- 1Immunology Programme, Life Sciences Institute, National University of Singapore, Singapore, Singapore
- 2Immunology Translational Research Program and Department of Microbiology and Immunology, Yong Loo Lin School of Medicine, National University of Singapore, Singapore, Singapore
γδT cells represent a small percentage of T cells in circulation but are found in large numbers in certain organs. They are considered to be innate immune cells that can exert cytotoxic functions on target cells without MHC restriction. Moreover, γδT cells contribute to adaptive immune response via regulating other immune cells. Under the influence of cytokines, γδT cells can be polarized to different subsets in the tumor microenvironment. In this review, we aimed to summarize the current understanding of antigen recognition by γδT cells, and the immune regulation mediated by γδT cells in the tumor microenvironment. More importantly, we depicted the polarization and plasticity of γδT cells in the presence of different cytokines and their combinations, which provided the basis for γδT cell-based cancer immunotherapy targeting cytokine signals.
Characteristics and Antigen Recognition of γδT Cells
Although γδT cells share the same progenitors with conventional αβT cells and develop in the thymus, they are considered as innate immune cells due to their major histocompatibility complex (MHC) unrestricted antigen recognition, as well as the expressions of Natural Killer Receptors (NKRs) and Toll-like Receptors (TLRs) along with rapid cytokine production. The majority of γδT cells are negative for CD4 and CD8. In both human and mice, γδT cells account for 5% of total peripheral T cells.
Based on the TCR δ chain usage, human γδT cells can be subtyped to Vδ1, Vδ2, Vδ3 and Vδ5 cells (Table 1). Vδ1 and Vδ2 are the major subsets, which are of great interest among human γδT cells. Human Vδ2 cells are generally paired with T cell receptor (TCR) γ9, also named as Vγ9Vδ2 cells. Vγ9Vδ2 cells are the dominant γδT subset in human peripheral blood mononuclear cells (PBMCs). Vγ9Vδ2 TCRs recognize phosphoantigens (PAgs) such as isopentenyl pyrophosphate (IPP), which is accumulated in tumor cells, and (E)-4-hydroxy-3- methyl-but-2-enyl pyrophosphate (HMBPP) that is produced during microbial infections (Figure 1A). Interestingly, although γδT cells bind PAgs in the MHC independent manner, PAgs-mediated activation of Vγ9Vδ2 requires butyrophilin (BTN) and BTN-like molecules (1). Recent studies reported that BTN2A1 associated with BTN3A1 to initiate antigen-presentation to Vγ9Vδ2 T cells (2, 3). Besides TCR-associated antigen recognition, Vγ9Vδ2 T cells also express NK receptors including NKG2D and DNAM1, which recognize MHC class I chain-related molecules (MICA, MICB), ULBP-binding proteins (ULBPs) and Nectin-like-5 that are broadly expressed on tumor cells (4).
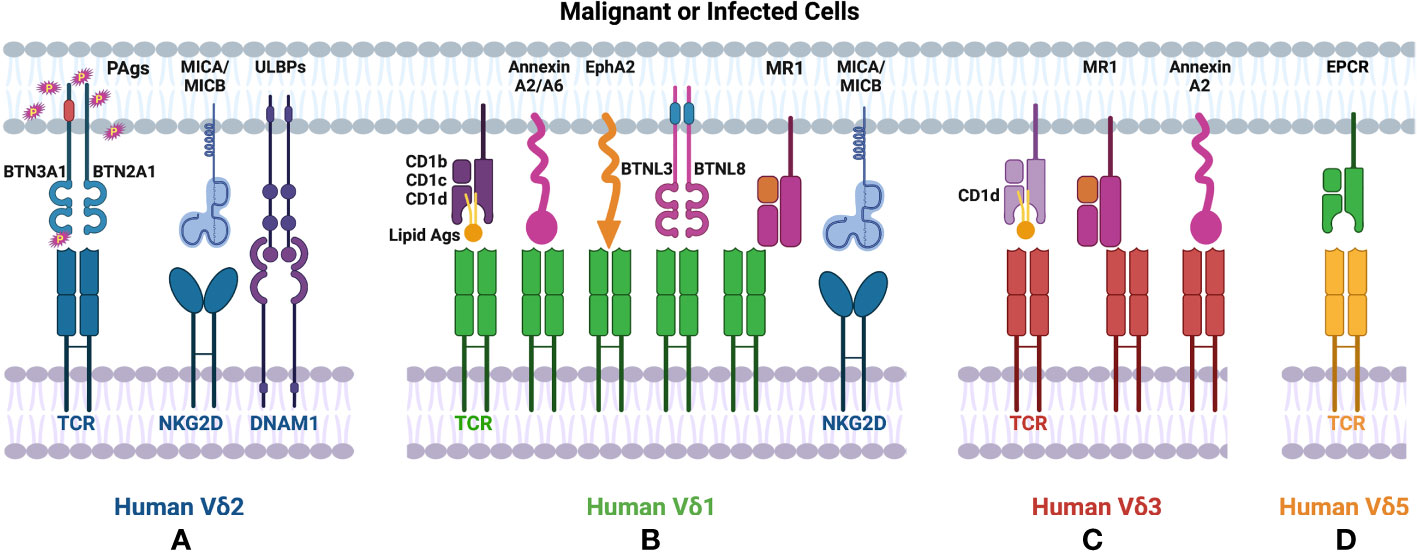
Figure 1 Ligands recognized by human γδT cells. (A) Human Vδ2 T cells recognize PAgs via TCR in a BTN molecule dependent manner. (B) TCRs of human Vδ1 cells recognize lipid antigens presented by CD1. Human Vδ1 also binds to Annexin A2/A6, EphA2, MR1 in an antigen-independent manner. (A, B) Both human Vδ1 and Vδ2 T cells express NKRs (such as NKG2D, DNAM1), which bind to MICA/MICB, ULBPs expressed on tumor cells. (C) Human Vδ3 cells interact with CD1d with/without antigen via TCR, also recognize Annexin A2 or MR1 without antigen loading. (D) Human Vd5 cells bind to EPCR via TCR.
Human Vδ1 cells are mainly distributed in epithelial tissues, such as skin, gut, spleen, and liver. Human Vδ1 cells constitute only up to 15% of human γδT cells in PBMCs (5), but they exhibit fast and marked expansion during CMV infections (6). γδT cell compartment involved in HCMV-specific response is non-Vγ9Vδ2 T cells with the TCRVδ1+ lymphocytes representing the prominent non-Vγ9Vδ2 γδ T cell subset (7–9). Furthermore, these Vδ1 cells display a mixed CD27-/CD45RA+ or CD27+/CD45RA+ phenotype that is identified as cytotoxic effector/memory populations in CMV+ individuals (10). These findings indicated the potential immune surveillance function of Vδ1 cells. Whereas the TCRγ chains paired with Vδ1 display high diversity and the antigens recognized by Vδ1 cells are not well revealed, it has been shown that CD1 molecules with or without loaded lipid antigens can specifically activate Vδ1 cells. The direct interactions between Vδ1 and CD1b, CD1c, or CD1d have been identified by CD1 tetramers, mutagenesis experiments and crystal structures (11–15). In addition to CD1-associated recognition, Vγ4Vδ1 cells have been reported to respond to BTNL3 and BTNL8 expressing cells via Vγ4 chain (Figure 1B) (16). Annexin A2 and Annexin A6 that are known as stress-induced phospholipid-binding proteins and involved in tumorigenesis also stimulated the proliferation and the production of TNF-α in Vγ4Vδ1 cells (17). Another newly identified stress-induced antigen that is recognized by Vδ1 TCR is ephrin type-A receptor 2 (EphA2) (Figure 1B), which is upregulated upon AMP-activated protein kinase (AMPK)-dependent metabolic reprogramming of cancer cells. It can be recognized co-ordinately by ephrin A to govern the activation of Vγ9Vδ1 cells (18). The involvement of EphA2 in Vδ1-mediated tumor cell lysis was demonstrated by reduced susceptibility to killing by EphA2 blocking (19). Human Vδ1 cells from peripheral blood and tissues exhibit autoreactivity to the monomorphic MHC-related protein 1 (MR1) without binding with any ligands, indicating MR1 as a ligand of Vδ1 γδTCR (20). Similar to Vδ2 cells, Vδ1 cells also mediate tumor cell lysis through recognizing ULBP3 and MICA by NKG2D (Figures 1A, B) (21–23).
Vδ3 cells account for ~0.2% of lymphocytes in PBMCs from healthy donors but are enriched in the liver and gut and can be expanded in patients with CMV activation and B cell chronic lymphocytic leukemia (24, 25). Human Vδ3 cells were identified as CD1d-restricted T cells and can mediate specific killing against CD1d+ cells (Figure 1C). Different from Vδ1 cells, Vδ3 cells can not recognize other CD1 molecules (such as CD1b,CD1c) (26). Annexin A2 was identified as the direct ligand of Vγ8Vδ3 TCR (Figure 1C) (17). Recently, human Vδ3 cells have also been shown to bind to MR1 in an antigen-independent manner (Figure 1C). Another notable population of human γδT cells is Vδ5 subset. Human Vγ4Vδ5 T cells were reported to bind directly with endothelial protein C receptor (EPCR) (Figure 1D), which is a MHC-like molecule and binds to phospholipid (27). However, the phospholipid binding is not required for the recognition between human Vδ5 cells and EPCR (28).
