- 1Department of Surgery, Duke University Medical Center, Durham, NC, United States
- 2Department of Molecular Genetics & Microbiology, Duke University School of Medicine, Durham, NC, United States
- 3Department of Biomedical Engineering, Duke University, Durham, NC, United States
- 4Division of Cardiovascular and Thoracic Surgery, Duke University Medical Center, Durham, NC, United States
Lung transplantation is the definitive therapy for patients living with end-stage lung disease. Despite significant progress made in the field, graft survival remains the lowest of all solid organ transplants. Additionally, the lung has among the lowest of organ utilization rates—among eligible donors, only 22% of lungs from multi-organ donors were transplanted in 2019. Novel strategies are needed to rehabilitate marginal organs and improve graft survival. Gene therapy is one promising strategy in optimizing donor allografts. Over-expression or inhibition of specific genes can be achieved to target various pathways of graft injury, including ischemic-reperfusion injuries, humoral or cellular rejection, and chronic lung allograft dysfunction. Experiments in animal models have historically utilized adenovirus-based vectors and the majority of literature in lung transplantation has focused on overexpression of IL-10. Although several strategies were shown to prevent rejection and prolong graft survival in preclinical models, none have led to clinical translation. The past decade has seen a renaissance in the field of gene therapy and two AAV-based in vivo gene therapies are now FDA-approved for clinical use. Concurrently, normothermic ex vivo machine perfusion technology has emerged as an alternative to traditional static cold storage. This preservation method keeps organs physiologically active during storage and thus potentially offers a platform for gene therapy. This review will explore the advantages and disadvantages of various gene therapy modalities, review various candidate genes implicated in various stages of allograft injury and summarize the recent efforts in optimizing donor lungs using gene therapy.
Introduction
Lung transplantation offers the only hope of curative therapy for patients living with end stage lung disease. Since the initial series of successful lung transplants performed in the 80s, both transplant outcomes and volume have improved substantially. The number of annual lung transplants performed has increased from 69 in 1988 to 4567 in 2016 worldwide (1). In the US, one-year survival following lung transplant is now close to 90%, and one in three recipients survive to ten years following transplant (2).
Despite these favorable trends, many challenges remain. In 2019, the donation rate for lung was 20.8 eligible donors per 100 eligible deaths (3), much lower than those of other solid organs. Sixty-four percent of donor lungs are not recovered due to a variety of reasons, many stemming from the inherent exposure and susceptibility to insults, such as infection, aspiration, trauma, smoking, and ventilator-associated injury. An additional 6.4% of donor lungs were recovered but not transplanted (2). Furthermore, survival after lung transplant remains the lowest of all solid organ transplants. Three major immune-mediated mechanisms account for most lung allograft failures: 1. Ischemic-reperfusion injury (IRI), with subsequent activation of the innate immune system, which is associated with primary graft dysfunction (PGD); 2. Cell-mediated, and to a lesser extent, antibody-mediated adaptive immune activation, which can cause acute rejection; and 3. Chronic graft dysfunction, a process that is poorly understood and thought to be related to inflammation and chronic airway remodeling.
Novel therapies that may maximize donor lung utilization and reduce immune-mediated graft failures are needed; gene therapy has emerged as a potential strategy for organ rehabilitation and optimization. The past decade has seen significant progress in gene therapy development as several products are now approved for clinical use (4). Concurrently, novel organ preservation strategies in normothermic Ex Vivo Lung Perfusion (EVLP) are gaining popularity after promising results in clinical trials (5, 6). EVLP techniques maintain lung allografts in a physiologically active and ventilated state, and thus offer an optimal platform for delivery of interventions such as gene therapy. In this review, we present progress in the use of gene therapy in lung transplantation, and highlight some important considerations related to the routes of delivery, vector choice and potential target genes.
Gene Therapy Approaches
The core of gene therapy is the use of exogenous DNA in promoting, modifying, or silencing protein expression. Gene therapy initially gained traction due to its potential to offer a durable cure for many inherited monogenic disorders with a single treatment (7). Although the field’s progress has ebbed and flowed, the past decade has witnessed a renaissance in gene therapy with several products receiving FDA approval following generations of efforts, including two AAV-based in vivo therapies: Luxturna® for Leber’s Congenital Amaurosis and Zolgensma® for spinal muscular atrophy (8). There is ongoing investigation to utilizing genetic therapies in other fields of medicine, including lung transplantation.
Several features unique to the field of transplantation are advantageous for genetic-based therapeutics (Figure 1). First, transplant patients are immunosuppressed, and it is hypothesized that innate immunity and subsequent immune activation against gene therapy vectors or foreign protein products can reduce therapeutic efficacy (9). Studies in both clinical and experimental animal models have demonstrated that immunosuppression facilitates gene therapy delivery and enhances gene expression (10–13). Second, considerable research in vector engineering has focused on optimizing tissue tropism, as most in vivo gene therapies are delivered systemically (14, 15). In transplantation however, organs are preserved ex vivo following procurement, which provides a unique opportunity for isolated organ-targeted treatment; this period of isolation could potentially enable utilization of higher vector doses and reduce the incidence of systemic adverse effects, such as those observed in early gene therapy clinical trials (16).
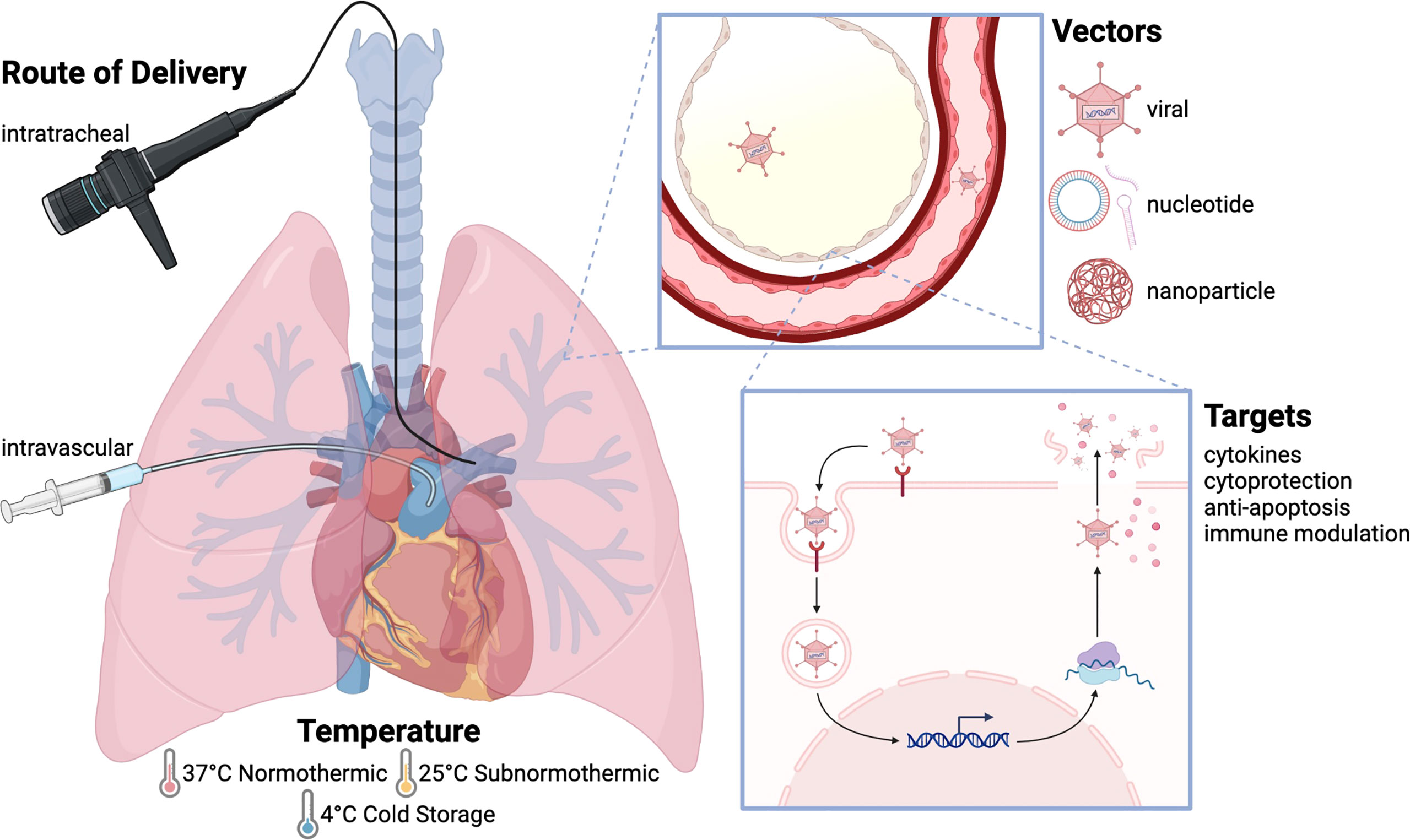
Figure 1 Approaches to gene therapy in lung transplantation. The basic principle of gene therapy is that gene sequences are delivered to target tissues using a vector, which is then taken up by these tissues allowing for gene expression. Gene therapy can be delivered using multiple routes, temperatures, and vectors, with numerous gene targets and uses. Intratracheal delivery is most commonly used, as it allows for localized treatment without systemic effects, although intravascular delivery has been used to target the vascular endothelium. Gene therapy is often delivered at physiologic temperature (37°C), although studies have also been performed at subnormothermic temperature (25°C) and cold storage temperature (4°C). Adenovirus is the most widely employed vector. Other viral vectors include AAV, lentivirus, and sendai virus. Nucleotide-based vectors include naked plasmid, shRNA, and siRNA, and nanoparticles have also been used to deliver gene products. The transfected genes include anti-inflammatory cytokines (IL-10, TGF-β), pro-inflammatory cytokines (IL-1, TNF-α), cytoprotective genes (NOS), anti-apoptotic molecules (caspase-3, FasL), and immune modulatory molecules (CTLA4-Ig, IDO).
Temperature of Delivery
Although administration of gene therapy to donor animals has generally been successful (11), it is not clinically relevant, as the treatment of human organ donors would raise significant ethical concerns. Thus, an ideal window for gene delivery to donor organs is between donor cross-clamp and reperfusion in the recipient. In lung transplantation, most grafts are preserved at 4°C or less, while clinical EVLP protocols to date preserve lung grafts at 37°C. In recent years, others have proposed alternative preservation strategies that may reduce inflammation where lungs were maintained with EVLP at sub-normothermic temperatures between 10 to 25°C (17–19).
Studies have demonstrated that gene expression can be detected in the target organ following reperfusion when gene therapies are delivered at different temperatures, ranging from 4°C to 37°C, across multiple organ systems, and in a variety of models (20–26). Thus, even though gene expression relies on substantial cellular machinery for transcription and translation at physiological temperature, there is evidence suggesting that gene delivery can be achieved during cold storage with subsequent transgene expression. Delivery of genes during static cold storage could offer an opportunity for translation to the clinical setting, as most organs are currently stored on ice.
Conceptually, preservation of the lung allograft closer to physiological temperature may improve vector uptake and gene expression as the graft is metabolically more active. After delivery of adenoviral vector encoding human IL-10 (hIL-10), Cypel et al. found increased hIL-10 concentrations in lung tissue in porcine lungs prior to reperfusion only when maintained on EVLP but not when kept under cold static conditions (23). Cassivi et al. found minimal transgene expression in donor lungs that received gene therapy during cold storage when comparing target protein expression at reperfusion between therapy delivery to the donor animal six hours before procurement, or after procurement during cold storage (27).
