- 1Department of Medical Microbiology and Immunology, University of Toledo College of Medicine and Life Sciences, Toledo, OH, United States
- 2Department of Medicine, University of Toledo College of Medicine and Life Sciences, Toledo, OH, United States
- 3Center for Biomedical Research, University of Texas Health Science Center, Tyler, TX, United States
Properdin acts as an essential positive regulator of the alternative pathway of complement by stabilizing enzymatic convertases. Identical properdin monomers form head-to-tail associations of oligomers in a reported 20:54:26 ratio (most often described as an approximate 1:2:1 ratio) of tetramers (P4), trimers (P3), and dimers (P2), in blood, under normal physiological conditions. Oligomeric size is proportional to properdin function with tetramers being more active, followed by trimers and dimers. Neutrophils are the most abundant granulocyte, are recruited to inflammatory microenvironments, and are a significant source of properdin, yet the ratio of properdin oligomers released from neutrophils is unknown. The oligomer ratio of neutrophil-derived properdin could have functional consequences in local microenvironments where neutrophils are abundant and complement drives inflammation. We investigated the oligomer properties of neutrophil-derived properdin, as compared to that of normal human sera, using a novel ELISA-based method that detects function of properdin in a way that was proportional to the oligomeric size of properdin (i.e., the larger the oligomer, the higher the detected function). Unexpectedly, neutrophil-derived properdin had 5-fold lower function than donor-matched serum-derived properdin. The lower function was due to a lower percentage of tetramers/trimers and more dimers, indicating a significantly different P4:P3:P2 ratio in neutrophil-derived properdin (18:34:48) as compared to donor-matched serum (29:43:29). Release of lower-order oligomers by neutrophils may constitute a novel regulatory mechanism to control the rate of complement activation in cellular microenvironments. Further studies to determine the factors that affect properdin oligomerization and whether, or how, the predominant dimers in neutrophil-derived properdin, assimilate to the ~1:2:1 ratio found in serum are warranted.
1. Introduction
The complement system is an essential component of the immune system consisting of three pathways: classical, lectin, and alternative, which are each activated differently, and converge at the generation of C3b. The alternative pathway (AP) is a surveillance system that is always active, whereby C3 in blood spontaneously hydrolyzes to produce C3(H2O) (C3 tick-over mechanism) (1). C3(H2O) binds Factor B, which is then cleaved by mature Factor D to form a fluid-phase C3 convertase known as C3(H2O)Bb (1) that cleaves circulating C3 to C3b. C3b can bind covalently to hydroxyl or amino groups on nearby surfaces where it interacts with Factors B and D to form a surface-bound C3 convertase, C3bBb. C3 convertases cleave C3 to generate C3 fragments (C3a and C3b). It is also argued that the AP is primarily an amplification loop of C3b generated from the classical and lectin pathways with contributions from non-complement proteases (reviewed in (2, 3)). In order for the AP to carry out essential amplification of all three complement pathways, the C3 convertase of the AP is stabilized by a positive regulatory protein, properdin, increasing the half-life of the convertase by 5-10-fold (4, 5). Subsequent binding of C3b near C3 convertases (6) forms C5 convertases that cleave C5, leading to formation of C5a and the membrane-attack complex (C5b-9) (reviewed in (7)). The complement fragments that are generated during complement activation serve as potent pro-inflammatory mediators that are critical for opsonization, immune modulation, chemotaxis, and numerous other functions.
A fine balance between complement activation and regulation ensures the host is protected from danger such as infection, but not overwhelmed by excessive, harmful inflammation. Regulation of the AP by negative regulators, both fluid-phase (AP: Factor H, Factor H-like protein 1; terminal pathway: clusterin, vitronectin) and membrane-bound (AP: CRIg, CD35, CD46, CD55; terminal pathway: CD35, CD46, CD55, CD59), are essential for ensuring complement activity does not result in unintentional damage to the host. In contrast, in order to increase the effectiveness of the AP, properdin serves as an essential positive regulator by 1) stabilizing AP convertases, as mentioned above; 2) enhancing proconvertase (C3bB) formation (8–11); 3) competing with Factor I to prevent cleavage of C3b into the inactive form, iC3b (8, 12–14); and 4) serving as a pattern recognition molecule to initiate AP convertase formation de novo by recruiting C3b or C3(H2O), followed by Factors B and D (9); however, the relevance of this initiating phenomenon in vivo remains unknown. Properdin is an oligomeric glycoprotein, which forms dimers (P2), trimers (P3), and tetramers (P4) of head-to-tail, non-covalent associations of identical monomeric subunits. In healthy serum, these oligomers are present in a stable 20:54:26 ratio of P4, P3, and P2 (15, 16). Each properdin monomer contains 442 amino-acid residues arranged into six complete thrombospondin type 1 repeat (TSR1-6) domains and a N-terminal TGF-β binding (TB) domain (17–19). Properdin oligomers form ring-shaped vertices upon interaction of the TB domain and TSR1 from one monomer with TSR4, TSR5, and TSR6 from another monomer (8, 12, 14). These vertices interact with C3b and Bb of convertases (or Factor B of proconvertases) at a binding site formed by TSR5 and a large loop of TSR6 (8, 11, 12). Properdin oligomerization directly correlates with properdin convertase-stabilizing function (i.e., P4 are more functional than P3, and P3 are more functional than P2) (15, 20). Electron microscopy suggests each properdin vertex concurrently binds a convertase, thus delineating the association between oligomer function and its stoichiometry (14). Simultaneous interactions of oligomeric properdin with multiple C3b molecules on an activator cell surface where C3b convertases are forming remains to be determined.
Properdin is present in serum at a concentration of 4-25 μg/ml (15). Unlike most complement proteins which are generated in the liver, properdin is released primarily from activated leukocytes. Cells known to either release or synthesize properdin mRNA include granulocytes, macrophages, monocytes, dendritic cells, primary T cells, mast cells, adipocytes, and endothelial cells (reviewed in (21)). Neutrophils, the most abundant leukocyte, release properdin stored in secondary granules synthesized during bone marrow maturation (22) in response to a variety of inflammatory agonists. Although it is accepted that properdin is present in serum in an approximate, 1:2:1 ratio of P4:P3:P2, the molecular composition of properdin when released by leukocytes is unknown. At local sites of inflammation, where neutrophils are abundant and subject to cytokine stimulation, the distribution of oligomers of neutrophil-derived properdin may further modulate local complement activity, beyond the increased properdin concentration.
In this study, the ability of properdin to capture and generate convertases de novo was utilized to develop a novel ELISA-based technique to evaluate the function of properdin in biological samples with a readout that correlated with the oligomeric distribution of properdin in the sample. For the first time, the oligomeric distribution of properdin released from neutrophils was characterized using this assay and confirmed by size exclusion chromatography. The novel assay revealed that the function of neutrophil-derived properdin was significantly lower than donor-matched serum properdin and the decreased function was confirmed to be due to the presence of predominantly P2 and P3.
2. Material and methods
2.1. Buffers
The following buffers were used: Gelatin veronal buffer (GVB=; 5 mM veronal, 145 mM NaCl, 0.004% NaN3, 0.1% gelatin, pH 7.3), MgEGTA [0.1 M MgCl2 and 0.1 M EGTA (ethylene glycol tetraacetic acid), pH 7.4], 0.5 M EDTA (0.25 M EDTA [Ethylenediaminetetraacetic acid] disodium salt-2H2O and 0.25 M EDTA tetrasodium salt-2H2O, pH 7.4), GVBE (GVB=, 10 mM EDTA), phosphate buffered saline (PBS; 10 mM NaH2PO4, 145 mM NaCl, pH 7.4), Hanks’ balanced salt solution with Ca2+ and Mg2+ (HBSS++; Gibco), and ELISA dilution buffer (PBS + 0.05% tween + 1% bovine serum albumin (BSA)).
2.2. Serum and complement proteins
Normal human serum (NHS) was purchased from Innovative Research or produced by our laboratory using healthy donors. The Institutional Review Board from the University of Toledo College of Medicine and Life Sciences approved the protocols, and written informed consent was obtained from all donors, in accordance with the Declaration of Helsinki. Properdin-depleted and C3-depleted serum were purchased from Complement Technology Inc. Unfractionated pure properdin was purified from normal human plasma as described previously (23). Rabbit erythrocytes (ER) were prepared from blood obtained from Rockland Immunochemicals.
2.3. Antibodies
The following antibodies were used: mouse monoclonal immunoglobulin G (IgG)1 anti-properdin 3A3E1 (biotin-labeled) and 6E9E6 (unlabeled) antibodies developed by our laboratory as previously described (24) and biotinylated mouse IgG1 anti-human C3/C3b/iC3b monoclonal (clone 7C12) (Cedarlane).
2.4. Human neutrophil cell isolation and activation
Human whole blood was collected via venipuncture from healthy donors. The Institutional Review Board from the University of Toledo College of Medicine and Life Sciences approved the protocols, and written informed consent was obtained from all donors, in accordance with the Declaration of Helsinki. Blood was drawn into BD vacutainer tubes containing 12 mg K3 (tripotassium) EDTA and polymorphonuclear (PMN) cells were isolated by using a Polymorphprep™ gradient solution (Axis Shield) following manufacturer’s instructions. PMN cells (5.0 x 107 cells/ml) in 0.2% BSA/HBSS++ buffer, were activated using 20 ng/ml of phorbol 12-myristate 13-acetate (PMA) (Enzo Life Science) for 30 min at 37°C. The PMNs were centrifuged at 600 x g for 10 min. The supernatant was removed and centrifuged at 13,000 g for 2 min to remove cell debris. PMNs without PMA treatment were prepared as a control. The supernatants from PMA-activated and non-activated PMNs were assayed for properdin concentration, as indicated below.
2.5. Sandwich ELISA to quantify properdin concentration
Properdin concentration of test samples was assayed by a sandwich ELISA as previously reported by our laboratory (25). The samples (i.e., fractions collected from: pure properdin, NHS, neutrophil supernatant, and donor-matched serum) were tested at varying dilutions in ELISA dilution buffer, as indicated in the figure legends. Other samples tested, but not shown, include neutrophil supernatant samples (diluted 1/80, 1/160, and 1/320), donor-matched serum, NHS, and C3-depleted serum (diluted 1/800, 1/1600, 1/3200, and 1/6400).
2.6. Separation of properdin oligomers from native properdin and biological samples
Physiological oligomeric forms of properdin P2, P3, P4, and non-physiological aggregated properdin (Pn) were isolated from purified properdin by size exclusion chromatography as previously reported with minor modifications (23). Briefly, 200 μg purified properdin in a total volume of 400 μl in PBS was loaded onto a Phenomenex BioSep 5 μM SEC s4000 500Å liquid chromatography column (600 x 7.8 mm) with guard column (75 x 7.8 mm) and was eluted at a flow rate of 0.3 ml/min in PBS. Fractions were collected in 250 μl volumes in glass tubes (12 x 75 mm) that were previously blocked with 500 μl GVB= overnight at 4°C and then emptied prior to sample collection. Collected fractions were stored at 4°C and used within one week of separation. Similarly, physiological oligomeric forms of properdin were separated from NHS and neutrophil supernatant by size exclusion chromatography by diluting the sample to contain 4 μg or 150 ng properdin (properdin concentration determined by sandwich ELISA) with 1% BSA and 20 mM EDTA in PBS, and syringe-filtered using 0.20 μM regenerated cellulose filter membranes (Phenomenex). Samples (400 μl final volume) were loaded onto a size chromatography column as described for purified properdin. For all samples that were run through the size exclusion column, the concentration of properdin within each fraction was determined using the sandwich ELISA described in section 2.5. A visual representation of the ratio of properdin oligomers in the sample was constructed by graphing the properdin concentration of each fraction. Peak fractions and no more than 3 fractions to the left or right of the peak fraction were selected for further analyses (i.e., properdin functional ELISA, hemolytic assay) of purified oligomers.
