- 1Department of Pediatric Hematology and Oncology, Cell and Gene Therapy, Bambino Gesù Children’s Hospital, IRCCS, Rome, Italy
- 2Department of Molecular Medicine, Sapienza University of Rome, Rome, Italy
- 3Department of Experimental Medicine, Sapienza University of Rome, Rome, Italy
- 4Department of Maternal and Child Health, Sapienza University of Rome, Rome, Italy
Optimal recovery of immune competence after periods of hematopoietic insults or stress is crucial to re-establish patient response to vaccines, pathogens and tumor antigens. This is particularly relevant for patients receiving high doses of chemotherapy or radiotherapy, who experience prolonged periods of lymphopenia, which can be associated with an increased risk of infections, malignant relapse, and adverse clinical outcome. While the thymus represents the primary organ responsible for the generation of a diverse pool of T cells, its function is profoundly impaired by a range of acute insults (including those caused by cytoreductive chemo/radiation therapy, infections and graft-versus-host disease) and by the chronic physiological deterioration associated with aging. Impaired thymic function increases the risk of infections and tumor antigen escape due to a restriction in T-cell receptor diversity and suboptimal immune response. Therapeutic approaches that can promote the renewal of the thymus have the potential to restore immune competence in patients. Previous work has documented the importance of the crosstalk between thymocytes and thymic epithelial cells in establishing correct architecture and function of thymic epithelium. This crosstalk is relevant not only during thymus organogenesis, but also to promote the recovery of its function after injuries. In this review, we will analyze the signals involved in the crosstalk between TECs and hematopoietic cells. We will focus in particular on how signals from T-cells can regulate TEC function and discuss the relevance of these pathways in restoring thymic function and T-cell immunity in experimental models, as well as in the clinical setting.
Introduction
Optimal immune recovery after periods of hematopoietic insults is key to reestablish patient immune competence and sustain response to vaccines, pathogens and tumor antigens. This is particularly relevant for patients receiving high doses of chemotherapy or radiotherapy, for instance, associated with the conditioning regimen employed in preparation to hematopoietic cell transplantation (HCT). These patients experience profound and prolonged periods of lymphopenia, which can be associated with an increased risk of developing life-threatening infections and, in cancer patients, tumor relapse. In fact, infections and relapse have been inversely correlated with the degree of immune reconstitution and account for greater than 50% of mortality after allogenic HCT (allo-HCT) (1–5).
The thymus represents the primary organ responsible for the maturation and differentiation of a broad pool of naïve T cells capable of recognizing an extremely large array of pathogens and tumor antigens. The process of T-cell development involves the migration of bone marrow-derived T-cell progenitors through the thymus and requires physical contact between developing thymocytes and the supporting thymic stromal microenvironment which consists of thymic epithelial cells (TECs), macrophages, endothelial cells (ECs), fibroblasts and dendritic cells (DCs) (6–8). Multiple developmental pathways, including Notch, Sonic Hedgehog, and WNT coordinate this complex hierarchical process (9–12). Despite its crucial role in generating T cells, thymic function may be profoundly impaired by acute insults, such as that caused by infections, stress, chemotherapy and radiotherapy. Delayed or defective recovery of thymic function has been associated with adverse clinical outcomes in patients receiving allo-HCT (13–18). Thymic function progressively declines with age, a well-known physiological process known as thymic involution (19). Age-associated thymic involution limits the recovery of thymic function after acute insults and significantly contributes to the decline of T-cell receptor (TCR) diversity in older individuals (20, 21). As a direct consequence, older patients are more prone to bacterial and viral infections and, possibly, to tumor antigen escape. The identification of clinical strategies that can restore thymic function and enhance immune reconstitution represent a major clinical need.
Through the mechanistic understanding of the molecules and pathways driving the maintenance of thymic function and its recovery after insults, several potential regenerative targets have been identified. They include growth factors (such as bone morphogenetic protein 4, stem cell factor, kit ligand and keratinocyte growth factor), the modulation of hormones (such as the inhibition of sex steroids and the use of growth hormone, insulin-like growth factor-1 and ghrelin), cytokines (such as interleukin (IL)-7, IL-12 and IL-21), chemokines (such as CXCL12/CXCR4) and the adoptive transfer of preformed T-cell progenitors, as well as ex vivo expanded thymus-derived endothelial cells (22). However, at present, none of these approaches is approved as a standard therapy to enhance thymic function and immune reconstitution.
Thymic Crosstalk Regulates Tissue Maintenance and Its Regeneration
Thymic crosstalk, a set of reciprocal regulations between thymocytes and the thymic environment, is critical to orchestrate thymocyte and TEC development, as well as to start thymic recovery after periods of stress or immunological injuries (23). Thymic epithelium represents a predominant stromal cell population within the thymus, which is classically divided into two subsets based on their spatial distribution and specialized function: cortical TECs (cTECs) and medullary TECs (mTECs) are responsible for positive and negative selection of thymocytes, respectively (10).
cTECs are critical for fate commitment, expansion, and positive selection of the developing thymocytes. On the other hand, mTECs are involved in the negative selection and maturation of thymocytes (2–4). mTECs can be further divided based on the expression of MHCII and additional molecules, such as CD40 and CD80/86. Within thymic microenvironment, while innate lymphoid cells (ILCs), endothelial cells and fibroblasts are mostly resistant to damage (24–26), thymic epithelium is particularly sensitive to the effects of chemotherapy and radiotherapy, with the MHCIIhigh mTEC subset representing the population most sensitive to insults, likely due to the high proliferative rate of these cells (27, 28).
TECs play a fundamental role in the development and selection of T cells providing key thymopoietic signals, including Interleukin-7 (IL-7), Notch-ligand Delta Like 1 and 4, as well as self-peptide–MHC complex. On the other hand, the maturation and maintenance of TECs is closely dependent on instructive signals provided by the bone marrow-derived lymphoid component. Indeed, thymocyte-derived signals are indispensable for the appropriate development and spatial organization of cTEC and mTEC subsets during late fetal development and adult life as revealed through the use of different genetic mouse models (29–31). Tcra KO and Zeta-chain-associated protein kinase 70 (Zap70) KO mice, in which thymocyte development is blocked at the double positive (DP) stage, showed severely impaired thymic medulla organization (32, 33). Similarly, Recombination activating gene (Rag)1 KO and Rag2 KO mice, in which thymocyte development is arrested at the double negative (DN) 3 stage, showed impaired medulla formation. Transgenic mice expressing high copies of the human CD3 epsilon molecule, which display a block at the DN1 stage of differentiation, showed impaired cortical thymic function and disrupted thymic architecture (34, 35). Importantly, transplantation of T-cell depleted bone marrow cells in severe combined immunodeficiency (SCID) mice, restored thymic architecture organization (36). In addition, the transfer of mature T cells into SCID mice promoted the recovery of the medullary epithelial structure, providing evidence that the regenerative signals on thymic epithelium can be instructed by both progenitor and mature T cells (37).
Interestingly, data suggesting that the infusion of mature T cells can boost thymic and immune recovery come also from clinical studies in which patients received allo-HCT followed by the adoptive transfer of donor T cells. Vago et al. demonstrated that the transfer of donor T cells genetically engineered to express the Herpes Simplex Virus thymidine kinase suicide gene (a safety switch system to be activated in case of graft-versus-host disease, GvHD) induced improved thymic function, as demonstrated by increased levels of T-cell receptor excision circles (TRECs) and recent thymic emigrants (RTEs) (38). Using chest tomography scans, this study also demonstrated that patients infused with modified T cells showed enlargement of active thymic tissue when compared to pre-transplant levels (38). In addition, recent observations collected at our center suggested that patients receiving donor T-cells genetically modified with the inducible Caspase 9 suicide gene showed rapid recovery of thymic function evaluated by the quantification of TRECs in patient peripheral blood (39). Data on enhanced immune recovery after the infusion of mature T-cells in patients, come also from studies in which the adoptive transfer of virus-specific T-cells generated a broad enhancement of the T-cell immunity (40, 41). The beneficial effect of the infused mature T cells on thymic function is likely to be transient in nature, but sufficient to provide regenerative signals, which result in faster recovery of thymic structure and accelerated immune reconstitution post damage. Nevertheless, it remains to be explored the long term persistence of these effects. The characterization of the underlying mechanisms of such effects could be of valuable importance to reveal pathways crucial for the regeneration of the human thymus that can be exploited to develop immune boosting therapies.
