- 1Department of Dermatology, University Hospital, Westfälische Wilhelms-University Münster, Münster, Germany
- 2Institute for Immunology, University Medical Center, Johannes Gutenberg University Mainz, Mainz, Germany
Dendritic cells (DC) are uniquely capable of initiating and directing immune responses. The range of their activities grounds in the heterogeneity of DC subsets and their functional plasticity. Numerical and functional DC changes influence the development and progression of disease, and correction of such dysregulations has the potential to treat disease causally. In this review, we discuss the major advances in our understanding of the regulation of DC lineage formation, differentiation, and function in the skin. We describe the alteration of DC in disease as well as possibilities for therapeutic reprogramming with a focus on tolerogenic DC. Because regulatory T cells (Treg) are indispensable partners of DC in the induction and control of tolerance, we pay special attention to the interactions with these cells. Above all, we would like to arouse fascination for this cell type and its therapeutic potential in skin diseases.
Introduction: DC Subtypes and Ontogeny
In humans, the haematopoiesis is initiated in the yolk-sac around days 16-18 of estimated gestational age (EGA), followed by the migration of immature hematopoietic stem cells, derived from the aorta-gonad-mesonephros, to the definitive location of pre-natal haematopoiesis; the fetal liver (1, 2). The adult myeloid compartment originates from precursor cells within the bone marrow. Common myeloid progenitors (CMP) have the ability to differentiate towards all adult myeloid cells in humans. The myeloid progenitor cell loses its capacity to produce megakaryocytes, erythroid, eosinophil and basophil cells as they become granulocyte macrophage DC progenitors (GMDP). GMDPs eventually acquire the phenotype of macrophage DC progenitors (MDP), which give rise to monocytes or CD123+ common DC progenitors (CDP). The latter is capable of differentiating towards CD141+ DC type 1 (cDC1) or CD1c+ DC type 2 (cDC2), as well as CD123+ plasmacytoid dendritic cells (pDC). Villani et al. (3) identified two distinct populations within cDC2 in blood and showed that CLEC9a could serve as a more suitable marker to identify cDC1 compared to CD141. Besides the bone-marrow precursors, monocytes can replenish the DC reservoir especially during inflammation (4). It appears that monocyte-derived DC (moDC) arise primarily from CD14+CD16− monocytes and carry the markers CD1c and CD1a in addition to CD11c (Table 1).
In humans, cDC1 and cDC2 are located in the dermis. Furthermore, the inflammatory environment in the skin promotes the development of moDC and the recruitment of pDC. The epidermis harbours Langerhans cells (LC) which are marked by the expression of Langerin, CD1a and E-cadherin (5). Although they demonstrate functional characteristics of DC, LC have been reclassified as tissue-resident macrophages (6). The same is true for dermal CD14+ cells, previously defined as DC, which most likely represent monocyte-derived macrophages (2). Lineage-tracing experiments in mice deciphered the role of transcription factors in DC development: cDC1 differentiation involves the transcription factors IRF8, IRF4, Id2 (7) and Batf3. Batf3 and IRF8 in particular appear to be indispensable for cDC1 development (5). Differentiation towards cDC2 in mice requires the expression of Id2 and Zeb2 (8), but IRF4 and Notch2/KLF4 expression is also required (5). Differentiation towards pDC requires the expression of IRF8 and IRF4 (5). Furthermore, ZEB2, a protein involved in epithelial-mesenchymal transition, has been described as a critical factor for the development of pDC. Deletion of zeb2 in murine bone marrow precursors lead to a drastic reduction of pDC in vitro and in vivo, presumably through the abrogation of zeb2-induced repression of id2. Loss of ZEB2 favours the development of cDC1 above pDC and appears to be of critical importance for the fate choice of CDP. Finally, moDC formation depends on the transcription factors MAFB and KLF4 (5) (Table 1).
Yolk-sac derived macrophages serve as transient progenitors of LC, but monocytes derived from the fetal liver replace them later on (9). In the adult skin, LC are self-sustaining with no contribution of bone marrow-derived cells (10). In mice, CSFR-1 receptor engagement (11) as well as transcription of runx3 and id2 are substantial in the maintenance of LC homeostasis.
In addition to phenotypic/functional classifications, DC classify by their migratory capabilities and differentiation stage. Attempts have been made to distinguish resident DC from migrating DC based on markers. For example, resident DC (pDC or cDC subgroups such as CD8+ DC or CD8-/CD11b+ DC) in lymphoid organs (12) or migrating DC from peripheral tissues and non-lymphoid organs (including CD103+/Langerin+/CD11b+) can be distinguished (13). However, however, all DC migrate both in steady state and in inflammation. In the blood, a population of pre-DC forms a subset that is functionally distinct from pDCs and cDCs. AXL and Siglec6 (CD327) expressing cells (AS DC) strongly stimulate T cells in lymphoid tissue. AS DC may also represent a mature cDC2 progenitor and are likely in transition to this subgroup (3).
The description of immature (iDC) and mature (mDC) phenotypes primarily refers to the maturation of DC in vitro. So-called iDC express CD11c but little major histocompatibility complex class II (MHC-II) and costimulatory molecules such as CD80, CD83 and CD86, while mDC show an upregulated expression of these costimulatory molecules as well as MHC-II (14). Maturation also occurs in vivo, of course, and the markers described in vitro are similarly altered.
Key Questions and Future Directions for the Field
The increasing use of scRNA-seq has led to the description of additional DC subtypes. Transcriptomes, however, incompletely reflect the cell state as gene transcription is stochastic, characterized by transcriptional bursts of varying intensity (15) and half-lives of individual mRNA molecules vary considerably (16). Therefore, there is a need to determine whether the putative heterogeneity of DC, as evidenced by scRNA-seq, also reflects functional differences.
Dendritic Cell Functions
DC are versatile cells that act as sensors for pathogens and gatekeepers of tolerance at the same time. They link innate signals (pattern recognition and early inflammatory mediators) to adaptive immune responses (T cell priming and Treg induction). DC functions are diverse and highly dependent on circumstances such as cytokines, tissue and cell origin. DC properties, including surface molecules and secreted soluble mediators, can result in tolerogenic as well as stimulatory effects. This means that their function can be beneficial in relation to peripheral tolerance induction or disadvantageous as in transplant rejection (17).
DC decide on the quality and quantity of the immune response simply by their degree of activation (Figure 2). As professional antigen-presenting dendritic cells (APCs), they take antigens from their environment, process them and provide co-stimulation that can prime naïve T cells unlike any other MHCII-carrying cell in the body.
Factors that drive DC activation are hallmarks of infection and inflammation such as danger (DAMP) or pathogen associated molecular patterns (PAMP) as well as inflammatory cytokines. DAMPs are molecules or cellular structures released because of cell death or trauma, as heat shock proteins, histones and mitochondrial components. PAMPs are components of pathogens such as LPS, peptidoglycans or bacterial and viral nucleic acids. Recognition of PAMPs or DAMPs via toll-like receptors (TLR), C-type lectins or RIG-I-like receptors or sensing of inflammatory cytokines such as tumor necrosis factor (TNF) alpha, interleukins 1 and 6 via cytokine receptors (18) induces a differentiation program in DC that has been termed “mature” (18). Such mature DC then express costimulatory molecules, MHC-II and chemokine receptors, which facilitate migration to the lymphoid organs and secretion of elevated levels of inflammatory cytokines. It must be noted, however, that although a DC exhibits characteristics that are termed “mature”, it may have non-immunogenic functions such as tolerance maintenance. At specific anatomical sites (thymus) DC exert tolerogenic functions despite being in a potentially inflammatory environment. Interesting in this regard is the NF-κB pathway. NF-κB is known as a target gene of TNF-α signalling but can also induce TNF- expression during DC maturation. Earlier reports suggest that NF-κB1 is involved in the repression of TNF-α expression in immature DCs by itself (19, 20). Steady-state DC actively induce a tolerance-promoting state (regulatory T-cell (Treg) induction) or render T-cells unresponsive (anergy) (21). Tolerogenic maturation has been shown to depend on IRF4, and ablation of IRF4 in DC impairs peripheral tolerance induction (22, 23) (Figure 1).
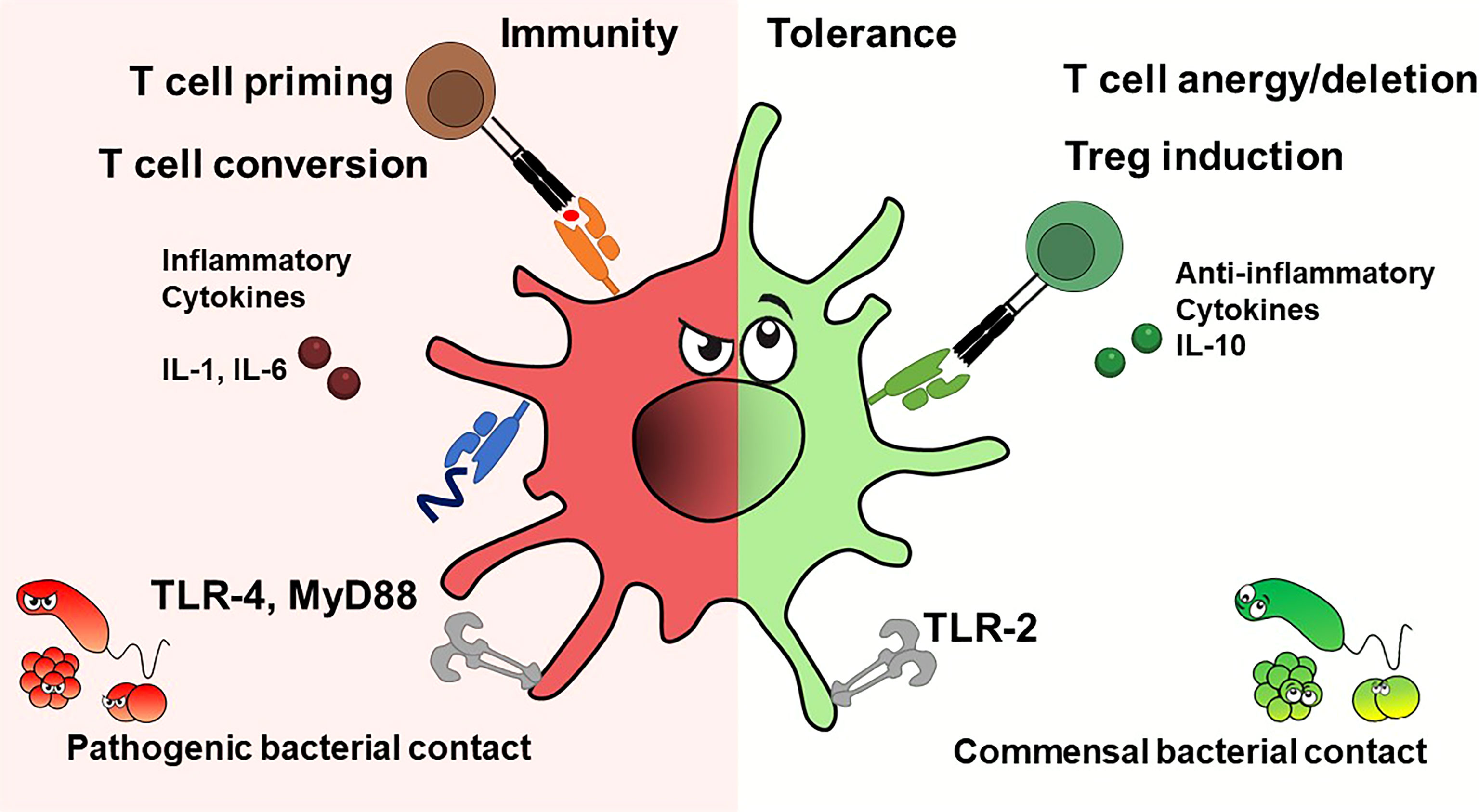
Figure 1 Dendritic cells exhibit immunostimulatory or tolerogenic potential depending on the context. DC's fate depends on cytokines and pathogen contact. Inflammatory cytokines and pathogens presenting TLR ligands promote T effector cells, while anti-inflammatory cytokines or interactions with commensal bacterial components produce tolerance, e.g. induce T cell anergy or deletion, or give rise to regulatory T cells.
