- Nantes Université, INSERM, Center for Research in Transplantation and Translational Immunology, UMR 1064, Nantes, France
The thymus is a primary lymphoid organ essential for the induction of central immune tolerance. Maturing T cells undergo several steps of expansion and selection mediated by thymic epithelial cells (TECs). In APECED and other congenital pathologies, a deficiency in genes that regulate TEC development or their ability to select non auto-reactive thymocytes results in a defective immune balance, and consequently in a general autoimmune syndrome. Restoration of thymic function is thus crucial for the emergence of curative treatments. The last decade has seen remarkable progress in both gene editing and pluripotent stem cell differentiation, with the emergence of CRISPR-based gene correction, the trivialization of reprogramming of somatic cells to induced pluripotent stem cells (iPSc) and their subsequent differentiation into multiple cellular fates. The combination of these two approaches has paved the way to the generation of genetically corrected thymic organoids and their use to control thymic genetic pathologies affecting self-tolerance. Here we review the recent advances in differentiation of iPSc into TECs and the ability of the latter to support a proper and efficient maturation of thymocytes into functional and non-autoreactive T cells. A special focus is given on thymus organogenesis and pathway modulation during iPSc differentiation, on the impact of the 2/3D structure on the generated TECs, and on perspectives for therapeutic strategies in APECED based on patient-derived iPSc corrected for AIRE gene mutations.
Introduction
Immune tolerance is primarily set in the thymus with the generation of non-autoreactive T cells originated from the maturation of thymocytes through intimate interactions with specialized sets of thymic epithelial cells (TECs). These complex interactions enable the selection of maturing thymocytes for the functionality and non autoreactivity of their T cell receptors (TCR). The step of negative selection, which is responsible for the induction of thymocyte self-tolerance, is mediated by TECs that reside in the thymic medulla and express the autoimmune regulator (AIRE) (1). Loss-of-function mutations in the AIRE gene result in the rare autoimmune polyendocrinopathy candidiasis ectodermal dystrophy syndrome (APECED), a life-threatening autoimmune disease characterized by severe autoimmune lesions in peripheral tissues (2). The direct cause of this syndrome is the impairment of negative selection due to AIRE deficiency, resulting in the escape of autoreactive T cells into the periphery. Current treatments of APECED are only symptomatic including the administration of immunosuppressants, antifungal drugs with a constant monitoring of candidiasis infection, and hormone replacement. Although these therapeutics have improved the course of APECED, they don’t address the root cause of the disease, leaving the patients at risk of premature death (3). Induced pluripotent stem cells (iPSc) are a promising tool for the development of new cellular and genetic therapies for APECED. These cells are reprogrammed somatic cells obtained by the induced expression of four genes (OCT4/POU5F1, KLF4, SOX2, MYC) (4). The remarkable proliferation potential of iPSc and their ability to differentiate into various cell fates (5–7) have paved the way to promising therapeutic strategies aiming to restore tissue functions, notably in rare genetic diseases. Several iPSc-derived cell therapies against various pathologies such as cancer, autoimmune disorders or Parkinson’s disease are currently being evaluated by clinical trials (8–10). However, some concerns have been raised regarding the overall safety of stem-cell based therapies, since reprogramming of somatic cells can lead to enhanced mutation susceptibility and selection of deleterious mutations originally present in a minority of somatic cells. In addition, with the implication of OCT4 in tumorigenesis, the risk of inducing teratoma and malignant tumor formation is to be seriously considered (11). Thus, stringent quality controls of the generated iPSc and their differentiated products, as well as the fine purification of the populations of interest are crucial (12). This review covers the recent developments of iPSc differentiation into TECs, the different approaches used to mimic thymic embryological development and how it can provide new strategies for therapeutic application against APECED.
Thymus Function: T Cell Maturation and Selection
In the thymus, maturing T cells undergo several steps of expansion and selection mediated by TECs that account for 0.5% of the thymic cellularity in the adult thymus (13) (Figure 1). TECs form the backbone of the stromal compartment and interact with a large number of thymocytes (14). They have been historically separated into cortical TECs (cTECs) and medullary TECs (mTECs) located in the outermost and the core areas of the thymic lobules, respectively. These two populations exhibit different phenotypes and play distinct roles in the control of T cell maturation. cTECs drive the commitment of the early thymic progenitors (ETPs) to the T cell lineage by providing Notch ligands such as Dll4 (15). They also control ETP homing and expansion through the secretion of chemokines and growth factors such as Ccl25, Cxcl12, Scf and Il-7 (16). At the later double-positive (DP) stage, thymocytes with TCR that are able to recognize peptide:major histocompatibility complex (MHC) receive survival signals from cTECs and are thereby positively selected (Figure 1). The peptides presented by cTECs are generated by a unique proteasome comprising the cortical marker ß5t encoded by the Psmb11 gene (15, 17). After the positive selection, thymocytes undergo a step of negative selection aiming to deplete those with a TCR having a high avidity for self-antigen peptides. This step is mediated by mTECs that attract single-positive (SP) thymocytes through the secretion of chemokines like Ccl21 (18). The negative selection is enabled by the unique ability of mTECs to express 90% of the genome (19–21), including the expression of a broad repertoire of tissue-restricted antigen (TRA) genes under the regulation of transcriptional activators such as FEZF2, CHD4 and especially AIRE (22–24). The non-conventional activation factor Aire controls the expression of a large fraction of these TRAs (25, 26) and is specifically expressed in the mature subpopulation of mTECs showing high levels of the major histocompatibility class II (MHCII) molecule (mTEChi) and a high turnover. Importantly, the last decade has seen many aspects of the Aire-driven regulation of TRA expression being uncovered (26, 27). In mice, the expression of TRA genes follows a stochastic order: they are co-expressed in modules randomly present in individual mTEChi spread out in the thymic medulla. This pattern of expression enables a complete screening of the TRA repertoire by the thymocytes passing through the medulla. The intensity of the interactions between TRA peptide:MHCII complexes at the surface of mTEChi and the TCR is determinant for the fate of thymocytes (1). Thus, thymocytes with a strong self-reactive TCR will undergo apoptosis, while those harboring a self-reactive TCR with an intermediate strength will be directed into the natural T regulator (nTreg) lineage which plays a major role in peripheral immune tolerance (28) (Figure 1). At their final stage of maturation, the thymocytes will enter the periphery through the cortico-medullary junction vasculature, as recent thymic emigrants (RTEs) characterized by their expression levels of CD69, CD62L (SELL), Qa2 and CCR7 (29, 30) (Figure 1).
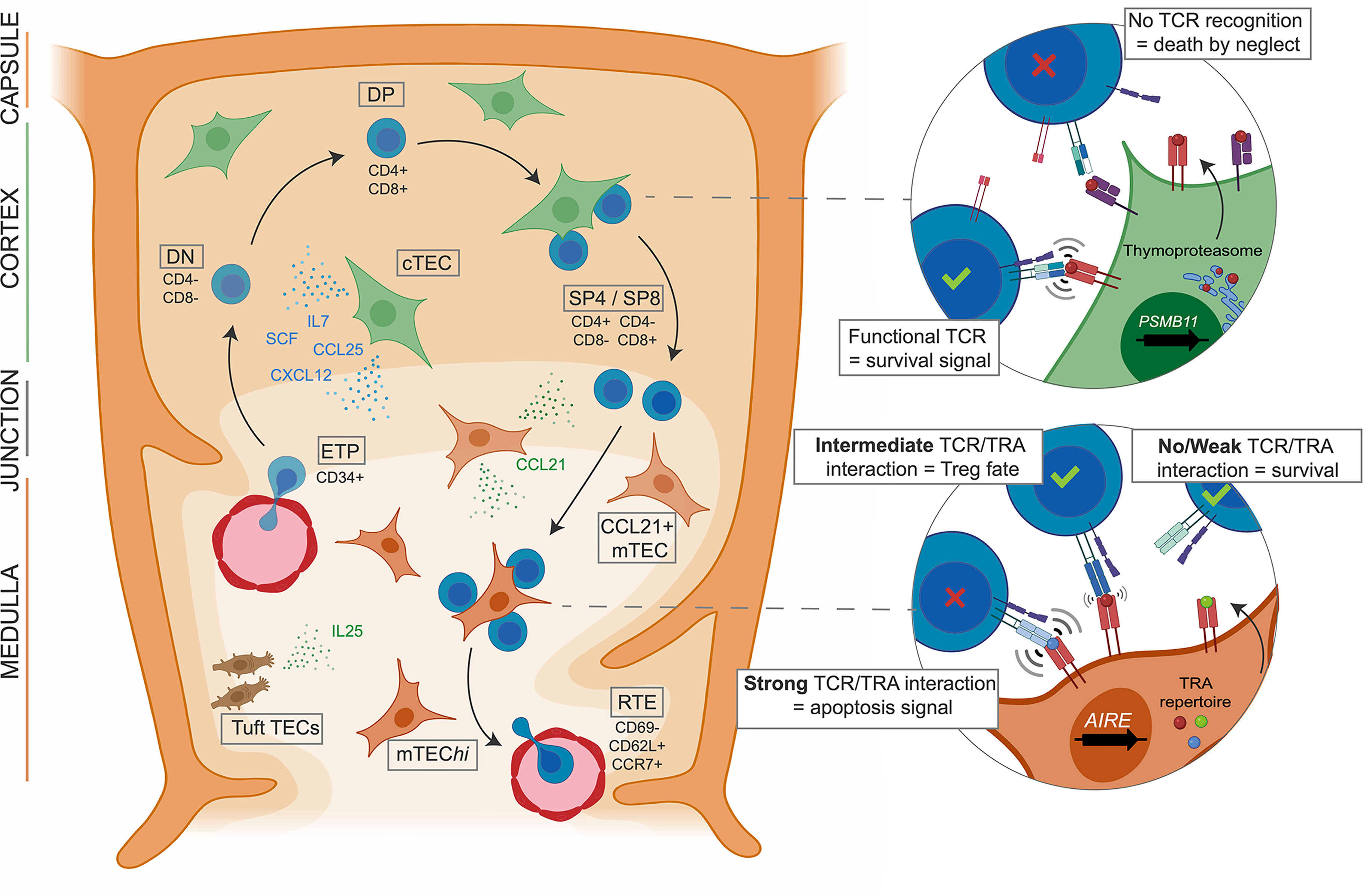
Figure 1 cTEC and mTEC orchestrate thymocyte maturation and selection. Early thymic progenitors (ETP) originating from the bone marrow enter the thymus and are attracted to the cortical region by chimiokines expressed by cTECs, also inducing growth and T lineage commitment by contact with NOTCH ligands. After TCR rearrangement CD4/CD8 DP thymocytes enter positive selection of the functional TCRs mediated by cTEC expressing the thymoproteasome. Resulting SP T cells are attracted to the medulla where thymocytes with auto-reactive TCRs are negatively selected by mature mTECs presenting the self-antigen repertoire under AIRE regulation. Thus, T cells with functional TCRs, able to recognize foreign antigens but tolerant to the self, are generated by the thymus.
TEC Heterogeneity and Maturation
From the homing of hematopoietic progenitors to the thymus to the escape of RTEs into the periphery, the maturation of T cells mostly relies on intimate interactions between thymocytes and TECs. These interactions are crucial for the maturation of TECs through a process referred to as thymic crosstalk (31–34). Indeed, TECs need to receive signals such as those mediated by Rankl and Cd40l to mature and survive (35). In recent years, advances in high-throughput single-cell (sc)RNA sequencing (scRNA-seq) have pushed further our understanding of TEC heterogeneity beyond the typical dichotomy between cTEC and mTEC in mice (16, 21, 36) and humans (37–39). Hence, new TEC populations were identified, notably populations composed of atypical tuft cells sharing similarities with intestinal tuft cells (16), myoid-like epithelial cells or neuroendocrine epithelial cells (37). While these populations are well characterized at the transcriptomic level, their functional role in the thymic microenvironment and their potential effect on thymocyte development remain elusive.
scRNA-seq studies of TEC heterogeneity in individuals of different ages also contributed to reveal the existence and identity of a TEC progenitor (TEP) population (36, 38). TEPs exhibit a cortical phenotype characterized by the expression of CD205 and ß5T (encoded by the LY75 and PSMB11 genes, respectively) (40–42). They were shown to be the source of mTECs and cTECs in fetal and neonatal thymuses (14, 43, 44). However, there is still a lack of evidence supporting this model in adults. After birth the thymus undergoes involution with a drastic decrease of its activity and cellularity, and shows a shift of the relative cTEC vs mTEC abundance in favor of the mTEC compartment (45, 46). In addition, it is assumed that TEPs enter quiescence in response to BMP4 and Activin A inhibitor follistatin (FSH) signaling (37, 47, 48). Thus, the emerging model for the origin of TECs relies on bipotent cTEC-like fetal TEPs entering quiescence upon aging and giving rise to lineage-restricted immature populations for the replenishment of medullary and cortical TEC compartments. However, additional studies based on single-cell fate-mapping need to be carried out to precisely understand the relationship between TECs and their progenitors upon aging. Another question left unanswered is the nature of signals underlying the medullary or cortical orientation of bipotent TEPs.
