- 1Division of Orthodontics and Dentofacial Orthopedics, Tohoku University Graduate School of Dentistry, Sendai, Miyagi, Japan
- 2Frontier Research Institute for Interdisciplinary Sciences, Tohoku University, Sendai, Miyagi, Japan
- 3Department of Biological Chemistry Graduate School of Pharmaceutical Sciences, Kyoto University, Kyoto, Japan
Docosahexaenoic acid (DHA) is an omega-3 fatty acid that has a range of positive impacts on human health, including anti-inflammatory effects and inhibition of osteoclast formation via G-protein-coupled receptor 120 (GPR120). Orthodontic force was reported to induce tumor necrosis factor-α (TNF-α) expression, which activates osteoclast differentiation during orthodontic tooth movement (OTM). The aim of this study was to investigate the influence of DHA on TNF-α-induced osteoclast formation and OTM in vivo. We examined osteoclast formation and bone resorption within the calvaria of both wild-type (WT) and GPR120-deficient (GPR120-KO) mice injected with phosphate-buffered saline (PBS), TNF-α, TNF-α and DHA, or DHA. DHA inhibited TNF-α-induced osteoclast formation and bone resorption in WT mice but had no effect in GPR120-KO mice. OTM experiments were performed in mouse strains with or without regular injection of DHA, and the effects of DHA on osteoclast formation in the alveolar bones during OTM were examined. DHA also suppressed OTM in WT but not GPR120-KO mice. Our data showed that DHA suppresses TNF-α-induced osteoclastogenesis and bone resorption via GPR120. TNF-α has considerable significance in OTM, and therefore, DHA may also inhibit TNF-α-induced osteoclast formation and bone resorption in OTM.
Introduction
Docosahexaenoic acid (DHA), a long-chain n-3 polyunsaturated fatty acid with 22 carbon atoms and 6 double bonds, is one of the polyunsaturated fatty acids. It has been shown to have benefits on several disorders, including cardiovascular disease (1), diabetes (2), inflammatory bowel disease, rheumatoid arthritis, dysmenorrhea, asthma, atherosclerosis, and autoimmune diseases (3–6). Moreover, supplements containing DHA have shown positive effects on some cancers, diabetes, and cardiovascular diseases (7, 8).
Osteoclasts, the derivative of the hematopoietic stem cells with multi-nucleus, are prerequisites for bone remodeling through destruction of the bone matrix and bone erosion such as rheumatoid arthritis, periprosthetic bone loss, periodontal disease, and postmenopausal osteoporosis (9). The differentiation and functional maturation of osteoclasts are mediated by two cytokines. One is the receptor activator of NF-kB ligand (RANKL), and the other is the macrophage colony-stimulating factor (M-CSF) (10). Additionally, tumor necrosis factor-α (TNF-α) promotes the differentiation of osteoclasts from osteoclast precursors derived from bone marrow cells both in vitro (11–13) and in vivo (14, 15). TNF-α has significant functions during osteoerosive disorders, including inflammation (16).
The G-protein-coupled receptors (GPRs) are seven transmembrane domain receptors that play crucial roles in many cellular functions and in the regulation of several physiological and pathological processes (17–20). Long-chain fatty acids bind the free fatty acid receptors (FFARs), GPR120 (FFAR4), GPR41 (FFAR3), GPR43 (FFAR2), GPR40 (FFAR1), and GPR84 (21, 22), among which GPR120 plays a key role in homeostatic metabolic regulation during immune processes and inflammation in vivo (23). Thus, the GPRs have attracted a great deal of attention as major drug development targets for several human diseases, and research has focused on GPR120 due to its role in inflammatory diseases, including obesity and diabetes (17, 22–27).
Dietary n-3 fatty acids prevent bone destruction in ovariectomized mice, and n-3, but not n-6, fatty acids inhibit osteoclast formation in vitro (28). Several in vitro studies showed that DHA has a suppressive effect on osteoclast formation and function and on the differentiation of human CD14+ monocytes into osteoclasts (29). DHA has also restrictive effects on osteoclast differentiation, activation, and function of the mouse monocyte cell line RAW 264.7 mediated via stimulation of RANKL in vitro (30). Our previous in vitro study showed that DHA attenuated osteoclast differentiation induced by both RANKL and TNF-α (31). Furthermore, DHA attenuates lipopolysaccharide (LPS)-induced osteoclast formation and bone resorption in vivo (31). These suppressive effects of DHA are mediated by the inhibition of TNF-α expression induced by LPS in macrophages and by inhibition of both osteoclast formation induced by RANKL and by TNF-α directly through GPR120 (31).
GPR120 signaling inhibits osteoclast formation by suppressing RANKL-mediated nuclear factor-kappa B (NF-kB) activation and nuclear factor of activated T-cell cytoplasmic (NFATc1) induction (32). Moreover, FFAs, including DHA, prevent the nuclear translocation of NF-κB in murine macrophages; however, the translocation of NF-κB subunit to the nuclei was not blocked in the case of FFAR4 absence (33). According to our previous study, NF-κB is involved in TNF-α-induced transcriptional activation of inflammation-associated genes. However, the effect of DHA on TNF-α-induced NF-κB pathway activation through GPR120 is still unclear.
Orthodontic tooth movement (OTM) with the application of orthodontic force involves the remodeling of alveolar bone and reconstruction of the periodontal ligament. Multiple factors are involved in the mechanism underlying OTM, including cytokines, growth factors, components of the bone matrix, and neurotransmitters, which mediate the differentiation and function of osteoblasts and osteoclasts, causing bone remodeling (34–38).
Several studies have demonstrated that mechanical force loading induced the production of TNF-α (39, 40). Moreover, TNF-α receptor-deficient mice reduced tooth movement and osteoclast formation compared to wild-type (WT) controls, which suggested that TNF-α has remarkable effects on osteoclast formation and bone resorption in OTM (41, 42). Our earlier research has found that DHA directly inhibits TNF-α-induced osteoclast formation, suggesting that DHA may also inhibit the TNF-α-induced osteoclastogenesis and bone destruction in OTM (31). However, the effects of DHA on OTM are yet to be elucidated.
The present research was performed with the aim of evaluating the effects of DHA injection on TNF-α-induced osteoclastogenesis and bone destruction in mice through GPR120, and elucidating the underlying mechanisms by in vitro experiments. Furthermore, we examined the influence of DHA on OTM and the levels of osteoclast activity mediated via GPR120.
Materials and methods
Animals and reagents
Male C57BL6/J mice, 8–12 weeks old, were purchased from CLEA Japan (Tokyo, Japan) to be used as WT mice. GPR120-KO mice were generated by crossing mice expressing Cre-recombinant activity under the influence of chicken actin promoter and C57BL6/J mice bearing Ffar4-flowed gene (Ffar4(dE1/dE1) mice) (31). All animal care and experiments were approved by the Tohoku University of Science Animal Care and Use Committee (2020DnA-007-05). DHA was obtained from Sigma-Aldrich (St. Louis, MO, USA). Recombinant murine TNF-α was obtained as described previously (14), and recombinant mouse M-CSF was obtained from CMG14-12, M-CSF-expressing cell line (43).
Histological analysis
WT and GPR120-KO mice were divided into four groups (n = 4), which received daily subcutaneous supracalvarial injection of the following for 5 consecutive days: negative control, phosphate-buffered saline (PBS); TNF-α (3 μg/day); DHA (100 µg/day) and TNF-α (3 μg/day); and DHA (100 µg/day). On day 6, all mice were sacrificed, and the calvariae were resected and then fixed immediately in 4% PBS-buffered formaldehyde. After fixation for 24 h at 4°C, 14% ethylenediaminetetraacetic acid (EDTA) was used to demineralize all calvariae for 3 days at room temperature. After dehydration in a tissue processer (TP1020; Leica Wetzlar, Germany), all calvariae were embedded using paraffin and then cut into 5-µm sections perpendicular to the sagittal suture using a microtome. Paraffin sections were stained with TRAP solution mixed with acetate buffer (pH 5.0), naphthol AS-MX phosphate (Sigma-Aldrich), Fast Red Violet LB Salt (Sigma-Aldrich), and 50 mM sodium tartrate. After staining, all sections were counterstained with hematoxylin. Cells were considered as osteoclasts if they were TRAP-positive and contain three or more nuclei (40). TRAP-positive osteoclasts at the suture mesenchyme of mice sagittal suture were counted and normalized to 400 × 400 µm2 surface area of each section.
To analyze OTM, the maxillae were fixed in 4% formaldehyde in PBS before being decalcified with 14% EDTA. Samples were dehydrated in a tissue processer (TP1020; Leica), before being embedded in paraffin. The upper left first molars of these mice were cut into horizontal sections at 100, 140, 180, 220, and 260 μm from the apical of the bifurcation area. All the sections were stained with TRAP solution and counterstained with hematoxylin. The mesial side of the labiolingual long axis of the distobuccal root was regarded as the compression side. Finally, we measured the number of osteoclasts on the alveolar surface of the compression side on the sections of different levels to analyze osteoclast formation (44).
Micro-CT analysis of bone destruction area
Mice treated with daily subcutaneous supracalvarial injection were sacrificed on day 6. Their calvariae were fixed immediately in 4% PBS-buffered formaldehyde and then scanned using microfocus computed tomography (micro-CT) (ScanXmate-E090; Comscan, Kanagawa, Japan). Software TRI/3DBON64 (RATOC System Engineering, Tokyo, Japan) was used to create 3D images of the calvariae. The resorption area was regarded as the black area between the sutures, in contrast to the bone surface, which appears white in the 3D CT images. To quantify the bone resorption area, a 50 × 70 pixel rectangle was selected at the intersection between the sagittal and coronal sutures, and the amount of bone resorption area to the total area was measured using ImageJ software (NIH, Bethesda, MD, USA) (40).
