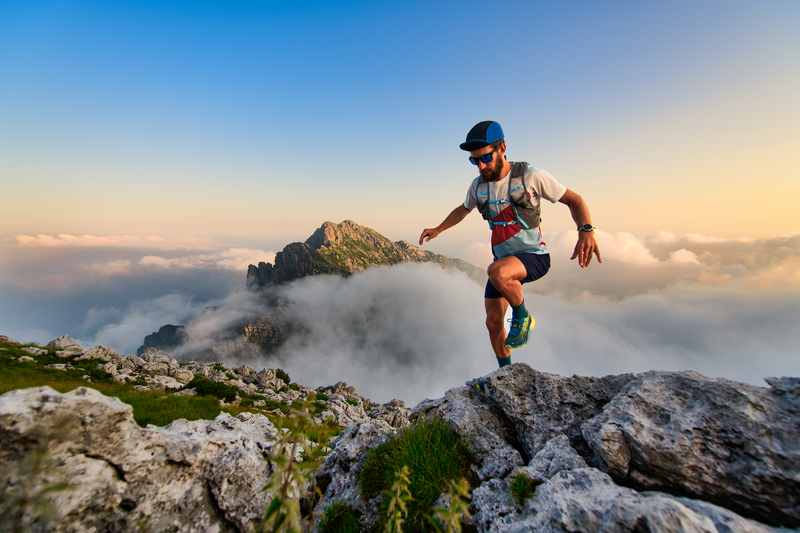
95% of researchers rate our articles as excellent or good
Learn more about the work of our research integrity team to safeguard the quality of each article we publish.
Find out more
ORIGINAL RESEARCH article
Front. Immunol. , 12 August 2022
Sec. Inflammation
Volume 13 - 2022 | https://doi.org/10.3389/fimmu.2022.918476
This article is part of the Research Topic Oral Neutrophils - The Good, the Bad, and the Ugly View all 13 articles
Background: Deep venous thrombosis (DVT) highly occurs in patients with severe COVID-19 and probably accounted for their high mortality. DVT formation is a time-dependent inflammatory process in which NETosis plays an important role. However, whether ginsenoside Rg5 from species of Panax genus could alleviate DVT and its underlying mechanism has not been elucidated.
Methods: The interaction between Rg5 and P2RY12 was studied by molecular docking, molecular dynamics, surface plasmon resonance (SPR), and molecular biology assays. The preventive effect of Rg5 on DVT was evaluated in inferior vena cava stasis–induced mice, and immunocytochemistry, Western blot, and calcium flux assay were performed in neutrophils from bone marrow to explore the mechanism of Rg5 in NETosis via P2RY12.
Results: Rg5 allosterically interacted with P2RY12, formed stable complex, and antagonized its activity via residue E188 and R265. Rg5 ameliorated the formation of thrombus in DVT mice; accompanied by decreased release of Interleukin (IL)-6, IL-1β, and tumor necrosis factor-α in plasma; and suppressed neutrophil infiltration and neutrophil extracellular trap (NET) release. In lipopolysaccharide- and platelet-activating factor–induced neutrophils, Rg5 reduced inflammatory responses via inhibiting the activation of ERK/NF-κB signaling pathway while decreasing cellular Ca2+ concentration, thus reducing the activity and expression of peptidyl arginine deiminase 4 to prevent NETosis. The inhibitory effect on neutrophil activity was dependent on P2RY12.
Conclusions: Rg5 could attenuate experimental DVT by counteracting NETosis and inflammatory response in neutrophils via P2RY12, which may pave the road for its clinical application in the prevention of DVT-related disorders.
Deep venous thrombosis (DVT) belongs to venous thromboembolic disorder and is the leading cause of pulmonary embolism, which eventually contributes to heart failure and even sudden death (1). DVT is generally characterized by abnormal coagulation of blood in deep veins of lower leg and thigh; however, it may occur in upper limb deep veins, visceral veins, and vena cava (2). DVT highly occurs in long-term sedentary people and post-surgery patients (3). Recently, clinical studies have found an association between high mortality in patients with COVID-19 and DVT (4). The clinical diagnosis of DVT mainly depends on clinical risk score, serum D-dimer, and color Doppler ultrasound. Anticoagulants such as Aspirin, antiplatelet drugs, Rivaroxaban, and low molecular–weight heparin are common clinical treatment for the prevention of DVT (5). Unfortunately, all of these treatments carry a remarkable risk of bleeding (6). Therefore, it is still urgent to develop effective antithrombotic drugs with less side effects to slow down the occurrence of DVT.
Neutrophils play an import role in thrombosis. Together with platelets, neutrophils are the first cells mobilized to the injury/infection sites to limit the dissemination of microbial infection by promoting blood coagulation. However, dysregulation or excessive stimulation may contribute to thrombotic processes (7). Neutrophils release neutrophil extracellular traps (NETs) upon excessive stimulation, a process called NETosis, which promotes thrombosis by forming a scaffold for adhesion of platelets, erythrocytes, and platelet adhesion molecules (8). NETs are extracellular, web-like structures mainly composed of decondensed chromatin and granule proteins such as histones, neutrophil elastase, myeloperoxidase (MPO), calprotectin, cathelicidins, defensins, and actin (9). When activated by Ca2+, peptidyl arginine deiminase 4 (PAD4) catalyzes the conversion of histone arginine to citrulline, resulting in the decondensation of heterochromatin and prompting NET formation. Preventing NETosis can decrease thrombogenicity, which may be beneficial for thrombosis prevention and become a promising therapy target for DVT (10).
P2RY12, as an adenosine diphosphate (ADP) receptor on the platelet surface, is a key player in platelet activation and a vital target of antithrombotic drugs such as Clopidogrel, Praugrel, and Ticagrelor (11). Upon activation by ADP, P2RY12 is coupled to the Gi2 protein, and its activation inhibits adenylate cyclase activity, thereby reducing the intracellular cyclic adenosine monophosphate (cAMP) level. In addition, Gi2 signaling gives rise to the activation of Phosphatidylinositol 3-kinase/protein kinase B (PI3K/AKT) pathway, which leads to a significant increase of granule release and platelet aggregation (12, 13), suggesting that dense granule release, procoagulant activity, and thrombosis are dependent on P2RY12 activation (14, 15). Interestingly, P2RY12 is expressed not only on the surface of platelets but also on several immune cells, including eosinophils, monocytes, macrophages, lymphocytes dendritic cells, and mast cells, which are involved in the inflammatory process of many diseases (16). Studies have shown that the P2RY12 plays an important role in early DVT formation (17), and P2RY12 inhibitor Clopidogrel, Ticagrelor, or Prasugrel can restrain platelet-leukocyte interaction (18–20). Although a study has demonstrated the reduction of platelet-leukocyte aggregation and NETs by Ticagrelor in patients with pneumonia (21), the direct relationship between P2RY12 and NETosis has not been elucidated yet.
Species of Panax genus, including Panax ginseng C.A. Meyer, Panax notoginseng (Burkill) F.H. Chen, and Panax quinquefolius L., are precious Chinese herbal medicines with beneficial effects in reinforcing immunity and reducing fatigue. Ginsenoside Rg5 (Figure 2A) is one of the natural saponins in P. ginseng and P. notoginseng, which has multiple pharmacological activities, such as anti-cancer, anti-inflammation, anti-diabetes, anti-obesity, neuroprotection, and cardioprotection (22). However, whether Rg5 could benefit DVT therapy has not been elucidated yet. In the present study, we firstly identified that Rg5 might bind to P2RY12 by molecular docking, which was confirmed by molecular dynamics (MD) simulation, surface plasmon resonance (SPR), and site mutation analysis. Furthermore, in DVT model mice, we found that Rg5 could attenuate thrombosis, which might be exerted by inhibiting NETosis through preventing NET release and inflammatory response by antagonizing P2RY12. These findings may pave the road for the clinical application of Rg5 in the prevention of DVT.