Promising results have been obtained over the last years, notably describing the role of Notch modulation in this fate decision (37, 49, 50). Indeed, Notch signaling has been shown to be essential for the mTEC specification of TEPs, notably through its fine-tuned regulation by the chromatin regulator HDAC3 (51). Thus, these findings support a model of a cTEC-like bipotent TEP population undergoing a default cortical differentiation, with Notch signalization promoting the mTEC transcriptional program. Despite the main paradigm of a TEC compartment of exclusive endoderm origin, Chakrabarti et al. recently showed that a population of bone-marrow hematopoietic progenitors transdifferentiate in true Foxn1-expressing TECs in the thymus (52). These hematopoietic bone marrow progenitors would migrate in the same manner as Cd34+ thymus seeding progenitors but would further differentiate into epithelial cells expressing cytokeratins and the master regulator of TEC development Foxn1. Moreover, Cd45+ Epcam+ cell could also give rise to Fsp1-expressing thymic fibroblasts. Thus, this fate mapping study identify a bone-marrow originating population able to transdifferentiate into TECs and fibroblasts to replenish the thymic stroma, suggesting that the TEC lineage development is more plastic than previously thought and may involve various progenitor populations originating from different embryological layers. However, proper characterization studies using high-throughput omics are still needed to precisely describe this newly identified cell population.
We mainly focus here on the role of TECs in thymocyte selection given the origin of APECED. However, other cell populations in the thymus have been shown to participate to this process. Indeed, dendritic cells (DC) have also the ability to induce clonal deletion and Treg generation (53–55). Different processes involving multiple DC subpopulations have been described. Briefly, migrating DCs can transport peripheral antigens to the thymus, negatively select thymocytes and induce Tregs (56, 57). Transendothelial DCs located in thymic vasculature capture blood circulating antigens and use them for selection (58, 59). Another source of antigens for DCs directly come from the thymic stroma through a mechanism of intercellular antigen transfer from the TECs (60, 61). Lymphotoxin β receptor (LTβR) expressed by mTECs has been shown to be central in this interaction by controlling the frequency and composition of intrathymic DC populations (62). DCs could also process self-antigens produced by thymic fibroblasts as a complementary source of self-antigens (63). Often neglected, thymic fibroblasts are now revealed as a crucial actor of the thymic microenvironment, with distinct subpopulations involved in functions as diverse as self-antigen production and regulation of both TEC and T cell maturation (63–65). Finally, these alternative sources of autoantigens or presenting cells are supported by studies in which disrupted thymuses with low TEC cellularity and morphological anomalies have no effect on mature T cell population frequency nor on the repertoire diversity (66).
Thymus Organogenesis
The first advances in the understanding of thymus organogenesis relied on comparative anatomical observations and histology studies of fetal tissues. These approaches revealed that the thymus is derived from the pharyngeal pouches that are transitory embryonic structures appearing between the third and fourth week of development in humans (67) and from E8 in mice (68, 69) (Figure 2). The pharyngeal pouches are invaginations originating from the most anterior foregut endoderm (67, 70, 71). It was shown in human fetuses that the thymus mostly derived from the third pharyngeal pouch (3PP) (72, 73). However, the embryological layer of origin of the thymus has long (74, 75) been uncertain. In the early 2000s, the single endodermal origin was demonstrated after ectopic transplantation experiments of pharyngeal endoderm proving that it is sufficient to give rise to a fully formed and functional thymus (68, 76).
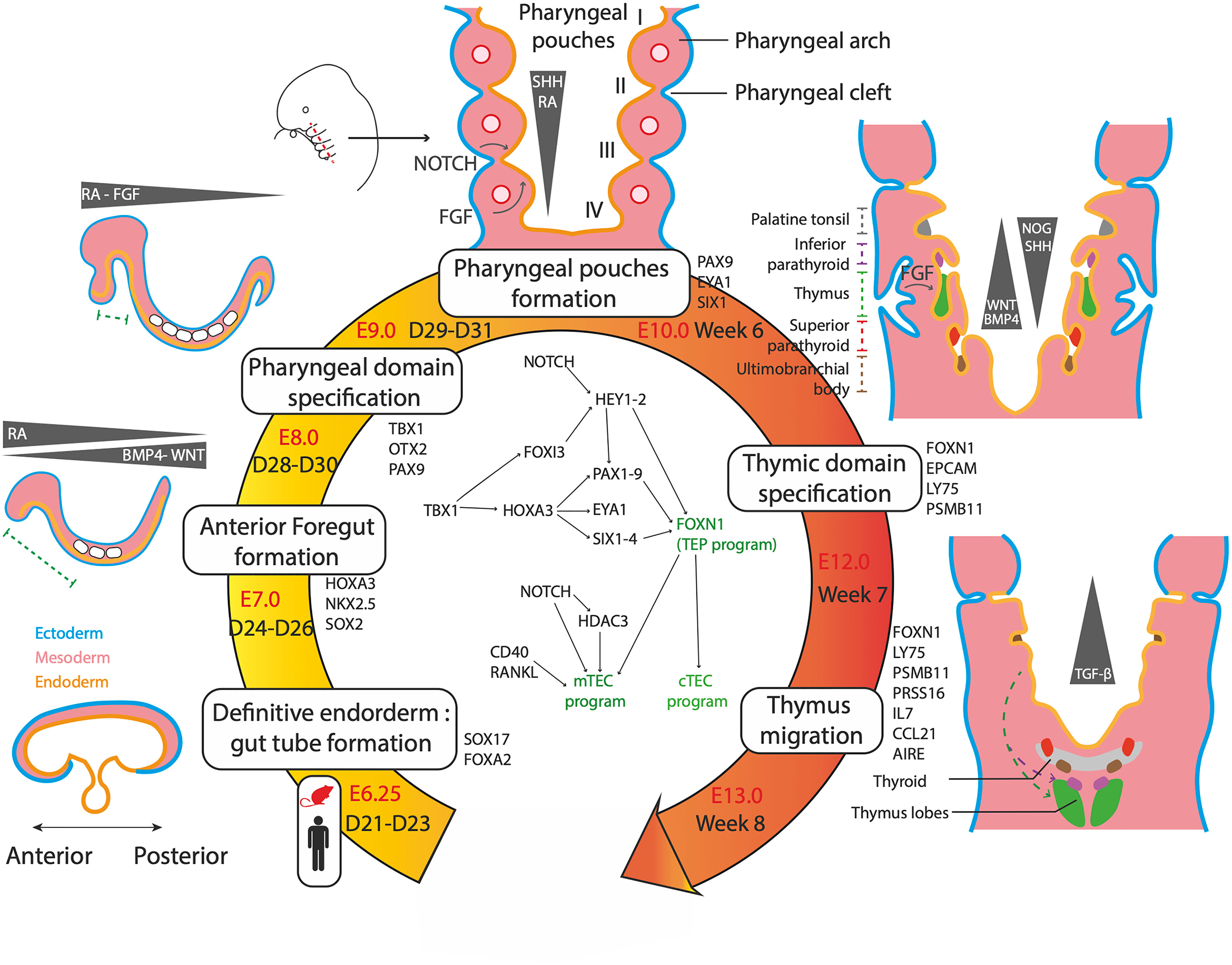
Figure 2 Genetic and molecular networks regulating the thymus organogenesis. The thymus is a definitive endoderm derivative. The main steps of organogenesis of the pharyngeal apparatus and thymus formation are described, with time scales in mouse and human, key markers genes and signalling molecules. First, gradients of cytokines and small molecules pattern the endoderm, resulting in an anterior domain between D24-D26 in human. Further patterning results in formation of the pharyngeal domain and its segmentation in pharyngeal arches at 6 weeks. The 3PP forms under singling involving retinoic acid (RA), Hedgehog (SHH), NOTCH and fibroblast growth factors (FGF). 3PP gives rise to the thymic primordium expressing FOXN1 by week 7. By week 8, the thymic primordium migrates under TGFβ signaling to its final position and TEC mature in cTEC and mTEC.
Although thymus organogenesis has been well described in mice (74, 75) the precise cellular and molecular mechanisms governing human thymus development are still elusive. In humans, the thymus forms from the 3PP endoderm at week 7 of development and initiates migration at 8.5 week (77). Involution of the 3PP endoderm results in a stratified epithelium of Cldn3/4-expressing cells polarized around a central lumen (78, 79) showing an early morphogenesis similar to other organs with epithelia organized in branching ducts, such as lung or pancreas. However, the definitive histological structure of the thymus is radically different, with formation of a 3D network of TECs that is far from the stratified epithelium constituting a branching architecture. This key aspect of thymic functionality, allowing the maximization of contacts between thymocytes and TECs, is mainly explained by the expression of the main TEC marker, Foxn1. This gene was first identified as the nude gene, originally described in the eponym hairless mice mutants exhibiting an absence of functional thymus (80). In mice, Foxn1 expression is detected in the 3PP endoderm as early as E9.5 and reaches high levels at E11 (80, 81). However, Foxn1 is not directly responsible for the commitment to thymic epithelial cell fate, since ectopically transplanted E9 3PP tissues, which do not express Foxn1 yet, are still able to generate fully developed thymuses (68). Nude mice show normal thymic primordium formation and migration but impaired maturation of the TEC compartment and consequently of T cell colonization (82). Thus, Foxn1 may be downstream of a regulatory network driving the commitment to thymic epithelial progenitors but would play a central role in the differentiation of TEPs into TECs (44, 68, 80, 83). Thymus rudiments from Foxn1 deficient mice shows an atypical branching structure, with formation of multiple lumens giving rise to a fully develop ductal system similar to the pancreas. In addition, ectopic expression of Foxn1 results in impaired epithelium formation and absence of lumen (84), showing Foxn1 ability to disrupt the classical tubular morphogenesis program. Finally, Foxn1 has been shown to be necessary for the expression of a full set of factors that control TEC transcriptional programs, such as Cxcl12, Ccl25, Dll4 and MHCII genes (85). More recently, Foxn1 has also been shown to control the expression of Cd40, Cd80, Aire and FgfrII that are crucial for TEC differentiation, amplification and function (85–87). Thus, by inhibiting tubulogenesis of the thymic epithelium and inducing expression of key genes of the TEC program, Foxn1 allows the structuration of the thymic environment and TEC generation (88).
Molecular Regulatory Networks in 3PP and Thymus Organogenesis
A complex interplay between the neural crest cells, the mesoderm-derived mesenchyme and the 3PP endoderm controls the fate, migration and expansion of cell populations in the developing thymus. The main genetic regulatory network is composed of the TBX1-HOXA3-PAX9 and EYA1-SIX1 cascades that are regulated by a set of signaling molecules secreted by the neural crest and mesoderm cells, such as retinoic acid (RA), proteins of the Wingless-int (WNT) family, bone morphogenetic proteins (BMP), fibroblast growth factors (FGF) and sonic hedgehog (SHH) proteins (Figure 2).
These factors, which are secreted by the mesoderm core and the neural crests in addition to the endoderm, guide the development of the thymic primordium. RA is a small non-peptidic vitamin A derivative that plays a key role throughout the embryonic development (89, 90). Gradients of RA have been shown to regulate the posterior pouch formation in several species (91–93). Disrupting RA activity during the development results in the absence of formation of posterior pharyngeal pouches (91, 93, 94). Moreover, RA was shown to be a key player in the early formation of pharyngeal pouches by regulating the expression of genes of central importance in their development, such as TBX1, HOXA3, PAX1 and PAX9 (85, 87, 89, 90, 95) (Figure 2). Proteins of the WNT family, including WNT4b and WNT5a (96–98), are expressed in the pharyngeal pouches and lead to the upregulation of FOXN1 by activating the canonical WNT/beta catenin pathway (96). Thus, modulation of the WNT signaling is critical to the formation of the thymic primordium and the maintenance of the thymic postnatal epithelium (97, 99, 100). Several studies have shown that genes of the WNT family are down-regulated in aged involuted thymuses (101–103) and are expressed in TECs under FOXN1 positive regulation (86, 100). However, a strong WNT signaling is detrimental to the thymic development (104) highlighting the importance of a proper modulation of WNT signals in TEC physiology. In mice, modulation of the Bmp pathway through a Bmp4-Noggin gradient in the thymic and parathyroid primordia, is responsible for a correct organ separation and thymic capsule formation (105, 106). Bmp4 has been shown to directly upregulate Foxn1 and FgfrIII (107). BMP signaling is thus closely integrated into FGF pathways that have been shown to play a crucial role in 3PP and thymic development (108, 109). Indeed, mutant mice for Fgf8 (110) and Fgfr2-IIIb, a receptor of Fgf7 and Fgf10 (87) show an impaired thymus development and arrest of TEC maturation. In zebrafish, the secretion of FGF8 in closeby mesoderm directs 3PP formation. However, later FGF signal inhibition through Spry is also necessary for thymic primordium migration and TEC proliferation (111). Similarly to the above molecules, Hedgehog plays multiple regulatory roles in the thymus. During the later steps of TEC maturation, SHH negatively impacts TEC proliferation but stimulates MHCII expression (112). SHH expression is restricted to the anteriormost pharyngeal apparatus, both in the endoderm and mesoderm, and plays a role in pharyngeal pouch patterning (113). At E10.5 SHH endorses a dorsalizing role, contrasting with the ventral thymic fate instructed by BMP4. These clues, added to the fact that SHH endodermal expression represses FOXN1, indicate that SHH signal inhibition is necessary to promote a thymic over parathyroid fate (114, 115). Overall, all these factors are involved in an integrated regulatory network orchestrating the specification, maturation and migration of the pharyngeal pouch derivatives.