2.7. Quantification of properdin oligomeric ratios in biological specimens
A systematic approach for determining the fraction boundaries of the properdin oligomer forms within each sample was applied using the plotted concentration data (method described in section 2.5) for each fraction. Figure 1 shows properdin concentration in each fraction derived from size exclusion chromatography of NHS (from donor #4). The width of the P4 peak was considered five fractions to the left of the P4 top fraction and all fractions to the right of the top fraction up until the fraction present in the valley between the P4 and P3. The fraction present at the valley between peaks was assigned to both P4 and P3 by dividing the concentration of that fraction equally between the two properdin oligomers. The width of the P2 peak was considered five fractions to the right of the P2 top fraction and all fractions to the left up until the valley between the P2 and P3 peaks. The fraction present at the valley between these peaks was assigned to both P2 and P3 by dividing the concentration of that fraction equally between the two properdin oligomers. The width of the P3 peak was defined as all fractions in between the P4/P3 valley and the P3/P2 valley. After assigning fractions to properdin oligomers, the concentration from fractions belonging to each properdin oligomer form was totaled. Then, all fractions, from all oligomers were summed for a grand total. The ratio of properdin oligomers was determined by dividing the total concentration of each properdin oligomer by the grand total and multiplying by 100. Comparable results were obtained when fitting the concentration data described in Figure 1 to three peaks using computer-generated curves applying Fityk software (26) assuming Gaussian distributions for each species (data not shown).
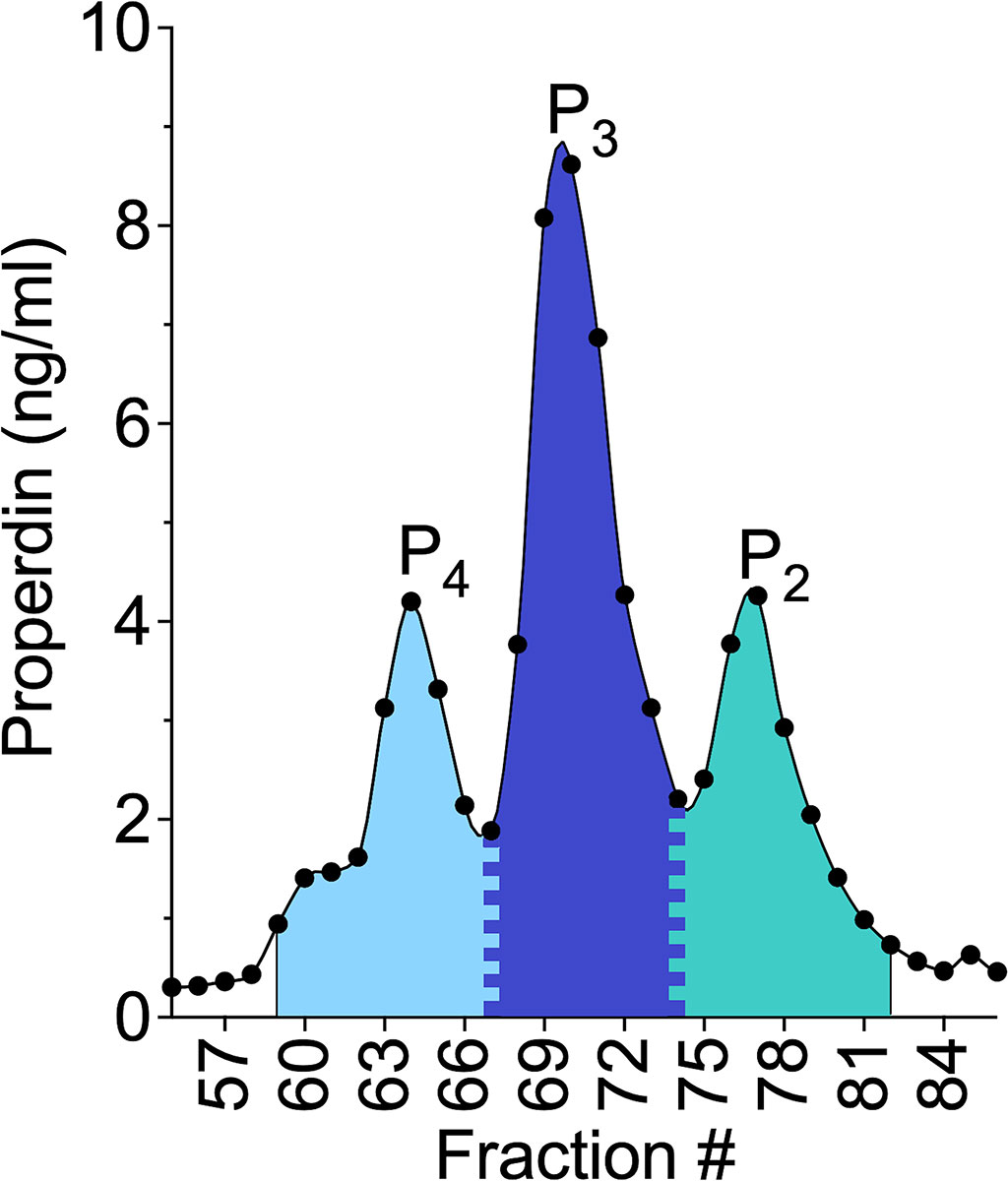
Figure 1 Visual representation of the method for determining the ratio of properdin oligomers using the properdin sandwich ELISA data from the fractions collected after performing size exclusion chromatography on NHS, from donor #4 as described in “Materials and Methods” (section 2.7);. The width of the P4 peak was considered five fractions to the left of the P4 top fraction and all fractions to the right of the top fraction up until the fraction present in the valley between the P4 and P3. The fraction present at the valley between peaks was assigned to both P4 and P3 by dividing the concentration of that fraction equally between the two properdin oligomers. The width of the dimer peak was considered five fractions to the right of the P2 top fraction and all fractions to the left up until the valley between the P2 and P3 peaks. The fraction present at the valley between these peaks was assigned to both P2 and P3 by dividing the concentration of that fraction equally between the two properdin oligomers. The width of the P3 peak was defined as all fractions in between the P4/P3 valley and the P3/P2 valley. After assigning fractions to properdin oligomers, the concentration from fractions belonging to each properdin oligomer form was totaled. Then, all fractions, from all oligomers were summed for a grand total. The ratio of properdin oligomers was determined by dividing the total concentration of each properdin oligomer by the grand total and multiplying by 100.
2.8. Development of an ELISA-based assay to assess properdin function
The following assay parameters were varied and overall optimal conditions allowing the greatest signal to noise ratio were chosen (data not shown): concentration of sensitizing anti-properdin monoclonal antibody 6E9E6, properdin concentration in the sample, concentration of properdin-depleted sera, properdin function reaction time, concentration of anti-C3b monoclonal antibody, dilution of horseradish peroxidase-streptavidin, and absorbance read time. Two versions of the assay that result in equivalent outcomes (using either medium-binding full-area or high-binding half-area ELISA plates) were standardized and carried out as described below.
2.8.1. Properdin functional ELISA to assess properdin function in purified properdin and in biological samples
A 96-well, high-binding, half-area, ELISA plate (Greiner Bio One) was coated with 50 μl/well of 1 μg/ml non-inhibitory anti-properdin monoclonal antibody 6E9E6 diluted in PBS overnight at 4°C. The plate was washed 4x with 130 μl/well 1x PBS containing 0.05% Tween-20 (PBST) and blocked for 2 h at 37°C with 125 μl/well 3% BSA in PBS. Properdin sources were diluted to equivalent concentrations within each assay (ng/ml; indicated in figures) in ELISA dilution buffer and 20 mM EDTA then added to the plate and incubated at 37°C for 1 h for properdin capture by the coating antibody, 6E9E6. Background was determined by adding properdin-depleted serum (for serum), PBS (for pure properdin), or 0.2% BSA with HBSS++ (for neutrophil supernatant), instead of a properdin source. The plate was washed 4x with 130 μl/well PBST and while on ice, 50 μl/well properdin-depleted serum diluted 1/10 in GVB= with 5 mM MgEGTA was added to the plate while cold water was added to empty wells not containing any sample to ensure even temperature distribution across the plate. The plate was incubated for 45 min at 37°C. 12.5 μl/well cold 0.1 M EDTA was added to all wells to stop complement activity, and the plate was washed 4x with 130 μl/well PBST. C3b deposited covalently on the plate was detected with 50 μl/well biotinylated anti-C3b monoclonal antibody at 200 ng/ml for 1 h at 37°C. The plate was washed 4x with PBST and incubated for 45 min at 37°C with horseradish peroxidase-streptavidin (Cedarlane) diluted 1:2500 in ELISA dilution buffer. The plate was washed 4x with 130 μl/well PBST and incubated for 30 min with 10-parts ABTS to 1-part ABTS enhancer at room temperature and absorbance was read at 405 nM on a Tecan Infinite M200 spectrophotometer. During each incubation, the plate was sealed with a plastic film. Data was normalized by dividing C3b deposition of each sample by C3b deposition of an internal plate control, NHS.
2.8.2. Properdin functional ELISA to assess properdin function in C3-depleted serum, and neutrophil supernatant and serum matched samples
This assay used full-area, medium-binding plates (Greiner Bio One), resulting in differences in some of the ELISA parameters as compared to section 2.8.1 as follows: (a) all volumes at all steps were doubled; (b) the plates were coated with 10 ug/ml of the 6E9E6 antibody; (c) the following sources of properdin were used: C3-depleted serum, NHS, neutrophil supernatant, or donor-matched serum; (d) properdin-depleted serum was used at 1/10-1/16 dilution and the incubation that followed was 90 min; (e) the biotinylated anti-C3b antibody was used at 20 ng/ml; and (f) the horseradish peroxidase-streptavidin was diluted 1/5000.
2.9. Properdin functional hemolytic assay to assess properdin function in purified properdin oligomers and in biological samples
2 x 107 ER were mixed with GVB= and the following reagents at the indicated final concentrations: 2.5 mM MgEGTA or 10 mM EDTA, 0-60 ng/ml of properdin from NHS, pure properdin, or properdin oligomers from pure properdin (P2, P3, and P4 and Pn), and 20% properdin depleted serum in a total 50 μl volume. Next, the mix was incubated for 10 min at 37°C, mixing the tubes every 5 min. The tubes were then placed on ice and 200 μl of cold GVBE was added to each tube to stop the reaction. The tubes were spun at 2000 g for 3 min at 4°C. The absorbance of 200 μl of each supernatant was measured in a microtiter plate at 414 nM. The % of hemolysis was calculated using the formula: [(A414-background A414 in the presence of EDTA)/(maximum A414 determined by water lysis-background A414 in the presence of EDTA)] x 100.