Ultimately, the optimal donor organ storage conditions for gene delivery is likely to be determined by the target gene of interest. For example, if the target is a mediator of IRI and a high concentration of the desired protein product would be needed at the time of reperfusion, then gene therapy during EVLP could be desirable in order to maintain metabolic activity for 6-8 hours prior to implantation. In contrast, gene delivery during cold storage could be sufficiently effective if targeting a later event such as acute or chronic rejection.
Routes of Delivery
Gene transfer to the lung allograft is generally achieved via the airway or intravascularly. Intra-tracheal delivery gives direct access to lung epithelial cells and, since the airway compartment is relatively isolated, the risk of systemic spread and off target effects upon reperfusion is minimized. In fact, most studies on lung-targeting gene therapy for the treatment of cystic fibrosis and α1-antitrypsin deficiency favor this approach (14, 28–30). Unsurprisingly, most studies on gene therapy in lung transplant have also elected to use the airway delivery approach (11, 23, 27, 31–33), because this route of delivery produces significantly greater gene expression in the lung overall, predominantly in respiratory epithelium, compared to other approaches (34, 35). However, one concern related to gene delivery via the airway is that it poorly targets the vascular endothelium (36). Vascular endothelium is the interface between the donor organ and the recipient circulation, and thus plays an important role in IRI and other immune mediated injuries.
As a result, investigators have turned to the intravenous delivery route, hoping to achieve better gene transfer in vascular endothelial cells. Thus, therapy is typically delivered via the pulmonary artery ex vivo or systemically in vivo. Early studies have demonstrated the feasibility of intravascular gene transfer to lungs in vivo or ex vivo using adenoviral vectors in rodent and large animal models (36–39). Target genes are detected in vascular endothelium and sometimes airway epithelium, but not alveolar epithelium, which is more readily transfected during trans-airway delivery (36, 40). In most cases, the efficiency of gene delivery was low, and off target delivery was detected in remote sites even when the viral vectors were injected ex vivo (34, 39).
Lastly, the lung is dually perfused by the pulmonary artery and the bronchial arteries. The bronchial artery receives about 1% of the cardiac output and provides systemic blood supply to the trachea and bronchi (41). Therefore, the bronchial artery can theoretically be utilized for gene delivery. There has been some speculation that gene delivery via the bronchial artery may improve targeting to the bronchial airways (36, 42), but further research is needed to demonstrate the efficacy of gene delivery through the bronchial artery.
Choice of Vector
Two general approaches exist to transfer genetic material into a target cell—viral and non-viral. Both have been applied in gene therapy studies in lung transplant (35, 43). The choice of vector is important, especially given the vector-associated safety issues in early gene therapy clinical trials (44). In general terms, viral vectors transduce the genes efficiently but can pose some safety concerns, while non-viral vectors tend to be safe and less immunogenic but are less efficient at gene transfer. Recent years have seen a shift in the field of gene therapy in terms of the choice of vector: the field has turned away from adenoviral-based vectors towards Adeno-associated virus (AAV) – based vectors for in vivo gene delivery. Lentiviral vectors are currently the leading platform for ex vivo gene modification in primary cell lines such as T cells (4). Non-viral vectors have also emerged, as the first siRNA-based drug Onpattro® delivered using lipid nanoparticle (LNP) technology was approved for the treatment of hereditary ATTR amyloidosis (45).
Adenoviral Vector
Adenovirus (Ad) is one of the first extensively studied vectors and the first effective in vivo gene therapy tested clinically (46). Early experiments in gene therapy for lung transplant and other solid organs have relied heavily on adenovirus-based vectors (20, 23, 32, 33, 35, 47). Ad vector has several advantages: 1. its ability to infect both non-dividing and dividing cells with high transduction efficacy; 2. the availability of scalable production systems; 3. a large insertional capacity for the target gene, around 7-8 kb; and 4. broad tissue tropism.
One major issue associated with Ad vector is its inherent high immunogenicity—unrelated to the transgene expression. A strong innate immune response against the capsid protein is well documented in animal models (48) and may trigger significant cytokine storm in susceptible individuals (44). Subsequently, robust host adaptive immune responses to the transgene product reduce the longevity of gene transfer. In a rat liver transplant model, Olthoff et al. successfully delivered CTLA4Ig to the donor liver using an Ad vector, although minimal levels of CTLA4Ig were observed beyond 20 days post-transplant (20). In a porcine lung transplant model, Machuca et al. delivered Ad vector encoding hIL-10 during EVLP. Although they were able to detect hIL-10 in the perfusate while on EVLP as early as 12 hours following Ad vector administration, serum levels of IL-10 post-transplant decrease drastically within the first week (33). In humans, the effective expression of Ad vector in vivo peaks at 1 week and is generally limited to about 2 weeks (49). Related to Ad’s immunogenic nature, the efficacy of repeat administration of Ad vectors is limited by the development of neutralizing antibodies directed against the vector following initial delivery. Additionally, pre-existing immunity against various Adenovirus serotypes exists population-wide, thus limiting the wide adoption of Ad vector (50).
As a result, Ad vector is increasingly being utilized for the treatment of cancer or vaccines, where a strong immune response is desired. In transplant, adenovirus vectors may remain of interest if rapid onset and short-term gene expression is desired (49).
Adeno-Associated Viral Vector
AAV is a nonpathogenic virus that requires co-infection with a helper virus to replicate in host cells. Although it can infect both dividing and growth-arrested cells, AAV infection causes no known disease in humans. Recombinant AAVs contain no viral proteins and cause less inflammatory response compared to Ad vector (15, 51, 52). Perhaps because of its excellent safety profile (53), AAV is currently the leading platform for gene delivery for the treatment of a variety of human diseases. Hundreds of phase I/II/III trials have been conducted to date using recombinant AAV vectors, with several AAV vector-based gene therapy products approved in Europe and USA (15, 54).
Additionally, AAV vectors are highly versatile with wide-ranging tissue tropism among serotypes. AAV vector platforms have been developed to target the central nervous system (CNS), eyes, liver, lung, heart, and muscle in a variety of preclinical and clinical models (50). Following delivery, AAV vectors typically persist in host cells in a stable episomal form and rarely can integrate at the site of double-strand breaks. (55, 56).
Because AAV is a single-stranded DNA (ss-DNA) virus, the ssDNA encoding the target gene needs to be converted into a double-stranded DNA (dsDNA) before transcription. As a result, transgene expression only rises to detectable levels in 1-2 weeks. In comparison, gene expression following Ad vector delivery becomes detectable within several hours. One alternative is the use of a self-complementary design, in which the packaged DNA product within the AAV vector undergoes intramolecular base pairing to form dsDNA (57). This way, transcription can begin as soon as the DNA reaches the nucleus. One disadvantage of the self-complementary design is that it further limits the packaging capacity of AAV to 3.3 kb (58).
In contrast to Ad vector, AAV vectors have been shown to achieve long-term transgene expression in experimental models and clinically. In a canine model of Hemophilia A, animals treated with AAV gene therapy have sustained factor VIII activity 10 years from the initial treatment. Clinical trials of AAV gene therapy in the liver have also demonstrated long-term efficacy (59, 60).
Of note, there are several important disadvantages associated with AAV vectors. Firstly, the target gene delivered by AAV is generally limited to less than 5 kb. However, the use of dual overlapping vector strategies could circumvent this limit (61). Secondly, transgene expression following AAV delivery generally does not reach clinically relevant levels until 1-2 weeks. Even with the self-complementary design, expression remains minimal within the first 24-48 hours. Such a timeline makes AAV a less-than-ideal vector candidate if IRI is the primary target of intervention.
To date, few AAV gene therapy studies have been published in lung transplant models. A recent study described the delivery of reporter gene using AAV8 during hypothermic oxygenated machine perfusion (HOPE) in a liver transplant model (62), and our group has similarly reported AAV9 delivery of a reporter gene to donor liver and lung during static cold storage (25, 26). Overall AAV has demonstrated its versatility and safety profiles in clinical trials and is thus most readily translatable for clinical uses. However, AAV’s utilization in targeting IRI specifically is likely limited due to its delayed action.
Lentiviral Vector
Lentiviral vectors have been increasingly used for gene therapy due to a number of unique characteristics: 1. They transduce non-dividing cells and offer efficient and durable gene expression (63, 64). This feature is appealing for lung applications given than 95% of all lung cells are quiescent (65). 2. Lentiviral vectors have a large packaging capacity (up to 9 kb). 3. They trigger minimal inflammation and immunogenic responses—a major advantage compared to adenoviral vectors (63). Their low immunogenicity profile allows for repeated doses with minimal decrease in efficacy (66). 4. Lentiviral vectors can be pseudotyped by altering their surface receptor recognition elements to target various cell types (67).
Lentiviral vectors have been used successfully to transfect lungs in murine models (65, 68, 69). In mice, airway delivery of lentivirus-encoding luciferase led to robust luciferase activity that was stable over 15 months, highlighting the potential of stable long-term transgene expression using lentiviral vectors in lungs. Of note, gene transduction occurred mainly in alveolar macrophages (70). Furthermore, Cooney et al. reported CFTR delivery via aerosolized FIV-based lentiviral gene therapy in a porcine cystic fibrosis model (71) with evidence of functional CFTR 2 weeks following gene therapy, demonstrating the efficacy of lentiviral vectors in a large-animal model.
Lentiviral-based gene therapy has also been tested in lung transplantation models. The Toronto group demonstrated in a mouse model that lentivirus-mediated trans-airway gene transfer to the donor lung results in persistent transgene expression up to 6 months in a syngeneic model (72). Of note, the efficacy of gene delivery to the donor lung was comparable whether the vector was given to the donor pretransplant or to the recipient posttransplant. A later study by the same group demonstrated the efficacy of a combination of ex vivo and in vivo lentiviral vector administration to the recipient lung (73).
Despite these advances, several concerns limit the application of lentiviral vectors to gene therapy. First, there is a risk of generating a replication-competent virus. Several modifications have been made to lentiviral vectors, including splitting the viral genome into separate plasmids as well as removing accessory virulence factors (64, 74). Second, there is a potential to cause insertional mutagenesis at the site of vector integration, leading to malignant transformation in the treated cells (75, 76). Lastly, large scale production of lentivirus for clinical use can be challenging (77). As such, optimization in pre-clinical models remains essential prior to utilizing lentiviral vectors in transplantation and machine perfusion.
Non-Viral
Given the intrinsic limitations (e.g. amount of genetic material deliverable, differential cell transfection) and safety considerations (e.g. infectious, oncologic) of viral vectors, non-viral based gene therapies have been explored. Naked delivery, the delivery of DNA without delivery agents, has shown overall mixed results with both plasmid-based therapy (78–80) and siRNA-based gene therapy (81, 82). The major limitations of these strategies remain poor stability and rapid degradation (45, 83), limited uptake of various types of cells in the lung (84), and possible off-target effects of unformulated siRNA (85).