Axis Patterning of the Definitive Endoderm and Emergence of the Foregut
Since the 3PP is a structure of the pharyngeal domain that is the anterior-most segment of the foregut endoderm, the identification of the molecular signals that sustain definitive endoderm patterning is a prerequisite for the control of the first steps of iPSc differentiation towards TECs. In mice, definitive endoderm specification is initiated during the gastrulation at E6.25, after which morphogenetic processes occur leading to the formation of the tubular gut structure by E8.0 (116) (Figure 2). Most of the pathways involved in pharyngeal pouch formation have also been shown to orchestrate the antero-posterior patterning of the gut tube. Indeed, it is now well established that the Bmp-Wnt-Fgf signaling has a posteriorizing effect on endoderm development and that its inhibition is required for the acquisition of foregut identity (117–119). In addition, the TGFß/Nodal inhibition and the RA signaling are also involved in the anteriorization of the endoderm (117). Recently, scRNA-seq datasets of foregut endoderm have been generated in mice between E3.5 and E12.5 (116, 120–122), including foregut mesoderm (116) and pharyngeal endoderm (122) lineages, thereby constituting atlases of endodermal and mesodermal cell populations involved in pharyngeal development. Hence, sets of genes specific to different stages of pharyngeal development could be identified, such as Pax9 and the Eya1-Six1 cascade for pharyngeal endoderm at E9.5 (Figure 2). More generally, these studies provide a model of pharyngeal endoderm development of unprecedented precision. In the case of the thymus, an early ventral foregut endoderm population expressing Nkx2-3 and 2-5 at E8.5 gives rise to the ventral pharynx expressing Bmp4 at E9.5 (116). It appeared that the key pathways involved at this stage of differentiation include FGF, NOTCH and RA signals arising from the surrounding mesoderm, as well as BMP autocrine ligands. Among the previously unrecognized pathways involved in anterior foregut development are those driven by HIPPO (121), EGF and NGF (120). At E10.5, the 3PP undergoes its formation upon activation of Eya1 and Six1. This cell population further differentiates into progenitors of parathyroid, ultimobranchial bodies and TEPs that begin to express Foxn1 at E11.5 and eventually give rise to early cTEC and mTEC populations at E12.5 (122).
Comfortingly, the same populations and signaling pathways are found in comparative studies with human embryos, validating that foregut endoderm organogenesis is conserved between mice and humans (77, 120). Finally, these studies provide key insights into which signaling pathways need to be modulated to direct differentiation of pluripotent human cells into TECs, even if most of the results presented above need to be confirmed by proper in vivo knock-out or lineage tracing studies.
Differentiation of Pluripotent Stem Cells Into the Thymic Lineage
Thanks to the growing understanding of signals driving the formation of the thymus, considerable progress has been made in the differentiation of pluripotent stem cells into the thymic epithelial lineage. We hereby review the different approaches, their achievements and limitations. A pioneer work was carried out by Lai & Jin, who successfully differentiated mouse embryonic stem cells (mESc) into cells showing a TEP phenotype (123) (Figure 3). Using a combination of FGFs, EGF and BMP4, they obtained a 24% proportion of EPCAM-positive cells after 10 days in a monolayer culture system. Despite this low proportion, EPCAM+ cells showed the expression of the TEP markers Pax1, Pax9 and Foxn1. These induced TEPs were able to successfully reconstitute a cortical and medullary compartment 6 weeks after engraftment under the kidney capsule, confirming their nature of bipotent progenitors. This regenerated thymus contained TCRαß+ CD3+ thymocytes expressing CD4 and CD8 in similar proportions than in native thymus.
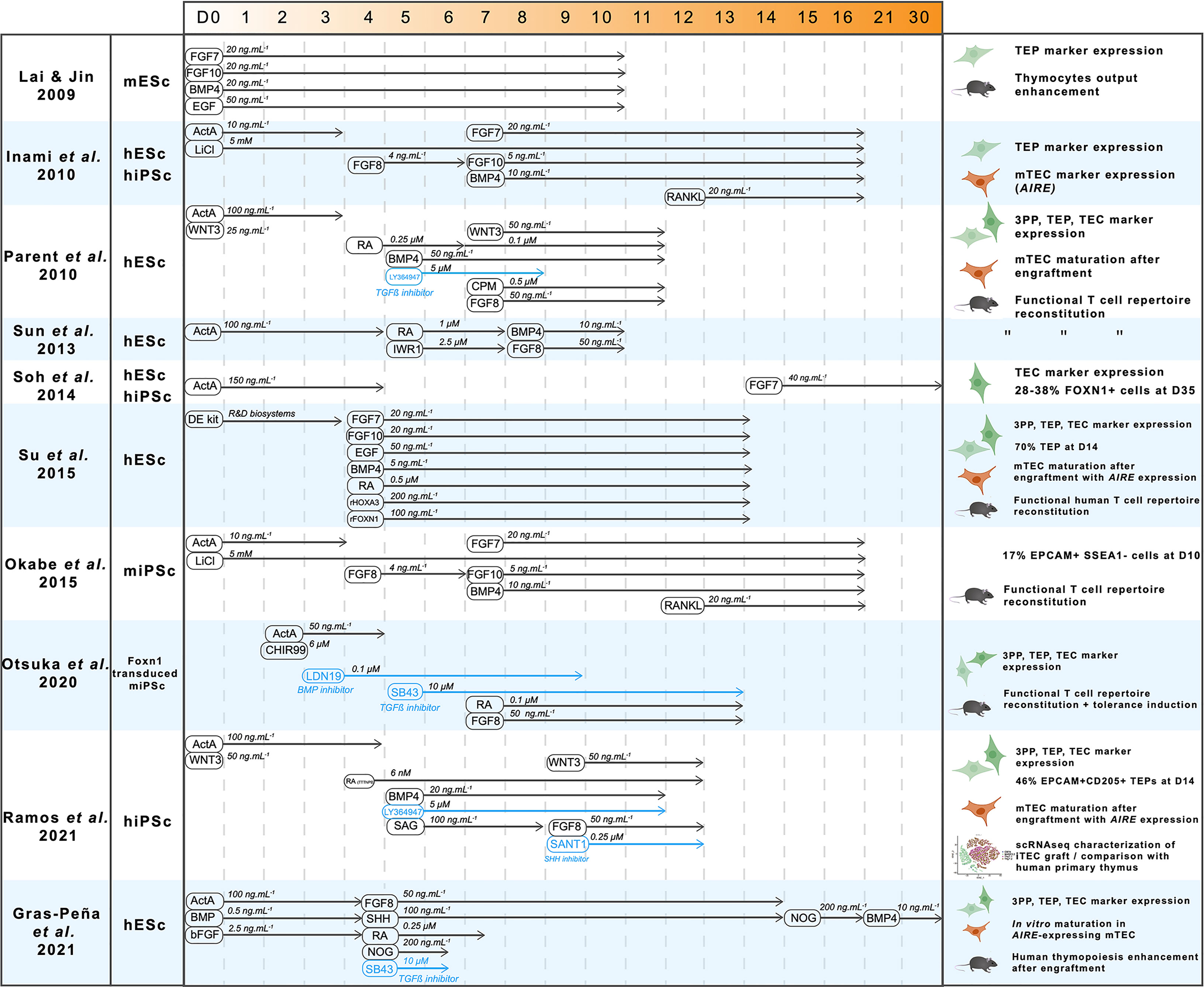
Figure 3 A synthesis of pluripotent stem cells thymic differentiation strategies. A decade of advances in thymic differentiation resulted in perfected protocols allowing production of mature mTEC by mimicking the thymic organogenesis in vitro. For each reference, cell type used, pathway-modulating molecules timings and concentrations are indicated, as well as results in terms of nature of the obtained cells and their ability to induce T cell maturation. ActivinA (ActA), Fibroblast growth factors 7,8,10 (FGF7-8-10), Bone Morphogenetic Protein 4 (BMP4), LiCl (Lithium Chloride), Retinoic acid (RA), Cyclopamine (CPM, hedgehog inhibitor), Sonic Hedgehog (SHH), LY364947 (TGFβ inhibitor), SB43 (TGFβ inhibitor), Wingless family member 3 (WNT3), IWR1 (WNT inhibitor), Epidermal Growth Factor (EGF), CHIR99 (WNT3 agonist), LDN19 (BMP inhibitor), Noggin (NOG), SANT1 (Hedgehog inhibitor), Smoothened Agonist (SAG, Hedgehog agonist).
Further advances were made by Inami et al. one year later (124). Using human iPSc and by adding RANK ligand (RANKL) to an optimized cytokine cocktail at day 12, they not only reproduce the differentiation into TEP with protein expression of their markers Pax1, Krt5, and Notch ligands DLL4 and DLL1, but for the first time they detected low levels of AIRE expression indicating further maturation of TEPs into mature mTECs. However, the functionality of this mTEC-enriched differentiated population was not addressed in this study, nor its heterogeneity which is an important parameter to control since a significant proportion of unwanted cell lineages are expected to arise from this differentiation and hinder inter-experimental reproducibility. In addition, the poor understanding of late TEC development and the lack of specific markers were major obstacles for a thorough analysis of the differentiated cells. To identify a combination of cell surface markers that are specific to TEPs, Soh et al. designed hESc reporter lines with a GFP cassette inserted into the exon 2 of the FOXN1 gene locus (125). Surprisingly, the differentiation of these hESc was successfully performed using a simple protocol consisting of exposure to Activin A for the first 4 days and supplementation of FGF7 from day 14 in embryoid bodies (Figure 3). Depending on the cell line, this protocol resulted in a strong proportion of GFP-positive cells (27-37%) after 35 days. Consistent with engagement in the thymic epithelial lineage, this GFP+ population was positive for TEC-specific markers such as KRT5, KRT14 and Notch ligands JAG2 and DLL4. However, characterization of the differentiated cells has not been performed further, leaving unanswered their maturation stage as well as their cortical and medullary identity. In addition, these cells were not able to support thymopoiesis since coculture with CD34+CD7+ proT cells failed to result in thymocyte differentiation.
Most of the progress made on the differentiation of pluripotent stem cells into TECs came from the studies of Parent et al. and Sun et al. that sought to optimize the differentiation protocol by monitoring the expression of markers of the intermediate foregut and pharyngeal pouch endoderm stages after cultivating hESc with different combinations of factors (126, 127) (Figure 3). These two studies showed that RA is needed for anteriorization of definitive endoderm, as well as BMP4 and WNT3 for acquisition of TEP identity. In addition, inhibition of WNT by IWR1, of TGFß by LY364947 at day 5, and Hedgehog by Cyclopamin (CPM) from day 7 to day 11 was shown to be required to increase FOXN1 expression. However, absence of unbiased experimental design did not allow an unambiguous and accurate identification of the effect of each factor on thymic differentiation, likely resulting in suboptimal results. Both protocols resulted in a significant upregulation of markers of thymic identity. However, no markers of TEC maturation were detectable. The induced TEPs were reaggregated and transplanted into nude mice to maturate further as described by Lai & Jin. The graft matured and was able to support thymopoiesis and reconstitute peripheral blood T cell compartments (127). Spectratype analysis of the TCR repertoire showed increased TCR Vß rearrangement diversity in mice engrafted with hESc-derived TEPs compared with controls (127). CD4+CD25+FOXP3+ Treg were also detected in engrafted mice (127). Importantly, the T cells generated in engrafted nude mice were functional showing IL2 secretion and proliferation after stimulation. They were also able to reject skin grafts. Since this model relies on cross-species reactivity, the same experiments were carried out in humanized mice with human hematopoietic progenitors. Similar results were obtained, therefore confirming the ability of thymic grafts to induce human T cell generation.
Additional approaches based on different strategies than directed differentiation, showed that the key factor FOXN1 was sufficient to induce TEC differentiation. First clues came from the study of Su et al., showing that culturing hESc with recombinant HOXA3 and FOXN1 resulted in significantly increased TEP yield (128). Additional evidence came from the reprogramming of fibroblasts by FOXN1 over-expression (86) showing that it is sufficient to drive differentiation towards the thymic epithelium fate with large, polygonal cells resembling TECs and expressing factors that sustain thymocyte development such as DLL4 and CCL25. These induced TECs (iTECs) were also able to mature ETPs into CD4+ and CD8+ SP T cells, both in vitro after 12 days of coculture and in vivo after engraftment in mice. In these mice, T cell functionality was confirmed with increased IL2 secretion in response to CD3/CD28 stimulation. These findings revealed that TECs can be generated from fibroblasts through the sole overexpression of FOXN1 thus highlighting a key role of this transcription factor in driving the TEC fate program. Together with the advancement of the delineation of the regulatory cascade inducing thymic fate, these results confirm that FOXN1 is necessary and sufficient for the induction of the TEC program, even if it does not induce thymic fate by itself. However, the reliability of the reprogramming approach to reconstitute the TEC compartment with its full heterogeneity needs to be evaluated and compared to the directed differentiation of iPSc that mimics thymus organogenesis. Several other studies have replicated these results, through directed differentiation alone (129) or in conjugation with Foxn1 overexpression (130, 131) and raised the question of induction of immune tolerance. As expected, Foxn1 overexpression in mouse iPSc significatively enhances the differentiation into TEPs, resulting in cells that express TEP markers Pax9, Dll4 and Foxn1 (129, 130) and undergo a proper TEC maturation after engraftment in mice. The effect of these iPSc-derived thymic grafts on immune tolerance has been studied by grafting skin biopsies from the same donor mouse strain from which the iPSc were generated in a recipient of another mouse strain after immune depletion by irradiation and anti-T antibody treatment (130) or directly in nude mice (129). Interestingly, recipient mice with a B6-derived thymic graft showed increased B6 skin graft survival. However, the cellular mechanisms leading to the induction of self-tolerance after iPSc-derived thymic graft needed to be more thoroughly examined.