Taken together, in contrast to αβT cells and other unconventional T cells, such as NKT and MAIT cells, human γδT cells usually recognize specific molecules in an antigen-independent manner except for Vδ2 cells. For example, Vδ1 and Vδ3 TCRs bind to the underside of MR1 and the side of the MR1 antigen-binding groove respectively. Vδ1 cells also respond to CD1 without the loading of lipid antigens. Vδ5 cells recognize EPCR without the involvement of antigens. Other than the recognition of these MHC-like structures in the absence of antigens, Vδ1 TCR can also interact with Annexin A2 and A6 and Vδ3 TCR can recognize Annexin A2 in an Ig-like manner.
With regard to murine γδT cells, they are generally grouped by the usage of TCR γ chains (Table 1). Vγ1 and Vγ4 are the predominant subsets in the splenic and circulating γδT cells (29). They are located in many mouse tissues. Vγ5 is invariably paired with Vδ1 and the Vγ5Vδ1 cells are found in dermis and are also named as dendritic epidermal T cells (DETC) (30). Vγ6 cells are mainly paired with Vδ1 or Vδ4 and can home to the mucosa of reproductive tissues and skin (30–32). Vγ7 cells are restricted to intestinal epithelial lymphocytes (33). However, the recognition of PAgs of γδTCR was not found in mouse. Only limited studies reported antigens recognized by murine γδT cells, such as H2−T10, H2−T22, and algae protein phycoerythrin (PE) (34–37). A recent study found that BTNL molecules shape the local Vγ7 and Vγ5 compartments in murine intestinal epithelium and skin (16, 38). The requirement of BTNL during the selection and maintenance of tissue-resident γδT cells indicates the potential interaction between γδTCR and BTNL. However, it is still not clear how the murine and human γδT cell subsets can be matched with each other, and it is difficult to translate some of the findings with murine γδT cells directly to human.
Anti-Tumor and Pro-Tumor Functions of γδT Cells Mediated by Cytokines and Receptor-Ligand Interactions
After the recognition of antigens or other stress-induced molecules expressed on tumor cells by TCR or NKR, γδT cells can mediate the direct tumor lysis by producing granzyme B, perforin, TNF-α and IFN-γ (Figure 2: top right) (39, 40). For example, human Vγ9Vδ2 T cells induced human hepatocellular carcinoma cell lysis in a DNAM-1-dependent manner (4). IL-17 produced by γδT17 cells significantly inhibited tumor development in mice and patients with lung cancer (41, 42). Additionally, activated γδT cells also express death induced ligands CD95L (also known as FasL) and TNF-related apoptosis-inducing ligand (TRAIL), which engage with death receptor CD95 (Fas) and TRAIL receptor, and apoptosis of infected or malignant cells (43–45). Similar to NK cells, the majority of γδT cells in peripheral blood express CD16. CD16 acts as an activation site triggering antibody dependent cellular cytotoxicity (ADCC) (Figure 2: top right) (46). A recent study showed that exosomes derived from human Vγ9Vδ2T cells (γδT-Exos) efficiently induced the apoptosis of tumor cells through death receptor ligation (Figure 2: top right) (47, 48).
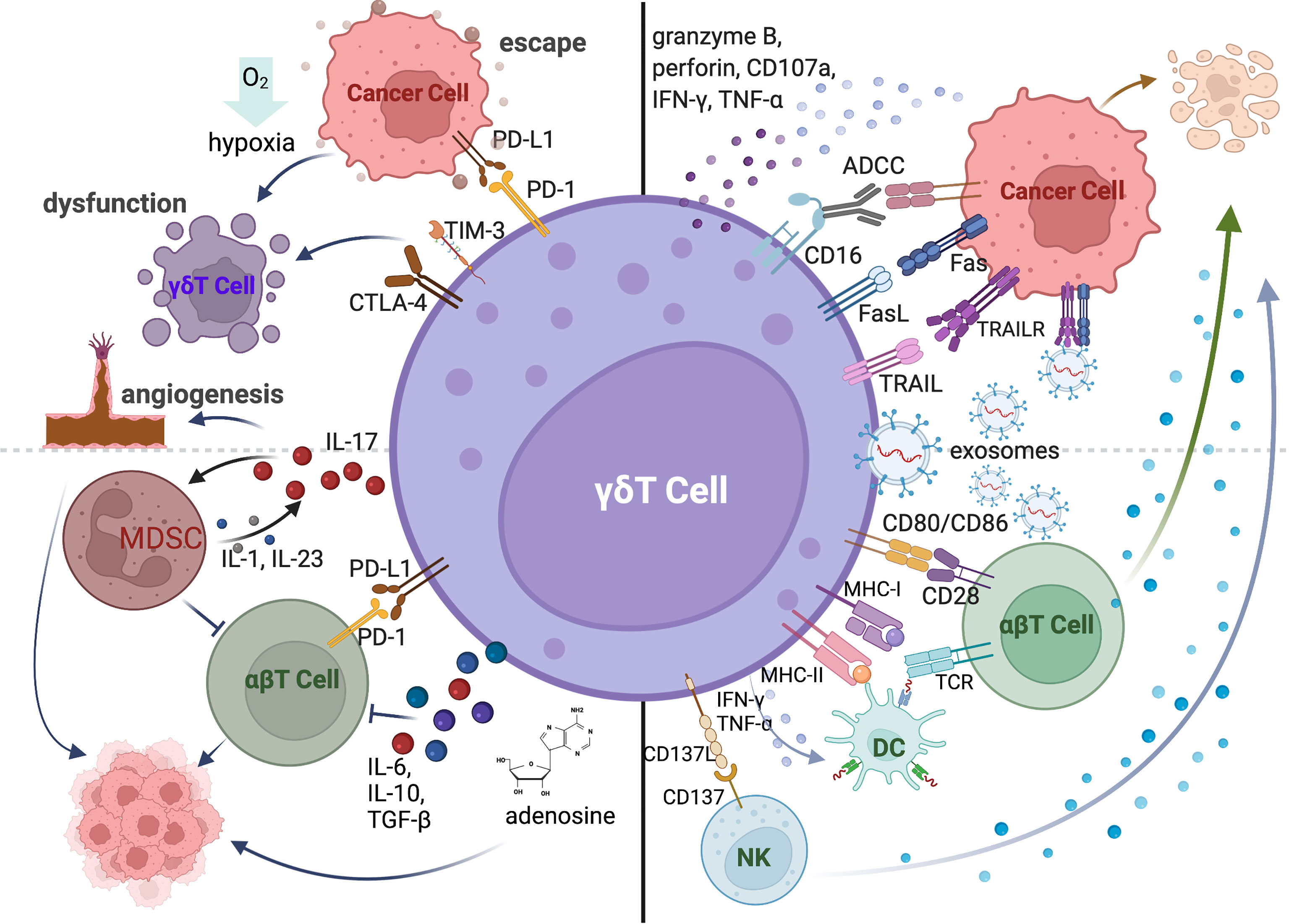
Figure 2 The anti-tumor and pro-tumor functions of γδT cells mediated by cytokines and receptor-ligand interactions. γδT cells can directly kill tumor cells by expressing death receptor ligands (FasL, TRAIL), producing cytotoxic molecules (granzyme B, perforin, CD107a, IFN-γ and TNF-α) and mediating ADCC via CD16 expression. The exosomes derived from γδT cells can also directly induce the apoptosis of cancer cells. γδT-APC can activate conventional T cells via MHC-I, MHC-II, and co-stimulatory molecules. γδT cells induce the maturation of DCs by secreting IFN-γ and TNF-α and trigger the activation of NK cells via CD137L. The pro-tumor function of γδT cells is mediated by the expression of co-inhibitory receptors. The co-inhibitory molecules contribute to tumor cell escape from immune surveillance. Hypoxic tumor microenvironment also induces the dysfunction of γδT cells. γδT cells also promote the tumor growth by recruiting immunosuppressive cells and inhibiting conventional T cells via producing IL-17, IL-6, IL-10, TGF-β or adenosine.
In addition to the direct killing against tumor cells, γδT cells can exert the indirect anti-tumor function by regulating other immune cells in the tumor microenvironment (Figure 2: bottom right). Human Vδ2 T cells are described as professional antigen-presenting cells, which can process antigens and provide co-stimulatory signals to induce the proliferation and differentiation of αβT cells (49). It is also reported that human γδT-APCs efficiently cross-present soluble antigens to CD8+T cells via MHC-I (50, 51). The high expression levels of APC-associated molecules and tumor antigen presenting capability of in vitro expanded human Vγ9Vδ2 T cells were also detected during the early stage of differentiation (52). Activated human γδT cells boost NK cell mediated killing of tumor cells through CD137L (53).