Regenerative Pathways
In this review, we will analyze the signals involved in the crosstalk between TECs and T-cells, looking beyond the process of thymocyte maturation and exploring how signals from T cells can regulate TEC function (Figure 1). Although several pathways (including Notch and Hedgehog) are known to have pivotal roles in T-cell and TEC development, we will highlight crosstalk signals described to regulate thymic function and T-cell immunity postnatally in experimental models, as well as in the clinical setting.
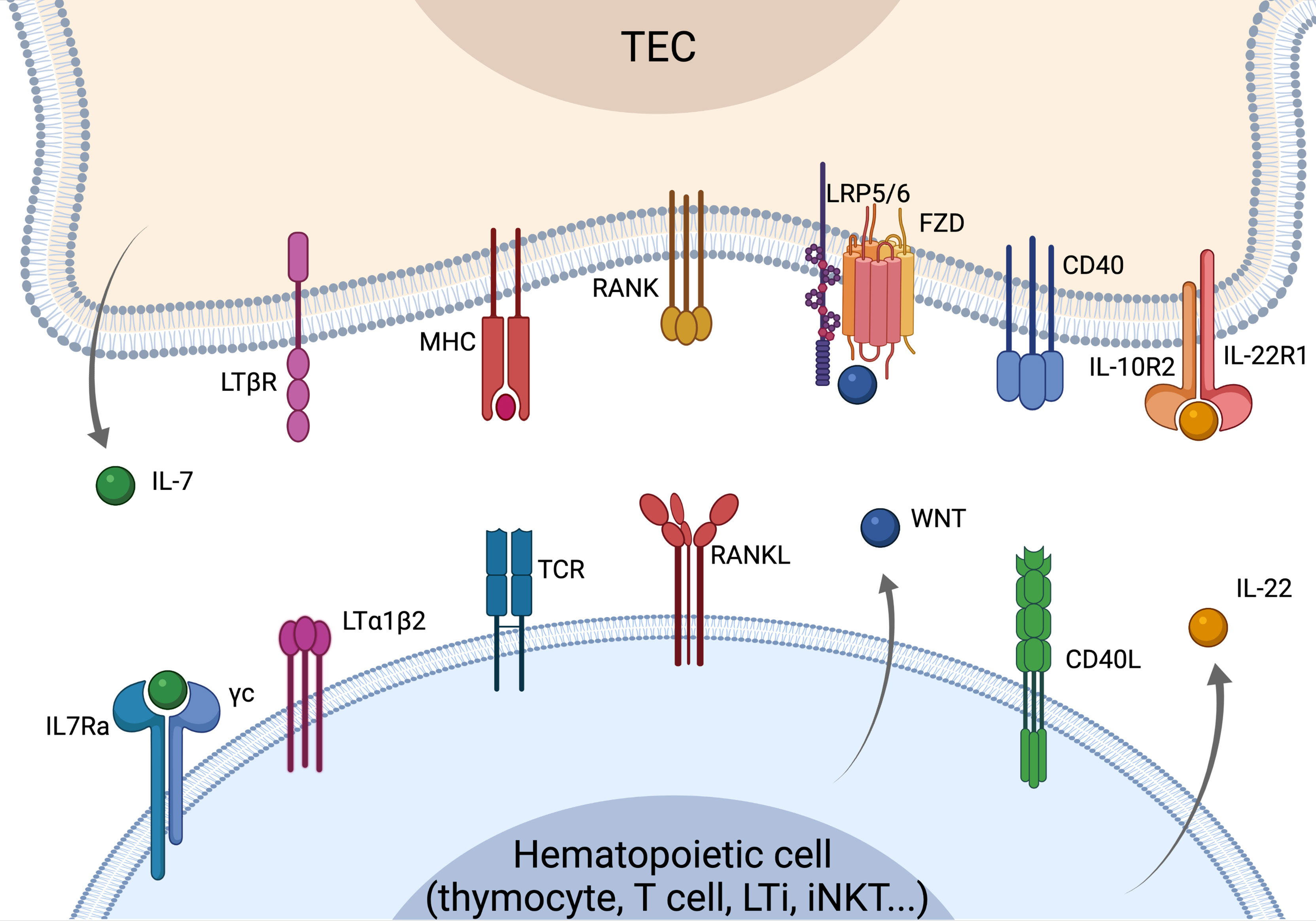
Figure 1 Overview of the crosstalk signals driving TEC development, differentiation and regeneration. Recover of thymus function is strictly dependent on the crosstalk signals between TECs and cells of the hematopoietic compartment. LTα and RANKL are mostly provided by SP thymocytes leading to mTEC maturation and differentiation. RANKL is overexpressed by CD4 thymocytes and LTi cells upon insults and can drive the recovery of thymus function. WNT signaling regulates TEC proliferation and homeostasis, while CD40L is involved in TEC maturation and proliferation. LTi- and T-cells-derived IL-22 is key in sustaining TEC proliferation and thymus recovery upon insults. IL-7 is mostly produced by cTEC acting as a key mediator of thymocyte maturation and proliferation. Elements of the figure were generated using Biorender.com.
RANKL
Receptor activator of nuclear factor kappa B ligand (RANKL) is a TNF superfamily member encoded by Tnfsf11 gene in mouse (42). Although a soluble form of RANKL (sRANKL) exists, this factor is expressed as a type II transmembrane protein whose ectodomain specifically interacts with its cognate receptor RANK (encoded by Tnfrsf11a). Thus, RANK-RANKL signaling is mostly mediated by the physical interaction of different cell types. RANK stimulation results in both canonical and non-canonical NF-kB signaling, together with MAPK activation (43). These events lead to the upregulation of genes involved in proliferation, survival and differentiation, thus resulting in pleiotropic effects on human physiology. First identified as a key component of bone metabolism, RANKL was then characterized as a crucial mediator in both organ development and immunity (44). In fact, despite having normal splenic architecture, Tnfrsf11a KO mice show null lymph-nodes organogenesis, while Tnfsf11 KO mice show reduced thymic size and block of thymocyte maturation between DN3 and DN4 stage of differentiation (45, 46).
Within the thymus, RANK is expressed by subsets of mTECs residing in both Aire+ and Aire- subpopulations (47, 48). On the other hand, RANKL is mostly provided by CD4 SP thymocytes and LTi cells, while CD8 SP thymocytes and invariant natural killer T (iNKT) cells contribute for the presentation of RANKL to a lesser extent (48–50). In this context, cell-cell interactions are of paramount importance in controlling central tolerance and T-cell production, as RANK signaling stimulates Aire+ mTEC maturation in concert with CD40 and LTα pathways (47, 51). Importantly, Aire+ mTECHI cells are also the primary cell population responsible for the production of osteoprotegerin (OPG) in the thymus, a soluble decoy receptor for RANKL encoded by Tnfrsf11b (52). OPG binding to RANKL inhibits its interaction with RANK. In fact, thymus tissues from Tnfrsf11b KO mice show increased mTEC cellularity (50, 53).
Besides its roles in thymic physiology, RANK-RANKL pathway is also implied in thymic regeneration upon immunological insults. In fact, RANKL is upregulated in CD4 thymocytes and LTi cells during thymus recovery in mice exposed to sublethal total body irradiation (SL-TBI) (54). On the other hand, a recent report demonstrated that increased CD4 T-cell-mediated RANK signaling in the thymus causes enhanced generation of mTEC. This results in an imbalance of cTEC and mTEC proportion, eventually leading to defective thymopoiesis (55). For its pivotal role in health and disease, the administration of RANKL or RANKL partial agonists has been exploited in mouse models reproducing particular clinical conditions, such as psoriasis and ischemic stroke (54, 56–58). Furthermore, in mouse models of HCT, sRANKL exogenous administration drives TEC regeneration, as demonstrated by increase in cellularity of thymic epithelial progenitor cells, cTEC and mTEC subsets (54). sRANKL-treated mice also showed early homing of lymphoid progenitors in the thymus and T-cell reconstitution (54). Moreover, Desanti et al. showed that stimulation of mTEC progenitors with RANK agonistic antibodies resulted in CD40 upregulation, thus suggesting a role in mTEC maturation (59).
