- Department of Biological Medicines & Shanghai Engineering Research Center of Immunotherapeutics, School of Pharmacy, Fudan University, Shanghai, China
As nano-sized materials prepared by isolating, disrupting and extruding cell membranes, cellular vesicles are emerging as a novel vehicle for immunotherapeutic drugs to activate antitumor immunity. Cell membrane-derived vesicles inherit the surface characteristics and functional properties of parental cells, thus having superior biocompatibility, low immunogenicity and long circulation. Moreover, the potent antitumor effect of cellular vesicles can be achieved through surface modification, genetic engineering, hybridization, drug encapsulation, and exogenous stimulation. The capacity of cellular vesicles to combine drugs of different compositions and functions in physical space provides a promising vehicle for combinational immunotherapy of cancer. In this review, the latest advances in cellular vesicles as vehicles for combinational cancer immunotherapy are systematically summarized with focuses on manufacturing processes, cell sources, therapeutic strategies and applications, providing an insight into the potential and existing challenges of using cellular vesicles for cancer immunotherapy.
Introduction
Immunotherapy brings great hope to cancer patients, but it also faces challenges such as low response rate, difficulty in eradication, and susceptibility to relapse. Combination therapy offers promising solutions to address these issues (1). The appropriate delivery system physically combines multiple drugs, providing an integrated solution to achieve tumor targeting, killing, and activation of immune systems simultaneously. The main problem with conventional drug delivery systems such as liposomes, polymer micelles, dendrimers and nanogels is their vulnerability to clearance by the reticuloendothelial system and other circulating immune cells, resulting in severe liver toxicity and inadequate enrichment in target sites (2). With this in mind, researchers focus on the study of biomimetic drug vehicles (3, 4). Cellular vesicle is an important area of interest within the field of biomimetic drug delivery vehicles. It mainly refers to plasma membrane structures extracted from parental cells under external intervention and prepared into nano-sized vesicles for drug delivery (5).
In this review, we seek to track the recent advances in the application of cell membrane vesicles as drug vehicles for cancer immunotherapy. We introduce the manufacturing workflow of cellular vesicles and summarize their characteristics from various parental origins. Then, the currently reported strategies of utilizing cellular vesicles to combat tumors are comprehensively reviewed. And finally, the comparion with other nanovehicles and challenges of cellular vesicles in cancer immunotherapy are discussed in depth with the aim of accelerating the clinical applications of this novel platform for cancer immunotherapy.
Manufacturing of Cellular Vesicles for Cancer Immunotherapy
Isolation
The typical process of isolating cellular vesicles consists of several steps (Figure 1A). Firstly, the parental cells are harvested and resuspended in a hypotonic buffer, rendering the cytoplasm swollen and susceptible to fragmentation by external forces (6, 7). Then, if the cytoplasmic components are to be removed, cells usually need to undergo approximately five freeze-thawing cycles and dounce homogenization to release intracellular proteins (6, 7). Next, cell membranes are separated from other cellular components by continuous high-speed or density gradient centrifugation. For example, the cells were subjected to centrifugation at 1000 g, 10000 g, and 100000 g to remove the nuclei, organelles, and other impurities, respectively (6). For density gradient centrifugation, cell membranes were prepared by centrifugation through discontinuous 30-40-55% sucrose (w/v) density gradient. At the interface of the different sucrose solutions, three lipid rings could be clearly detected, where the fraction between 30% and 40% sucrose retained the most plasma membrane proteins and was then collected and prepared for vesicles (7–9). Finally, they are sonicated for several minutes and repeatedly extruded through about three layers of polycarbonate membranes with stepwise decreasing pore size to obtain cellular vesicles (6, 10). Besides, to obtain vesicles that retain cytoplastic proteins and RNAs, cellular vesicles can be purified by OptiPrep density gradient centrifugation. Briefly, the cells underwent serial extrusion of the polycarbonate membranes, followed by centrifugation at 100000 g through 10% and 50% OptiPrep medium, and vesicles were harvested at the junction of the two layers (11–14). Aside from the above typical procedures, cellular vesicles can be induced by cytochalasin B or obtained by nitrogen cavitation (15–17).
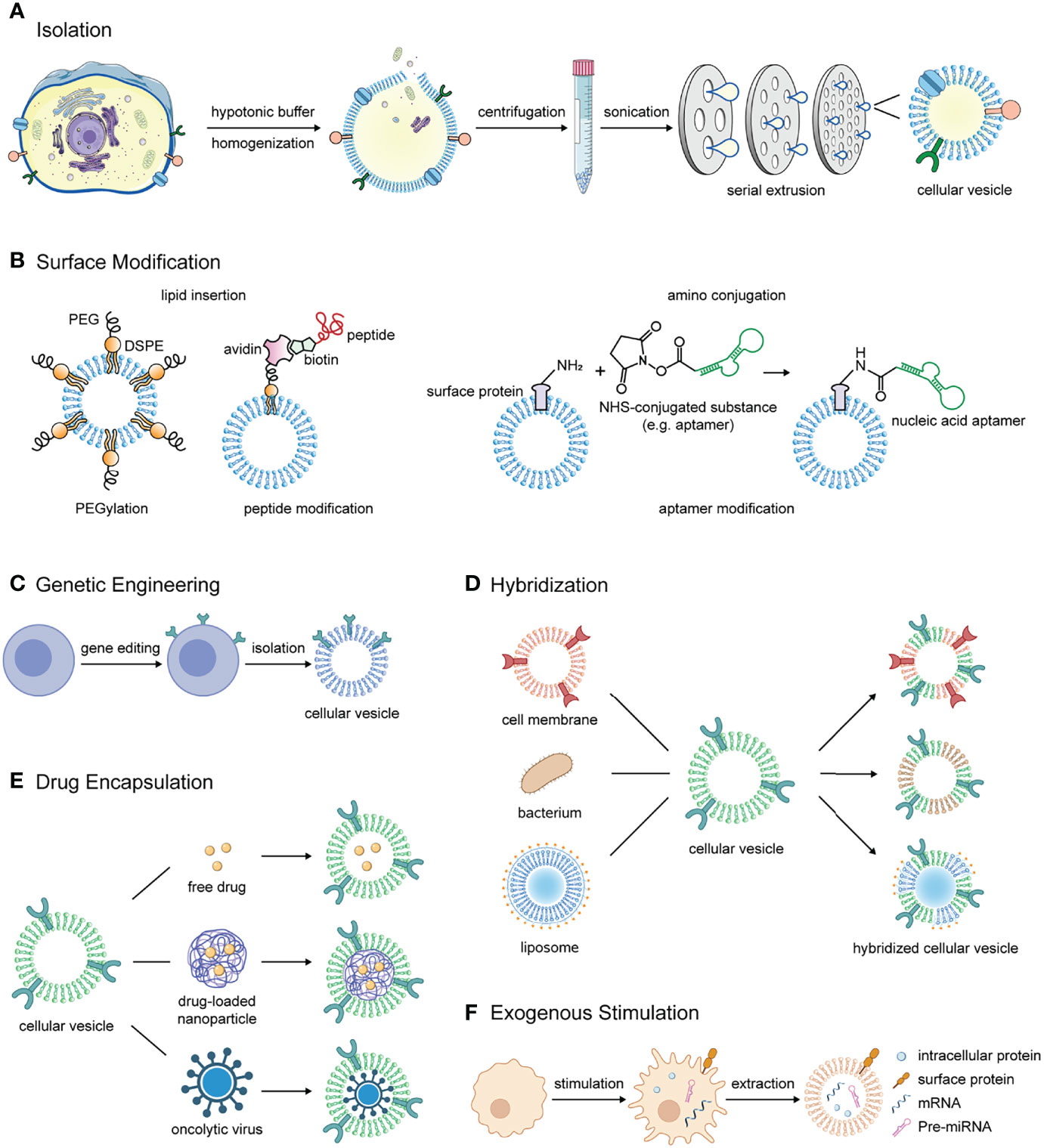
Figure 1 The manufacturing process, modification methods, and application strategies of cellular vesicles in combinational immunotherapy of cancer. (A) The process of isolating cell membrane-derived vesicles from parental cells. (B) Methods for modifying substances such as polyethylene glycol, tumor targeting peptide and nucleic acid aptamer on the surface of cellular vesicles. (C) Cellular vesicles inherit antitumor proteins that the parental cells overexpress through gene editing. (D) Cellular vesicles are hybridized with different materials such as cell membranes from other sources, bacterial membranes and liposomes to obtain multiple components and functions for cancer immunotherapy. (E) Free drugs, drug-loaded nanoparticles or oncolytic viruses are encapsulated in cellular vesicles for delivery to the tumor lesions to activate antitumor immunity. (F) In response to exogenous stimulation, immune cells produce a variety of tumor growth inhibitors including surface markers, intracellular proteins, mRNA for pro-inflammatory cytokines, and certain miRNAs, which can be retained in cellular vesicles.
