhibit the TH1 cell response to cutaneous antigen exposure. J Allergy Clin Immunol (2021) 147:2305–15.e3. doi: 10.1016/J.JACI.2020.11.036
118. Faulkner H, Humphreys N, Renauld JC, Van Snick J, Grencis R. Interleukin-9 is involved in host protective immunity to intestinal nematode infection. Eur J Immunol (1997) 27:2536–40. doi: 10.1002/eji.1830271011
119. Faulkner H, Renauld JC, Van Snick J, Grencis RK. Interleukin-9 enhances resistance to the intestinal nematode Trichuris muris. Infect Immun (1998) 66:3832–40. doi: 10.1128/IAI.66.8.3832-3840.1998
120. Hültner L, Kölsch S, Stassen M, Kaspers U, Kremer JP, Mailhammer R, et al. In activated mast cells, IL-1 up-regulates the production of several Th2-related cytokines including IL-9. J Immunol (2000) 164:5556–63. doi: 10.4049/jimmunol.164.11.5556
121. Stassen M, Arnold M, Hültner L, Müller C, Neudörfl C, Reineke T, et al. Murine bone marrow-derived mast cells as potent producers of IL-9: Costimulatory function of IL-10 and kit ligand in the presence of IL-1. J Immunol (2000) 164:5549–55. doi: 10.4049/jimmunol.164.11.5549
122. Theoharides TC, Kempuraj D, Kourelis T, Manola A. Human mast cells stimulate activated T cells: Implications for multiple sclerosis. Ann N Y Acad Sci (2008) 1144:74–82. doi: 10.1196/ANNALS.1418.029
123. Bodduluri SR, Mathis S, Maturu P, Krishnan E, Satpathy SR, Chilton PM, et al. Mast cell–dependent CD8+ T-cell recruitment mediates immune surveillance of intestinal tumors in ApcMin/þ mice. Cancer Immunol Res (2018) 6:332–47. doi: 10.1158/2326-6066.CIR-17-0424/470586
124. Cowen T, Trigg P, Eady RAJ. Distribution of mast cells in human dermis: Development of a mapping technique. Br J Dermatol (1979) 100:635–40. doi: 10.1111/J.1365-2133.1979.TB08066.X
125. Liu J, Harberts E, Tammaro A, Girardi N, Filler RB, Fishelevich R, et al. IL-9 regulates allergen-specific Th1 responses in allergic contact dermatitis. J Invest Dermatol (2014) 134:1903–11. doi: 10.1038/JID.2014.61
126. Wang Z, MacLeod DT, Di Nardo A. Commensal bacteria lipoteichoic acid increases skin mast cell antimicrobial activity against vaccinia viruses. J Immunol (2012) 189:1551–8. doi: 10.4049/JIMMUNOL.1200471
127. Wang Z, Mascarenhas N, Eckmann L, Miyamoto Y, Sun X, Kawakami T, et al. Skin microbiome promotes mast cell maturation by triggering stem cell factor production in keratinocytes. J Allergy Clin Immunol (2017) 139:1205–16.e6. doi: 10.1016/J.JACI.2016.09.019
128. Columbo M, Bochner BS. Human skin mast cells adhere to vitronectin via the αvβ3 integrin receptor (CD51/CD61). J Allergy Clin Immunol (2001) 107:554. doi: 10.1067/MAI.2001.113238
129. Kurashima Y, Amiya T, Fujisawa K, Shibata N, Suzuki Y, Kogure Y, et al. The enzyme Cyp26b1 mediates inhibition of mast cell activation by fibroblasts to maintain skin-barrier homeostasis. Immunity (2014) 40:530–41. doi: 10.1016/J.IMMUNI.2014.01.014
130. Okayama Y, Ono Y, Nakazawa T, Church MK, Mori M. Human skin mast cells produce TNF-alpha by substance p. Int Arch Allergy Immunol (1998) 117:48–51. doi: 10.1159/000053571
131. Furuta T, Imajo-Ohmi S, Fukuda H, Kano S, Miyake K, Watanabe N. Mast cell-mediated immune responses through IgE antibody and toll-like receptor 4 by malarial peroxiredoxin. Eur J Immunol (2008) 38:1341–50. doi: 10.1002/EJI.200738059
132. Gibbs BF, Wierecky J, Welker P, Henz BM, Wolff HH, Grabbe J. Human skin mast cells rapidly release preformed and newly generated TNF-alpha and IL-8 following stimulation with anti-IgE and other secretagogues. Exp Dermatol (2001) 10:312–20. doi: 10.1034/j.1600-0625.2001.100503.x
133. Wershil BK, Wang ZS, Gordon JR, Galli SJ. Recruitment of neutrophils during IgE-dependent cutaneous late phase reactions in the mouse is mast cell-dependent. partial inhibition of the reaction with antiserum against tumor necrosis factor-alpha. J Clin Invest (1991) 87:446–53. doi: 10.1172/jci115016