Besides ligand-receptor interactions, cytokine production is the pivotal pathway to regulate other immune cells. Like conventional T cells, γδT cells can be polarized to different subsets based on the secreted cytokines, including IFN-γ-producing γδT cells (γδT-IFN or γδT1), IL-4-producing γδT cells (γδT2), IL-17-producing γδT cells (γδT17) and Foxp3+ regulatory γδT cells (γδTreg). These cytokine-producing γδT cells exist in both human and mouse and can regulate other immune cell functions via their signature cytokine productions (Figure 2: bottom right). For instance, activated γδT1 cells promoted the maturation of DCs via IFN-γ dependent manner in mouse (54). Human freshly isolated γδT1 cells also induced the upregulation of HLA-DR, CD86, CD83 and release of IFN-γ, IL-6, and TNF-α of monocyte-derived DCs through the production of TNF-α and IFN-γ (55, 56). Both human Vδ2 and Vδ3 cells can promote B cell differentiation, antibody maturation and cytokine production (25, 55). IL-4 producing mouse Vγ1Vδ6 T cells can drive the proliferation and IgA secretion of Germinal Centre (GC) B cells (57). In additions, γδT17 cells promoted the infiltration of CTLs within the tumor bed via IL-17 production after chemotherapy (58).
Although the anti-tumor functions of γδT cells have been shown in many murine models and in cancer patients, the pro-tumor activities of γδT cells were also reported in numerous studies (Figure 2: left). Co-inhibitory molecules can be upregulated on human and murine γδT cells in tumors, which can bind to the co-inhibitory receptors expressed on αβT cells to restrain their activation, infiltration, and anti-tumor efficiency (59). The expressions of PD-1, TIM3 and TIGIT also induced the exhaustion and dysfunction of γδT cells in AML and MM patients (60). Moreover, co-inhibitory receptors on γδT cells contribute to the tumor immune escape by interaction with immunosuppressive molecules (Figure 2: top left) (61). Meanwhile, hypoxic tumor microenvironment induced by metabolic status of cancer cells is a critical factor in mediating immunosuppression. The anti-tumor function of γδT cells can be inhibited by hypoxia via the downregulation of NKG2D and CD107a expressions (62, 63). Over the past decade, IL-17-producing γδT cells have been found to associate with enhanced tumor growth and metastasis. γδT is one of the major sources of IL-17 in the tumor microenvironment and reduced tumor burden was observed in IL-17-producing Vγ4-depleted and IL-17-deficient mice (64). γδT17 cells recruit myeloid-derived suppressor cells (MDSCs) to the tumor site, which can suppress CD8+T cell responses (64, 65). Consistently, this is also demonstrated in human colorectal cancer (66). In addition, IL-17-produing γδT cells can accelerate tumor progression by promoting angiogenesis and mobilizing pro-tumor macrophages (67, 68). γδTreg cells were found to impair DC maturation and function and CD8+T cell-mediated anti-tumor function in cancer patients via TGF-β, IL-6 or IL-10 dependent or independent manner (69, 70). Moreover, CD39+ γδTregs were implicated in the immunosuppressive environment via producing adenosine in human colorectal cancer (71). The IL-6-adenosine positive feedback loop between CD73+ γδTregs and cancer-associated fibroblast (CAF) was also involved in tumor progression in breast cancer patients (72).
The role of γδT cells during tumor development is still controversial. Their functions could be cancer type specific. For example, human Vδ1 cells exhibit potent cytotoxicity against colon cancer cells and B-cell chronic lymphocytic leukemia (73, 74), whereas Vδ2 cells are shown to kill a wide variety of tumors including acute myeloid leukemia, multiple myeloma and lung cancer (60, 75). On the other hand, some γδT subsets may exert different functions in the same type of cancer under different treatment conditions/environment. γδT17 cells promoted CTL infiltration into colon cancer after chemotherapy (58), whereas they have been reported to inhibit anti-tumor immune response via promoting the recruitment, proliferation, and survival of MDSCs in colorectal cancer and hepatocellular carcinoma (66). Therefore, γδT cell function during tumor development may be greatly influenced by the cytokines present in the tumor microenvironment under specific conditions.
Cytokine-Mediated Regulation of γδT Cell Function
IL-2 is the commonly used cytokine for expanding human and murine γδT cells. IL-2 is identified as T cell growth factor and is necessary for the proliferation and differentiation of naïve T cells into effector T cells (76). However, γδ T cells produce relatively less IL-2 than αβ T cells (77). Due to the PAgs recognition of human Vγ9Vδ2 T cells, the combination of IL-2 with synthetic PAgs, such as Zoledronate (Zol) and BrHPP, was widely used for the generation of human Vγ9Vδ2 T cells from PBMCs for γδT cell-based immunotherapy (Figure 3). Adoptive transfer of pamidronate-expanded Vγ9Vδ2 cells alone effectively prevented EBV-induced B cell lymphoproliferative disease (EBV-LPD) in mouse and the injection of pamidronate significantly controlled the development through specific activation and expansion of Vγ9Vδ2 cells in humanized mice (78). The adoptive transfer of IL-2/PAgs ex vivo expanded Vγ9Vδ2 cells from autologous or allogeneic hosts exhibited potent anti-tumor effects in a variety of cancer patients, such as gastric cancer, osteolytic breast cancer, prostate cancer, and colorectal cancer and so on (79–81). The in vivo administration of pamidronate/Zol and low-dose IL-2 also triggered the proliferation of γδT cells in clinical trials and engaged the anti-tumor response without appreciable toxicity in patients (82–84).
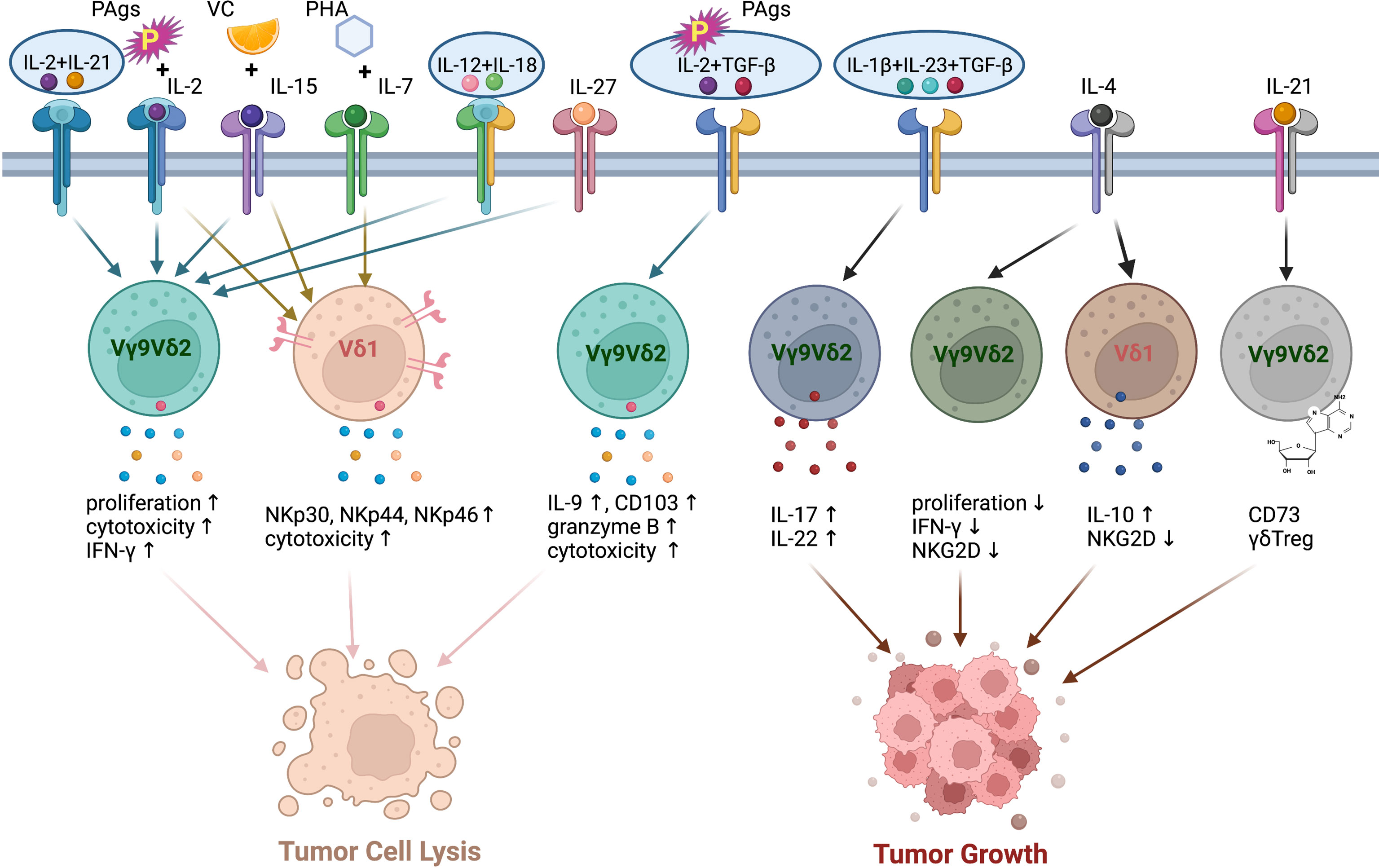
Figure 3 The polarization of human γδT cells induced by different cytokine combination. PAgs and IL-2 with the addition of VC and IL-15, IL-12+IL-18, IL-27, IL-21+IL-2 enhance the cytotoxicity of human Vγ9Vδ2 T cells. IL-2 or IL-15 induces the expressions of NKp30, NKp44, NKp46 on human Vδ1 cells. PHA and IL-7 enhance the cytotoxic capacity of human Vδ1 cells. TGF-β increases the anti-tumor cytotoxicity of human Vγ9Vδ2 T cells in the presence of PAgs and IL-2. The combination of IL-1β, IL-23 and TGF-β promotes the differentiation of Vγ9Vδ2 T cells to IL-17-producing γδT cells. IL-4 reduces the proliferation, NKG2D expression and IFN-γ production of Vγ9Vδ2 T cells via promoting IL-10 secretion of Vδ1 cells. IL-21 alone induces Vγ9Vδ2 T cell differentiation to CD73+ γδTreg cells, which promote tumor growth.