Preparation of osteoblasts
The calvariae of 5- to 6-day-old WT and GPR120-KO mice were dissected. Collagenase solution (0.2% w/v; Wako Pure Chemical Industries, Osaka, Japan) was prepared in isolation buffer (3 mM K2HPO4, 10 mM NaHCO3, 60 mM sorbitol, 70 mM NaCl, 1 mM CaCl2, 0.5% [w/v] glucose, 0.1% (w/v) bovine serum albumin [BSA], and 25 mM 4-(2-hydroxyethyl)-1-piperazineethanesulfonic acid). EDTA was prepared at a concentration of 5 mM with 0.1% BSA in PBS and filtered through a 0.2-µm filter. Calvariae were subjected to digestion via collagenase and EDTA, for 20 and 15 min, respectively, at 37°C on a shaker. Fraction 1 (collagenase), fraction 2 (EDTA), fraction 3 (collagenase), fraction 4 (collagenase), fraction 5 (EDTA), and the digests were collected. Fractions 3–5 were considered as the osteoblast high fractions. Cells were cultured in alpha minimal essential medium (α-MEM) (Wako), containing 10% fetal bovine serum (FBS) and 100 IU/ml penicillin G and 100 μg/ml streptomycin, overnight. The adherent cells were collected using trypsin-EDTA (Life Technologies, Grand Island, NY, USA). The cells were cultured in α-MEM containing 10% FBS for 3 days with the culture media changed every 2 days. Adherent cells were used as osteoblasts (45).
RNA preparation and real-time RT-PCR analysis
The calvariae of mice from all eight groups of the in vivo experiments were frozen in liquid nitrogen and then crushed using Micro Smash MS-100R (Tomy Seiko, Tokyo, Japan) in 800 μl of TRIzol reagent (Invitrogen, Carlsbad, CA, USA). RNA was also prepared from osteoblasts of the mouse strains from the in vitro experiments. Cells were incubated in α-MEM with either PBS, TNF-α (100 ng/ml), TNF-α (100 ng/ml) and DHA (100 ng/ml), or DHA (100 ng/ml) for 3 days. Total RNA was used to synthesize cDNAs of each group of samples with oligo-dT primers (Invitrogen) and reverse transcriptase in a total volume of 20 μl. Each reaction contained 2 μl of cDNA and 23 μl of a mixture consisting of SYBR Premix Ex Taq (Takara, Shiga, Japan) and 50 pmol/μl of primers. TRAP, RANKL, and OPG mRNA expression levels were evaluated using real-time RT-PCR using a Thermal Cycler Dice Real-Time System (Takara). The PCR cycling conditions were started with an initial denaturation step at 95°C for 10 s, followed by 45–60 cycles of amplification, with each cycle consisting of a denaturation step at 95°C for 5 s and an annealing step at 60°C for 30 s. To determine the relative expression levels of RANKL mRNAs, glyceraldehyde 3-phosphate dehydrogenase (GAPDH) was used as a reference gene. The primer sequences used for cDNA amplification were as follows: GAPDH, 5′-GGTGGAGCCAAAAGGGTCA-3′ and 5′-GGGGGCTAAGCAGTTGGT-3′; TRAP, 5′-AACTTGCGACCATTGTTA-3′ and 5′-GGGGACCTTTCGTTGATGT-3′; RANKL, 5′-CCTGAGGCCAGCCATTT-3′ and 5′-CTTGGCCCAGCCTCGAT-3′; OPG, 5′-CTTAGGTCC AACTACAGAGGAAC-3′ and 5′-ATCAGAGCCTCATCACCTT-3′; and Cathepsin K 5′-GCAGAGGTTGTACTATGA-3′ and 5′-GCAGGCGTTGTTCTTATT-3′ (46).
Preparation of osteoclast precursors and co-culture of both GPR120-KO osteoclast precursors and WT or GPR120-KO osteoblasts
The bone marrow cells of GPR120-KO mice were prepared by flushing from the femora and tibiae and cultured in α-MEM with 10% FBS, 100 IU/ml penicillin G, and 100 μg/ml streptomycin together with 100 ng/ml M-CSF for 3 days. Attached cells were obtained by trypsin-EDTA (Life Technologies). These cells were collected as osteoclast precursors. GPR120-KO osteoclast precursors and WT or GPR120-KO osteoblasts were co-cultured in the presence of 10−6 M PGE2+10−8 M 1,25(OH)2D3 (Sigma-Aldrich) with or without TNF-α (100 ng/ml) and with or without DHA in 96-well plates. Co-culturing was continued for 4 days after which it was ended by fixation with 4% formaldehyde and stained with TRAP as described previously (47). Cells were considered as osteoclasts if they were TRAP positive and contained three or more nuclei.
Immunofluorescence
Osteoblasts isolated from WT or GPR120KO mice were seeded in 96-well plates in α-MEM at a density of 3 × 10³ per well overnight at 37°C. Cells were then stimulated with PBS, TNF-α (100 ng/ml), TNF-α (100 ng/ml) and DHA (100 ng/ml), or DHA (100 ng/ml) for 1 h. After this, we removed the culture medium and washed it three times using PBS. We then fixed it in 4% (w/v) formaldehyde solution in PBS for 15 min at room temperature. The fixative solution in the 96-well plates was removed and washed by PBS before adding 0.5% Triton X-100 (v/v) in PBS for 15 min. All the wells were washed with PBS and blocked with 3% BSA in PBS. Wells were incubated with p65 (D14E12) XP Rabbit mAb (Cell Signaling Technology, MA, USA; 1:400 dilution) diluted in 3% BSA in PBS to 1:400 overnight at 4°C. Cells were washed and incubated with Alexa Fluor 555 conjugate (Cell Signaling Technology, MA, USA; 1:100 dilution) in 3% BSA in PBS for 1 h in the dark at room temperature. Finally, cells were incubated with 4′,6-diamidino-2-phenylindole (DAPI) for 5 min before visualizing fluorescent cells under a fluorescence microscope (Olympus IX71, Tokyo, Japan).
Preparation of OTM and measurement of the distance of tooth movement
OTM was performed as described previously (48). Twelve-week-old WT or GPR120-KO male mice received injections of PBS or DHA (100 µg/day) every 2 days during OTM using a 30 G 10-mm needle under anesthesia. There was a total of seven injections for each mouse during the 12-day OTM. The injection site was as close to the gingiva on the buccal side as possible, and there was only one injection site at a time. The upper incisor and upper left first molar were bound with a nickel–titanium (Ni–Ti) closed-coil spring (Tomy Seiko) under anesthesia. This appliance was fixed with a stainless-steel wire (0.01 mm in diameter) and attached to a hole through the upper anterior alveolar of the mice to move the upper left first molar mesially with 10g tractive force. After 12 days of force loading, the distance between the upper first molar and the second molar was measured with silicone impressions of the maxilla, and the distance was compared between groups. These alveolar bones were also used for histological analysis.
Statistical analysis
All data were expressed as the mean ± standard deviation (SD). Statistical analyses were performed using Scheffé’s test and t-test. In all analyses, p < 0.05 represented statistical significance.
Results
DHA inhibited osteoclast formation induced by TNF-α through GPR120 activation in vivo
We examined whether osteoclast formation induced by TNF-α is inhibited by DHA through GPR120 in vivo. TNF-α and DHA were injected into the calvariae of both WT and GPR120-deficient (GPR120-KO) mice. Following 5 consecutive days of TNF-α injection, large numbers of TRAP-positive cells with three or more nuclei were recognized along the suture mesenchyme on histological sections. In contrast, WT mice co-administered TNF-α and DHA showed a significant reduction in the mean number of TRAP-positive cells compared to those treated with TNF-α alone (Figures 1A, B). However, the inhibitory effect of DHA was attenuated in GPR120-KO mice (Figures 1A, B). Furthermore, TRAP, RANKL, and Cathepsin K mRNA levels were significantly lower in WT mice co-administered TNF-α and DHA than in those administered TNF-α alone (Figures 1C, D), so as the ratio of RANKL/OPG. However, there were no significant differences in TRAP, RANKL, or Cathepsin K mRNA levels in GPR120-KO mice administered TNF-α with or without DHA injection (Figures 1C–E), and the ratio of RANKL/OPG was also at the same level in these two groups of GPR120 KO mice.
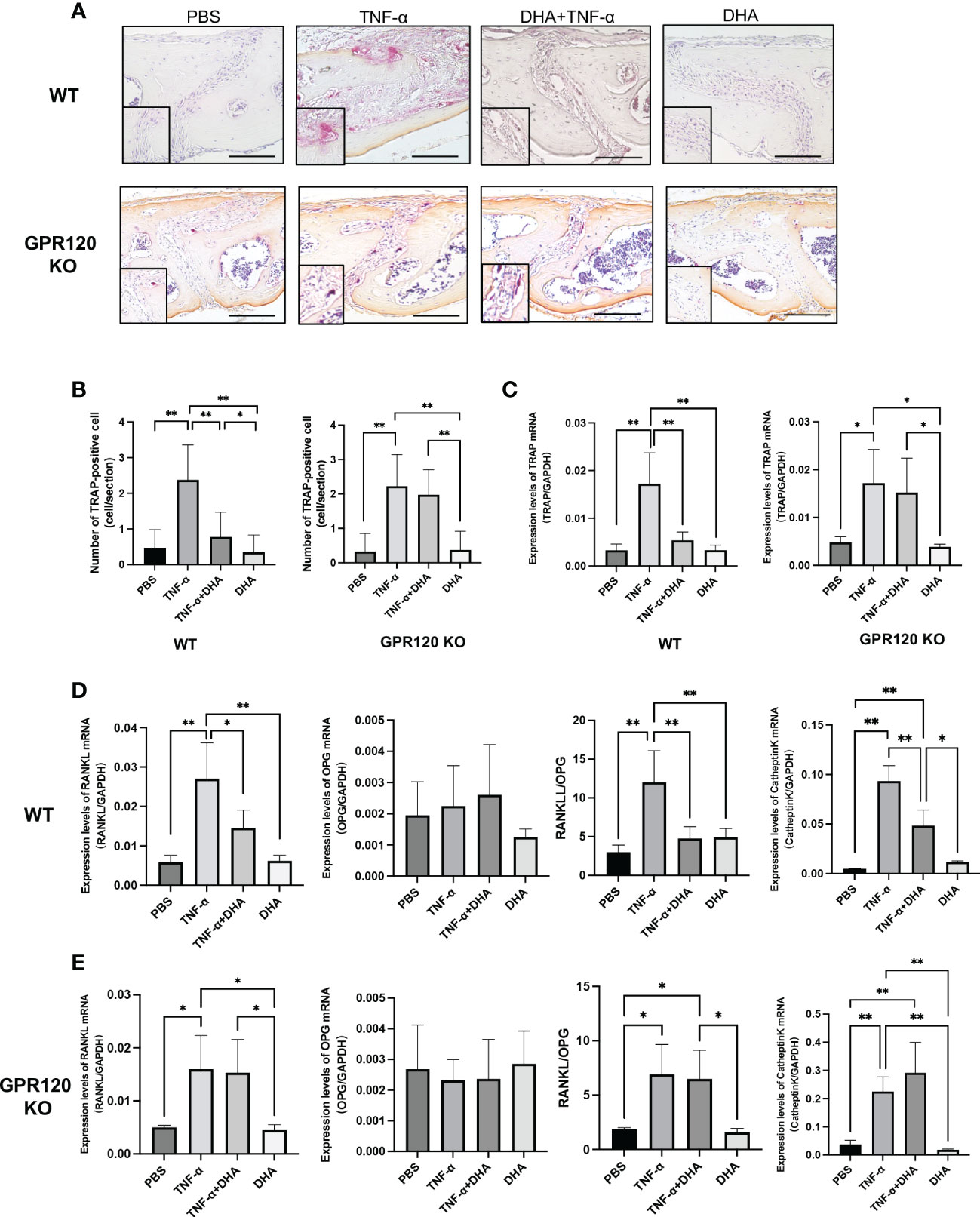
Figure 1 DHA inhibited osteoclast formation induced by TNF-α via GPR120 in vivo. (A) Microscopic images of TRAP-stained sections of mouse calvariae. Calvariae of WT and GPR120-KO mice receiving a 5-day daily subcutaneous injection of PBS, TNF-α, TNF-α + DHA, or DHA only to supracalvaria were cut into 5-µm sections. After staining with TRAP solution, hematoxylin was used as a counterstain for those sections. (B) TRAP-positive cells number in the sagittal suture of four groups of WT and GPR120-KO mice administered PBS, TNF-α, TNF-α + DHA, or DHA. (C) TRAP mRNA levels in WT and GPR120-KO mouse calvariae were determined using real-time RT-PCR. (D) RANKL, OPG, and Cathepsin K mRNA levels and the ratio of RANKL/OPG in WT mouse calvariae were determined using real-time RT-PCR. (E) RANKL, OPG, and Cathepsin K mRNA levels and the ratio of RANKL/OPG in GPR120-KO mouse calvariae were determined using real-time RT-PCR. The results are given as means ± SD. Scheffé’s test was used to determine the statistical significance of differences (n = 4; *p < 0.05, **p < 0.01). Scale bars = 100 µm.