Ginsenoside Rg5 (Cat# BP1651, purity > 95%) was purchased from Biopurify Phytochemicals, Ltd. (Chengdu, China). Rivaroxaban (Cat# MB1878, purity > 98%) was obtained from Meilun Biotechnology Co., Ltd. (Dalian, China). Platelet-activating factor (PAF) (Cat# GC14535) was provided by GlpBio Technology, Inc. (CA, USA). Lipopolysaccharide (LPS) from Escherichia coli 0111:B4 was obtained from Sigma-Aldrich Company (MO, USA). 2-Methylthioadenosine diphosphate (2MesADP) trisodium salt was purchased from Tocris Bioscience (MN, USA). n-Dodecyl-β-D-Maltopyranoside (DDM) (Cat# D310) was bought from Anatrace (OH, USA).
Antibodies against AKT (Cat# T55561F), phospho-AKT-Ser473 (Cat# T40067F), phospho-ERK1 (T202/Y204) + ERK2 (T185) (Cat# T40072F), p44/42 MAPK (ERK1/2) (Cat# T40071F), and GAPDH (Cat# M20006F) were purchased from Abmart (Shanghai, China). Nuclear factor-kappa B (NF-kB) (Cat# 8242S), p-NF-κB-Ser536 (Cat# 3033L), and β-actin (Cat# 12413) antibodies were obtained from Cell Signaling Technology (C.S.T.) Co. (MA, USA). Anti-PAD4 antibody (Cat# ab214810) and anti-Histone H3 (citrulline R2 + R8 + R17) antibody (Cat# ab5103) were purchased from Abcam (Cambridge, England). Anti-MPO antibody (Cat# GB11224) was purchased from Servicebio (Wuhan, China).
Wide-type C57BL/6 male mice (25 ± 2 g) were provided by Shanghai Sippe-Bk Lab Animal Co., Ltd. and were adapted for 1 week before use. P2RY12-knockout (KO) mice were kindly provided by Dr. Jun-ling Liu from Ruijin Hospital, Shanghai Jiaotong University School of Medicine (Shanghai, China), and genotyped as described previously (23). All mice received humane care and were kept in a standard environment with a 12/12-h cyclic lighting schedule (lights on at 07:00 am) in the Laboratory Animal Center of Shanghai University of Traditional Chinese Medicine (SHUTCM, Shanghai, China). The temperature and humidity were maintained at 25 ± 2°C and 45 ± 5%, respectively.
BMNs from the femur and tibia of wild type (WT) or P2RY12-KO mice were prepared according to the instruction of the Mouse Bone Marrow Neutrophil Extraction Kit (Solarbio, Beijing, China).
BMNs were seeded in 24-well plate at 2 × 106/ml per well and treated with PBS or 6.25, 12.5, and 18.75 μM Rg5 for 2 h followed by stimulation of LPS (20 μg/ml) or 50 μM PAF for 30 min. Then, the cells were collected for Western blot analysis, and cell-free supernatant was collected for further analysis. DNA content in supernatant was measured by using Quant-iT PicoGreen dsDNA Reagent and Kits (Invitrogen, CA, USA). The concentration of DNA released by neutrophils treated with 0.3% Triton was set as 100%, and the relative proportion of cell-free DNA (cfDNA) concentration in each sample was calculated by comparing with the former.
Serum or medium concentrations of D-dimer, IL-6, IL-1β, tumor necrosis factor-α (TNF-α) and citrullinated histones 3 (CitH3) were measured by using respective ELISA kits (Lengton Bioscience, Shanghai, China). The absorbance was detected at 450 or 570 nm on a microplate reader (FlexStation 3, Molecular Devices, CA, USA).
To examine the effect of Rg5 on the formation of NETs, the BMNs were seeded in 24-well plates with coverslips pre-coated with poly-D-lysine. After treatment of 18.75 μM Rg5 for 2 h followed by stimulation of LPS (5 μg/ml) or 20 μM PAF for 3 h, the cells were fixated by 4% Paraformaldehyde (PFA) for 10 min. Consequently, the cells were blocked with 5% donkey serum for 1 h and incubated overnight with antibody against CitH3 (1:1,000) at 4°C, followed by Alexa 488–conjugated secondary antibody (1:800). Finally, the coverslips were mounted on slides with mounting medium containing Diamidinyl phenyl indole (DAPI) in the dark. The microscopy images were captured by Olympus slide scanner (VS120, Japan) and analyzed by ImageJ (version 1.46r).
The P2RY12–antagonist complex structure (Protein Data Bank Code: 4NTJ) was obtained to predict the potential binding mode of Rg5 with P2RY12. As described previously (24), AutoDock Vina (version 1.1.2), Maestro (version 11.4, Schrödinger, LLC, New York, NY, 2021), and molecular operating environment (MOE, Chemical Computing Group, version 2019.0101) softwares were used to calculate the binding capability of Rg5 and other compounds. The exponential consensus ranking (ECR) analysis (25) was used to assign a rank to each ligand based on the molecular docking score provided by different docking programs to combine the results of multiple docking programs.
P2RY12 protein were expressed and purified as described previously (26) and concentrated to approximately 1 mg/ml for further usage.
The binding affinity between Rg5 and P2RY12 was measured using Biacore T200 (GE healthcare, MA, USA). P2RY12 protein was immobilized on the chip in the presence of 1× HEPES Buffered Saline-EDTA P20 (HBS-EP) with 0.03% DDM buffer (pH 7.4) by using the His Capture Kit (GE healthcare, MA, USA). Various concentrations of Rg5 (0.098, 0.195, 0.391, 0.781, 0.562, and 3.125 μM) or Ticagrelor (0.031, 0.062, 0.125, 0.25, 0.5, and 1 μM) dissolved in 5% DMSO were passed at 30 μl/min for 120 s over the P2RY12 protein, followed by a dissociation step of 120 s. The Equilibrium dissociation constant (KD) value was obtained by fitting the data to a steady state affinity model using Biacore T200 Evaluation Software (version 3.0).
The stable MD trajectory of the P2RY12–Rg5, P2RY12–Ticagrelor, or P2RY12–Aspirin complex was estimated by the Molecular mechanics/poisson-boltzmann surface area (MM/PBSA) technique implemented in AMBER14 as described previously (27). The MM/PBSA method combined molecular mechanics and continuous solvent model was used to predict the binding free energy of P2RY12 protein and ligands.
CHO-P2RY12 cells overexpressing P2RY12 were cultured in Dulbecco's modified eagle medium (DMEM) high-glucose medium containing 10% fetal bovine serum at 37°C. To examine the inhibitory effect of Rg5 against P2RY12 signaling, CHO-P2RY12 cells were seeded at a density of 1 × 105 cells per well in six-well plates for 24 h and serum-starved for 12 h. Then, the cells were treated with Rg5 (3.125, 6.25, 12.5, 25, and 50 μM) for 2 h followed by stimulation of 2MesADP (100 nM) for 5 min. Afterward, the cells were collected for further Western blot analysis.