Interestingly, human tolerogenic DC (IL10-DC) matured in vitro in the presence of interleukin-10 induce functional regulatory T-cells regardless of their degree of maturation (CD83 low and high) (24, 25). In addition, DC express distinct toll-like receptors (TLRs), which supports their functional specialization, a process that is highly context- and thus cytokine-dependent. A tolerance-promoting function via IL-10 has been reported for (26, 27). Because of its barrier function, the skin also harbours commensal bacteria, which colonize the barrier and thus protect the body from colonization by pathogens. The immune system senses patterns expressed by skin commensals. As previously shown, CD103+ DC take up antigens or commensals in the skin or hair follicles and travel to the draining lymph nodes to activate T cells. Primed T cells then migrate back to the skin where they augment the antimicrobial properties of keratinocytes via T cell-produced IL-17 (28). Aside from being potent inducers of CD4+ and CD8+ T cell-mediated adaptive responses, DC can also have direct effects on bacteria. The production of TNF-α and inducible nitric oxide synthase (iNOS) by so-called TipDC has shown that DC can eliminate bacteria in the murine L. monocytogenes infection model. TipDC had allostimulatory capacity in mixed leukocyte reactions but were not required for effective priming of CD4 and CD8 T cells in vivo (29). Skin resident LC phagocytes serve as skin guardians, orchestrate immune responses against pathogens, but have also been implicated to induce tolerance to skin’s own antigens under steady-state conditions (30, 31). Local ablation of epidermal LC worsened the dermal phenotype in a lupus mouse model but had no systemic effect on the effects of autoimmune responses. LC regulate skin tolerance via IL-10-producing CD4+ T cells, but IL-10 levels in skin-draining lymph nodes were unaffected (32) (Figure 1).
Human pDC constitutively express the serine protease granzyme B (GrB) (33). GrB production in human pDC is stimulated by IL-21 but inhibited by autocrine production of type I interferons (34). Similarly, IL-10 can increase GrB secretion from pDC, whereas Toll-like receptor agonists and CD40 ligand strongly inhibit them (35). GrB+ pDC suppress T cell proliferation in a GrB-dependent, perforin-independent manner, a process reminiscent of Treg. GrB-secreting pDC may therefore play a regulatory role in tumor immune defense, antiviral immune responses, and autoimmune processes. In inflammatory processes, CD8⁺ T cells trigger perforin-mediated apoptosis in pDC, limiting their proinflammatory activity and possibly avoiding autoimmunity (36).
Key Questions and Future Directions for the Field
Future research in this field must link the phenotypic characterization of the different DC subpopulations to the known functions of DC biology. Is there really a large number of different DC or are there simply states of activation in certain organs at certain times that are functionally indistinguishable?
Altered DC Function in Disease
As key cells of tolerance and immunity, dendritic cells are directly involved in the development and progression of diseases. Here, we would like to give examples of disease-causing and course-determining DC alterations in cutaneous tumors, autoimmune diseases, and inflammation of the skin.
Skin Cancer
The DC network of the skin may be one of the first contacts that growing skin tumors have with the immune system. In what way such first contacts influence tumor growth is unclear. However, avoidance of inflammatory responses and immune cell immigration induced by them might help tumors to remain “cold,” i.e., not detected or even ignored by the immune system. If inflammation and immune cell immigration occur, tumors induce numerical and functional changes in progenitor cells and mature DC that attenuate or undermine the immune response against them. Following examples categorize DC alterations in skin cancer:
Altered DC Recruitment or Survival
Lesions of squamous cell carcinoma (SCC) have significantly reduced numbers of LCs and CD11c+ dermal DC, resulting in fewer DC available for effective T-cell priming (37, 38).
Melanomas recruit pDC via CXCR4/CXCL12 (39). IFN-induced expression of CXCR3 ligands (CXCL9, CXCL10, and CXCL11) produced by pDC in turn promotes the attraction of additional pDC (40). Through increased attraction, melanomas appear to recruit primarily immature pDC (also called plasmacytoid monocytes) (39).
Redirection of Monocyte Precursors
IL-6 and macrophage colony-stimulating factor (M-CSF) produced by melanomas promote monocyte differentiation into macrophages rather than DC, limiting effective T-cell responses (41). Melanomas concomitantly produce GM3 and GD3 gangliosides that inhibit DC differentiation from monocyte-derived progenitors and induce apoptosis in monocyte-derived DC (42, 43). Similarly, melanoma-derived cyclooxygenase-1 (COX-1) and COX2 prostanoids block DC differentiation (44).
Exploitation of Immature DC
Tumor-infiltrating immature DC and pre-DC promote angiogenesis in tumors by secreting endothelial growth factors (e.g., VEGF) and producing other factors that increase the sensitivity of endothelial cells to growth factors (45, 46). Conversely, tumors induce endothelial transdifferentiation in DC progenitor cells, which form a scaffold for the subsequent lining of tumors with endothelial cells (47).
DC Subversion
Skin tumors undermine anti-tumor immunity not only by altering the differentiation of DC precursors, but also by interfering with the activity of fully differentiated DC:
DC treated with melanoma lysate produce less IL-12p70 albeit without showing changes in maturation markers (e.g., CD40, CD80, CD83) (48). However, melanoma-infiltrating DC also appear to become resistant to some maturation stimuli (49). In turn, DC from regressing melanoma metastases exhibit a stronger T-cell stimulatory potential than DC in progressively growing metastases (50).
More recently melanomas have been found to convert infiltrating cDC2 cells into myeloid cells characterized by decreased CD1c expression and expression of monocyte/TAM markers such as CD14, CD163, CD206, and MerTK (51). Thus, CD14+ DC appear to arise not only from monocytes but can also develop from cDC2 in the tumor microenvironment (52). In a three-dimensional melanoma model with multicellular tumor spheroids, lactic acid produced by melanomas suppressed the production of proinflammatory cytokines, including IL-12, by monocyte-derived DC (53).
Melanoma-recruited pDC lose the propensity to produce type I interferons (IFN I), which play an important role in cancer immunization (54, 55). Such altered pDC then support melanoma progression by promoting regulatory immunity through OX40L and ICOSL (56).
Treg contribute to immune tolerance to tumors (57, 58) as evidenced by the fact that their ablation in mouse models improves the anti-tumor immune response (59). However, while early Treg deletion triggers complete rejection of transplanted tumors (60), deletion at later time points only limits tumor growth, suggesting that Treg establish mechanisms in the tumor microenvironment that become Treg independent. Nevertheless, depletion of Treg at late growth phases of tumors also increases the number of functional cytotoxic T cells in tumor tissue and enhances responses to vaccination against tumor antigens (60). However, some observations point to a more complex role for Treg in tumor growth. By limiting inflammatory processes that drive malignant transformation (61), Treg may protect against the development of certain tumor types (62). While sarcomas appear to escape, immune control via editing rather than immune repression, lung adenocarcinomas escape because the anti-tumor response itself was suppressed (63). Tumor-infiltrating T cells also often do not appear suppressed, but rather chronically activated and functionally altered (64, 65). Taking into account that Treg primarily form stable contacts with DC in tissues, the effect of Treg in tumors appears to be based less on regulation of T cells and more on DC. How tumor-induced changes in DC affect their interaction with Treg seems for the most part unexplored. It is also conceivable that tumor cells alone do not alter DC in tumors, but interaction with Treg does.
Autoimmune Skin Diseases
The development of autoimmune diseases bases on alterations in central or peripheral immune tolerance. As early as the 1980s [ten years after their discovery as a separate antigen presenting cell population (66)], DC were observed to act as drivers of T cells in autoimmune lesions (67) and to transfer autoimmunity to naive recipients (68).
Interestingly, observations made in the 1970s (69) that led to the definition of Foxp3+ Treg in the 1990s (70) are also closely related to autoimmune diseases. Foxp3+ Treg prevent autoimmunity by limiting activation and differentiation of autoreactive T cells through interaction with DC (71), in particular, by regulating the expression of costimulatory molecules (72) and suppressing canonical autophagy (73).
Despite methodological difficulty in reliably identifying human Treg (74, 75), a number of papers have demonstrated that most autoimmune diseases are accompanied by defects in the number or function of peripheral blood Treg, and these observations could be confirmed by in vivo disease models (76). Most notably, experimental models demonstrated that attenuation of Treg activity is causal and not a consequence of autoimmune disease (77).
Preventive Role in Autoimmune Pathogenesis?
Novel models of constitutive and inducible DC ablation and DC-specific gene targeting have further elucidated the role of DC in autoimmune diseases. Enforced antigen expression in steady state DC induces profound and irreversible peripheral unresponsiveness. Both, induction of Treg and anergization or deletion of autoreactive T cells have been shown to be involved in peripheral tolerance induction by DC (21, 78–81). However, constitutive cDC, pDC or CD8+ cDC ablation (82–85) results in only a small reduction in Treg numbers but does not cause autoimmunity and DC ablation in an autoimmune-prone background ameliorates rather than exacerbates disease (86). However, these observations do not mean that DC are redundant for peripheral tolerance, because in the absence of DC, effector cells do not form.
Presumably, their functional state rather than their phenotype determines the tolerogenic properties of DC. Although distinct tolerogenic DC can be generated under artificial conditions (87, 88), they are not identifiable in the steady state. It has been suggested that expression of the autoimmune regulator (AIRE), which induces the presentation of a wide range of self-antigens, contributes to central and peripheral tolerogenic DC activity (89, 90), however, in human DC its association with tolerogenic DC activity has been called into question (91).
Independent of a phenotypic distinction of tolerogenic DC populations, there are observations showing that DC counteract autoimmunity by activating regulatory cells. In pemphigus, for example, LC counteract the development of autoimmunity by activating keratinocyte antigen-specific Treg (92).
Contributing Role in Autoimmune Pathogenesis
While early adoptive transfer experiments had already shown that DC can trigger autoimmune responses (67, 68), a number of anti-inflammatory genes (including SHP1, STAT3, αvβ8-integrin) have since been identified whose absence in DC triggers spontaneous autoimmune responses (93–95). In addition the formation of type I interferons by pDC appears to be involved in all autoimmune processes. Recent observations show that the gut microbiome induces IFN-I production in pDC, which drives a specific epigenomic and metabolic state in cDC that instructs cDC for pathogen control. Interestingly, however, IFN-I-mediated cDC instruction lowers the threshold for self-reactivity (96). In this context, it is noteworthy that of the 81 human autoimmune diseases identified by Hayter and Cook (97), most involve the barrier tissues gut and skin. As in the gut, a continuous confrontation with microbiota takes place in the skin (98). Whether this leads, as in the gut, to an instruction of skin DC is, to our knowledge, not investigated. On the other hand, there are increasing indications that gut commensal-mediated immune instruction becomes systemic and influences immune responses in the skin including autoimmune responses (99).
Disease Repercussions on DC and Their Significance for Disease Progression
In addition to changes in DC that drive the development of autoimmunity, there are retroactive changes in disease that determine disease progression.
DC Accumulation and Altered Cytokine Formation
Accumulation of pDC is characteristic of autoimmune skin lesions. In several autoimmune diseases, increased recruitment of DC to target tissues leads to a decrease in the number of DC in the blood. In rheumatoid arthritis (RA) there is also -presumably in response to the decrease in numbers in the blood- an increased generation of pre-plasmacytoid dendritic cells in bone marrow (100). In systemic lupus erythematosus (SLE) circulating pDCs become functionally abnormal (101).
Defective Migration
Local immune activation, in addition to increased attraction of pDC, also ensures that DC already in the tissue increasingly migrate to draining lymph nodes, or, conversely, are retained at the site of inflammation. For example, in autoimmune dermatitis, the migration of LC is impaired (102). The defect in LC migration develops before the onset of skin lesions and correlates with the onset and severity of dermatitis. However, there appear to be both non-migrating LC and migrating cells that look like LC and share some of their characteristics (103).
Inflammatory Skin Diseases
Inflammatory skin diseases are due to barrier damage or activation of innate and acquired immunity. In contrast to defined autoimmune diseases, inflammatory skin diseases often have an autoimmune component for which it is not conclusively clear whether it is causal.
Psoriasis
Psoriasis is a common (2% of the U.S. population) chronic inflammatory skin disease characterized by thickening and scaling of the epidermis due to increased proliferation of keratinocytes (104). Psoriasis often develops on damaged skin (Köbner phenomenon), suggesting that innate danger signals may trigger psoriatic inflammation. It is now widely believed that psoriasis is of autoimmune origin (105, 106).
Psoriasis is associated with a greatly increased number of inflammatory DC in diseased skin that act as potent T-cell activators (107). In both psoriasis and relevant mouse models, the IL-23/IL-17/IL-22 axis plays an important role in the disease. In IL-23-induced psoriasis and in Imiquimod-induced psoriasis (a model based on the observation that patients treated with Aldara cream experience flares of psoriasis (108), which most closely match gene expression found in psoriatic skin (109)), the critical DC are TNF-α and IL-1β-producing monocyte-derived DC, including a population of inflammatory Langerhans cells. Flt3L-dependent DC and resident LC appear to be dispensable for inflammation (110).