More recently, comprehensive studies came out and pushed this topic further. Indeed, Ramos et al. and Gras-peña et al. optimized the differentiation protocols, notably by modulating temporal Hedgehog signaling (132, 133) (Figure 3). Interestingly, both protocols include Hedgehog activation during the step of pharyngeal endoderm induction, contrary to what was done in a previous report (126). Hence, the fine temporal modulation of the Hedgehog-specific pathways may be key to trigger a proper differentiation towards pharyngeal endoderm. Other examples of temporal modulations are also observed with the inhibition of BMP through Noggin between day 15 to day 21 followed by a more classical activation of BMP4 from day 21, resulting in a significant 10-fold increase of PAX9 expression (133). Another key insight from this study was the addition of FGF8b during endoderm anteriorization at day 4.5 which results in a 5-fold increase of FOXN1 expression. Remarkably, TECs derived from hESc following this protocol could be maintained for up to 30 days in classical 2D culture and they expressed the thymic markers FOXN1, PAX9, EYA1, SIX1 and AIRE at similar levels than in the human fetal thymus. Another crucial aim is the characterization of the induced TECs at the single-cell level. After a directed differentiation protocol yielding 46% of TEPs (EPCAM+ CD205+) at day 14, Ramos et al. reaggregated the cells and perform their engraftment in nude mice. After 14 to 19 weeks, the thymic grafts were analyzed by bulk and scRNA-seq. Confirmation of further maturation in TECs was provided by high levels of expression of HLA-DRA and DLL4. Remarkably, the scRNA-seq data of these grafts showed a common clustering of TECs derived from iPSc with primary TECs from postnatal thymuses. However, subclustering of the TEC population revealed a distinct separation between the two types of samples, with induced TECs mainly composed of TEPs and differentiating TECs, while cells from the more mature cTEC and mTEChi clusters were originating from the primary samples.
These data shed a new light on the mechanisms leading to TEP differentiation, notably in pointing out the roles of Activin A and the Notch pathway detected by the expression of INHBA and DLK1 in the TEP cluster. This direct differentiation protocol allows precise differentiation of iPSc into TEPs that mature into functional TECs in vivo with a transcriptomic profile close to primary TECs. Further application of scRNA-seq techniques to TEC differentiation could lead to a deeper understanding of the mechanisms driving the generation of the diverse TEC populations and leverage this new knowledge to differentiate specific TEC subpopulations.
Together, TEC differentiation from pluripotent stem cells has shown significant improvement in recent years with a continuous improvement of the protocols giving rise to TEPs, with greater yield and purity. Conversely, less progress was achieved in the approaches aiming to obtain mature TECs from TEPs. Regarding this stage of maturation, important clues came from the study of the thymic crosstalk with the finding that the interactions between TECs and developing thymocytes are necessary to the maturation of TECs. It was also shown that the thymic crosstalk could be mimicked in vitro using cytokines including but not restricted to RANKL. At a functional level, these induced TECs (iTEC) can support the maturation of thymocytes and reconstitute the T cell compartment in vivo. T cells cocultured with iTEC proliferate and secrete cytokines after stimulation. They are also able to improve skin graft rejection, thus showing evidence of functionality. Interestingly, nTreg can also be generated by iTEC grafts and induce immune tolerance to syngeneic skin grafts.
Although all the above protocols succeeded in generating TEPs with various efficiency, considerable obstacles still need to be addressed to obtain a functional thymic organoid in vitro. This highlights the fact that our current level of control over thymic iPSc differentiation is still incomplete and that most of the modulated pathways in these protocols may not be necessary nor sufficient.
Heterogeneity of the directed differentiation products is also a crucial stake for clinical applications since undifferentiated iPSc can lead to teratoma formation after transplantation. Purification strategies and treatment by selective anti-iPSc molecules such as YM155 (133) are promising approaches to mitigate this risk. This differentiation heterogeneity could also counter-intuitively positively affect the generation of iPSc-derived TECs. As described above, multiple cell types collaborate for thymic functionality. Thus, a differentiation strategy yielding not only isolated TECs but also thymic fibroblast or even T progenitors and dendritic cells would improve TEC differentiation.
Finally, a long-term culture system accurately reproducing in vivo thymic microenvironment still needs to be developed. Although TECs derived from iPSc can be maintained in classical 2D culture for up to 30 days (133), a culture system closer to the 3D sponge-like structure of the thymic stroma could significantly improve TEC differentiation and viability.
3D Culture and Organoids
In classical 2D culture, primary TECs show a progressive loss of AIRE and FOXN1 expression (134) and of their ability to express the full set of TRA genes (135). Since the 3D structure is an important factor for TEC maintenance (136), recapitulating such an organization in culture systems for thymic differentiation could improve yields and viability of iTECs. Several 3D models have been developed and tested on primary TECs. One of them relies on the coculture of mTECs with dermal fibroblasts embedded in a fibrin hydrogel, allowing proliferation, phenotype conservation, and further maturation of these cells (135). In comparison to simpler 2D culture systems, this organotypic 3D culture allows mTECs to keep their primary ability to express TRA genes. Other synthetic hydrogels have been developed to reconstitute the thymic microenvironment, such as a self-assembling synthetic hydrogel formed by EAK16-II/EAKII-H6 peptides (137). This gel was shown to induce the organization of primary TECs in 3D clusters and maintain the expression of FOXN1 and EPCAM. After transplantation in nude mice, these hydrogel-embedded TECs supported the generation of T cell populations that were able to induce self-tolerance, as assessed by mixed leukocyte reactions. However, no cortico-medullary TEC segregation was observed in the reconstituted thymus, and the generated T cells were considerably biased toward CD8+ SP T cells. Additional systems were also generated to support the 3D culture of TECs, such as those based on fibronectin functionalized fibrous meshes (138), gelatin spheric microgels (139) or type I collagen hydrogel (140). Despite the various protein compositions of their matrices, these systems supported an enhanced proliferation, spreading and maintenance of the main TEC populations. However, the collagen hydrogel seeded with mice TEC did not seem to support thymopoiesis in vivo (140). Although synthetic hydrogels are a promising tool to culture TECs, more thorough research and optimization are needed to accurately mimic the thymic microenvironment.
An alternative approach to these synthetic systems is to directly use the primary thymus matrix to benefit from the native diversity of its constituting proteins and 3D structure. Decellularization of primary thymic lobes results in scaffolds that conserve the microstructure and the protein composition of the thymic microenvironment (141–143). These scaffolds support TEC growth and differentiation with the conservation of FOXN1 expression and the reformation of distinct medullary and cortical compartments. Moreover, after engraftment, the reconstituted thymuses can support the generation of functional T cells that can induce donor-specific tolerance in a skin graft model (141, 143). However, these approaches are dependent on a primary source of mouse or human thymuses, thus hindering clinical use.
Lastly, beside TEC differentiation, recent studies aiming to obtain functional T cells from hematopoietic progenitors or iPSc have also highlighted the importance of 3D culture systems to leverage obstacles in order to generate TEC organoids. Development of artificial thymic organoids (ATO) (144–146) has been achieved through reaggregation of a bone marrow stromal cell line engineered to express the Notch ligand Dll4 with either hematopoietic stem cells or mesodermal progenitors derived from iPSc. The cocultured cells formed a 3D structure cultured in an air-liquid interface. Using bone marrow stem cells from mice of three different backgrounds, the ATO system was able to consistently reproduce thymopoiesis, and generate mature single-positive CD4 and CD8 cells (145, 146). Remarkably, this system resulted in comparable results when using mesodermal progenitors derived from hESc and human iPSc (145). Thus, thymic organoids can recapitulate T cell differentiation in vitro, therefore showing the importance of 3D structure in comparison to less efficient 2D cocultures (147). However, CD4 single-positive T cell frequency was lower than expected, probably because of the absence of TECs in this system, resulting in an impaired MHCII signaling. Another crucial drawback is the conservation of numerous clones naturally eliminated in the thymus, illustrating the absence of negative selection in the ATOs.
Clinical Applications to APECED and Perspectives
Congenital pathologies affecting TEC development and function lead to severe conditions of autoimmunity or lymphopenia. APECED is caused by loss-of-function mutations in the AIRE gene resulting in a general autoimmune syndrome. Patients receive a personalized combination of treatments targeting the symptoms and leading to clinical improvements. However, no curative strategy is available yet, and APECED patients still risk premature death. Remarkably, the recent breakthroughs in TEC generation from iPSc lead to new perspectives for treatment of APECED (Figure 4A). Reprogramming patient cells and correcting the AIRE mutations through gene editing techniques would produce iPSc that could be used to regenerate 3D thymic tissues. Transplantation of these autologous engineered thymic tissues would restore thymic function and limit the risk of autoimmunity. Indeed, the transplanted tissues would be syngeneic and T cells generated would be educated to the patient’s autoantigen repertoire, reconstituting its immune system (Figure 4A).
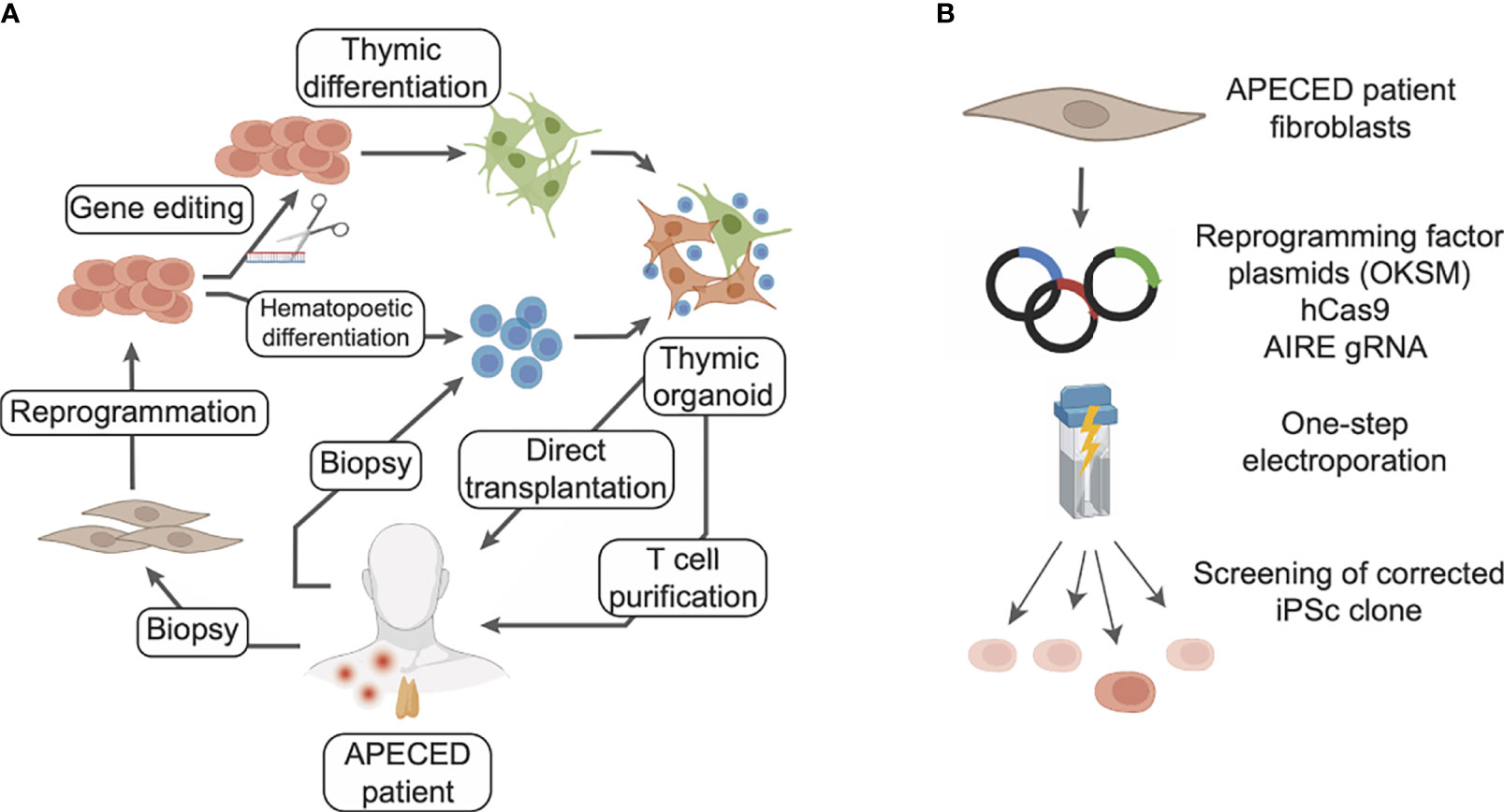
Figure 4 Potential cellular therapy strategies for treating APECED with induced TECs to restore thymic functionality. (A) Fibroblasts from the patient can be reprogrammed to iPSc and the defective gene corrected with gene editing tools. Differentiation of these iPSc into thymic epithelial cells, in combination with T cell progenitors differentiated from the same iPSc or directly purified from patient blood, would allow generation of artificial thymic organoids. These organoids could be either directly transplanted or used to generate competent T cells ex vivo to reconstitute the patient immune system. In this scenario T cell progenitors could be purified from patient blood or differentiated from patient iPSc. (B) Gene editing of a deficient gene from a patient can rely on CRISPR/Cas9 and be combined with reprogramming steps for the obtention of iPSc.