Surface Modification
Surface modification of cellular vesicles is a vital strategy to improve their stability and tumor targeting ability. The modification methods include lipid insertion and amino conjugation. In terms of modification contents, they mainly include peptides, nucleic acid aptamers, and polyethylene glycol (PEG) (Figure 1B).
Attachment of tumor-targeting peptides is one of the most commonly used modification. One approach is to resort to lipid insertion and biotin-avidin interaction (18). Another approach is conjugation via N-hydroxysuccinimide (NHS) group. NHS esters are capable of covalent coupling with primary amines on proteins. Therefore, NHS-PEG-folic acid could be linked with amino groups of vesicle proteins for targeting tumors highly expressing folate receptors (19, 20). The modification of nucleic acid aptamers can also be performed by amino coupling to achieve specific targeting of tumors with high expressions of nucleolin (15). PEGylation is able to increase the dispersion stability and prolong the circulation time (21–23).
Potential of Cellular Vesicles From Diverse Parental Cells in Cancer Immunotherapy
Tumor Cells
Tumor cell membrane-derived vesicles have some unique properties in cancer therapy including persistent existence, homotypic targeting, and antigen stimulation. Tumor cells can evade clearance by macrophages through expressing innate immune checkpoint CD47 to convey a negative signal of phagocytosis (24). This property was well preserved on its cellular vesicles and enabled a prolonged circulation in vivo. However, other immunosuppressive molecules expressed on tumor cells such as PD-L1, Galectin-9 and Siglec-15 may also be retained on cellular vesicles and inhibit the function of tumor-infiltrating lymphocytes (25–27).
Another important rationale for employing cancer cell vesicles as antitumor drug vehicles is homotypic targeting, which is probably through surface adhesion molecules such as N-cadherin and galectin-3 (28, 29). In a tumor self-targeting study, researchers prepared four kinds of cell membrane-encapsulated magnetic nanoparticles derived from different tumor cell lines. In the in vivo competition of tumor “homing”, vesicles derived from heterologous tumor cells were notably weaker than that from homologous cells (28). This finding was also verified in patient-derived xenograft (PDX) models. The fluorescence intensity of tumor cell membrane-encapsulated nanoparticles at the tumor site was 3 and 10 times higher than that of erythrocyte membrane-coated nanoparticles and bare nanoparticles, respectively. For tumor cell vesicles derived from various patients, 2.5 to 10-fold higher tumor-targeting capacity was observed when the source of the donor membrane was consistent with the host compared to inconsistent cases (30).
Carrying tumor antigens is also a non-negligible advantage of tumor cell-derived cellular vesicles. Tumor cell vesicles inherit tumor-associated antigens and tumor-specific antigens and can therefore be equipped with immunological adjuvant for the preparation of cancer vaccine (21, 31–33).
Immune Cells
The advantages of selecting immune cells as the source of cellular vesicles for antitumor drug vehicles are ease of genetic modification, natural cargo of antitumor components, and the ability to evade immune surveillance, target tumor cells and present tumor antigens.
Abnormal nuclear structure in erythrocytes and platelets as well as excessively active DNA replication and mutation in tumor cells pose additional impediments to gene editing. As for the immune cells, genetic engineering technologies have been widely used in manufacturing immune cells with chimeric antigen receptors (CARs) (34–37). Coating IR780-loaded mesoporous silica nanoparticles with GPC3-specific CAR-T cell membranes enhanced tumor-targeting capability, with tumors weighing less than half the weight of normal T cell membrane-coated nanoparticle treatment group (38).
Another noteworthy point is that vesicles extruded from immune cells carry naturally expressed pro-inflammatory and antitumor substances (13). Programmed cell death-1 (PD-1) and transforming growth factor-beta receptor (TGF-βR) expressed on T cells are considered to inhibit the antitumor effects of CD8+ T cells, however their retention on T-cell vesicles in turn neutralizes PD-L1 and TGF-β in the tumor microenvironment (TME). Meanwhile, antitumor substances expressed by T cells such as granzyme B and FasL can still induce apoptosis of tumor cells through their vesicles (13, 39). Studies on macrophages showed that cellular vesicles derived from M1-type macrophages contained high levels of IL-6 and tumor necrosis factor-alpha (TNF-α), which presented pro-inflammatory and tumoricidal effects in vivo (11, 14). Intravenous injection of M1 macrophage-derived vesicles alone was demonstrated to promote tumor-associated macrophage polarization toward M1 type and improve CD8+ T cell infiltration in TME (14).