CD40L
CD40L is a transmembrane protein and a tumor necrosis factor (TNF) superfamily component playing key roles in both innate and adaptive immunity (60). CD40L is expressed by activated T and B cells, basophils, monocytes, NK and mast cells and signals through physical interaction with its cognate receptor CD40 (61). The latter is a transmembrane costimulatory receptor firstly identified on B cells as a factor responsible for their activation and proliferation (62). In subsequent studies, CD40 was also reported to be expressed by activated T cells, DCs, fibroblasts, epithelial and endothelial cells (60, 63–66). CD40 signaling drives upregulation of co-stimulatory molecules, cytokine production and cross-presentation of the antigen in DCs, thus promoting DC-mediated T-cell activation (60, 67). Moreover, it was shown that CD8 and CD4 T cells directly communicate through CD40-CD40L interaction and this pathway is indispensable for the generation of CD8 T-cell memory (66).
As CD40-CD40L axis plays a crucial function in antigen presenting cell (APC) regulation, several studies investigated the role of CD40 signaling within the thymus in the context of T-cell development and selection, and self-tolerance induction (68). Here, similarly and in synergy with RANKL, CD40L stimulates mTEC maturation in the postnatal thymus, with both Cd40 KO and Cd40lg KO mice showing a reduction in mTECs without affecting cTEC compartment (27, 51). On the other hand, Dunn et al. produced transgenic mice expressing CD40L cDNA under the control of the proximal lck promoter (69). These mice carrying Cd40lg overexpression in thymocytes showed alterations in organ architecture, with an abnormal mTEC proportion and reduction in thymus cortex (69).
Within the thymic medulla, RANKL and CD40L are upregulated in CD4 single positive (SP) thymocytes, this finding suggesting a key role of CD4 SP in regulating mTEC maturation and homeostasis (50, 59, 70). However, flow-cytometry analyses highlighted a great heterogeneity within CD4 SP population, with CD25-CD4+TCRβhigh thymocytes showing the highest RANKL positivity during the early SP stage (CD69+), while being mostly CD40L+ in subsequent maturation steps (CD69-) (59). This temporal regulation of TNF family ligands expression in thymocytes is paralleled by a RANKL-dependent CD40 upregulation in mTECs, eventually leading to mTEC proliferation and maturation (59).
Lymphotoxin-α
Lymphotoxin-α (LTα) is another member of TNF superfamily that was originally identified as a soluble factor secreted by lymphocytes having cytotoxic effects on tumor cells (71, 72). Subsequent studies showed that, besides its soluble homotrimer (LTα3) form, LTα could associate with the transmembrane protein LTβ resulting in the membrane-bound heterotrimer LTα1β2 (73). The latter signals through cell-cell interactions with its cognate receptor LTβR, resulting in both canonical and non-canonical activation of NF-kB pathway (74, 75). This signaling has several implications in immunity including the regulation of lymphoid organ development. In fact, both Lta, Ltb and Ltbr KO mice show similar phenotypes lacking lymph nodes and Peyer’s patches, and abnormal splenic architecture (76–78).
Besides activated T and B cells, LTα1β2 is also expressed by NKs and type 3 ILCs (ILC3). On the other hand, LTβR is mainly expressed by epithelial and endothelial cells among macrophages and DCs (72).
Within the thymus, LTβR is expressed by the entire stromal compartment, especially by TECs, while LTα1β2 is mostly provided by single positive thymocytes (70, 79). Here, LTβ or LTβR deficiency leads to aberrant mTEC development and altered medulla organization (79–82). In particular, it was shown that LTα/LTβR signaling mediated by mature thymocytes is indispensable for the generation of terminally differentiated mTECs, as demonstrated by involucrin expression (83).
Besides its role in steady state, LTα is also important during insult recovery, as demonstrated by the fact that both Lta and Ltbr KO mice show impaired thymic recovery in in vivo models of HCT (47, 84). Upon SL-TBI, LTα1β2 upregulation is induced in radio-resistant LTi cells leading to thymic recovery through the stimulation of TEC proliferation and survival (54). On the other hand, LTα/LtβR signaling is also implied in T-cell progenitors homing and mature T-cells egress from the thymus in both steady state and HCT settings (84–86). For these reasons, LtβR agonistic antibody administration following HCT has been evaluated in mouse models, leading to an increase in thymic output and immune reconstitution (85).
Interleukin-7
IL-7 is a stromal-derived, non-redundant cytokine having a key role in regulating immunity and immune reconstitution (87). The active form of human IL-7 is a glycoprotein of 25 kDa that is mainly produced within the lymphoid organs and that signals through the IL−7 receptor (IL−7R) (2). The latter is a hetero−dimer consisting of IL−7Rα and the common cytokine receptor γ−chain (γc). Triggering of the receptor mediates anti−apoptotic and co−stimulatory proliferative signals, mostly on T- and B-cell lineages (88). In the thymus, IL-7 is primarily produced by TECs and fibroblasts (22). Using a IL-7 reporter mouse, it has been shown that TECs expressing high levels of IL-7 reside within a subset of cTECs defined as CD205+Ly51+CD40low (89). Cooperatively with Notch1, IL-7 provides proliferative signals to DN and DP thymocytes (90) and also sustains the recombination of the T-cell receptor γ−chain (TCRγ) locus (87). On the other hand, besides receiving maturation signals throughout their development, thymocytes control mTEC gene expression and differentiation, thus regulating the formation of a proper thymic microenvironment architecture (50, 70). For instance, thymocytes can downregulate Il7 expression by TECs in a negative feedback fashion (91). In fact, lymphopenic Rag2 Il2rg double KO mouse strain shows a markedly increased proportion of IL−7+ TECs compared to WT mice (91). IL-7R-deficient mice show defective thymic microenvironment, especially in corticomedullary structure, and reduced mTEC development (92, 93). While this phenotype is most likely due to a failure of the crosstalk normally provided by IL-7-dependent thymocytes and other cells of the hematopoietic lineage, a possible direct impact of IL-7 on thymic stromal cells is currently unknown. Interestingly, as discussed above, Vago et al. observed that serum levels of IL-7 peaked after every infusion of donor T-cells in transplanted patients, this suggesting that mature T-cells may induce IL-7 production, although the underlying mechanism is still largely obscure (38).
In the periphery, IL-7 has a key role in T-cell homeostatic proliferation and its production is tightly regulated, as the levels of IL-7 in the peripheral blood increase during lymphopenia remaining high until T-cell pool returns to steady state conditions (18, 94, 95). Given its crucial role in T-cell homeostasis, exogenous administration of IL-7 has been tested in several clinical conditions (87). In the context of HCT, IL-7 administration drives both CD4 and CD8 T-cell expansion, and this phenomenon is accompanied by an increase of TCR repertoire diversity (96). Most recently, IL-7 administration has been used in a murine model of age-related lymphopenia. Aged mice were subjected to IL-7 treatment and both numbers of CD4 and CD8 naïve T-cells in spleen and lymph nodes rose to levels similar to those observed in adult mice (97).
Interleukin-22
IL-22 is a monomeric cytokine released as a 179 amino acid monomeric protein (98). As IL-7, IL-22 is a non-conventional cytokine targeting stromal rather than hematopoietic compartment. In fact, the main targets of IL-22 are epithelial cells and fibroblasts within the thymus, liver, kidneys, lung and pancreas (99). On the other hand, the main contributors for IL-22 production are αβ and γδ T-cells, as well as ILCs, although fibroblasts, neutrophils and macrophages are also reported as secondary sources of IL-22 (99–104).
While systemic expression of IL22 is low during steady state, its production is induced upon negative stimuli, such as tissue injury and inflammation (99). During these pathologic conditions, IL-22 exerts controversial effects, being involved in both epithelial tissue regeneration and upregulation of different inflammatory mediators, including TNF, IL-6 and LPS-binding protein (105–107).
Within the thymus, IL-22 is involved in stromal regeneration following insults. In fact, IL-22 upregulation occurs in thymus-resident lymphoid tissue inducer (LTi) cells in mice exposed to SL-TBI (107). In turn, IL-22 production acts directly on mTEC compartment, providing proliferation and survival signals to the damaged tissue (107). Besides endogenous IL-22 production in injured thymus, recent findings demonstrated that exogenous administration of IL-22 could also promote faster thymic recovery. In fact, murine models of HCT showed that donor-derived T-cells are a major contributor for IL-22 production upon transplantation, leading to TEC proliferation and thymus recovery (108, 109). Moreover, exogenous IL-22 administration accelerates thymic regeneration after insults (107, 109).