Reprogramming of somatic cells from APECED patients has been shown feasible despite the role that AIRE plays in the regulation of ESc pluripotency and in their self-renewal (148, 149). Indeed iPSc have recently been generated from APECED patient cells (150), showing that the AIRE R257X mutation does not impair cell reprogramming, iPSc proliferation and pluripotency. In this study, PBMCs from 2 female APECED patients were transduced by a Sendai virus vector, yielding 10 iPSc clones in which the AIRE mutation was confirmed. These iPSc show similar proliferation and expression of pluripotency markers than iPSc with functional AIRE, therefore validating the use of iPSc-based approaches in APECED. If AIRE has not been reportedly corrected in iPSc, this approach has been demonstrated in several models of monogenic pathologies, affecting diverse organs such as retina, kidney and liver (151–153). In these studies, the mutated genes RPGR, IFT140 and LDLR causing Retinitis pigmentosa, nephronophthisis and homozygous familial hypercholesterolemia, respectively, were corrected in iPSc from patient cells. The corrected iPSc were then differentiated into retinal organoids, kidney organoids or hepatocyte-like cells, all of them showing a rescued phenotype and functionality (151–153). The CRISPR/Cas9 system was used for gene edition in these 3 studies, delivering plasmids and Cas9 to the iPSc by electroporation (152, 153), or in a one-step protocol during reprogramming (151). In the latter, patient dermal fibroblasts are electroporated by two pulses at 1,400 V for 20 ms with a cocktail of plasmids coding for the reprogramming factors, a guide RNA for the target gene, its corrected sequence, and a spCas9-gem. This specific Cas9 variants have been developed for gene editing iPSc (154). Thus, given those converging clues, using CRISPR/Cas9 to edit AIRE in iPSc derived from APECED patients could be a valid curative strategy (Figure 4B).
Beyond APECED, other pathologies affecting the thymus are also caused by genetic defects. DiGeorges syndrome is caused by a microdeletion of the TBX1-containing chromosome region 22q11.2, nude SCID by mutations in the FOXN1 gene and Otofaciocervical Syndrome type 2 in the PAX1 gene (155). These conditions lead to partial or total athymia and life-threatening lymphopenia. Curative treatments of these diseases could also rely on transplantation of autologous engineered thymic tissues to restore thymic function while limiting the risk of autoimmunity. Nonetheless, a crucial limitation is the long lapse of time needed to reprogram iPSc and to differentiate them into functional thymic tissue. Applied to APECED, early diagnosis would be crucial, since this approach cannot cure the damage already caused by autoimmunity, even though promising immunotherapies are emerging to treat autoimmune manifestations (156). For pathologies causing lymphopenia, early diagnosis would also be vital to limit the risk of potentially lethal infections during the time needed to generate the engineered thymic tissues. To envision the use of such therapies, additional challenges would need to be met with the need to develop clinical-grade differentiation protocols not relying on any xenogenous reactives and based on well-accepted synthetic hydrogels for 3D culture. Finally, the risk of transplanting iSPc-derived TECs should be carefully assessed to limit teratoma formation, with purification of differentiated cells and anti-pluripotency treatment.
Because of the complex interactions between cell populations in the thymus, the crucial importance of its 3D organization and the still-improving understanding of TEC biology, generation of in vitro culture systems closely reproducing the thymus is a major challenge. In recent years, great advances have been made in the understanding of thymus organogenesis, the generation of TECs from pluripotent stem cells and 3D culture systems. These complementary progress will very likely result in preclinical applications for the treatment of pathologies affecting T cell development in the thymus.
Author Contributions
NP and MG designed/outlined the manuscript; NP wrote the manuscript and MG edited the manuscript.
Funding
NP was supported by “la fondation d’entreprise ProGreffe” and by the EJP-Rare Disease JTC2019 program TARID project (ANR-19-RAR4-0011-05) to MG.
Conflict of Interest
The authors declare that the research was conducted in the absence of any commercial or financial relationships that could be construed as a potential conflict of interest.
Publisher’s Note
All claims expressed in this article are solely those of the authors and do not necessarily represent those of their affiliated organizations, or those of the publisher, the editors and the reviewers. Any product that may be evaluated in this article, or claim that may be made by its manufacturer, is not guaranteed or endorsed by the publisher.
Abbreviations
AIRE, autoimmune regulator; APECED, polyendocrinopathy candidiasis ectodermal dystrophy syndrome; ATO, artificial thymic organoid; DN, double negative CD4-CD8- thymocyte; DP, double positive CD4+CD8+ thymocyte; ESc, embryonic stem cells; ETP, early thymic progenitor; iPSc, induced pluripotent stem cells; mTEChi, MHC-II high medullary thymic epithelial cell; nTreg, natural regulatory T cell; RA, retinoic acid; RTE, recent thymic emigrant; SCID, severe combined immunodeficiency; TCR, T cell receptor; TEC, thymic epithelial cells; TEP, thymic epithelial progenitor; TRA, tissue restricted autoantigen; 3PP, third pharyngeal pouch.
References
1. Kadouri N, Nevo S, Goldfarb Y, Abramson J. Thymic Epithelial Cell Heterogeneity: TEC by TEC. Nat Rev Immunol (2019) 20:239–253. doi: 10.1038/s41577-019-0238-0
2. Perheentupa J. Autoimmune Polyendocrinopathy-Candidiasis-Ectodermal Dystrophy. J Clin Endocrinol Metab (2006) 91(8):2843–50. doi: 10.1210/jc.2005-2611
3. Borchers J, Pukkala E, Mäkitie O, Laakso S. Patients With APECED Have Increased Early Mortality Due to Endocrine Causes, Malignancies and Infections. J Clin Endocrinol Metab (2020) 105(6):dgaa140. doi: 10.1210/clinem/dgaa140
4. Takahashi K, Yamanaka S. Induction of Pluripotent Stem Cells From Mouse Embryonic and Adult Fibroblast Cultures by Defined Factors. Cell (2006) 126(4):663–76. doi: 10.1016/j.cell.2006.07.024
5. Yoshida Y, Yamanaka S. Induced Pluripotent Stem Cells 10 Years Later. Circ Res (2017) 120(12):1958–68. doi: 10.1161/CIRCRESAHA.117.311080
6. Tukker AM, Wijnolts FMJ, de Groot A, Westerink RHS. Human iPSC-Derived Neuronal Models for In Vitro Neurotoxicity Assessment. NeuroToxicology (2018) 67:215–25. doi: 10.1016/j.neuro.2018.06.007
7. Kondo Y, Toyoda T, Inagaki N, Osafune K. iPSC Technology-Based Regenerative Therapy for Diabetes. J Diabetes Investig (2018) 9(2):234–43. doi: 10.1111/jdi.12702
8. Fate Therapeutics Announces FDA Clearance of Landmark IND for FT500 iPSC-Derived, Off-The-Shelf NK Cell Cancer Immunotherapy. Fate Therapeutics Inc (2021).
9. Parmar M, Grealish S, Henchcliffe C. The Future of Stem Cell Therapies for Parkinson Disease. Nat Rev Neurosci (2020) 21(2):103–15. doi: 10.1038/s41583-019-0257-7
10. Kumar A, Das JK, Peng HY, Wang L, Ren Y, Xiong X, et al. Chapter 14 - Current Development in iPSC-Based Therapy for Autoimmune Diseases. In: Advances in Stem Cell Biology, Birbrair A, editor. Recent Advances in iPSCs for Therapy. Academic Press (2021) , 3:315–338. Available at: https://www.sciencedirect.com/science/article/pii/B9780128222294000012.
11. Pan GJ, Chang ZY, Schöler HR, Pei D. Stem Cell Pluripotency and Transcription Factor Oct4. Cell Res (2002) 12(5):321–9. doi: 10.1038/sj.cr.7290134
12. Desgres M, Menasché P. Clinical Translation of Pluripotent Stem Cell Therapies: Challenges and Considerations. Cell Stem Cell (2019) 25(5):594–606. doi: 10.1016/j.stem.2019.10.001
13. Sakata M, Ohigashi I, Takahama Y. Cellularity of Thymic Epithelial Cells in the Postnatal Mouse. J Immunol (2018) 200(4):1382–8. doi: 10.4049/jimmunol.1701235
14. Abramson J, Anderson G. Thymic Epithelial Cells. Annu Rev Immunol (2017) 35:85–118. doi: 10.1146/annurev-immunol-051116-052320
15. Ohigashi I, Zuklys S, Sakata M, Mayer CE, Zhanybekova S, Murata S, et al. Aire-Expressing Thymic Medullary Epithelial Cells Originate From β5t-Expressing Progenitor Cells. Proc Natl Acad Sci (2013) 110(24):9885–90. doi: 10.1073/pnas.1301799110
16. Bornstein C, Nevo S, Giladi A, Kadouri N, Pouzolles M, Gerbe F, et al. Single-Cell Mapping of the Thymic Stroma Identifies IL-25-Producing Tuft Epithelial Cells. Nature (2018) 559(7715):622–6. doi: 10.1038/s41586-018-0346-1
17. Apavaloaei A, Brochu S, Dong M, Rouette A, Hardy MP, Villafano G, et al. PSMB11 Orchestrates the Development of CD4 and CD8 Thymocytes via Regulation of Gene Expression in Cortical Thymic Epithelial Cells. J Immunol (2019) 202(3):966–78. doi: 10.4049/jimmunol.1801288
18. Kozai M, Kubo Y, Katakai T, Kondo H, Kiyonari H, Schaeuble K, et al. Essential Role of CCL21 in Establishment of Central Self-Tolerance in T Cells. J Exp Med (2017) 214(7):1925–35. doi: 10.1084/jem.20161864
19. Sansom SN, Shikama-Dorn N, Zhanybekova S, Nusspaumer G, Macaulay IC, Deadman ME, et al. Population and Single-Cell Genomics Reveal the Aire Dependency, Relief From Polycomb Silencing, and Distribution of Self-Antigen Expression in Thymic Epithelia. Genome Res (2014) 24(12):1918–31. doi: 10.1101/gr.171645.113
20. Danan-Gotthold M, Guyon C, Giraud M, Levanon EY, Abramson J. Extensive RNA Editing and Splicing Increase Immune Self-Representation Diversity in Medullary Thymic Epithelial Cells. Genome Biol (2016) 17(1):219. doi: 10.1186/s13059-016-1079-9
21. Dhalla F, Baran-Gale J, Maio S, Chappell L, Hollander GA, Ponting CP. Biologically Indeterminate Yet Ordered Promiscuous Gene Expression in Single Medullary Thymic Epithelial Cells. EMBO J (2020) 39(1):e101828. doi: 10.15252/embj.2019101828
22. Takaba H, Morishita Y, Tomofuji Y, Danks L, Nitta T, Komatsu N, et al. Fezf2 Orchestrates a Thymic Program of Self-Antigen Expression for Immune Tolerance. Cell (2015) 163(4):975–87. doi: 10.1016/j.cell.2015.10.013
23. Tomofuji Y, Takaba H, Suzuki HI, Benlaribi R, Martinez CDP, Abe Y, et al. Chd4 Choreographs Self-Antigen Expression for Central Immune Tolerance. Nat Immunol (2020) 21(8):892–901. doi: 10.1038/s41590-020-0717-2
24. Björses P, Halonen M, Palvimo JJ, Kolmer M, Aaltonen J, Ellonen P, et al. Mutations in the AIRE Gene: Effects on Subcellular Location and Transactivation Function of the Autoimmune Polyendocrinopathy-Candidiasis–Ectodermal Dystrophy Protein. Am J Hum Genet (2000) 66(2):378–92. doi: 10.1086/302765