IL-15, another proinflammatory cytokine in IL-2 superfamily, has been shown to contribute to the effector functions and maintain the survival of human NK cells via IL-15-AKT-XBP1s signalling pathway (Figure 3) (85). It is also a promising candidate for enhancing the expansion and cytotoxicity of γδT cells. With the stimulation of IL-2 or IL-15, human Vδ1 cells were selectively induced to express NKp30, NKp44 and NKp46 in a PI3K/AKT dependent manner. The expression of NCRs is associated with increased production of granzyme B and improved cytotoxicity against tumor cells (86, 87). Although low IL-2 and additional IL-15 did not affect NKR expression level on human Vγ9Vδ2 cells, IL-15 significantly increased the expressions of perforin, granzyme B, granulysin and T-bet, which led to enhanced cytotoxic capacity of Vγ9Vδ2 cells. A recent study showed that IL-15 and vitamin C (VC) promoted the proliferation and differentiation and reduced the apoptosis of human Vγ9Vδ2 T cells in vitro (88). Moreover, these cells possessed improved cytotoxicity, both in vitro and in humanized mouse model. The adoptive transfer of IL-15+VC expanded Vγ9Vδ2 T cells prolonged the survival of patients with late-stage lung cancer or liver cancer (89). IL-15 receptor α signalling limited the development of IL-17-produing γδT cells in a mouse model (90). A global increase of γδT17 cells was found in IL-15Rα-KO mice, but only modest dysregulation of IL-17 production was observed on γδT cells from IL-15-KO mice (90).
Other members of IL-2 cytokine family, including IL-4, IL-7, and IL-21, can also act on γδT cells (Figure 3). IL-4 was demonstrated to negatively regulate the anti-tumor function of γδT cells via inhibiting the expression of NKG2D and promoting the IL-10 production from Vδ1 cells, which in turn suppressed IFN-γ production and the proliferation of Vδ2 cells (91). IL-7 was used to expand Vδ1 cells from PBMCs in the presence of PHA in vitro. These expanded Vδ1 cells exhibited great anti-tumor function and prolonged the survival of human colon carcinoma xenografted mice via expressing high levels of cytotoxicity-related molecules, chemokine receptors and NCRs (73). However, IL-7 selectively promoted the IL-17 production of human Vδ1, Vδ2 from cord blood and murine CD27- γδT cells (92). The combination of IL-2 and IL-21 directly enhanced the cytotoxicity of human γδT cells to hepatocellular carcinoma cells in vitro (93). In the presence of IL-21, PAgs-expanded Vγ9Vδ2 T cells expressed high level of CXCR5, which enhanced their potential to support antibody production by B cells (94). On the other hand, IL-21-stimulated Vγ9Vδ2 T cells can differentiate to CD73+ γδTreg cells, which exert immunosuppressive function via inhibiting T cell responses (95).
The synergistic function of IL-12 and IL-18 in inducing the IFN-γ production of T cells and NK cells has been demonstrated (96–99). Similarly, IL-12 and IL-18 also induced the production of IFN-γ and increased cytotoxicity in γδT cells in an antigen-independent manner (Figure 3) (100, 101). However, the combination of IL-12 and IL-18 led to the upregulation of TIM3 on γδT cells (102). That might indicate the exhaustion or dysfunction of γδT cell under the treatment of IL-12/18. IL-27 is a heterodimeric cytokine of IL-12 cytokine family. The expression of IL-12R on T cells can be induced by IL-27 (103). The expression of IL-27R was also detected on human Vγ9Vδ2 cells. As expected, IL-27 enhanced the cytotoxicity of human Vγ9Vδ2 T cells by promoting the production of cytotoxic molecules (Figure 3) (104).
In addition to cytokines inducing IFN-γ production in γδT cells, IL-17-inducing cytokines are responsible for the polarization of γδT17 cells. It is well known that combination of IL-1β, IL-6, IL-23 and TGF-β induce Th17 differentiation in mouse (105). In human, IL-1 and IL-23 but not TGF-β and IL-6 serve as a rheostat tuning the magnitude of Th17 development (106). The stimulation of IL-1 and IL-23 also promoted RORγt, IL-17, IL-21, and IL-22 expression by γδT cells without the engagement of T cell receptor in mouse (107, 108). TGF-β was found to play a key role in the generation of murine γδT17 in thymus during the postnatal period (109). In adults, IL-1β, TGF-β and IL-23 are required for the commitment of human Vγ9Vδ2 T cells to IL-17-producing γδT cells, which also produce IL-22 (110). The function of IL-6 during the differentiation of γδT17 is uncertain. However, the cocktail of cytokines (IL-1β, TGF-β, IL-6 and IL-23) was used to selectively generate IL-17+ Vγ9Vδ2 T cells in vitro (111). These expanded IL-17+ Vγ9Vδ2 T cells produce IL-17 but neither IL-22 nor IFN-γ. The expressions of granzyme B, TRAIL, FasL and CD161 on IL-17+ Vγ9Vδ2 T cells indicated that they contributed to host immune responses against infectious microorganisms. By contrast, TGF-β surprisingly augmented the cytotoxic activity of human Vδ2 T cells when they were stimulated with PAgs and IL-2 or IL-15 in the presence of TGF-β. TGF-β enhanced the migration and anti-tumor function of Vδ2 T cells through upregulating the expressions of CD54, CD103, IFN-γ, IL-9 and granzyme B (112, 113).
In conclusion, γδT cells display high functional plasticity depending on the cytokine environment (Figure 3). In view of the cytokine-dependent polarization of γδT cells, it is crucial to understand the roles of various cytokines regulating γδT cell function, which can guide the effective γδT cell-based cancer immunotherapy.
Current γδT Cell-Based Cancer Immunotherapies
Currently, the majority of the preclinical and clinical studies on γδT cell-based cancer immunotherapy focus on adoptive transfer of expanded γδT cells and its combination with other treatments (Table 2, Figure 4: top left and bottom left). Due to the feasible expansion of human Vγ9Vδ2 T cells using PAgs or aminobisphosphonates, Zol has been used to expand human γδT cells for adoptive transfer or directly injected to induce the proliferation of human γδT cells in vivo for cancer immunotherapy (115, 136).
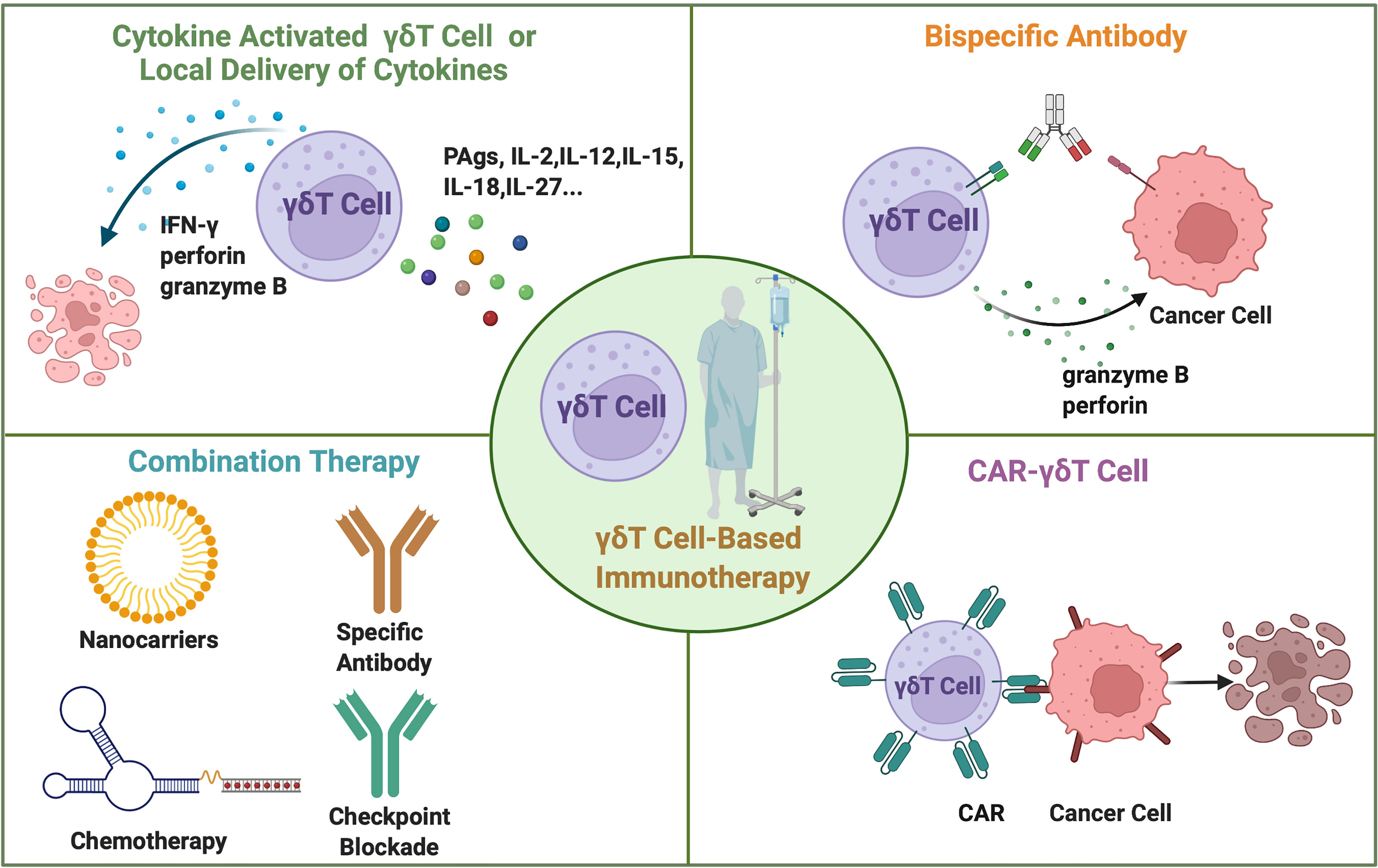
Figure 4 The current approaches for γδT cell-based cancer immunotherapy. The adoptive transfer of cytokine-activated γδT cells in vitro or locally administration of cytokines in vivo. Combination therapy includes γδT cell transfer combined with specific antibody therapy, immune checkpoint blockade, chemotherapy, and nanoparticles. Bispecific antibodies simultaneously bind to γδT cells and cancer cells. Gene modified CAR-γδT cells directly recognize the cancer cells and mediate cancer cell lysis.