Several chemical carriers have thus been developed to overcome those limitations including lipid-, peptide-, polymer-, and nanoparticle-based carriers (8, 86–88). Of those approaches, nanoparticle-based gene therapy is most promising with currently more than ten FDA-approved lipid nanoparticle (LNP) drug delivery systems (45). LNPs readily achieve delivery of large amounts of genetic information, are easy to scale up, and cause minimal inflammation and immune responses (89, 90). Importantly, several groups recently reported generation of lung-targeting LNPs (91–93), a major step in developing lung-specific gene therapies.
Ex vivo Lung Perfusion Platform
The past decade has witnessed a strong push for the expansion of the donor pool. This is driven primarily by increased utilization of organs that have been thought to confer an increased risk of graft failure. Transplant centers are increasingly using donation after cardiac death (DCD) donor lungs and adopting a more liberal approach to donor lung acceptance, including using long cold ischemic time organs and organs from donors over 60-years-old, Hepatitis C Virus (HCV) positive donors, and donors with a significant smoking history (94–97).
Coincidentally, the adoption of novel preservation technology, i.e. ex vivo lung perfusion (EVLP), has allowed improved evaluation of donor lungs under physiological conditions, which is particularly useful for traditionally marginal organs. In fact, a recent multicenter trial in the US described a portable EVLP device that has led to an 87% donor lung utilization rate, four times the rate observed when lungs are preserved on cold ice (98). In addition to its capacity to evaluate lung allografts, the EVLP platform also provides a window to administer therapies that recondition donor lungs and minimize injury following reperfusion. The Toronto group has previously examined the efficacy of high dose antimicrobials on bacterial and fungal infections (99), ultraviolet C light on controlling hepatitis C viral concentration (100, 101), and Rituximab on depletion of B cells latently infected with Epstein Barr virus (102) in discarded human lungs. Others have investigated various anti-inflammatory compounds in animal models (103–105).
EVLP provides an optimal platform for the delivery of gene therapy. Airway and vasculature are both accessible while on EVLP and thus available for vector administration. As the organs are maintained under physiologically active conditions, vector uptake and transgene expression can readily occur. Additionally, any downstream effects or potential side effects associated with gene therapy can be monitored. Aside from the Ad vector, the initiation of transgene expression following gene therapy administration can take days. While most clinical EVLP protocols limit organ preservation to 12 hours, it may be possible to prolong this window of intervention using EVLP with additional external nutrition and dialysis support to the donor graft (106, 107), thus allowing a longer window for transgene expression.
Several aspects of EVLP merit special consideration regarding the delivery of gene therapy. First, various EVLP devices and protocols exist, but all maintain the organ at physiological temperature. As a result, most studies on gene therapy during EVLP are done under normothermic conditions. However, EVLP performed at subnormothermic temperatures may be useful in delivering gene therapy. Our group recently reported less inflammation to the donor graft when EVLP was performed at 25 °C compared to 37°C in a rat model (19). A similar result was also noted by Arni et al. (108). Gene delivery during subnormothermic EVLP has not yet been attempted, and more research is needed to determine the optimal temperature for delivery and subsequent transgene expression. Secondly, several EVLP protocols use RBC-based perfusate. In the case of Ad vector, various blood components, including RBC, PBMC, plasma and serum, have been found to interfere with vector transduction of cell lines in vitro (47). Therefore, EVLP protocols with RBC-free perfusate may be ideal to minimize interference of gene delivery. Third, if the vector is to be delivered through the vasculature, the circulating volume of perfusate is likely to play a significant role in the vector dose (and therefore cost) required to induce effective transduction. Lastly, while there has been recent enthusiasm regarding the utility of EVLP, not all donor organs require EVLP prior to implantation. EVLP preservation requires additional time and resources compared to static cold storage. Gene delivery to donor organs does not necessarily require EVLP, though EVLP could help facilitate transgene expression when targeting processes occurring soon after reperfusion.
Current Status of Gene Therapy in Lung Transplant
Gene therapy has been utilized to target several challenges in lung transplantation, including IRI, acute rejection, and chronic graft dysfunction (Figure 2, Table 1).
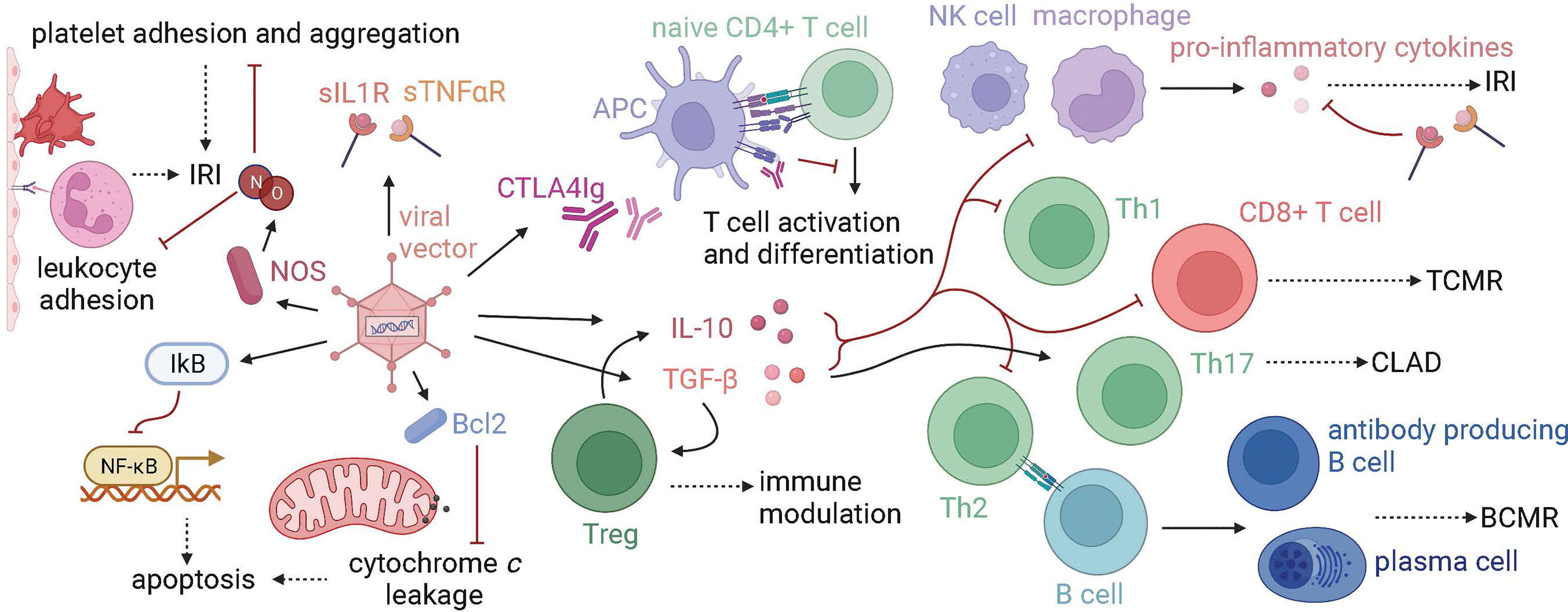
Figure 2 Gene therapy targets in lung transplantation. Graft injury at many timepoints in transplantation, from procurement to years following transplant, has been targeted using gene therapy. Proteins that modulate the immune system, prevent apoptosis, or protect the endothelium have been transfected using vectors, namely viral vectors such as adenovirus. Anti-inflammatory cytokines such as IL-10 and TGF-beta decrease innate immune responses, T cell effector and helper functions, and B cell costimulation, which makes them a target in the treatment of IRI, TCMR, BCMR, and CLAD. Tregs are activated by TGF-beta, produce IL-10, and have immune-modulating functions. Other immune-modulating proteins such as CTLA4Ig, which blocks T cell activation, and soluble IL1 and TNF-alpha receptors (sIL1R, sTNF-alphaR), which block the downstream effects of proinflammatory cytokines, have been used in gene therapy models. NOS is a target for ameliorating IRI, as the production of NO decreases both platelet and leukocyte adhesion. Anti-apoptotic molecules, such as IkB and Bcl2 have also been used in IRI models. (IRI, ischemia-reperfusion injury; TCMR, T cell-mediated rejection; CLAD, chronic lung allograft dysfunction; BCMR, B cell-mediated rejection).
Ischemia-Reperfusion Injury
IL-10 is the most widely employed target of gene therapy approaches to mitigate IRI given its broad anti-inflammatory properties. Exogenous administration of IL-10 has previously demonstrated the ability to attenuate lung IRI (109). In murine transplant models, administration of IL-10 gene therapy is most often delivered intratracheally to the donor in vivo using an Ad vector, which results in improved post-reperfusion lung function, with decreased expression of TNF-α, IFN-γ, and nitric oxide synthetase (NOS) (110–112). In addition, the delivery of IL-10 gene therapy to the donor graft in vivo causes a shift from necrosis to apoptosis as the dominant mode of IRI-induced cell death in a rat lung transplant model (113). Intramuscular Ad vector encoding IL-10 similarly ameliorates IRI in small animal lung transplantation models but was found to require a higher vector titer and trigger systemic effects that local delivery avoids (114).
As detailed in earlier sections, one challenge of the use of Ad vector is a vector-induced inflammatory response, especially when administered in vivo to the donor, in both small and large animal models (11, 115). In fact, even empty Ad virion post gene delivery can continue to serve as a significant source of stress and inflammatory response (116). One potential solution is to allow sufficient time for IL-10 transgene expression in the donor prior to organ retrieval so that by the time of retrieval, the anti-inflammatory effect of IL-10 has reversed the vector-induced inflammation. de Perrot et al. also demonstrated methylprednisolone given prior to Ad vector administration reduces these inflammatory effects (11).
An alternative approach is to remove the impact of donor immune system and deliver Ad vector ex vivo. A comparison of in vivo and ex vivo intratracheal delivery of AdhIL-10 in a porcine transplant model saw improved lung function and decreased inflammation on histology in the ex vivo group despite cytokine levels comparable to those of the in vivo group, perhaps due to the absence of circulating leukocytes in ex vivo perfusion circuits (32). The same group also demonstrated the utility of ex vivo intratracheal delivery in discarded human lungs, reproducing the findings observed in small animal models (23). AdhIL-10 delivery to discarded human lungs resulted in maintenance of pulmonary physiologic parameters, decreased IL-1β and IL-8, and restoration of tight junctions on histology, indicating successful suppression of inflammatory injury in lung allografts (23).
TGF-β is generally considered an anti-inflammatory cytokine, although its roles in fibrosis and promoting Th17 cells are also well established (117). As applied to IRI, TGF-β gene transfer has been utilized as monotherapy and in combination with IL-10 transduction. IM administration of naked plasmid encoding activated TGF-β1 prior to lung transplantation and following prolonged ischemia decreases cytokines such as TNF-α, IFN-γ, and IL-2, reduces neutrophil sequestration, and improves IRI in a small animal model (80). Unsurprisingly, the combination of TGF-β1 and IL-10 transfection potentiates amelioration of IRI and reduction of pro-inflammatory cytokines (79). Conversely, other studies have utilized Ad vectors that produce inhibitors of pro-inflammatory cytokines such as TNF-α and IL-1. Soluble TNF-α and IL-1 receptors inhibit the inflammatory effects of these cytokines, thus ameliorating IRI in rodent models (118, 119).