2.10. Statistical analyses
Data was analyzed by unpaired t-test, one-way ANOVA with Tukey’s post-test, or two-way ANOVA with Bonferroni’s post-test using GraphPad Prism version 9.2.0 for Mac OS X (GraphPad Software, San Diego, California, USA), or by ANCOVA analysis followed by Tukey’s post hoc test using SAS 9.4 (SAS Institute Inc., Cary, NC, USA) (as indicated in figure legends or results section). P values less than 0.05 were considered significant.
3. Results
3.1. Development of an ELISA-based method to characterize properdin function in biological samples
Given that properdin oligomer distribution influences overall properdin function (P4 have greater function than P3, and P3 have greater function than P2), an assay that could detect functional differences in biological samples was developed. This was accomplished using a multiplexed (96-well format), ELISA-based approach (Figure 2). In order to measure the function of properdin captured from a biological source, a non-inhibitory, anti-properdin monoclonal antibody (6E9E6) was used as a capture antibody. In addition, to ensure that equivalent amounts of properdin (ng/ml) could be captured, irrespective of the oligomerization state, 6E9E6 was used because it did not detect differences between P2, P3, and, P4 (24). Before the next step, the concentration of properdin in the samples was determined using the sandwich ELISA described in the “Materials and Methods” (section 2.5). The next step of the functional properdin ELISA consisted in adding to the 6E9E6-sensitized wells, equivalent concentrations of properdin within biological samples (i.e., serum, neutrophil supernatant) or pure properdin, in the presence of EDTA (to prevent complement activation). After properdin capture, the plate was washed, so that only captured properdin remained, and incubated with properdin-depleted serum with MgEGTA (to facilitate AP activity only). Using properdin-depleted serum as a source of complement proteins for de novo convertase formation on the captured properdin, ensured properdin activity was only contributed by the captured properdin. The efficiency of properdin to initiate AP activity (i.e., properdin function) was determined by the level of C3b covalently bound to the BSA coating the plate, which was detected by an anti-C3b monoclonal antibody. The inter- and intra-assay coefficient of variation (CV) for the assay format described in “Materials and Methods” section 2.8.1, was 19.6% and 5.3%, respectively. For the format described in section 2.8.2, the inter-assay and intra-assay CV was 9.7% and 6.5%, respectively. Additional validation is described below.
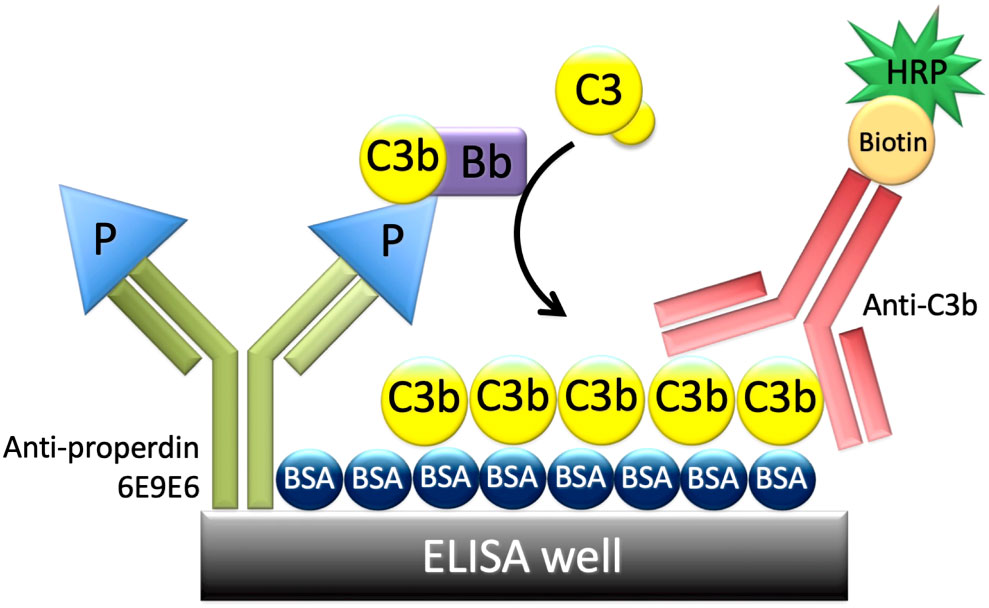
Figure 2 Principle of the properdin functional ELISA. An ELISA plate was coated with a non-inhibitory anti-properdin monoclonal antibody 6E9E6 (in green), blocked with BSA, and then properdin was captured from various sources (purified properdin, serum, neutrophil supernatant). The plate was washed, and properdin-depleted serum was added as a source of complement proteins including C3b, C3(H2O), Factor B, and Factor D. C3b or C3(H2O) and Factor B bind to properdin to form C3 convertases, C3bBb, or C3(H2O)Bb. Convertases cleave C3 and C3b is deposited covalently on BSA and detected with biotin-labeled anti-C3b (in red) followed by horseradish peroxidase-streptavidin, resulting in a colorimetric reaction.
3.1.1. The properdin functional ELISA can detect differences between properdin oligomers
The functional ELISA was validated by evaluating the ability of different captured properdin oligomers to promote C3b deposition. For this purpose, size exclusion chromatography was used to separate physiological oligomeric forms (P2, P3, and P4) and non-physiological aggregates (Pn) from unfractionated pure properdin. Physiological oligomers in the context of NHS were also separated. After fractionating the samples containing properdin, the concentration of properdin in the collected fractions was determined by sandwich ELISA and the resulting concentrations were plotted to visualize the oligomer distribution in the sample for purified properdin (Figure 3A) and NHS (Figure 3B). Both properdin sources showed P4, P3, and P2 peaks at similar ratios (i.e., ~18:57:25 for unfractionated pure properdin and ~20:52:27 for NHS) and the unfractionated pure properdin contained a small Pn peak.
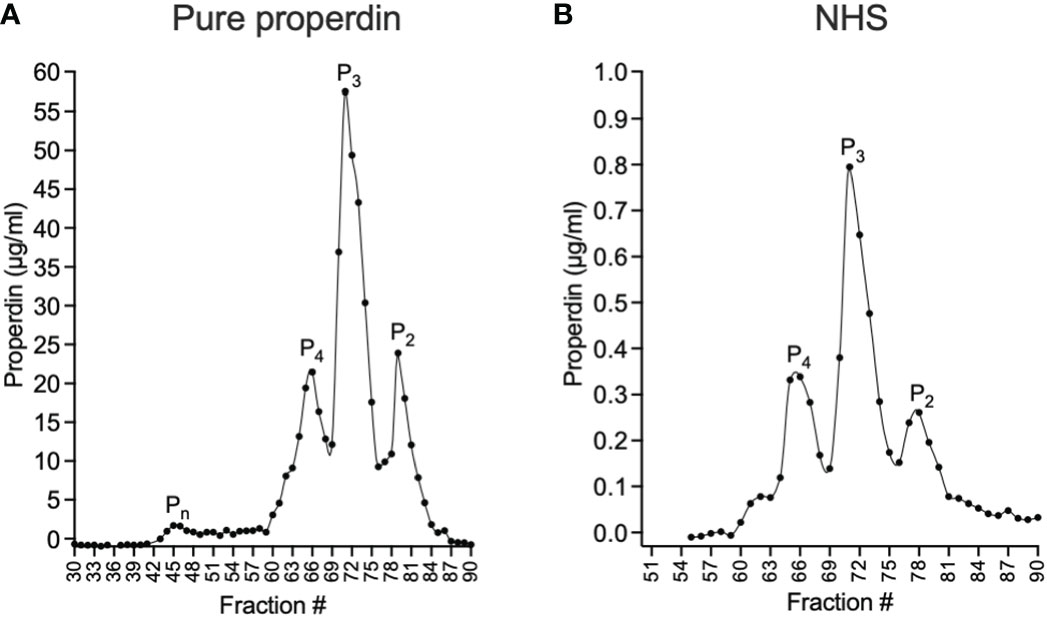
Figure 3 Separation of properdin forms in purified properdin and NHS by size exclusion chromatography and quantification of properdin in the fractions by sandwich ELISA. Properdin from a pure, unfractionated properdin preparation (A) or in NHS (B) was separated into tetramers (P4), trimers (P3), dimers (P2) and non-physiological aggregates (Pn) by size exclusion chromatography and the concentration of properdin in the collected fractions (diluted 1:3200 (A) and 1:100 (B)) was determined by sandwich ELISA as described in “Materials and Methods” (section 2.5 and 2.6) and graphed.
The function in the selected chromatography fractions of each separated properdin oligomers were assayed at equivalent concentrations in the functional ELISA as described in “Materials and Methods” (section 2.8.1). The assay detected significant differences in function between individual properdin oligomers separated from both pure properdin and serum. P2 from purified properdin were 30% and 53% as active as P4 and P3 from purified properdin, respectively. In the case of oligomers separated from serum, P2 were 37% and 64% as active as P4 and P3, respectively. P3 from pure properdin and serum were 58% as active as P4 from pure properdin and serum. The function of properdin in NHS was closest to pure P3 and physiological P2/P3, as expected given NHS lacks the Pn forms (15, 27). Pn, which are the highly aggregated, non-physiological oligomers of properdin, showed the highest function, and unfractionated pure properdin, which contains Pn and the physiological oligomers, resulted in the second highest function (Figures 4A, B).