DHA inhibited bone resorption induced by TNF-α through GPR120 activation in vivo
After scanning using micro-CT, the ratio of bone resorption area to the total area of mice calvariae was analyzed. Mice injected with TNF-α showed a considerably larger bone resorption area than the PBS control or DHA groups. However, the bone resorption area was markedly decreased in the TNF-α and DHA co-administration group. In contrast, there were no significant differences in bone resorption area in GPR120-KO administered TNF-α with or without DHA (Figures 2A, B).
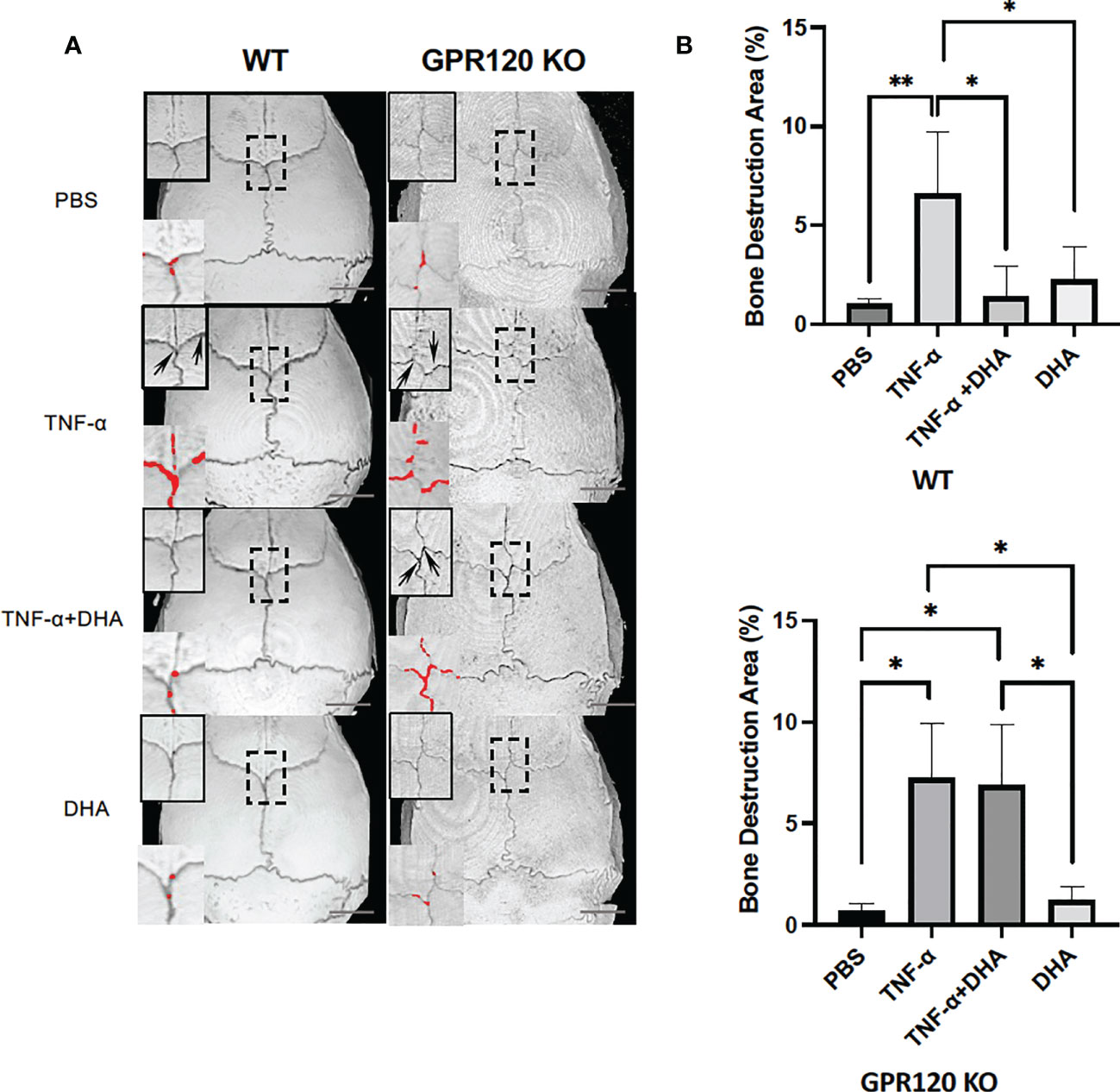
Figure 2 DHA inhibited TNF-α-induced bone resorption in vivo. (A) Three-dimensional reconstructed images of WT and GPR120 mouse calvariae. After receiving daily injections of PBS, TNF-α, TNF-α + DHA, or DHA only for 5 days, calvariae were resected and scanned using micro-CT. Three-dimensional images were created using TRI/3DBON64 software. Bone destruction areas are indicated by red dots and upper left corner zoom in of each image with arrows. (B) The ratio of bone destruction area to total calvarial bone area in WT and GPR120-KO mice. The results are given as means ± SD. Scheffé’s test was used to determine the statistical significance of differences (n = 4; *p < 0.05, **p < 0.01) Scale bars = 2 mm.
DHA inhibited TNF-α-induced RANKL expression in osteoblasts through GPR120 activation in vitro
We analyzed RANKL mRNA expression levels in osteoblasts in vitro to determine the mechanism by which DHA inhibits osteoclast formation through activation of GPR120. RANKL mRNA expression level was higher in the group treated with TNF-α alone than that in the control group (PBS) or the DHA-treated group. In contrast, the group treated with both TNF-α and DHA showed a lower RANKL mRNA expression than the group treated with TNF-α. On the contrary, the expression level of RANKL mRNA was similar in GPR120-KO osteoblasts treated with TNF-α and with TNF-α plus DHA (Figure 3).
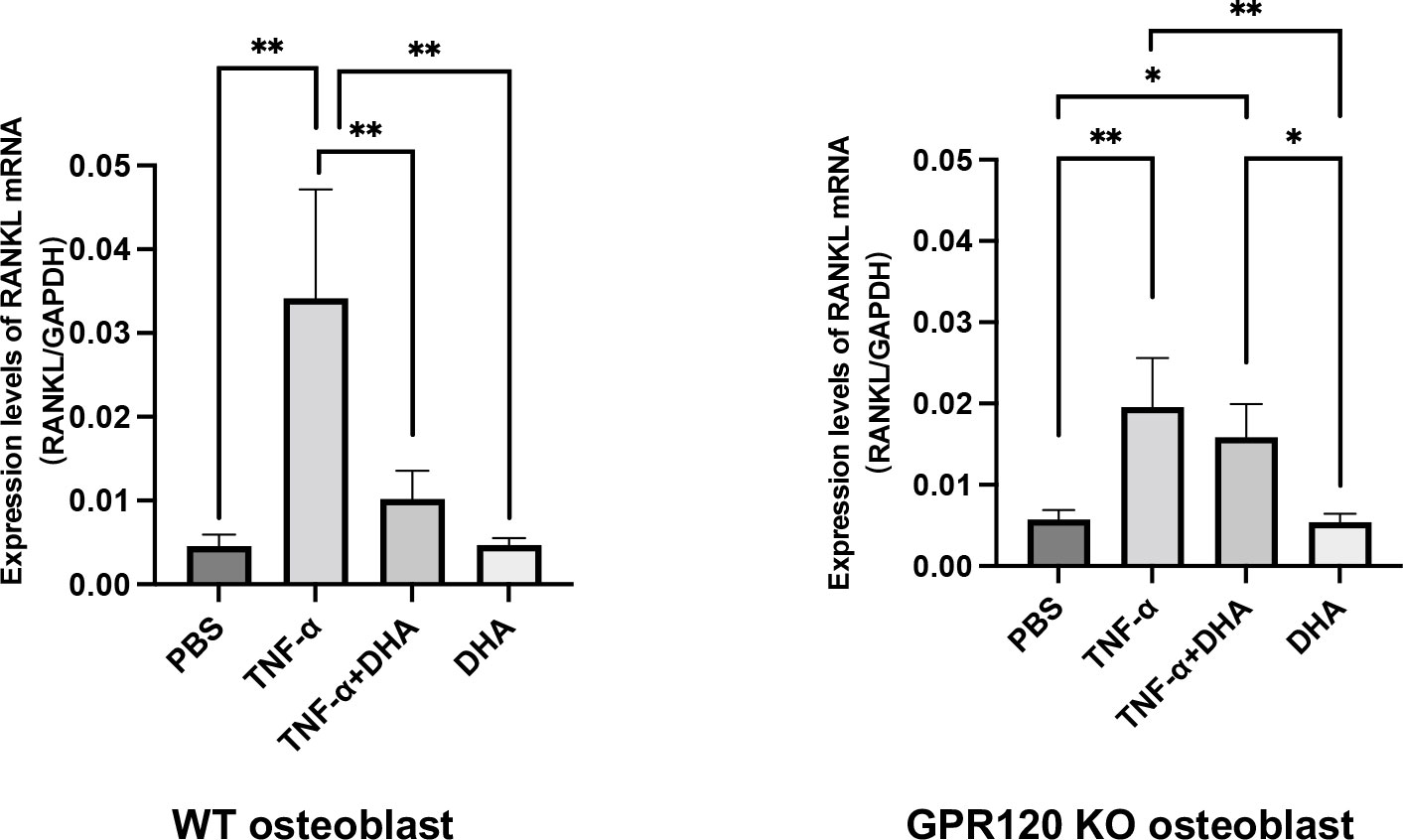
Figure 3 DHA inhibited RANKL expression in osteoblasts via GPR120 in vitro. RANKL mRNA levels of osteoblasts were demonstrated using real-time RT-PCR. Osteoblasts of WT and GPR120-KO mice were cultured with PBS, TNF-α, TNF-α + DHA, or DHA only. The results are given as means ± SD. Scheffé’s test was used to determine the statistical significance of differences (n = 4; *p < 0.05, **p < 0.01).