Lichen Planus
Lichen planus is a chronic recurrent inflammatory disease of the skin and mucous membranes associated with apoptosis of basal keratinocytes (111). For some time, autoimmune T cells against desmoglein 3- and COL17 have been suspected to play a role in this disease (112). Studies on DC indicate that LC play roles in the pathology of the disease as they accumulate alongside DC in oral lymphoid foci (113). As in virtually all skin autoimmune diseases, in addition to myeloid DC, pDC were observed to secrete IFN-α and granzyme B and possibly help lymphocytes in tissue destruction.
Herpesvirus infections are associated with several autoimmune diseases, including SLE, multiple sclerosis (MS), and RA (114). Importantly, HSV infect DC and alter their function (115). Remissions of lichen planus have been associated with a decrease in protein expression of HHV-7 herpesviruses in pDC (116). Either viruses infect inflamed skin more easily or viral infections lead to the destruction of keratinocytes and thus start reactions against self-antigens.
Key Questions and Future Directions for the Field
The study of human disease in relation to the role of DC struggles with the chicken-and-egg problem. Are DC drivers of the disease or are changes in DC merely a consequence of the disease? Mouse models of skin diseases such as psoriasis recapitulate the symptoms of psoriatic patients, but are based on triggers that do not occur as such in the pathogenesis of human diseases. In addition, it is unclear which molecules define tolerogenic and immunogenic DC.
Therapeutic DC Reprogramming
In Vivo Targeting of DC for Therapeutic Approaches
The important potential use of tolerogenic DC in immunotherapeutic applications include allergic and autoimmune disorders and transplantation medicine. Thus, methods for rapid and reliable large-scale production of DC ex vivo from peripheral blood of patients have been of great academic and clinical interest and multiple clinical studies have been performed as mentioned below. However, difficulties in obtaining DC from the blood in sufficient numbers, high manufacturing costs and comprehensive requirements for GCP-based production have led to advancements for in vivo targeting of per se tolerogenic DC or for induction of tolerogenic phenotypes in vivo, respectively (117–119). In addition to avoidance of costly ex vivo isolation, these approaches have the advantage of targeting of DC subsets in their natural environment and offer the availability to a broad range of patients because of donor independency (Figure 2).
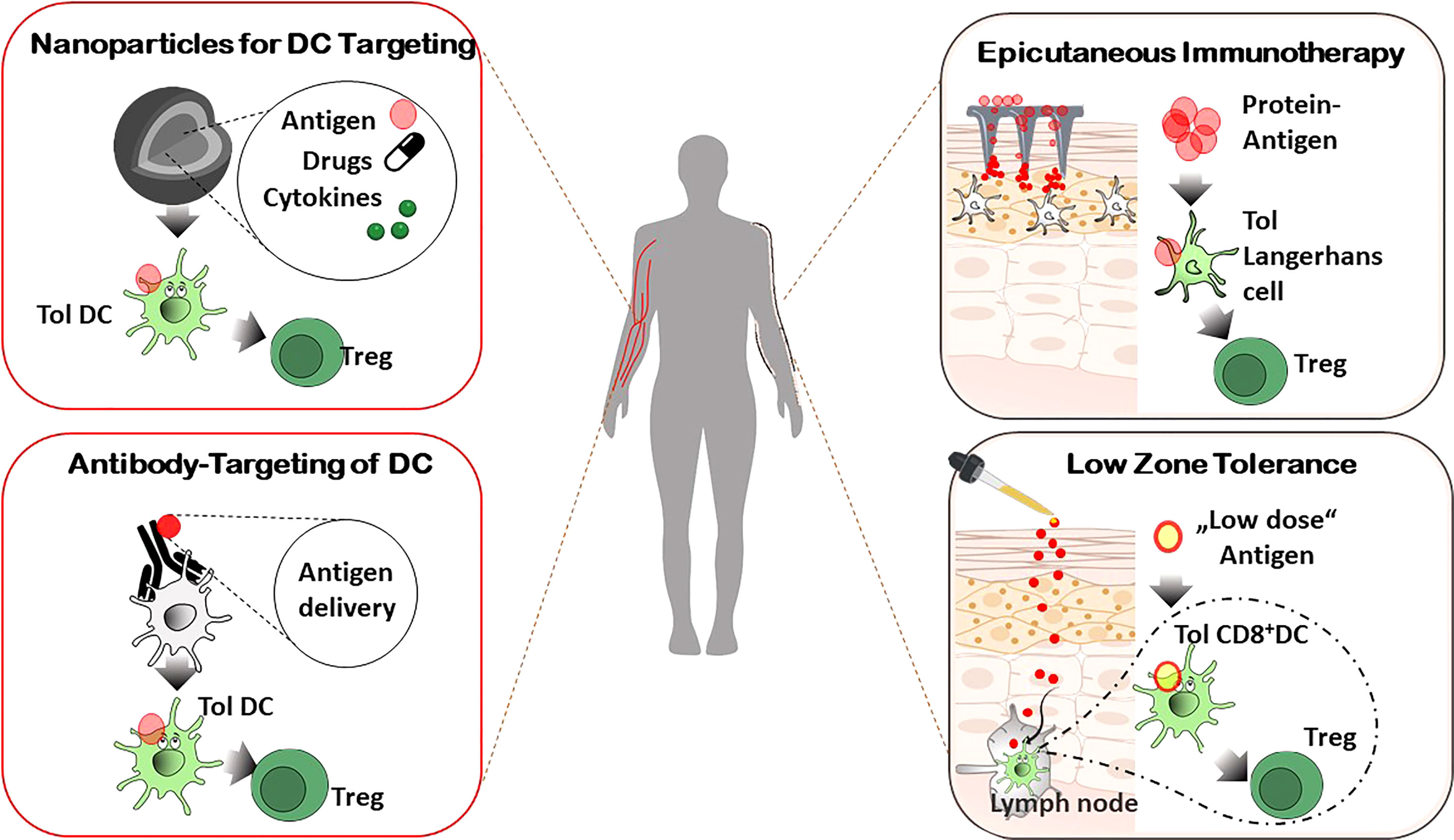
Figure 2 In vivo targeting of DC for Treg induction. Systemic administration of specifically designed nanoparticles or antibodies combined with antigen delivery for tolerogenic DC targeting in vivo, affects the resulting immune response of allergic and autoimmune diseases in an antigen-specific manner. Epicutaneous application of antigens results in activation of tolerogenic LC in the skin (by dermal patches, Epicutaneous immunotherapy) or tolerogenic DC in skin draining lymph nodes (repetitive exposure to low doses of allergens, Low zone tolerance), respectively, and subsequent control of allergic immune reactions by Treg.
Antibody-Mediated DC Targeting In Vivo
An alternative strategy for antigen-specific tolerance induction by DC in vivo is the use of antigen-delivering antibodies (119, 120). Here, a particular antigen is delivered via antibodies that bind to molecules expressed by DC. The rationale is the specific targeting of tolerogenic DC and/or the induction of intracellular tolerogenic pathways after internalization and processing of the antigen (Figure 2). So, the processed antigen will be presented in a tolerogenic context, resulting in T cell anergy, T cell deletion, and/or activation and expansion of Treg, respectively. Though many studies focus on the induction of immunity in cancer, in vivo targeting of DC was achieved to affect the immune responses in allergies, autoimmune diseases and transplantation rejections as well. Antibody-dependent targeting of DEC-205, an endocytotic receptor highly expressed on cDC1, resulted in antigen-specific unresponsiveness in the absence of adjuvants, whereas presence of maturation stimuli led to pronounced immune responses (21, 118). With regard to the pro-tolerogenic properties of DEC205+ DC if given without adjuvants, antibodies against the c-type lectin receptor DEC205 have been administered in different mouse models of autoimmune diseases (e.g. EAE, diabetes, colitis and arthritis) and graft-versus-host disease (121–125), resulting in tolerogenic mechanisms and prevention of inflammatory immune reactions. Dependent on the type of disorder and the antigen used, T cell deletion or T cell anergy of antigen-specific effector T cells and/or Treg activation have been identified as underlying mechanism for tolerance induction. Importantly, a phase I clinical trial in which human DC were targeted in vivo with anti-DEC205 linked to the tumor antigen NY-ESO-1 in the presence of maturation factors resulted in a robust cellular and humoral immune response. This proof-of-concept study confirms the preclinical data that in vivo targeting of DC leads to an antigen-specific modulation of immune reactions (126). Further studies reported alternative molecules for antibody-mediated targeting of tolerogenic DC as CD207, Treml4, Siglecs, DCIR2, or CLEC9A (DNGR1). Antigen delivery by these antibodies in the vast majority of the studies promoted the activation and/or differentiation of regulatory FOXP3+ regulatory T cells (118, 127) (Figure 2).
Use of Nanoparticles for Tolerogenic DC Targeting In Vivo
Driven by the initial success in DC-targeting nanocarriers for cancer treatment, promising strategies in this field are under development to modulate the immune system in autoimmunity, allergies, and transplantation medicine (128). DC-specific antibodies can be linked to nanoparticles (NP), allowing for a cell-specific targeting, as well as drug-delivery to induce DC with a tolerogenic phenotype, thereby preventing harmful inflammatory immune responses (Figure 2). For successful nanoparticle-based immune modulation through DC targeting in vivo, there are some aspects to be considered: 1. physicochemical properties of the NP (material, size, shape, charge, surface modification etc.), 2. disease-specific antigens, 3. DC targeting molecules, 4. co-delivery of drugs or adjuvants with functional properties for tolerance induction and 5. route of administration (119, 128). When using nanoparticles for regulation of immune reactions in autoimmune and allergic diseases, all these parameters can be optimized to boost DC targeting and to control unwanted immune responses. Multiple receptors, including DC-SIGN, mannose and Fc receptors, CD40, or CD11c, respectively, have been used for DC specific targeting in the context of nanomedicine (128). Immunosuppressive drugs and other tolerogenic agents (e.g. siRNA, antisense oligonucleotides) can be packaged into nanoparticles and be co-delivered with (auto-)antigens into DC, resulting in immune tolerance, e.g. by generation and activation of Treg (129) (Figure 2). In preclinical in vivo models it was demonstrated that poly(lactic-co-glycolic acid) (PLGA) nanoparticles carrying rapamycin were capable of inducing durable immunological tolerance to co-administered proteins via induction of tolerogenic DC and subsequent increased numbers of Treg. This immune modulation resulted in an inhibition of antigen-specific hypersensitivity reaction that was superior to the efficacy of rapamycin administered on its own (130). In another approach, nanoparticles were generated that target disease relevant peptides toward MHC class II molecules which then initiate the expansion of antigen-specific regulatory Tr1 cells and regulatory B cells leading to abrogated immunological and clinical symptoms in several murine autoimmune models such as type 1 diabetes, rheumatoid arthritis, multiple sclerosis and inflammatory bowel disease (131). The field of transplantation medicine uses nanoparticles to induce a donor-specific, long-lasting tolerance. Bryant et al. showed that PLGA-based nanoparticles loaded with donor antigens combined with low dose rapamycin at the time of transplant protected transplanted islet allografts from rejection (132).
The skin as Anatomical Site for DC – Mediated Tolerance Induction
Among specific anatomical sites, the skin represents a crucial barrier that is in constant exchange with exogenous antigens and commensal pathogens, requiring control of immune responses orchestrated by cutaneous and skin-draining DC. As mentioned above, the induction of tolerance vs. immunity depends on the DC subset, immune mediators and receptors involved etc. but as well as on the dose and application route of the antigen. With regard to the antigen dosage, the allergic contact dermatitis (ACD), one of the most frequent occupational skin disorder, is induced in patients and mice by epicutaneous exposure to high doses of contact allergens (haptens). They are taken up by cutaneous DC that prime the allergen-specific ACD effector T cells in the skin-draining LN. A second contact with the same allergen results in activation of these allergen-specifc ACD effector T cells and the clinical manifestations of the ACD (133, 134). In contrast, repetitive epicutaneous applications of very low doses of haptens (low zone tolerance) circumvent the activation of cutaneous DC and in contrast induce an allergen-specific tolerance via stimulation of regulatory FOXP3 + T cells and activation and differentiation of CD8+ tolerogenic DC in skin-draining LN. The latter ones produce TNF that foster the apoptosis of ACD effector T cells, thereby inhibiting the allergic cutaneous inflammation of ACD (135–137) (Figure 2). Thus, the dosage of the contact allergen determines the outcome of the immune response and the development of the resulting skin disease via activation of stimulating vs tolerogenic DC, followed by regulatory T cell differentiation respectively.