Other than targeting IL-10 and TGF-β, both of which are broadly anti-inflammatory cytokines, researchers have investigated specific pathways implicated in IRI or cell death. Ad vectors have been used to deliver several cytoprotective genes in a number of IRI small animal studies. During IRI, nitric oxide synthase (NOS) in the endothelium produces less nitric oxide (NO), which, under normal conditions, inhibits leukocyte adhesion and platelet adhesion and aggregation. Systemic in vivo administration of endothelial NOS using an Ad vector improved oxygenation and reduced neutrophil sequestration 24 hours posttransplant (120). Similar results were seen with the delivery of heat shock protein 70 (HSP70), a protein known to increase resistance to IRI, to the donor lung (121). Anti-apoptotic proteins have also been targeted in lung IRI experiments. IκB, an inhibitor of NFκB, and Bcl-2, a protein that prevents cytochrome c leakage from the mitochondria, both decrease induction of apoptosis and ameliorate IRI when delivered using an Ad vector in rodent models (122, 123). Short hairpin RNA (shRNA) and small interfering RNA (siRNA) are other delivery mode that has been used to suppress apoptosis. Use of intratracheal shRNAs targeting caspase-3 and p38α, a mitogen-activated protein kinase, prevents apoptosis and protects the lung from IRI (124, 125). Similarly, intratracheal Fas siRNA decreases pulmonary cell apoptosis and edema without decreasing cytokine release or neutrophil infiltration (126).
Acute Rejection
The role of several anti-inflammatory cytokines has been evaluated in acute rejection (AR) models. In a major MHC mismatched rat lung transplant model, Oishi et al. showed decreased AR scores, pulmonary edema, hemorrhage, and necrosis along with decreased inflammatory cytokines 6 days posttransplant in those that received hIL-10 plasmid ex vivo (127). Similar results were demonstrated by Hirayama et al. in a minor MHC mismatch model, where hIL-10 was delivered using a lentiviral vector given both pretransplant to donors and posttransplant to recipients (72). In another set of experiments, Itano et al. initially did not see any difference in physiologic parameters or rejection score when viral IL-10 (vIL-10) was delivered endobronchially using a naked plasmid to the donor lung (128); however, vIL-10 delivery via the pulmonary vein using a liposomal vector did result in improvement of physiologic parameters and AR score (129). Interestingly, transgene expression was confirmed in both cases, but semi-quantitative western blot showed better expression of vIL-10 when delivered via a liposomal vector,. In a later study, Osada et al. delivered vIL-10 using an Ad vector in vivo to the airway and failed to see any significant difference in AR score 6-day posttransplant, despite successful transgene expression (130). The inherent immunogenicity of the Ad vector may add to the alloimmune-mediated damage and interfere with vIL-10’s effect on the acute rejection phenotype. Most recently, Machuca et al. examined the delivery of AdhIL-10 in a porcine lung transplant survival model. At day 7 posttransplant, lungs that received IL-10 gene therapy had better gas exchange and lower inflammation scores. Additionally, recipient lymphocytes from the AdhIL-10 group show less proliferation and IFN-γ production on mixed lymphocyte reaction (33). Apart from IL-10, the delivery of several other anti-inflammatory cytokines using gene therapy has been evaluated in acute rejection models with mixed results. Endobronchial plasmid delivery of TGF-β1 resulted in improved PaO2 and decreased rejection scores in mice (131). Ex vivo delivery of lipid-mediated TGF-β1 improved oxygenation without improving rejection scores (132). Furthermore, several studies delivered genes encoding anti-inflammatory cytokines intramuscularly, and evaluated the impact of systemic production of those cytokines on lung transplant outcomes (133–135).
As in IRI experiments, cytoprotective genes have also been utilized in AR studies with variable success. Transbronchial administration of adenoviral eNOS does not reduce AR scores despite increase in NO production (136). In contrast, Sendai virus-liposome complex-mediated transfection of oligo-deoxynucleotide (ODN), an NFκB inhibitor, improved graft function and oxygenation and decreased rejection scores (137). Targeting anti-apoptotic molecules such as FasL improved graft function and rejection scores when paired with cyclosporine A (138).
Another approach is to deliver transgenes that specifically target adaptive immunity. IDO degrades tryptophan and suppresses T cell responses via tryptophan metabolites (139, 140). Treatment of donor lungs with viral or non-viral vectors encoding IDO inhibited T-cell response and decreased AR scores, cell necrosis, and apoptosis (141, 142). CTLA4Ig inhibits T cell costimulation by disrupting B7-CD28 interaction, thus suppressing T cell activation. CTLA4Ig delivery to donor lung allografts using an Ad vector, did not reduce lymphocyte infiltration or AR scores on day 4 posttransplant in spite of transgene expression (143). Lastly, a German group expanded on this approach by attempting to silence graft major histocompatibility complex (MHC) using a gene therapy approach in a porcine model. MHC mismatch can trigger alloimmune-mediated responses and thus deletion of MHC on graft endothelium could reduce graft immunogenicity. The authors delivered a lentiviral vector encoding short hairpin RNA (shRNA) targeting beta-2-microglobulin and class II-transactivator transcripts during EVLP. Using this approach, they were able to demonstrate the deletion of swine leukocyte antigen (SLA) from the majority of endothelial cells following vector delivery in vitro (144). Similar approach has demonstrated efficacy in a murine kidney model (24).
Chronic Graft Dysfunction
Chronic lung allograft dysfunction (CLAD) remains a poorly understood process. Acute rejection, IRI leading to PGD, gastroesophageal reflux and recurrent infections are known risk factors for CLAD. In the current era, there is now emerging evidence that CLAD is related to aberrant airway repair, leading to airway remodeling, following airway epithelial injury. Th17 response is implicated in CLAD, and so is autoimmunity to various cryptic self-antigens.
Few investigators have utilized gene therapy to target CLAD, likely because few therapeutic targets are established for CLAD and a high fidelity animal model is lacking. IL-10 gene therapy has been delivered using multiple vectors—such as adenovirus, lentivirus, and sendai virus—in chronic rejection models and has been shown to attenuate bronchiolitis obliterans (BO) following lung transplantation (70, 145, 146). A combined ex and in vivo dual approach using lentiviral IL-10 was found to inhibit both acute and chronic rejection in a mouse model with decreased fibrosis and airway obliteration on histology (73). Jiang et al. successfully delivered hypoxia inducible factor 1α (HIF-1α) to the donor trachea by preserving the donor trachea in solution containing Ad vector, which attenuated airway fibrotic remodeling in a tracheal transplant model. HIF-1α transfection prevents loss of the epithelial lining and improves airway microvascular perfusion, while inducing expression of angiogenic factors such as PLGF and VEGF (147).
Conclusion
Gene therapy has emerged as a potential therapeutic intervention in lung transplantation. Despite the interest in the field and overall positive experience in small and large animal models, the clinical translation of gene therapy in lung transplant remains limited. This is partly because most studies have been performed using Ad vectors, which have raised safety concerns in clinical trial and are now reserved for special indications. AAV is currently the leading platform for in vivo therapy; however, it has yet to be applied to lung transplantation. Transbronchial delivery of gene therapy is most widely used in experimental models and provides great transduction efficiency without off target effects. However, vascular delivery or novel modifications to the viral vectors may be necessary to target the vascular endothelium, which serves as the interphase between donor organ and recipient immune system. Several aspects of immune-mediated injuries in lung transplant can be targeted using gene therapy, including IRI (IL-10, HO-1, anti-apoptotic proteins), acute rejection (anti-inflammatory cytokines, co-inhibitory molecules, silence of immunogenic molecules) and CLAD. Ultimately, there is not a perfect one-for-all vector for gene therapy in lung transplant. There may, however, be an optimal vector for the delivery of a specific target gene of interest that will ensure sufficient transgene expression at the right time and in the right place.
For genes that target IRI, the protein product needs to be present at the time of reperfusion. Therefore, a vector such as an adenoviral vector with a fast onset is desired, and practically, organs will require a period of EVLP prior to implantation to facilitate protein synthesis. In contrast, if a late immune insult is targeted, it is preferred to use a vector that persists long-term and can evade the host immune system well. The recent popularization of EVLP has provided a unique platform for gene therapy delivery, but it remains to be seen whether this platform is necessary for all gene therapy.
Lastly, most pre-clinical gene therapy studies target one cytokine or immunomodulatory molecules. Practically, it is unlikely that modulation of a single pathway will solve complex processes such as IRI or CLAD. Co-administration of multiple vectors targeting multiple pathways may be necessary (148). Additionally, many cytokines are pleotropic with inhibition of some inflammatory pathways and activation of others. For example, IL-10 generally inhibits Th1 cytokines and NF-κB activity but promotes B cell proliferation and antibody production. TGF-β induces Tregs but promotes highly inflammatory TH17 cells. Therefore, constitutive expression of various cytokines may lead to undesired effects. Inflammation inducible vector has been reported in the literature and may potentially provide a solution (149).
Author Contributions
QG, AB and MH conceived this review. QG, IA and ID preformed literature review and drafted the manuscript. IA, SK, NA, AA, RK performed critical review and revision of the manuscript. MH, AA and AB performed critical review and revision of the manuscript and oversaw the drafting process. All authors contributed to the article and approved the submitted version.
Conflict of Interest
MH consults for Bridge to Life, Paragonix, Medtronic, and Intuitive Surgical and has research funding from Cystic Fibrosis Foundation, Biomedinnovations, and Transmedics. AA is co-founder and director at StrideBio and TorqueBio. He is also an advisor to Kriya Therapeutics, Atsena Therapeutics, Ring Therapeutics and Mammoth Bio.
The remaining authors declare that the research was conducted in the absence of any commercial or financial relationships that could be construed as a potential conflict of interest.
Publisher’s Note
All claims expressed in this article are solely those of the authors and do not necessarily represent those of their affiliated organizations, or those of the publisher, the editors and the reviewers. Any product that may be evaluated in this article, or claim that may be made by its manufacturer, is not guaranteed or endorsed by the publisher.
Acknowledgments
All figures were created using BioRender.com.