Although IL-22 administration is currently being evaluated for the treatment of several conditions, only few trials are exploring the infusion of IL-22 or their agonists in the HCT setting (NCT02406651, NCT04539470). While these studies are primarily focused on acute GvHD treatment or prevention, the recent results herein reviewed suggest the possibility to use IL-22 in restoring thymic function during the first period after the transplant.
WNT
WNT-signaling plays an important role during thymic development and in the maintenance of its function in adult life (11). In humans, 19 different WNT family members have been identified along with 15 WNT receptors and coreceptors. WNT regulates the stabilization of β-catenin which, in the absence of any WNT signaling, is degraded in a cytoplasmatic “destruction complex” consisting of glycogen synthase kinase 3β (GSK), adenomatous polyposis coli (APC), axis inhibition protein (AXIN) and casein kinase (CK). After the binding of WNT to a member of the Frizzled receptor family and its coreceptors low-density lipoprotein-receptor related proteins (LRP) 5 and 6, the β-catenin is no longer degraded leading to its accumulation, activation and translocation to the cell nucleus where it regulates downstream transcription factors of the TCF/LEF family. The crucial role of WNT in the thymus has been demonstrated in several genetic models. Tcf-1 KO mice showed altered T-cell differentiation with a partial block at the double negative and immature single positive stages (110). Mice carrying a constituency active form of β-catenin in TECs show altered thymic organogenesis, reduced TEC proliferation and loss of TEC identity (111). The inhibition of WNT signaling through the forced expression of the canonical WNT inhibitor DKK1 leads to loss of TEC progenitors and thymic degeneration (112). Downregulation of WNT signaling has been also linked to the age-associated involution of the human thymus (113).
While stromal cells, such as TECs, are the major producers of WNT family members, cells of the hematopoietic lineage can also express WNTs. WNT proteins, such as WNT4 and WNT5b, expressed by TECs and thymocytes sustain the proliferation of TECs, which is partially achieved by increasing the expression of the key thymopoietic factor FoxN1 (114–116). Upregulation of FoxN1 expression represents a major step towards the regeneration of thymic function. Previous studies demonstrated that FoxN1 and its downstream genes are upregulated during the endogenous process of thymic reconstitution after sublethal dose of radiation (24). Importantly, induction of FoxN1 expression alone is sufficient to reverse thymic involution and regenerate the organ in mice (117). Together, these data demonstrate that the levels of FoxN1 tightly control thymic regeneration and the identification of factors regulating its expression could have a strong rationale for thymic boosting approaches. Whether mature T-cells can express members of the WNT family and induce the upregulation of FoxN1 in TECs when transferred in vivo would represent an interesting regenerative approach to investigate.
Conclusions
Several strategies have been proposed to restore thymic function after injuries and insults. Among these, the administration of chemokines and growth factors have been explored in several preclinical mouse studies displaying very promising results. However, when transferred to the clinic, the same strategies have shown modest regenerative potential. Until now, increasing thymic function and T-cell production remains a major challenge for the treatment of several conditions, especially in the early phase following HCT. Besides the HCT setting, boosting thymic function is of paramount importance for the treatment of other T-cell deficiencies associated with pathological, as well as physiological conditions. Thymic involution is a well-known phenomenon associated with a progressive decline of thymic size and output with age which paralleled with a decrease in immune surveillance in the elderly (118–120). Therapeutic approaches that can promote thymic function in older individuals can increase peripheral T-cell diversity, enhance the immunity against pathogens and response to vaccines, and, possibly, reduce the risk of malignancy through better immune-surveillance mechanisms against transformed cells. As previously discussed, the work by Vago et al. demonstrated that the infusion of mature donor T cells can rejuvenate the thymus of adult transplanted patients (aged 17-66). Whether a similar approach can restore TEC functionality in older individuals, in which the residual thymic tissue is limited (121) remains an avenue to be explored. On the other hand, it is highly unlikely that the same approach can mediate beneficial effects in restoring thymic function and the process of T-cell development in patients with intrinsic genetic defects which alter TEC function, for instance as a consequence of FoxN1 deficiency in patients affected by the nude/severe combined immunodeficiency. In fact, these defects cannot be mitigated by changes in the hematopoietic compartment as suggested by the inefficacy of bone marrow transplantation in these patients (122).
Author Contributions
MR, MC, IS, MF, FL and EV wrote, drafted, and edited the manuscript. All authors contributed to the article and approved the submitted version.
Funding
EV was supported by grants from the Amy Strelzer Manasevit Research Program; the Italian Association for Cancer Research (AIRC); and the Italian Ministry of Health (“Ricerca Corrente”). FL was supported by grants from AIRC (Special Program Metastatic disease: the key unmet need in oncology 5 per mille 2018 Project Code 21147 and Accelerator Award 2017 INCAR); Ministero dell’Istruzione, dell’Università e della Ricerca, PRIN ID 2017 WC8499_004; Ministero della Salute, RF-2016-02364388. IS and MPF, THYMINNOVA (IP-2020-02-2431).
Conflict of Interest
The authors declare that the research was conducted in the absence of any commercial or financial relationships that could be construed as a potential conflict of interest.
Publisher’s Note
All claims expressed in this article are solely those of the authors and do not necessarily represent those of their affiliated organizations, or those of the publisher, the editors and the reviewers. Any product that may be evaluated in this article, or claim that may be made by its manufacturer, is not guaranteed or endorsed by the publisher.
References
1. Small TN, Papadopoulos EB, Boulad F, Black P, Castro-Malaspina H, Childs BH, et al. Comparison of Immune Reconstitution After Unrelated and Related T-Cell- Depleted Bone Marrow Transplantation: Effect of Patient Age and Donor Leukocyte Infusions. Blood (1999) 93:467–80. doi: 10.1182/blood.v93.2.467
2. Maury S, Mary JY, Rabian C, Schwarzinger M, Toubert A, Scieux C, et al. Prolonged Immune Deficiency Following Allogeneic Stem Cell Transplantation: Risk Factors and Complications in Adult Patients. Br J Haematol (2001) 115:630–41. doi: 10.1046/j.1365-2141.2001.03135.x