The idea to modulate an allergic immune response by application of allergens via the skin was also further analysed for protein antigens, and called epicutaneous immunotherapy (EPIT) (138). In animal models of food allergies and autoimmune diseases, repetitive applications of an adhesive dermal patch containing antigens protect from inflammation and clinical symptoms via uptake of antigens by cutaneous LC and subsequent regulatory T cell activation (119, 138, 139) (Figure 2). These promising studies have paved the way for several clinical trials of EPIT that have been completed or are ongoing for milk, peanuts and pollen allergies with encouraging results in terms of safety and tolerability (138).
Clinical Translation of Tolerogenic DC
For clinical applications, the most available options so far have been to induce immature DC ex vivo, and treat them with agents promoting the differentiation into tolerogenic DC phenotypes, which can be given during the entire culture period in the absence of maturation stimuli or only during the maturation phaseThe latter protocols combine the culture of CD14+ DC precursor cells in the presence of maturation factors (e.g., LPS or IL-1+IL-6+TNF+PGE2) with additional pharmacological agents, immunosuppressive mediators, exposure to apoptotic cells or gene modification (119, 140, 141). Here, an aberrant DC maturation occurs, resulting in the differentiation of a stable human tolerogenic DC phenotype. Such monocyte-derived DC recapitulate the properties and functionality of naturally occurring DC. Co-culture of monocyte-derived DC with immunosuppressive drugs (e.g. rapamycin, dexamethasone) or cytokines (e.g. TGF-beta, IL-10) or other bioactive agents (e.g. vitamin D3, hepatocyte growth factor, complement factor H, neuropeptide vasoactive intestinal peptide) leads to tolerogenic properties of the differentiated DC populations (140, 142–148). Most of these substances dampen the antigen-presenting properties of DC by reducing expression of costimulatory and MHC class molecules. On the other hand, immunosuppressive surface molecules (e.g. ILTs, PD-L1) or soluble mediators (IL-10, TGF-beta, IDO) are highly expressed by tolerogenic DC subsets (145, 149–151). These differentiated tolerogenic DC are capable to directly suppress the effector functions of other immune cells, such as T or B cells, induce T cell anergy or T cell apoptosis and/or the differentiation and activation of Treg with high suppressive activity.
Since a comparative study by Boks et al. identified IL-10 modulated tolerogenic DC as promising candidates for antigen-specific tolerance induction in vitro, we will highlight the generation and properties of these DC subset in more detail. In the first culturing step IL-10 DC differentiate in the presence of IL-4 and GM-CSF to immature DC. The two most prominent protocols differ in the duration of IL-10 supplementation: throughout the culture period (DCIL10) or only during the last two days of culture, in the presence of a maturation cocktail (25, 152–156). Gregori et al. showed that DCIL10 express CD14, CD16 and ILT2-4 but also typical DC markers such as CD83 and CD86 molecules (156). DCIL10 are inducers of regulatory IL10 secreting Tr1 cells that express CD49b and Lag3 and the induction of these Tr1 cells was largely dependent on IL-10 secretion by DCIL10.
In contrast, human IL-10 DC, generated in presence of IL-10 only during the last two days of the maturation phase, were inducers of anergic T cells as well as of Treg, which was independent of IL-10 secretion by DC. The Treg efficiently suppressed syngeneic CD4+ effector T cells and cytotoxic CD8+ T cells in a cell-to-cell contact dependent and antigen-specific manner (142, 153). The induced T cell anergy was associated with an increased expression of MAP kinase p38 and its effector molecules MEK 2 and 3. The latter ones facilitated the upregulation of the cyclin-dependent inhibitor 1B (p27Kip1), resulting in a cell-cycle arrest in the G1 phase and, thereby in an anergic state of the T cells (154, 155).
As mentioned above, a comparative study by Boks et al. identified IL10-modulated DC (IL-10 DC) as the most potent candidates for tolerance induction as they exhibit important prerequisites for clinical applications in humans such as potent migratory capacity, efficient Treg induction, and the stability of tolerogenic phenotype under inflammatory conditions (146). Investigation of our own laboratory confirmed these theses as we identified two subsets of the tolerogenic IL-10 DC, CD83highCCR7+ and CD83lowCCR7- IL-10 DC. Both tolerogenic DC subpopulations exhibited the capacity to induce Treg, but Treg generated in the presence of the CD83high IL-10 DC subset displayed a superior suppressive capacity. In addition, the CD83high DC subset was extremely stable in a pro-inflammatory environment and, due to the increased CCR7, expression showed a very high migratory capacity which is required for DC/T cell-contact in lymphatic tissues (25), identifying this CD83highCCR7+ IL-10 DC subset as promising candidate for clinical applications.
Clinical Studies With Ex Vivo Generated Human Tolerogenic DC
The increasing knowledge of tolerogenic DC biology, the promising results from in vitro studies with human DC and numerous preclinical animal studies have paved the way for several completed and ongoing clinical phase I trials in autoimmune diseases and transplantation medicine (141) (Figure 3). Published studies in patients suffering from diabetes (157), arthritis (158, 159), multiple sclerosis (160, 161) and Crohn´s disease (162) used different protocols for induction of tolerogenic DC phenotypes, including dexamethasone alone or plus vitamin D3 or vitamin A, respectively. Vitamin D3 alone, an NF-kappaB inhibitor, or antisense ODN against CD40, CD80 and CD86 were also used. In the vast majority of the trials the tolerogenic DC were loaded with disease specific antigens/peptides for induction of a specific tolerance reaction. The study results demonstrated a range from no adverse effects until grade 1/2, indicating a high safety profile for tolerogenic DC applications in vivo. Analysis of the clinical outcome showed a therapeutic response in a part of the patients, often measured in reduction of disease-specific scores. Immuno-monitoring in some trials revealed a decrease in cytokine production, reduced effector T cell responses, and intriguingly, an increase of the Treg/effector T cell ratio or of the frequency of the Tr1 regulatory T cell subset (158) (Figure 3).
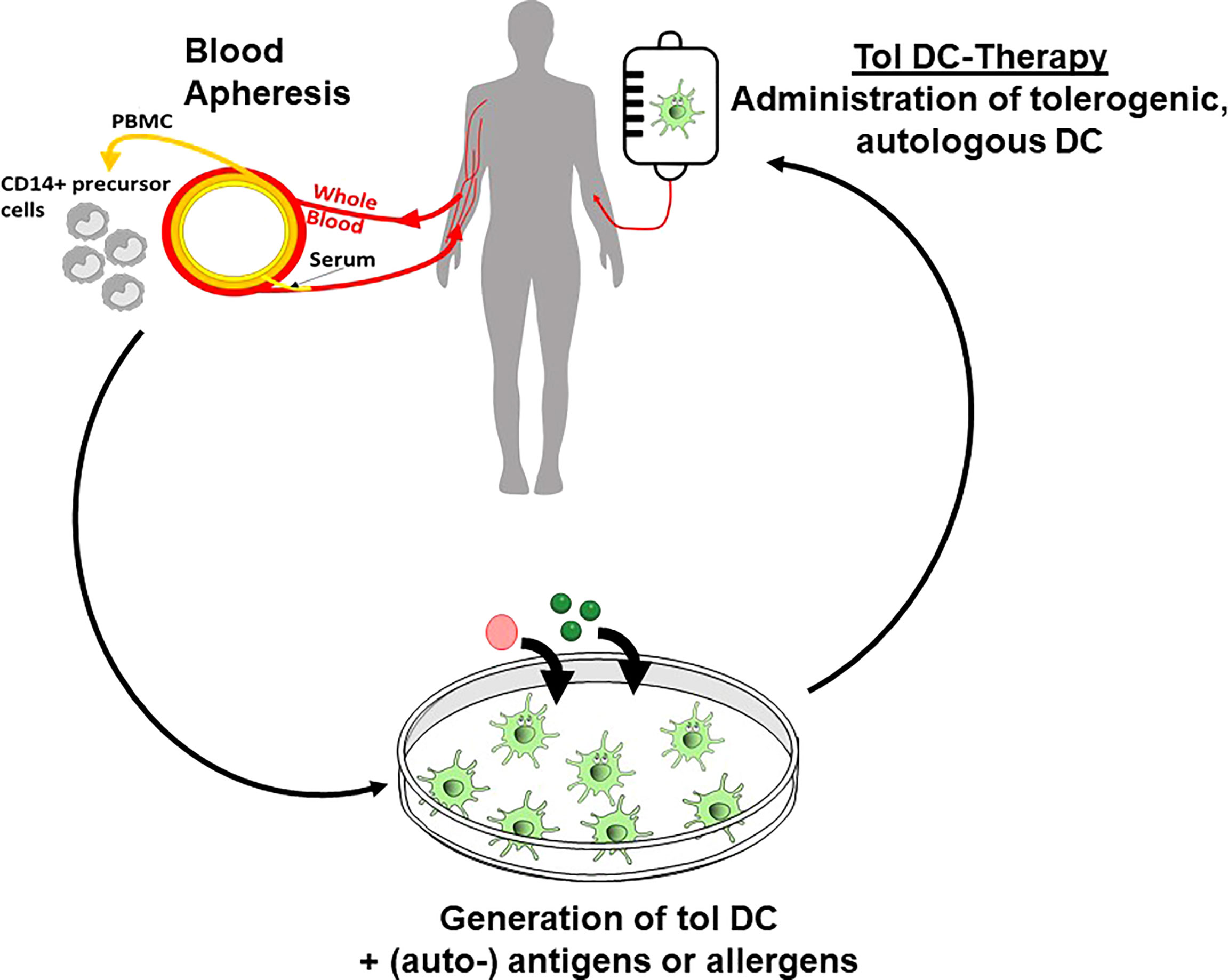
Figure 3 Tolerogenic DC in clinical applications. Ex vivo generation of tolerogenic DC starts with isolation of CD14+ monocytic precursor cells from the peripheral blood of the patients. After subsequent in vitro culture in the presence of tolerogenic agents and loading with (auto-) antigens, the tolerogenic DC are re-injected into the patients to affect the inflammatory immune reaction in allergic and autoimmune disorders or in transplantation rejection.
In transplantation medicine, data from six phase I/II trails assessing the use of various regulatory cell products, including tolerogenic DC, in kidney transplantation have been analysed. The results demonstrate that regulatory cell therapy is associated with fewer infectious complications, but similar rejection rates, and is therefore a potentially useful therapeutic approach to minimise the burden of general immunosuppression (163). A meta- analysis of 48 records of clinical trials confirmed these data, showing the safety of tolerance-inducing cell-products (including DC) in patients with autoimmune disorders or receiving organ transplantation (160).
Key Questions and Future Directions
In general, the clinical trials in this field have confirmed the safety and feasibility of autologous ex vivo generated tolerogenic DC for therapeutic approaches in autoimmunity and transplantation medicine. However, tolerogenic DC use in real clinical practice has many problems to solve beforehand. Harmonization of study protocols and systematic inclusion of immunological outcome measures would highly improve the development of tolerogenic DC-based treatment approaches. These needs have initiated the implication of consortia in the USA (“The immune tolerance network”) and Europe (“Action to focus and accelerate cell-based tolerance inducing therapies”).
As the generation of autologous ex vivo tolerogenic DC exhibit several pitfalls as mentioned, alternative strategies of in vivo DC targeting by antibody- and nanoparticle-based techniques or antigen delivery by way of the skin (EPIT, LZT) can be considered for tolerance induction. However, these systems have been primarily tested in preclinical models and further translational trials have to be performed to show the efficacy, feasibility and safety of these novel strategies for tolerance induction via DC targeting in vivo.
Author Contributions
CB, VR, and KS contributed to conception and design of the manuscript. NS, CB, VR, and KS wrote the first draft of the manuscript. HP wrote sections of the manuscript. VR designed figures and JT created table 1. All authors contributed to manuscript revision, read, and approved the submitted version.
Funding
This work was supported by the German Research Foundation (Deutsche Forschungsgemeinschaft, DFG) with TR156/A4/C05-246807620, SFB1009/B11-194468054, SFB1066/B06-213555243 and SFB1450/C06-431460824 (all to KS), TR156/C5-246807620 (to VR), TR156/B02-246807620 (to HP), SFB1066/B08-213555243 (to CB).
Conflict of Interest
The authors declare that the research was conducted in the absence of any commercial or financial relationships that could be construed as a potential conflict of interest.
Publisher’s Note
All claims expressed in this article are solely those of the authors and do not necessarily represent those of their affiliated organizations, or those of the publisher, the editors and the reviewers. Any product that may be evaluated in this article, or claim that may be made by its manufacturer, is not guaranteed or endorsed by the publisher.