References
1. Chambers DC, Cherikh WS, Harhay MO, Hayes D Jr., Hsich E, Khush KK, et al. The International Thoracic Organ Transplant Registry of the International Society for Heart and Lung Transplantation: Thirty-Sixth Adult Lung and Heart-Lung Transplantation Report-2019; Focus Theme: Donor and Recipient Size Match. J Heart Lung Transplant (2019) 38:1042–55. doi: 10.1016/j.healun.2019.08.001
2. Valapour M, Lehr CJ, Skeans MA, Smith JM, Miller E, Goff R, et al. OPTN/SRTR 2019 Annual Data Report: Lung. Am J Transplant (2021) 21 Suppl 2:441–520. doi: 10.1111/ajt.16495
3. Israni AK, Zaun D, Rosendale JD, Schaffhausen C, Mckinney W, Snyder JJ. OPTN/SRTR 2019 Annual Data Report: Deceased Organ Donors. Am J Transplant (2021) 21(Suppl 2):521–58. doi: 10.1111/ajt.16491
4. Dunbar CE, High KA, Joung JK, Kohn DB, Ozawa K, Sadelain M. Gene Therapy Comes of Age. Science (2018) 359:1–10. doi: 10.1126/science.aan4672
5. Cypel M, Yeung JC, Liu M, Anraku M, Chen F, Karolak W, et al. Normothermic Ex Vivo Lung Perfusion in Clinical Lung Transplantation. N Engl J Med (2011) 364:1431–40. doi: 10.1056/NEJMoa1014597
6. Warnecke G, Van Raemdonck D, Smith MA, Massard G, Kukreja J, Rea F, et al. Normothermic Ex-Vivo Preservation With the Portable Organ Care System Lung Device for Bilateral Lung Transplantation (INSPIRE): A Randomised, Open-Label, non-Inferiority, Phase 3 Study. Lancet Respir Med (2018) 6:357–67. doi: 10.1016/S2213-2600(18)30136-X
7. Friedmann T, Roblin R. Gene Therapy for Human Genetic Disease? Science (1972) 175:949–55. doi: 10.1126/science.175.4025.949
8. Bulaklak K, Gersbach CA. The Once and Future Gene Therapy. Nat Commun (2020) 11:1–4. doi: 10.1038/s41467-020-19505-2
9. Muhuri M, Maeda Y, Ma H, Ram S, Fitzgerald KA, Tai PW, et al. Overcoming Innate Immune Barriers That Impede AAV Gene Therapy Vectors. J Clin Invest (2021) 131:1–14. doi: 10.1172/JCI143780
10. Cassivi SD, Liu M, Boehler A, Pierre A, Tanswell AK, O'brodovich H, et al. Transplant Immunosuppression Increases and Prolongs Transgene Expression Following Adenoviral-Mediated Transfection of Rat Lungs. J Heart Lung Transplant (2000) 19:984–94. doi: 10.1016/S1053-2498(00)00166-2
11. De Perrot M, Fischer S, Liu M, Imai Y, Martins S, Sakiyama S, et al. Impact of Human Interleukin-10 on Vector-Induced Inflammation and Early Graft Function in Rat Lung Transplantation. Am J Respir Cell Mol Biol (2003) 28:616–25. doi: 10.1165/rcmb.2002-0109OC
12. Corti M, Elder M, Falk D, Lawson L, Smith B, Nayak S, et al. B-Cell Depletion is Protective Against Anti-AAV Capsid Immune Response: A Human Subject Case Study. Mol Ther Methods Clin Dev (2014) 1:14033–. doi: 10.1038/mtm.2014.33
13. Meliani A, Boisgerault F, Hardet R, Marmier S, Collaud F, Ronzitti G, et al. Antigen-Selective Modulation of AAV Immunogenicity With Tolerogenic Rapamycin Nanoparticles Enables Successful Vector Re-Administration. Nat Commun (2018) 9:1–13. doi: 10.1038/s41467-018-06621-3
14. Van Haasteren J, Hyde SC, Gill DR. Lessons Learned From Lung and Liver in-Vivo Gene Therapy: Implications for the Future. Expert Opin Biol Ther (2018) 18:959–72. doi: 10.1080/14712598.2018.1506761
15. Wang D, Tai PWL, Gao G. Adeno-Associated Virus Vector as a Platform for Gene Therapy Delivery. Nat Rev Drug Discovery (2019) 18:358–78. doi: 10.1038/s41573-019-0012-9
16. Verdera HC, Kuranda K, Mingozzi F. AAV Vector Immunogenicity in Humans: A Long Journey to Successful Gene Transfer. Mol Ther (2020) 28:723–46. doi: 10.1016/j.ymthe.2019.12.010
17. Ali A, Wang A, Ribeiro RVP, Beroncal EL, Baciu C, Galasso M, et al. Static Lung Storage at 10°C Maintains Mitochondrial Health and Preserves Donor Organ Function. Sci Transl Med (2021) 13:eabf7601. doi: 10.1126/scitranslmed.abf7601
18. Arni S, Maeyashiki T, Opitz I, Inci I. Subnormothermic Ex Vivo Lung Perfusion Attenuates Ischemia Reperfusion Injury From Donation After Circulatory Death Donors. PloS One (2021) 16:e0255155–e0255155. doi: 10.1371/journal.pone.0255155
19. Gloria JN, Yerxa J, Kesseli SJ, Davis RP, Samoylova ML, Barbas AS, et al. Subnormothermic Ex Vivo Lung Perfusion Attenuates Graft Inflammation in a Rat Transplant Model. J Thorac Cardiovasc Surg (2021). doi: 10.1016/j.jtcvs.2021.01.066
20. Olthoff KM, Judge TA, Gelman AE, Da Shen X, Hancock WW, Turka LA, et al. Adenovirus-Mediated Gene Transfer Into Cold-Preserved Liver Allografts: Survival Pattern and Unresponsiveness Following Transduction With CTLA4Ig. Nat Med (1998) 4:194–200. doi: 10.1038/nm0298-194
21. Yano M, Hiratsuka M, Nagahiro I, Mora BN, Scheule RK, Patterson GA. Ex Vivo Transfection of Pulmonary Artery Segments in Lung Isografts. Ann Thorac Surg (1999) 68:1805–9. doi: 10.1016/S0003-4975(99)00719-5
22. Brasile L, Stubenitsky BM, Haisch CE, Kon M, Kootstra G. Repair of Damaged Organs In Vitro. Am J Transplant (2005) 5:300–6. doi: 10.1111/j.1600-6143.2005.00682.x
23. Cypel M, Liu M, Rubacha M, Yeung JC, Hirayama S, Anraku M, et al. Functional Repair of Human Donor Lungs by IL-10 Gene Therapy. Sci Transl Med (2009) 1:4ra9. doi: 10.1126/scitranslmed.3000266
24. Yuzefovych Y, Valdivia E, Rong S, Hack F, Rother T, Schmitz J, et al. Genetic Engineering of the Kidney to Permanently Silence MHC Transcripts During Ex Vivo Organ Perfusion. Front Immunol (2020) 11:265. doi: 10.3389/fimmu.2020.00265
25. Kesseli S, Krischak M, Gao QM, Halpern S, Zhang M, Abraham N, et al. Adeno-Associated Virus 9 Mediates Gene Transduction During Static Cold Storage in Rodent Liver Transplantation. Am J Transplant (2022) 22:67–7.
26. Kesseli SJ, Krischak MK, Gao Q, Halpern SE, Zhang M, Song M, et al. Intra-Tracheal Adeno-Associated Virus Mediates Gene Transduction During Static Cold Storage in Rodent Lung Transplantation. J Heart Lung Transplant (2022) 41:S38–9. doi: 10.1016/j.healun.2022.01.087
27. Cassivi SD, Cardella JA, Fischer S, Liu M, Slutsky AS, Keshavjee S. Transtracheal Gene Transfection of Donor Lungs Prior to Organ Procurement Increases Transgene Levels at Reperfusion and Following Transplantation. J Heart Lung Transplant (1999) 18:1181–8. doi: 10.1016/S1053-2498(99)00095-9
28. Rosenfeld MA, Siegfried W, Yoshimura K, Yoneyama K, Fukayama M, Stier LE, et al. Adenovirus-Mediated Transfer of a Recombinant Alpha 1-Antitrypsin Gene to the Lung Epithelium In Vivo. Science (1991) 252:431–4. doi: 10.1126/science.2017680
29. Rosenfeld MA, Yoshimura K, Trapnell BC, Yoneyama K, Rosenthal ER, Dalemans W, et al. In Vivo Transfer of the Human Cystic Fibrosis Transmembrane Conductance Regulator Gene to the Airway Epithelium. Cell (1992) 68:143–55. doi: 10.1016/0092-8674(92)90213-V
30. Steines B, Dickey DD, Bergen J, Excoffon KJ, Weinstein JR, Li X, et al. CFTR Gene Transfer With AAV Improves Early Cystic Fibrosis Pig Phenotypes. JCI Insight (2016) 1:e88728. doi: 10.1172/jci.insight.88728
31. Janssens SP, Bloch KD, Nong Z, Gerard RD, Zoldhelyi P, Collen D. Adenoviral-Mediated Transfer of the Human Endothelial Nitric Oxide Synthase Gene Reduces Acute Hypoxic Pulmonary Vasoconstriction in Rats. J Clin Invest (1996) 98:317–24. doi: 10.1172/JCI118795
32. Yeung JC, Wagnetz D, Cypel M, Rubacha M, Koike T, Chun YM, et al. Ex Vivo Adenoviral Vector Gene Delivery Results in Decreased Vector-Associated Inflammation Pre- and Post-Lung Transplantation in the Pig. Mol Ther (2012) 20:1204–11. doi: 10.1038/mt.2012.57
33. Machuca TN, Cypel M, Bonato R, Yeung JC, Chun YM, Juvet S, et al. Safety and Efficacy of Ex Vivo Donor Lung Adenoviral IL-10 Gene Therapy in a Large Animal Lung Transplant Survival Model. Hum Gene Ther (2017) 28:757–65. doi: 10.1089/hum.2016.070
34. Kanaan SA, Kozower BD, Suda T, Daddi N, Tagawa T, Ritter JH, et al. Intratracheal Adenovirus-Mediated Gene Transfer is Optimal in Experimental Lung Transplantation. J Thorac Cardiovasc Surg (2002) 124:1130–6. doi: 10.1067/mtc.2002.123702
35. Sato M, Keshavjee S. Gene Therapy in Lung Transplantation. Curr Gene Ther (2006) 6:439–58. doi: 10.2174/156652306777934810
36. Lemarchand P, Jones M, Danel C, Yamada I, Mastrangeli A, Crystal RG. In Vivo Adenovirus-Mediated Gene Transfer to Lungs via Pulmonary Artery. J Appl Physiol (1985) (1994) 76:2840–5. doi: 10.1152/jappl.1994.76.6.2840
37. Muller DW, Gordon D, San H, Yang Z, Pompili VJ, Nabel GJ, et al. Catheter-Mediated Pulmonary Vascular Gene Transfer and Expression. Circ Res (1994) 75:1039–49. doi: 10.1161/01.RES.75.6.1039
38. Schachtner SK, Rome JJ, Hoyt RF Jr., Newman KD, Virmani R, Dichek DA. In Vivo Adenovirus-Mediated Gene Transfer via the Pulmonary Artery of Rats. Circ Res (1995) 76:701–9. doi: 10.1161/01.RES.76.5.701
39. Chapelier A, Danel C, Mazmanian M, Bacha EA, Sellak H, Gilbert MA, et al. Gene Therapy in Lung Transplantation: Feasibility of Ex Vivo Adenovirus-Mediated Gene Transfer to the Graft. Hum Gene Ther (1996) 7:1837–45. doi: 10.1089/hum.1996.7.15-1837