In addition, vesicles originating from certain immune cells can evade clearance, target tumor sites, recognize cancer cells and present tumor antigens. The use of monocyte- and macrophage-derived vesicles as nanovehicles emphasizes on their ability to evade clearance by the mononuclear phagocyte system and the expression of α4β1 that interacts with the vascular cell adhesion molecule-1 (VCAM-1) of metastatic tumors (8, 40, 41). It was reported that macrophage J774 membrane-encapsulated nanoparticles exhibited delayed liver accumulation, with integrity maintained for up to 40 min, which is 2-fold longer than that of naked nanoparticles. Also, 25% of adherent particles coated with macrophage membrane were not phagocytized by Kupffer cells, significantly higher than uncoated nanoparticles (~ 9%). Drug delivery with macrophage vesicles increased the particle density at the tumor sites by approximately twofold (8). Besides, T-cell vesicles were shown to carry high levels of lymphocyte function-associated antigen-1 (LFA-1), which mediates the targeting of tumor sites via binding to intercellular adhesion molecule-1 (ICAM-1) highly expressed on tumor cells and inflamed endothelium (8, 39). Natural killer (NK) cell vesicles also have some degree of tumor homing ability due to aberrant expression of ligands for NK cell receptors (e.g., NKG2-D) in tumors (9). Neutrophil-derived vesicles have been shown to target tumor cells and inflammatory endothelium through three pairs of interactions including LFA-1/ICAM-1, β1 integrin/VCAM-1, and CD44/L-selectin (42). And DC vesicles could present tumor antigens to activate T cells (43, 44).
Erythrocytes and Platelets
The abundance in quantity and simplicity in composition have led to the extensive use of erythrocyte membranes as drug vehicles (45). Similar to tumor cells, erythrocytes also highly express CD47 to protect themselves from the attack of macrophages, thus prolong the circulation time (46–48).
Platelets play a significant role in tumor metastasis (49). In analogy to erythrocytes, platelets regulate self-homeostasis in the circulation by expressing phagocytic negative signal CD47 (50). Besides, it is worth noting that platelet-derived vesicles contain P-selectin protein. CD44 is highly expressed and acts as the primary P-selectin ligand on certain types of carcinoma cells. Therefore platelet-derived vesicles probably have some degree of tumor-targeting ability (51–53). However, it was also reported that platelet-derived vesicles rapidly bound to blood monocytes due to P-selectin-mediated adhesion, which would reduce their stability and half-life (54).
Other Types of Cells
In addition to the cells aforementioned, cellular vesicles obtained from fibroblasts and bacterial membrane for cancer treatment have also been reported. Fibroblast membrane-derived vesicles are superior in penetration into the TME, and bacterial membranes contribute to activation of the innate immune system (55–57).
Strategies and Applications of Cellular Vesicles in Combinational Immunotherapy of Cancer
Genetic Engineering of Cellular Vesicles
Gene editing allows cells to express proteins targeting tumor lesions or substances regulating immunity of TME, and the functionality can be perpetuated to their extruded vesicles (Figure 1C). For example, lentivirus carrying sequence of signal regulatory protein alpha (SIRPα), a ligand of CD47, and plasmid inserted with PD-1 sequence were transfected into different tumor cells. After being prepared into cellular vesicles separately, they were fabricated into fusion vesicles. The fusion vesicles were demonstrated to possess high levels of both SIRPα and PD-1 on the surface and have the ability to block innate and adaptive immune checkpoints simultaneously (10). To achieve targeted killing of tumor cells, CAR-T cells were extruded into cellular vesicles for hepatocellular carcinoma treatment (38). Besides, cellular vesicles were also engineered to overexpress antigens to stimulate T cell activation (58).
Hybridization of Cellular Vesicles From Different Type of Cells or With Other Materials
Hybridized cellular vesicles inherit function characteristics of both parental materials (Figure 1D). Fused or hybridized cellular vesicles can be produced by breaking up, mixing and then co-extruding through polycarbonate porous membranes. A large number of works have investigated the fusion of two or even more types of cell membrane vesicles to achieve multi-functionality (10, 14, 29, 59).
Bacteria are one of the most common immune stimulants, and Escherichia coli (E. coli) membrane vesicles have been successfully employed to transport tumor antigens and act as vaccines in the absence of adjuvants (60, 61). Researchers constructed fusion vesicles by hybridizing E. coli outer membrane vesicles and tumor cell vesicles in order to simultaneously augment innate and adaptive immunity for personalized tumor immunotherapy. The fusion vesicles could be effectively enriched in lymph nodes and inhibited the growth and lung metastasis of colorectal and breast tumors (57). In another similar study, tumor cell membrane and E. coli cytomembrane were co-extruded with nanoparticles to obtain hybrid vaccine. In 4T1 tumor model, the tumor-suppressive effect of fusion vesicles was significantly better than the simple combinational dosing of tumor vesicle-nanoparticles and E. coli vesicle-nanoparticles, with 60-day survival rates improved from 0 to 93% and 25%, respectively (62).
In addition, the advantages of liposomes can also be conferred to cellular vesicles by hybridization (9, 41). For instance, decoration of emtansine-carrying liposomes with macrophage membranes displayed a significant suppressing effect on lung metastasis of breast cancer (41).
Entrapping Drugs in the Hollow Cores of Cellular Vesicles
The hollow-core structure of cellular vesicles provides space for loading antitumor drugs and entrapping nanoparticles (Figure 1E). Encapsulation of free therapeutic agents can be achieved by co-incubation or remote loading (12, 63). And simple mixing of cellular vesicles and nanoparticles followed by co-extrusion is sufficient to prepare the desired cell membrane-coated nanoparticles (64). However, the surface charge interaction needs to be taken into account before wrapping the nanoparticles (65, 66). Studies of cellular vesicles loading chemotherapeutic drugs such as docetaxel, doxorubicin, camptothecin and oxaliplatin have been extensively reported (9, 18, 43, 48). These drugs can induce immunogenic cell death, and the combination with cellular vesicles confers an enhanced immune response. One of the interesting attempts was the combination of mature DC vesicles with oxaliplatin-loaded nanoparticles. Chemotherapy led to immunogenic cell death, and the camouflaged mature dendrosomes initiated T cell responses by presenting tumor antigens, thus amplifying antitumor immune responses (43). Cellular vesicles were also widely applied in cancer photothermal therapy, such as membrane-camouflaged indocyanine green nanoparticles, black phosphorus quantum dot and melanin nanoparticles (20, 38, 67–69). Oncolytic viruses can also be enveloped in cellular vesicles aiming to evade antiviral neutralizing antibodies and enhance tumor-targeting ability (70).