3. Storek J, Joseph A, Espino G, Dawson MA, Douek DC, Sullivan KM, et al. Immunity of Patients Surviving 20 to 30 Years After Allogeneic or Syngeneic Bone Marrow Transplantation. Blood (2001) 98:3505–12. doi: 10.1182/blood.V98.13.3505
4. Storek J, Gooley T, Witherspoon RP, Sullivan KM, Storb R. Infectious Morbidity in Long-Term Survivors of Allogeneic Marrow Transplantation is Associated With Low CD4 T Cell Counts. Am J Hematol (1997) 54:131–8. doi: 10.1002/(SICI)1096-8652(199702)54:2<131::AID-AJH6>3.0.CO;2-Y
5. Jenq RR, Van Den Brink MRM. Allogeneic Haematopoietic Stem Cell Transplantation: Individualized Stem Cell and Immune Therapy of Cancer. Nat Rev Cancer (2010) 10:213–21. doi: 10.1038/nrc2804
6. Han J, Zúñiga-Pflücker JC. A 2020 View of Thymus Stromal Cells in T Cell Development. J Immunol (2021) 206:249–56. doi: 10.4049/jimmunol.2000889
7. Takahama Y. Journey Through the Thymus: Stromal Guides for T-Cell Development and Selection. Nat Rev Immunol (2006) 6:127–35. doi: 10.1038/nri1781
8. Petrie HT, Zúñiga-Pflücker JC. Zoned Out: Functional Mapping of Stromal Signaling Microenvironments in the Thymus. Annu Rev Immunol (2007) 25:649–79. doi: 10.1146/annurev.immunol.23.021704.115715
9. Saldaña JI, Solanki A, Lau C-I, Sahni H, Ross S, Furmanski AL, et al. Sonic Hedgehog Regulates Thymic Epithelial Cell Differentiation. J Autoimmun (2016) 68:86–97. doi: 10.1016/j.jaut.2015.12.004
10. Kadouri N, Nevo S, Goldfarb Y, Abramson J. Thymic Epithelial Cell Heterogeneity: TEC by TEC. Nat Rev Immunol (2020) 20:239–53. doi: 10.1038/s41577-019-0238-0
11. Famili F, Naber BAE, Vloemans S, De Haas EFE, Tiemessen MM, Staal FJT. Discrete Roles of Canonical and non-Canonical Wnt Signaling in Hematopoiesis and Lymphopoiesis. Cell Death Dis (2015) 6:1–10. doi: 10.1038/cddis.2015.326
12. Tsaouli G, Barbarulo A, Vacca A, Screpanti I, Felli MP. Molecular Mechanisms of Notch Signaling in Lymphoid Cell Lineages Development: NF-κb and Beyond. Adv Exp Med Biol (2020) 1227:145–64. doi: 10.1007/978-3-030-36422-9_10
13. Wils EJ, van der Holt B, Broers AEC, Posthumus-Van Sluijs SJ, Gratama JW, Braakman E, et al. Insufficient Recovery of Thymopoiesis Predicts for Opportunistic Infections in Allogeneic Hematopoietic Stem Cell Transplant Recipients. Haematologica (2011) 96:1846–54. doi: 10.3324/haematol.2011.047696
14. Talvensaari K, Clave E, Douay C, Rabian C, Garderet L, Busson M, et al. A Broad T-Cell Repertoire Diversity and an Efficient Thymic Function Indicate a Favorable Long-Term Immune Reconstitution After Cord Blood Stem Cell Transplantation. Blood (2002) 99:1458–64. doi: 10.1182/blood.V99.4.1458
15. Clave E, Rocha V, Talvensaari K, Busson M, Douay C, Appert ML, et al. Prognostic Value of Pretransplantation Host Thymic Function in HLA-Identical Sibling Hematopoietic Stem Cell Transplantation. Blood (2005) 105:2608–13. doi: 10.1182/blood-2004-04-1667
16. Clave E, Lisini D, Douay C, Giorgiani G, Busson M, Zecca M, et al. Thymic Function Recovery After Unrelated Donor Cord Blood or T-Cell Depleted HLA-Haploidentical Stem Cell Transplantation Correlates With Leukemia Relapse. Front Immunol (2013) 4:54. doi: 10.3389/fimmu.2013.00054
17. Clave E, Lisini D, Douay C, Giorgiani G, Busson M, Zecca M, et al. A Low Thymic Function is Associated With Leukemia Relapse in Children Given T-Cell-Depleted HLA-Haploidentical Stem Cell Transplantation. Leukemia (2012) 26:1886–8. doi: 10.1038/leu.2012.59
18. Politikos I, Kim HT, Nikiforow S, Li L, Brown J, Antin JH, et al. IL-7 and SCF Levels Inversely Correlate With T Cell Reconstitution and Clinical Outcomes After Cord Blood Transplantation in Adults. PloS One (2015) 10:1–15. doi: 10.1371/journal.pone.0132564
19. Thomas R, Wang W, Su D-M. Contributions of Age-Related Thymic Involution to Immunosenescence and Inflammaging. Immun Ageing (2020) 17:2. doi: 10.1186/s12979-020-0173-8
20. Nikolich-Žugich J. The Twilight of Immunity: Emerging Concepts in Aging of the Immune System. Nat Immunol (2018) 19:10–9. doi: 10.1038/s41590-017-0006-x
21. Mittelbrunn M, Kroemer G. Hallmarks of T Cell Aging. Nat Immunol (2021) 22:687–98. doi: 10.1038/s41590-021-00927-z
22. Velardi E, Tsai JJ, van den Brink MRM. T Cell Regeneration After Immunological Injury. Nat Rev Immunol (2021) 21:277–91. doi: 10.1038/s41577-020-00457-z
23. Lopes N, Sergé A, Ferrier P, Irla M. Thymic Crosstalk Coordinates Medulla Organization and T-Cell Tolerance Induction. Front Immunol (2015) 6:365. doi: 10.3389/fimmu.2015.00365
24. Wertheimer T, Velardi E, Tsai J, Cooper K, Xiao S, Kloss CC, et al. Production of BMP4 by Endothelial Cells is Crucial for Endogenous Thymic Regeneration. Sci Immunol (2018) 3. doi: 10.1126/sciimmunol.aal2736
25. Kelly RM, Goren EM, Taylor PA, Mueller SN, Stefanski HE, Osborn MJ, et al. Short-Term Inhibition of P53 Combined With Keratinocyte Growth Factor Improves Thymic Epithelial Cell Recovery and Enhances T-Cell Reconstitution After Murine Bone Marrow Transplantation. Blood (2010) 115:1088–97. doi: 10.1182/blood-2009-05-223198
26. Zhang SL, Wang X, Manna S, Zlotoff DA, Bryson JL, Blazar BR, et al. Chemokine Treatment Rescues Profound T-Lineage Progenitor Homing Defect After Bone Marrow Transplant Conditioning in Mice. Blood (2014) 124:296–304. doi: 10.1182/blood-2014-01-552794
27. Gray DHD, Seach N, Ueno T, Milton MK, Liston A, Lew AM, et al. Developmental Kinetics, Turnover, and Stimulatory Capacity of Thymic Epithelial Cells. Blood (2006) 108:3777–85. doi: 10.1182/blood-2006-02-004531
28. Gray D, Abramson J, Benoist C, Mathis D. Proliferative Arrest and Rapid Turnover of Thymic Epithelial Cells Expressing Aire. J Exp Med (2007) 204:2521–8. doi: 10.1084/jem.20070795
29. Klug DB, Carter C, Gimenez-Conti IB, Richie ER. Cutting Edge: Thymocyte-Independent and Thymocyte-Dependent Phases of Epithelial Patterning in the Fetal Thymus. J Immunol (2002) 169:2842–5. doi: 10.4049/jimmunol.169.6.2842
30. Shores EW, Van Ewijk W, Singer A. Maturation of Medullary Thymic Epithelium Requires Thymocytes Expressing Fully Assembled CD3-TCR Complexes. Int Immunol (1994) 6:1393–402. doi: 10.1093/intimm/6.9.1393
31. Cejalvo T, Munoz JJ, Tobajas E, Fanlo L, Alfaro D, García-Ceca J, et al. Ephrin-B–Dependent Thymic Epithelial Cell–Thymocyte Interactions Are Necessary for Correct T Cell Differentiation and Thymus Histology Organization: Relevance for Thymic Cortex Development. J Immunol (2013) 190:2670–81. doi: 10.4049/jimmunol.1201931
32. Palmer DB, Viney JL, Ritter MA, Hayday AC, Owen MJ. Expression of the Alpha Beta T-Cell Receptor is Necessary for the Generation of the Thymic Medulla. Dev Immunol (1993) 3:175–9. doi: 10.1155/1993/56290
33. Negishi I, Motoyama N, Nakayama K, Nakayama K, Senju S, Hatakeyama S, et al. Essential Role for ZAP-70 in Both Positive and Negative Selection of Thymocytes. Nature (1995) 376:435–8. doi: 10.1038/376435a0