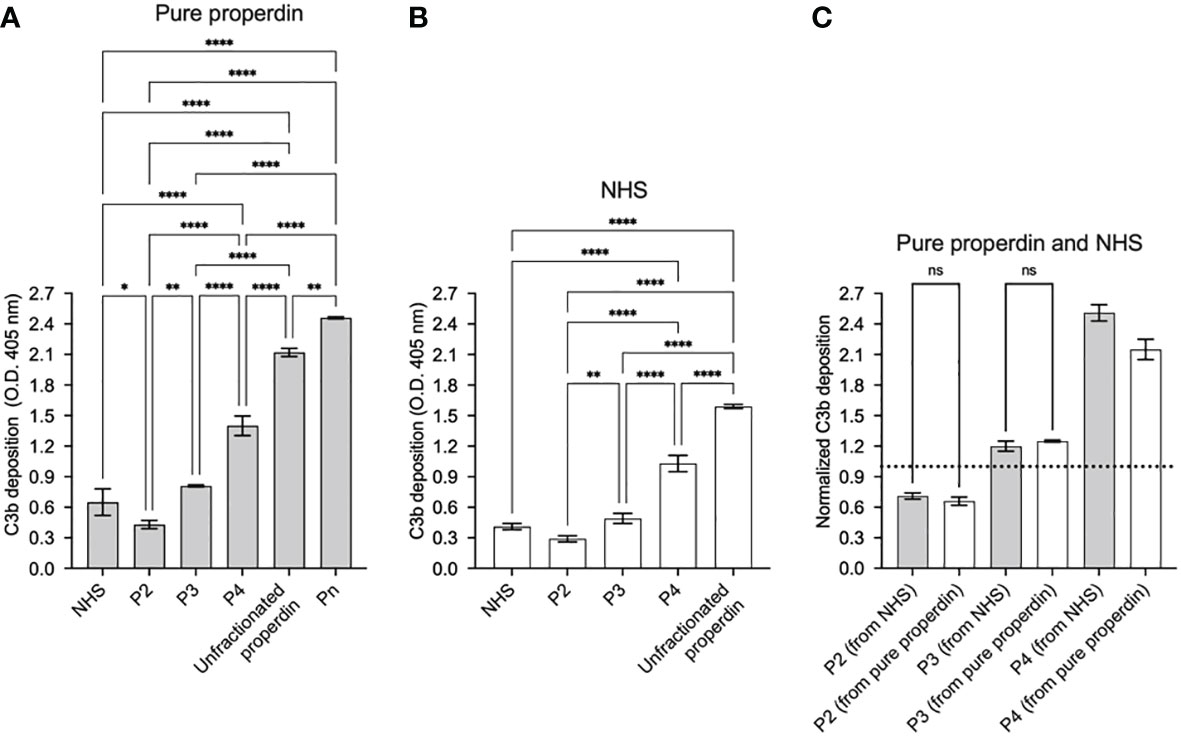
Figure 4 Properdin functional ELISA can distinguish function between properdin oligomers derived from pure properdin and serum. Properdin function was quantified by the properdin functional ELISA where the readout was C3b deposition as described in “Materials and Methods” (section 2.8.1). Data is graphed minus background of samples containing no properdin during the properdin capture step. All samples were tested at equivalent concentrations (200 ng/ml). Properdin function was quantified in properdin oligomer samples (Pn, P4, P3, and P2) that were separated from pure properdin (A) and in P4, P3, and P2 from NHS (B). Both (A) and (B) include assessment of function of unfractionated NHS and unfractionated pure properdin as controls. The data is a representative of 3 experiments and is graphed as mean and standard deviation of triplicates. (C) C3b deposition normalized to an internal plate control (NHS, dotted line) for P2, P3, and P4 derived from pure properdin (as shown in A) and NHS (as shown in B). Significant differences in C3b deposition between samples was assessed by one-way ANOVA with Tukey’s multiple comparison test; p<0.0001 ****, p<0.01 **, p<0.05 *, p≥ 0.05 non-significant (only shown for panel C).
The utility of the functional ELISA to detect dose-dependent functional differences between unfractionated pure properdin that has P2, P3, P4, and aggregated Pn forms, and serum-derived properdin that lacks Pn forms was also tested. The function of 16 concentrations (0-500 ng/ml) of unfractionated pure properdin and properdin within serum was assessed. This resulted in a concentration-dependent increase in both serum-derived and unfractionated pure properdin with properdin in serum having lower function at the tested doses (Figure 5), indicating that the properdin functional assay detected dose-dependent increases in properdin function and wide functional differences between samples with distinct oligomeric composition.
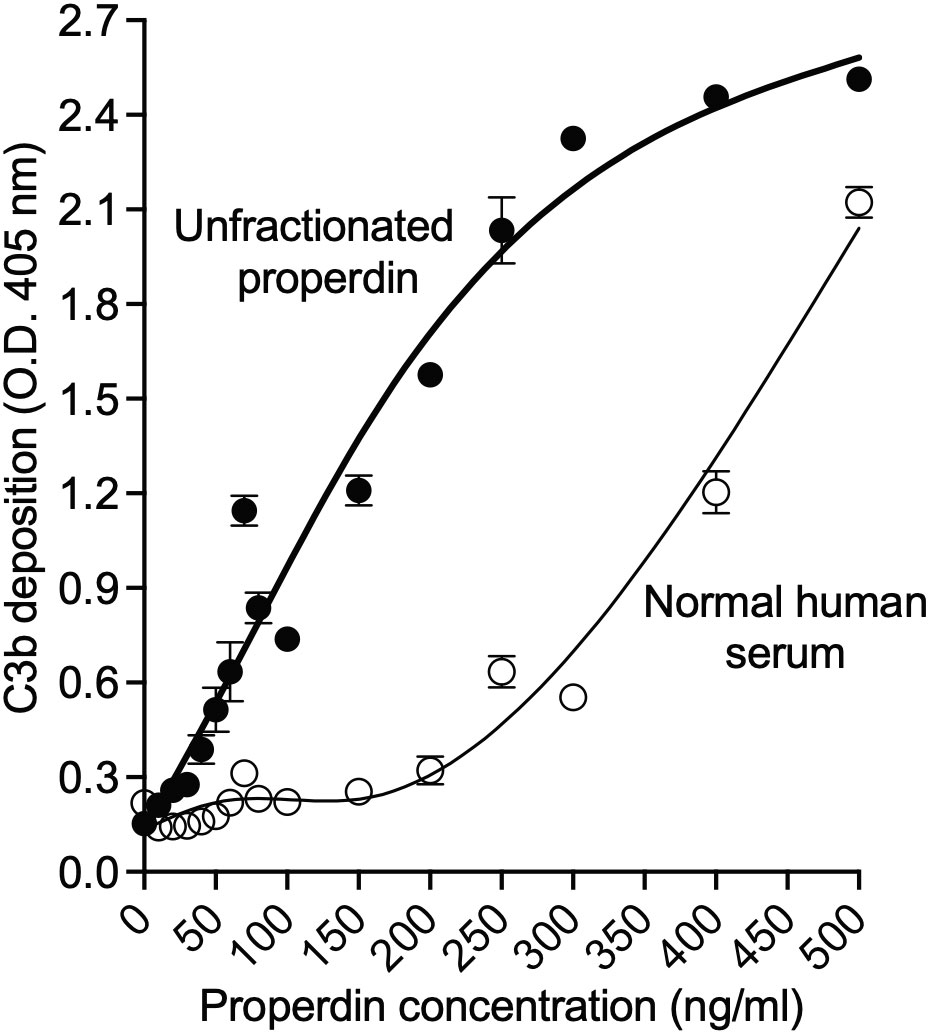
Figure 5 Properdin functional assay detected dose-dependent increases in properdin function and wide functional differences between samples with distinct oligomeric composition. Properdin concentration of unfractionated pure properdin and of properdin in the context of NHS was determined by sandwich ELISA as described in “Materials and Methods” (section 2.5), followed by assessment of function in the samples using the properdin functional ELISA as described in Materials and Methods (section 2.8.1). Unfractionated pure properdin and properdin in NHS were assessed at varying properdin concentrations, as indicated in the x-axis. Data was graphed as mean and standard deviation of triplicates from one experiment.
3.1.2. The hemolytic properdin functional assay does not detect greater properdin function when higher order properdin oligomers are present
To determine if another assay, in addition to the functional ELISA, can detect functional differences in samples with distinct oligomer composition, we also evaluated the function of unfractionated pure properdin, properdin in NHS, and properdin in individual properdin oligomers (P2, P3, P4, and Pn) in a properdin functional hemolytic assay using ER, which are susceptible to AP lysis (Figure 6). Using ANCOVA analysis with Tukey’s post hoc test, the data indicate that although NHS has similar function as P2 at all concentrations tested, no differences were observed between unfractionated pure properdin (that contains contaminating Pn) and P3, and P4 had the same activity as P3. In addition, Pn resulted in the lowest hemolysis, most likely due to consumption of complement in the serum, as described previously for high order properdin oligomers (15). Overall, the data indicates that under the conditions tested, the hemolytic assay cannot detect oligomer-dependent differences in properdin function.
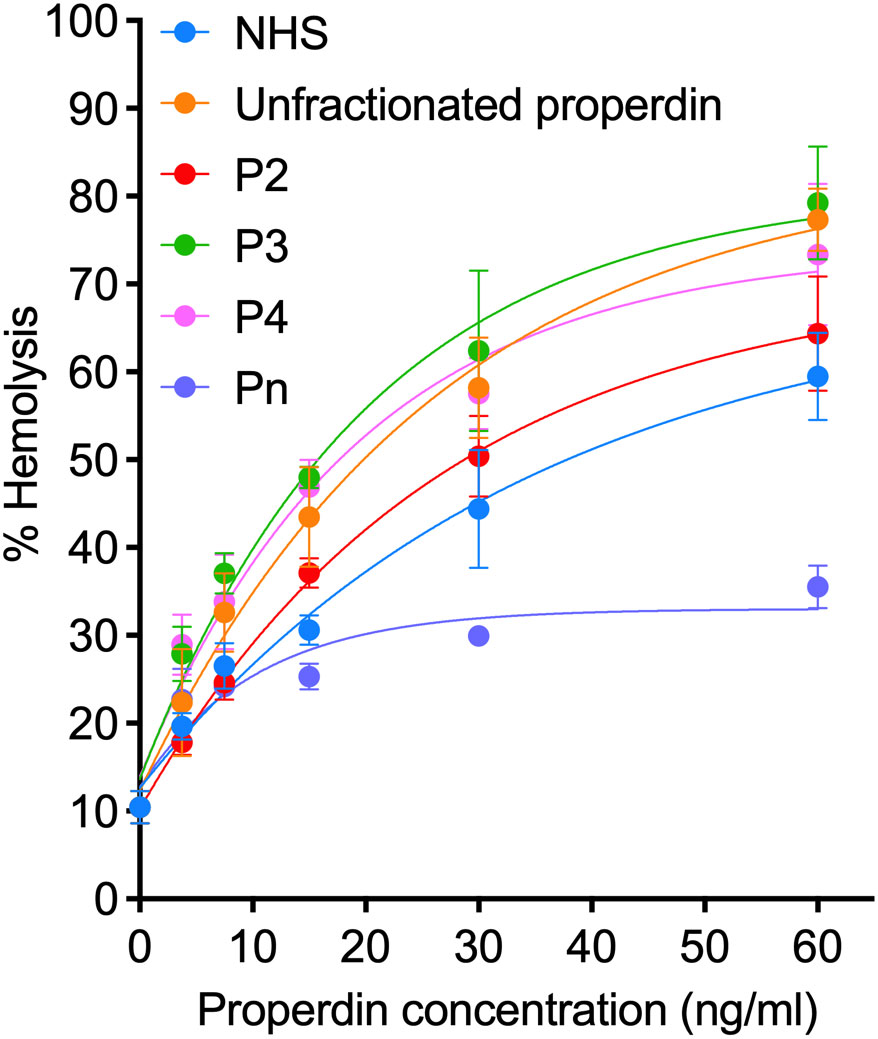
Figure 6 Properdin functional hemolytic assay does not detect greater function in higher order oligomers. Properdin function was quantified in unfractionated pure properdin and NHS, along with properdin oligomers separated from pure properdin (Pn, P4, P3, and P2), using a properdin functional hemolytic assay where % hemolysis is the read out as described in “Materials and Methods” (section 2.9). Data was graphed as mean and standard deviation of two independent experiments with duplicates.
3.1.3. Properdin functional ELISA does not capture serum-derived properdin in combination with C3(H2O) or C3b
The readout of the functional ELISA is the deposition of C3b generated by C3 convertases initiated by properdin. A previous report described that properdin can be found complexed to certain proteins in serum, such as C3(H2O), C3 fragments, clusterin, IgG, and C3b-C3b-IgG complexes (28). In order to rule out interference from C3b deposition being potentially contributed by C3(H20) or C3b associated with properdin during the step when properdin was captured from serum, we compared the function of properdin within NHS to properdin captured from C3-depleted serum using the functional ELISA as described in the “Materials and Methods” (section 2.8.2). The assay detected no differences in function between serum-derived properdin and properdin derived from C3-depleted serum at the tested concentrations of properdin (Figure 7), indicating that if any C3(H2O) or C3b was being captured with the properdin it does not interfere in the assay itself.