Stimulating Production of Natural Antitumor Substances Before Vesicle Extraction
Stimulating immune cells to express endogenous antitumor substances and then extruding for vesicles is also an important strategy (Figure 1F). Vesicles isolated from activated or polarized immune cells were able to retain not only the membrane protein properties of the parental cells but also their intracellular proteins, mRNA and miRNA under appropriate preparation (11, 13, 14). It was reported that M1-type macrophages-derived nanovesicles contained high levels of mRNA of multiple pro-inflammatory cytokines and they could promote macrophage polarization toward M1 and infiltration of CD8+ T cells into tumors (11, 14).
Comparison of Cellular Vesicles With Other Nanovehicles
The advantages of non-biomimetic nanovehicles (e.g., liposomes, polymer micelles, dendrimer, nanogels, mesoporous silica, metallic nanoparticles) include high yield, diverse chemical modifications and precise regulation of physicochemical properties. For example, pH-sensitive dendrimers, temperature-responsive nanogels and magnetic nanoparticles enable specific drug release, size switching, sol-gel transition, and magnetic hyperthermia at tumor sites (71–74). In comparison with that, cellular vesicles preserved membrane characteristics and functions of parental cells, thus exhibiting better biocompatibility, low immunogenicity, negligible toxicity, long circulation, and natural targeting ability (75).
Exosome is also emerging as an important drug delivery platform for cancer immunotherapy. Compared to cell membrane vesicles, the difference between exosomal surface proteins and cytoplasmic membranes offer unique possibilities for exosome as drug vehicles. For instance, co-expression of peptides with proteins highly expressed on exosome surface (e.g., tetraspanin CD9/CD63/CD81, LAMP-2B and lactadherin) by genetic engineering allows for their enrichment on exosomes (76–81). Anchoring drugs to the exosomal marker via a medium such as CP05 peptide-mediated CD63 linkage simplifies drug loading approaches (82). However, for cancer therapy, exosomes are mostly administered at doses of 100-600 μg exosomal proteins per mouse, which implies consumption of approximately 1 L cell supernatant, severely hindering the application of exosomes as drug vehicles (83, 84). Cell membrane vesicles and exosomes share most of the characteristics of biomimetic nanovehicles, yet the former has superiority in terms of yield, production stability, and size homogeneity (11, 12). Cell membrane vesicles are administered at doses similar to exosomes, but with yields up to 30-300 μg vesicle proteins/107 cells (12, 17, 41).
Future Perspectives
Through genetic modifications, membrane hybridization, drug encapsulation and exogenous stimulation, cellular vesicles were engineered to provide ideal vehicles for cancer immunotherapy drugs, including not only surface proteins but also internal nanoparticles, proteins, nucleic acids, and small-molecule drugs (Table 1).
Cellular vesicles hold great therapeutic promise as drug vehicles for combinational cancer immunotherapy, but translating these concepts into practical treatment approaches has proven challenging. Firstly, a key consideration of choosing cellular vesicles as a biomimetic cancer immunotherapy drug carrier is the low immunogenicity, but this also puts forward a requirement that donor cells have to be highly compatible with the recipient to avoid host rejection response. In the meantime, cellular vesicles homing to tumor lesions rely on homotypic recognition and targeting of tumor cells (28). The requirement for autologous cells, especially autologous tumor cells, limits their transformation to clinical applications. Currently, there are only two clinical trials using tumor cell membrane-derived vesicles for the treatment of malignant pleural effusion (NCT01854866 and NCT02657460) (85). Secondly, the hydrodynamic diameter of proteins is usually around 10 nm, while the diameter of the prepared cellular vesicles is approximately 100 to 200 nm. Therefore, the protein density and topology may need to be considered when overexpressing proteins of interest on the vesicle surface to preserve their biological activity as much as possible. Thirdly, when preparing multifunctional cellular vesicles by hybridization, it was noted that the ratio of two different membranes would affect the function of hybridized vesicles (69). In the case of mixed erythrocyte and tumor cell membrane-derived vesicles, an increase in the proportion of erythrocyte membranes displayed prolonged circulation, while an increase in the proportion of tumor cell membranes improved homotypic targeting ability (69). However, this was difficult to control as precisely as mixed liposome preparation and relied on empirical studies on most occasions. Besides, cellular vesicles are prone to spontaneous aggregation in external solutions. The extracted cell membranes can be stored at ultra-low temperature for a long time but not for extruded cellular vesicles. And there are many other problems to be solved in terms of manufacturing, storage, stability and efficiency.
In conclusion, cellular vesicles inherit the cell membrane and part of cytoplasmic components and functions of parental cells. They can serve as ideal drug vehicles for cancer combinational immunotherapy. Future research is particularly needed in the areas of engineering strategies, long-term stability and in vivo fate.
Author Contributions
CX drafted the paper and prepared the table and figures. XZ and DJ conceived and proofread the manuscript. All authors contributed to the article and approved the submitted version.
Funding
This study was supported by Shanghai Sailing Program (21YF1401900), National Natural Science Foundation of China (82073752, 81773620, and 81803529), Scientific and Innovative Action Plan of Shanghai (20S11904700 and 20JC1411000).
Conflict of Interest
The authors declare that the research was conducted in the absence of any commercial or financial relationships that could be construed as a potential conflict of interest.
Publisher’s Note
All claims expressed in this article are solely those of the authors and do not necessarily represent those of their affiliated organizations, or those of the publisher, the editors and the reviewers. Any product that may be evaluated in this article, or claim that may be made by its manufacturer, is not guaranteed or endorsed by the publisher.