References
1. Hettinger J, Richards DM, Hansson J, Barra MM, Joschko A-C, Krijgsveld J, et al. Origin of Monocytes and Macrophages in a Committed Progenitor. Nat Immunol (2013) 14:821–30. doi: 10.1038/ni.2638
2. Mcgovern N, Chan JKY, Ginhoux F. Dendritic Cells in Humans-From Fetus to Adult. Int Immunol (2015) 27:65–72. doi: 10.1093/intimm/dxu091
3. Villani AC, Satija R, Reynolds G, Sarkizova S, Shekhar K, Fletcher J, et al. Single-Cell RNA-Seq Reveals New Types of Human Blood Dendritic Cells, Monocytes, and Progenitors. Science (2017) 356:eaah4573. doi: 10.1126/science.aah4573
4. Mehta H, Angsana J, Bissonnette R, Munoz-Elias EJ, Sarfati M. Inflammatory Skin Disorders: Monocyte-Derived Cells Take Center Stage. Front Immunol (2021) 12. doi: 10.3389/fimmu.2021.691806
5. Collin M, Bigley V. Human Dendritic Cell Subsets: An Update. Immunology (2018) 154:3–20. doi: 10.1111/imm.12888
6. Doebel T, Voisin B, Nagao K. Langerhans Cells - The Macrophage in Dendritic Cell Clothing. Trends Immunol (2017) 38:817–28. doi: 10.1016/j.it.2017.06.008
7. Wu XD, Briseno CG, Grajales-Reyes GE, Haldar M, Iwata A, Kretzer NM, et al. Transcription Factor Zeb2 Regulates Commitment to Plasmacytoid Dendritic Cell and Monocyte Fate. Proc Natl Acad Sci U S A (2016) 113:14775–80. doi: 10.1073/pnas.1611408114
8. Scott CL, Soen B, Martens L, Skrypek N, Saelens W, Taminau J, et al. The Transcription Factor Zeb2 Regulates Development of Conventional and Plasmacytoid DCs by Repressing Id2. J Exp Med (2016) 213:897–911. doi: 10.1084/jem.20151715
9. Hoeffel G, Wang YL, Greter M, See P, Teo P, Malleret B, et al. Adult Langerhans Cells Derive Predominantly From Embryonic Fetal Liver Monocytes With a Minor Contribution of Yolk Sac-Derived Macrophages. J Exp Med (2012) 209:1167–81. doi: 10.1084/jem.20120340
10. Mildner A, Jung S. Development and Function of Dendritic Cell Subsets. Immunity (2014) 40:642–56. doi: 10.1016/j.immuni.2014.04.016
11. Ginhoux F, Tacke F, Angeli V, Bogunovic M, Loubeau M, Dai XM, et al. Langerhans Cells Arise From Monocytes In Vivo. Nat Immunol (2006) 7:265–73. doi: 10.1038/ni1307
12. Segura E. Review of Mouse and Human Dendritic Cell Subsets. Methods Mol Biol (2016) 1423:3–15. doi: 10.1007/978-1-4939-3606-9_1
13. Balan S, Saxena M, Bhardwaj N. Dendritic Cell Subsets and Locations. Int Rev Cell Mol Biol (2019) 348:1–68. doi: 10.1016/bs.ircmb.2019.07.004
14. Patente TA, Pinho MP, Oliveira AA, Evangelista GCM, Bergami-Santos PC, Barbuto J. Human Dendritic Cells: Their Heterogeneity and Clinical Application Potential in Cancer Immunotherapy. Front Immunol (2019) 9. doi: 10.3389/fimmu.2018.03176
15. Larsson AJM, Johnsson P, Hagemann-Jensen M, Hartmanis L, Faridani OR, Reinius B, et al. Genomic Encoding of Transcriptional Burst Kinetics. Nature (2019) 565:251–4. doi: 10.1038/s41586-018-0836-1
16. Schwanhausser B, Busse D, Li N, Dittmar G, Schuchhardt J, Wolf J, et al. Global Quantification of Mammalian Gene Expression Control. Nature (2011) 473:337–42. doi: 10.1038/nature10098
17. Yuan S, Chen Y, Zhang M, Wang Z, Hu Z, Ruan Y, et al. Overexpression of miR-223 Promotes Tolerogenic Properties of Dendritic Cells Involved in Heart Transplantation Tolerance by Targeting Irak1. Front Immunol (2021) 12:676337. doi: 10.3389/fimmu.2021.676337
18. Bermejo-Jambrina M, Eder J, Helgers LC, Hertoghs N, Nijmeijer BM, Stunnenberg M, et al. C-Type Lectin Receptors in Antiviral Immunity and Viral Escape. Front Immunol (2018) 9:590. doi: 10.3389/fimmu.2018.00590
19. Lutz MB, Schuler G. Immature, Semi-Mature and Fully Mature Dendritic Cells: Which Signals Induce Tolerance or Immunity? Trends Immunol (2002) 23:445–9. doi: 10.1016/S1471-4906(02)02281-0
20. Dissanayake D, Hall H, Berg-Brown N, Elford AR, Hamilton SR, Murakami K, et al. Nuclear Factor-Kappab1 Controls the Functional Maturation of Dendritic Cells and Prevents the Activation of Autoreactive T Cells. Nat Med (2011) 17:1663–7. doi: 10.1038/nm.2556
21. Hawiger D, Inaba K, Dorsett Y, Guo M, Mahnke K, Rivera M, et al. Dendritic Cells Induce Peripheral T Cell Unresponsiveness Under Steady State Conditions In Vivo. J Exp Med (2001) 194:769–79. doi: 10.1084/jem.194.6.769
22. Ardouin L, Luche H, Chelbi R, Carpentier S, Shawket A, Montanana Sanchis F, et al. Broad and Largely Concordant Molecular Changes Characterize Tolerogenic and Immunogenic Dendritic Cell Maturation in Thymus and Periphery. Immunity (2016) 45:305–18. doi: 10.1016/j.immuni.2016.07.019
23. Vander Lugt B, Riddell J, Khan AA, Hackney JA, Lesch J, Devoss J, et al. Transcriptional Determinants of Tolerogenic and Immunogenic States During Dendritic Cell Maturation. J Cell Biol (2017) 216:779–92. doi: 10.1083/jcb.201512012
24. Torres-Aguilar H, Aguilar-Ruiz SR, Gonzalez-Perez G, Munguia R, Bajana S, Meraz-Rios MA, et al. Tolerogenic Dendritic Cells Generated With Different Immunosuppressive Cytokines Induce Antigen-Specific Anergy and Regulatory Properties in Memory CD4+ T Cells. J Immunol (2010) 184:1765–75. doi: 10.4049/jimmunol.0902133
25. Kryczanowsky F, Raker V, Graulich E, Domogalla MP, Steinbrink K. IL-10-Modulated Human Dendritic Cells for Clinical Use: Identification of a Stable and Migratory Subset With Improved Tolerogenic Activity. J Immunol (2016) 197:3607–17. doi: 10.4049/jimmunol.1501769
26. Dillon S, Agrawal S, Banerjee K, Letterio J, Denning TL, Oswald-Richter K, et al. Yeast Zymosan, a Stimulus for TLR2 and Dectin-1, Induces Regulatory Antigen-Presenting Cells and Immunological Tolerance. J Clin Invest (2006) 116:916–28. doi: 10.1172/JCI27203
27. Chamorro S, Garcia-Vallejo JJ, Unger WW, Fernandes RJ, Bruijns SC, Laban S, et al. TLR Triggering on Tolerogenic Dendritic Cells Results in TLR2 Up-Regulation and a Reduced Proinflammatory Immune Program. J Immunol (2009) 183:2984–94. doi: 10.4049/jimmunol.0801155
28. Naik S, Bouladoux N, Linehan JL, Han SJ, Harrison OJ, Wilhelm C, et al. Commensal-Dendritic-Cell Interaction Specifies a Unique Protective Skin Immune Signature. Nature (2015) 520:104–8. doi: 10.1038/nature14052
29. Serbina NV, Salazar-Mather TP, Biron CA, Kuziel WA, Pamer EG. TNF/iNOS-Producing Dendritic Cells Mediate Innate Immune Defense Against Bacterial Infection. Immunity (2003) 19:59–70. doi: 10.1016/S1074-7613(03)00171-7
30. Steinman RM, Nussenzweig MC. Avoiding Horror Autotoxicus: The Importance of Dendritic Cells in Peripheral T Cell Tolerance. Proc Natl Acad Sci U S A (2002) 99:351–8. doi: 10.1073/pnas.231606698
31. Clausen BE, Kel JM. Langerhans Cells: Critical Regulators of Skin Immunity? Immunol Cell Biol (2010) 88:351–60. doi: 10.1038/icb.2010.40
32. King JK, Philips RL, Eriksson AU, Kim PJ, Halder RC, Lee DJ, et al. Langerhans Cells Maintain Local Tissue Tolerance in a Model of Systemic Autoimmune Disease. J Immunol (2015) 195:464–76. doi: 10.4049/jimmunol.1402735
33. Rissoan MC, Duhen T, Bridon JM, Bendriss-Vermare N, Peronne C, De Saint Vis B, et al. Subtractive Hybridization Reveals the Expression of Immunoglobulin-Like Transcript 7, Eph-B1, Granzyme B, and 3 Novel Transcripts in Human Plasmacytoid Dendritic Cells. Blood (2002) 100:3295–303. doi: 10.1182/blood-2002-02-0638
34. Salvi V, Vermi W, Cavani A, Lonardi S, Carbone T, Facchetti F, et al. IL-21 May Promote Granzyme B-Dependent NK/Plasmacytoid Dendritic Cell Functional Interaction in Cutaneous Lupus Erythematosus. J Invest Dermatol (2017) 137:1493–500. doi: 10.1016/j.jid.2017.03.016
35. Jahrsdorfer B, Vollmer A, Blackwell SE, Maier J, Sontheimer K, Beyer T, et al. Granzyme B Produced by Human Plasmacytoid Dendritic Cells Suppresses T-Cell Expansion. Blood (2010) 115:1156–65. doi: 10.1182/blood-2009-07-235382
36. Mossu A, Daoui A, Bonnefoy F, Aubergeon L, Saas P, Perruche S. Plasmacytoid Dendritic Cells Die by the CD8 T Cell-Dependent Perforin Pathway During Acute Nonviral Inflammation. J Immunol (2016) 197:1672–82. doi: 10.4049/jimmunol.1501875
37. Galan A, Ko CJ. Langerhans Cells in Squamous Cell Carcinoma vs. Pseudoepitheliomatous Hyperplasia of the Skin. J Cutan Pathol (2007) 34:950–2. doi: 10.1111/j.1600-0560.2007.00741.x
38. Bluth MJ, Zaba LC, Moussai D, Suarez-Farinas M, Kaporis H, Fan L, et al. Myeloid Dendritic Cells From Human Cutaneous Squamous Cell Carcinoma are Poor Stimulators of T-Cell Proliferation. J Invest Dermatol (2009) 129:2451–62. doi: 10.1038/jid.2009.96
39. Vermi W, Bonecchi R, Facchetti F, Bianchi D, Sozzani S, Festa S, et al. Recruitment of Immature Plasmacytoid Dendritic Cells (Plasmacytoid Monocytes) and Myeloid Dendritic Cells in Primary Cutaneous Melanomas. J Pathol (2003) 200:255–68. doi: 10.1002/path.1344
40. Vanbervliet B, Bendris-Vermare N, Massacrier C, Homey B, De Bouteiller O, Briere F, et al. The Inducible CXCR3 Ligands Control Plasmacytoid Dendritic Cell Responsiveness to the Constitutive Chemokine Stromal Cell-Derived Factor 1 (SDF-1)/Cxcl12. J Exp Med (2003) 198:823–30. doi: 10.1084/jem.20020437
41. Banchereau J, Palucka AK. Dendritic Cells as Therapeutic Vaccines Against Cancer. Nat Rev Immunol (2005) 5:296–306. doi: 10.1038/nri1592
42. Peguet-Navarro J, Sportouch M, Popa I, Berthier O, Schmitt D, Portoukalian J. Gangliosides From Human Melanoma Tumors Impair Dendritic Cell Differentiation From Monocytes and Induce Their Apoptosis. J Immunol (2003) 170:3488–94. doi: 10.4049/jimmunol.170.7.3488
43. Bennaceur K, Popa I, Chapman JA, Migdal C, Peguet-Navarro J, Touraine JL, et al. Different Mechanisms are Involved in Apoptosis Induced by Melanoma Gangliosides on Human Monocyte-Derived Dendritic Cells. Glycobiology (2009) 19:576–82. doi: 10.1093/glycob/cwp015
44. Sombroek CC, Stam AG, Masterson AJ, Lougheed SM, Schakel MJ, Meijer CJ, et al. Prostanoids Play a Major Role in the Primary Tumor-Induced Inhibition of Dendritic Cell Differentiation. J Immunol (2002) 168:4333–43. doi: 10.4049/jimmunol.168.9.4333
45. Coukos G, Benencia F, Buckanovich RJ, Conejo-Garcia JR. The Role of Dendritic Cell Precursors in Tumour Vasculogenesis. Br J Cancer (2005) 92:1182–7. doi: 10.1038/sj.bjc.6602476