DHA inhibited TNF-α-enhanced osteoclast formation in co-culture via GPR120 activation in osteoblasts
To examine whether TNF-α induced RANKL in osteoblasts and enhanced osteoclastogenesis, GPR120-KO osteoclast precursors were used to avoid the direct effects of DHA on differentiation of osteoclast precursors to osteoclasts. Osteoblasts from WT mice or GPR120-KO and GPR120-KO osteoclast precursors were cultured with PBS as a control, TNF-α (100 ng/ml), TNF-α (100 ng/ml) and DHA (100 ng/ml), or DHA (100 ng/ml) in the presence of 10−8 M 1,25(OH)2D3 and 10−6 M prostaglandin E2. The numbers of TRAP-positive cells were measured in co-cultures, and these results indicated a significant decrease in the TRAP-positive cell number associated with DHA treatment in co-culture using WT osteoblasts but not GPR120-KO osteoblasts (Figures 4A–D).
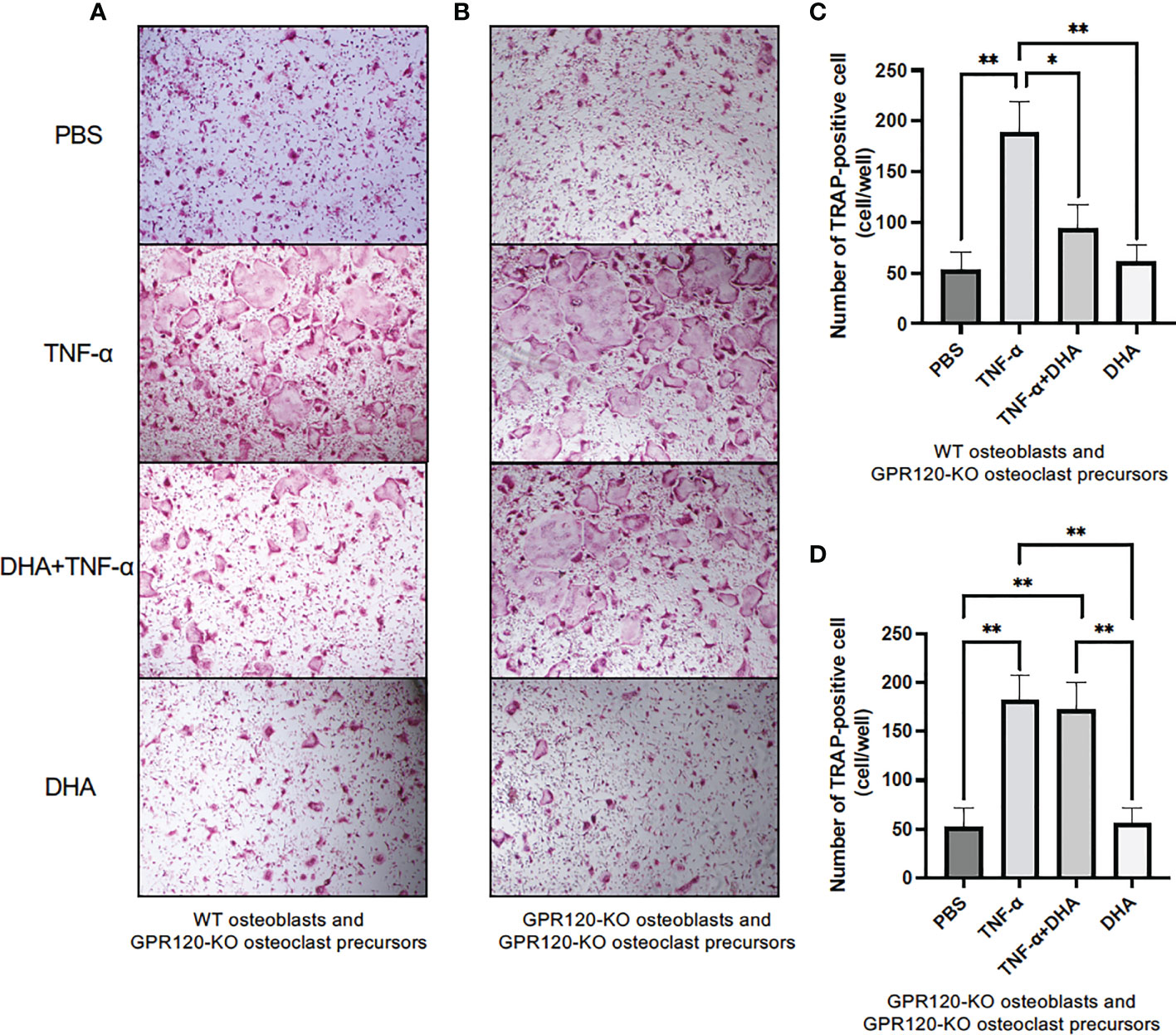
Figure 4 DHA inhibited TNF-α-induced osteoclast formation in co-culture using GPR120-KO osteoclast precursors to avoid the effects of DHA on osteoclastogenesis and WT osteoblasts or GPR120-KO osteoblasts. (A) Images of TRAP-positive cells and (C) the numbers of TRAP-positive cells in co-cultures of GPR120-KO osteoclast precursors and WT osteoblasts cultured with PBS, TNF-α (100 ng/mL), TNF-α + DHA, or DHA only in the presence of prostaglandin E2 and 1,25(OH)2D3 for 4 days. (B) Images of TRAP-positive cells and (D) the numbers of TRAP-positive cells in co-cultures of GPR120-KO osteoclast precursors with GPR120-KO osteoblasts cultured with PBS, TNF-α (100 ng/ml), TNF-α + DHA, or DHA only in the presence of prostaglandin E2 and 1,25(OH)2D3 for 4 days. The results are given as means ± SD. Scheffé’s test was used to determine the statistical significance of differences (n = 4; *p < 0.05, **p < 0.01).
DHA inhibited NF-κB activation induced by TNF-α in osteoblast through GPR120
To investigate the role of DHA in the transcription factor NF-κB pathway, we divided the WT and KO cells into four groups and applied stimulation of PBS as a control, TNF-α (100 ng/ml), TNF-α (100 ng/ml) and DHA (100 ng/ml), or DHA (100 ng/ml). We found that NF-κB nuclear localization significantly increased in WT osteoblasts when osteoblasts received TNF-α stimulation, while it was reduced when DHA and TNF-α were co-administered (Figures 5A, B). This suggests that DHA inhibits the NF-κB signaling pathway, which is activated by TNF-α. However, this inhibitory effect was eliminated in GPR120 KO mice, and DHA did not exert an inhibitory effect on the NF-κB pathway activated by TNF-α in the osteoblasts of GPR120-KO mice (Figures 5A, B).
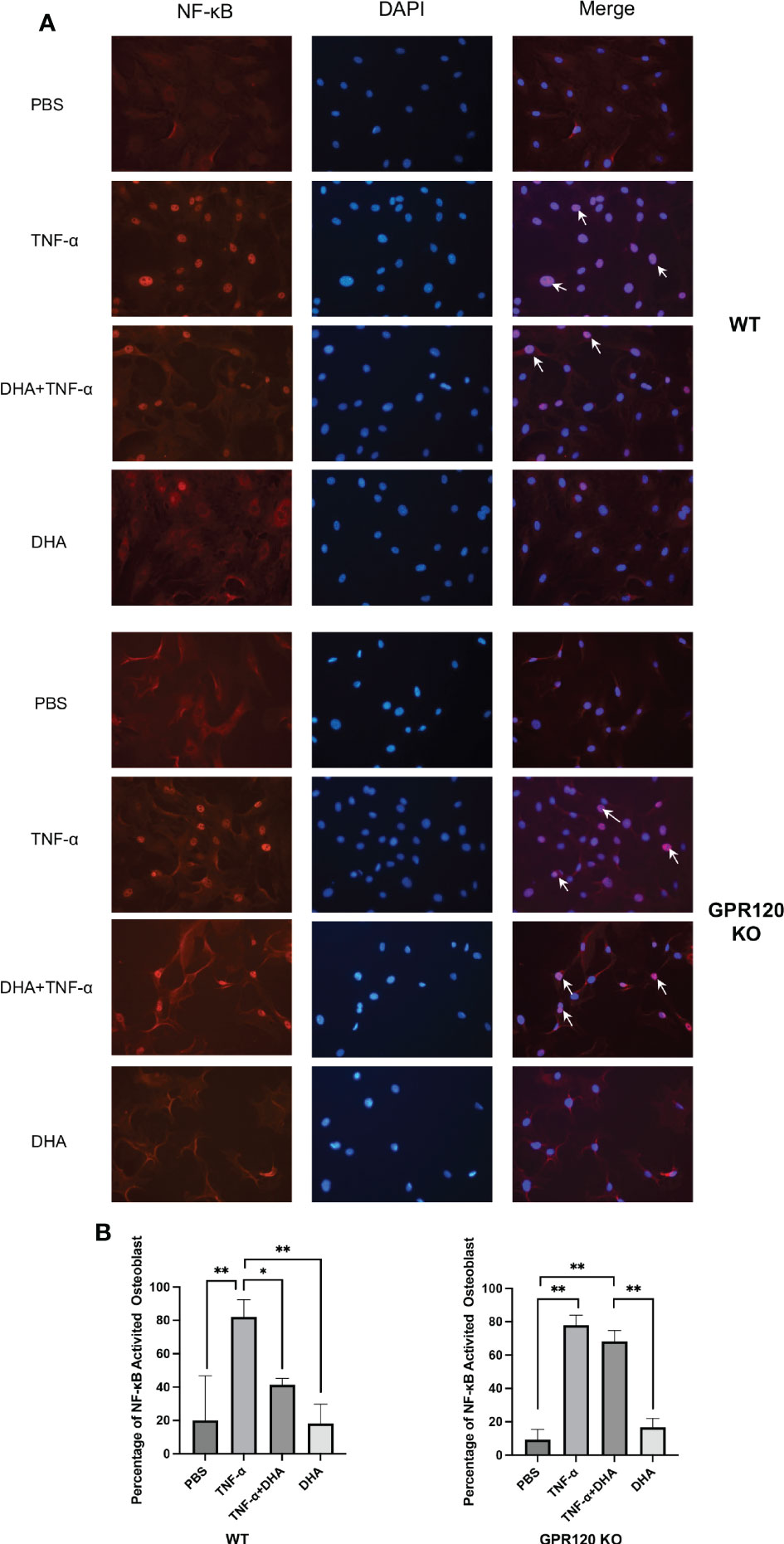
Figure 5 Effect of DHA on NF-κB activation by TNF-α in osteoblast. (A) Fluorescent images of WT osteoblast stained for NF-κB p65 antibody and Alexa Flour 555; DAPI was used as control. NF-κB activated osteoblast were indicated by arrows. (B) NF-κB activated osteoblasts number to the total number of osteoblasts. The results are given as means ± SD. Scheffé’s test was used to determine the statistical significance of differences (n = 4; *p < 0.05, **p < 0.01). Scale bars = 50 μm.