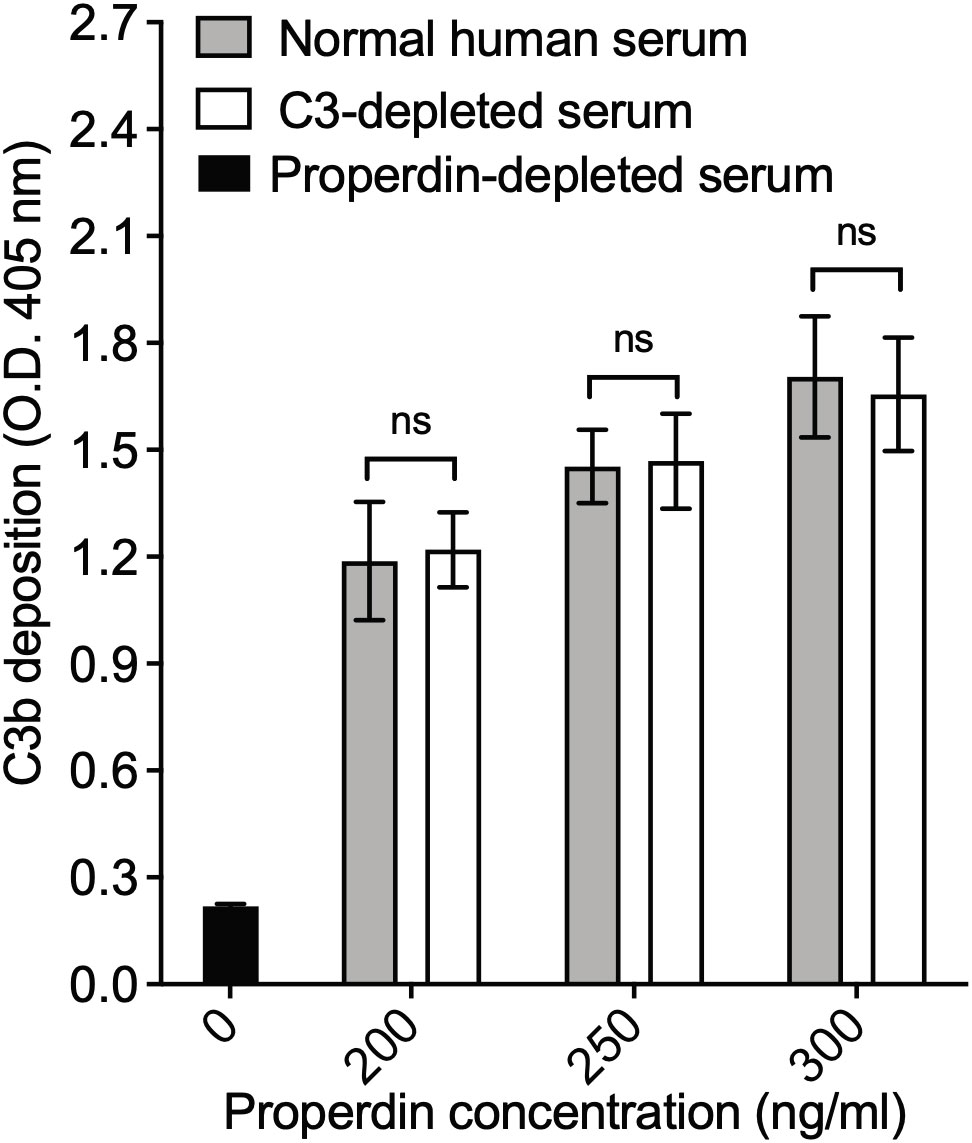
Figure 7 Properdin functional ELISA does not capture properdin in combination with C3 components. Properdin function was quantified by the properdin functional ELISA where the readout is C3b deposition as described in “Materials and Methods” section 2.8.2. Data is graphed minus background of samples containing no properdin during the properdin capture step. All samples were tested at equivalent concentrations (200-300 ng/ml). Results are shown as mean and standard deviation of two independent experiments with triplicates. Significant differences in C3b deposition between samples was assessed by two-way ANOVA with Bonferroni post hoc test where p≥ 0.05 non-significant (ns).
3.2. Assessment of neutrophil-derived properdin oligomeric distribution
3.2.1. Human neutrophil-derived properdin has lower function than properdin in donor-matched serum as detected by the properdin functional ELISA
The ability of the functional ELISA to detect functional differences between samples that contain different oligomer composition (e.g., between NHS and pure properdin or individual oligomers), suggested that the assay can serve as a first indication of potential differences in distribution of properdin oligomers within a sample. Thus, the assay was used to screen the function of properdin within activated neutrophil supernatants isolated from the blood of heathy human volunteers. Neutrophils release properdin upon activation and are an important source of serum properdin (29). Activated neutrophils release properdin stored within secondary granules (22) into the local, cellular microenvironments, which may contribute to the overall inflammatory response within these environments. However, the distribution of neutrophil-derived properdin oligomers (which would correlate to properdin function) is unknown. To address this gap, the function between serum-derived and neutrophil-derived properdin was assessed. To carry out a comparison at the individual level, serum and neutrophils from the same donor were collected. The neutrophils were activated using PMA, the cellular supernatants were collected, and the concentration of properdin in the supernatants was determined by using the sandwich ELISA as described in the “Materials and Methods” (section 2.5). The concentration of properdin in serum was between 8-19 μg/ml and 649-923 ng/ml in activated neutrophil supernatant. On average, the concentration of properdin released from the cellular supernatant of non-activated neutrophils was 170 +/- 128 ng/ml (data not shown). The function of properdin in serum and in the neutrophil supernatants were next assessed at equivalent properdin concentrations in the functional ELISA as described in the “Materials and Methods” (section 2.8.2). The function for neutrophil-derived properdin was significantly lower (an average 5-fold lower) than serum-derived properdin (Figure 8). Given that properdin function quantified in the functional ELISA was a property of oligomerization (Figure 4), we next assessed whether the lower function associated with neutrophil-derived properdin was due to an alteration in the ratio of properdin oligomers distinct from the approximate 1:2:1 ratio reported for serum-derived properdin.
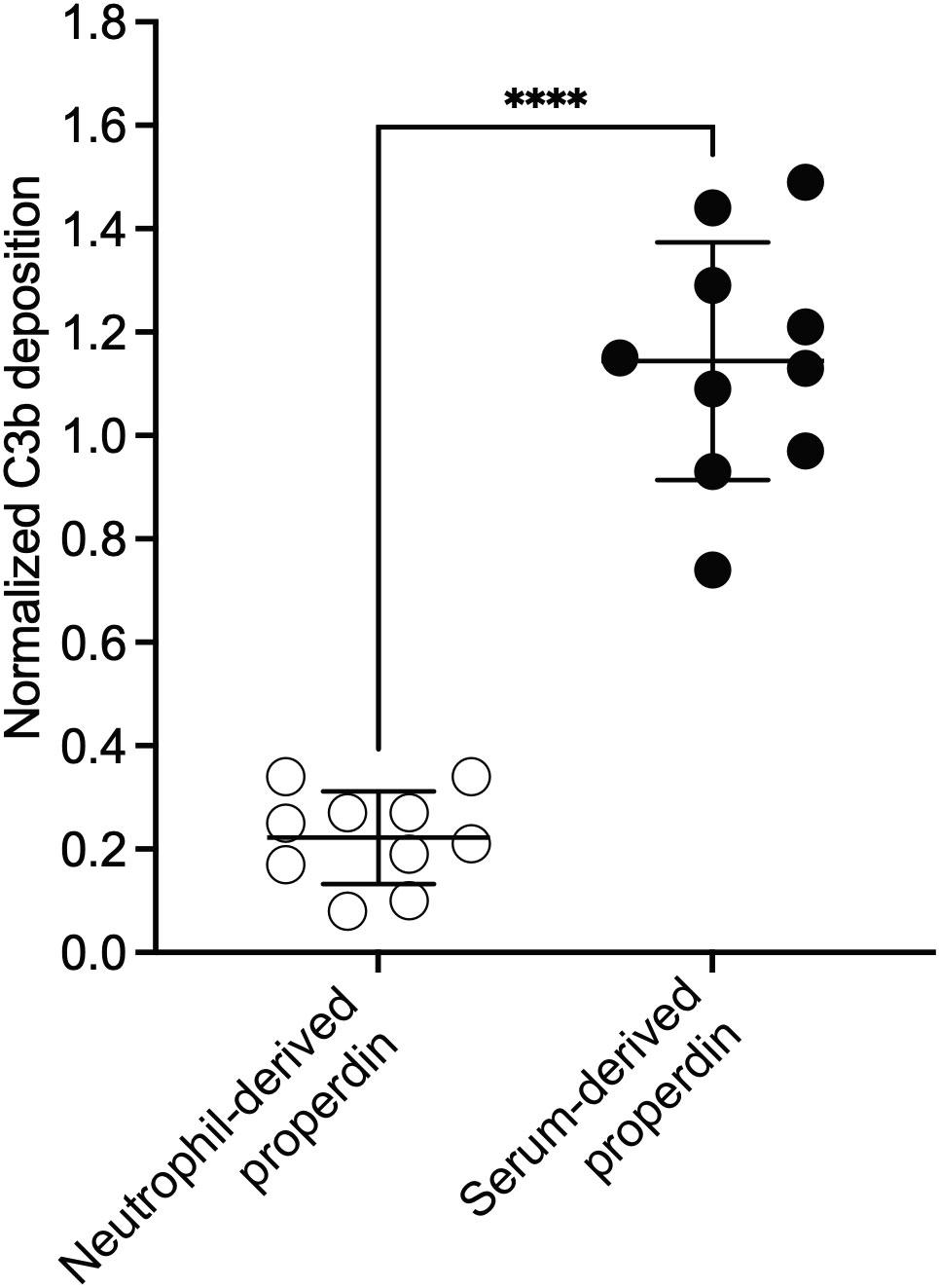
Figure 8 Neutrophil-derived properdin had significantly lower function than serum-derived properdin, when assessed in the properdin functional ELISA, at equivalent concentrations. Properdin function was quantified by properdin functional ELISA where the readout was C3b deposition as described in “Materials and Methods” (section 2.8.2). Data is graphed minus background of samples containing no properdin during the properdin capture step. Properdin function in phorbol-12-myristate-13-acetate (PMA) activated neutrophil supernatant and serum collected from 10 healthy donors was quantified. All samples were tested at equivalent concentrations (300 ng/ml). Results are shown as mean and standard deviation from two independent experiments with triplicates. Significant differences in C3b deposition between samples was assessed by unpaired t test; p< 0.0001****.