134. Dudeck J, Ghouse SM, Lehmann CH, Hoppe A, Schubert N, Nedospasov SA, et al. Mast-Cell-Derived TNF amplifies CD8(+) dendritic cell functionality and CD8(+) T cell priming. Cell Rep (2015) 13:399–411. doi: 10.1016/j.celrep.2015.08.078
135. McLachlan JB, Hart JP, Pizzo SV, Shelburne CP, Staats HF, Gunn MD, et al. Mast cell-derived tumor necrosis factor induces hypertrophy of draining lymph nodes during infection. Nat Immunol (2003) 4:1199–205. doi: 10.1038/ni1005
136. Mantri CK, St. John AL. Immune synapses between mast cells and γδ T cells limit viral infection. J Clin Invest (2019) 129:1094–108. doi: 10.1172/JCI122530
137. Hackler Y, Siebenhaar F, Löhning M, Maurer M, Muñoz M. Mast cells modulate antigen-specific CD8+ T cell activation during LCMV infection. Front Immunol (2021) 12:688347. doi: 10.3389/FIMMU.2021.688347
138. Aoki R, Kawamura T, Goshima F, Ogawa Y, Nakae S, Nakao A, et al. Mast cells play a key role in host defense against herpes simplex virus infection through TNF-α and IL-6 production. J Invest Dermatol (2013) 133:2170–9. doi: 10.1038/JID.2013.150
139. Agier J, Pastwińska J, Brzezińska-Błaszczyk E. An overview of mast cell pattern recognition receptors. Inflammation Res (2018) 67:737–46. doi: 10.1007/S00011-018-1164-5
140. Kothari R, Dong X. Scratching the surface of itch receptors. Trends Pharmacol Sci (2022) 43:168–70. doi: 10.1016/J.TIPS.2021.12.005
141. McNeil BD, Pundir P, Meeker S, Han L, Undem BJ, Kulka M, et al. Identification of a mast-Cell-Specific receptor crucial for pseudo-allergic drug reactions. Nature (2015) 519:237–41. doi: 10.1038/nature14022
142. Green DP, Limjunyawong N, Gour N, Pundir P, Dong X. A mast-Cell-Specific receptor mediates neurogenic inflammation and pain. Neuron (2019) 101:412–20.e3. doi: 10.1016/j.neuron.2019.01.012
143. Nakamura Y, Kambe N, Saito M, Nishikomori R, Nishikomiri R, Kim Y-G, et al. Mast cells mediate neutrophil recruitment and vascular leakage through the NLRP3 inflammasome in histamine-independent urticaria. J Exp Med (2009) 206:1037–46. doi: 10.1084/jem.20082179
144. Nakamura Y, Franchi L, Kambe N, Meng G, Strober W, Núñez G. Critical role for mast cells in interleukin-1β-Driven skin inflammation associated with an activating mutation in the Nlrp3 protein. Immunity (2012) 37:85–95. doi: 10.1016/J.IMMUNI.2012.04.013
145. Reber LL, Sibilano R, Starkl P, Roers A, Grimbaldeston MA, Tsai M, et al. Imaging protective mast cells in living mice during severe contact hypersensitivity. JCI Insight (2017) 2:1–13. doi: 10.1172/jci.insight.92900
Keywords: Mast cells, chemotaxis, cell heterogeneity, chemokines, cytokines, MRGPRX2
Citation: West PW and Bulfone-Paus S (2022) Mast cell tissue heterogeneity and specificity of immune cell recruitment. Front. Immunol. 13:932090. doi: 10.3389/fimmu.2022.932090
Received: 29 April 2022; Accepted: 30 June 2022;
Published: 29 July 2022.
Edited by:
Satoshi Tanaka, Kyoto Pharmaceutical University, JapanReviewed by:
Hydar Ali, University of Pennsylvania, United StatesCopyright © 2022 West and Bulfone-Paus. This is an open-access article distributed under the terms of the Creative Commons Attribution License (CC BY). The use, distribution or reproduction in other forums is permitted, provided the original author(s) and the copyright owner(s) are credited and that the original publication in this journal is cited, in accordance with accepted academic practice. No use, distribution or reproduction is permitted which does not comply with these terms.
*Correspondence: Silvia Bulfone-Paus, c2lsdmlhLmJ1bGZvbmUtcGF1c0BtYW5jaGVzdGVyLmFjLnVr