DHA inhibited OTM via GPR120 activation
A 12-day OTM was examined in WT and GPR120-KO mice treated with PBS or DHA. The OTM distance on day 12 was significantly different between control (PBS) and DHA-treated WT mice (143.9 ± 10.0 μm and 99.2 ± 11.4 μm, respectively) (Figures 6A, B). In contrast, the OTM distance in control (PBS) and DHA-treated GPR120-KO mice were at the same level (147.0 ± 7.8 μm and 141.0 ± 16.8 μm, respectively) (Figures 6A, B).
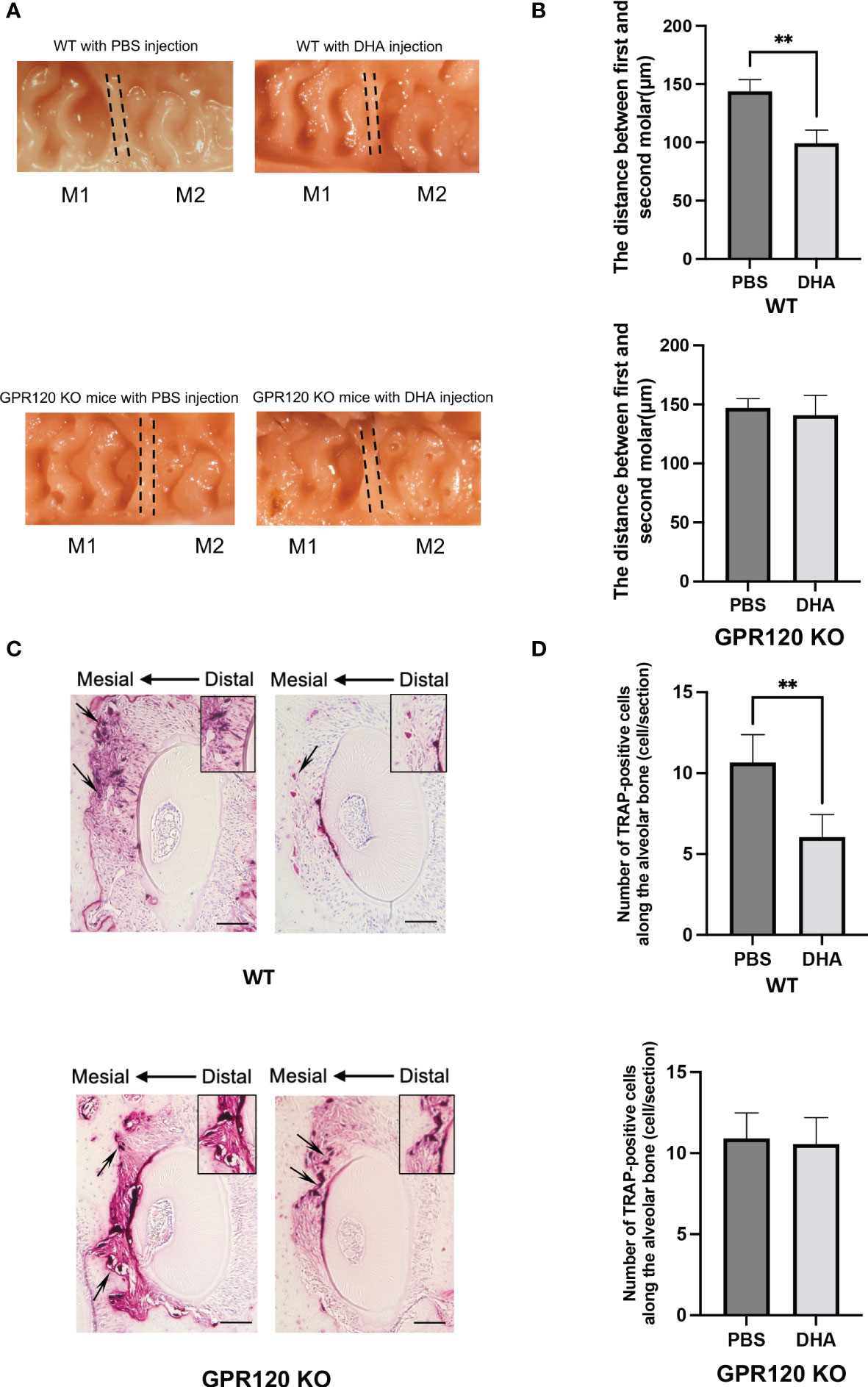
Figure 6 Tooth movement in WT and GPR120-KO mice treated with or without DHA after 12 days of OTM. (A) Images of a 12-day duration of orthodontic tooth movement in WT and GPR120-KO mice with PBS or DHA. (B) Measurement of the distance between the first and the second molar in WT and GPR120-KO mice with PBS or DHA after 12-day force loading. (C) TRAP-stained histological sections of the distobuccal root of the maxillary left first molar of WT and GPR120-KO mice after a 12-day duration of orthodontic tooth movement. The arrows show the osteoclasts formation and bone resorption area along the root. (D) The number of TRAP-positive cells along the alveolar bone surface around the distobuccal root of the left maxillary first molar after 12-day orthodontic tooth movement of WT and GPR120-KO mice treated with PBS or DHA. The results are given as means ± SD. Scheffé’s test was used to determine the statistical significance of differences (n = 4; **p < 0.01). Scale bars = 50 μm.
Sections of five different levels from the distobuccal root of the upper left first molar were subjected to TRAP staining. A large number of TRAP-positive cells were noticed along the alveolar bone in the compression side of the tooth in control WT mice on day 12, but DHA-treated WT mice showed fewer TRAP-positive cells (Figures 6C, D). However, the number of TRAP-positive cells was similar in DHA-treated GPR120-KO mice and the control group on day 12 (Figures 6C, D).
Discussion
DHA has been reported to have several positive impacts on individual health, including anti-inflammatory effects. DHA was reported to inhibit osteoclast formation via the omega-3 fatty acid receptor, GPR120. Orthodontic force induces TNF-α, which activates osteoclast differentiation during OTM. In this study, we investigated the impact of DHA on osteoclast formation, bone resorption induced by TNF-α, and OTM in vivo. Our results indicated that DHA attenuated TNF-α-induced osteoclast formation and bone resorption in WT mice but had no noticeable effect in GPR120-KO mice in vivo, suggesting that the attenuation of TNF-α-induced osteoclast bone resorption by DHA is mediated through GPR120. We previously reported that DHA directly inhibits osteoclast formation via GPR120 in osteoclast precursors. However, the effect of DHA on the expression of RANKL induced by TNF-α in osteoblasts is yet to be elucidated. Therefore, in the present study, we evaluated whether DHA inhibited TNF-α-induced RANKL expression in osteoblasts. The results showed that DHA inhibited TNF-α-induced RANKL expression in osteoblasts. Furthermore, to examine whether DHA inhibited TNF-α-induced RANKL expression in osteoblasts can indirectly inhibit osteoclast formation, we used GPR120-KO osteoclast precursors to avoid the direct effects of DHA and GPR120-KO osteoblasts to eliminate the effects of DHA. Our data demonstrated that DHA inhibited TNF-α-induced osteoclast formation in a co-culture system via activation of GPR120 in osteoblasts. As TNF-α plays a crucial role in OTM, DHA may inhibit TNF-α-induced osteoclast formation and bone resorption during OTM. Therefore, we performed OTM experiments in both WT and GPR120-KO mice with PBS or DHA treatment, which indicated that DHA also suppressed OTM in WT but not in GPR120-KO mice.