3.2.2. The function of neutrophil-derived properdin was due to a properdin oligomer ratio distribution that was different from serum, skewed towards dimers
To address whether the lower function of neutrophil-derived properdin, as compared to serum, was due to differences in oligomer distribution, size exclusion chromatography coupled with quantification of properdin of the fractions were performed and oligomeric ratios for these samples were calculated as described in the “Materials and Methods” (section 2.7). The ratio of properdin oligomers derived from serum (Figure 9A) was an expected ratio of 29:43:29 (P4:P3:P2). The ratio of properdin oligomers in activated neutrophil supernatants from 5 donors (Figures 9B–F) was characterized by a lower amount of P4/P3 and higher amount of P2 resulting in oligomeric ratios of 19:33:48, 23:37:39, 19:31:50, 15:36:49, and 16:31:53 (average 18:34:48). Overall, the data indicate that the ratio of properdin oligomers released from PMA-activated neutrophils was different from NHS and favors lower-order forms.
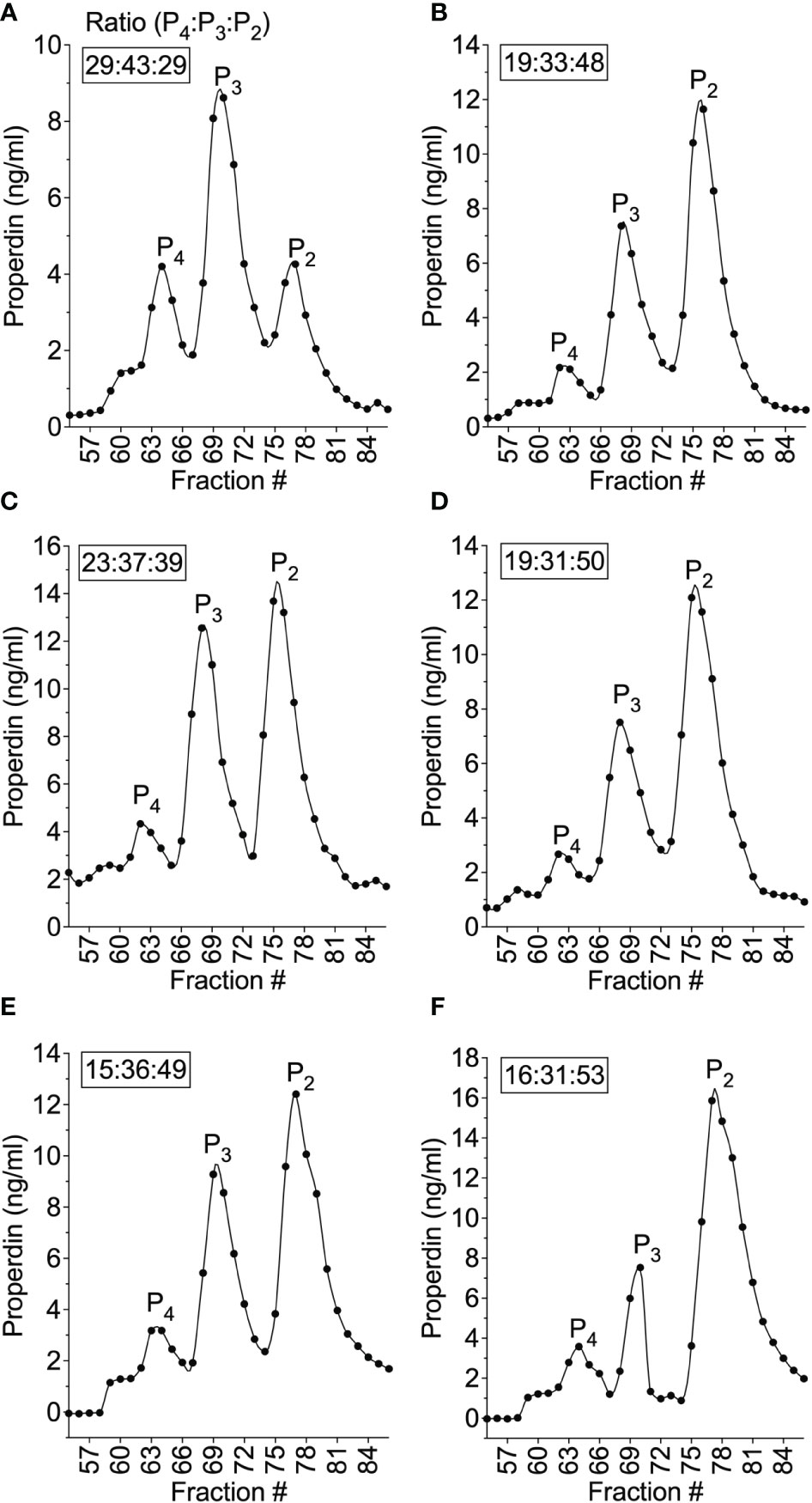
Figure 9 Properdin oligomeric distribution differed between neutrophil-derived and serum-derived properdin and was skewed towards dimers. The ratios of properdin oligomers in serum from donor #4 (A) and PMA-activated neutrophil supernatant from donor #4 (B), and PMA-activated neutrophil supernatant from donor #6 (C), #7 (D), #8 (E), #9 (F) were determined by size exclusion chromatography and properdin concentration by sandwich ELISA as described in “Materials and Methods” (section 2.5 and 2.6) and the data was graphed by properdin concentration. The ratios of properdin oligomers were calculated as described in “Materials and Methods” (section 2.7). and indicated as an inset to each graph.
4. Discussion
Neutrophils account for a significant source (~19-32%) of circulating properdin (29). It is likely that neutrophils are major contributors of properdin in inflammatory microenvironments, because they are the first cells recruited to sites of infection where they facilitate the clearance of microorganisms via phagocytosis, the release of cytotoxic molecules from secondary granules, and the formation of neutrophil extracellular traps (NETs) (reviewed in (30, 31)). Neutrophils also participate in adaptive immune regulation through interactions with antigen-presenting cells and lymphocytes (reviewed in (32)). Neutrophils secrete properdin stored within granules (22) in response to a variety of inflammatory agonists. Properdin carries out a series of essential canonical and non-canonical functions including promotion of: oxidative burst in neutrophils (33), complement activation on NETs (34, 35), complement-mediated platelet-granulocyte formation (20) and also can serve as a ligand for natural killer cells, leading to antimicrobial outcomes independent of complement activation (36). Properdin released from neutrophils can bind activated platelets (37) and apoptotic T-cells (38). Any of these functions may affect inflammatory states both in circulation and especially in cellular microenvironments. Because the magnitude of the function of properdin was directly related to the ratio of properdin oligomers (Figure 4), identifying the oligomerization state of properdin when it is released from neutrophils becomes essential, yet remained unknown. In this study, we developed an ELISA-based assay that can measure the function of properdin, dependent on the oligomerization state of properdin in the sample, which allowed us to address this question.
Properdin is known to be essential for stabilizing AP convertases; however, properdin has been shown, via surface plasmon resonance, to also serve as a focal point for de novo convertase assembly in vitro (9). This convertase-initiating property was used to develop the properdin functional assay. The assay relied on a non-inhibitory, monoclonal, anti-human properdin antibody (6E9E6) to capture properdin from various sources including pure properdin, serum, and neutrophil supernatant (Figure 2). 6E9E6 was specifically chosen given its non-inhibitory nature and the fact that it cannot distinguish between properdin oligomers when assayed at equivalent ng/ml concentrations (24). Thus, this allows the readout of the assay (C3b deposition) to represent properdin function alone, as a property of oligomer sizes present in the sample.
The function of properdin oligomers derived from either pure properdin or from serum was evaluated to validate the functional assay. For this purpose, properdin from both sources was fractionated into oligomeric forms by size exclusion chromatography, followed by quantification of properdin in each fraction (Figure 3). As expected, the ratio of properdin oligomers in both sources followed the approximate 1:2:1 ratio of P4, P3, and P2 and pure properdin contained a small peak consisting of non-physiological aggregates (Pn) that form after repeat freeze/thaw cycles or prolonged storage whereas NHS contained a minor peak prior to P4, which may indicate the presence of pentamers or hexamers, which have been identified in NHS by others (14–16, 27, 39). The addition of the sandwich ELISA for determining concentration of the fractions, following properdin oligomeric separation within serum by size exclusion chromatography, became necessary to define the oligomers within the complex mixture of proteins found within serum. The functional properdin ELISA and size exclusion chromatography are complementary techniques. The ELISA served as an initial high-throughput assay that allows testing of several samples (>28, in triplicate, plus appropriate controls) for detection of differences in overall oligomer-related function between samples, while the size exclusion chromatography was run on individual selected samples to confirm oligomer distribution in relation to the ELISA-based function. In addition, the functional properdin ELISA, given its ability to detect function proportional to size while using very small amounts of properdin (ng), may serve as a practical screening tool for assuring absence of contaminating Pn in purified properdin preparations.
When assessing the function of properdin oligomers in the functional ELISA, there was a significant increase in C3b deposition between each properdin oligomer (P2, P3, and P4) collected from both purified properdin and serum (Figure 4). In both sources of properdin, P2 were less active than P3, and P3 were less active than P4. As expected, the function of serum-derived properdin was comparable to the function of P3 from purified properdin and P2/P3 in the context of serum, as serum-derived properdin contains predominantly P3, and does not contain non-physiological Pn (15, 27). Properdin function was the highest in non-physiological, aggregated properdin (Pn), followed by unfractionated pure properdin that contains a mixture of physiological and non-physiological oligomers. Although the Pn fraction only represents a small amount of the total properdin within unfractionated properdin, there are several characteristics of properdin that indicate Pn are highly potent complement activators: (a) Pn elute as a minor peak (Figure 3A) with an approximate molecular mass greater than 106 Da (27); (b) structural studies indicate convertases are formed at vertices of properdin oligomers (14); (c) the Pn fraction, when added to serum, completely consumes complement (15). Altogether, this indicates that Pn act similar to an activating surface, likely due to the high, variable number of available vertices and thus, even small amounts of Pn in the context of unfractionated properdin may have exponential effects on the resulting C3b deposition.
The assay was further validated by demonstrating that it can detect concentration-dependent functional differences between unfractionated pure properdin and serum-derived properdin (Figure 5). Unfractionated pure properdin, which contains highly aggregated oligomers, had higher function, as expected, than properdin derived from serum across different properdin concentrations (50-500 ng/ml). It was evident that at the lower and higher concentrations, the margin of difference between these two properdin sources narrows. Hence, for evaluating properdin function, the concentration at which properdin is prepared during the capture step must be considered. Altogether, the data indicate the convertase-initiating/stabilizing capacity of properdin oligomers increased with size and highlights the feasibility of applying the functional ELISA to detect functional differences between properdin sources and, in turn, determine whether the ratio of properdin oligomers is skewed towards P2 or P4.