References
1. Colli LM, Machiela MJ, Zhang H, Myers TA, Jessop L, Delattre O, et al. Landscape of Combination Immunotherapy and Targeted Therapy to Improve Cancer Management. Cancer Res (2017) 77(13):3666–71. doi: 10.1158/0008-5472.Can-16-3338
2. Zhang YN, Poon W, Tavares AJ, McGilvray ID, Chan WCW. Nanoparticle-Liver Interactions: Cellular Uptake and Hepatobiliary Elimination. J Control Release (2016) 240:332–48. doi: 10.1016/j.jconrel.2016.01.020
3. Chen YX, Wei CX, Lyu YQ, Chen HZ, Jiang G, Gao XL. Biomimetic Drug-Delivery Systems for the Management of Brain Diseases. Biomater Sci (2020) 8(4):1073–88. doi: 10.1039/c9bm01395d
4. Wang H, Liu Y, He R, Xu D, Zang J, Weeranoppanant N, et al. Cell Membrane Biomimetic Nanoparticles for Inflammation and Cancer Targeting in Drug Delivery. Biomater Sci (2020) 8(2):552–68. doi: 10.1039/c9bm01392j
5. Thanuja MY, Anupama C, Ranganath SH. Bioengineered Cellular and Cell Membrane-Derived Vehicles for Actively Targeted Drug Delivery: So Near and Yet So Far. Adv Drug Deliv Rev (2018) 132:57–80. doi: 10.1016/j.addr.2018.06.012
6. Li C, Zhao Z, Luo Y, Ning T, Liu P, Chen Q, et al. Macrophage-Disguised Manganese Dioxide Nanoparticles for Neuroprotection by Reducing Oxidative Stress and Modulating Inflammatory Microenvironment in Acute Ischemic Stroke. Adv Sci (Weinh) (2021) 8(20):e2101526. doi: 10.1002/advs.202101526
7. Zhang L, Li R, Chen H, Wei J, Qian H, Su S, et al. Human Cytotoxic T-Lymphocyte Membrane-Camouflaged Nanoparticles Combined With Low-Dose Irradiation: A New Approach to Enhance Drug Targeting in Gastric Cancer. Int J Nanomed (2017) 12:2129–42. doi: 10.2147/ijn.S126016
8. Parodi A, Quattrocchi N, van de Ven AL, Chiappini C, Evangelopoulos M, Martinez JO, et al. Synthetic Nanoparticles Functionalized With Biomimetic Leukocyte Membranes Possess Cell-Like Functions. Nat Nanotechnol (2013) 8(1):61–8. doi: 10.1038/nnano.2012.212
9. Pitchaimani A, Nguyen TDT, Aryal S. Natural Killer Cell Membrane Infused Biomimetic Liposomes for Targeted Tumor Therapy. Biomaterials (2018) 160:124–37. doi: 10.1016/j.biomaterials.2018.01.018
10. Meng QF, Zhao Y, Dong C, Liu L, Pan Y, Lai J, et al. Genetically Programmable Fusion Cellular Vesicles for Cancer Immunotherapy. Angew Chem Int Ed Engl (2021) 60(50):26320–26. doi: 10.1002/anie.202108342
11. Choo YW, Kang M, Kim HY, Han J, Kang S, Lee JR, et al. M1 Macrophage-Derived Nanovesicles Potentiate the Anticancer Efficacy of Immune Checkpoint Inhibitors. ACS Nano (2018) 12(9):8977–93. doi: 10.1021/acsnano.8b02446
12. Jang SC, Kim OY, Yoon CM, Choi DS, Roh TY, Park J, et al. Bioinspired Exosome-Mimetic Nanovesicles for Targeted Delivery of Chemotherapeutics to Malignant Tumors. ACS Nano (2013) 7(9):7698–710. doi: 10.1021/nn402232g
13. Hong J, Kang M, Jung M, Lee YY, Cho Y, Kim C, et al. T-Cell-Derived Nanovesicles for Cancer Immunotherapy. Adv Mater (2021) 33(33):e2101110. doi: 10.1002/adma.202101110
14. Rao L, Wu L, Liu Z, Tian R, Yu G, Zhou Z, et al. Hybrid Cellular Membrane Nanovesicles Amplify Macrophage Immune Responses Against Cancer Recurrence and Metastasis. Nat Commun (2020) 11(1):4909. doi: 10.1038/s41467-020-18626-y
15. Peng LH, Zhang YH, Han LJ, Zhang CZ, Wu JH, Wang XR, et al. Cell Membrane Capsules for Encapsulation of Chemotherapeutic and Cancer Cell Targeting in Vivo. ACS Appl Mater Interfaces (2015) 7(33):18628–37. doi: 10.1021/acsami.5b05065
16. Gao J, Chu D, Wang Z. Cell Membrane-Formed Nanovesicles for Disease-Targeted Delivery. J Control Release (2016) 224:208–16. doi: 10.1016/j.jconrel.2016.01.024
17. Li R, He Y, Zhu Y, Jiang L, Zhang S, Qin J, et al. Route to Rheumatoid Arthritis by Macrophage-Derived Microvesicle-Coated Nanoparticles. Nano Lett (2019) 19(1):124–34. doi: 10.1021/acs.nanolett.8b03439
18. Chai Z, Ran D, Lu L, Zhan C, Ruan H, Hu X, et al. Ligand-Modified Cell Membrane Enables the Targeted Delivery of Drug Nanocrystals to Glioma. ACS Nano (2019) 13(5):5591–601. doi: 10.1021/acsnano.9b00661
19. Mishra PR, Jain NK. Folate Conjugated Doxorubicin-Loaded Membrane Vesicles for Improved Cancer Therapy. Drug Deliv (2003) 10(4):277–82. doi: 10.1080/drd_10_4_277
20. Li X, Zhao X, Pardhi D, Wu Q, Zheng Y, Zhu H, et al. Folic Acid Modified Cell Membrane Capsules Encapsulating Doxorubicin and Indocyanine Green for Highly Effective Combinational Therapy. Vivo Acta Biomater (2018) 74:374–84. doi: 10.1016/j.actbio.2018.05.006
21. Ochyl LJ, Bazzill JD, Park C, Xu Y, Kuai R, Moon JJ. PEGylated Tumor Cell Membrane Vesicles as a New Vaccine Platform for Cancer Immunotherapy. Biomaterials (2018) 182:157–66. doi: 10.1016/j.biomaterials.2018.08.016
22. Kooijmans SAA, Fliervoet LAL, van der Meel R, Fens M, Heijnen HFG, van Bergen En Henegouwen PMP, et al. PEGylated and Targeted Extracellular Vesicles Display Enhanced Cell Specificity and Circulation Time. J Control Release (2016) 224:77–85. doi: 10.1016/j.jconrel.2016.01.009