40. Canonico AE, Conary JT, Meyrick BO, Brigham KL. Aerosol and Intravenous Transfection of Human Alpha 1-Antitrypsin Gene to Lungs of Rabbits. Am J Respir Cell Mol Biol (1994) 10:24–9. doi: 10.1165/ajrcmb.10.1.8292378
41. Walker CM, Rosado-De-Christenson ML, Martínez-Jiménez S, Kunin JR, Wible BC. Bronchial Arteries: Anatomy, Function, Hypertrophy, and Anomalies. RadioGraphics (2015) 35:32–49. doi: 10.1148/rg.351140089
42. Bakhai A, Sheridan DJ, Coutelle CC. "Bronchial Artery Delivery of Viral Vectors for Gene Delivery in Cystic Fibrosis; Superior to Airway Delivery?". BMC Pulm Med (2002) 2:2. doi: 10.1186/1471-2466-2-2
43. Rahim F, Ebrahimi A. Gene Therapy Modalities in Lung Transplantation. Transpl Immunol (2014) 31:165–72. doi: 10.1016/j.trim.2014.08.001
44. Raper SE, Chirmule N, Lee FS, Wivel NA, Bagg A, Gao GP, et al. Fatal Systemic Inflammatory Response Syndrome in a Ornithine Transcarbamylase Deficient Patient Following Adenoviral Gene Transfer. Mol Genet Metab (2003) 80:148–58. doi: 10.1016/j.ymgme.2003.08.016
45. Akinc A, Maier MA, Manoharan M, Fitzgerald K, Jayaraman M, Barros S, et al. The Onpattro Story and the Clinical Translation of Nanomedicines Containing Nucleic Acid-Based Drugs. Nat Nanotechnology (2019) 14:1084–7. doi: 10.1038/s41565-019-0591-y
46. Zabner J, Couture LA, Gregory RJ, Graham SM, Smith AE, Welsh MJ. Adenovirus-Mediated Gene Transfer Transiently Corrects the Chloride Transport Defect in Nasal Epithelia of Patients With Cystic Fibrosis. Cell (1993) 75:207–16. doi: 10.1016/0092-8674(93)80063-K
47. Bishawi M, Roan JN, Milano CA, Daneshmand MA, Schroder JN, Chiang Y, et al. A Normothermic Ex Vivo Organ Perfusion Delivery Method for Cardiac Transplantation Gene Therapy. Sci Rep (2019) 9:1–9. doi: 10.1038/s41598-019-43737-y
48. Wold WSM, Toth K. Adenovirus Vectors for Gene Therapy, Vaccination and Cancer Gene Therapy. Curr Gene Ther (2013) 13:421–33. doi: 10.2174/1566523213666131125095046
49. Crystal RG. Adenovirus: The First Effective In Vivo Gene Delivery Vector. Hum Gene Ther (2014) 25:3–11. doi: 10.1089/hum.2013.2527
50. Bulcha JT, Wang Y, Ma H, Tai PWL, Gao G. Viral Vector Platforms Within the Gene Therapy Landscape. Signal Transduction Targeted Ther (2021) 6:53. doi: 10.1038/s41392-021-00487-6
51. Zaiss AK, Liu Q, Bowen GP, Wong NC, Bartlett JS, Muruve DA. Differential Activation of Innate Immune Responses by Adenovirus and Adeno-Associated Virus Vectors. J Virol (2002) 76:4580–90. doi: 10.1128/JVI.76.9.4580-4590.2002
52. Somanathan S, Breous E, Bell P, Wilson JM. AAV Vectors Avoid Inflammatory Signals Necessary to Render Transduced Hepatocyte Targets for Destructive T Cells. Mol Ther (2010) 18:977–82. doi: 10.1038/mt.2010.40
53. Büning H, Schmidt M. Adeno-Associated Vector Toxicity-To Be or Not to be? Mol Ther (2015) 23:1673–5. doi: 10.1038/mt.2015.182
54. Srivastava A. In Vivo Tissue-Tropism of Adeno-Associated Viral Vectors. Curr Opin Virol (2016) 21:75–80. doi: 10.1016/j.coviro.2016.08.003
55. Hayashi H, Kubo Y, Izumida M, Matsuyama T. Efficient Viral Delivery of Cas9 Into Human Safe Harbor. Sci Rep (2020) 10:21474. doi: 10.1038/s41598-020-78450-8
56. Sabatino DE, Mccarty DM. Topics in AAV Integration Come Front and Center at ASGCT AAV Integration Roundtable. Mol Ther (2021) 29:3319–20. doi: 10.1016/j.ymthe.2021.10.024
57. Mccarty DM. Self-Complementary AAV Vectors; Advances and Applications. Mol Ther (2008) 16:1648–56. doi: 10.1038/mt.2008.171
58. Naso MF, Tomkowicz B, Perry WL 3rd, Strohl WR. Adeno-Associated Virus (AAV) as a Vector for Gene Therapy. BioDrugs (2017) 31:317–34. doi: 10.1007/s40259-017-0234-5
59. Nathwani AC, Reiss UM, Tuddenham EG, Rosales C, Chowdary P, Mcintosh J, et al. Long-Term Safety and Efficacy of Factor IX Gene Therapy in Hemophilia B. N Engl J Med (2014) 371:1994–2004. doi: 10.1056/NEJMoa1407309
60. George LA, Sullivan SK, Giermasz A, Rasko JEJ, Samelson-Jones BJ, Ducore J, et al. Hemophilia B Gene Therapy With a High-Specific-Activity Factor IX Variant. N Engl J Med (2017) 377:2215–27. doi: 10.1056/NEJMoa1708538
61. Chamberlain K, Riyad JM, Weber T. Expressing Transgenes That Exceed the Packaging Capacity of Adeno-Associated Virus Capsids. Hum Gene Ther Methods (2016) 27:1–12. doi: 10.1089/hgtb.2015.140
62. Bonaccorsi-Riani E, Gillooly A, Brüggenwirth IMA, Martins PN. Delivery of Genetic Load During Ex Situ Liver Machine Perfusion With Potential for CRISPR-Cas9 Gene Editing: An Innovative Strategy for Graft Treatment. Hepatobiliary Pancreat Dis Int (2021) 20:503–5. doi: 10.1016/j.hbpd.2021.04.006
63. Castellani S, Conese M. Lentiviral Vectors and Cystic Fibrosis Gene Therapy. Viruses (2010) 2:395–412. doi: 10.3390/v2020395
64. Liu YP, Berkhout B. HIV-1-Based Lentiviral Vectors. Methods Mol Biol (2014) 1087:273–84. doi: 10.1007/978-1-62703-670-2_22
65. Limberis MP, Bell CL, Heath J, Wilson JM. Activation of Transgene-Specific T Cells Following Lentivirus-Mediated Gene Delivery to Mouse Lung. Mol Ther (2010) 18:143–50. doi: 10.1038/mt.2009.190
66. Sinn PL, Arias AC, Brogden KA, Mccray PB Jr. Lentivirus Vector can be Readministered to Nasal Epithelia Without Blocking Immune Responses. J Virol (2008) 82:10684–92. doi: 10.1128/JVI.00227-08
67. Donnelley M, Parsons DW. Gene Therapy for Cystic Fibrosis Lung Disease: Overcoming the Barriers to Translation to the Clinic. Front Pharmacol (2018) 9:1381. doi: 10.3389/fphar.2018.01381
68. Buckley SM, Howe SJ, Sheard V, Ward NJ, Coutelle C, Thrasher AJ, et al. Lentiviral Transduction of the Murine Lung Provides Efficient Pseudotype and Developmental Stage-Dependent Cell-Specific Transgene Expression. Gene Ther (2008) 15:1167–75. doi: 10.1038/gt.2008.74
69. Wilson AA, Murphy GJ, Hamakawa H, Kwok LW, Srinivasan S, Hovav AH, et al. Amelioration of Emphysema in Mice Through Lentiviral Transduction of Long-Lived Pulmonary Alveolar Macrophages. J Clin Invest (2010) 120:379–89. doi: 10.1172/JCI36666
70. Hirayama S, Sato M, Liu M, Loisel-Meyer S, Yeung JC, Wagnetz D, et al. Local Long-Term Expression of Lentivirally Delivered IL-10 in the Lung Attenuates Obliteration of Intrapulmonary Allograft Airways. Hum Gene Ther (2011) 22:1453–60. doi: 10.1089/hum.2010.225
71. Cooney AL, Abou Alaiwa MH, Shah VS, Bouzek DC, Stroik MR, Powers LS, et al. Lentiviral-Mediated Phenotypic Correction of Cystic Fibrosis Pigs. JCI Insight (2016) 1:1–9. doi: 10.1172/jci.insight.88730
72. Hirayama S, Sato M, Loisel-Meyer S, Matsuda Y, Oishi H, Guan Z, et al. Lentivirus IL-10 Gene Therapy Down-Regulates IL-17 and Attenuates Mouse Orthotopic Lung Allograft Rejection. Am J Transplant (2013) 13:1586–93. doi: 10.1111/ajt.12230
73. Oishi H, Juvet SC, Martinu T, Sato M, Medin JA, Liu M, et al. A Novel Combined Ex Vivo and In Vivo Lentiviral Interleukin-10 Gene Delivery Strategy at the Time of Transplantation Decreases Chronic Lung Allograft Rejection in Mice. J Thorac Cardiovasc Surg (2018) 156:1305–15. doi: 10.1016/j.jtcvs.2018.05.003
74. Milone MC, O'doherty U. Clinical Use of Lentiviral Vectors. Leukemia (2018) 32:1529–41. doi: 10.1038/s41375-018-0106-0
75. Montini E, Cesana D, Schmidt M, Sanvito F, Bartholomae CC, Ranzani M, et al. The Genotoxic Potential of Retroviral Vectors is Strongly Modulated by Vector Design and Integration Site Selection in a Mouse Model of HSC Gene Therapy. J Clin Invest (2009) 119:964–75. doi: 10.1172/JCI37630
76. Rothe M, Modlich U, Schambach A. Biosafety Challenges for Use of Lentiviral Vectors in Gene Therapy. Curr Gene Ther (2013) 13:453–68. doi: 10.2174/15665232113136660006
77. Martínez-Molina E, Chocarro-Wrona C, Martínez-Moreno D, Marchal JA, Boulaiz H. Large-Scale Production of Lentiviral Vectors: Current Perspectives and Challenges. Pharmaceutics (2020) 12:1–20. doi: 10.3390/pharmaceutics12111051
78. D'ovidio F, Daddi N, Suda T, Grapperhaus K, Patterson AG. Efficient Naked Plasmid Cotransfection of Lung Grafts by Extended Lung/Plasmid Exposure Time. Ann Thorac Surg (2001) 71:1817–23. doi: 10.1016/S0003-4975(01)02593-0
79. Daddi N, Suda T, D'ovidio F, Kanaan SA, Tagawa T, Grapperhaus K, et al. Recipient Intramuscular Cotransfection of Naked Plasmid Transforming Growth Factor Beta1 and Interleukin 10 Ameliorates Lung Graft Ischemia-Reperfusion Injury. J Thorac Cardiovasc Surg (2002) 124:259–69. doi: 10.1067/mtc.2002.122295
80. Daddi N, Kanaan SA, Suda T, Tagawa T, D'ovidio F, Grapperhaus K, et al. Recipient Intramuscular Administration of Naked Plasmid TGF-Beta1 Attenuates Lung Graft Reperfusion Injury. J Heart Lung Transplant (2003) 22:1323–34. doi: 10.1016/j.healun.2003.09.011
81. Lam JK, Liang W, Chan HK. Pulmonary Delivery of Therapeutic siRNA. Adv Drug Delivery Rev (2012) 64:1–15. doi: 10.1016/j.addr.2011.02.006