The coding sequence of P2RY12 (Homo sapiens, Gene ID: 64805) was cloned into PCMV6 vector and site-directed mutated by using the Mut Express II Fast Mutagenesis Kit (Vazyme, Nanjing, China). The primers (Generay Biotech, Shanghai, China) were listed as follows: P2RY12_E188A mutation (forward, 5′- GTCTGGCATGCGATAGTAAATTACATCTGTC-3′; reverse, 5′- GTAATTTACTATCGCATGCCAGACTAGACCG-3′), P2RY12_R265A mutation (forward, 5′- CAAACCGCTGATGTCTTTGACTGCACTGCTGAA-3′; reverse, 5′- GACATCAGCGGTTTGGCTCAGGGTGTAAGGAATT-3′), P2RY12_D266A mutation (forward, 5′- ACCCGGGCGGTCTTTGACTGCACTGCTGAAAATA -3′; reverse, 5′- AAAGACCGCCCGGGTTTGGCTCAGGGTGTAA -3′), and P2RY12_R265A and D266A mutation (forward, 5′- CTGAGCCAAACCGCTGCGGTCTTTGACTGC -3′; reverse, 5′- GTGCAGTCAAAGACCGCAGCGGTTTGGCTC -3′). Plasmid was extracted according to the steps in the EndoFree Midi Kit (Cwbiotech, Taizhou, China) and transfected into CHO cells for 12 h by Tecfect DNA transfection reagent (TEYE Co, Shanghai, China). CHO cells were cultured and treated under the same conditions as CHO-P2RY12 cells, which were collected for further Western blot analysis.
The methods of protein samples preparation and Western blot analysis were described previously (28). After incubation in primary antibodies (1:1,000) and secondary antibody (1:5,000), the protein bands were visualized by using the ECL Enhanced Kit (ABclonal Technology, Wuhan, China). The photographs were taken and analyzed by using Tanon 5200 Multi (Shanghai, China).
DVT model was established as described previously (29). In brief, the IVC caudal to the left renal vein was ligated for 12 h to achieve stasis induction of thrombosis. None of the mice showed any bleeding during surgery.
A total of 48 mice were randomly divided into six groups: (1) Sham group; (2) DVT group; (3) Rg5 of 1.25 mg/kg + DVT group; (4) Rg5 of 2.5 mg/kg + DVT group; (5) Rg5 of 5 mg/kg + DVT group; and (6) Rivaroxaban of 0.3 mg/kg + DVT group. Rg5 is dissolved in saline containing 2% ethanol for injection, and Rivaroxaban was dissolved in a special solution (Polyethylene glycol/Saline/Glycerin = 996 g/100 g/60g). Rg5 or Rivaroxaban was intravenously given at 15 min prior to thrombus induction. IVC of mice in sham group was separated without ligation. After the formation of DVT, arterial blood was collected immediately and mixed with 10% sodium citrate for anticoagulation followed by centrifugation at 3,000 rpm for 15 min to obtain the plasma. The IVC between the left renal vein and the iliac crest bifurcation was separated, whose length and wet weight were measured. Afterward, the IVC was fixed by 4% PFA for further hematoxylin and eosin (HE) staining and immunohistochemistry.
For HE staining, the 5-μm-thick sections were dewaxed and stained by HE as described previously (30). For immunohistochemistry, the sections were deparaffinzed and rehydrated, followed by antigen retrieval as described elsewhere (31). After deactivation of endogenous peroxidase with H2O2, they were blocked with 3% Bull serum albumin (BSA) and incubated with the anti-MPO antibody (1:2,000) and the secondary antibody (1:200). The immuno-reactive cells were visualized after Diaminobenzidine (DAB) chromogenic reaction. Finally, the images were captured by Olympus slide scanner (VS120, Japan) and analyzed by ImageJ (version 1.46r).
Calcium flux in BMNs was monitored by using the Screen Quest™ Calbryte-520 Probenecid-Free and Wash-Free Calcium Assay Kit (AAT Bioquest, CA, USA). In brief, BMNs were seeded at 2 × 106 cells/ml in 96-well plate. After Rg5 (18.75 μM) treatment for 2 h, BMNs were loaded with Calbryte 520 AM dye for 45 min. Then, the cells were stimulated with PAF (20 μM) and monitored immediately on a fluorescence microplate reader (FlexStation 3, Molecular Devices, CA, USA) with excitation wavelength at 490 nm and emission wavelength at 525 nm at 37°C for 60 min.
BMNs were stimulated with PAF (20 μM) for 3 h after pre-treated with Rg5 (18.75 μM) for 2 h. Then, the cells were collected and lysed to extract the proteins, which were incubated with 10 mM ethyl N-benzoyl-L-argininate hydrochloride (BAEE) at 37°C for 10 min. The ammonia content produced through the enzymatic hydrolysis of BAEE by PAD4 was measured according to the procedure described by the manufacturer (Blood Ammonia Content Detection Kit). Finally, the absorbance was measured at 630 nm on a microplate reader (FlexStation 3).
The data were presented as mean ± SEM to describe the differences among multiple groups. Differences among groups were analyzed by one-way ANOVA with Dunnett’s analysis (n ≥ 4) and Kruskal–Wallis test (n = 3) using GraphPad Prism 5.0. The value of P < 0.05 was considered statistically significant.
Because whether P2RY12 is involved in NETosis has not been elucidated yet, we firstly investigated its role in NETosis. As shown in Figure 1A, PAF dose-dependently induced the production of cfDNA in both WT and P2RY12-KO neutrophils. However, when stimulated with the same dose of PAF, higher than 50 μM, P2RY12-KO neutrophils produced significantly less cfDNA than WT neutrophils (P < 0.05, P < 0.001). Similarly, when stimulated at the concentration higher than 20 μg/ml, LPS also induced much more release of cfDNA in WT neutrophils than that in P2RY12-KO neutrophils (P < 0.01 and P < 0.001). After PAF or LPS stimulation, much more NETs in reticular structure, which were mainly composed of DNA and CitH3 derived from chromatin depolymerization in the nucleus, were released from WT neutrophils than that from P2RY12-KO neutrophils (Figure 1B, P < 0.01). Moreover, PAF-induced remarkably increased production of inflammatory factors, such as IL-6, IL-1β, and TNF-α in WT neutrophils (Figure 1C, P < 0.05); by contrast, it induced slight increment of IL-6, IL-1β, and TNF-α in P2RY12-KO neutrophils. Similarly, LPS induced more release of inflammatory cytokines in WT neutrophils than that in P2RY12-KO neutrophils (Figure 1D). These results implicated that P2RY12 is critical for the NETosis and inflammatory response in neutrophils.
Figure 1 Effects of P2RY12 on PAF and LPS-induced NET release and inflammatory responses. (A) Different concentrations of PAF or LPS induced the release of cfDNA from WT or P2RY12-KO neutrophils (n = 4). (B) Immunofluorescent staining and statistical analysis of CitH3 and DAPI staining of WT or P2RY12-KO neutrophils induced by PAF and LPS, respectively (n = 4). (C, D) Release of IL-6, IL-1β, and TNF-α in medium of WT or P2RY12-KO neutrophils induced by PAF and LPS, respectively (n = 4). All the data are shown as the mean ± SEM. *P < 0.05, **P < 0.01, ***P < 0.001 vs. WT control; ###P < 0.001 vs. P2RY12-KO Control; &P < 0.05, &&P < 0.01, &&&P < 0.001, WT vs. P2RY12-KO. #P < 0.05, ##P < 0.01.
As aforementioned, P2RY12 is important for neutrophil activation, particularly NETosis, we next carried out virtual screening by using ECR strategy, which was adopted to better describe the comprehensive binding affinity of the P2RY12–ligand complex predicted by Autodock Vina, Glide, and MOE softwares. We used ranking values rather than docking scores in subsequent docking analyses. According to the ranking results, the binding affinity between Rg5 and P2RY12 was similar to that between Ticagrelor and P2RY12 (Table 1). To further investigate the stability of Rg5 binding to P2RY12, MD analysis was performed. As shown in Figure 2B, the electrostatic interaction (ΔEele) between Rg5 and P2RY12 was higher than that between Ticagrelor and P2RY12, and the total free energy (ΔGTot) of Rg5 was also slightly higher than that of Ticagrelor. In addition, as shown in Figure 2C, compared with the stable state of Ticagrelor in P2RY12, although the complex of Rg5 and P2RY12 fluctuated before 25 ns, it quickly leveled off later; and Aspirin remained in a state of fluctuation in P2RY12. The Root mean square deviation (RMSD) trajectories of Rg5 and Pocket also fluctuated slightly at the beginning but became stable later on. To confirm the direct interaction, we conducted SPR assay. As shown in Figure 2D, Rg5 dose-dependently bound to P2RY12 with a KD of 0.33 μM, which was similar to that of Ticagrelor (0.102 μM). These results suggested that Rg5 could bind to P2RY12 steadily and affect the activity of the latter. On this premise, we used CHO-P2Y12-OE cells to explore whether the binding of Rg5 to P2RY12 could interfere the downstream signaling of the latter. As demonstrated in Figure 2E, Rg5 itself had no effect on P2RY12 signaling, but it inhibited 2MesADP-induced phosphorylation of AKT and ERK in a dose-dependent manner, suggesting the antagonic effect of Rg5 on P2RY12.