Due to the successful application of chimeric antigen receptor (CAR) technology in αβT cells, it has also been applied in γδT cell therapy (Figure 4: bottom right). The study of allogeneic CAR-Vδ1 T cells targeting CD20 antigen exhibited strong anti-tumor activity and minimum xenogeneic graft-versus-host diseases (GVHD) post transplantation (137). This result further supports the clinical evaluation of ADI-001, an allogeneic CD20-CAR-Vδ1 T cell-associated clinical trial (NCT04735471). CAR-Vδ2 T cells also showed promising results in clearing tumor in vivo (138). Mucin 1 (MUC1) with the Tn epitope is a tumor associated antigen that is highly expressed on the surface of a variety of cancer cells. MUC1-Tn CAR-modified Vγ9Vδ2 T cells exhibited similar or stronger anti-tumor effect against breast cancer cell and gastric cancer cell in vitro compared with CAR-αβT cells. MUC1-Tn-CAR-Vγ9Vδ2 T cells more effectively suppressed tumor growth than Vγ9Vδ2 T cells in a xenograft murine gastric cancer model (138).
Many recent studies focus on antibody-induced γδ T cell activation (Figure 4: top right). Fab fragment of anti-CD3e antibody UCHT1 could bind to γδTCR and enhance the tumor killing of Vγ9Vδ2 T cells (139). Aude De Gassart et al. constructed a humanized antibody, ICT01, that could activate Vγ9Vδ2T cells (140). This antibody activated γδT cells that could kill various tumor cell lines and primary tumor cells but not normal healthy cells. Rajkumar Ganesan et al. designed a bispecific antibody, anti-TRGV9/anti-CD123, that could simultaneously bind to the Vγ9 chain of Vγ9Vδ2 T cells and AML target antigen, CD123, then induce the recruitment and activation of Vγ9Vδ2 T cells to target AML blasts (141). Recently, it is demonstrated that tribody activated γδT cells efficiently. Hans H Oberg et al. reported that tribody [(HER2)2 X CD16] is more effective than anti-HER2 monoclonal antibodies in enhancing γδT cell killing against HER2-expressing cancer cells (142). Similarly, tribody of (Her2)2X Vγ9 targets human Vγ9 T cells and HER2-expressing tumor cells to induce γδT cell-mediated tumor killing (143).
The combination therapy of γδT cells with chemotherapy, monoclonal antibody, immune checkpoint blockade or surgery can exert better anti-tumor efficacy than monotherapy (Figure 4: bottom left). The combination of γδT cells with locoregional therapy enhanced clinical efficacy (134). The study using rituximab combined with obinutuzumab and daratumumab activated γδT cells expanded the therapeutic potential of distinctive tumor-antigen-targeting mAbs induced ADCC by γδT cells (144). Targeting the costimulatory signals such as CD137 agonist antibody may promote the anti-tumor functions of Vγ9Vδ2 T cells (145). γδT cell therapy enhanced chemotherapy-induced cytotoxicity to advanced bladder cancer cells (146). Chemotherapeutic agent temozolomide (TMZ) may promote the anti-tumor efficacy of the adoptively transferred ex vivo expanded γδT cells for malignant glioblastoma (147). A few studies demonstrated that nanoparticles could also enhance γδ T cells function. In a recent work, it was found that selenium nanoparticles (SeNPs) pre-treatment strengthened the anti-tumor cytotoxicity of Vγ9Vδ2 T cells by increasing the expression of cytotoxicity related molecules, such as NKG2D, CD16, and IFN-γ (148). Chitosan nanoparticles (CSNPs) also exhibited the role of enhancing anti-tumor immune responses of γδT cells (149). Immune checkpoint blockade using anti-PD-1 mAb promoted Vγ9Vδ2 T cell cytotoxicity against PC-2 tumors in immunodeficient NSG mice (150). Furthermore, combination of Tim-3 blocking antibody and bispecific antibody MT110 (anti-CD3 and anti-EpCAM) enhanced the anti-tumor efficacy of the adoptively transferred γδT cells (151). However, autologous γδT cells combined with gemcitabine therapy for patients with curatively resected pancreatic cancer revealed no significant difference compared with those receiving gemcitabine alone (132), suggesting better understanding of the mechanism of action during different treatment is required to achieved effective combination treatment outcome with γδT cells. Cytokine combinations promoting γδT cell function revealed in pre-clinical studies are yet to be evaluated in clinical trials.
Challenges and Potential Strategies Targeting Cytokine Signals to Improve γδT Cell-Based Immunotherapy
γδT cell-based immunotherapy mainly faces three challenges in achieving improved outcomes for cancer patients. The first challenge is the in vitro generation/expansion of activated γδT cells with superior cytotoxicity. Although adoptive transfer or in vivo expanded human Vγ9Vδ2 T cells exhibited good safety profile, it did not achieve clinical benefit in some patients (123). To boost the cytotoxicity of expanded γδT cells and overcome the immune suppressive tumor microenvironment, cytokine stimulated allogeneic Vγ9Vδ2 cells or Vδ1 cells have been used for clinical trials. A recent study on 132 late-stage cancer patients confirmed the safety and efficacy of IL-15 and VC activated allogeneic Vγ9Vδ2 T cells (89). The addition of IL-15 resulted in the activation, proliferation and increased cytotoxic capacity of γδ T cells (152). To activate cytokine signals, expanded Vδ1 T cells were engineered with a GPC-3 CAR and secreted IL-15 (sIL-15) which significantly controlled tumor growth without inducing GVHD. Moreover, GPC-3-CAR/sIL-15 Vδ1 T cells displayed greater proliferation and stronger anti-tumor responses when compared with GPC-3-CAR Vδ1 T cells lacking sIL-15, suggesting IL-15 signal was critical for CAR Vδ1 T cell function (153). The adoptive transfer of IL-7-expanded human Vδ1 cells also displayed improved cytotoxicity and prolonged the survival of human colon carcinoma xenografted mice (73).
Secondly, rapid exhaustion is a big challenge for maintaining survival and durable anti-tumor functions of γδT cells. Persistent stimulation of human γδT cells with PAgs often induces γδT cell exhaustion (154). It was demonstrated that CD137 costimulation promoted the proliferation and prolonged the survival of Vγ9Vδ2 T cells in vitro and in vivo (145). Moreover, Endogenous IL-15 acted as a potential factor to support the survival of human Vγ9Vδ2 T cells in vivo in the absence of exogenous IL-2 (120). The dysfunction of T cells is also associated with the immunosuppressive tumor microenvironment which will be discussed in the following session.