34. Wang B, Biron C, She J, Higgins K, Sunshine MJ, Lacy E, et al. A Block in Both Early T Lymphocyte and Natural Killer Cell Development in Transgenic Mice With High-Copy Numbers of the Human CD3E Gene. Proc Natl Acad Sci U S A (1994) 91:9402–6. doi: 10.1073/pnas.91.20.9402
35. Holländer GA, Wang B, Nichogiannopoulou A, Platenburg PP, van Ewijk W, Burakoff SJ, et al. Developmental Control Point in Induction of Thymic Cortex Regulated by a Subpopulation of Prothymocytes. Nature (1995) 373:350–3. doi: 10.1038/373350a0
36. Shores EW, Van Ewijk W, Singer A. Disorganization and Restoration of Thymic Medullary Epithelial Cells in T Cell Receptor-Negative Scid Mice: Evidence That Receptor-Bearing Lymphocytes Influence Maturation of the Thymic Microenvironment. Eur J Immunol (1991) 21:1657–61. doi: 10.1002/eji.1830210711
37. Surh CD, Ernst B, Sprent J. Growth of Epithelial Cells in the Thymic Medulla is Under the Control of Mature T Cells. J Exp Med (1992) 176:611–6. doi: 10.1084/jem.176.2.611
38. Vago L, Oliveira G, Bondanza A, Noviello M, Soldati C, Ghio D, et al. T-Cell Suicide Gene Therapy Prompts Thymic Renewal in Adults After Hematopoietic Stem Cell Transplantation. Blood (2012) 120:1820–30. doi: 10.1182/blood-2012-01-405670
39. Catanoso M, Clave E, Douay C, Algeri M, Fournier I, Bertaina V, et al. T- and B-Cell Neogenesis Recovers Efficiently in Children With Acute Leukemia Given an Alpha-Beta T-Cell Depleted Haplo-HSCT Followed By Infusion of Donor T-Cells Genetically Modified With Inducible Caspase 9 Suicide Gene (BPX-501 Cells). Blood (2018) 132:4560–0. doi: 10.1182/blood-2018-99-116536
40. Perruccio K, Tosti A, Burchielli E, Topini F, Ruggeri L, Carotti A, et al. Transferring Functional Immune Responses to Pathogens After Haploidentical Hematopoietic Transplantation. Blood (2005) 106:4397–406. doi: 10.1182/blood-2005-05-1775
41. Comoli P, De Palma R, Siena S, Nocera A, Basso S, Del Galdo F, et al. Adoptive Transfer of Allogeneic Epstein-Barr Virus (EBV)-Specific Cytotoxic T Cells With In Vitro Antitumor Activity Boosts LMP2-Specific Immune Response in a Patient With EBV-Related Nasopharyngeal Carcinoma. Ann Oncol (2004) 15:113–7. doi: 10.1093/annonc/mdh027
42. Sobacchi C, Menale C, Villa A. The RANKL-RANK Axis: A Bone to Thymus Round Trip. Front Immunol (2019) 10:629. doi: 10.3389/fimmu.2019.00629
43. Akiyama T, Shinzawa M, Akiyama N. RANKL-RANK Interaction in Immune Regulatory Systems. World J Orthop (2012) 3:142–50. doi: 10.5312/wjo.v3.i9.142
44. Rao S, Cronin SJF, Sigl V, Penninger JM. RANKL and RANK: From Mammalian Physiology to Cancer Treatment. Trends Cell Biol (2018) 28:213–23. doi: 10.1016/j.tcb.2017.11.001
45. Kong YY, Yoshida H, Sarosi I, Tan HL, Timms E, Capparelli C, et al. OPGL is a Key Regulator of Osteoclastogenesis, Lymphocyte Development and Lymph-Node Organogenesis. Nature (1999) 397:315–23. doi: 10.1038/16852
46. Dougall WC, Glaccum M, Charrier K, Rohrbach K, Brasel K, De Smedt T, et al. RANK is Essential for Osteoclast and Lymph Node Development. Genes Dev (1999) 13:2412–24. doi: 10.1101/gad.13.18.2412
47. Irla M. RANK Signaling in the Differentiation and Regeneration of Thymic Epithelial Cells. Front Immunol (2021) 11:623265. doi: 10.3389/fimmu.2020.623265
48. McCarthy NI, Cowan JE, Nakamura K, Bacon A, Baik S, White AJ, et al. Osteoprotegerin-Mediated Homeostasis of Rank + Thymic Epithelial Cells Does Not Limit Foxp3 + Regulatory T Cell Development. J Immunol (2015) 195:2675–82. doi: 10.4049/jimmunol.1501226
49. White AJ, Lucas B, Jenkinson WE, Anderson G. Invariant NKT Cells and Control of the Thymus Medulla. J Immunol (2018) 200:3333–9. doi: 10.4049/jimmunol.1800120
50. Hikosaka Y, Nitta T, Ohigashi I, Yano K, Ishimaru N, Hayashi Y, et al. The Cytokine RANKL Produced by Positively Selected Thymocytes Fosters Medullary Thymic Epithelial Cells That Express Autoimmune Regulator. Immunity (2008) 29:438–50. doi: 10.1016/j.immuni.2008.06.018
51. Akiyama T, Shimo Y, Yanai H, Qin J, Ohshima D, Maruyama Y, et al. The Tumor Necrosis Factor Family Receptors RANK and CD40 Cooperatively Establish the Thymic Medullary Microenvironment and Self-Tolerance. Immunity (2008) 29:423–37. doi: 10.1016/j.immuni.2008.06.015
52. Tsukasaki M, Asano T, Muro R, Huynh NCN, Komatsu N, Okamoto K, et al. OPG Production Matters Where It Happened. Cell Rep (2020) 32:108124. doi: 10.1016/j.celrep.2020.108124
53. Akiyama N, Shinzawa M, Miyauchi M, Yanai H, Tateishi R, Shimo Y, et al. Limitation of Immune Tolerance-Inducing Thymic Epithelial Cell Development by Spi-B-Mediated Negative Feedback Regulation. J Exp Med (2014) 211:2425–38. doi: 10.1084/jem.20141207
54. Lopes N, Vachon H, Marie J, Irla M. Administration of RANKL Boosts Thymic Regeneration Upon Bone Marrow Transplantation. EMBO Mol Med (2017) 9:835–51. doi: 10.15252/emmm.201607176
55. Yin C, Pei XY, Shen H, Gao YN, Sun XY, Wang W, et al. Thymic Homing of Activated CD4+ T Cells Induces Degeneration of the Thymic Epithelium Through Excessive RANK Signaling. Sci Rep (2017) 7:1–12. doi: 10.1038/s41598-017-02653-9
56. Lo Iacono N, Blair HC, Poliani PL, Marrella V, Ficara F, Cassani B, et al. Osteopetrosis Rescue Upon RANKL Administration to Rankl-/- Mice: A New Therapy for Human RANKL-Dependent ARO. J Bone Miner Res (2012) 27:2501–10. doi: 10.1002/jbmr.1712
57. Shimamura M, Nakagami H, Shimizu H, Wakayama K, Kawano T, Ikeda Y, et al. Therapeutic Effects of Systemic Administration of the Novel RANKL-Modified Peptide, MHP1, for Ischemic Stroke in Mice. BioMed Res Int (2018) 2018:1–9. doi: 10.1155/2018/4637084
58. Ju N, Shimamura M, Hayashi H, Ikeda Y, Yoshida S, Nakamura A, et al. Preventative Effects of the Partial RANKL Peptide MHP1-AcN in a Mouse Model of Imiquimod-Induced Psoriasis. Sci Rep (2019) 9:1–8. doi: 10.1038/s41598-019-51681-0
59. Desanti GE, Cowan JE, Baik S, Parnell SM, White AJ, Penninger JM, et al. Developmentally Regulated Availability of RANKL and CD40 Ligand Reveals Distinct Mechanisms of Fetal and Adult Cross-Talk in the Thymus Medulla. J Immunol (2012) 189:5519–26. doi: 10.4049/jimmunol.1201815
60. Elgueta R, Benson MJ, De Vries VC, Wasiuk A, Guo Y, Noelle RJ. Molecular Mechanism and Function of CD40/CD40L Engagement in the Immune System. Immunol Rev (2009) 229:152–72. doi: 10.1111/j.1600-065X.2009.00782.x
61. Karnell JL, Rieder SA, Ettinger R, Kolbeck R. Targeting the CD40-CD40L Pathway in Autoimmune Diseases: Humoral Immunity and Beyond. Adv Drug Delivery Rev (2019) 141:92–103. doi: 10.1016/j.addr.2018.12.005
62. Clark EA, Ledbetter JA. Activation of Human B Cells Mediated Through Two Distinct Cell Surface Differentiation Antigens, Bp35 and Bp50. Proc Natl Acad Sci U S A (1986) 83:4494–8. doi: 10.1073/pnas.83.12.4494
63. Fries KM, Sempowski GD, Gaspari AA, Blieden T, Looney RJ, Phipps RP. CD40 Expression by Human Fibroblasts. Clin Immunol Immunopathol (1995) 77:42–51. doi: 10.1016/0090-1229(95)90135-3
64. Karmann K, Hughes CCW, Schechner J, Fanslowt WC, Pober JS. CD40 on Human Endothelial Cells: Inducibility by Cytokines and Functional Regulation of Adhesion Molecule Expression. Proc Natl Acad Sci USA (1995) 92:4342–6. doi: 10.1073/pnas.92.10.4342