We and others have shown that binding of purified properdin to necrotic cells and zymosan is dose-dependently inhibited when serum is added to the pure properdin (23, 40). Likewise, when necrotic cells and zymosan are incubated with NHS (heat inactivated or in presence of EDTA, to eliminate complement activity), native properdin in the serum does not bind. The functional assay described herein does not rely on the ability of properdin to bind to a surface. Instead, it utilizes a non-inhibitory monoclonal anti-properdin antibody to capture properdin from any sample, followed by washing, which leaves only properdin that has been captured. Thus, the captured properdin provides a site for de novo convertase formation when properdin-depleted sera is added in the following step. It remains possible that hypothetical inhibitory factors could be pulled down with the properdin during the capture step. However, Figure 4C shows no difference in the level of C3b deposition between properdin oligomers (P2 and P3) separated from pure properdin or captured from NHS, suggesting the absence of inhibitory factors in serum that would have contributed to differences in properdin function between these two sources. Properdin function is higher in P4 captured from NHS when compared to pure properdin (Figure 4C), which while unexpected, rules out the presence of inhibitory factors in serum that would contribute to reduced properdin function. To rule out the possibility that properdin was being captured along with C3(H2O) or C3 fragments in biological samples such as serum [as described previously (28)], which could potentially interfere in the outcome, the functional properdin ELISA was carried out with C3-depleted serum as a source of properdin and the results were compared to NHS as a properdin source, at equivalent concentrations (Figure 7). The deposition of C3b was not significantly different between properdin sources, suggesting that all the observed function was due to de novo convertases forming on the captured properdin and not due to the presence of C3b or C3(H2O) captured with properdin, which could have accelerated the formation of convertases and/or increased the signal per se. This was further supported by the data indicating a similar level of function between serum-derived properdin and pure P3 oligomers (Figure 4A). The outcome of the properdin functional ELISA was compared to the function in a properdin functional hemolytic assay that uses AP-sensitive ER, properdin-depleted sera, and a properdin source (Figure 6). Unlike the functional ELISA (Figure 4), the hemolytic assay was not able to consistently detect greater function in higher order oligomers (i.e., Pn>P4>P3>P2), even when the hemolytic assay parameters were varied, including time allotted for complement activation and percentage of properdin depleted serum (data not shown). Pn resulted in little activity, which has been previously shown to be due to complement consumption in the fluid phase that does not occur for physiological properdin oligomers (15). This previous study that detected Pn-mediated complement consumption using neuraminidase-treated sheep erythrocytes, also detected greater activity in P4 versus P3 and P3 versus P2 (15), unlike our hemolysis assay. Differences between the method used may, in part, explain the differences in findings. The functional properdin ELISA, does not result in consumption of Pn in the fluid phase, because properdin is captured to the solid phase first, then complement proteins (in the context of properdin-depleted sera) are added in a subsequent step to facilitate convertase assembly on the captured properdin, followed by C3b deposition. This contrasts with hemolytic assays in which properdin sources are mixed simultaneously with properdin-depleted sera (complement protein source) and the erythrocytes, permitting complement activation and consumption on properdin aggregates (i.e., Pn) in the fluid phase instead of properdin mediating surface-driven complement-mediated hemolysis.
Given that neutrophils serve important roles at sites of inflammation and release properdin when activated, the functional assay was applied to characterize the oligomeric ratio of neutrophil-derived properdin. The functional assay demonstrated that neutrophil-derived properdin was associated with a 5-fold reduction in C3b deposition compared to donor matched serum-derived properdin (Figure 8). The cause for this was identified using size exclusion chromatography where the data indicated that neutrophils release properdin predominantly as P2, while P3 and P4 levels are reduced as compared to properdin in NHS (Figures 9A–E). This is the first study to demonstrate properdin released from neutrophils exist in a ratio distinct from properdin present in healthy serum. In agreement with the notion that properdin-producing cells may secrete properdin at different oligomeric states, a previous study described T-cell-derived properdin as having approximately 100-fold more activity than serum-derived properdin (41), although the reason for this enhanced activity was never reported. Given that properdin is secreted from numerous sources in addition to neutrophils (reviewed in (21)), these data suggest the ratio of properdin oligomeric forms may differ according to cell type, although whether the release of lower order properdin oligomers is unique to neutrophils remains unknown. In addition, neutrophils release properdin in response to numerous activators including bacterial lipopolysaccharide, tumor necrosis factor-α, interleukin-8, granulocyte macrophage colony stimulating factor, C5a, N-formylmethionine-leucyl-phenylalanine (fMLP), PMA, interferon-α, and influenza A virus (33, 42, 43). It remains to be determined if neutrophils stimulated by activators other than PMA leads to the release of properdin oligomers at distinct ratios.
The finding that neutrophils release properdin oligomers as mainly P2 is especially relevant in the context of inflammatory microenvironments where changes in the ratio of properdin oligomers may influence AP activity and may thus represent a previously unknown complement regulatory mechanism. Properdin released from neutrophils as predominantly lower order oligomers may be advantageous to maintain a basal, low level AP activity near the site of neutrophil activation in the microenvironment to avoid premature consumption of properdin. This would be due to higher order properdin oligomers, specifically P4, being the first oligomeric form to be utilized by AP convertases and preferentially consumed (15), given it has the most vertices to interact with convertases (14) and thus the greatest avidity for surfaces on which convertases are formed (27). Properdin released mainly as P2 may avoid its premature consumption and enable it to eventually assemble to the approximate 1:2:1 ratio in serum and carry out its important functions in circulation, in cellular microenvironments, and at cell-cell interfaces.
In general, protein oligomerization states may be affected by changes in (a) protein concentration; (b) physiological conditions including temperature, pH, ionic strength; or (c) molecular signaling by ligand binding or post-translational modifications (reviewed in (44, 45)). Specifically, properdin oligomerization may be influenced by post-translational modifications such as O-linked glycans present in TSR4 (8). Moreover, denaturation with guanidine converts properdin into an equal mix of P2:P3:P4 (1:1:1) oligomers (15, 39). Properdin oligomerization is also sensitive to changes in pH. Properdin exposed to acidic, denaturing conditions (pH 2.3) redistributed to a mixture containing similar ratios of all three physiological oligomers and returned to an approximate 1:2:1 ratio of oligomers upon renaturing at pH 7.4 (15). Acidic pH is characteristic of inflammatory microenvironments, such as the tumor microenvironment (reviewed in (46)), synovial fluid of traumatic and osteoarthritic joints (47), and bacterial biofilms (48). Interestingly, intercellular pH increases when neutrophils are stimulated with C5a (49) or fMLP (50) (also reviewed in (51)) that could potentially influence properdin oligomerization. In our study, the pH of the neutrophil supernatant was neutral, suggesting that it is possible that other factors may influence oligomer state. However, it also suggests that neutrophil oligomer ratio may actually be mostly steady-state and that the combination of different oligomer ratios of properdin derived from different cell sources results in the final approximate 1:2:1 ratio in serum. In agreement with the possibility of consistent ratios, exchange from one oligomer form to another has been reported as slow (19) and that the oligomers are highly stable (15) in vitro.
Overall, future studies are warranted to understand the molecular mechanisms responsible for the oligomerization ratio of neutrophil-derived properdin and whether, or how, it assimilates to the approximate 1:2:1 ratio in serum.
In conclusion, our study utilized a novel ELISA-based functional assay to describe a previously unknown phenomenon whereby neutrophil-derived properdin was released in an oligomeric ratio (predominantly P2) that was distinct from serum. This knowledge contributes to understanding molecular mechanisms of AP regulation in inflammatory microenvironments.
Data availability statement
The original contributions presented in the study are included in the article/supplementary material. Further inquiries can be directed to the corresponding author.
Ethics statement
The studies involving human participants were reviewed and approved by University of Toledo Institutional Review Board (IRB). The patients/participants provided their written informed consent to participate in this study.
Author contributions
Experiments were designed by SRM, SSM, NG, VF, and were conducted by SRM and SSM. The results were analyzed and interpreted and written into the manuscript by SRM, SSM, NG, and VF. MP provided key technical design contributions. All authors critically reviewed the manuscript. All authors contributed to the article and approved the submitted version.
Funding
This work was supported by a grant from The Medical Research Society of the University of Toledo Foundation (VF), National Institute of Health R01HL112937 (VF), The American Heart Association Student Scholarship in Cardiovascular Disease (NG), and The American Association of Immunologists Careers in Immunology Fellowship Program (VF and SMM).
Acknowledgments
We thank Sean Ehinger for excellent technical support.
Conflict of interest
During part of this work, VF served as a consultant for, and received grant funding from, Apellis Pharmaceuticals. These relationships no longer exist.
The remaining authors declare that the research was conducted in the absence of any commercial or financial relationships that could be constructed as a potential conflict of interest.
Publisher’s note
All claims expressed in this article are solely those of the authors and do not necessarily represent those of their affiliated organizations, or those of the publisher, the editors and the reviewers. Any product that may be evaluated in this article, or claim that may be made by its manufacturer, is not guaranteed or endorsed by the publisher.
References
1. Pangburn MK, Schreiber RD, Müller-Eberhard HJ. Formation of the initial C3 convertase of the alternative complement pathway. acquisition of C3b-like activities by spontaneous hydrolysis of the putative thioester in native C3. J Exp Med (1981) 154:856–67. doi: 10.1084/jem.154.3.856
2. Harrison RA. The properdin pathway: an "alternative activation pathway" or a "critical amplification loop" for C3 and C5 activation? Semin Immunopathol (2018) 40:15–35. doi: 10.1007/s00281-017-0661-x
3. Ekdahl KN, Mohlin C, Adler A, Åman A, Manivel VA, Sandholm K, et al. Is generation of C3(H2O) necessary for activation of the alternative pathway in real life? Mol Immunol (2019) 114:353–61. doi: 10.1016/j.molimm.2019.07.032
4. Fearon DT, Austen KF. Properdin: binding to C3b and stabilization of the C3b-dependent C3 convertase. J Exp Med (1975) 142:856–63. doi: 10.1084/jem.142.4.856
5. Medicus RG, Götze O, Müller-Eberhard HJ. Alternative pathway of complement: Recruitment of precursor properdin by the labile C3/C5 convertase and the potentiation of the pathway. J Exp Med (1976) 144:1076–93. doi: 10.1084/jem.144.4.1076
6. Berends ET, Gorham RD Jr., Ruyken M, Soppe JA, Orhan H, Aerts PC, et al. Molecular insights into the surface-specific arrangement of complement C5 convertase enzymes. BMC Biol (2015) 13:93. doi: 10.1186/s12915-015-0203-8
7. Merle NS, Church SE, Fremeaux-Bacchi V, Roumenina LT. Complement system part I - molecular mechanisms of activation and regulation. Front Immunol (2015) 6:262. doi: 10.3389/fimmu.2015.00262
8. van den Bos RM, Pearce NM, Granneman J, Brondijk THC, Gros P. Insights into enhanced complement activation by structures of properdin and its complex with the c-terminal domain of C3b. Front Immunol (2019) 10:2097. doi: 10.3389/fimmu.2019.02097
9. Hourcade DE. The role of properdin in the assembly of the alternative pathway C3 convertases of complement. J Biol Chem (2006) 281:2128–32. doi: 10.1074/jbc.M508928200
10. DiScipio RG. The binding of human complement proteins C5, factor b, β1H and properdin to complement fragment C3b on zymosan. Biochem J (1981) 199:485–96. doi: 10.1042/bj1990485
11. Pedersen DV, Roumenina L, Jensen RK, Gadeberg TA, Marinozzi C, Picard C, et al. Functional and structural insight into properdin control of complement alternative pathway amplification. EMBO J (2017) 36:1084–99. doi: 10.15252/embj.201696173
12. Pedersen DV, Gadeberg TAF, Thomas C, Wang Y, Joram N, Jensen RK, et al. Structural basis for properdin oligomerization and convertase stimulation in the human complement system. Front Immunol (2019) 10:2007. doi: 10.3389/fimmu.2019.02007
13. Farries TC, Lachmann PJ, Harrison RA. Analysis of the interactions between properdin, the third component of complement (C3), and its physiological activation products. Biochem J (1988) 252:47–54. doi: 10.1042/bj2520047
14. Alcorlo M, Tortajada A, Rodríguez de Córdoba S, Llorca O. Structural basis for the stabilization of the complement alternative pathway C3 convertase by properdin. Proc Natl Acad Sci USA (2013) 110:13504–09. doi: 10.1073/pnas.1309618110
15. Pangburn MK. Analysis of the natural polymeric forms of human properdin and their functions in complement activation. J Immunol (1989) 142:202–7 doi: 1016/S0021-9258(17)43086-9.