DHA was reported to prevent bone loss in ovariectomized mice by inhibiting the production and activation of osteoclasts in vivo (28). Moreover, experiments in rats have shown that perinatal supplementation with DHA reduced bone resorption, decreased osteoclast density, and increased bone mass (49). Treatment of oral Porphyromonas gingivalis infection-induced periodontitis model rats with fish oil containing DHA was reported to result in a marked reduction in alveolar bone resorption (50). Our previous study has suggested that DHA inhibited osteoclastogenesis and bone destruction induced by LPS in vivo, and this effect was ameliorated by the selective GPR120 antagonist, AH7614, in the LPS and DHA treatment group, indicating that DHA prevents LPS-induced osteoclastogenesis through GPR120 in vivo (31). Furthermore, TNF-α induces osteoclast formation both in vivo and in vitro (11–15), and we previously have reported that DHA inhibited TNF-α-induced osteoclast formation in vitro (31). However, the effects of DHA on TNF-α-induced osteoclast formation and bone resorption in vivo were not clear. Therefore, this study was conducted to examine whether DHA inhibited TNF-α-induced osteoclast formation. Daily injection of 100 μg of DHA to the supracalvaria for 5 days suppressed osteoclast formation induced by TNF-α. The impact of DHA on TNF-α-induced bone destruction was examined based on the ratio of bone destruction area to total area on micro-CT images. Consistent with previous studies, the TNF-α and DHA administration group showed significantly reduced bone resorption compared to the group administered TNF-α alone, indicating that DHA suppressed osteoclast formation and bone destruction in vivo (29–31, 51, 52). Using GPR120-KO mice, we also examined whether the suppression of osteoclastogenesis and bone destruction induced by TNF-α via DHA in vivo was mediated through GPR120. Although TNF-α-induced osteoclastogenesis and bone destruction suppressed by DHA in vivo was detected in WT mice, the effects were not observed in GPR120-KO mice. Taken together, the suppression effect of DHA on osteoclastogenesis and bone resorption mediated by TNF-α in vivo is mediated through GPR120.
DHA was concluded to suppress the production of inflammatory cytokines by several types of cells. DHA was shown to prevent IL-6 and TNF-α expression in primary mouse macrophages and the mouse macrophage cell line RAW247.6 by binding to GPR120 (23). DHA inhibits the inflammatory cytokines, such as IL-6, and TNF-α and IL-1β expression induced by LPS in THP-1 cells, which is the human monocytic leukemia cell line (53). Additionally, DHA downregulates the production of IL-1β in bone-marrow-derived macrophages (54). Our previous study showed that the TNF-α mRNA expression level A in macrophages and RANKL mRNA in stromal cells were reduced in mice treated with DHA and LPS compared to those treated with LPS alone (31). There are two possible mechanisms by which DHA suppresses TNF-α-induced osteoclastogenesis and bone resorption in vivo. First, previous studies have shown that DHA may directly inhibit TNF-α-induced osteoclastogenesis by affecting cell differentiation in vivo. Our previous study has shown that DHA directly inhibits TNF-α-induced differentiation of osteoclast progenitors into osteoclasts in vitro (31). Second, DHA may inhibit the TNF-α-induced expression of RANKL, which promotes osteoclast formation, in osteoblasts in vivo. RANKL and TNF-α are known to be essential factors in osteoclast formation (10–13), and several studies have shown that TNF-α induces the expression of RANKL in stromal cells, including osteoblasts and osteocytes (55–62). In this study, RANKL and Cathepsin K mRNA expression increased in mice treated with TNF-α but decreased in mice treated with a combination of DHA and TNF-α. These results correspond with the hypothesis that DHA suppresses osteoclast formation by inhibiting TNF-α-induced RANKL expression. We also examined whether DHA suppresses TNF-α-induced RANKL and Cathepsin K mRNA expression via GPR120 in vivo through experiments using GPR120-KO mice. Our results showed that TNF-α-induced RANKL and Cathepsin K mRNA expression was not inhibited by DHA in GPR120-KO mice, indicating that DHA inhibits TNF-α-induced RANKL and Cathepsin K production via GPR120 in vivo. Taken together, our observations suggest that the inhibitory effect of DHA on TNF-α-induced osteoclast formation in vivo may be due to both reduction in RANKL, Cathepsin K expression, and RANKL/OPG ratio, and a direct effect of DHA on TNF-α-induced osteoclast formation via GPR120 in osteoclast precursors as well.
This study showed that DHA inhibited TNF-α-induced RANKL expression in osteoblasts. As our previous study has shown that DHA directly inhibited TNF-α-induced osteoclast formation (31), we examined whether the inhibition of TNF-α-induced osteoclast formation by DHA was mediated via inhibition of RANKL expression in osteoblasts in a co-culture system using GPR120-KO osteoclast precursors to eliminate the direct effects of DHA on osteoclast precursors and WT osteoblasts. TNF-α was shown to enhance osteoclast formation; however, DHA inhibited TNF-α-enhanced osteoclast formation in co-cultures of GPR120-KO osteoclast precursors and WT osteoblasts. Furthermore, we examined whether DHA inhibited TNF-α-induced osteoclast formation via GPR120 in a co-culture system using GPR120-KO osteoclast precursors and GPR120-KO osteoblasts. No inhibition of osteoclast formation was observed in this co-culture system, suggesting that DHA acts on osteoblasts to decrease RANKL expression induced by TNF-α via activation of GPR120 in osteoblasts.
NF-κB is an important mediator in the process of inflammation, which facilitates intracellular signaling of inflammatory cytokines. Dysregulated NF-κB activation is a sign of chronic inflammatory diseases (63). The p50/p65 dimer of the NF-κB subunits is found in the cytoplasm, where its activation is repressed by IκBα. Upon stimulation in inflammatory conditions such as in rheumatoid arthritis by inflammatory cytokines, the IκB kinase (IKK) complex phosphorylates IκBα for subsequent ubiquitination and proteasome-mediated degradation, and IκBα dissociation induces NF-κB p65 subunit localization to the nucleus and binding to specific gene promoters to regulate pro- and anti-inflammatory protein expression. In this study, we examined the nuclear localization of NF-κB P65 subunit to figure out the effects of DHA on NF-κB signaling in WT and GPR120 KO mice. The results demonstrated that the nuclear localization of P65 was obviously increased in the TNF-α-stimulated group of both WT and GPR120-KO mice. Furthermore, the ratio of NF-κB activated cell was significantly decreased in WT DHA and TNF-α co-administrated group. However, the NF-κB-activated cell remained at the same level in the co-administrated group as TNF-α only group in GPR120-KO mice. These results indicate that DHA inhibits NF-κB activation induced by TNF-α in osteoblast through GPR120.
Several reports have shown that the compressive force in OTM induces the expression of TNF-α, which plays a significant role in osteoclast formation (64–69). Our previous study has also shown that TNF-α plays a crucial role in OTM in mouse models, where the distance of tooth movement was shorter in TNF-receptor-deficient mice than WT mice (41). These results indicated that TNF-α has a significant influence on OTM and therefore suggested that DHA may inhibit TNF-α-induced osteoclast formation during OTM. Regular injection of DHA was shown to inhibit OTM and osteoclast formation during OTM in WT, but not GPR120-KO mice, indicating that the effects of DHA on OTM and osteoclast formation during OTM are mediated via GPR120. Previous studies using chimeric TNF-receptor-deficient mice and WT mice have shown that stromal cells made a greater contribution to TNF-α-induced osteoclast formation than macrophages in vivo (14, 15). These studies concluded that TNF-α-responsive stromal cells contributed more to TNF-α-induced osteoclast formation than macrophages did in vivo. In another study, we also used chimeric TNF-receptor-deficient mice and WT mice to examine the contributions of each cell type in vivo during OTM, and the results indicated that the osteoclast number and distance of OTM were higher in chimeric mice with TNF receptors on stromal cells (70). These findings showed that the TNF-α responsiveness of stromal cells is an essential factor for osteoclastogenesis and bone destruction in OTM. In our present study, DHA was shown to inhibit TNF-α-induced RANKL expression and osteoclast formation in vivo. Additionally, DHA inhibited RANKL expression in osteoblasts via TNF-α in vitro. Furthermore, DHA inhibited OTM and osteoclast formation during OTM. These results suggested that DHA may inhibit TNF-α-responsive osteoblasts in OTM. The results of the present study taken together with those of previous studies suggest that DHA may inhibit TNF-α expression, TNF-α-induced osteoclast formation, and RANKL expression in TNF-α-responsive osteoblasts via GPR120 in OTM.
Conclusions
In this study, we concluded that DHA suppresses bone resorption induced by TNF-α in vivo via GPR120, and the same tendency was also demonstrated in OTM. Additionally, DHA directly restrains RANKL expression in osteoblasts in vitro via GPR120. The mechanisms by which DHA inhibits osteoclast formation and bone destruction induced by TNF-α in vivo were associated with its inhibitory effect on TNF-α-induced RANKL expression in osteoblasts via GPR120 (Figure 7).
Data availability statement
The original contributions presented in the study are included in the article/supplementary material, further inquiries can be directed to the corresponding author.
Ethics statement
All animal care and experiments were approved by the Tohoku University of Science Animal Care and Use Committee.
Author contributions
Conceptualization, JM and HK. Methodology, HK. Validation, JM and HK. Formal analysis, JM, SO, and TN. Investigation, JM, HK, AM, FO, TN, AP, YN, RK, AK, and KK. Resources, HK. Data curation, JM. Writing (original draft preparation), JM. Writing (review and editing), HK. Supervision, AI and IM. Project administration, HK. Funding acquisition, AK, HK, and IM. All authors contributed to the article and approved the submitted version.
Funding
This work was supported in part by JSPS KAKENHI grants from the Japan Society for the Promotion of Science (No. 20K18748 to AK, No. 19K10397 to HK, and No. 18K09862 to IM).
Conflict of interest
The authors declare that the research was conducted in the absence of any commercial or financial relationships that could be construed as a potential conflict of interest.
Publisher’s note
All claims expressed in this article are solely those of the authors and do not necessarily represent those of their affiliated organizations, or those of the publisher, the editors and the reviewers. Any product that may be evaluated in this article, or claim that may be made by its manufacturer, is not guaranteed or endorsed by the publisher.
Abbreviations
DHA, docosahexaenoic acid; GPR120, G protein-coupled receptor 120; TNF-α, tumor necrosis factor-α; OTM, orthodontic tooth movement; WT, wild-type; GPR120-KO, GPR120-deficient; RANKL, receptor activator of nuclear factor kappa-B ligand; NF-kB, nuclear factor-kappa B; M-CSF, macrophage colony stimulating factor; GPRs, G protein-coupled receptors; LPS, lipopolysaccharide; TRAP, tartrate-resistant acid phosphatase; IL, interleukin; PGE2, prostaglandin E2; 1,25(OH)2D3, 1,25 dihydroxyvitamin D3.