23. Suk JS, Xu Q, Kim N, Hanes J, Ensign LM. PEGylation as a Strategy for Improving Nanoparticle-Based Drug and Gene Delivery. Adv Drug Deliv Rev (2016) 99(Pt A):28–51. doi: 10.1016/j.addr.2015.09.012
24. Zhang W, Huang Q, Xiao W, Zhao Y, Pi J, Xu H, et al. Advances in Anti-Tumor Treatments Targeting the CD47/Sirpα Axis. Front Immunol (2020) 11:18. doi: 10.3389/fimmu.2020.00018
25. Cervantes-Villagrana RD, Albores-García D, Cervantes-Villagrana AR, García-Acevez SJ. Tumor-Induced Neurogenesis and Immune Evasion as Targets of Innovative Anti-Cancer Therapies. Signal Transd Target Ther (2020) 5(1):99. doi: 10.1038/s41392-020-0205-z
26. Yang R, Sun L, Li CF, Wang YH, Yao J, Li H, et al. Galectin-9 Interacts With PD-1 and TIM-3 to Regulate T Cell Death and Is a Target for Cancer Immunotherapy. Nat Commun (2021) 12(1):832. doi: 10.1038/s41467-021-21099-2
27. Sun J, Lu Q, Sanmamed MF, Wang J. Siglec-15 as an Emerging Target for Next-Generation Cancer Immunotherapy. Clin Cancer Res (2021) 27(3):680–88. doi: 10.1158/1078-0432.Ccr-19-2925
28. Zhu JY, Zheng DW, Zhang MK, Yu WY, Qiu WX, Hu JJ, et al. Preferential Cancer Cell Self-Recognition and Tumor Self-Targeting by Coating Nanoparticles With Homotypic Cancer Cell Membranes. Nano Lett (2016) 16(9):5895–901. doi: 10.1021/acs.nanolett.6b02786
29. Song J, Jung H, You G, Mok H. Cancer-Cell-Derived Hybrid Vesicles From MCF-7 and HeLa Cells for Dual-Homotypic Targeting of Anticancer Drugs. Macromol Biosci (2021) 21(7):e2100067. doi: 10.1002/mabi.202100067
30. Rao L, Yu G-T, Meng Q-F, Bu L-L, Tian R, Lin L-S, et al. Cancer Cell Membrane-Coated Nanoparticles for Personalized Therapy in Patient-Derived Xenograft Models. Adv Funct Mater (2019) 29(51):1905671. doi: 10.1002/adfm.201905671
31. Fang RH, Hu CM, Luk BT, Gao W, Copp JA, Tai Y, et al. Cancer Cell Membrane-Coated Nanoparticles for Anticancer Vaccination and Drug Delivery. Nano Lett (2014) 14(4):2181–8. doi: 10.1021/nl500618u
32. Yang R, Xu J, Xu L, Sun X, Chen Q, Zhao Y, et al. Cancer Cell Membrane-Coated Adjuvant Nanoparticles With Mannose Modification for Effective Anticancer Vaccination. ACS Nano (2018) 12(6):5121–29. doi: 10.1021/acsnano.7b09041
33. Liu B, Yang Y, Chao Y, Xiao Z, Xu J, Wang C, et al. Equipping Cancer Cell Membrane Vesicles With Functional DNA as a Targeted Vaccine for Cancer Immunotherapy. Nano Lett (2021) 21(22):9410-18. doi: 10.1021/acs.nanolett.1c02582
34. Bailey SR, Maus MV. Gene Editing for Immune Cell Therapies. Nat Biotechnol (2019) 37(12):1425–34. doi: 10.1038/s41587-019-0137-8
35. Sloas C, Gill S, Klichinsky M. Engineered CAR-Macrophages as Adoptive Immunotherapies for Solid Tumors. Front Immunol (2021) 12:783305. doi: 10.3389/fimmu.2021.783305
36. Schmidt P, Raftery MJ, Pecher G. Engineering NK Cells for CAR Therapy-Recent Advances in Gene Transfer Methodology. Front Immunol (2020) 11:611163. doi: 10.3389/fimmu.2020.611163
37. Xu C, Ju D, Zhang X. Chimeric Antigen Receptor T-Cell Therapy: Challenges and Opportunities in Lung Cancer. Antib Ther (2022) 5(1):73–83. doi: 10.1093/abt/tbac006
38. Ma W, Zhu D, Li J, Chen X, Xie W, Jiang X, et al. Coating Biomimetic Nanoparticles With Chimeric Antigen Receptor T Cell-Membrane Provides High Specificity for Hepatocellular Carcinoma Photothermal Therapy Treatment. Theranostics (2020) 10(3):1281–95. doi: 10.7150/thno.40291
39. Kang M, Hong J, Jung M, Kwon SP, Song SY, Kim HY, et al. T-Cell-Mimicking Nanoparticles for Cancer Immunotherapy. Adv Mater (2020) 32(39):e2003368. doi: 10.1002/adma.202003368
40. Krishnamurthy S, Gnanasammandhan MK, Xie C, Huang K, Cui MY, Chan JM. Monocyte Cell Membrane-Derived Nanoghosts for Targeted Cancer Therapy. Nanoscale (2016) 8(13):6981–5. doi: 10.1039/c5nr07588b
41. Cao H, Dan Z, He X, Zhang Z, Yu H, Yin Q, et al. Liposomes Coated With Isolated Macrophage Membrane Can Target Lung Metastasis of Breast Cancer. ACS Nano (2016) 10(8):7738–48. doi: 10.1021/acsnano.6b03148
42. Kang T, Zhu Q, Wei D, Feng J, Yao J, Jiang T, et al. Nanoparticles Coated With Neutrophil Membranes Can Effectively Treat Cancer Metastasis. ACS Nano (2017) 11(2):1397–411. doi: 10.1021/acsnano.6b06477
43. Chen X, Ling X, Xia J, Zhu Y, Zhang L, He Y, et al. Mature Dendritic Cell-Derived Dendrosomes Swallow Oxaliplatin-Loaded Nanoparticles to Boost Immunogenic Chemotherapy and Tumor Antigen-Specific Immunotherapy. Bioactive Mater (2021) 15:15–28. doi: 10.1016/j.bioactmat.2021.12.020
44. Ochyl LJ, Moon JJ. Dendritic Cell Membrane Vesicles for Activation and Maintenance of Antigen-Specific T Cells. Adv Healthc Mater (2019) 8(4):e1801091. doi: 10.1002/adhm.201801091
45. Ihler GM, Glew RH, Schnure FW. Enzyme Loading of Erythrocytes. Proc Natl Acad Sci USA (1973) 70(9):2663–6. doi: 10.1073/pnas.70.9.2663
46. Lutz HU. Innate Immune and non-Immune Mediators of Erythrocyte Clearance. Cell Mol Biol (Noisy-le-grand) (2004) 50(2):107–16.