16. Smith CA, Pangburn MK, Vogel CW, Müller-Eberhard HJ. Molecular architecture of human properdin, a positive regulator of the alternative pathway of complement. J Biol Chem (1984) 259:4582–8 doi: 10.4049/jimmunol.142.1.202.
17. Nolan KF, Reid KBM. Complete primary structure of human properdin: A positive regulator of the alternative pathway of the serum complement system. Biochem Soc Trans (1990) 18:1161–62. doi: 10.1042/bst0181161
18. Sun Z, Reid KBM, Perkins SJ. The dimeric and trimeric solution structures of the multidomain complement protein properdin by X-ray scattering, analytical ultracentrifugation and constrained modelling. J Mol Biol (2004) 343:1327–43. doi: 10.1016/j.jmb.2004.09.001
19. Pedersen DV, Pedersen MN, Mazarakis SM, Wang Y, Lindorff-Larsen K, Arleth L, et al. Properdin oligomers adopt rigid extended conformations supporting function. Elife. (2021) 10:e63356. doi: 10.7554/eLife.63356
20. Blatt AZ, Saggu G, Kulkarni KV, Cortes C, Thurman JM, Ricklin D, et al. Properdin-mediated C5a production enhances stable binding of platelets to granulocytes in human whole blood. J Immunol (2016) 196:4671–80. doi: 10.4049/jimmunol.1600040
21. Chen JY, Cortes C, Ferreira VP. Properdin: a multifaceted molecule involved in inflammation and diseases. Mol Immunol (2018) 102:58–72. doi: 10.1016/j.molimm.2018.05.018
22. Wirthmueller U, Dewald B, Thelen M, Schäfer MK, Stover C, Whaley K, et al. Properdin, a positive regulator of complement activation, is released from secondary granules of stimulated peripheral blood neutrophils. J Immunol (1997) 158:4444 doi: 10.4049/jimmunol.158.9.4444.
23. Ferreira VP, Cortes C, Pangburn MK. Native polymeric forms of properdin selectively bind to targets and promote activation of the alternative pathway of complement. Immunobiology. (2010) 215:932–40. doi: 10.1016/j.imbio.2010.02.002
24. Chen JY, Galwankar NS, Emch HN, Menon SS, Cortes C, Thurman JM, et al. Properdin is a key player in lysis of red blood cells and complement activation on endothelial cells in hemolytic anemias caused by complement dysregulation. Front Immunol (2020) 11:1460. doi: 10.3389/fimmu.2020.01460
25. Bain W, Li H, van der Geest R, Moore SR, Olonisakin TF, Ahn B, et al. Increased alternative complement pathway function and improved survival during critical illness. Am J Respir Crit Care Med (2020) 202:230–40. doi: 10.1164/rccm.201910-2083OC
26. Wojdyr M. Fityk: A general-purpose peak fitting program. J Appl Crystallogr (2010) 43:1126–28. doi: 10.1107/S0021889810030499
27. Farries TC, Finch JT, Lachmann PJ, Harrison RA. Resolution and analysis of ‘native’ and a’ctivated’ properdin. Biochem J (1987) 243:507–17. doi: 10.1042/bj2430507
28. Pauly D, Nagel BM, Reinders J, Killian T, Wulf M, Ackermann S, et al. A novel antibody against human properdin inhibits the alternative complement system and specifically detects properdin from blood samples. PLos One (2014) 9:e96371. doi: 10.1371/journal.pone.0096371
29. Tsyrkunou A, Agarwal S, Koirala B, Finberg RW, Nath R, Barton B, et al. Properdin levels in individuals with chemotherapy-induced neutropenia. Open Forum Infect Dis (2016) 4:ofw250. doi: 10.1093/ofid/ofw250
30. de Bont CM, Boelens WC, Pruijn GJM. NETosis, complement, and coagulation: A triangular relationship. Cell Mol Immunol (2019) 16:19–27. doi: 10.1038/s41423-018-0024-0
31. Mortaz E, Alipoor SD, Adcock IM, Mumby S, Koenderman L. Update on neutrophil function in severe inflammation. Front Immunol (2018) 9:2171. doi: 10.3389/fimmu.2018.02171
32. Leliefeld PHC, Koenderman L, Pillay J. How neutrophils shape adaptive immune responses. Front Immunol (2015) 6:471. doi: 10.3389/fimmu.2015.00471
33. Camous L, Roumenina L, Bigot S, Brachemi S, Frémeaux-Bacchi V, Lesavre P, et al. Complement alternative pathway acts as a positive feedback amplification of neutrophil activation. Blood. (2011) 117:1340–9. doi: 10.1182/blood-2010-05-283564
34. Yuen J, Pluthero FG, Douda DN, Riedl M, Cherry A, Ulanova M, et al. NETosing neutrophils activate complement both on their own NETs and bacteria via alternative and non-alternative pathways. Front Immunol (2016) 7:137. doi: 10.3389/fimmu.2016.00137
35. Wang H, Wang C, Zhao M-H, Chen M. Neutrophil extracellular traps can activate alternative complement pathways. Clin Exp Immunol (2015) 181:518–27. doi: 10.1111/cei.12654
36. Narni-Mancinelli E, Gauthier L, Baratin M, Guia S, Fenis A, Deghmane A-E, et al. Complement factor p is a ligand for the natural killer cell-activating receptor NKp46. Sci Immunol (2017) 2:eaam9628. doi: 10.1126/sciimmunol.aam9628
37. Saggu G, Cortes C, Emch HN, Ramirez G, Worth RG, Ferreira VP. Identification of a novel mode of complement activation on stimulated platelets mediated by properdin and C3(H2O). J Immunol (2013) 190:6457–67. doi: 10.4049/jimmunol.1300610
38. Kemper C, Mitchell LM, Zhang L, Hourcade DE. The complement protein properdin binds apoptotic T cells and promotes complement activation and phagocytosis. Proc Natl Acad Sci USA (2008) 105:9023–28. doi: 10.1073/pnas.0801015105
39. Medicus RG, Esser AF, Fernandez HN, Müller-Eberhard HJ. Native and activated properdin: Interconvertibility and identity of amino- and carboxy-terminal sequences. J Immunol (1980) 124:602–06 doi: 10.4049/jimmunol.124.2.602.
40. van Essen MF, Schlagwein N, van den Hoven EMP, van Gijlswijk-Janssen DJ, Lubbers R, van den Bos RM, et al. Initial properdin binding contributes to alternative pathway activation at the surface of viable and necrotic cells. Eur J Immunol (2022) 52:597–608. doi: 10.1002/eji.202149259
41. Schwaeble W, Dippold WG, Schäfer MK, Pohla H, Jonas D, Luttig B, et al. Properdin, a positive regulator of complement activation, is expressed in human T cell lines and peripheral blood T cells. J Immunol (1993) 151:2521. doi: 10.4049/jimmunol.151.5.2521.
42. Uchiyama S, Keller N, Schlaepfer E, Grube C, Schuepbach RA, Speck RF, et al. Interferon α–enhanced clearance of group a streptococcus despite neutropenia. J Infect Dis (2016) 214:321–28. doi: 10.1093/infdis/jiw157
43. Varghese PM, Mukherjee S, Al-Mohanna FA, Saleh SM, Almajhdi FN, Beirag N, et al. Human properdin released by infiltrating neutrophils can modulate influenza a virus infection. Front Immunol (2021) 12:747654. doi: 10.3389/fimmu.2021.747654
44. Nooren IMA, Thornton JM. Structural characterisation and functional significance of transient protein–protein interactions. J Mol Biol (2003) 325:991–1018. doi: 10.1016/S0022-2836(02)01281-0
45. Hashimoto K, Nishi H, Bryant S, Panchenko AR. Caught in self-interaction: Evolutionary and functional mechanisms of protein homooligomerization. Phys Biol (2011) 8:35007. doi: 10.1088/1478-3975/8/3/035007
46. Kato Y, Ozawa S, Miyamoto C, Maehata Y, Suzuki A, Maeda T, et al. Acidic extracellular microenvironment and cancer. Cancer Cell Int (2013) 13:89. doi: 10.1186/1475-2867-13-89
47. Henrietta JE, Eileen M-JM. On the viscosity and pH of synovial fluid and the pH of blood. J Bone Joint Surg (1959) 41-B:388–400. doi: 10.1302/0301-620x.41b2.388
48. Deng Q, Sun P, Zhang L, Liu Z, Wang H, Ren J, et al. Porphyrin MOF dots–based, function-adaptive nanoplatform for enhanced penetration and photodynamic eradication of bacterial biofilms. Adv Funct Mater (2019) 29:1903018. doi: 10.1002/adfm.201903018
49. Denk S, Neher MD, Messerer DAC, Wiegner R, Nilsson B, Rittirsch D, et al. Complement C5a functions as a master switch for the pH balance in neutrophils exerting fundamental immunometabolic effects. J Immunol (2017) 198:4846–54. doi: 10.4049/jimmunol.1700393
50. Simchowitz L. Intracellular pH modulates the generation of superoxide radicals by human neutrophils. J Clin Invest (1985) 76:1079–89. doi: 10.1172/jci112061
Keywords: complement system, alternative pathway, properdin, neutrophil, oligomers
Citation: Moore SR, Menon SS, Galwankar NS, Khuder SA, Pangburn MK and Ferreira VP (2023) A novel assay that characterizes properdin function shows neutrophil-derived properdin has a distinct oligomeric distribution. Front. Immunol. 13:918856. doi: 10.3389/fimmu.2022.918856
Received: 12 April 2022; Accepted: 21 December 2022;
Published: 12 January 2023.
Edited by:
Nicole Thielens, UMR5075 Institut de Biologie Structurale (IBS), FranceReviewed by:
Cees Van Kooten, Leiden University, NetherlandsGregers Rom Andersen, Aarhus University, Denmark
Copyright © 2023 Moore, Menon, Galwankar, Khuder, Pangburn and Ferreira. This is an open-access article distributed under the terms of the Creative Commons Attribution License (CC BY). The use, distribution or reproduction in other forums is permitted, provided the original author(s) and the copyright owner(s) are credited and that the original publication in this journal is cited, in accordance with accepted academic practice. No use, distribution or reproduction is permitted which does not comply with these terms.
*Correspondence: Viviana P. Ferreira, Vml2aWFuYS5GZXJyZWlyYUB1dG9sZWRvLmVkdQ==
†These authors have contributed equally to this work