46. Sprague L, Muccioli M, Pate M, Meles E, Mcginty J, Nandigam H, et al. The Interplay Between Surfaces and Soluble Factors Define the Immunologic and Angiogenic Properties of Myeloid Dendritic Cells. BMC Immunol (2011) 12:12–35. doi: 10.1186/1471-2172-12-35
47. Gottfried E, Kreutz M, Haffner S, Holler E, Iacobelli M, Andreesen R, et al. Differentiation of Human Tumour-Associated Dendritic Cells Into Endothelial-Like Cells: An Alternative Pathway of Tumour Angiogenesis. Scandinavian J Immunol (2007) 65:329–35. doi: 10.1111/j.1365-3083.2007.01903.x
48. Jackson AM, Mulcahy LA, Zhu XW, O'donnell D, Patel PM. Tumour-Mediated Disruption of Dendritic Cell Function: Inhibiting the MEK1/2-P44/42 Axis Restores IL-12 Production and Th1-Generation. Int J Cancer (2008) 123:623–32. doi: 10.1002/ijc.23530
49. Vicari AP, Chiodoni C, Vaure C, Ait-Yahia S, Dercamp C, Matsos F, et al. Reversal of Tumor-Induced Dendritic Cell Paralysis by CpG Immunostimulatory Oligonucleotide and Anti-Interleukin 10 Receptor Antibody. J Exp Med (2002) 196:541–9. doi: 10.1084/jem.20020732
50. Enk AH, Jonuleit H, Saloga J, Knop J. Dendritic Cells as Mediators of Tumor-Induced Tolerance in Metastatic Melanoma. Int J Cancer (1997) 73:309–16. doi: 10.1002/(SICI)1097-0215(19971104)73:3<309::AID-IJC1>3.0.CO;2-3
51. Michea P, Noel F, Zakine E, Czerwinska U, Sirven P, Abouzid O, et al. Adjustment of Dendritic Cells to the Breast-Cancer Microenvironment is Subset Specific. Nat Immunol (2018) 19:885–97. doi: 10.1038/s41590-018-0145-8
52. Di Blasio S, Van Wigcheren GF, Becker A, Van Duffelen A, Gorris M, Verrijp K, et al. The Tumour Microenvironment Shapes Dendritic Cell Plasticity in a Human Organotypic Melanoma Culture. Nat Commun (2020) 11:2749. doi: 10.1038/s41467-020-16583-0
53. Gottfried E, Kunz-Schughart LA, Ebner S, Mueller-Klieser W, Hoves S, Andreesen R, et al. Tumor-Derived Lactic Acid Modulates Dendritic Cell Activation and Antigen Expression. Blood (2006) 107:2013–21. doi: 10.1182/blood-2005-05-1795
54. Dunn GP, Bruce AT, Sheehan KC, Shankaran V, Uppaluri R, Bui JD, et al. A Critical Function for Type I Interferons in Cancer Immunoediting. Nat Immunol (2005) 6:722–9. doi: 10.1038/ni1213
55. Dunn GP, Koebel CM, Schreiber RD. Interferons, Immunity and Cancer Immunoediting. Nat Rev Immunol (2006) 6:836–48. doi: 10.1038/nri1961
56. Aspord C, Leccia MT, Charles J, Plumas J. Plasmacytoid Dendritic Cells Support Melanoma Progression by Promoting Th2 and Regulatory Immunity Through OX40L and ICOSL. Cancer Immunol Res (2013) 1:402–15. doi: 10.1158/2326-6066.CIR-13-0114-T
57. Kim JM, Rasmussen JP, Rudensky AY. Regulatory T Cells Prevent Catastrophic Autoimmunity Throughout the Lifespan of Mice. Nat Immunol (2007) 8:191–7. doi: 10.1038/ni1428
58. Lahl K, Sparwasser T. In Vivo Depletion of FoxP3+ Tregs Using the DEREG Mouse Model. Methods Mol Biol (Clifton N.J.) (2011) 707:157–72. doi: 10.1007/978-1-61737-979-6_10
59. Sutmuller RP, Van Duivenvoorde LM, Van Elsas A, Schumacher TN, Wildenberg ME, Allison JP, et al. Synergism of Cytotoxic T Lymphocyte-Associated Antigen 4 Blockade and Depletion of CD25(+) Regulatory T Cells in Antitumor Therapy Reveals Alternative Pathways for Suppression of Autoreactive Cytotoxic T Lymphocyte Responses. J Exp Med (2001) 194:823–32. doi: 10.1084/jem.194.6.823
60. Klages K, Mayer CT, Lahl K, Loddenkemper C, Teng MWL, Ngiow SF, et al. Selective Depletion of Foxp3+ Regulatory T Cells Improves Effective Therapeutic Vaccination Against Established Melanoma. Cancer Res (2010) 70:7788–99. doi: 10.1158/0008-5472.CAN-10-1736
61. Rao VP, Poutahidis T, Ge Z, Nambiar PR, Boussahmain C, Wang YY, et al. Innate Immune Inflammatory Response Against Enteric Bacteria Helicobacter Hepaticus Induces Mammary Adenocarcinoma in Mice. Cancer Res (2006) 66:7395–400. doi: 10.1158/0008-5472.CAN-06-0558
62. Gounaris E, Blatner NR, Dennis K, Magnusson F, Gurish MF, Strom TB, et al. T-Regulatory Cells Shift From a Protective Anti-Inflammatory to a Cancer-Promoting Proinflammatory Phenotype in Polyposis. Cancer Res (2009) 69:5490–7. doi: 10.1158/0008-5472.CAN-09-0304
63. Joshi NS, Akama-Garren EH, Lu Y, Lee DY, Chang GP, Li A, et al. Regulatory T Cells in Tumor-Associated Tertiary Lymphoid Structures Suppress Anti-Tumor T Cell Responses. Immunity (2015) 43:579–90. doi: 10.1016/j.immuni.2015.08.006
64. Predina J, Eruslanov E, Judy B, Kapoor V, Cheng GJ, Wang LC, et al. Changes in the Local Tumor Microenvironment in Recurrent Cancers may Explain the Failure of Vaccines After Surgery. Proc Natl Acad Sci U S A (2013) 110:1582–3. doi: 10.1073/pnas.1211850110
65. Li H, van der Leun AM, Yofe I, Lubling Y, Gelbard-Solodkin D, Van Akkooi ACJ, et al. Dysfunctional CD8 T Cells Form a Proliferative, Dynamically Regulated Compartment Within Human Melanoma. Cell (2019) 176:775–789.e718.
66. Steinman RM, Cohn ZA. Identification of a Novel Cell Type in Peripheral Lymphoid Organs of Mice. I. Morphology, Quantitation, Tissue Distribution. J Exp Med (1973) 137:1142–62. doi: 10.1084/jem.137.5.1142
67. Olsson T, Holmdahl R, Klareskog L, Forsum U. Ia-Expressing Cells and T Lymphocytes of Different Subsets in Peripheral Nerve Tissue During Experimental Allergic Neuritis in Lewis Rats. Scand J Immunol (1983) 18:339–43. doi: 10.1111/j.1365-3083.1983.tb01805.x
68. Knight SC, Mertin J, Stackpoole A, Clark J. Induction of Immune Responses In Vivo With Small Numbers of Veiled (Dendritic) Cells. Proc Natl Acad Sci U S A (1983) 80:6032–5. doi: 10.1073/pnas.80.19.6032
69. Nishizuka Y, Sakakura T. Thymus and Reproduction: Sex-Linked Dysgenesia of the Gonad After Neonatal Thymectomy in Mice. Science (1969) 166:753–5. doi: 10.1126/science.166.3906.753
70. Sakaguchi S, Sakaguchi N, Asano M, Itoh M, Toda M. Immunologic Self-Tolerance Maintained by Activated T Cells Expressing IL-2 Receptor Alpha-Chains (CD25). Breakdown of a Single Mechanism of Self-Tolerance Causes Various Autoimmune Diseases. J Immunol (Baltimore Md.: 1950) (1995) 155:1151–64.
71. Liu Z, Gerner MY, Van Panhuys N, Levine AG, Rudensky AY, Germain RN. Immune Homeostasis Enforced by Co-Localized Effector and Regulatory T Cells. Nature (2015) 528:225–30. doi: 10.1038/nature16169
72. Bolton HA, Zhu E, Terry AM, Guy TV, Koh WP, Tan SY, et al. Selective Treg Reconstitution During Lymphopenia Normalizes DC Costimulation and Prevents Graft-Versus-Host Disease. J Clin Invest (2015) 125:3627–41. doi: 10.1172/JCI76031
73. Alissafi T, Banos A, Boon L, Sparwasser T, Ghigo A, Wing K, et al. Tregs Restrain Dendritic Cell Autophagy to Ameliorate Autoimmunity. J Clin Invest (2017) 127:2789–804. doi: 10.1172/JCI92079
74. Allan SE, Crome SQ, Crellin NK, Passerini L, Steiner TS, Bacchetta R, et al. Activation-Induced FOXP3 in Human T Effector Cells Does Not Suppress Proliferation or Cytokine Production. Int Immunol (2007) 19:345–54. doi: 10.1093/intimm/dxm014
75. Santegoets SJ, Dijkgraaf EM, Battaglia A, Beckhove P, Britten CM, Gallimore A, et al. Monitoring Regulatory T Cells in Clinical Samples: Consensus on an Essential Marker Set and Gating Strategy for Regulatory T Cell Analysis by Flow Cytometry. Cancer Immunol Immunother (2015) 64:1271–86. doi: 10.1007/s00262-015-1729-x
76. Miyara M, Gorochov G, Ehrenstein M, Musset L, Sakaguchi S, Amoura Z. Human FoxP3+ Regulatory T Cells in Systemic Autoimmune Diseases. Autoimmun Rev (2011) 10:744–55. doi: 10.1016/j.autrev.2011.05.004
77. Sakaguchi S, Yamaguchi T, Nomura T, Ono M. Regulatory T Cells and Immune Tolerance. Cell (2008) 133:775–87. doi: 10.1016/j.cell.2008.05.009
78. Probst HC, Lagnel J, Kollias G, Van Den Broek M. Inducible Transgenic Mice Reveal Resting Dendritic Cells as Potent Inducers of CD8(+) T Cell Tolerance. Immunity (2003) 18:713–20. doi: 10.1016/S1074-7613(03)00120-1
79. Hawiger D, Masilamani RF, Bettelli E, Kuchroo VK, Nussenzweig MC. Immunological Unresponsiveness Characterized by Increased Expression of CD5 on Peripheral T Cells Induced by Dendritic Cells In Vivo. Immunity (2004) 20:695–705. doi: 10.1016/j.immuni.2004.05.002
80. Probst HC, Mccoy K, Okazaki T, Honjo T, Van Den Broek MF. Resting Dendritic Cells Induce Peripheral CD8+T Cell Tolerance Through PD-1 and CTLA-4. Immunology (2005) 116:43–3. doi: 10.1038/ni1165
81. Muth S, Schutze K, Schild H, Probst HC. Release of Dendritic Cells From Cognate CD4(+) T-Cell Recognition Results in Impaired Peripheral Tolerance and Fatal Cytotoxic T-Cell Mediated Autoimmunity. Proc Natl Acad Sci U S A (2012) 109:9059–64. doi: 10.1073/pnas.1110620109
82. Birnberg T, Bar-On L, Sapoznikov A, Caton ML, Cervantes-Barragan L, Makia D, et al. Lack of Conventional Dendritic Cells Is Compatible With Normal Development and T Cell Homeostasis, But Causes Myeloid Proliferative Syndrome. Immunity (2008) 29:986–97. doi: 10.1016/j.immuni.2008.10.012
83. Edelson BT, Kc W, Juang R, Kohyama M, Benoit LA, Klekotka PA, et al. Peripheral CD103+ Dendritic Cells Form a Unified Subset Developmentally Related to CD8alpha+ Conventional Dendritic Cells. J Exp Med (2010) 207:823–36. doi: 10.1084/jem.20091627
84. Bar-On L, Birnberg T, Kim KW, Jung S. Dendritic Cell-Restricted CD80/86 Deficiency Results in Peripheral Regulatory T-Cell Reduction But is Not Associated With Lymphocyte Hyperactivation. Eur J Immunol (2011) 41:291–8. doi: 10.1002/eji.201041169
85. Cervantes-Barragan L, Lewis KL, Firner S, Thiel V, Hugues S, Reith W, et al. Plasmacytoid Dendritic Cells Control T-Cell Response to Chronic Viral Infection. Proc Natl Acad Sci U S A (2012) 109:3012–7. doi: 10.1073/pnas.1117359109
86. Teichmann LL, Ols ML, Kashgarian M, Reizis B, Kaplan DH, Shlomchik MJ. Dendritic Cells in Lupus are Not Required for Activation of T and B Cells But Promote Their Expansion, Resulting in Tissue Damage. Immunity (2010) 33:967–78. doi: 10.1016/j.immuni.2010.11.025
87. Kriegel MA, Rathinam C, Flavell RA. Pancreatic Islet Expression of Chemokine CCL2 Suppresses Autoimmune Diabetes via Tolerogenic CD11c+ CD11b+ Dendritic Cells. Proc Natl Acad Sci U S A (2012) 109:3457–62. doi: 10.1073/pnas.1115308109
88. Li D, Zhao B, Luo Y, Limbara S, Zhao B, Zou X, et al. Transplantation of Aire-Overexpressing Bone Marrow-Derived Dendritic Cells Delays the Onset of Type 1 Diabetes. Int Immunopharmacol (2017) 49:13–20. doi: 10.1016/j.intimp.2017.05.023