47. Zhang X, Fan J, Ju D. Insights Into CD47/Sirpα Axis-Targeting Tumor Immunotherapy. Antib Ther (2018) 1(2):37–42. doi: 10.1093/abt/tby006
48. Malhotra S, Dumoga S, Sirohi P, Singh N. Red Blood Cells-Derived Vesicles for Delivery of Lipophilic Drug Camptothecin. ACS Appl Mater Interfaces (2019) 11(25):22141–51. doi: 10.1021/acsami.9b04827
49. Schlesinger M. Role of Platelets and Platelet Receptors in Cancer Metastasis. J Hematol Oncol (2018) 11(1):125. doi: 10.1186/s13045-018-0669-2
50. Olsson M, Bruhns P, Frazier WA, Ravetch JV, Oldenborg PA. Platelet Homeostasis Is Regulated by Platelet Expression of CD47 Under Normal Conditions and in Passive Immune Thrombocytopenia. Blood (2005) 105(9):3577–82. doi: 10.1182/blood-2004-08-2980
51. Hanley WD, Napier SL, Burdick MM, Schnaar RL, Sackstein R, Konstantopoulos K. Variant Isoforms of CD44 are P- and L-Selectin Ligands on Colon Carcinoma Cells. FASEB J (2006) 20(2):337–9. doi: 10.1096/fj.05-4574fje
52. Alves CS, Burdick MM, Thomas SN, Pawar P, Konstantopoulos K. The Dual Role of CD44 as a Functional P-Selectin Ligand and Fibrin Receptor in Colon Carcinoma Cell Adhesion. Am J Physiol Cell Physiol (2008) 294(4):C907–16. doi: 10.1152/ajpcell.00463.2007
53. Iannacone M. Platelet-Mediated Modulation of Adaptive Immunity. Semin Immunol (2016) 28(6):555–60. doi: 10.1016/j.smim.2016.10.008
54. Chimen M, Evryviadou A, Box CL, Harrison MJ, Hazeldine J, Dib LH, et al. Appropriation of Gpibα From Platelet-Derived Extracellular Vesicles Supports Monocyte Recruitment in Systemic Inflammation. Haematologica (2020) 105(5):1248–61. doi: 10.3324/haematol.2018.215145
55. Li J, Zhen X, Lyu Y, Jiang Y, Huang J, Pu K. Cell Membrane Coated Semiconducting Polymer Nanoparticles for Enhanced Multimodal Cancer Phototheranostics. ACS Nano (2018) 12(8):8520–30. doi: 10.1021/acsnano.8b04066
56. Kuerban K, Gao X, Zhang H, Liu J, Dong M, Wu L, et al. Doxorubicin-Loaded Bacterial Outer-Membrane Vesicles Exert Enhanced Anti-Tumor Efficacy in Non-Small-Cell Lung Cancer. Acta Pharm Sin B (2020) 10(8):1534–48. doi: 10.1016/j.apsb.2020.02.002
57. Zou MZ, Li ZH, Bai XF, Liu CJ, Zhang XZ. Hybrid Vesicles Based on Autologous Tumor Cell Membrane and Bacterial Outer Membrane To Enhance Innate Immune Response and Personalized Tumor Immunotherapy. Nano Lett (2021) 21(20):8609–18. doi: 10.1021/acs.nanolett.1c02482
58. Ukrainskaya V, Rubtsov Y, Pershin D, Podoplelova N, Terekhov S, Yaroshevich I, et al. Antigen-Specific Stimulation and Expansion of CAR-T Cells Using Membrane Vesicles as Target Cell Surrogates. Small (2021) 17(45):e2102643. doi: 10.1002/smll.202102643
59. Dehaini D, Wei X, Fang RH, Masson S, Angsantikul P, Luk BT, et al. Erythrocyte-Platelet Hybrid Membrane Coating for Enhanced Nanoparticle Functionalization. Adv Mater (2017) 29(16):1606209. doi: 10.1002/adma.201606209
60. Chen DJ, Osterrieder N, Metzger SM, Buckles E, Doody AM, DeLisa MP, et al. Delivery of Foreign Antigens by Engineered Outer Membrane Vesicle Vaccines. Proc Natl Acad Sci USA (2010) 107(7):3099–104. doi: 10.1073/pnas.0805532107
61. Fessler J, Matson V, Gajewski TF. Exploring the Emerging Role of the Microbiome in Cancer Immunotherapy. J Immunother Cancer (2019) 7(1):108. doi: 10.1186/s40425-019-0574-4
62. Chen L, Qin H, Zhao R, Zhao X, Lin L, Chen Y, et al. Bacterial Cytoplasmic Membranes Synergistically Enhance the Antitumor Activity of Autologous Cancer Vaccines. Sci Transl Med (2021) 13(601):eabc2816. doi: 10.1126/scitranslmed.abc2816
63. Zhang X, Angsantikul P, Ying M, Zhuang J, Zhang Q, Wei X, et al. Remote Loading of Small-Molecule Therapeutics Into Cholesterol-Enriched Cell-Membrane-Derived Vesicles. Angew Chem Int Ed Engl (2017) 56(45):14075–79. doi: 10.1002/anie.201707598
64. Luk BT, Hu CM, Fang RH, Dehaini D, Carpenter C, Gao W, et al. Interfacial Interactions Between Natural RBC Membranes and Synthetic Polymeric Nanoparticles. Nanoscale (2014) 6(5):2730–7. doi: 10.1039/c3nr06371b
65. Hu CM, Fang RH, Luk BT, Chen KN, Carpenter C, Gao W, et al. ‘Marker-Of-Self’ Functionalization of Nanoscale Particles Through a Top-Down Cellular Membrane Coating Approach. Nanoscale (2013) 5(7):2664–8. doi: 10.1039/c3nr00015j
66. Xia Q, Zhang Y, Li Z, Hou X, Feng N. Red Blood Cell Membrane-Camouflaged Nanoparticles: A Novel Drug Delivery System for Antitumor Application. Acta Pharm Sin B (2019) 9(4):675–89. doi: 10.1016/j.apsb.2019.01.011
67. Han Y, Pan H, Li W, Chen Z, Ma A, Yin T, et al. T Cell Membrane Mimicking Nanoparticles With Bioorthogonal Targeting and Immune Recognition for Enhanced Photothermal Therapy. Adv Sci (Weinh) (2019) 6(15):1900251. doi: 10.1002/advs.201900251
68. Liang X, Ye X, Wang C, Xing C, Miao Q, Xie Z, et al. Photothermal Cancer Immunotherapy by Erythrocyte Membrane-Coated Black Phosphorus Formulation. J Control Release (2019) 296:150–61. doi: 10.1016/j.jconrel.2019.01.027