25. Anderson MS, Venanzi ES, Klein L, Chen Z, Berzins SP, Turley SJ, et al. Projection of an Immunological Self Shadow Within the Thymus by the Aire Protein. Science (2002) 298(5597):1395–401. doi: 10.1126/science.1075958
26. Giraud M, Yoshida H, Abramson J, Rahl PB, Young RA, Mathis D, et al. Aire Unleashes Stalled RNA Polymerase to Induce Ectopic Gene Expression in Thymic Epithelial Cells. Proc Natl Acad Sci U S A (2012) 109(2):535–40. doi: 10.1073/pnas.1119351109
27. Giraud M, Jmari N, Du L, Carallis F, Nieland TJF, Perez-Campo FM, et al. An RNAi Screen for Aire Cofactors Reveals a Role for Hnrnpl in Polymerase Release and Aire-Activated Ectopic Transcription. Proc Natl Acad Sci U S A (2014) 111(4):1491–6. doi: 10.1073/pnas.1323535111
28. Li MO, Rudensky AY. T Cell Receptor Signaling in the Control of Regulatory T Cell Differentiation and Function. Nat Rev Immunol (2016) 16(4):220–33. doi: 10.1038/nri.2016.26
29. Cowan JE, Parnell SM, Nakamura K, Caamano JH, Lane PJL, Jenkinson EJ, et al. The Thymic Medulla is Required for Foxp3+ Regulatory But Not Conventional CD4+ Thymocyte Development. J Exp Med (2013) 210(4):675–81. doi: 10.1084/jem.20122070
30. Teh CE, Daley SR, Enders A, Goodnow CC. T-Cell Regulation by Casitas B-Lineage Lymphoma (Cblb) is a Critical Failsafe Against Autoimmune Disease Due to Autoimmune Regulator (Aire) Deficiency. Proc Natl Acad Sci U S A (2010) 107(33):14709–14. doi: 10.1073/pnas.1009209107
31. Holländer GA, Wang B, Nichogiannopoulou A, Platenburg PP, van Ewijk W, Burakoff SJ, et al. Developmental Control Point in Induction of Thymic Cortex Regulated by a Subpopulation of Prothymocytes. Nature (1995) 373(6512):350–3. doi: 10.1038/373350a0
32. Irla M, Guerri L, Guenot J, Sergé A, Lantz O, Liston A, et al. Antigen Recognition by Autoreactive CD4+ Thymocytes Drives Homeostasis of the Thymic Medulla. PloS One (2012) 7(12):e52591. doi: 10.1371/journal.pone.0052591
33. Irla M, Hugues S, Gill J, Nitta T, Hikosaka Y, Williams IR, et al. Autoantigen-Specific Interactions With CD4+ Thymocytes Control Mature Medullary Thymic Epithelial Cell Cellularity. Immunity (2008) 29(3):451–63. doi: 10.1016/j.immuni.2008.08.007
34. Lopes N, Sergé A, Ferrier P, Irla M. Thymic Crosstalk Coordinates Medulla Organization and T-Cell Tolerance Induction. Front Immunol (2015) 6:365/abstract. doi: 10.3389/fimmu.2015.00365/abstract
35. Irla M. RANK Signaling in the Differentiation and Regeneration of Thymic Epithelial Cells. Front Immunol (2021) 11:623265. doi: 10.3389/fimmu.2020.623265
36. Wells KL, Miller CN, Gschwind AR, Wei W, Phipps JD, Anderson MS, et al. Combined Transient Ablation and Single-Cell RNA-Sequencing Reveals the Development of Medullary Thymic Epithelial Cells. Elife (2020) 9:e60188. doi: 10.7554/elife.60188
37. Bautista JL, Cramer NT, Miller CN, Chavez J, Berrios DI, Byrnes LE, et al. Single-Cell Transcriptional Profiling of Human Thymic Stroma Uncovers Novel Cellular Heterogeneity in the Thymic Medulla. Nat Commun (2021) 12(1):1096. doi: 10.1038/s41467-021-21346-6
38. Park JE, Botting RA, Domínguez Conde C, Popescu DM, Lavaert M, Kunz DJ, et al. A Cell Atlas of Human Thymic Development Defines T Cell Repertoire Formation. Science (2020) 367(6480):eaay3224. doi: 10.1126/science.aay3224
39. Zeng Y, Liu C, Gong Y, Bai Z, Hou S, He J, et al. Single-Cell RNA Sequencing Resolves Spatiotemporal Development of Pre-Thymic Lymphoid Progenitors and Thymus Organogenesis in Human Embryos. Immunity (2019) 51(5):930–948.e6. doi: 10.1016/j.immuni.2019.09.008
40. Alawam AS, Anderson G, Lucas B. Generation and Regeneration of Thymic Epithelial Cells. Front Immunol (2020) 11:858. doi: 10.3389/fimmu.2020.00858
41. Baik S, Jenkinson EJ, Lane PJL, Anderson G, Jenkinson WE. Generation of Both Cortical and Aire+ Medullary Thymic Epithelial Compartments From CD205+ Progenitors. Eur J Immunol (2013) 43(3):589–94. doi: 10.1002/eji.201243209
42. Alves NL, Takahama Y, Ohigashi I, Ribeiro AR, Baik S, Anderson G, et al. Serial Progression of Cortical and Medullary Thymic Epithelial Microenvironments. Eur J Immunol (2014) 44(1):16–22. doi: 10.1002/eji.201344110
43. Rossi SW, Jenkinson WE, Anderson G, Jenkinson EJ. Clonal Analysis Reveals a Common Progenitor for Thymic Cortical and Medullary Epithelium. Nature (2006) 441(7096):988–91. doi: 10.1038/nature04813
44. Bleul CC, Corbeaux T, Reuter A, Fisch P, Mönting JS, Boehm T. Formation of a Functional Thymus Initiated by a Postnatal Epithelial Progenitor Cell. Nature (2006) 441(7096):992–6. doi: 10.1038/nature04850
45. Chen L, Xiao S, Manley NR. Foxn1 is Required to Maintain the Postnatal Thymic Microenvironment in a Dosage-Sensitive Manner. Blood (2009) 113(3):567–74. doi: 10.1182/blood-2008-05-156265
46. O’Neill KE, Bredenkamp N, Tischner C, Vaidya HJ, Stenhouse FH, Peddie CD, et al. Foxn1 Is Dynamically Regulated in Thymic Epithelial Cells During Embryogenesis and at the Onset of Thymic Involution. PloS One (2016) 11(3):e0151666. doi: 10.1371/journal.pone.0151666
47. Lepletier A, Hun ML, Hammett MV, Wong K, Naeem H, Hedger M, et al. nterplay Between Follistatin, Activin A, and BMP4 Signaling Regulates Postnatal Thymic Epithelial Progenitor Cell Differentiation During Aging. Cell Rep (2019) 27(13):3887–901. doi: 10.1016/j.celrep.2019.05.045
48. Barsanti M, Lim JMC, Hun ML, Lister N, Wong K, Hammett MV, et al. A Novel Foxn1eGFP/+ Mouse Model Identifies Bmp4-Induced Maintenance of Foxn1 Expression and Thymic Epithelial Progenitor Populations. Eur J Immunol (2017) 47(2):291–304. doi: 10.1002/eji.201646553
49. Liu D, Kousa AI, O’Neill KE, Rouse P, Popis M, Farley AM, et al. Canonical Notch Signaling Controls the Early Thymic Epithelial Progenitor Cell State and Emergence of the Medullary Epithelial Lineage in Fetal Thymus Development. Dev Camb Engl (2020) 147(12):dev178582. doi: 10.1242/dev.178582
50. Li J, Gordon J, Chen ELY, Xiao S, Wu L, Zúñiga-Pflücker JC, et al. NOTCH1 Signaling Establishes the Medullary Thymic Epithelial Cell Progenitor Pool During Mouse Fetal Development. Dev Camb Engl (2020) 147(12):dev178988. doi: 10.1242/dev.178988
51. Goldfarb Y, Kadouri N, Levi B, Sela A, Herzig Y, Cohen RN, et al. HDAC3 is a Master Regulator of mTEC Development. Cell Rep (2016) 15(3):651–65. doi: 10.1016/j.celrep.2016.03.048
52. Chakrabarti S, Hoque M, Jamil NZ, Singh VJ, Pollacksmith D, Meer N, et al. Bone Marrow-Derived Cells Contribute to the Maintenance of Thymic Stroma Including TECs. J Immunol Res (2022) 2022:e6061746. doi: 10.1155/2022/6061746
53. Vobořil M, Brabec T, Dobeš J, Šplíchalová I, Březina J, Čepková A, et al. Toll-Like Receptor Signaling in Thymic Epithelium Controls Monocyte-Derived Dendritic Cell Recruitment and Treg Generation. Nat Commun (2020) 11(1):2361. doi: 10.1038/s41467-020-16081-3
54. Wang H, Zúñiga-Pflücker JC. Thymic Microenvironment: Interactions Between Innate Immune Cells and Developing Thymocytes. Front Immunol (2022) 13:885280. doi: 10.3389/fimmu.2022.885280
55. Hasegawa H, Matsumoto T. Mechanisms of Tolerance Induction by Dendritic Cells In Vivo. Front Immunol (2018) 9:350. doi: 10.3389/fimmu.2018.00350
56. Proietto AI, van Dommelen S, Zhou P, Rizzitelli A, D’Amico A, Steptoe RJ, et al. Dendritic Cells in the Thymus Contribute to T-Regulatory Cell Induction. Proc Natl Acad Sci U S A (2008) 105(50):19869–74. doi: 10.1073/pnas.0810268105
57. Bonasio R, Scimone ML, Schaerli P, Grabie N, Lichtman AH, von Andrian UH. Clonal Deletion of Thymocytes by Circulating Dendritic Cells Homing to the Thymus. Nat Immunol (2006) 7(10):1092–100. doi: 10.1038/ni1385
58. Vollmann EH, Rattay K, Barreiro O, Thiriot A, Fuhlbrigge RA, Vrbanac V, et al. Specialized Transendothelial Dendritic Cells Mediate Thymic T-Cell Selection Against Blood-Borne Macromolecules. Nat Commun (2021) 12(1):6230. doi: 10.1038/s41467-021-26446-x
59. Zegarra-Ruiz DF, Kim DV, Norwood K, Kim M, Wu WJH, Saldana-Morales FB, et al. Thymic Development of Gut-Microbiota-Specific T Cells. Nature (2021) 594(7863):413–7. doi: 10.1038/s41586-021-03531-1
60. Kroger CJ, Spidale NA, Wang B, Tisch R. Thymic Dendritic Cell Subsets Display Distinct Efficiencies and Mechanisms of Intercellular MHC Transfer. J Immunol Baltim Md (2017) 198(1):249–56. doi: 10.4049/jimmunol.1601516
61. Koble C, Kyewski B. The Thymic Medulla: A Unique Microenvironment for Intercellular Self-Antigen Transfer. J Exp Med (2009) 206(7):1505–13. doi: 10.1084/jem.20082449
62. Cosway EJ, Lucas B, James KD, Parnell SM, Carvalho-Gaspar M, White AJ, et al. Redefining Thymus Medulla Specialization for Central Tolerance. J Exp Med (2017) 214(11):3183–95. doi: 10.1084/jem.20171000
63. Nitta T, Tsutsumi M, Nitta S, Muro R, Suzuki EC, Nakano K, et al. Fibroblasts as a Source of Self-Antigens for Central Immune Tolerance. Nat Immunol (2020) 21(10):1172–80. doi: 10.1038/s41590-020-0756-8
64. Nitta T, Ota A, Iguchi T, Muro R, Takayanagi H. The Fibroblast: An Emerging Key Player in Thymic T Cell Selection. Immunol Rev (2021) 302(1):68–85. doi: 10.1111/imr.12985
65. Nitta T, Takayanagi H. Non-Epithelial Thymic Stromal Cells: Unsung Heroes in Thymus Organogenesis and T Cell Development. Front Immunol (2021) 11:620894/full. doi: 10.3389/fimmu.2020.620894/full
66. Muñoz JJ, García-Ceca J, Montero-Herradón S, Sánchez del Collado B, Alfaro D, Zapata A. Can a Proper T-Cell Development Occur in an Altered Thymic Epithelium? Lessons From EphB-Deficient Thymi. Front Endocrinol (2018) 9:135. doi: 10.3389/fendo.2018.00135
67. Graham A, Richardson J. Developmental and Evolutionary Origins of the Pharyngeal Apparatus. EvoDevo (2012) 3(1):24. doi: 10.1186/2041-9139-3-24
68. Gordon J, Wilson VA, Blair NF, Sheridan J, Farley A, Wilson L, et al. Functional Evidence for a Single Endodermal Origin for the Thymic Epithelium. Nat Immunol (2004) 5(5):546–53. doi: 10.1038/ni1064
69. Gordon J, Manley NR. Mechanisms of Thymus Organogenesis and Morphogenesis. Development (2011) 138(18):3865–78. doi: 10.1242/dev.059998
70. Sperber GH, Guttmann SGHSGD, Steven M. Craniofacial Development (Book for Windows & Macintosh). Craniofacial Development PMPH-USA (2001) 220 p.
71. Frisdal A, Trainor PA. Development and Evolution of the Pharyngeal Apparatus. WIREs Dev Biol (2014) 3(6):403–18. doi: 10.1002/wdev.147
72. Norris EH. The Morphogenesis and Histogenesis of the Thymus Gland in Man: In Which the Origin of the Hassall’s Corpuscles of the Human Thymus is Discovered. Contrib Embryol (1938) 27(166):191–208.