89. Lei Y, Ripen AM, Ishimaru N, Ohigashi I, Nagasawa T, Jeker LT, et al. Aire-Dependent Production of XCL1 Mediates Medullary Accumulation of Thymic Dendritic Cells and Contributes to Regulatory T Cell Development. J Exp Med (2011) 208:383–94. doi: 10.1084/jem.20102327
90. Lindmark E, Chen Y, Georgoudaki AM, Dudziak D, Lindh E, Adams WC, et al. AIRE Expressing Marginal Zone Dendritic Cells Balances Adaptive Immunity and T-Follicular Helper Cell Recruitment. J Autoimmun (2013) 42:62–70. doi: 10.1016/j.jaut.2012.11.004
91. Crossland KL, Abinun M, Arkwright PD, Cheetham TD, Pearce SH, Hilkens CMU, et al. AIRE is Not Essential for the Induction of Human Tolerogenic Dendritic Cells. Autoimmunity (2016) 49:211–8. doi: 10.3109/08916934.2016.1148692
92. Kitashima DY, Kobayashi T, Woodring T, Idouchi K, Doebel T, Voisin B, et al. Langerhans Cells Prevent Autoimmunity via Expansion of Keratinocyte Antigen-Specific Regulatory T Cells. EBioMedicine (2018) 27:293–303. doi: 10.1016/j.ebiom.2017.12.022
93. Travis MA, Reizis B, Melton AC, Masteller E, Tang Q, Proctor JM, et al. Loss of Integrin Alpha(V)Beta8 on Dendritic Cells Causes Autoimmunity and Colitis in Mice. Nature (2007) 449:361–5. doi: 10.1038/nature06110
94. Kim SJ, Zou YR, Goldstein J, Reizis B, Diamond B. Tolerogenic Function of Blimp-1 in Dendritic Cells. J Exp Med (2011) 208:2193–9. doi: 10.1084/jem.20110658
95. Kaneko T, Saito Y, Kotani T, Okazawa H, Iwamura H, Sato-Hashimoto M, et al. Dendritic Cell-Specific Ablation of the Protein Tyrosine Phosphatase Shp1 Promotes Th1 Cell Differentiation and Induces Autoimmunity. J Immunol (2012) 188:5397–407. doi: 10.4049/jimmunol.1103210
96. Schaupp L, Muth S, Rogell L, Kofoed-Branzk M, Melchior F, Lienenklaus S, et al. Microbiota-Induced Type I Interferons Instruct a Poised Basal State of Dendritic Cells. Cell (2020) 181:1080–1096.e1019.
97. Hayter SM, Cook MC. Updated Assessment of the Prevalence, Spectrum and Case Definition of Autoimmune Disease. Autoimmunity Reviews (2012) 11:754–65. doi: 10.1016/j.autrev.2012.02.001
98. Shen W, Li W, Hixon JA, Bouladoux N, Belkaid Y, Dzutzev A, et al. Adaptive Immunity to Murine Skin Commensals. Proc Natl Acad Sci U S A (2014) 111:E2977–2986. doi: 10.1073/pnas.1401820111
99. De Pessemier B, Grine L, Debaere M, Maes A, Paetzold B, Callewaert C, et al. Gut-Skin Axis: Current Knowledge of the Interrelationship between Microbial Dysbiosis and Skin Conditions. Microorganisms (2021) 9(2):353. doi: 10.3390/microorganisms9020353
100. Hirohata S, Yanagida T, Tomita T, Yoshikawa H. Increased Generation of Pre-Plasmacytoid Dendritic Cells in Bone Marrow of Rheumatoid Arthritis. Mod Rheumatol (2014) 24:443–7. doi: 10.3109/14397595.2013.843759
101. Jin O, Kavikondala S, Mok MY, Sun L, Gu J, Fu R, et al. Abnormalities in Circulating Plasmacytoid Dendritic Cells in Patients With Systemic Lupus Erythematosus. Arthritis Res Ther (2010) 12:R137. doi: 10.1186/ar3075
102. Eriksson AU, Singh RR. Cutting Edge: Migration of Langerhans Dendritic Cells is Impaired in Autoimmune Dermatitis. J Immunol (2008) 181:7468–72. doi: 10.4049/jimmunol.181.11.7468
103. Sheng JP, Chen Q, Wu XT, Dong YW, Mayer J, Zhang JL, et al. Fate Mapping Analysis Reveals a Novel Murine Dermal Migratory Langerhans-Like Cell Population. Elife (2021) 10:e65412. doi: 10.7554/eLife.65412
104. Lowes MA, Suarez-Farinas M, Krueger JG. Immunology of Psoriasis. Annu Rev Immunol (2014) 32:227–55. doi: 10.1146/annurev-immunol-032713-120225
105. Arakawa A, Siewert K, Stohr J, Besgen P, Kim SM, Ruhl G, et al. Melanocyte Antigen Triggers Autoimmunity in Human Psoriasis. J Exp Med (2015) 212:2203–12. doi: 10.1084/jem.20151093
106. Lande R, Botti E, Jandus C, Dojcinovic D, Fanelli G, Conrad C, et al. The Antimicrobial Peptide LL37 Is a T-Cell Autoantigen in Psoriasis. Nat Commun (2015) 6:5621. doi: 10.1038/ncomms7595
107. Zaba LC, Fuentes-Duculan J, Eungdamrong NJ, Abello MV, Novitskaya I, Pierson KC, et al. Psoriasis Is Characterized by Accumulation of Immunostimulatory and Th1/Th17 Cell-Polarizing Myeloid Dendritic Cells. J Invest Dermatol (2009) 129:79–88. doi: 10.1038/jid.2008.194
108. Flutter B, Nestle FO. TLRs to Cytokines: Mechanistic Insights From the Imiquimod Mouse Model of Psoriasis. Eur J Immunol (2013) 43:3138–46. doi: 10.1002/eji.201343801
109. Suarez-Farinas M, Arbeit R, Jiang WW, Ortenzio FS, Sullivan T, Krueger JG. Suppression of Molecular Inflammatory Pathways by Toll-Like Receptor 7, 8, and 9 Antagonists in a Model of IL-23-Induced Skin Inflammation. PLos One (2013) 8(2):e84634. doi: 10.1371/journal.pone.0084634
110. Singh TP, Zhang HH, Borek I, Wolf P, Hedrick MN, Singh SP, et al. Monocyte-Derived Inflammatory Langerhans Cells and Dermal Dendritic Cells Mediate Psoriasis-Like Inflammation. Nat Commun (2016) 7:563–604. doi: 10.1038/ncomms13581
111. Alrashdan MS, Cirillo N, Mccullough M. Oral Lichen Planus: A Literature Review and Update. Arch Dermatol Res (2016) 308:539–51. doi: 10.1007/s00403-016-1667-2
112. Schmidt T, Solimani F, Pollmann R, Stein R, Schmidt A, Stulberg I, et al. T(H)1/T(H)17 Cell Recognition of Desmoglein 3 and Bullous Pemphigoid Antigen 180 in Patients With Lichen Planus. J Allergy Clin Immunol (2018) 142:669–72. doi: 10.1016/j.jaci.2018.02.044
113. Santoro A, Majorana A, Roversi L, Gentili F, Marrelli S, Vermi W, et al. Recruitment of Dendritic Cells in Oral Lichen Planus. J Pathol (2005) 205:426–34. doi: 10.1002/path.1699
115. Farias MA, Duarte LF, Tognarelli EI, Gonzalez PA. Herpes Simplex Virus Interference With Immunity: Focus on Dendritic Cells. Virulence (2021) 12:2583–607. doi: 10.1080/21505594.2021.1980990
116. De Vries HJC, Teunissen MBM, Zorgdrager F, Picavet D, Cornelissen M. Lichen Planus Remission is Associated With a Decrease of Human Herpes Virus Type 7 Protein Expression in Plasmacytoid Dendritic Cells. Arch Dermatol Res (2007) 299:213–9. doi: 10.1007/s00403-007-0750-0
117. Hasegawa H, Matsumoto T. Mechanisms of Tolerance Induction by Dendritic Cells In Vivo. Front Immunol (2018) 9:350. doi: 10.3389/fimmu.2018.00350
118. Castenmiller C, Keumatio-Doungtsop BC, Van Ree R, De Jong EC, Van Kooyk Y. Tolerogenic Immunotherapy: Targeting DC Surface Receptors to Induce Antigen-Specific Tolerance. Front Immunol (2021) 12:643240. doi: 10.3389/fimmu.2021.643240
119. Passeri L, Marta F, Bassi V, Gregori S. Tolerogenic Dendritic Cell-Based Approaches in Autoimmunity. Int J Mol Sci (2021) 22(16):8415. doi: 10.3390/ijms22168415
120. Iberg CA, Hawiger D. Natural and Induced Tolerogenic Dendritic Cells. J Immunol (2020) 204:733–44. doi: 10.4049/jimmunol.1901121
121. Stern JNH, Keskin DB, Kato Z, Waldner H, Schallenberg S, Anderson A, et al. Promoting Tolerance to Proteolipid Protein-Induced Experimental Autoimmune Encephalomyelitis Through Targeting Dendritic Cells. Proc Natl Acad Sci U S A (2010) 107:17280–5. doi: 10.1073/pnas.1010263107
122. Ettinger M, Gratz IK, Gruber C, Hauser-Kronberger C, Johnson TS, Mahnke K, et al. Targeting of the Hnc16a Collagen Domain to Dendritic Cells Induces Tolerance to Human Type XVII Collagen. Exp Dermatol (2012) 21:395–8. doi: 10.1111/j.1600-0625.2012.01474.x
123. Mukherjee G, Geliebter A, Babad J, Santamaria P, Serreze DV, Freeman GJ, et al. DEC-205-Mediated Antigen Targeting to Steady-State Dendritic Cells Induces Deletion of Diabetogenic CD8 T Cells Independently of PD-1 and PD-L1. Int Immunol (2013) 25:651–60. doi: 10.1093/intimm/dxt031
124. Spiering R, Margry B, Keijzer C, Petzold C, Hoek A, Wagenaar-Hilbers J, et al. DEC205+ Dendritic Cell-Targeted Tolerogenic Vaccination Promotes Immune Tolerance in Experimental Autoimmune Arthritis. J Immunol (2015) 194:4804–13. doi: 10.4049/jimmunol.1400986
125. Iberg CA, Hawiger D. Targeting Dendritic Cells With Antigen-Delivering Antibodies for Amelioration of Autoimmunity in Animal Models of Multiple Sclerosis and Other Autoimmune Diseases. Antibodies (Basel) (2020) 9(2):23. doi: 10.3390/antib9020023
126. Dhodapkar MV, Sznol M, Zhao B, Wang D, Carvajal RD, Keohan ML, et al. Induction of Antigen-Specific Immunity With a Vaccine Targeting NY-ESO-1 to the Dendritic Cell Receptor DEC-205. Sci Transl Med (2014) 6:232ra251. doi: 10.1126/scitranslmed.3008068
127. Joffre OP, Sancho D, Zelenay S, Keller AM, Reis E Sousa C. Efficient and Versatile Manipulation of the Peripheral CD4+ T-Cell Compartment by Antigen Targeting to DNGR-1/CLEC9A. Eur J Immunol (2010) 40:1255–65. doi: 10.1002/eji.201040419
128. Cifuentes-Rius A, Desai A, Yuen D, Johnston APR, Voelcker NH. Inducing Immune Tolerance With Dendritic Cell-Targeting Nanomedicines. Nat Nanotechnol (2021) 16:37–46. doi: 10.1038/s41565-020-00810-2
129. Stead SO, Kireta S, Mcinnes SJP, Kette FD, Sivanathan KN, Kim J, et al. Murine and Non-Human Primate Dendritic Cell Targeting Nanoparticles for in Vivo Generation of Regulatory T-Cells. ACS Nano (2018) 12:6637–47. doi: 10.1021/acsnano.8b01625
130. Kishimoto TK, Ferrari JD, Lamothe RA, Kolte PN, Griset AP, O'neil C, et al. Improving the Efficacy and Safety of Biologic Drugs With Tolerogenic Nanoparticles. Nat Nanotechnol (2016) 11:890–9. doi: 10.1038/nnano.2016.135
131. Clemente-Casares X, Blanco J, Ambalavanan P, Yamanouchi J, Singha S, Fandos C, et al. Expanding Antigen-Specific Regulatory Networks to Treat Autoimmunity. Nature (2016) 530:434–40. doi: 10.1038/nature16962
132. Bryant J, Hlavaty KA, Zhang X, Yap WT, Zhang L, Shea LD, et al. Nanoparticle Delivery of Donor Antigens for Transplant Tolerance in Allogeneic Islet Transplantation. Biomaterials (2014) 35:8887–94. doi: 10.1016/j.biomaterials.2014.06.044
133. Kostner L, Anzengruber F, Guillod C, Recher M, Schmid-Grendelmeier P, Navarini AA. Allergic Contact Dermatitis. Immunol Allergy Clin North Am (2017) 37:141–52. doi: 10.1016/j.iac.2016.08.014
134. Azeem M, Kader H, Kerstan A, Hetta HF, Serfling E, Goebeler M, et al. Intricate Relationship Between Adaptive and Innate Immune System in Allergic Contact Dermatitis. Yale J Biol Med (2020) 93:699–709.