Figure 2 Interaction between Rg5 and P2RY12. (A) Chemical structure for Rg5. (B) Comparison of binding free energy between Rg5, Ticagrelor, and Aspirin binding to P2RY12. ΔEvdw, van der Waals energy; ΔEele, electrostatic energy; ΔEPB, Poisson–Boltzmann energy; ΔESA, nonpolar energy; ΔGTot, total binding free energy. (C) Stability analysis of Rg5, Ticagrelor, and Aspirin binding to P2RY12. (D) SPR analyses of Rg5 and Ticagrelor binding to P2RY12. (E) Protein expression and phosphorylation levels of AKT (n = 3) and ERK (n = 3). All the data are shown as the mean ± SEM. *P < 0.05, **P < 0.01 vs. 2MesADP group.
To find out the exact binding sites, we first carried out molecular docking analysis of Rg5 and P2RY12. As shown in Figures 3A, B, Rg5 was predicted to interact with P2RY12 at amino acid residues E188, R256, T260, R265, and D266. Furthermore, energy decomposition of amino acid residues based on MM/PBSA free energy calculation showed that amino acid residues S101, E188, R256, R265, and D266 were important in the binding process of Rg5 and P2RY12 (Figure 3C). Because R256 was reported to play a pivotal role for the binding of ADP with P2RY12, and the mutation of R256 weakened the activation of P2RY12 by ADP, we chose E188, R265, and D266 for site-directed mutagenesis. As shown in Figure 3D, a series of P2RY12 variants were generated and overexpressed in CHO cells followed by activation of 2MesADP. However, Rg5 still could antagonize 2MesADP-induced phosphorylation of AKT and ERK unless E188 and R265 was simultaneously mutated into alanine. According to the docking results in Figure 3D, there were two main binding modes of Rg5 in P2RY12 mutants. As shown in Figure 3E, Rg5 mainly bound to two extracellular pockets, which were separated by residues Y105 and K280. Pocket 2 was the binding site of Rg5 in the WT conformation, E188A mutant, and E188A and R265A mutant of P2RY12; whereas in other mutants, Rg5 bound to the more advantageous Pocket 1. The interaction between Rg5 and binding pocket in Figure 3F showed the absence of hydrogen bonding with E188 and R265 in the interaction of Rg5 and E188A and R265A mutant, which resulted in the weakened binding effect of Rg5 to P2RY12. Ranking results in Table 2 also verified that the E188A and R265A mutant had the weakest affinity with Rg5, suggesting that E188 and R265 play an important role in the interaction between Rg5 and P2RY12.
Figure 3 Interaction sites of Rg5 with P2RY12. (A) P2RY12 and Rg5 complex structure, and binding pocket with Rg5. (B) Two-dimensional interaction map of interactions between Rg5 and P2RY12. (C) Energy decomposition of amino acid residues in P2RY12 and Rg5 based on MM/PBSA free energy calculation. (D) Structures of P2RY12 mutation variants with Rg5 bound were shown in the upper panel, and Western blot analysis in the lower panel displayed the protein expression and phosphorylation levels of AKT (n = 4) and ERK (n = 4). All the data are shown as the mean ± SEM. *P < 0.05, **P < 0.01, ***P < 0.001 vs. 2MesADP group. (E) Hypothetical binding modes of Rg5 to P2RY12 mutation variants obtained by molecular docking. The binding conformation of Rg5 in each P2RY12 mutant were colored as follows: white, original; slate, E188A; orange, E188A and R265A; magenta, R165A; pink, D266A; cyan, E188A and D266A; yellow, R265A and D266A; green, E188A and R265A and D266A. (F) A view of the P2RY12 mutant/Rg5 complex.
As shown in Figure 4, IVC ligation caused significant thrombosis in mice (Figure 4A). Accordingly, the wet weight and length of thrombus in the DVT group mice were increased markedly, compared with the sham group (P < 0.001). Rg5 pretreatment, especially at dosages of 2.5 and 5 mg/kg, significantly reduced thrombus formation (P < 0.05 or P < 0.001). Meanwhile, Rg5 pretreatment at higher dosages suppressed the plasma D-dimer content in DVT mice (Figure 4D, P < 0.05). Similarly, the positive control drug, Rivaroxiban (0.3 mg/kg), also attenuated thrombus formation, as well as the plasma D-dimer content in DVT mice. These results demonstrated that Rg5 could prevent thrombosis in deep vein. On the other hand, DVT is closely relevant to thrombophlebitis. As shown in Figure 4E, much more inflammatory cells infiltrated in the veins of DVT mice compared with the sham mice. Moreover, MPO staining exposed that most of the accumulated inflammatory cells were neutrophils (Figure 4F). After pretreatment of Rg5, the infiltration of inflammatory cells including neutrophils in vein was reduced (P < 0.01 or P < 0.001). Rg5 treatment (2.5 and 5 mg/kg) also significantly inhibited the release of inflammatory cytokines IL-6, TNF-α, and IL-1β in plasma (Figures 4H–J, P < 0.05 or P < 0.001). In addition, DVT is closely related to NETs produced by neutrophils. As the biomarker of NET formation, the content of citrullinated histone H3 (CitH3) was elevated after induction of DVT, which was significantly reduced after Rg5 (5 mg/kg) pre-administration (Figure 4K, P < 0.05). Interestingly, the positive drug, Rivaroxiban (0.3 mg/kg), could also suppress neutrophil infiltration and inflammatory response, as well as CitH3 expression. These results suggested that Rg5 could inhibit NET release and inflammatory response in mice.
Figure 4 Effect of Rg5 administration on thrombosis and inflammation in DVT mice. (A) Morphology of venous thrombosis. (B, C) Thrombus wet weight and length (n = 8). (D) Plasma D-dimer content (n = 7). (E) HE staining of inferior vena cava. (F) MPO immunohistochemical staining of inferior vena cava. (G) MPO positive cell density (n = 6). (H–K) Plasma contents of IL-6, IL-1β, TNF-α, and CitH3 (n = 7). Data are shown as the mean ± SEM. *P < 0.05, **P < 0.01, ***P < 0.001 vs. DVT group.
On the premise that Rg5 had no effect on neutrophils viability (Figure 5A), we first investigated whether Rg5 had an inhibitory effect on NETosis induced by PAF or LPS. As shown in Figure 5B, Rg5 pre-treatment significantly reduced the chromatin depolymerization and the expression of CitH3 in PAF-induced WT neutrophils (P < 0.001). However, in PAF-induced P2RY12-KO neutrophils, Rg5 did not show the same effect. Similar results were found in LPS-induced WT and P2RY12-KO neutrophils (Figure 5C). In addition, Rg5 treatment dose-dependently reduced cfDNA release and inflammatory factor production in culture medium of WT neutrophils induced by PAF and LPS (Figures 5D, E). However, in PAF-induced P2RY12-KO neutrophils, Rg5 pre-treatment at 18.75 μM slightly suppressed the cfDNA release, whereas it could not inhibit further inflammatory factor release (Figure 5D). Similarly, in LPS-induced P2RY12-KO neutrophils, Rg5 still showed slight inhibitory effects on the production of IL-1β (Figure 5E). These results suggested that Rg5 inhibited NETosis mainly through P2RY12.