The third challenge is the immunosuppression mechanisms in cancer patients that can impair the anti-tumor functions of the infused/activated γδT cells. The lack of IL-2 and IL-21 in HCC patients was associated with the PD-1 expression and reduced cytotoxicity of human γδT cells (93). In a murine HCC model, IL-23 overexpression in the liver induced the polarization of γδT cells to IL-17-producing γδT cells (155). Then γδT17 cells promoted tumor growth via recruiting immunosuppressive myeloid-derived suppressor cells (MDSCs). TGF-β is a pivotal immunosuppressive cytokine that secreted by immunosuppressive cell subsets (such as MDSCs and Treg) and tumor cells (156). Mouse Foxp3+ γδT cells can be induced by TGF-β and inhibit T cell activation (157). To avoid γδT cell exhaustion and circumvent tumour immunosuppressive microenvironment, it is a feasible approach to target cytokine signals via administering exogenous stimulating cytokines or blocking the immunosuppressive cytokines. As systemic administration of cytokines usually induces toxicity in patients (158, 159), local delivery of cytokine can limit the systemic toxicity and offer an approach to benefit from the therapeutic effects of the activating cytokines. The local delivery of mRNAs encoding interleukin-12 (IL-12) single chain, interferon-α, granulocyte-macrophage colony-stimulating factor, or IL-15 sushi led to robust anti-tumor immune responses and tumor regression in multiple murine models (160). These findings provided preclinical evidence for modifying the tumor microenvironment via local administration of cytokines. It is possible to induce highly cytotoxic γδT cells through modulations of tumor microenvironment through the induction or delivery of cytokines that can specially promote the anti-tumor functions of γδT cells (Figure 4: top left).
Conclusion and Future Directions
Taken together, γδT cells are promising cellular products for adoptive cancer immunotherapy. γδT cells mediate anti-tumor effects by direct killing and indirect immune regulatory function to other immune cells. γδTCR can recognize specific molecules often in an antigen-independent manner. γδT cells can differentiate into various subsets producing signature cytokines, which can have anti-tumor or pro-tumor functions. In the meantime, this differentiation is greatly influenced by the cytokines present in the microenvironment. γδT cell-based cancer immunotherapy has a good safety profile in the clinical trials but its clinical efficacy needs further improvement. Combination therapies involving γδT cells have had some clinical successes, including chemotherapy, CAR therapy, and checkpoint blockade therapy. IL-2 and IL-15 have been explored for their functions to activate γδT cells in clinical trials. However, other cytokines and combinations that can activate γδT cells are yet to be evaluated in clinical trials. First, cytokines or cytokine combinations can be used to expand, activate, and polarize γδT cells ex vivo to generate potent cellular products for adoptive therapy. Cytokine signals can also be modulated to prolong the survival of the transferred γδT cells in vivo. Second, cytokine can be incorporated into CAR γδT cell therapy to facilitate CAR γδT cell function and prolong their survival in vivo via autocrine mechanism, which can avoid the toxicity induced by systemic cytokine treatment. Third, cytokine signal on γδT cells can be triggered via antibody binding in the form of bi-specific or tri-specific antibody targeting tumor antigens. The additional cytokine signal can facilitate γδT cell function and survival. Thus, detailed understanding of the effects of cytokines and cytokine combinations on γδT cell anti-tumor function is critical for designing effective therapeutic strategies to incorporate cytokine signals into various γδT cell-based cancer immunotherapy to achieve superior clinical efficacy.
Author Contributions
All authors listed have made a substantial, direct, and intellectual contribution to the work and approved it for publication.
Funding
This work has been supported by Singapore National Research Foundation grant NRF-CRP19-2017-04.
Conflict of Interest
The authors declare that the research was conducted in the absence of any commercial or financial relationships that could be construed as a potential conflict of interest.
Publisher’s Note
All claims expressed in this article are solely those of the authors and do not necessarily represent those of their affiliated organizations, or those of the publisher, the editors and the reviewers. Any product that may be evaluated in this article, or claim that may be made by its manufacturer, is not guaranteed or endorsed by the publisher.
Acknowledgments
Figures in this paper were drawn using BioRender (@biorender.com).
References
1. Vavassori S, Kumar A, Wan GS, Ramanjaneyulu GS, Cavallari M, El Daker S, et al. Butyrophilin 3a1 Binds Phosphorylated Antigens and Stimulates Human Gammadelta T Cells. Nat Immunol (2013) 14(9):908–16. doi: 10.1038/ni.2665
2. Rigau M, Ostrouska S, Fulford TS, Johnson DN, Woods K, Ruan Z, et al. Butyrophilin 2a1 Is Essential for Phosphoantigen Reactivity by Gammadelta T Cells. Science (2020) 367(6478):1–10. doi: 10.1126/science.aay5516
3. Laplagne C, Ligat L, Foote J, Lopez F, Fournie JJ, Laurent C, et al. Self-Activation of Vgamma9vdelta2 T Cells by Exogenous Phosphoantigens Involves Tcr and Butyrophilins. Cell Mol Immunol (2021) 18(8):1861–70. doi: 10.1038/s41423-021-00720-w
4. Toutirais O, Cabillic F, Le Friec G, Salot S, Loyer P, Le Gallo M, et al. Dnax Accessory Molecule-1 (Cd226) Promotes Human Hepatocellular Carcinoma Cell Lysis by Vgamma9vdelta2 T Cells. Eur J Immunol (2009) 39(5):1361–8. doi: 10.1002/eji.200838409
5. Sandberg Y, Almeida J, Gonzalez M, Lima M, Barcena P, Szczepanski T, et al. Tcrgammadelta+ Large Granular Lymphocyte Leukemias Reflect the Spectrum of Normal Antigen-Selected Tcrgammadelta+ T-Cells. Leukemia (2006) 20(3):505–13. doi: 10.1038/sj.leu.2404112
6. Davey MS, Willcox CR, Joyce SP, Ladell K, Kasatskaya SA, McLaren JE, et al. Clonal Selection in the Human Vdelta1 T Cell Repertoire Indicates Gammadelta Tcr-Dependent Adaptive Immune Surveillance. Nat Commun (2017) 8:14760. doi: 10.1038/ncomms14760
7. Kaminski H, Marseres G, Cosentino A, Guerville F, Pitard V, Fournie JJ, et al. Understanding Human Gammadelta T Cell Biology Toward a Better Management of Cytomegalovirus Infection. Immunol Rev (2020) 298(1):264–88. doi: 10.1111/imr.12922
8. Pizzolato G, Kaminski H, Tosolini M, Franchini DM, Pont F, Martins F, et al. Single-Cell Rna Sequencing Unveils the Shared and the Distinct Cytotoxic Hallmarks of Human Tcrvdelta1 and Tcrvdelta2 Gammadelta T Lymphocytes. Proc Natl Acad Sci USA (2019) 116(24):11906–15. doi: 10.1073/pnas.1818488116
9. Scheper W, van Dorp S, Kersting S, Pietersma F, Lindemans C, Hol S, et al. Gammadeltat Cells Elicited by Cmv Reactivation After Allo-Sct Cross-Recognize Cmv and Leukemia. Leukemia (2013) 27(6):1328–38. doi: 10.1038/leu.2012.374
10. Pitard V, Roumanes D, Lafarge X, Couzi L, Garrigue I, Lafon ME, et al. Long-Term Expansion of Effector/Memory Vdelta2-Gammadelta T Cells Is a Specific Blood Signature of Cmv Infection. Blood (2008) 112(4):1317–24. doi: 10.1182/blood-2008-01-136713
11. Reijneveld JF, Ocampo TA, Shahine A, Gully BS, Vantourout P, Hayday AC, et al. Human Gammadelta T Cells Recognize Cd1b by Two Distinct Mechanisms. Proc Natl Acad Sci USA (2020) 117(37):22944–52. doi: 10.1073/pnas.2010545117