65. Galy AH, Spits H. CD40 is Functionally Expressed on Human Thymic Epithelial Cells. J Immunol (1992) 149:775 LP–782.
66. Bourgeois C, Rocha B, Tanchot C. A Role for CD40 Expression on CD8+ T Cells in the Generation of CD8+ T Cell Memory. Science (2002) 297:2060–3. doi: 10.1126/science.1072615
67. Elmetwali T, Salman A, Wei W, Hussain SA, Young LS, Palmer DH. CD40L Membrane Retention Enhances the Immunostimulatory Effects of CD40 Ligation. Sci Rep (2020) 10:1–15. doi: 10.1038/s41598-019-57293-y
68. Akiyama T, Shinzawa M, Akiyama N. TNF Receptor Family Signaling in the Development and Functions of Medullary Thymic Epithelial Cells. Front Immunol (2012) 3:278. doi: 10.3389/fimmu.2012.00278
69. Dunn RJ, Luedecker CJ, Haugen HS, Clegg CH, Farr AG. Thymic Overexpression of CD40 Ligand Disrupts Normal Thymic Epithelial Organization. J Histochem Cytochem (1997) 45:129–41. doi: 10.1177/002215549704500116
70. Irla M, Hugues S, Gill J, Nitta T, Hikosaka Y, Williams IR, et al. Autoantigen-Specific Interactions With CD4+ Thymocytes Control Mature Medullary Thymic Epithelial Cell Cellularity. Immunity (2008) 29:451–63. doi: 10.1016/j.immuni.2008.08.007
71. Williams TW, Granger GA. Lymphocyte In Vitro Cytotoxicity: Lymphotoxins of Several Mammalian Species [35]. Nature (1968) 219:1076–7. doi: 10.1038/2191076a0
72. Borelli A, Irla M. Lymphotoxin: From the Physiology to the Regeneration of the Thymic Function. Cell Death Differ (2021) 28:2305–14. doi: 10.1038/s41418-021-00834-8
73. Browning JL, Ngam-ek A, Lawton P, DeMarinis J, Tizard R, Chow EP, et al. Lymphotoxin Beta, a Novel Member of the TNF Family That Forms a Heteromeric Complex With Lymphotoxin on the Cell Surface. Cell (1993) 72:847–56. doi: 10.1016/0092-8674(93)90574-a
74. Crowe PD, VanArsdale TL, Walter BN, Ware CF, Hession C, Ehrenfels B, et al. A Lymphotoxin-Beta-Specific Receptor. Science (1994) 264:707–10. doi: 10.1126/science.8171323
75. Upadhyay V, Fu YX. Lymphotoxin Signalling in Immune Homeostasis and the Control of Microorganisms. Nat Rev Immunol (2013) 13:270–9. doi: 10.1038/nri3406
76. Koni PA, Sacca R, Lawton P, Browning JL, Ruddle NH, Flavell RA. Distinct Roles in Lymphoid Organogenesis for Lymphotoxins Alpha and Beta Revealed in Lymphotoxin Beta-Deficient Mice. Immunity (1997) 6:491–500. doi: 10.1016/s1074-7613(00)80292-7
77. Fütterer A, Mink K, Luz A, Kosco-Vilbois MH, Pfeffer K. The Lymphotoxin Beta Receptor Controls Organogenesis and Affinity Maturation in Peripheral Lymphoid Tissues. Immunity (1998) 9:59–70. doi: 10.1016/s1074-7613(00)80588-9
78. De Togni P, Goellner J, Ruddle NH, Streeter PR, Fick A, Mariathasan S, et al. Abnormal Development of Peripheral Lymphoid Organs in Mice Deficient in Lymphotoxin. Science (1994) 264:703–7. doi: 10.1126/science.8171322
79. Boehm T, Scheu S, Pfeffer K, Bleul CC. Thymic Medullary Epithelial Cell Differentiation, Thymocyte Emigration, and the Control of Autoimmunity Require Lympho-Epithelial Cross Talk via Ltβr. J Exp Med (2003) 198:757–69. doi: 10.1084/jem.20030794
80. Sun L, Li H, Luo H, Zhao Y. Thymic Epithelial Cell Development and its Dysfunction in Human Diseases. BioMed Res Int (2014) 2014:206929. doi: 10.1155/2014/206929
81. Mouri Y, Yano M, Shinzawa M, Shimo Y, Hirota F, Nishikawa Y, et al. Lymphotoxin Signal Promotes Thymic Organogenesis by Eliciting RANK Expression in the Embryonic Thymic Stroma. J Immunol (2011) 186:5047–57. doi: 10.4049/jimmunol.1003533
82. Wu W, Shi Y, Xia H, Chai Q, Jin C, Ren B, et al. Epithelial Ltβr Signaling Controls the Population Size of the Progenitors of Medullary Thymic Epithelial Cells in Neonatal Mice. Sci Rep (2017) 7:1–11. doi: 10.1038/srep44481
83. White AJ, Nakamura K, Jenkinson WE, Saini M, Sinclair C, Seddon B, et al. Lymphotoxin Signals From Positively Selected Thymocytes Regulate the Terminal Differentiation of Medullary Thymic Epithelial Cells. J Immunol (2010) 185:4769–76. doi: 10.4049/jimmunol.1002151
84. Shi Y, Wu W, Chai Q, Li Q, Hou Y, Xia H, et al. Ltβr Controls Thymic Portal Endothelial Cells for Haematopoietic Progenitor Cell Homing and T-Cell Regeneration. Nat Commun (2016) 7:12369. doi: 10.1038/ncomms12369
85. Lucas B, James KD, Cosway EJ, Parnell SM, Tumanov AV, Ware CF, et al. Lymphotoxin β Receptor Controls T Cell Progenitor Entry to the Thymus. J Immunol (2016) 197:2665–72. doi: 10.4049/jimmunol.1601189
86. James KD, Cosway EJ, Lucas B, White AJ, Parnell SM, Carvalho-Gaspar M, et al. Endothelial Cells Act as Gatekeepers for Ltβrdependent Thymocyte Emigration. J Exp Med (2018) 215:2984–93. doi: 10.1084/jem.20181345
87. MacKall CL, Fry TJ, Gress RE. Harnessing the Biology of IL-7 for Therapeutic Application. Nat Rev Immunol (2011) 11:330–42. doi: 10.1038/nri2970
88. Jiang Q, Li WQ, Aiello FB, Mazzucchelli R, Asefa B, Khaled AR, et al. Cell Biology of IL-7, a Key Lymphotrophin. Cytokine Growth Factor Rev (2005) 16:513–33. doi: 10.1016/j.cytogfr.2005.05.004
89. Ribeiro AR, Rodrigues PM, Meireles C, Di Santo JP, Alves NL. Thymocyte Selection Regulates the Homeostasis of IL-7–Expressing Thymic Cortical Epithelial Cells. In Vivo. J Immunol (2013) 191:1200–9. doi: 10.4049/jimmunol.1203042
90. González-García S, García-Peydró M, Alcain J, Toribio ML. Notch1 and IL-7 Receptor Signalling in Early T-Cell Development and Leukaemia. Curr Top Microbiol Immunol (2012) 360:47–73. doi: 10.1007/82_2012_231
91. Alves NL, Huntington ND, Mention J-J, Richard-Le Goff O, Di Santo JP. Cutting Edge: A Thymocyte-Thymic Epithelial Cell Cross-Talk Dynamically Regulates Intrathymic IL-7 Expression. In Vivo. J Immunol (2010) 184:5949–53. doi: 10.4049/jimmunol.1000601
92. Moore AJ, In TS, Trotman-Grant A, Yoganathan K, Montpellier B, Guidos CJ, et al. A Key Role for IL-7R in the Generation of Microenvironments Required for Thymic Dendritic Cells. Immunol Cell Biol (2017) 95:933–42. doi: 10.1038/icb.2017.74
93. Duah M, Li L, Shen J, Lan Q, Pan B, Xu K. Thymus Degeneration and Regeneration. Front Immunol (2021) 12:706244. doi: 10.3389/fimmu.2021.706244
94. Bolotin E, Annett G, Parkman R, Weinberg K. Serum Levels of IL-7 in Bone Marrow Transplant Recipients: Relationship to Clinical Characteristics and Lymphocyte Count. Bone Marrow Transplant (1999) 23:783–8. doi: 10.1038/sj.bmt.1701655
95. Fry TJ, Connick E, Falloon J, Lederman MM, Liewehr DJ, Spritzler J, et al. A Potential Role for Interleukin-7 in T-Cell Homeostasis. Blood (2001) 97:2983–90. doi: 10.1182/blood.V97.10.2983
96. Perales MA, Goldberg JD, Yuan J, Koehne G, Lechner L, Papadopoulos EB, et al. Recombinant Human Interleukin-7 (CYT107) Promotes T-Cell Recovery After Allogeneic Stem Cell Transplantation. Blood (2012) 120:4882–91. doi: 10.1182/blood-2012-06-437236