Figure 5 Effects of Rg5 on PAF or LPS-induced release of NETs and inflammatory factors in WT or P2RY12-KO neutrophils. (A) Effect of Rg5 on WT or P2RY12-KO neutrophils viability (n = 4). (B, C) Immunofluorescent staining and statistical analysis of CitH3 in WT or P2RY12-KO neutrophils stimulated by PAF or LPS. (n = 6). (D, E) DNA concentration (n = 4) and IL-6, IL-1β, and TNF-α concentrations (n = 4) in the medium of WT or P2RY12-KO neutrophils stimulated by PAF or LPS. All the data are shown as the mean ± SEM. *P < 0.05, **P < 0.01, ***P < 0.001 vs. PAF or LPS group.
As PAD4 plays an important role in NETosis, we next examined whether Rg5 could influence the activity of PAD4 and whether this action was dependent on P2RY12. As shown in Figure 6A, Rg5 treatment significantly reduced PAF-induced calcium flux. In contrast, Rg5 could not further mitigate PAF-induced calcium flux in P2RY12-KO neutrophils (Figure 6A). In terms of PAD4 expression, 18.75 μM Rg5 treatment significantly mitigated the increase of PAD4 induced by PAF in WT neutrophils (Figure 6B, P < 0.05). Accordingly, the catalytic activity of PAD4 in WT neutrophils was suppressed by Rg5, compared with PAF group cells (Figure 6C, P < 0.01 or P < 0.001). When P2RY12 was deleted, Rg5 displayed no effect on the expression and activity of PAD4 in neutrophils. These results implicated that Rg5 suppressed PAF-induced elevation of PAD4 in neutrophils through P2RY12. Thereby, we investigated whether Rg5 could regulate the signaling pathway associated with inflammatory cytokine production and whether this effect was dependent on P2RY12. As shown in Figure 6D, PAF-induced a marked rise in the phosphorylation of ERK and NF-κB, which could be counteracted by Rg5 treatment (P < 0.05, P < 0.01, or P < 0.001). PAF could also induce the phosphorylation of ERK and NF-κB in P2RY12-KO neutrophils. However, Rg5 treatment did not suppress the activated ERK and NF-κB in P2RY12-KO cells. Similarly, Rg5 failed to inhibit LPS-activated ERK/NF-κB pathway in P2RY12-KO neutrophils (Figure 6E). These results indicated that Rg5 suppressed the inflammatory pathway in neutrophils depending on P2RY12.
Figure 6 Effects of Rg5 on PAF or LPS-induced activation of PAD4 and ERK/NF-κB signaling pathway in WT or P2RY12-KO neutrophils. (A) Calcium flux in WT or P2RY12-KO neutrophils stimulated by PAF (n = 3). (B) Protein expression of PAD4 in WT or P2RY12-KO neutrophils stimulated by PAF (n = 5). (C) Activity of PAD4 in WT or P2RY12-KO neutrophils stimulated by PAF (n = 4). (D, E) Western Blot results and statistical analysis of protein expression and phosphorylation levels of ERK (n = 5) and NF-κB (n = 5) in WT or P2RY12-KO neutrophils stimulated by PAF or LPS. All the data are shown as the mean ± SEM. *P < 0.05, **P < 0.01, ***P < 0.001 vs. PAF or LPS group.
In this study, we demonstrated that Rg5 inhibited signal transduction in neutrophils and reduced DVT formation by interacting with P2RY12. On the one hand, Rg5 suppressed inflammatory response by inhibiting cytokine production through ERK/NF-κB signaling pathway; on the other hand, it reduced NETosis by preventing intracellular calcium mobilization, leading to the loss of PAD4 activity. Our results suggested that Rg5 might be a promising candidate for the prevention of DVT by counteracting neutrophil activation through P2RY12.
P2RY12 has been shown as a drug target for the prevention of platelet aggregation for a long time and is also involved in inflammation. For instance, knockout of P2RY12 or blocking of P2RY12 with Clopidogrel significantly reduced the volume of venous thrombosis in mice (18, 32). Clopidogrel, Cangrelor, and Ticagrelor have been used widely in clinic for the treatment of thrombogenesis by acting as P2RY12 antagonists, but some patients experienced side effects such as bleeding or recurrent ischemia (33). Interestingly, in our experiment, the injection of Rg5 could prolong the coagulation time compared with that of Cangrelor, but the bleeding time of Rg5 was shorter than that of Cangrelor (data not shown), suggesting that Rg5 might have less bleeding risk compared with Cangrelor. However, further research remains to be done in the future to confirm the effect.
Clopidogrel has been proven to inhibit the production of proinflammatory mediator in plasma, particularly IL-6, TNFα, and IL-1β, and reduce platelet–neutrophil interactions (18, 21, 34). However, whether P2RY12 plays an important role in the activation of neutrophils has not been clearly elucidated. A recent report demonstrated that, in the sepsis model of P2RY12-KO mice, there was no prominent increase of neutrophils in the serum and inflammation sites (35). However, there was no further investigation to disclose the underlying mechanism. In the present study, we demonstrated that both LPS and PAF stimulation enhanced inflammatory response and NET release in neutrophils; however, the effect of which was weakened in P2RY12-deficient neutrophils. These results implicated that P2RY12 is important for NETosis.
Rg5 has been shown to have multiple pharmacological activities, including anti-inflammatory, antitumor, neuroprotective, and cardioprotective properties (21). However, up to date, there is no report that clearly clarifies the direct molecular target. In our virtual screening, we found that Rg5 showed strong affinity with P2RY12, which was similar to Ticagrelor, a well-known P2RY12 antagonist. To confirm the binding, we conducted SPR assay and MD simulation, respectively, which revealed that Rg5 could dose-dependently bind to P2RY12 and form stable complex rapidly. Moreover, site-mutation analysis exposed that two amino acid residues E188 and R265, which did not directly interact with 2MesADP (36), were critical sites for the binding of Rg5 to P2RY12 and activation of downstream signaling. The roles of these two amino acids have been mentioned in previous studies, both of which were related to the activation of P2RY12 (37, 38). However, a study has also indicated that E188 and R265 were not the active sites for P2RY12; they mainly affect the conformational states of the protein (26). In agreement with the conjecture, the P2RY12 mutant constructed by homologous modeling suggested that the allosteric effect caused by mutations of E188 and R265 might account for the diminished antagonism of Rg5 to P2RY12. These results robustly corroborated the direct binding and antagonic activity of Rg5 to P2RY12.