73. Van Dyke JH. On the Origin of Accessory Thymus Tissue, Thymus IV: The Occurrence in Man. Anat Rec (1941) 79(2):179–209. doi: 10.1002/ar.1090790204
74. Blackburn CC, Manley NR. Developing a New Paradigm for Thymus Organogenesis. Nat Rev Immunol (2004) 4(4):278–89. doi: 10.1038/nri1331
75. Manley NR, Condie BG. Transcriptional Regulation of Thymus Organogenesis and Thymic Epithelial Cell Differentiation. In: Liston A, editor. Progress in Molecular Biology and Translational Science. Academic Press (2010) 92:103–120. Available at: http://www.sciencedirect.com/science/article/pii/S187711731092005X.
76. Le Douarin NM, Jotereau FV. Tracing of Cells of the Avian Thymus Through Embryonic Life in Interspecific Chimeras. J Exp Med (1975) 142(1):17–40. doi: 10.1084/jem.142.1.17
77. Farley AM, Morris LX, Vroegindeweij E, Depreter MLG, Vaidya H, Stenhouse FH, et al. Dynamics of Thymus Organogenesis and Colonization in Early Human Development. Development (2013) 140(9):2015–26. doi: 10.1242/dev.087320
78. Hamazaki Y. Adult Thymic Epithelial Cell (TEC) Progenitors and TEC Stem Cells: Models and Mechanisms for TEC Development and Maintenance. Eur J Immunol (2015) 45(11):2985–93. doi: 10.1002/eji.201545844
79. Muñoz JJ, Zapata AG. Epithelial Development Based on a Branching Morphogenesis Program: The Special Condition of Thymic Epithelium In: Heinbockel T, Shields VD, editors. Histology [Internet]. London: IntechOpen [cited 2022 Jun 09]. (2018). doi: 10.5772/intechopen.81193
80. Nehls M, Pfeifer D, Schorpp M, Hedrich H, Boehm T. New Member of the Winged-Helix Protein Family Disrupted in Mouse and Rat Nude Mutations. Nature (1994) 372(6501):103–7. doi: 10.1038/372103a0
81. Gordon J, Bennett AR, Blackburn CC, Manley NR. Gcm2 and Foxn1 Mark Early Parathyroid- and Thymus-Specific Domains in the Developing Third Pharyngeal Pouch. Mech Dev (2001) 103(1–2):141–3. doi: 10.1016/S0925-4773(01)00333-1
82. Blackburn CC, Augustine CL, Li R, Harvey RP, Malin MA, Boyd RL, et al. The Nu Gene Acts Cell-Autonomously and is Required for Differentiation of Thymic Epithelial Progenitors. Proc Natl Acad Sci (1996) 93(12):5742–6. doi: 10.1073/pnas.93.12.5742
83. Nehls M, Kyewski B, Messerle M, Waldschütz R, Schüddekopf K, Smith AJH, et al. Two Genetically Separable Steps in the Differentiation of Thymic Epithelium. Science (1996) 272(5263):886–9. doi: 10.1126/science.272.5263.886
84. Prowse DM, Lee D, Weiner L, Jiang N, Magro CM, Baden HP, et al. Ectopic Expression of the Nude Gene Induces Hyperproliferation and Defects in Differentiation: Implications for the Self-Renewal of Cutaneous Epithelia. Dev Biol (1999) 212(1):54–67. doi: 10.1006/dbio.1999.9328
85. Nowell CS, Bredenkamp N, Tetélin S, Jin X, Tischner C, Vaidya H, et al. Foxn1 Regulates Lineage Progression in Cortical and Medullary Thymic Epithelial Cells But is Dispensable for Medullary Sublineage Divergence. PloS Genet (2011) 7(11):e1002348. doi: 10.1371/journal.pgen.1002348
86. Bredenkamp N, Nowell CS, Blackburn CC. Regeneration of the Aged Thymus by a Single Transcription Factor. Development (2014) 141(8):1627–37. doi: 10.1242/dev.103614
87. Revest JM, Suniara RK, Kerr K, Owen JJT, Dickson C. Development of the Thymus Requires Signaling Through the Fibroblast Growth Factor Receptor R2-IIIb. J Immunol (2001) 167(4):1954–61. doi: 10.4049/jimmunol.167.4.1954
88. Muñoz JJ, Cejalvo T, Tobajas E, Fanlo L, Cortés A, Zapata AG. 3D Immunofluorescence Analysis of Early Thymic Morphogenesis and Medulla Development. Histol Histopathol (2015) 30(5):589–99. doi: 10.14670/HH-30.589
89. Rhinn M, Dollé P. Retinoic Acid Signalling During Development. Development (2012) 139(5):843–58. doi: 10.1242/dev.065938
90. Graham A, Okabe M, Quinlan R. The Role of the Endoderm in the Development and Evolution of the Pharyngeal Arches. J Anat (2005) 207(5):479–87. doi: 10.1111/j.1469-7580.2005.00472.x
91. Wendling O, Dennefeld C, Chambon P, Mark M. Retinoid Signaling is Essential for Patterning the Endoderm of the Third and Fourth Pharyngeal Arches. Dev Camb Engl (2000) 127(8):1553–62. doi: 10.1242/dev.127.8.1553
92. Kopinke D, Sasine J, Swift J, Stephens WZ, Piotrowski T. Retinoic Acid is Required for Endodermal Pouch Morphogenesis and Not for Pharyngeal Endoderm Specification. Dev Dyn (2006) 235(10):2695–709. doi: 10.1002/dvdy.20905
93. Quinlan R, Gale E, Maden M, Graham A. Deficits in the Posterior Pharyngeal Endoderm in the Absence of Retinoids. Dev Dyn (2002) 225(1):54–60. doi: 10.1002/dvdy.10137
94. Niederreither K, Vermot J, Roux IL, Schuhbaur B, Chambon P, Dollé P. The Regional Pattern of Retinoic Acid Synthesis by RALDH2 is Essential for the Development of Posterior Pharyngeal Arches and the Enteric Nervous System. Development (2003) 130(11):2525–34. doi: 10.1242/dev.00463
95. Arnold JS, Werling U, Braunstein EM, Liao J, Nowotschin S, Edelmann W, et al. Inactivation of Tbx1 in the Pharyngeal Endoderm Results in 22q11ds Malformations. Development (2006) 133(5):977–87. doi: 10.1242/dev.02264
96. Balciunaite G, Keller MP, Balciunaite E, Piali L, Zuklys S, Mathieu YD, et al. Wnt Glycoproteins Regulate the Expression of FoxN1, the Gene Defective in Nude Mice. Nat Immunol (2002) 3(11):1102–8. doi: 10.1038/ni850
97. Osada M, Jardine L, Misir R, Andl T, Millar SE, Pezzano M. DKK1 Mediated Inhibition of Wnt Signaling in Postnatal Mice Leads to Loss of TEC Progenitors and Thymic Degeneration. PloS One (2010) 5(2):e9062. doi: 10.1371/journal.pone.0009062
98. Jin S OJ, Stellabotte F, Choe CP. Foxi1 Promotes Late-Stage Pharyngeal Pouch Morphogenesis Through Ectodermal Wnt4a Activation. Dev Biol (2018) 441(1):12–8. doi: 10.1016/j.ydbio.2018.06.011
99. Osada M, Ito E, Fermin HA, Vazquez-Cintron E, Venkatesh T, Friedel RH, et al. The Wnt Signaling Antagonist Kremen1 is Required for Development of Thymic Architecture. Clin Dev Immunol (2006) 13(2–4):299–319. doi: 10.1080/17402520600935097
100. Brunk F, Augustin I, Meister M, Boutros M, Kyewski B. Thymic Epithelial Cells Are a Nonredundant Source of Wnt Ligands for Thymus Development. J Immunol (2015) 195(11):5261–71. doi: 10.4049/jimmunol.1501265
101. Wei T, Zhang N, Guo Z, Chi F, Song Y, Zhu X. Wnt4 Signaling is Associated With the Decrease of Proliferation and Increase of Apoptosis During Age-Related Thymic Involution. Mol Med Rep (2015) 12(5):7568–76. doi: 10.3892/mmr.2015.4343
102. Griffith AV, Fallahi M, Venables T, Petrie HT. Persistent Degenerative Changes in Thymic Organ Function Revealed by an Inducible Model of Organ Regrowth. Aging Cell (2012) 11(1):169–77. doi: 10.1111/j.1474-9726.2011.00773.x
103. Kvell K, Varecza Z, Bartis D, Hesse S, Parnell S, Anderson G, et al. Wnt4 and LAP2alpha as Pacemakers of Thymic Epithelial Senescence. PloS One (2010) 5(5):e10701. doi: 10.1371/journal.pone.0010701
104. Swann JB, Happe C, Boehm T. Elevated Levels of Wnt Signaling Disrupt Thymus Morphogenesis and Function. Sci Rep (2017) 7(1):785. doi: 10.1038/s41598-017-00842-0
105. Gordon J, Manley NR. Tissue-Specific Requirements for BMP Signaling During Thymus and Parathyroid Morphogenesis. Dev Biol (2006) 295(1):455. doi: 10.1016/j.ydbio.2006.04.402
106. Patel SR, Gordon J, Mahbub F, Blackburn CC, Manley NR. Bmp4 and Noggin Expression During Early Thymus and Parathyroid Organogenesis. Gene Expr Patterns (2006) 6(8):794–9. doi: 10.1016/j.modgep.2006.01.011
107. Tsai PT, Lee RA, Wu H. BMP4 Acts Upstream of FGF in Modulating Thymic Stroma and Regulating Thymopoiesis. Blood (2003) 102(12):3947–53. doi: 10.1182/blood-2003-05-1657
108. Lovely CB, Swartz ME, McCarthy N, Norrie JL, Eberhart JK. Bmp Signaling Mediates Endoderm Pouch Morphogenesis by Regulating Fgf Signaling in Zebrafish. Development (2016) 143(11):2000–11. doi: 10.1242/dev.129379
109. Macatee TL, Hammond BP, Arenkiel BR, Francis L, Frank DU, Moon AM. Ablation of Specific Expression Domains Reveals Discrete Functions of Ectoderm- and Endoderm-Derived FGF8 During Cardiovascular and Pharyngeal Development. Development (2003) 130(25):6361–74. doi: 10.1242/dev.00850
110. Abu-Issa R, Smyth G, Smoak I, Meyers EN. Fgf8 is Required for Pharyngeal Arch and Cardiovascular Development in the Mouse. Development (2002) 129(19):4613–25. doi: 10.1242/dev.129.19.4613
111. Gardiner JR, Jackson AL, Gordon J, Lickert H, Manley NR, Basson MA. Localised Inhibition of FGF Signalling in the Third Pharyngeal Pouch is Required for Normal Thymus and Parathyroid Organogenesis. Development (2012) 139(18):3456–66. doi: 10.1242/dev.079400
112. Saldaña JI, Solanki A, Lau CI, Sahni H, Ross S, Furmanski AL, et al. Sonic Hedgehog Regulates Thymic Epithelial Cell Differentiation. J Autoimmun (2016) 68:86–97. doi: 10.1016/j.jaut.2015.12.004
113. Moore-Scott BA, Manley NR. Differential Expression of Sonic Hedgehog Along the Anterior–Posterior Axis Regulates Patterning of Pharyngeal Pouch Endoderm and Pharyngeal Endoderm-Derived Organs. Dev Biol (2005) 278(2):323–35. doi: 10.1016/j.ydbio.2004.10.027
114. Bain VE, Gordon J, O’Neil JD, Ramos I, Richie ER, Manley NR. Tissue-Specific Roles for Sonic Hedgehog Signaling in Establishing Thymus and Parathyroid Organ Fate. Dev Camb Engl (2016) 143(21):4027–37. doi: 10.1242/dev.141903
115. Garg V, Yamagishi C, Hu T, Kathiriya IS, Yamagishi H, Srivastava D. Tbx1, a DiGeorge Syndrome Candidate Gene, Is Regulated by Sonic Hedgehog During Pharyngeal Arch Development. Dev Biol (2001) 235(1):62–73. doi: 10.1006/dbio.2001.0283
116. Han L, Chaturvedi P, Kishimoto K, Koike H, Nasr T, Iwasawa K, et al. Single Cell Transcriptomics Identifies a Signaling Network Coordinating Endoderm and Mesoderm Diversification During Foregut Organogenesis. Nat Commun (2020) 11(1):4158. doi: 10.1038/s41467-020-17968-x
117. Davenport C, Diekmann U, Budde I, Detering N, Naujok O. Anterior–Posterior Patterning of Definitive Endoderm Generated From Human Embryonic Stem Cells Depends on the Differential Signaling of Retinoic Acid, Wnt-, and BMP-Signaling. Stem Cells (2016) 34(11):2635–47. doi: 10.1002/stem.2428
118. Green MD, Chen A, Nostro MC, d’Souza SL, Schaniel C, Lemischka IR, et al. Generation of Anterior Foregut Endoderm From Human Embryonic and Induced Pluripotent Stem Cells. Nat Biotechnol (2011) 29(3):267–72. doi: 10.1038/nbt.1788