135. Luckey U, Maurer M, Schmidt T, Lorenz N, Seebach B, Metz M, et al. T Cell Killing by Tolerogenic Dendritic Cells Protects Mice From Allergy. J Clin Invest (2011) 121:3860–71. doi: 10.1172/JCI45963
136. Luckey U, Schmidt T, Pfender N, Romer M, Lorenz N, Martin SF, et al. Crosstalk of Regulatory T Cells and Tolerogenic Dendritic Cells Prevents Contact Allergy in Subjects With Low Zone Tolerance. J Allergy Clin Immunol (2012) 130:781. doi: 10.1016/j.jaci.2012.06.022
137. Schmidt T, Lorenz N, Raker VK, Schmidgen MI, Mahnke K, Enk A, et al. Allergen-Specific Low Zone Tolerance Is Independent of MRP8/14-, TLR4-, TLR7-, and TLR9-Mediated Immune Processes. J Invest Dermatol (2018) 138:452–5. doi: 10.1016/j.jid.2017.09.020
138. Wang J, Sampson HA. Safety and Efficacy of Epicutaneous Immunotherapy for Food Allergy. Pediatr Allergy Immunol (2018) 29:341–9. doi: 10.1111/pai.12869
139. Dioszeghy V, Mondoulet L, Laoubi L, Dhelft V, Plaquet C, Bouzereau A, et al. Antigen Uptake by Langerhans Cells Is Required for the Induction of Regulatory T Cells and the Acquisition of Tolerance During Epicutaneous Immunotherapy in OVA-Sensitized Mice. Front Immunol (2018) 9. doi: 10.3389/fimmu.2018.01951
140. Domogalla MP, Rostan PV, Raker VK, Steinbrink K. Tolerance Through Education: How Tolerogenic Dendritic Cells Shape Immunity. Front Immunol (2017) 8. doi: 10.3389/fimmu.2017.01764
141. Morante-Palacios O, Fondelli F, Ballestar E, Martinez-Caceres EM. Tolerogenic Dendritic Cells in Autoimmunity and Inflammatory Diseases. Trends Immunol (2021) 42:59–75. doi: 10.1016/j.it.2020.11.001
142. Steinbrink K, Wolfl M, Jonuleit H, Knop J, Enk AH. Induction of Tolerance by IL-10-Treated Dendritic Cells. J Immunol (1997) 159:4772–80.
143. Piemonti L, Monti P, Allavena P, Sironi M, Soldini L, Leone BE, et al. Glucocorticoids Affect Human Dendritic Cell Differentiation and Maturation. J Immunol (1999) 162:6473–81.
144. Gonzalez-Rey E, Chorny A, Fernandez-Martin A, Ganea D, Delgado M. Vasoactive Intestinal Peptide Generates Human Tolerogenic Dendritic Cells That Induce CD4 and CD8 Regulatory T Cells. Blood (2006) 107:3632–8. doi: 10.1182/blood-2005-11-4497
145. Unger WW, Laban S, Kleijwegt FS, van der Slik AR, Roep BO. Induction of Treg by Monocyte-Derived DC Modulated by Vitamin D3 or Dexamethasone: Differential Role for PD-L1. Eur J Immunol (2009) 39:3147–59. doi: 10.1002/eji.200839103
146. Boks MA, Kager-Groenland JR, Haasjes MSP, Zwaginga JJ, Van Ham SM, Ten Brinke A. IL-10-Generated Tolerogenic Dendritic Cells are Optimal for Functional Regulatory T Cell Induction - A Comparative Study of Human Clinical-Applicable DC. Clin Immunol (2012) 142:332–42. doi: 10.1016/j.clim.2011.11.011
147. Olivar R, Luque A, Cardenas-Brito S, Naranjo-Gomez M, Blom AM, Borras FE, et al. The Complement Inhibitor Factor H Generates an Anti-Inflammatory and Tolerogenic State in Monocyte-Derived Dendritic Cells. J Immunol (2016) 196:4274–90. doi: 10.4049/jimmunol.1500455
148. Svajger U, Rozman PJ. Recent Discoveries in Dendritic Cell Tolerance-Inducing Pharmacological Molecules. Int Immunopharmacol (2020) 81:106275. doi: 10.1016/j.intimp.2020.106275
149. Grohmann U, Volpi C, Fallarino F, Bozza S, Bianchi R, Vacca C, et al. Reverse Signaling Through GITR Ligand Enables Dexamethasone to Activate IDO in Allergy. Nat Med (2007) 13:579–86. doi: 10.1038/nm1563
150. Stallone G, Pontrelli P, Infante B, Gigante M, Netti GS, Ranieri E, et al. Rapamycin Induces ILT3(high)ILT4(high) Dendritic Cells Promoting a New Immunoregulatory Pathway. Kidney Int (2014) 85:888–97. doi: 10.1038/ki.2013.337
151. Adnan E, Matsumoto T, Ishizaki J, Onishi S, Suemori K, Yasukawa M, et al. Human Tolerogenic Dendritic Cells Generated With Protein Kinase C Inhibitor are Optimal for Functional Regulatory T Cell Induction - A Comparative Study. Clin Immunol (2016) 173:96–108. doi: 10.1016/j.clim.2016.09.007
152. Steinbrink K, Jonuleit H, Muller G, Knop J, Enk AH. IL-10-Treated Human Dendritic Cells Induce a Melanoma-Antigen-Specific Anergy in CD8(+) T Cells Resulting in a Failure to Lyse Tumor Cells. J Invest Dermatol (1998) 110:479–9. doi: 10.1016/S0923-1811(98)83039-9
153. Steinbrink K, Jonuleit H, Müller G, Schuler G, Knop J, Enk AH. Interleukin-10-Treated Human Dendritic Cells Induce a Melanoma-Antigen-Specific Anergy in CD8(+) T Cells Resulting in a Failure to Lyse Tumor Cells. Blood (1999) 93:1634–42. doi: 10.1182/blood.V93.5.1634.405k11_1634_1642
154. Kubsch S, Graulich E, Knop J, Steinbrink K. Suppressor Activity of Anergic T Cells Induced by IL-10-Treated Human Dendritic Cells: Association With IL-2- and CTLA-4-Dependent G1 Arrest of the Cell Cycle Regulated by p27Kip1. Eur J Immunol (2003) 33:1988–97. doi: 10.1002/eji.200323600
155. Adler HS, Kubsch S, Graulich E, Ludwig S, Knop J, Steinbrink K. Activation of MAP Kinase P38 is Critical for the Cell-Cycle-Controlled Suppressor Function of Regulatory T Cells. Blood (2007) 109:4351–9. doi: 10.1182/blood-2006-09-047563
156. Gregori S, Tomasoni D, Pacciani V, Scirpoli M, Battaglia M, Magnani CF, et al. Differentiation of Type 1 T Regulatory Cells (Tr1) by Tolerogenic DC-10 Requires the IL-10-Dependent ILT4/HLA-G Pathway. Blood (2010) 116:935–44. doi: 10.1182/blood-2009-07-234872
157. Giannoukakis N, Phillips B, Finegold D, Harnaha J, Trucco M. Phase I (Safety) Study of Autologous Tolerogenic Dendritic Cells in Type 1 Diabetic Patients. Diabetes Care (2011) 34:2026–32. doi: 10.2337/dc11-0472
158. Benham H, Nel HJ, Law SC, Mehdi AM, Street S, Ramnoruth N, et al. Citrullinated Peptide Dendritic Cell Immunotherapy in HLA Risk Genotype-Positive Rheumatoid Arthritis Patients. Sci Transl Med (2015) 7:290ra287. doi: 10.1126/scitranslmed.aaa9301
159. Bell GM, Anderson AE, Diboll J, Reece R, Eltherington O, Harry RA, et al. Autologous Tolerogenic Dendritic Cells for Rheumatoid and Inflammatory Arthritis. Ann Rheum Dis (2017) 76:227–34. doi: 10.1136/annrheumdis-2015-208456
160. Willekens B, Presas-Rodriguez S, Mansilla MJ, Derdelinckx J, Lee WP, Nijs G, et al. Tolerogenic Dendritic Cell-Based Treatment for Multiple Sclerosis (MS): A Harmonised Study Protocol for Two Phase I Clinical Trials Comparing Intradermal and Intranodal Cell Administration. BMJ Open (2019) 9:e030309. doi: 10.1136/bmjopen-2019-030309
161. Zubizarreta I, Florez-Grau G, Vila G, Cabezon R, Espana C, Andorra M, et al. Immune Tolerance in Multiple Sclerosis and Neuromyelitis Optica With Peptide-Loaded Tolerogenic Dendritic Cells in a Phase 1b Trial. Proc Natl Acad Sci U S A (2019) 116:8463–70. doi: 10.1073/pnas.1820039116
162. Jauregui-Amezaga A, Cabezon R, Ramirez-Morros A, Espana C, Rimola J, Bru C, et al. Intraperitoneal Administration of Autologous Tolerogenic Dendritic Cells for Refractory Crohn's Disease: A Phase I Study. J Crohns Colitis (2015) 9:1071–8. doi: 10.1093/ecco-jcc/jjv144
Keywords: treg cells, tolerance, dendritic cells, cellular immunotherapy, DC targeted vaccines, interleukin 10
Citation: Scheib N, Tiemann J, Becker C, Probst HC, Raker VK and Steinbrink K (2022) The Dendritic Cell Dilemma in the Skin: Between Tolerance and Immunity. Front. Immunol. 13:929000. doi: 10.3389/fimmu.2022.929000
Received: 26 April 2022; Accepted: 30 May 2022;
Published: 28 June 2022.
Edited by:
Helmut Jonuleit, Johannes Gutenberg University Mainz, GermanyReviewed by:
Manfred B. Lutz, Julius Maximilian University of Würzburg, GermanyMarkus Philipp Radsak, Johannes Gutenberg University Mainz, Germany
Copyright © 2022 Scheib, Tiemann, Becker, Probst, Raker and Steinbrink. This is an open-access article distributed under the terms of the Creative Commons Attribution License (CC BY). The use, distribution or reproduction in other forums is permitted, provided the original author(s) and the copyright owner(s) are credited and that the original publication in this journal is cited, in accordance with accepted academic practice. No use, distribution or reproduction is permitted which does not comply with these terms.
*Correspondence: Verena Katharina Raker, VmVyZW5hLlJha2VyQHVrbXVlbnN0ZXIuZGU=