References
1. Calder PC. N-3 fatty acids, inflammation, and immunity–relevance to postsurgical and critically ill patients. Lipids (2004) 39(12):1147–61. doi: 10.1007/s11745-004-1342-z
2. Woodman RJ, Watts GF, Puddey IB, Burke V, Mori TA, Hodgson JM, et al. Leukocyte count and vascular function in type 2 diabetic subjects with treated hypertension. Atherosclerosis (2002) 163(1):175–81. doi: 10.1016/s0021-9150(01)00770-5
3. Goldberg RJ. Katz J. a meta-analysis of the analgesic effects of omega-3 polyunsaturated fatty acid supplementation for inflammatory joint pain. Pain (2007) 129(1-2):210–23. doi: 10.1016/j.pain.2007.01.020
4. Galarraga B, Ho M, Youssef HM, Hill A, McMahon H, Hall C, et al. Cod liver oil (N-3 fatty acids) as an non-steroidal anti-inflammatory drug sparing agent in rheumatoid arthritis. Rheumatol (Oxford) (2008) 47(5):665–9. doi: 10.1093/rheumatology/ken024
5. Fritsche K. Fatty acids as modulators of the immune response. Annu Rev Nutr (2006) 26:45–73. doi: 10.1146/annurev.nutr.25.050304.092610
6. Zheng JS, Huang T, Yang J, Fu YQ, Li D. Marine n-3 polyunsaturated fatty acids are inversely associated with risk of type 2 diabetes in asians: A systematic review and meta-analysis. PloS One (2012) 7(9):e44525. doi: 10.1371/journal.pone.0044525
7. Connor WE. Importance of n-3 fatty acids in health and disease. Am J Clin Nutr (2000) 71(1 Suppl):171S–5S. doi: 10.1093/ajcn/71.1.171S
8. Laviano A, Rianda S, Molfino A, Rossi Fanelli F. Omega-3 fatty acids in cancer. Curr Opin Clin Nutr Metab Care (2013) 16(2):156–61. doi: 10.1097/MCO.0b013e32835d2d99
9. Crotti TN, Dharmapatni AA, Alias E, Haynes DR. Osteoimmunology: Major and costimulatory pathway expression associated with chronic inflammatory induced bone loss. J Immunol Res (2015) 2015:281287. doi: 10.1155/2015/281287
10. Teitelbaum SL. Osteoclasts: What do they do and how do they do it? Am J Pathol (2007) 170(2):427–35. doi: 10.2353/ajpath.2007.060834
11. Azuma Y, Kaji K, Katogi R, Takeshita S, Kudo A. Tumor necrosis factor-alpha induces differentiation of and bone resorption by osteoclasts. J Biol Chem (2000) 275(7):4858–64. doi: 10.1074/jbc.275.7.4858
12. Kobayashi K, Takahashi N, Jimi E, Udagawa N, Takami M, Kotake S, et al. Tumor necrosis factor alpha stimulates osteoclast differentiation by a mechanism independent of the Odf/Rankl-rank interaction. J Exp Med (2000) 191(2):275–86. doi: 10.1084/jem.191.2.275
13. Fuller K, Murphy C, Kirstein B, Fox SW, Chambers TJ. Tnfalpha potently activates osteoclasts, through a direct action independent of and strongly synergistic with rankl. Endocrinology (2002) 143(3):1108–18. doi: 10.1210/endo.143.3.8701
14. Kitaura H, Sands MS, Aya K, Zhou P, Hirayama T, Uthgenannt B, et al. Marrow stromal cells and osteoclast precursors differentially contribute to tnf-Alpha-Induced osteoclastogenesis in vivo. J Immunol (2004) 173(8):4838–46. doi: 10.4049/jimmunol.173.8.4838
15. Kitaura H, Zhou P, Kim HJ, Novack DV, Ross FP, Teitelbaum SL. M-csf mediates tnf-induced inflammatory osteolysis. J Clin Invest (2005) 115(12):3418–27. doi: 10.1172/JCI26132
16. Wong M, Ziring D, Korin Y, Desai S, Kim S, Lin J, et al. Tnfalpha blockade in human diseases: Mechanisms and future directions. Clin Immunol (2008) 126(2):121–36. doi: 10.1016/j.clim.2007.08.013
17. Wellhauser L, Belsham DD. Activation of the omega-3 fatty acid receptor Gpr120 mediates anti-inflammatory actions in immortalized hypothalamic neurons. J Neuroinflamm (2014) 11:60. doi: 10.1186/1742-2094-11-60
18. Oh DY, Lagakos WS. The role of G-Protein-Coupled receptors in mediating the effect of fatty acids on inflammation and insulin sensitivity. Curr Opin Clin Nutr Metab Care (2011) 14(4):322–7. doi: 10.1097/MCO.0b013e3283479230
19. Talukdar S, Olefsky JM, Osborn O. Targeting Gpr120 and other fatty acid-sensing gpcrs ameliorates insulin resistance and inflammatory diseases. Trends Pharmacol Sci (2011) 32(9):543–50. doi: 10.1016/j.tips.2011.04.004
20. Yonezawa T, Kurata R, Yoshida K, Murayama MA, Cui X, Hasegawa A. Free fatty acids-sensing G protein-coupled receptors in drug targeting and therapeutics. Curr Med Chem (2013) 20(31):3855–71. doi: 10.2174/09298673113209990168
21. Itoh Y, Kawamata Y, Harada M, Kobayashi M, Fujii R, Fukusumi S, et al. Free fatty acids regulate insulin secretion from pancreatic beta cells through Gpr40. Nature (2003) 422(6928):173–6. doi: 10.1038/nature01478
22. Hirasawa A, Tsumaya K, Awaji T, Katsuma S, Adachi T, Yamada M, et al. Free fatty acids regulate gut incretin glucagon-like peptide-1 secretion through Gpr120. Nat Med (2005) 11(1):90–4. doi: 10.1038/nm1168
23. Oh DY, Talukdar S, Bae EJ, Imamura T, Morinaga H, Fan W, et al. Gpr120 is an omega-3 fatty acid receptor mediating potent anti-inflammatory and insulin-sensitizing effects. Cell (2010) 142(5):687–98. doi: 10.1016/j.cell.2010.07.041
24. Ichimura A, Hirasawa A, Poulain-Godefroy O, Bonnefond A, Hara T, Yengo L, et al. Dysfunction of lipid sensor Gpr120 leads to obesity in both mouse and human. Nature (2012) 483(7389):350–4. doi: 10.1038/nature10798
25. Yan Y, Jiang W, Spinetti T, Tardivel A, Castillo R, Bourquin C, et al. Omega-3 fatty acids prevent inflammation and metabolic disorder through inhibition of Nlrp3 inflammasome activation. Immunity (2013) 38(6):1154–63. doi: 10.1016/j.immuni.2013.05.015
26. Oh DY, Walenta E, Akiyama TE, Lagakos WS, Lackey D, Pessentheiner AR, et al. A Gpr120-selective agonist improves insulin resistance and chronic inflammation in obese mice. Nat Med (2014) 20(8):942–7. doi: 10.1038/nm.3614
27. Yore MM, Syed I, Moraes-Vieira PM, Zhang T, Herman MA, Homan EA, et al. Discovery of a class of endogenous mammalian lipids with anti-diabetic and anti-inflammatory effects. Cell (2014) 159(2):318–32. doi: 10.1016/j.cell.2014.09.035
28. Sun D, Krishnan A, Zaman K, Lawrence R, Bhattacharya A, Fernandes G. Dietary n-3 fatty acids decrease osteoclastogenesis and loss of bone mass in ovariectomized mice. J Bone Miner Res (2003) 18(7):1206–16. doi: 10.1359/jbmr.2003.18.7.1206
29. Kasonga AE, Deepak V, Kruger MC, Coetzee M. Arachidonic acid and docosahexaenoic acid suppress osteoclast formation and activity in human Cd14+ monocytes, in vitro. PloS One (2015) 10(4):e0125145. doi: 10.1371/journal.pone.0125145
30. Rahman MM, Bhattacharya A, Fernandes G. Docosahexaenoic acid is more potent inhibitor of osteoclast differentiation in raw 264.7 cells than eicosapentaenoic acid. J Cell Physiol (2008) 214(1):201–9. doi: 10.1002/jcp.21188
31. Kishikawa A, Kitaura H, Kimura K, Ogawa S, Qi J, Shen WR, et al. Docosahexaenoic acid inhibits inflammation-induced osteoclast formation and bone resorption in vivo through Gpr120 by inhibiting tnf-alpha production in macrophages and directly inhibiting osteoclast formation. Front Endocrinol (Lausanne) (2019) 10:157. doi: 10.3389/fendo.2019.00157
32. Kim HJ, Yoon HJ, Kim BK, Kang WY, Seong SJ, Lim MS, et al. G Protein-coupled receptor 120 signaling negatively regulates osteoclast differentiation, survival, and function. J Cell Physiol (2016) 231(4):844–51. doi: 10.1002/jcp.25133
33. Kasonga AE, Kruger MC, Coetzee M. Free fatty acid receptor 4-Beta-Arrestin 2 pathway mediates the effects of different classes of unsaturated fatty acids in osteoclasts and osteoblasts. Biochim Biophys Acta Mol Cell Biol Lipids (2019) 1864(3):281–9. doi: 10.1016/j.bbalip.2018.12.009
34. Alhashimi N, Frithiof L, Brudvik P, Bakhiet M. Chemokines are upregulated during orthodontic tooth movement. J Interferon Cytokine Res (1999) 19(9):1047–52. doi: 10.1089/107999099313271
35. Terai K, Takano-Yamamoto T, Ohba Y, Hiura K, Sugimoto M, Sato M, et al. Role of osteopontin in bone remodeling caused by mechanical stress. J Bone Miner Res (1999) 14(6):839–49. doi: 10.1359/jbmr.1999.14.6.839
36. Krishnan V, Davidovitch Z. On a path to unfolding the biological mechanisms of orthodontic tooth movement. J Dent Res (2009) 88(7):597–608. doi: 10.1177/0022034509338914
37. Masella RS, Meister M. Current concepts in the biology of orthodontic tooth movement. Am J Orthod Dentofacial Orthop (2006) 129(4):458–68. doi: 10.1016/j.ajodo.2005.12.013
38. Meikle MC. The tissue, cellular, and molecular regulation of orthodontic tooth movement: 100 years after Carl sandstedt. Eur J Orthod (2006) 28(3):221–40. doi: 10.1093/ejo/cjl001
39. Alhashimi N, Frithiof L, Brudvik P, Bakhiet M. Orthodontic tooth movement and De novo synthesis of proinflammatory cytokines. Am J Orthod Dentofacial Orthop (2001) 119(3):307–12. doi: 10.1067/mod.2001.110809