Platelet aggregation and inflammation are related to the pathogenesis of DVT. During the occurrence of DVT in clinic, slow or restricted blood flow in veins leads to damage of venous endothelial cells due to hypoxia and further releases cytokines such as inflammatory factors, PAF, and chemokines, which induces the activation and aggregation of platelets and white blood cells. When systemic or local infection occurs, in addition to similar responses described above, platelets and leukocytes activate rapidly in response to direct pathogen stimulation, leading to a significantly increased risk of DVT (39). Activated platelets are recruited and transported to the venous wall where they attach directly to endothelial cells or to leukocytes to form heterogeneous aggregates, which is a critical step in DVT initiation (40). P2RY12 inhibitors Ticagrelor and Clopidogrel have been shown to significantly reduce the formation of platelet–neutrophil or platelet–monocyte aggregates and improve systemic inflammatory responses (41). The process of thrombosis is usually accompanied by the transformation of acute inflammation to chronic inflammation, and inflammation is closely related to DVT (42, 43). Recent studies have found that inflammation factors like IL-6, IL-1, and TNF-α were elevated in both DVT patients and mice (44, 45) and activated platelets and coagulation system, which further promoted thrombosis (46, 47). Neutrophils are essential in the development of thrombotic inflammation in DVT. In the DVT mouse model, 1 h after inferior vena cava stenosis, white blood cells began to appear and adhere to the venous endothelium. Six hours later, white blood cells covered the entire endothelial surface, of which more than 80% were neutrophils and the remaining 20% were monocytes. Moreover, when neutrophils are eliminated, DVT formation will be inhibited, indicating that the importance of neutrophils in DVT occurrence cannot be ignored (48). Activated neutrophils release NETs, which were found in the plasma and thrombus of patients with DVT (49, 50). Although monocytes also release extracellular traps (51), studies showed that neutrophils were the source of these extracellular traps (48), and a large number of NETs were also found in the thrombosis model (52). Meanwhile, treatment with DNase 1, known to degrade NETs, reduced the frequency of thrombosis (53), indicating that neutrophils and NETs in DVT are functionally important. In this study, we found that Rg5 inhibits DVT formation by reducing the release of inflammatory cytokines and the expression of NET biomarker CitH3 in mice. We induced bacterial and aseptic inflammation in vitro with LPS and PAF, respectively, and found that Rg5 reduced the inflammatory response of neutrophils by inhibiting P2RY12. This study focused only on the antagonistic effect of Rg5 against P2RY12 of neutrophils, rather than that of the platelets or other blood cells, like monocytes. However, Rg5 has been reported to have a significant anti-platelet aggregation effect (54). Combined with the fact that Rg5 can inhibit the phosphorylation of AKT and ERK signals downstream of P2RY12 and antagonize its activity, we speculate that Rg5 can inhibit platelet activation and platelet–white blood cell interaction by targeting P2RY12. Therefore, we believe that Rg5 may be an ideal treatment option for DVT.
P2RY12, as a member of the GPCR family, transmits signals mainly through two pathways: one modulates the cAMP level and PI3K/AKT pathway by binding to Gi protein, and the other influences Mitogen activated protein kinases (MAPK) signaling pathways such as ERK pathway by binding to β-arrestin or Gi protein (55). Previous studies have reported that the transcription and expression of inflammatory cytokines such as TNF-α, IL-1β, and IL-6 were related to the phosphorylation of NF-κB signal (56), which was affected by ERK pathway (57). PAD4 is highly expressed in neutrophil nuclei and relies on calcium mobilization to catalyze the conversion of several arginine sites on histones into citrulline (58), which plays an important role in the formation of NETs (59, 60). It has also been found that Rg5 can play an anti-inflammatory role by interfering NLRP3 signaling pathway and reducing inflammatory cytokines, whereas PAD4 also has the ability to upregulate NLRP3 inflammatory granules through post-transcription (61). Meanwhile, the activation of P2RY12 by ADP is closely associated with NLRP3- and NF-κB–mediated inflammatory responses (62). Therefore, on the basis of the inhibitory effect of Rg5 on P2RY12 activation in this study, the interference of Rg5 on NLRP3 signaling via P2RY12 may also explain the inhibitory effect of Rg5 on neutrophil inflammation. In the present study, Rg5 was found to inhibit ERK/NF-κB activation and inflammatory factor production in neutrophils induced by both LPS and PAF. Meanwhile, Rg5 restrained calcium influx, PAD4 activity, and NET release in PAF/LPS-induced neutrophils. However, in P2RY12 deficiency neutrophils, the suppressive effects of Rg5 on the aforementioned parameters were diminished. These results implicated that Rg5 modulated ERK/NF-κB and cAMP/PAD4 signaling pathway in neutrophils depending on P2RY12.
In summary, this study demonstrated that Rg5 as a natural P2RY12 antagonist could inhibit NETosis during thrombosis to slow down the formation of DVT. Our study provides a theoretical basis for the clinical application of Rg5 for the prevention of DVT.
The raw data supporting the conclusions of this article will be made available by the authors, without undue reservation.
The animal study was reviewed and approved by Shanghai University of Traditional Chinese Medicine.
ZC, GW, and XX performed the experiments, analyzed the data, and wrote the paper. ZW and XW designed the study. HL, JL, and HS participated in its design and helped to draft the manuscript. MC and SL gave valuable suggestions about the study. All authors contributed to the article and approved the submitted version.
This work was supported by Shanghai Municipal Natural Science Foundation (22ZR1461100). Major International (Regional) Joint Research Project of NSFC (81920108033), Guangxi Science and Technology Base and Talent Special Project (Guike AD20297068).
The authors declare that the research was conducted in the absence of any commercial or financial relationships that could be construed as a potential conflict of interest.
All claims expressed in this article are solely those of the authors and do not necessarily represent those of their affiliated organizations, or those of the publisher, the editors and the reviewers. Any product that may be evaluated in this article, or claim that may be made by its manufacturer, is not guaranteed or endorsed by the publisher.
1. Badireddy M, Mudipalli VR. Deep venous thrombosis prophylaxis. In: StatPearls. Treasure Island (FL: StatPearls Publishing (2021).
2. Bevis PM, Smith FC. Deep vein thrombosis. Surg (Oxford) (2016) 34:159–64. doi: 10.1016/j.mpsur.2016.02.001
3. Diana Yap FS, Ng ZY, Wong CY, Muhamad Saifuzzaman MK, Yang LB. Appropriateness of deep vein thrombosis (DVT) prophylaxis use among medical inpatients: a DVT risk alert tool (DRAT) study. Med J Malaysia (2019) 74:45–50.