12. Pellicci DG, Uldrich AP, Le Nours J, Ross F, Chabrol E, Eckle SB, et al. The Molecular Bases of Delta/Alphabeta T Cell-Mediated Antigen Recognition. J Exp Med (2014) 211(13):2599–615. doi: 10.1084/jem.20141764
13. Roy S, Ly D, Castro CD, Li NS, Hawk AJ, Altman JD, et al. Molecular Analysis of Lipid-Reactive Vdelta1 Gammadelta T Cells Identified by Cd1c Tetramers. J Immunol (2016) 196(4):1933–42. doi: 10.4049/jimmunol.1502202
14. Luoma AM, Castro CD, Mayassi T, Bembinster LA, Bai L, Picard D, et al. Crystal Structure of Vdelta1 T Cell Receptor in Complex With Cd1d-Sulfatide Shows Mhc-Like Recognition of a Self-Lipid by Human Gammadelta T Cells. Immunity (2013) 39(6):1032–42. doi: 10.1016/j.immuni.2013.11.001
15. Uldrich AP, Le Nours J, Pellicci DG, Gherardin NA, McPherson KG, Lim RT, et al. Cd1d-Lipid Antigen Recognition by the Gammadelta Tcr. Nat Immunol (2013) 14(11):1137–45. doi: 10.1038/ni.2713
16. Di Marco Barros R, Roberts NA, Dart RJ, Vantourout P, Jandke A, Nussbaumer O, et al. Epithelia Use Butyrophilin-Like Molecules to Shape Organ-Specific Gammadelta T Cell Compartments. Cell (2016) 167(1):203–18.e17. doi: 10.1016/j.cell.2016.08.030
17. Marlin R, Pappalardo A, Kaminski H, Willcox CR, Pitard V, Netzer S, et al. Sensing of Cell Stress by Human Gammadelta Tcr-Dependent Recognition of Annexin A2. Proc Natl Acad Sci USA (2017) 114(12):3163–8. doi: 10.1073/pnas.1621052114
18. Harly C, Joyce SP, Domblides C, Bachelet T, Pitard V, Mannat C, et al. Human Gammadelta T Cell Sensing of Ampk-Dependent Metabolic Tumor Reprogramming Through Tcr Recognition of Epha2. Sci Immunol (2021) 6(61):1–14. doi: 10.1126/sciimmunol.aba9010
19. Hudecek R, Kohlova B, Siskova I, Piskacek M, Knight A. Blocking of Epha2 on Endometrial Tumor Cells Reduces Susceptibility to Vdelta1 Gamma-Delta T-Cell-Mediated Killing. Front Immunol (2021) 12:752646. doi: 10.3389/fimmu.2021.752646
20. Le Nours J, Gherardin NA, Ramarathinam SH, Awad W, Wiede F, Gully BS, et al. A Class of Gammadelta T Cell Receptors Recognize the Underside of the Antigen-Presenting Molecule Mr1. Science (2019) 366(6472):1522–7. doi: 10.1126/science.aav3900
21. Groh V, Steinle A, Bauer S, Spies T. Recognition of Stress-Induced Mhc Molecules by Intestinal Epithelial Gammadelta T Cells. Science (1998) 279(5357):1737–40. doi: 10.1126/science.279.5357.1737
22. Poggi A, Venturino C, Catellani S, Clavio M, Miglino M, Gobbi M, et al. Vdelta1 T Lymphocytes From B-Cll Patients Recognize Ulbp3 Expressed on Leukemic B Cells and Up-Regulated by Trans-Retinoic Acid. Cancer Res (2004) 64(24):9172–9. doi: 10.1158/0008-5472.CAN-04-2417
23. Wu J, Groh V, Spies T. T Cell Antigen Receptor Engagement and Specificity in the Recognition of Stress-Inducible Mhc Class I-Related Chains by Human Epithelial Gamma Delta T Cells. J Immunol (2002) 169(3):1236–40. doi: 10.4049/jimmunol.169.3.1236
24. Hunter S, Willcox CR, Davey MS, Kasatskaya SA, Jeffery HC, Chudakov DM, et al. Human Liver Infiltrating Gammadelta T Cells Are Composed of Clonally Expanded Circulating and Tissue-Resident Populations. J Hepatol (2018) 69(3):654–65. doi: 10.1016/j.jhep.2018.05.007
25. Petrasca A, Melo AM, Breen EP, Doherty DG. Human Vdelta3(+) Gammadelta T Cells Induce Maturation and Igm Secretion by B Cells. Immunol Lett (2018) 196:126–34. doi: 10.1016/j.imlet.2018.02.002
26. Mangan BA, Dunne MR, O'Reilly VP, Dunne PJ, Exley MA, O'Shea D, et al. Cutting Edge: Cd1d Restriction and Th1/Th2/Th17 Cytokine Secretion by Human Vdelta3 T Cells. J Immunol (2013) 191(1):30–4. doi: 10.4049/jimmunol.1300121
27. Mohan Rao LV, Esmon CT, Pendurthi UR. Endothelial Cell Protein C Receptor: A Multiliganded and Multifunctional Receptor. Blood (2014) 124(10):1553–62. doi: 10.1182/blood-2014-05-578328
28. Willcox CR, Pitard V, Netzer S, Couzi L, Salim M, Silberzahn T, et al. Cytomegalovirus and Tumor Stress Surveillance by Binding of a Human Gammadelta T Cell Antigen Receptor to Endothelial Protein C Receptor. Nat Immunol (2012) 13(9):872–9. doi: 10.1038/ni.2394
29. Born WK, Yin Z, Hahn YS, Sun D, O'Brien RL. Analysis of Gamma Delta T Cell Functions in the Mouse. J Immunol (2010) 184(8):4055–61. doi: 10.4049/jimmunol.0903679
30. Castillo-Gonzalez R, Cibrian D, Sanchez-Madrid F. Dissecting the Complexity of Gammadelta T-Cell Subsets in Skin Homeostasis, Inflammation, and Malignancy. J Allergy Clin Immunol (2021) 147(6):2030–42. doi: 10.1016/j.jaci.2020.11.023
31. Itohara S, Farr AG, Lafaille JJ, Bonneville M, Takagaki Y, Haas W, et al. Homing of a Gamma Delta Thymocyte Subset With Homogeneous T-Cell Receptors to Mucosal Epithelia. Nature (1990) 343(6260):754–7. doi: 10.1038/343754a0
32. Marchitto MC, Dillen CA, Liu H, Miller RJ, Archer NK, Ortines RV, et al. Clonal Vgamma6(+)Vdelta4(+) T Cells Promote Il-17-Mediated Immunity Against Staphylococcus Aureus Skin Infection. Proc Natl Acad Sci USA (2019) 116(22):10917–26. doi: 10.1073/pnas.1818256116
33. Melandri D, Zlatareva I, Chaleil RAG, Dart RJ, Chancellor A, Nussbaumer O, et al. The Gammadeltatcr Combines Innate Immunity With Adaptive Immunity by Utilizing Spatially Distinct Regions for Agonist Selection and Antigen Responsiveness. Nat Immunol (2018) 19(12):1352–65. doi: 10.1038/s41590-018-0253-5
34. Crowley MP, Reich Z, Mavaddat N, Altman JD, Chien Y. The Recognition of the Nonclassical Major Histocompatibility Complex (Mhc) Class I Molecule, T10, by the Gammadelta T Cell, G8. J Exp Med (1997) 185(7):1223–30. doi: 10.1084/jem.185.7.1223
35. Shin S, El-Diwany R, Schaffert S, Adams EJ, Garcia KC, Pereira P, et al. Antigen Recognition Determinants of Gammadelta T Cell Receptors. Science (2005) 308(5719):252–5. doi: 10.1126/science.1106480
36. Zeng X, Wei YL, Huang J, Newell EW, Yu H, Kidd BA, et al. Gammadelta T Cells Recognize a Microbial Encoded B Cell Antigen to Initiate a Rapid Antigen-Specific Interleukin-17 Response. Immunity (2012) 37(3):524–34. doi: 10.1016/j.immuni.2012.06.011
37. Jensen KD, Su X, Shin S, Li L, Youssef S, Yamasaki S, et al. Thymic Selection Determines Gammadelta T Cell Effector Fate: Antigen-Naive Cells Make Interleukin-17 and Antigen-Experienced Cells Make Interferon Gamma. Immunity (2008) 29(1):90–100. doi: 10.1016/j.immuni.2008.04.022
38. Jandke A, Melandri D, Monin L, Ushakov DS, Laing AG, Vantourout P, et al. Butyrophilin-Like Proteins Display Combinatorial Diversity in Selecting and Maintaining Signature Intraepithelial Gammadelta T Cell Compartments. Nat Commun (2020) 11(1):3769. doi: 10.1038/s41467-020-17557-y
39. Vantourout P, Hayday A. Six-Of-the-Best: Unique Contributions of Gammadelta T Cells to Immunology. Nat Rev Immunol (2013) 13(2):88–100. doi: 10.1038/nri3384
40. Silva-Santos B, Serre K, Norell H. Gammadelta T Cells in Cancer. Nat Rev Immunol (2015) 15(11):683–91. doi: 10.1038/nri3904
41. Cui K, Mei X, Cheng M. Increased Interleukin-17a-Producing Gammadeltat Cells Predict Favorable Survival in Elderly Patients With Luad and Lusc. J Cancer Res Clin Oncol (2021) 147(11):3289–98. doi: 10.1007/s00432-021-03742-z
42. Cheng M, Chen Y, Huang D, Chen W, Xu W, Chen Y, et al. Intrinsically Altered Lung-Resident Gammadeltat Cells Control Lung Melanoma by Producing Interleukin-17a in the Elderly. Aging Cell (2020) 19(2):e13099. doi: 10.1111/acel.13099
43. Pennington DJ, Vermijlen D, Wise EL, Clarke SL, Tigelaar RE, Hayday AC. The Integration of Conventional and Unconventional T Cells That Characterizes Cell-Mediated Responses. Adv Immunol (2005) 87:27–59. doi: 10.1016/S0065-2776(05)87002-6