40. Ohori F, Kitaura H, Ogawa S, Shen WR, Qi J, Noguchi T, et al. Il-33 inhibits tnf-Alpha-Induced osteoclastogenesis and bone resorption. Int J Mol Sci (2020) 21(3):1130. doi: 10.3390/ijms21031130
41. Kitaura H, Yoshimatsu M, Fujimura Y, Eguchi T, Kohara H, Yamaguchi A, et al. An anti-C-Fms antibody inhibits orthodontic tooth movement. J Dent Res (2008) 87(4):396–400. doi: 10.1177/154405910808700405
42. Yoshimatsu M, Shibata Y, Kitaura H, Chang X, Moriishi T, Hashimoto F, et al. Experimental model of tooth movement by orthodontic force in mice and its application to tumor necrosis factor receptor-deficient mice. J Bone Miner Metab (2006) 24(1):20–7. doi: 10.1007/s00774-005-0641-4
43. Takeshita S, Kaji K, Kudo A. Identification and characterization of the new osteoclast progenitor with macrophage phenotypes being able to differentiate into mature osteoclasts. J Bone Miner Res (2000) 15(8):1477–88. doi: 10.1359/jbmr.2000.15.8.1477
44. Noguchi T, Kitaura H, Ogawa S, Qi J, Shen WR, Ohori F, et al. Tnf-alpha stimulates the expression of rank during orthodontic tooth movement. Arch Oral Biol (2020) 117:104796. doi: 10.1016/j.archoralbio.2020.104796
45. Bakker AD, Klein-Nulend J. Osteoblast isolation from murine calvaria and long bones. Methods Mol Biol (2012) 816:19–29. doi: 10.1007/978-1-61779-415-5_2
46. Shima K, Kimura K, Ishida M, Kishikawa A, Ogawa S, Qi J, et al. C-X-C motif chemokine 12 enhances lipopolysaccharide-induced osteoclastogenesis and bone resorption in vivo. Calcif Tissue Int (2018) 103(4):431–42. doi: 10.1007/s00223-018-0435-z
47. Nara Y, Kitaura H, Ogawa S, Shen WR, Qi J, Ohori F, et al. Anti-C-Fms antibody prevents osteoclast formation and bone resorption in Co-culture of osteoblasts and osteoclast precursors in vitro and in ovariectomized mice. Int J Mol Sci (2020) 21(17):6120. doi: 10.3390/ijms21176120
48. Shen WR, Kitaura H, Qi J, Ogawa S, Ohori F, Noguchi T, et al. Local administration of high-dose diabetes medicine exendin-4 inhibits orthodontic tooth movement in mice. Angle Orthod (2021) 91(1):111–8. doi: 10.2319/021320-103.1
49. Fong L, Muhlhausler BS, Gibson RA, Xian CJ. Perinatal maternal dietary supplementation of Omega3-fatty acids transiently affects bone marrow microenvironment, osteoblast and osteoclast formation, and bone mass in Male offspring. Endocrinology (2012) 153(5):2455–65. doi: 10.1210/en.2011-1917
50. Kesavalu L, Vasudevan B, Raghu B, Browning E, Dawson D, Novak JM, et al. Omega-3 fatty acid effect on alveolar bone loss in rats. J Dent Res (2006) 85(7):648–52. doi: 10.1177/154405910608500713
51. Boeyens JC, Deepak V, Chua WH, Kruger MC, Joubert AM, Coetzee M. Effects of Omega3- and Omega6-polyunsaturated fatty acids on rankl-induced osteoclast differentiation of Raw264.7 cells: A comparative in vitro study. Nutrients (2014) 6(7):2584–601. doi: 10.3390/nu6072584
52. Kim HJ, Ohk B, Yoon HJ, Kang WY, Seong SJ, Kim SY, et al. Docosahexaenoic acid signaling attenuates the proliferation and differentiation of bone marrow-derived osteoclast precursors and promotes apoptosis in mature osteoclasts. Cell Signal (2017) 29:226–32. doi: 10.1016/j.cellsig.2016.11.007
53. Weldon SM, Mullen AC, Loscher CE, Hurley LA, Roche HM. Docosahexaenoic acid induces an anti-inflammatory profile in lipopolysaccharide-stimulated human thp-1 macrophages more effectively than eicosapentaenoic acid. J Nutr Biochem (2007) 18(4):250–8. doi: 10.1016/j.jnutbio.2006.04.003
54. Williams-Bey Y, Boularan C, Vural A, Huang NN, Hwang IY, Shan-Shi C, et al. Omega-3 free fatty acids suppress macrophage inflammasome activation by inhibiting nf-kappab activation and enhancing autophagy. PloS One (2014) 9(6):e97957. doi: 10.1371/journal.pone.0097957
55. Marahleh A, Kitaura H, Ohori F, Kishikawa A, Ogawa S, Shen WR, et al. Tnf-alpha directly enhances osteocyte rankl expression and promotes osteoclast formation. Front Immunol (2019) 10:2925. doi: 10.3389/fimmu.2019.02925
56. Rossa C, Ehmann K, Liu M, Patil C, Kirkwood KL. Mkk3/6-P38 mapk signaling is required for il-1beta and tnf-Alpha-Induced rankl expression in bone marrow stromal cells. J Interferon Cytokine Res (2006) 26(10):719–29. doi: 10.1089/jir.2006.26.719
57. Kitaura H, Marahleh A, Ohori F, Noguchi T, Shen WR, Qi J, et al. Osteocyte-related cytokines regulate osteoclast formation and bone resorption. Int J Mol Sci (2020) 21(14):5169. doi: 10.3390/ijms21145169
58. Yang B, Sun H, Xu X, Zhong H, Wu Y, Wang J. Yap1 inhibits the induction of tnf-Alpha-Stimulated bone-resorbing mediators by suppressing the nf-kappab signaling pathway in Mc3t3-E1 cells. J Cell Physiol (2020) 235(5):4698–708. doi: 10.1002/jcp.29348
59. Di Benedetto G, Lempereur L, Valle D, Greco EA, Bernardini R, Lenzi A, et al. Redundant modulatory effects of proinflammatory cytokines in human osteoblastic cells in vitro. Clin Exp Rheumatol (2018) 36(6):959–69.
60. Pacios S, Xiao W, Mattos M, Lim J, Tarapore RS, Alsadun S, et al. Osteoblast lineage cells play an essential role in periodontal bone loss through activation of nuclear factor-kappa b. Sci Rep (2015) 5:16694. doi: 10.1038/srep16694
61. Wada N, Maeda H, Yoshimine Y, Akamine A. Lipopolysaccharide stimulates expression of osteoprotegerin and receptor activator of nf-kappa b ligand in periodontal ligament fibroblasts through the induction of interleukin-1 beta and tumor necrosis factor-alpha. Bone (2004) 35(3):629–35. doi: 10.1016/j.bone.2004.04.023
62. Hofbauer LC, Lacey DL, Dunstan CR, Spelsberg TC, Riggs BL, Khosla S. Interleukin-1beta and tumor necrosis factor-alpha, but not interleukin-6, stimulate osteoprotegerin ligand gene expression in human osteoblastic cells. Bone (1999) 25(3):255–9. doi: 10.1016/s8756-3282(99)00162-3
63. Liu T, Zhang L, Joo D, Sun SC. Nf-kappab signaling in inflammation. Signal Transduct Target Ther (2017) 2:e17023. doi: 10.1038/sigtrans.2017.23
64. Andrade I Jr., Silva TA, Silva GA, Teixeira AL, Teixeira MM. The role of tumor necrosis factor receptor type 1 in orthodontic tooth movement. J Dent Res (2007) 86(11):1089–94. doi: 10.1177/154405910708601113
65. Basaran G, Ozer T, Kaya FA, Kaplan A, Hamamci O. Interleukine-1beta and tumor necrosis factor-alpha levels in the human gingival sulcus during orthodontic treatment. Angle Orthod (2006) 76(5):830–6. doi: 10.1043/0003-3219(2006)076[0830:IATNFL]2.0.CO;2
66. Garlet TP, Coelho U, Silva JS, Garlet GP. Cytokine expression pattern in compression and tension sides of the periodontal ligament during orthodontic tooth movement in humans. Eur J Oral Sci (2007) 115(5):355–62. doi: 10.1111/j.1600-0722.2007.00469.x
67. Lowney JJ, Norton LA, Shafer DM, Rossomando EF. Orthodontic forces increase tumor necrosis factor alpha in the human gingival sulcus. Am J Orthod Dentofacial Orthop (1995) 108(5):519–24. doi: 10.1016/s0889-5406(95)70052-8
68. Ren Y, Hazemeijer H, de Haan B, Qu N, de Vos P. Cytokine profiles in crevicular fluid during orthodontic tooth movement of short and long durations. J Periodontol (2007) 78(3):453–8. doi: 10.1902/jop.2007.060261
69. Uematsu S, Mogi M, Deguchi T. Interleukin (Il)-1 beta, il-6, tumor necrosis factor-alpha, epidermal growth factor, and beta 2-microglobulin levels are elevated in gingival crevicular fluid during human orthodontic tooth movement. J Dent Res (1996) 75(1):562–7. doi: 10.1177/00220345960750010801
Keywords: osteoclast, TNF-α, GPR120, DHA, orthodontic tooth movement
Citation: Ma J, Kitaura H, Ogawa S, Ohori F, Noguchi T, Marahleh A, Nara Y, Pramusita A, Kinjo R, Kanou K, Kishikawa A, Ichimura A and Mizoguchi I (2023) Docosahexaenoic acid inhibits TNF-α-induced osteoclast formation and orthodontic tooth movement through GPR120. Front. Immunol. 13:929690. doi: 10.3389/fimmu.2022.929690
Received: 10 May 2022; Accepted: 30 December 2022;
Published: 18 January 2023.
Edited by:
Sun-Kyeong Lee, University of Connecticut Health Center, United StatesReviewed by:
I-Ping Chen, University of Connecticut Health Center, United StatesZaki Hakami, Jazan University, Saudi Arabia
Copyright © 2023 Ma, Kitaura, Ogawa, Ohori, Noguchi, Marahleh, Nara, Pramusita, Kinjo, Kanou, Kishikawa, Ichimura and Mizoguchi. This is an open-access article distributed under the terms of the Creative Commons Attribution License (CC BY). The use, distribution or reproduction in other forums is permitted, provided the original author(s) and the copyright owner(s) are credited and that the original publication in this journal is cited, in accordance with accepted academic practice. No use, distribution or reproduction is permitted which does not comply with these terms.
*Correspondence: Hideki Kitaura, aGlkZWtpLmtpdGF1cmEuYjRAdG9ob2t1LmFjLmpw