82. Zoulikha M, Xiao Q, Boafo GF, Sallam MA, Chen Z, He W. Pulmonary Delivery of siRNA Against Acute Lung Injury/Acute Respiratory Distress Syndrome. Acta Pharm Sin B (2022) 12:600–20. doi: 10.1016/j.apsb.2021.08.009
83. Hu B, Zhong L, Weng Y, Peng L, Huang Y, Zhao Y, et al. Therapeutic siRNA: State of the Art. Signal Transduct Target Ther (2020) 5:101. doi: 10.1038/s41392-020-0207-x
84. Moschos SA, Frick M, Taylor B, Turnpenny P, Graves H, Spink KG, et al. Uptake, Efficacy, and Systemic Distribution of Naked, Inhaled Short Interfering RNA (siRNA) and Locked Nucleic Acid (LNA) Antisense. Mol Ther (2011) 19:2163–8. doi: 10.1038/mt.2011.206
85. Kleinman ME, Yamada K, Takeda A, Chandrasekaran V, Nozaki M, Baffi JZ, et al. Sequence- and Target-Independent Angiogenesis Suppression by siRNA via TLR3. Nature (2008) 452:591–7. doi: 10.1038/nature06765
86. Niidome T, Huang L. Gene Therapy Progress and Prospects: Nonviral Vectors. Gene Ther (2002) 9:1647–52. doi: 10.1038/sj.gt.3301923
87. Sung JC, Pulliam BL, Edwards DA. Nanoparticles for Drug Delivery to the Lungs. Trends Biotechnol (2007) 25:563–70. doi: 10.1016/j.tibtech.2007.09.005
88. Van Rijt SH, Bein T, Meiners S. Medical Nanoparticles for Next Generation Drug Delivery to the Lungs. Eur Respir J (2014) 44:765–74. doi: 10.1183/09031936.00212813
89. Zhao Y, Huang L. Lipid Nanoparticles for Gene Delivery. Adv Genet (2014) 88:13–36. doi: 10.1016/B978-0-12-800148-6.00002-X
90. Riley MK, Vermerris W. Recent Advances in Nanomaterials for Gene Delivery-A Review. Nanomaterials (Basel) (2017) 7:1–19. doi: 10.3390/nano7050094
91. Cheng Q, Wei T, Farbiak L, Johnson LT, Dilliard SA, Siegwart DJ. Selective Organ Targeting (SORT) Nanoparticles for Tissue-Specific mRNA Delivery and CRISPR-Cas Gene Editing. Nat Nanotechnol (2020) 15:313–20. doi: 10.1038/s41565-020-0669-6
92. Alfaro R, Legaz I, Gonzalez-Martinez G, Jimenez-Coll V, Martinez-Banaclocha H, Galian JA, et al. Monitoring of B Cell in Kidney Transplantation: Development of a Novel Clusters Analysis and Role of Transitional B Cells in Transplant Outcome. Diagnostics (Basel) (2021) 11:1–20. doi: 10.3390/diagnostics11040641
93. Qiu M, Tang Y, Chen J, Muriph R, Ye Z, Huang C, et al. Lung-Selective mRNA Delivery of Synthetic Lipid Nanoparticles for the Treatment of Pulmonary Lymphangioleiomyomatosis. Proc Natl Acad Sci U.S.A. (2022) 119:1–10. doi: 10.1073/pnas.2116271119
94. Englum BR, Ganapathi AM, Speicher PJ, Gulack BC, Snyder LD, Davis RD, et al. Impact of Donor and Recipient Hepatitis C Status in Lung Transplantation. J Heart Lung Transplant (2016) 35:228–35. doi: 10.1016/j.healun.2015.10.012
95. Mulvihill MS, Gulack BC, Ganapathi AM, Speicher PJ, Englum BR, Hirji SA, et al. The Association of Donor Age and Survival is Independent of Ischemic Time Following Deceased Donor Lung Transplantation. Clin Transplant (2017) 31:1–9. doi: 10.1111/ctr.12993
96. Choi AY, Jawitz OK, Raman V, Halpern SE, Haney JC, Klapper JA, et al. Predictors of Older Donor Lung Use: Are We Too Good at Saying No? Ann Thorac Surg (2020) 110:1683–90. doi: 10.1016/j.athoracsur.2020.04.050
97. Swaminathan AC, Todd JL, Palmer SM. Advances in Human Lung Transplantation. Annu Rev Med (2021) 72:135–49. doi: 10.1146/annurev-med-080119-103200
98. Loor G, Warnecke G, Villavicencio MA, Smith MA, Kukreja J, Ardehali A, et al. Portable Normothermic Ex-Vivo Lung Perfusion, Ventilation, and Functional Assessment With the Organ Care System on Donor Lung Use for Transplantation From Extended-Criteria Donors (EXPAND): A Single-Arm, Pivotal Trial. Lancet Respir Med (2019) 7:975–84. doi: 10.1016/S2213-2600(19)30200-0
99. Nakajima D, Cypel M, Bonato R, Machuca TN, Iskender I, Hashimoto K, et al. Ex Vivo Perfusion Treatment of Infection in Human Donor Lungs. Am J Transplant (2016) 16:1229–37. doi: 10.1111/ajt.13562
100. Galasso M, Feld JJ, Watanabe Y, Pipkin M, Summers C, Ali A, et al. Inactivating Hepatitis C Virus in Donor Lungs Using Light Therapies During Normothermic Ex Vivo Lung Perfusion. Nat Commun (2019) 10:481. doi: 10.1038/s41467-018-08261-z
101. Cypel M, Feld JJ, Galasso M, Pinto Ribeiro RV, Marks N, Kuczynski M, et al. Prevention of Viral Transmission During Lung Transplantation With Hepatitis C-Viraemic Donors: An Open-Label, Single-Centre, Pilot Trial. Lancet Respir Med (2020) 8:192–201. doi: 10.1016/S2213-2600(19)30268-1
102. Ku TJY, Ribeiro RVP, Ferreira VH, Galasso M, Keshavjee S, Kumar D, et al. Ex-Vivo Delivery of Monoclonal Antibody (Rituximab) to Treat Human Donor Lungs Prior to Transplantation. EBioMedicine (2020) 60:102994. doi: 10.1016/j.ebiom.2020.102994
103. Emaminia A, Lapar DJ, Zhao Y, Steidle JF, Harris DA, Laubach VE, et al. Adenosine A₂A Agonist Improves Lung Function During Ex Vivo Lung Perfusion. Ann Thorac Surg (2011) 92:1840–6. doi: 10.1016/j.athoracsur.2011.06.062
104. Charles EJ, Mehaffey JH, Sharma AK, Zhao Y, Stoler MH, Isbell JM, et al. Lungs Donated After Circulatory Death and Prolonged Warm Ischemia are Transplanted Successfully After Enhanced Ex Vivo Lung Perfusion Using Adenosine A2B Receptor Antagonism. J Thorac Cardiovasc Surg (2017) 154:1811–20. doi: 10.1016/j.jtcvs.2017.02.072
105. Cosgun T, Iskender I, Yamada Y, Arni S, Lipiski M, Van Tilburg K, et al. Ex Vivo Administration of Trimetazidine Improves Post-Transplant Lung Function in Pig Model. Eur J Cardiothorac Surg (2017) 52:171–7. doi: 10.1093/ejcts/ezx053
106. Eshmuminov D, Becker D, Bautista Borrego L, Hefti M, Schuler MJ, Hagedorn C, et al. An Integrated Perfusion Machine Preserves Injured Human Livers for 1 Week. Nat Biotechnol (2020) 38:189–+. doi: 10.1038/s41587-019-0374-x
107. Takahashi M, Andrew Cheung HY, Watanabe T, Zamel R, Cypel M, Liu M, et al. Strategies to Prolong Homeostasis of Ex Vivo Perfused Lungs. J Thorac Cardiovasc Surg (2021) 161:1963–73. doi: 10.1016/j.jtcvs.2020.07.104
108. Arni S, Maeyashiki T, Citak N, Opitz I, Inci I. Subnormothermic Ex Vivo Lung Perfusion Temperature Improves Graft Preservation in Lung Transplantation. Cells (2021) 10:1–13. doi: 10.3390/cells10040748
109. Eppinger MJ, Ward PA, Bolling SF, Deeb GM. Regulatory Effects of Interleukin-10 on Lung Ischemia-Reperfusion Injury. J Thorac Cardiovasc Surg (1996) 112:1301–5. doi: 10.1016/S0022-5223(96)70144-7
110. Itano H, Zhang W, Ritter JH, Mccarthy TJ, Mohanakumar T, Patterson GA. Adenovirus-Mediated Gene Transfer of Human Interleukin 10 Ameliorates Reperfusion Injury of Rat Lung Isografts. J Thorac Cardiovasc Surg (2000) 120:947–56. doi: 10.1067/mtc.2000.109240
111. Fischer S, Liu M, Maclean AA, De Perrot M, Ho M, Cardella JA, et al. In Vivo Transtracheal Adenovirus-Mediated Transfer of Human Interleukin-10 Gene to Donor Lungs Ameliorates Ischemia-Reperfusion Injury and Improves Early Posttransplant Graft Function in the Rat. Hum Gene Ther (2001) 12:1513–26. doi: 10.1089/10430340152480249
112. Tagawa T, Suda T, Daddi N, Kozower BD, Kanaan SA, Mohanakumar T, et al. Low-Dose Endobronchial Gene Transfer to Ameliorate Lung Graft Ischemia-Reperfusion Injury. J Thorac Cardiovasc Surg (2002) 123:795–802. doi: 10.1067/mtc.2002.119067
113. Fischer S, De Perrot M, Liu M, Maclean AA, Cardella JA, Imai Y, et al. Interleukin 10 Gene Transfection of Donor Lungs Ameliorates Posttransplant Cell Death by a Switch From Cellular Necrosis to Apoptosis. J Thorac Cardiovasc Surg (2003) 126:1174–80. doi: 10.1016/S0022-5223(03)00114-4
114. Kozower BD, Kanaan SA, Tagawa T, Suda T, Grapperhaus K, Daddi N, et al. Intramuscular Gene Transfer of Interleukin-10 Reduces Neutrophil Recruitment and Ameliorates Lung Graft Ischemia-Reperfusion Injury. Am J Transplant (2002) 2:837–42. doi: 10.1034/j.1600-6143.2002.20905.x
115. Martins S, De Perrot M, Imai Y, Yamane M, Quadri SM, Segall L, et al. Transbronchial Administration of Adenoviral-Mediated Interleukin-10 Gene to the Donor Improves Function in a Pig Lung Transplant Model. Gene Ther (2004) 11:1786–96. doi: 10.1038/sj.gt.3302357
116. Stilwell JL, Samulski RJ. Role of Viral Vectors and Virion Shells in Cellular Gene Expression. Mol Ther (2004) 9:337–46. doi: 10.1016/j.ymthe.2003.11.007
117. Meng X-M, Nikolic-Paterson DJ, Lan HY. TGF-β: The Master Regulator of Fibrosis. Nat Rev Nephrol (2016) 12:325–38. doi: 10.1038/nrneph.2016.48
118. Tagawa T, Kozower BD, Kanaan SA, Daddi N, Suda T, Oka T, et al. Tumor Necrosis Factor Inhibitor Gene Transfer Ameliorates Lung Graft Ischemia-Reperfusion Injury. J Thorac Cardiovasc Surg (2003) 126:1147–54. doi: 10.1016/S0022-5223(03)00584-1
119. Tagawa T, Dharmarajan S, Hayama M, Ishiyama T, Suda T, Itano H, et al. Endobronchial Gene Transfer of Soluble Type I Interleukin-1 Receptor Ameliorates Lung Graft Ischemia-Reperfusion Injury. Ann Thorac Surg (2004) 78:1932–9. doi: 10.1016/j.athoracsur.2004.06.008