69. Jiang Q, Liu Y, Guo R, Yao X, Sung S, Pang Z, et al. Erythrocyte-Cancer Hybrid Membrane-Camouflaged Melanin Nanoparticles for Enhancing Photothermal Therapy Efficacy in Tumors. Biomaterials (2019) 192:292–308. doi: 10.1016/j.biomaterials.2018.11.021
70. Lv P, Liu X, Chen X, Liu C, Zhang Y, Chu C, et al. Genetically Engineered Cell Membrane Nanovesicles for Oncolytic Adenovirus Delivery: A Versatile Platform for Cancer Virotherapy. Nano Lett (2019) 19(5):2993–3001. doi: 10.1021/acs.nanolett.9b00145
71. Xia C, Yin S, Xu S, Ran G, Deng M, Mei L, et al. Low Molecular Weight Heparin-Coated and Dendrimer-Based Core-Shell Nanoplatform With Enhanced Immune Activation and Multiple Anti-Metastatic Effects for Melanoma Treatment. Theranostics (2019) 9(2):337–54. doi: 10.7150/thno.29026
72. Li HJ, Du JZ, Liu J, Du XJ, Shen S, Zhu YH, et al. Smart Superstructures With Ultrahigh pH-Sensitivity for Targeting Acidic Tumor Microenvironment: Instantaneous Size Switching and Improved Tumor Penetration. ACS Nano (2016) 10(7):6753–61. doi: 10.1021/acsnano.6b02326
73. Zhou X, He X, Shi K, Yuan L, Yang Y, Liu Q, et al. Injectable Thermosensitive Hydrogel Containing Erlotinib-Loaded Hollow Mesoporous Silica Nanoparticles as a Localized Drug Delivery System for NSCLC Therapy. Adv Sci (Weinh) (2020) 7(23):2001442. doi: 10.1002/advs.202001442
74. Singh A, Jain S, Sahoo SK. Magnetic Nanoparticles for Amalgamation of Magnetic Hyperthermia and Chemotherapy: An Approach Towards Enhanced Attenuation of Tumor. Mater Sci Eng C Mater Biol Appl (2020) 110:110695. doi: 10.1016/j.msec.2020.110695
75. Xu CH, Ye PJ, Zhou YC, He DX, Wei H, Yu CY. Cell Membrane-Camouflaged Nanoparticles as Drug Carriers for Cancer Therapy. Acta Biomater (2020) 105:1–14. doi: 10.1016/j.actbio.2020.01.036
76. Liang G, Kan S, Zhu Y, Feng S, Feng W, Gao S. Engineered Exosome-Mediated Delivery of Functionally Active miR-26a and Its Enhanced Suppression Effect in HepG2 Cells. Int J Nanomed (2018) 13:585–99. doi: 10.2147/IJN.S154458
77. Kanuma T, Yamamoto T, Kobiyama K, Moriishi E, Masuta Y, Kusakabe T, et al. CD63-Mediated Antigen Delivery Into Extracellular Vesicles via DNA Vaccination Results in Robust CD8(+) T Cell Responses. J Immunol (2017) 198(12):4707–15. doi: 10.4049/jimmunol.1600731
78. Dooley K, McConnell RE, Xu K, Lewis ND, Haupt S, Youniss MR, et al. A Versatile Platform for Generating Engineered Extracellular Vesicles With Defined Therapeutic Properties. Mol Ther (2021) 29(5):1729–43. doi: 10.1016/j.ymthe.2021.01.020
79. Tian Y, Li S, Song J, Ji T, Zhu M, Anderson GJ, et al. A Doxorubicin Delivery Platform Using Engineered Natural Membrane Vesicle Exosomes for Targeted Tumor Therapy. Biomaterials (2014) 35(7):2383–90. doi: 10.1016/j.biomaterials.2013.11.083
80. Limoni SK, Moghadam MF, Moazzeni SM, Gomari H, Salimi F. Engineered Exosomes for Targeted Transfer of siRNA to HER2 Positive Breast Cancer Cells. Appl Biochem Biotechnol (2019) 187(1):352–64. doi: 10.1007/s12010-018-2813-4
81. Rountree RB, Mandl SJ, Nachtwey JM, Dalpozzo K, Do L, Lombardo JR, et al. Exosome Targeting of Tumor Antigens Expressed by Cancer Vaccines Can Improve Antigen Immunogenicity and Therapeutic Efficacy. Cancer Res (2011) 71(15):5235–44. doi: 10.1158/0008-5472.Can-10-4076
82. Gao X, Ran N, Dong X, Zuo B, Yang R, Zhou Q, et al. Anchor Peptide Captures, Targets, and Loads Exosomes of Diverse Origins for Diagnostics and Therapy. Sci Transl Med (2018) 10(444):eaat0195. doi: 10.1126/scitranslmed.aat0195
83. Gupta D, Zickler AM, El Andaloussi S. Dosing Extracellular Vesicles. Adv Drug Deliv Rev (2021) 178:113961. doi: 10.1016/j.addr.2021.113961
84. Kimiz-Gebologlu I, Oncel SS. Exosomes: Large-Scale Production, Isolation, Drug Loading Efficiency, and Biodistribution and Uptake. J Control Release (2022) 347:533–43. doi: 10.1016/j.jconrel.2022.05.027
Keywords: cellular vesicle, drug delivery vehicle, cancer immunotherapy, combination therapy, membrane hybridization, drug encapsulation
Citation: Xu C, Ju D and Zhang X (2022) Cell Membrane-Derived Vesicle: A Novel Vehicle for Cancer Immunotherapy. Front. Immunol. 13:923598. doi: 10.3389/fimmu.2022.923598
Received: 19 April 2022; Accepted: 14 June 2022;
Published: 07 July 2022.
Edited by:
Alessandra Zingoni, Sapienza University of Rome, ItalyReviewed by:
Abhalaxmi Singh, University of Illinois at Chicago, United StatesCopyright © 2022 Xu, Ju and Zhang. This is an open-access article distributed under the terms of the Creative Commons Attribution License (CC BY). The use, distribution or reproduction in other forums is permitted, provided the original author(s) and the copyright owner(s) are credited and that the original publication in this journal is cited, in accordance with accepted academic practice. No use, distribution or reproduction is permitted which does not comply with these terms.
*Correspondence: Dianwen Ju, ZGlhbndlbmp1QGZ1ZGFuLmVkdS5jbg==; Xuyao Zhang, eHV5YW96aGFuZ0BmdWRhbi5lZHUuY24=