119. Kearns NA, Genga RMJ, Ziller M, Kapinas K, Peters H, Brehm MA, et al. Generation of Organized Anterior Foregut Epithelia From Pluripotent Stem Cells Using Small Molecules. Stem Cell Res (2013) 11(3):1003–12. doi: 10.1016/j.scr.2013.06.007
120. Li LC, Wang X, Xu ZR, Wang YC, Feng Y, Yang L, et al. Single-Cell Patterning and Axis Characterization in the Murine and Human Definitive Endoderm. Cell Res (2021) 31(3):326–44. doi: 10.1038/s41422-020-00426-0
121. Nowotschin S, Setty M, Kuo YY, Liu V, Garg V, Sharma R, et al. The Emergent Landscape of the Mouse Gut Endoderm at Single-Cell Resolution. Nature (2019) 569(7756):361–7. doi: 10.1038/s41586-019-1127-1
122. Magaletta ME, Lobo M, Kernfeld EM, Aliee H, Huey JD, Parsons TJ, et al. Integration of Single-Cell Transcriptomes and Chromatin Landscapes Reveals Regulatory Programs Driving Pharyngeal Organ Development. Nat Commun (2022) 13(1):457. doi: 10.1038/s41467-022-28067-4
123. Lai L, Jin J. Generation of Thymic Epithelial Cell Progenitors by Mouse Embryonic Stem Cells. Stem Cells (2009) 27:3012–3020. doi: 10.1002/stem.238
124. Inami Y, Yoshikai T, Ito S, Nishio N, Suzuki H, Sakurai H, et al. Differentiation of Induced Pluripotent Stem Cells to Thymic Epithelial Cells by Phenotype. Immunol Cell Biol (2011) 89(2):314–21. doi: 10.1038/icb.2010.96
125. Soh CL, Giudice A, Jenny RA, Elliott DA, Hatzistavrou T, Micallef SJ, et al. FOXN1GFP/w Reporter hESCs Enable Identification of Integrin-β4, HLA-DR, and EpCAM as Markers of Human PSC-Derived FOXN1+ Thymic Epithelial Progenitors. Stem Cell Rep (2014) 2(6):925–37. doi: 10.1016/j.stemcr.2014.04.009
126. Parent AV, Russ HA, Khan IS, LaFlam TN, Metzger TC, Anderson MS, et al. Generation of Functional Thymic Epithelium From Human Embryonic Stem Cells That Supports Host T Cell Development. Cell Stem Cell (2013) 13(2):219–229. doi: 10.1016/j.stem.2013.04.004
127. Sun X, Xu J, Lu H, Liu W, Miao Z, Sui X, et al. Directed Differentiation of Human Embryonic Stem Cells Into Thymic Epithelial Progenitor-Like Cells Reconstitutes the Thymic Microenvironment In Vivo. Cell Stem Cell (2013) 13(2):230–6. doi: 10.1016/j.stem.2013.06.014
128. Su M, Hu R, Jin J, Yan Y, Song Y, Sullivan R, et al. Efficient In Vitro Generation of Functional Thymic Epithelial Progenitors From Human Embryonic Stem Cells. Sci Rep (2015) 5(1):1–8. doi: 10.1038/srep09882
129. Okabe M, Ito S, Nishio N, Tanaka Y, Isobe KI. Thymic Epithelial Cells Induced From Pluripotent Stem Cells by a Three-Dimensional Spheroid Culture System Regenerates Functional T Cells in Nude Mice. Cell Reprogramming (2015) 17(5):368–75. doi: 10.1089/cell.2015.0006
130. Otsuka R, Wada H, Tsuji H, Sasaki A, Murata T, Itoh M, et al. Efficient Generation of Thymic Epithelium From Induced Pluripotent Stem Cells That Prolongs Allograft Survival. Sci Rep (2020) 10(1):1–8. doi: 10.1038/s41598-019-57088-1
131. Chhatta AR, Cordes M, Hanegraaf MAJ, Vloemans S, Cupedo T, Cornelissen JJ, et al. De Novo Generation of a Functional Human Thymus From Induced Pluripotent Stem Cells. J Allergy Clin Immunol (2019) 144(5):1416–1419.e7. doi: 10.1016/j.jaci.2019.05.042
132. Ramos SA, Morton JJ, Yadav P, Reed B, Alizadeh SI, Shilleh AH, et al. Generation of Functional Human Thymic Cells From Induced Pluripotent Stem Cells. J Allergy Clin Immunol (2022) 149:767–781.e6. doi: 10.1016/j.jaci.2021.07.021
133. Gras-Peña R, Danzl NM, Khosravi-Maharlooei M, Campbell SR, Ruiz AE, Parks CA, et al. Human Stem Cell-Derived Thymic Epithelial Cells Enhance Human T-Cell Development in a Xenogeneic Thymus. J Allergy Clin Immunol (2022) 149:1755–1771. doi: 10.1016/j.jaci.2021.09.038
134. Kont V, Laan M, Kisand K, Merits A, Scott HS, Peterson P. Modulation of Aire Regulates the Expression of Tissue-Restricted Antigens. Mol Immunol (2008) 45(1):25–33. doi: 10.1016/j.molimm.2007.05.014
135. Pinto S, Schmidt K, Egle S, Stark HJ, Boukamp P, Kyewski B. An Organotypic Coculture Model Supporting Proliferation and Differentiation of Medullary Thymic Epithelial Cells and Promiscuous Gene Expression. J Immunol (2013) 190(3):1085–93. doi: 10.4049/jimmunol.1201843
136. Silva CS, Reis RL, Martins A, Neves NM. Recapitulation of Thymic Function by Tissue Engineering Strategies. Adv Healthc Mater 10:2100773. doi: 10.1002/adhm.202100773
137. Tajima A, Liu W, Pradhan I, Bertera S, Lakomy RA, Rudert WA, et al. Promoting 3-D Aggregation of FACS Purified Thymic Epithelial Cells With EAK 16-II/EAKIIH6 Self-Assembling Hydrogel. J Vis Exp (2016) 112):54062. doi: 10.3791/54062
138. Silva CS, Pinto RD, Amorim S, Pires RA, Correia-Neves M, Reis RL, et al. Fibronectin-Functionalized Fibrous Meshes as a Substrate to Support Cultures of Thymic Epithelial Cells. Biomacromolecules (2020) 21(12):4771–80. doi: 10.1021/acs.biomac.0c00933
139. Suraiya AB, Hun ML, Truong VX, Forsythe JS, Chidgey AP. Gelatin-Based 3d Microgels for In Vitro T Lineage Cell Generation. ACS Biomater Sci Eng (2020) 6(4):2198–208. doi: 10.1021/acsbiomaterials.9b01610
140. Bortolomai I, Sandri M, Draghici E, Fontana E, Campodoni E, Marcovecchio GE, et al. Gene Modification and Three-Dimensional Scaffolds as Novel Tools to Allow the Use of Postnatal Thymic Epithelial Cells for Thymus Regeneration Approaches. Stem Cells Transl Med (2019) 8(10):1107–22. doi: 10.1002/sctm.18-0218
141. Asnaghi MA, Barthlott T, Gullotta F, Strusi V, Amovilli A, Hafen K, et al. Thymus Extracellular Matrix-Derived Scaffolds Support Graft-Resident Thymopoiesis and Long-Term In Vitro Culture of Adult Thymic Epithelial Cells. Adv Funct Mater (2021) 31:2010747. doi: 10.1002/adfm.202010747
142. Hun M, Barsanti M, Wong K, Ramshaw J, Werkmeister J, Chidgey AP. Native Thymic Extracellular Matrix Improves In Vivo Thymic Organoid T Cell Output, and Drives In Vitro Thymic Epithelial Cell Differentiation. Biomaterials (2017) 118:1–15. doi: 10.1016/j.biomaterials.2016.11.054
143. Fan Y, Tajima A, Goh SK, Geng X, Gualtierotti G, Grupillo M, et al. Bioengineering Thymus Organoids to Restore Thymic Function and Induce Donor-Specific Immune Tolerance to Allografts. Mol Ther (2015) 23(7):1262–77. doi: 10.1038/mt.2015.77
144. Seet CS, He C, Bethune MT, Li S, Chick B, Gschweng EH, et al. Generation of Mature T Cells From Human Hematopoietic Stem and Progenitor Cells in Artificial Thymic Organoids. Nat Methods (2017) 14(5):521–30. doi: 10.1038/nmeth.4237
145. Montel-Hagen A, Sun V, Casero D, Tsai S, Zampieri A, Jackson N, et al. In Vitro Recapitulation of Murine Thymopoiesis From Single Hematopoietic Stem Cells. Cell Rep (2020) 33(4):108320. doi: 10.1016/j.celrep.2020.108320
146. Montel-Hagen A, Seet CS, Li S, Chick B, Zhu Y, Chang P, et al. Organoid-Induced Differentiation of Conventional T Cells From Human Pluripotent Stem Cells. Cell Stem Cell (2019) 24(3):376–389.e8. doi: 10.1016/j.stem.2018.12.011
147. Kennedy M, Awong G, Sturgeon CM, Ditadi A, LaMotte-Mohs R, Zúñiga-Pflücker JC, et al. T Lymphocyte Potential Marks the Emergence of Definitive Hematopoietic Progenitors in Human Pluripotent Stem Cell Differentiation Cultures. Cell Rep (2012) 2(6):1722–35. doi: 10.1016/j.celrep.2012.11.003
148. Bin G, Jiarong Z, Shihao W, Xiuli S, Cheng X, Liangbiao C, et al. Aire Promotes the Self-Renewal of Embryonic Stem Cells Through Lin28. Stem Cells Dev (2012) 21(15):2878–90. doi: 10.1089/scd.2012.0097
149. Gu B, Zhang J, Chen Q, Tao B, Wang W, Zhou Y, et al. Aire Regulates the Expression of Differentiation-Associated Genes and Self-Renewal of Embryonic Stem Cells. Biochem Biophys Res Commun (2010) 394(2):418–23. doi: 10.1016/j.bbrc.2010.03.042
150. Karvonen E, Krohn KJE, Ranki A, Hau A. Generation and Characterization of iPS Cells Derived From APECED Patients for Gene Correction. Front Endocrinol (2022) 13:794327. doi: 10.3389/fendo.2022.794327
151. Forbes TA, Howden SE, Lawlor K, Phipson B, Maksimovic J, Hale L, et al. Patient-iPSC-Derived Kidney Organoids Show Functional Validation of a Ciliopathic Renal Phenotype and Reveal Underlying Pathogenetic Mechanisms. Am J Hum Genet (2018) 102(5):816–31. doi: 10.1016/j.ajhg.2018.03.014
152. Deng WL, Gao ML, Lei XL, Lv JN, Zhao H, He KW, et al. Gene Correction Reverses Ciliopathy and Photoreceptor Loss in iPSC-Derived Retinal Organoids From Retinitis Pigmentosa Patients. Stem Cell Rep (2018) 10(4):1267–81. doi: 10.1016/j.stemcr.2018.02.003
153. Okada H, Nakanishi C, Yoshida S, Shimojima M, Yokawa J, Mori M, et al. Function and Immunogenicity of Gene-Corrected iPSC-Derived Hepatocyte-Like Cells in Restoring Low Density Lipoprotein Uptake in Homozygous Familial Hypercholesterolemia. Sci Rep (2019) 9(1):4695. doi: 10.1038/s41598-019-41056-w
154. Howden SE, McColl B, Glaser A, Vadolas J, Petrou S, Little MH, et al. A Cas9 Variant for Efficient Generation of Indel-Free Knockin or Gene-Corrected Human Pluripotent Stem Cells. Stem Cell Rep (2016) 7(3):508–17. doi: 10.1016/j.stemcr.2016.07.001
155. Kreins AY, Bonfanti P, Davies EG. Current and Future Therapeutic Approaches for Thymic Stromal Cell Defects. Front Immunol (2021) 12:655354. doi: 10.3389/fimmu.2021.655354
Keywords: thymus, IPSC, thymic epithelial cells (TEC), differentiation, APECED, tolerance
Citation: Provin N and Giraud M (2022) Differentiation of Pluripotent Stem Cells Into Thymic Epithelial Cells and Generation of Thymic Organoids: Applications for Therapeutic Strategies Against APECED. Front. Immunol. 13:930963. doi: 10.3389/fimmu.2022.930963
Received: 28 April 2022; Accepted: 26 May 2022;
Published: 29 June 2022.
Edited by:
Rachid Tazi Ahnini, The University of Sheffield, United KingdomReviewed by:
Agustin G. Zapata, Complutense University of Madrid, SpainAnn Chidgey, Monash University, Australia
Rachel Thomas, Alcon Research, LLC, United States
Copyright © 2022 Provin and Giraud. This is an open-access article distributed under the terms of the Creative Commons Attribution License (CC BY). The use, distribution or reproduction in other forums is permitted, provided the original author(s) and the copyright owner(s) are credited and that the original publication in this journal is cited, in accordance with accepted academic practice. No use, distribution or reproduction is permitted which does not comply with these terms.
*Correspondence: Matthieu Giraud, bWF0dGhpZXUuZ2lyYXVkQGluc2VybS5mcg==