97. Uhrlaub JL, Jergović M, Bradshaw CM, Sonar S, Coplen CP, Dudakov J, et al. Quantitative Restoration of Immune Defense in Old Animals Determined by Naïve Antigen-Specific CD8 T Cell Numbers. Aging Cell (2022), 21:e13582. doi: 10.1111/acel.13582
98. Nagem RAP, Colau D, Dumoutier L, Renauld JC, Ogata C, Polikarpov I. Crystal Structure of Recombinant Human Interleukin-22. Structure (2002) 10:1051–62. doi: 10.1016/S0969-2126(02)00797-9
99. Dudakov JA, Hanash AM, van den Brink MRM. Interleukin-22: Immunobiology and Pathology. Annu Rev Immunol (2015) 33:747–85. doi: 10.1146/annurev-immunol-032414-112123
100. Crellin NK, Trifari S, Kaplan CD, Satoh-Takayama N, Di Santo JP, Spits H. Regulation of Cytokine Secretion in Human CD127+ LTi-Like Innate Lymphoid Cells by Toll-Like Receptor 2. Immunity (2010) 33:752–64. doi: 10.1016/j.immuni.2010.10.012
101. Wolk K, Kunz S, Asadullah K, Sabat R. Cutting Edge: Immune Cells as Sources and Targets of the IL-10 Family Members? J Immunol (2002) 168:5397–402. doi: 10.4049/jimmunol.168.11.5397
102. Sanos SL, Bui VL, Mortha A, Oberle K, Heners C, Johner C, et al. Rorγt and Commensal Microflora are Required for the Differentiation of Mucosal Interleukin 22-Producing NKp46+ Cells. Nat Immunol (2009) 10:83–91. doi: 10.1038/ni.1684
103. Zindl CL, Lai JF, Lee YK, Maynard CL, Harbour SN, Ouyang W, et al. IL-22-Producing Neutrophils Contribute to Antimicrobial Defense and Restitution of Colonic Epithelial Integrity During Colitis. Proc Natl Acad Sci U S A (2013) 110:12768–73. doi: 10.1073/pnas.1300318110
104. Hansson M, Silverpil E, Lindén A, Glader P. Interleukin-22 Produced by Alveolar Macrophages During Activation of the Innate Immune Response. Inflammation Res (2013) 62:561–9. doi: 10.1007/s00011-013-0608-1
105. Sabat R, Ouyang W, Wolk K. Therapeutic Opportunities of the IL-22-IL-22R1 System. Nat Rev Drug Discovery (2014) 13:21–38. doi: 10.1038/nrd4176
106. Witte E, Witte K, Warszawska K, Sabat R, Wolk K. Interleukin-22: A Cytokine Produced by T, NK and NKT Cell Subsets, With Importance in the Innate Immune Defense and Tissue Protection. Cytokine Growth Factor Rev (2010) 21:365–79. doi: 10.1016/j.cytogfr.2010.08.002
107. Dudakov JA, Hanash AM, Jenq RR, Young LF, Ghosh A, Singer NV, et al. Interleukin-22 Drives Endogenous Thymic Regeneration in Mice. Science (80-) (2012) 336:91–5. doi: 10.1126/science.1218004
108. Pan B, Wang D, Li L, Shang L, Xia F, Zhang F, et al. IL-22 Accelerates Thymus Regeneration via Stat3/Mcl-1 and Decreases Chronic Graft-Versus-Host Disease in Mice After Allotransplants. Biol Blood Marrow Transplant (2019) 25:1911–9. doi: 10.1016/j.bbmt.2019.06.002
109. Pan B, Zhang F, Lu Z, Li L, Shang L, Xia F, et al. Donor T-Cell-Derived Interleukin-22 Promotes Thymus Regeneration and Alleviates Chronic Graft-Versus-Host Disease in Murine Allogeneic Hematopoietic Cell Transplant. Int Immunopharmacol (2019) 67:194–201. doi: 10.1016/j.intimp.2018.12.023
110. Verbeek S, Izon D, Hofhuis F, Robanus-Maandag E, te Riele H, van de Wetering M, et al. An HMG-Box-Containing T-Cell Factor Required for Thymocyte Differentiation. Nature (1995) 374:70–4. doi: 10.1038/374070a0
111. Zuklys S, Gill J, Keller MP, Hauri-Hohl M, Zhanybekova S, Balciunaite G, et al. Stabilized β-Catenin in Thymic Epithelial Cells Blocks Thymus Development and Function. J Immunol (2009) 182:2997–3007. doi: 10.4049/jimmunol.0713723
112. Osada M, Jardine L, Misir R, Andl T, Millar SE, Pezzano M. DKK1 Mediated Inhibition of Wnt Signaling in Postnatal Mice Leads to Loss of TEC Progenitors and Thymic Degeneration. PloS One (2010) 5:e9062. doi: 10.1371/journal.pone.0009062
113. Ferrando-Martínez S, Ruiz-Mateos E, Dudakov JA, Velardi E, Grillari J, Kreil DP, et al. WNT Signaling Suppression in the Senescent Human Thymus. Journals Gerontol - Ser A Biol Sci Med Sci (2015) 70:273–81. doi: 10.1093/gerona/glu030
114. Heinonen KM, Vanegas JR, Brochu S, Shan J, Vainio SJ, Perreault C. Wnt4 Regulates Thymic Cellularity Through the Expansion of Thymic Epithelial Cells and Early Thymic Progenitors. Blood (2011) 118:5163–73. doi: 10.1182/blood-2011-04-350553
115. Balciunaite G, Keller MP, Balciunaite E, Piali L, Zuklys S, Mathieu YD, et al. Wnt Glycoproteins Regulate the Expression of FoxNI, the Genes Defective in Nude Mice. Nat Immunol (2002) 3:1102–8. doi: 10.1038/ni850
116. Vaidya HJ, Briones Leon A, Blackburn CC. FOXN1 in Thymus Organogenesis and Development. Eur J Immunol (2016) 46:1826–37. doi: 10.1002/eji.201545814
117. Bredenkamp N, Nowell CS, Clare Blackburn C. Regeneration of the Aged Thymus by a Single Transcription Factor. Dev (2014) 141:1627–37. doi: 10.1242/dev.103614
118. Montecino-rodriguez E, Berent-maoz B, Dorshkind K, Montecino-rodriguez E, Berent-maoz B, Dorshkind K. Causes, Consequences, and Reversal of Immune System Aging Find the Latest Version : Review Series Causes, Consequences, and Reversal of Immune System Aging. J Clin Invest (2013) 123:958–65. doi: 10.1172/JCI64096.958
119. Lord JM. The Effect of Ageing of the Immune System on Vaccination Responses. Hum Vaccin Immunother (2013) 9:1364–7. doi: 10.4161/hv.24696
120. Berben L, Floris G, Wildiers H, Hatse S. Cancer and Aging: Two Tightly Interconnected Biological Processes. Cancers (Basel) (2021) 13. doi: 10.3390/cancers13061400
121. Drabkin MJ, Meyer JI, Kanth N, Lobel S, Fogel J, Grossman J, et al. Age-Stratified Patterns of Thymic Involution on Multidetector Ct. J Thorac Imaging (2018) 33:409–16. doi: 10.1097/RTI.0000000000000349
Keywords: immune reconstitution, thymus, T cells, immune-senescence, thymic epithelial cells
Citation: Rosichini M, Catanoso M, Screpanti I, Felli MP, Locatelli F and Velardi E (2022) Signaling Crosstalks Drive Generation and Regeneration of the Thymus. Front. Immunol. 13:920306. doi: 10.3389/fimmu.2022.920306
Received: 14 April 2022; Accepted: 17 May 2022;
Published: 06 June 2022.
Edited by:
Nicolai Stanislas van Oers, University of Texas Southwestern Medical Center, United StatesReviewed by:
Dong-Ming Su, University of North Texas Health Science Center, United StatesAnn Chidgey, Monash University, Australia
Copyright © 2022 Rosichini, Catanoso, Screpanti, Felli, Locatelli and Velardi. This is an open-access article distributed under the terms of the Creative Commons Attribution License (CC BY). The use, distribution or reproduction in other forums is permitted, provided the original author(s) and the copyright owner(s) are credited and that the original publication in this journal is cited, in accordance with accepted academic practice. No use, distribution or reproduction is permitted which does not comply with these terms.
*Correspondence: Enrico Velardi, ZW5yaWNvLnZlbGFyZGlAb3BiZy5uZXQ=