120. Suda T, Mora BN, D'ovidio F, Cooper JA, Hiratsuka M, Zhang W, et al. In Vivo Adenovirus-Mediated Endothelial Nitric Oxide Synthase Gene Transfer Ameliorates Lung Allograft Ischemia-Reperfusion Injury. J Thorac Cardiovasc Surg (2000) 119:297–304. doi: 10.1016/S0022-5223(00)70185-1
121. Hiratsuka M, Mora BN, Yano M, Mohanakumar T, Patterson GA. Gene Transfer of Heat Shock Protein 70 Protects Lung Grafts From Ischemia-Reperfusion Injury. Ann Thorac Surg (1999) 67:1421–7. doi: 10.1016/S0003-4975(99)00164-2
122. Cooke DT, Hoyt EG, Robbins RC. Overexpression of Human Bcl-2 in Syngeneic Rat Donor Lungs Preserves Posttransplant Function and Reduces Intragraft Caspase Activity and Interleukin-1beta Production. Transplantation (2005) 79:762–7. doi: 10.1097/01.TP.0000153368.08861.15
123. Ishiyama T, Dharmarajan S, Hayama M, Moriya H, Grapperhaus K, Patterson GA. Inhibition of Nuclear Factor kappaB by IkappaB Superrepressor Gene Transfer Ameliorates Ischemia-Reperfusion Injury After Experimental Lung Transplantation. J Thorac Cardiovasc Surg (2005) 130:194–201. doi: 10.1016/j.jtcvs.2005.02.040
124. Zhang YX, Fan H, Shi Y, Xu ST, Yuan YF, Zheng RH, et al. Prevention of Lung Ischemia-Reperfusion Injury by Short Hairpin RNA-Mediated Caspase-3 Gene Silencing. J Thorac Cardiovasc Surg (2010) 139:758–64. doi: 10.1016/j.jtcvs.2009.09.027
125. Lv X, Tan J, Liu D, Wu P, Cui X. Intratracheal Administration of P38α Short-Hairpin RNA Plasmid Ameliorates Lung Ischemia-Reperfusion Injury in Rats. J Heart Lung Transplant (2012) 31:655–62. doi: 10.1016/j.healun.2012.03.001
126. Del Sorbo L, Costamagna A, Muraca G, Rotondo G, Civiletti F, Vizio B, et al. Intratracheal Administration of Small Interfering RNA Targeting Fas Reduces Lung Ischemia-Reperfusion Injury. Crit Care Med (2016) 44:e604–613. doi: 10.1097/CCM.0000000000001601
127. Oishi H, Okada Y, Kikuchi T, Hoshikawa Y, Sado T, Noda M, et al. Transbronchial Human Interleukin-10 Gene Transfer Reduces Acute Inflammation Associated With Allograft Rejection and Intragraft Interleukin-2 and Tumor Necrosis Factor-Alpha Gene Expression in a Rat Model of Lung Transplantation. J Heart Lung Transplant (2010) 29:360–7. doi: 10.1016/j.healun.2009.10.002
128. Itano H, Zhang WJ, Ritter JH, Mccarthy TJ, Yew NS, Mohanakumar T, et al. Endobronchial Transfection of Naked Viral Interleukin-10 Gene in Rat Lung Allotransplantation. Ann Thorac Surg (2001) 71:1126–33. doi: 10.1016/S0003-4975(00)02651-5
129. Itano H, Mora BN, Zhang WJ, Ritter JH, Mccarthy TJ, Yew NS, et al. Lipid-Mediated Ex Vivo Gene Transfer of Viral Interleukin 10 in Rat Lung Allotransplantation. J Thorac Cardiovasc Surg (2001) 122:29–38. doi: 10.1067/mtc.2001.114636
130. Okada Y, Zuo XJ, Toyoda M, Marchevsky A, Matloff JM, Oishi H, et al. Adenovirus Mediated IL-10 Gene Transfer to the Airway of the Rat Lung for Prevention of Lung Allograft Rejection. Transpl Immunol (2006) 16:95–8. doi: 10.1016/j.trim.2006.03.012
131. D'ovidio F, Yano M, Ritter JH, Mohanakumar T, Patterson GA. Endobronchial Transfection of Naked TGF-Beta1 cDNA Attenuates Acute Lung Rejection. Ann Thorac Surg (1999) 68:1008–13. doi: 10.1016/S0003-4975(99)00784-5
132. Yano M, Mora BN, Ritter JM, Scheule RK, Yew NS, Mohanakumar T, et al. Ex Vivo Transfection of Transforming Growth Factor-Beta1 Gene to Pulmonary Artery Segments in Lung Grafts. J Thorac Cardiovasc Surg (1999) 117:705–13. doi: 10.1016/S0022-5223(99)70290-4
133. Suda T, D'ovidio F, Daddi N, Ritter JH, Mohanakumar T, Patterson GA. Recipient Intramuscular Gene Transfer of Active Transforming Growth Factor-Beta 1 Attenuates Acute Lung Rejection. Ann Thorac Surg (2001) 71:1651–6. doi: 10.1016/S0003-4975(01)02528-0
134. Tagawa T, Kozower BD, Kanaan SA, Daddi N, Muraoka M, Oka T, et al. Gene Transfer of Tumor Necrosis Factor Inhibitor Improves the Function of Lung Allografts. J Thorac Cardiovasc Surg (2004) 127:1558–63. doi: 10.1016/j.jtcvs.2003.09.023
135. Suda T, Daddi N, Tagawa T, Kanaan SA, Kozower BD, Ritter JH, et al. Recipient Intramuscular Cotransfection of Transforming Growth Factor Beta 1 and Interleukin 10 Ameliorates Acute Lung Graft Rejection. J Thorac Cardiovasc Surg (2005) 129:926–31. doi: 10.1016/j.jtcvs.2004.07.055
136. Jeppsson A, Pellegrini C, O'brien T, Miller VM, Tazelaar HD, Taner CB, et al. Gene Transfer of Endothelial Nitric Oxide Synthase to Pulmonary Allografts: Impact on Acute Rejection. Transpl Int (2000) 13 Suppl 1:S591–596. doi: 10.1007/s001470050409
137. Ohmori K, Takeda S, Miyoshi S, Minami M, Nakane S, Ohta M, et al. Attenuation of Lung Injury in Allograft Rejection Using NF-kappaB Decoy Transfection-Novel Strategy for Use in Lung Transplantation. Eur J Cardiothorac Surg (2005) 27:23–7. doi: 10.1016/j.ejcts.2004.09.016
138. Schmid RA, Stammberger U, Hillinger S, Gaspert A, Boasquevisque CH, Malipiero U, et al. Fas Ligand Gene Transfer Combined With Low Dose Cyclosporine A Reduces Acute Lung Allograft Rejection. Transpl Int (2000) 13 Suppl 1:S324–328. doi: 10.1111/j.1432-2277.2000.tb02051.x
139. Shimizu T, Nomiyama S, Hirata F, Hayaishi O. Indoleamine 2,3-Dioxygenase. Purification and Some Properties. J Biol Chem (1978) 253:4700–6. doi: 10.1016/S0021-9258(17)30447-7
140. Hwu P, Du MX, Lapointe R, Do M, Taylor MW, Young HA. Indoleamine 2,3-Dioxygenase Production by Human Dendritic Cells Results in the Inhibition of T Cell Proliferation. J Immunol (2000) 164:3596–9. doi: 10.4049/jimmunol.164.7.3596
141. Swanson KA, Zheng Y, Heidler KM, Mizobuchi T, Wilkes DS. CDllc+ Cells Modulate Pulmonary Immune Responses by Production of Indoleamine 2,3-Dioxygenase. Am J Respir Cell Mol Biol (2004) 30:311–8. doi: 10.1165/rcmb.2003-0268OC
142. Liu H, Liu L, Fletcher BS, Visner GA. Novel Action of Indoleamine 2,3-Dioxygenase Attenuating Acute Lung Allograft Injury. Am J Respir Crit Care Med (2006) 173:566–72. doi: 10.1164/rccm.200509-1413OC
143. Ugurlu MM, Griffin MD, O'brien T, Tazelaar HD, Miller VM, Mcgregor CG. The Effects of CTLA-4Ig on Acute Lung Allograft Rejection: A Comparison of Intrabronchial Gene Therapy With Systemic Administration of Protein. Transplantation (2001) 71:1867–71. doi: 10.1097/00007890-200106270-00029
144. Figueiredo C, Carvalho Oliveira M, Chen-Wacker C, Jansson K, Hoffler K, Yuzefovych Y, et al. Immunoengineering of the Vascular Endothelium to Silence MHC Expression During Normothermic Ex Vivo Lung Perfusion. Hum Gene Ther (2019) 30:485–96. doi: 10.1089/hum.2018.117
145. Boehler A, Chamberlain D, Xing Z, Slutsky AS, Jordana M, Gauldie J, et al. Adenovirus-Mediated Interleukin-10 Gene Transfer Inhibits Post-Transplant Fibrous Airway Obliteration in an Animal Model of Bronchiolitis Obliterans. Hum Gene Ther (1998) 9:541–51. doi: 10.1089/hum.1998.9.4-541
146. Shoji F, Yonemitsu Y, Okano S, Yoshino I, Nakagawa K, Nakashima Y, et al. Airway-Directed Gene Transfer of Interleukin-10 Using Recombinant Sendai Virus Effectively Prevents Post-Transplant Fibrous Airway Obliteration in Mice. Gene Ther (2003) 10:213–8. doi: 10.1038/sj.gt.3301847
147. Jiang X, Khan MA, Tian W, Beilke J, Natarajan R, Kosek J, et al. Adenovirus-Mediated HIF-1α Gene Transfer Promotes Repair of Mouse Airway Allograft Microvasculature and Attenuates Chronic Rejection. J Clin Invest (2011) 121:2336–49. doi: 10.1172/JCI46192
148. Mesaki K, Juvet S, Guan Z, Hu J, Davidson AR, Cypel M, et al. Multiplex Targeted Epigenome Editing Utilizing CRSPR/Cas9 for Potent Anti-Inflammatory Gene Therapy in Lung Transplant. J Heart Lung Transplant (2021) 40:S53. doi: 10.1016/j.healun.2021.01.1869
Keywords: lung transplant, gene therapy, viral vector, Adeno-associated viral vector (AAV vector), Adenoviral (Ad) vector
Citation: Gao Q, DeLaura IF, Anwar IJ, Kesseli SJ, Kahan R, Abraham N, Asokan A, Barbas AS and Hartwig MG (2022) Gene Therapy: Will the Promise of Optimizing Lung Allografts Become Reality? Front. Immunol. 13:931524. doi: 10.3389/fimmu.2022.931524
Received: 29 April 2022; Accepted: 09 June 2022;
Published: 01 July 2022.
Edited by:
Jordi Ochando, Icahn School of Medicine at Mount Sinai, United StatesReviewed by:
Ernest G Chan, University of Pittsburgh Medical Center, United StatesMasashi Furukawa, University of Pittsburgh Medical Center, United States
Copyright © 2022 Gao, DeLaura, Anwar, Kesseli, Kahan, Abraham, Asokan, Barbas and Hartwig. This is an open-access article distributed under the terms of the Creative Commons Attribution License (CC BY). The use, distribution or reproduction in other forums is permitted, provided the original author(s) and the copyright owner(s) are credited and that the original publication in this journal is cited, in accordance with accepted academic practice. No use, distribution or reproduction is permitted which does not comply with these terms.
*Correspondence: Matthew G. Hartwig, bWF0dGhldy5oYXJ0d2lnQGR1a2UuZWR1