4. Kollias A, Kyriakoulis KG, Lagou S, Kontopantelis E, Stergiou GS, Syrigos K. Venous thromboembolism in COVID-19: A systematic review and meta-analysis. Vasc Med (2021) 26:415–25. doi: 10.1177/1358863X21995566
5. Lichota A, Szewczyk EM, Gwozdzinski K. Factors affecting the formation and treatment of thrombosis by natural and synthetic compounds. Int J Mol Sci (2020) 21:7975. doi: 10.3390/ijms21217975
6. Weitz JI, Chan NC. Novel antithrombotic strategies for treatment of venous thromboembolism. Blood (2020) 135:351–9. doi: 10.1182/blood.2019000919
7. Pfeiler S, Stark K, Massberg S, Engelmann B. Propagation of thrombosis by neutrophils and extracellular nucleosome networks. Haematologica (2017) 102:206–13. doi: 10.3324/haematol.2016.142471
8. Fuchs TA, Brill A, Duerschmied D, Schatzberg D, Monestier M, Myers DD Jr, et al. Extracellular DNA traps promote thrombosis. Proc Natl Acad Sci USA (2010) 107:15880–5. doi: 10.1073/pnas.1005743107
9. Urban CF, Ermert D, Schmid M, Abu-Abed U, Goosmann C, Nacken W, et al. Neutrophil extracellular traps contain calprotectin, a cytosolic protein complex involved in host defense against candida albicans. PloS Pathog (2009) 5:e1000639. doi: 10.1371/journal.ppat.1000639
10. Papayannopoulos V. Neutrophil extracellular traps in immunity and disease. Nat Rev Immunol (2018) 18:134–47. doi: 10.1038/nri.2017.105
11. Qutub MA, Chong AY, So DY. Current evidence on platelet P2Y12 receptor inhibitors: Is there still a role for clopidogrel in 2015? Can J Cardiol (2015) 31:1481–4. doi: 10.1016/j.cjca.2015.04.019
12. Niu H, Chen X, Gruppo RA, Li D, Wang Y, Zhang L, et al. Integrin αIIb-mediated PI3K/Akt activation in platelets. PloS One (2012) 7:e47356. doi: 10.1371/journal.pone.0047356
13. Guidetti GF, Canobbio I, Torti M. PI3K/Akt in platelet integrin signaling and implications in thrombosis. Adv Biol Regul (2015) 59:36–52. doi: 10.1016/j.jbior.2015.06.001
14. Kim S, Kunapuli SP. P2Y12 receptor in platelet activation. Platelets (2011) 22:56–60. doi: 10.3109/09537104.2010.497231
15. Speich HE, Bhal V, Houser KH, Caughran AT, Lands LT, Houng AK, et al. Signaling via P2Y12 may be critical for early stabilization of platelet aggregates. J Cardiovasc Pharmacol (2014) 63:520–7. doi: 10.1097/FJC.0000000000000076
16. Mansour A, Bachelot-Loza C, Nesseler N, Gaussem P, Gouin-Thibault I. P2Y12 inhibition beyond thrombosis: Effects on inflammation. Int J Mol Sci (2020) 21:1391. doi: 10.3390/ijms21041391
17. Guenther F, Herr N, Mauler M, Witsch T, Roming F, Hein L, et al. Contrast ultrasound for the quantification of deep vein thrombosis in living mice: effects of enoxaparin and P2Y12 receptor inhibition. J Thromb Haemost (2013) 11:1154–62. doi: 10.1111/jth.12206
18. Wang XL, Deng HF, Li T, Miao SY, Xiao ZH, Liu MD, et al. Clopidogrel reduces lipopolysaccharide-induced inflammation and neutrophil-platelet aggregates in an experimental endotoxemic model. J Biochem Mol Toxicol (2019) 33:e22279. doi: 10.1002/jbt.22279
19. Rahman M, Gustafsson D, Wang Y, Thorlacius H, Braun OÖ. Ticagrelor reduces neutrophil recruitment and lung damage in abdominal sepsis. Platelets (2014) 25:257–63. doi: 10.3109/09537104.2013.809520
20. Judge HM, Buckland RJ, Sugidachi A, Jakubowski JA, Storey RF. The active metabolite of prasugrel effectively blocks the platelet P2Y12 receptor and inhibits procoagulant and pro-inflammatory platelet responses. Platelets (2008) 19:125–33. doi: 10.1080/09537100701694144
21. Sexton TR, Zhang G, Macaulay TE, Callahan LA, Charnigo R, Vsevolozhskaya OA, et al. Ticagrelor reduces thromboinflammatory markers in patients with pneumonia. JACC Basic Transl Sci (2018) 3:435–49. doi: 10.1016/j.jacbts.2018.05.005
22. Liu MY, Liu F, Gao YL, Yin JN, Yan WQ, Liu JG, et al. Pharmacological activities of ginsenoside Rg5 (Review). Exp Ther Med (2021) 22:840. doi: 10.3892/etm.2021.10272
23. Zheng F, Zhou Q, Cao Y, Shi H, Wu H, Zhang B, et al. P2Y12 deficiency in mouse impairs noradrenergic system in brain, and alters anxiety-like neurobehavior and memory. Genes Brain Behav (2019) 18:e12458. doi: 10.1111/gbb.12458
24. Liao Q, Chen Z, Tao Y, Zhang B, Wu X, Yang L, et al. An integrated method for optimized identification of effective natural inhibitors against SARS-CoV-2 3CLpro. Sci Rep (2021) 11:22796. doi: 10.1038/s41598-021-02266-3
25. Palacio-Rodríguez K, Lans I, Cavasotto CN, Cossio P. Exponential consensus ranking improves the outcome in docking and receptor ensemble docking. Sci Rep (2019) 9:5142. doi: 10.1038/s41598-019-41594-3
26. Zhang K, Zhang J, Gao ZG, Zhang D, Zhu L, Han GW, et al. Structure of the human P2Y12 receptor in complex with an antithrombotic drug. Nature (2014) 509:115–8. doi: 10.1038/nature13083
27. Miller BR 3rd, McGee TD Jr, JM S, Homeyer N, Gohlke H, Roitberg AE. MMPBSA.py: An efficient program for end-state free energy calculations. J Chem Theory Comput (2012) 8:3314–21. doi: 10.1021/ct300418h
28. Xu Q, Chen Z, Zhu B, Wang G, Jia Q, Li Y, et al. A-type cinnamon procyanidin oligomers protect against 1-Methyl-4-Phenyl-1,2,3,6-Tetrahydropyridine-Induced neurotoxicity in mice through inhibiting the P38 mitogen-activated protein Kinase/P53/BCL-2 associated X protein signaling pathway. J Nutr (2020) 150:1731–7. doi: 10.1093/jn/nxaa128
29. Wrobleski SK, Farris DM, Diaz JA, Myers DD Jr, Wakefield TW. Mouse complete stasis model of inferior vena cava thrombosis. J Vis Exp (2011) 52:2738. doi: 10.3791/2738
30. Yang L, Xing F, Han X, Li Q, Wu H, Shi H, et al. Astragaloside IV regulates differentiation and induces apoptosis of activated CD4+ T cells in the pathogenesis of experimental autoimmune encephalomyelitis. Toxicol Appl Pharmacol (2019) 362:105–15. doi: 10.1016/j.taap.2018.10.024
31. Varma MR, Varga AJ, Knipp BS, Sukheepod P, Upchurch GR, Kunkel SL, et al. Neutropenia impairs venous thrombosis resolution in the rat. J Vasc Surg (2003) 38:1090–8. doi: 10.1016/S0741-5214(03)00431-2
32. Bird JE, Wang X, Smith PL, Barbera F, Huang C, Schumacher WA. A platelet target for venous thrombosis? P2Y1 deletion or antagonism protects mice from vena cava thrombosis. J Thromb Thrombolysis (2012) 34:199–207. doi: 10.1007/s11239-012-0745-3
33. Terrier J, Daali Y, Fontana P, Csajka C, Reny JL. Towards personalized antithrombotic treatments: Focus on P2Y12 inhibitors and direct oral anticoagulants. Clin Pharmacokinet (2019) 58:1517–32. doi: 10.1007/s40262-019-00792-y
34. Xiao Z, Théroux P. Clopidogrel inhibits platelet-leukocyte interactions and thrombin receptor agonist peptide-induced platelet activation in patients with an acute coronary syndrome. J Am Coll Cardiol (2004) 43:1982–8. doi: 10.1016/j.jacc.2003.10.071
35. Liverani E, Rico MC, Tsygankov AY, Kilpatrick LE, Kunapuli SP. P2Y12 receptor modulates sepsis-induced inflammation. Arterioscler Thromb Vasc Biol (2016) 36:961–71. doi: 10.1161/ATVBAHA.116.307401
36. Zhang J, Zhang K, Gao ZG, Paoletta S, Zhang D, Han GW, et al. Agonist-bound structure of the human P2Y12 receptor. Nature (2014) 509:119–22. doi: 10.1038/nature13288
37. Ignatovica V, Megnis K, Lapins M, Schiöth HB, Klovins J. Identification and analysis of functionally important amino acids in human purinergic 12 receptor using a saccharomyces cerevisiae expression system. FEBS J (2012) 279:180–91. doi: 10.1111/j.1742-4658.2011.08410.x
38. Schmidt P, Ritscher L, Dong EN, Hermsdorf T, Cöster M, Wittkopf D, et al. Identification of determinants required for agonistic and inverse agonistic ligand properties at the ADP receptor P2Y12. Mol Pharmacol (2013) 83:256–66. doi: 10.1124/mol.112.082198
39. Beristain-Covarrubias N, Perez-Toledo M, Thomas MR, Henderson IR, Watson SP, Cunningham AF. Understanding infection-induced thrombosis: Lessons learned from animal models. Front Immunol (2019) 10:2569. doi: 10.3389/fimmu.2019.02569
40. Diaz JA, Obi AT, Myers DD Jr, Wrobleski SK, Henke PK, Mackman N, et al. Critical review of mouse models of venous thrombosis. Arterioscler Thromb Vasc Biol (2012) 32:556–62. doi: 10.1161/ATVBAHA.111.244608
41. Thomas MR, Outteridge SN, Ajjan RA, Phoenix F, Sangha GK, Faulkner RE, et al. Platelet P2Y12 inhibitors reduce systemic inflammation and its prothrombotic effects in an experimental human model. Arterioscler Thromb Vasc Biol (2015) 35:2562–70. doi: 10.1161/ATVBAHA.115.306528
42. Branchford BR, Carpenter SL. The role of inflammation in venous thromboembolism. Front Pediatr (2018) 6:142. doi: 10.3389/fped.2018.00142
43. Vazquez-Garza E, Jerjes-Sanchez C, Navarrete A, Joya-Harrison J, Rodriguez D. Venous thromboembolism: thrombosis, inflammation, and immunothrombosis for clinicians. J Thromb Thrombolysis (2017) 44:377–85. doi: 10.1007/s11239-017-1528-7
44. Candido S, Lumera G, Barcellona G, Vetri D, Tumino E, Platania I, et al. Direct oral anticoagulant treatment of deep vein thrombosis reduces IL-6 expression in peripheral mono-nuclear blood cells. Exp Ther Med (2020) 20:237. doi: 10.3892/etm.2020.9367
45. Meng Y, Yin Q, Ma Q, Qin H, Zhang J, Zhang B, et al. FXII regulates the formation of deep vein thrombosis via the PI3K/AKT signaling pathway in mice. Int J Mol Med (2021) 47:87. doi: 10.3892/ijmm.2021.4920
46. Zhang Y, Zhang Z, Wei R, Miao X, Sun S, Liang G, et al. IL (Interleukin)-6 contributes to deep vein thrombosis and is negatively regulated by miR-338-5p. Arterioscler Thromb Vasc Biol (2020) 40:323–34. doi: 10.1161/ATVBAHA.119.313137
47. Bester J, Pretorius E. Effects of IL-1β, IL-6 and IL-8 on erythrocytes, platelets and clot viscoelasticity. Sci Rep (2016) 6:32188. doi: 10.1038/srep32188
48. von Brühl ML, Stark K, Steinhart A, Chandraratne S, Konrad I, Lorenz M, et al. Monocytes, neutrophils, and platelets cooperate to initiate and propagate venous thrombosis in mice in vivo. J Exp Med (2012) 209:819–35. doi: 10.1084/jem.20112322
49. Liu L, Zhang W, Su Y, Chen Y, Cao X, Wu J. The impact of neutrophil extracellular traps on deep venous thrombosis in patients with traumatic fractures. Clin Chim Acta (2021) 519:231–8. doi: 10.1016/j.cca.2021.04.021
50. van Montfoort ML, Stephan F, Lauw MN, Hutten BA, Van Mierlo GJ, Solati S, et al. Circulating nucleosomes and neutrophil activation as risk factors for deep vein thrombosis. Arterioscler Thromb Vasc Biol (2013) 33:147–51. doi: 10.1161/ATVBAHA.112.300498
51. Granger V, Faille D, Marani V, Noël B, Gallais Y, Szely N, et al. Human blood monocytes are able to form extracellular traps. J Leukoc Biol (2017) 102:775–81. doi: 10.1189/jlb.3MA0916-411R
52. Campos J, Ponomaryov T, De Prendergast A, Whitworth K, Smith CW, Khan AO, et al. Neutrophil extracellular traps and inflammasomes cooperatively promote venous thrombosis in mice. Blood Adv (2021) 5:2319–24. doi: 10.1182/bloodadvances.2020003377
53. Brill A, Fuchs TA, Savchenko AS, Thomas GM, Martinod K, De Meyer SF, et al. Neutrophil extracellular traps promote deep vein thrombosis in mice. J Thromb Haemost (2012) 10:136–44. doi: 10.1111/j.1538-7836.2011.04544.x
54. Lee JG, Lee YY, Kim SY, Pyo JS, Yun-Choi HS, Park JH. Platelet antiaggregating activity of ginsenosides isolated from processed ginseng. Pharmazie (2009) 64:602–4.
55. Cattaneo M. P2Y12 receptors: structure and function. J Thromb Haemost (2015) 13(Suppl 1):S10–S16. doi: 10.1111/jth.12952
56. Lawrence T. The nuclear factor NF-kappaB pathway in inflammation. Cold Spring Harb Perspect Biol (2009) 1:a001651. doi: 10.1101/cshperspect.a001651
57. Lu CH, Li KJ, Wu CH, Shen CY, Kuo YM, Hsieh SC, et al. The FcγRIII engagement augments PMA-stimulated neutrophil extracellular traps (NETs) formation by granulocytes partially via cross-talk between syk-ERK-NF-κB and PKC-ROS signaling pathways. Biomedicines (2021) 9:1127. doi: 10.3390/biomedicines9091127
58. Thiam HR, Wong SL, Qiu R, Kittisopikul M, Vahabikashi A, Goldman AE, et al. NETosis proceeds by cytoskeleton and endomembrane disassembly and PAD4-mediated chromatin decondensation and nuclear envelope rupture. Proc Natl Acad Sci USA (2020) 117:7326–37. doi: 10.1073/pnas.1909546117
59. Li P, Li M, Lindberg MR, Kennett MJ, Xiong N, Wang Y. PAD4 is essential for antibacterial innate immunity mediated by neutrophil extracellular traps. J Exp Med (2010) 207:1853–62. doi: 10.1084/jem.20100239
60. Tan C, Aziz M, Wang P. The vitals of NETs. J Leukoc Biol (2021) 110:797–808. doi: 10.1002/JLB.3RU0620-375R
61. Münzer P, Negro R, Fukui S, di Meglio L, Aymonnier K, Chu L, et al. NLRP3 inflammasome assembly in neutrophils is supported by PAD4 and promotes NETosis under sterile conditions. Front Immunol (2021) 12:683803. doi: 10.3389/fimmu.2021.683803
Keywords: deep venous thrombosis, ginsenoside Rg5, P2RY12, neutrophil extracellular traps, inflammation
Citation: Chen Z, Wang G, Xie X, Liu H, Liao J, Shi H, Chen M, Lai S, Wang Z and Wu X (2022) Ginsenoside Rg5 allosterically interacts with P2RY12 and ameliorates deep venous thrombosis by counteracting neutrophil NETosis and inflammatory response. Front. Immunol. 13:918476. doi: 10.3389/fimmu.2022.918476
Received: 12 April 2022; Accepted: 21 July 2022;
Published: 12 August 2022.
Edited by:
Clifford Taggart, Queen’s University Belfast, United KingdomReviewed by:
Girish S Kesturu, Tumkur University, IndiaCopyright © 2022 Chen, Wang, Xie, Liu, Liao, Shi, Chen, Lai, Wang and Wu. This is an open-access article distributed under the terms of the Creative Commons Attribution License (CC BY). The use, distribution or reproduction in other forums is permitted, provided the original author(s) and the copyright owner(s) are credited and that the original publication in this journal is cited, in accordance with accepted academic practice. No use, distribution or reproduction is permitted which does not comply with these terms.
*Correspondence: Xiaojun Wu, eGlhb2p1bnd1MzIwQDEyNi5jb20=; Zhengtao Wang, enR3YW5nQHNodXRjbS5lZHUuY24=
Disclaimer: All claims expressed in this article are solely those of the authors and do not necessarily represent those of their affiliated organizations, or those of the publisher, the editors and the reviewers. Any product that may be evaluated in this article or claim that may be made by its manufacturer is not guaranteed or endorsed by the publisher.
Research integrity at Frontiers
Learn more about the work of our research integrity team to safeguard the quality of each article we publish.