44. Roessner K, Wolfe J, Shi C, Sigal LH, Huber S, Budd RC. High Expression of Fas Ligand by Synovial Fluid-Derived Gamma Delta T Cells in Lyme Arthritis. J Immunol (2003) 170(5):2702–10. doi: 10.4049/jimmunol.170.5.2702
45. Tawfik D, Groth C, Gundlach JP, Peipp M, Kabelitz D, Becker T, et al. Trail-Receptor 4 Modulates Gammadelta T Cell-Cytotoxicity Toward Cancer Cells. Front Immunol (2019) 10:2044. doi: 10.3389/fimmu.2019.02044
46. Braakman E, van de Winkel JG, van Krimpen BA, Jansze M, Bolhuis RL. Cd16 on Human Gamma Delta T Lymphocytes: Expression, Function, and Specificity for Mouse Igg Isotypes. Cell Immunol (1992) 143(1):97–107. doi: 10.1016/0008-8749(92)90008-d
47. Wang X, Xiang Z, Liu Y, Huang C, Pei Y, Wang X, et al. Exosomes Derived From Vdelta2-T Cells Control Epstein-Barr Virus-Associated Tumors and Induce T Cell Antitumor Immunity. Sci Transl Med (2020) 12(563):1–16. doi: 10.1126/scitranslmed.aaz3426
48. Wang X, Zhang Y, Mu X, CR Tu, Chung Y, Tsao SW, et al. Exosomes Derived From Gammadelta-T Cells Synergize With Radiotherapy and Preserve Antitumor Activities Against Nasopharyngeal Carcinoma in Immunosuppressive Microenvironment. J Immunother Cancer (2022) 10(2):e003832. doi: 10.1136/jitc-2021-003832
49. Brandes M, Willimann K, Moser B. Professional Antigen-Presentation Function by Human Gammadelta T Cells. Science (2005) 309(5732):264–8. doi: 10.1126/science.1110267
50. Meuter S, Eberl M, Moser B. Prolonged Antigen Survival and Cytosolic Export in Cross-Presenting Human Gammadelta T Cells. Proc Natl Acad Sci USA (2010) 107(19):8730–5. doi: 10.1073/pnas.1002769107
51. Brandes M, Willimann K, Bioley G, Levy N, Eberl M, Luo M, et al. Cross-Presenting Human Gammadelta T Cells Induce Robust Cd8+ Alphabeta T Cell Responses. Proc Natl Acad Sci USA (2009) 106(7):2307–12. doi: 10.1073/pnas.0810059106
52. Holmen Olofsson G, Idorn M, Carnaz Simoes AM, Aehnlich P, Skadborg SK, Noessner E, et al. Vgamma9vdelta2 T Cells Concurrently Kill Cancer Cells and Cross-Present Tumor Antigens. Front Immunol (2021) 12:645131. doi: 10.3389/fimmu.2021.645131
53. Maniar A, Zhang X, Lin W, Gastman BR, Pauza CD, Strome SE, et al. Human Gammadelta T Lymphocytes Induce Robust Nk Cell-Mediated Antitumor Cytotoxicity Through Cd137 Engagement. Blood (2010) 116(10):1726–33. doi: 10.1182/blood-2009-07-234211
54. Wang B, Tian Q, Guo D, Lin W, Xie X, Bi H. Activated Gammadelta T Cells Promote Dendritic Cell Maturation and Exacerbate the Development of Experimental Autoimmune Uveitis (Eau) in Mice. Immunol Invest (2021) 50(2-3):164–83. doi: 10.1080/08820139.2020.1716786
55. Petrasca A, Doherty DG. Human Vdelta2(+) Gammadelta T Cells Differentially Induce Maturation, Cytokine Production, and Alloreactive T Cell Stimulation by Dendritic Cells and B Cells. Front Immunol (2014) 5:650. doi: 10.3389/fimmu.2014.00650
56. Ismaili J, Olislagers V, Poupot R, Fournie JJ, Goldman M. Human Gamma Delta T Cells Induce Dendritic Cell Maturation. Clin Immunol (2002) 103(3 Pt 1):296–302. doi: 10.1006/clim.2002.5218
57. Ullrich L, Lueder Y, Juergens AL, Wilharm A, Barros-Martins J, Bubke A, et al. Il-4-Producing Vgamma1(+)/Vdelta6(+) Gammadelta T Cells Sustain Germinal Center Reactions in Peyer's Patches of Mice. Front Immunol (2021) 12:729607. doi: 10.3389/fimmu.2021.729607
58. Ma Y, Aymeric L, Locher C, Mattarollo SR, Delahaye NF, Pereira P, et al. Contribution of Il-17-Producing Gamma Delta T Cells to the Efficacy of Anticancer Chemotherapy. J Exp Med (2011) 208(3):491–503. doi: 10.1084/jem.20100269
59. Daley D, Zambirinis CP, Seifert L, Akkad N, Mohan N, Werba G, et al. Gammadelta T Cells Support Pancreatic Oncogenesis by Restraining Alphabeta T Cell Activation. Cell (2016) 166(6):1485–99.e15. doi: 10.1016/j.cell.2016.07.046
60. Brauneck F, Weimer P, Schulze Zur Wiesch J, Weisel K, Leypoldt L, Vohwinkel G, et al. Bone Marrow-Resident Vdelta1 T Cells Co-Express Tigit With Pd-1, Tim-3 or Cd39 in Aml and Myeloma. Front Med (Lausanne) (2021) 8:763773. doi: 10.3389/fmed.2021.763773
61. Beatty GL, Gladney WL. Immune Escape Mechanisms as a Guide for Cancer Immunotherapy. Clin Cancer Res (2015) 21(4):687–92. doi: 10.1158/1078-0432.CCR-14-1860
62. Park JH, Kim HJ, Kim CW, Kim HC, Jung Y, HS L, et al. Tumor Hypoxia Represses Gammadelta T Cell-Mediated Antitumor Immunity Against Brain Tumors. Nat Immunol (2021) 22(3):336–46. doi: 10.1038/s41590-020-00860-7
63. Sureshbabu SK, Chaukar D, Chiplunkar SV. Hypoxia Regulates the Differentiation and Anti-Tumor Effector Functions of Gammadeltat Cells in Oral Cancer. Clin Exp Immunol (2020) 201(1):40–57. doi: 10.1111/cei.13436
64. Ma S, Cheng Q, Cai Y, Gong H, Wu Y, Yu X, et al. Il-17a Produced by Gammadelta T Cells Promotes Tumor Growth in Hepatocellular Carcinoma. Cancer Res (2014) 74(7):1969–82. doi: 10.1158/0008-5472.CAN-13-2534
65. Coffelt SB, Kersten K, Doornebal CW, Weiden J, Vrijland K, Hau CS, et al. Il-17-Producing Gammadelta T Cells and Neutrophils Conspire to Promote Breast Cancer Metastasis. Nature (2015) 522(7556):345–8. doi: 10.1038/nature14282
66. Wu P, Wu D, Ni C, Ye J, Chen W, Hu G, et al. Gammadeltat17 Cells Promote the Accumulation and Expansion of Myeloid-Derived Suppressor Cells in Human Colorectal Cancer. Immunity (2014) 40(5):785–800. doi: 10.1016/j.immuni.2014.03.013
67. Rei M, Goncalves-Sousa N, Lanca T, Thompson RG, Mensurado S, Balkwill FR, et al. Murine Cd27(-) Vgamma6(+) Gammadelta T Cells Producing Il-17a Promote Ovarian Cancer Growth Via Mobilization of Protumor Small Peritoneal Macrophages. Proc Natl Acad Sci USA (2014) 111(34):E3562–70. doi: 10.1073/pnas.1403424111
68. Wakita D, Sumida K, Iwakura Y, Nishikawa H, Ohkuri T, Chamoto K, et al. Tumor-Infiltrating Il-17-Producing Gammadelta T Cells Support the Progression of Tumor by Promoting Angiogenesis. Eur J Immunol (2010) 40(7):1927–37. doi: 10.1002/eji.200940157
69. Kuhl AA, Pawlowski NN, Grollich K, Blessenohl M, Westermann J, Zeitz M, et al. Human Peripheral Gammadelta T Cells Possess Regulatory Potential. Immunology (2009) 128(4):580–8. doi: 10.1111/j.1365-2567.2009.03162.x
70. Peng G, Wang HY, Peng W, Kiniwa Y, Seo KH, Wang RF. Tumor-Infiltrating Gammadelta T Cells Suppress T and Dendritic Cell Function Via Mechanisms Controlled by a Unique Toll-Like Receptor Signaling Pathway. Immunity (2007) 27(2):334–48. doi: 10.1016/j.immuni.2007.05.020
71. Hu G, Wu P, Cheng P, Zhang Z, Wang Z, Yu X, et al. Tumor-Infiltrating Cd39(+)Gammadeltatregs Are Novel Immunosuppressive T Cells in Human Colorectal Cancer. Oncoimmunology (2017) 6(2):e1277305. doi: 10.1080/2162402X.2016.1277305
72. Hu G, Cheng P, Pan J, Wang S, Ding Q, Jiang Z, et al. An Il6-Adenosine Positive Feedback Loop Between Cd73(+) Gammadeltatregs and Cafs Promotes Tumor Progression in Human Breast Cancer. Cancer Immunol Res (2020) 8(10):1273–86. doi: 10.1158/2326-6066.CIR-19-0923
73. Wu D, Wu P, Wu X, Ye J, Wang Z, Zhao S, et al. Ex Vivo Expanded Human Circulating Vdelta1 Gammadeltat Cells Exhibit Favorable Therapeutic Potential for Colon Cancer. Oncoimmunology (2015) 4(3):e992749. doi: 10.4161/2162402X.2014.992749
74. Siegers GM, Dhamko H, Wang XH, Mathieson AM, Kosaka Y, Felizardo TC, et al. Human Vdelta1 Gammadelta T Cells Expanded From Peripheral Blood Exhibit Specific Cytotoxicity Against B-Cell Chronic Lymphocytic Leukemia-Derived Cells. Cytotherapy (2011) 13(6):753–64. doi: 10.3109/14653249.2011.553595
75. Kakimi K, Matsushita H, Murakawa T, Nakajima J. Gammadelta T Cell Therapy for the Treatment of Non-Small Cell Lung Cancer. Transl Lung Cancer Res (2014) 3(1):23–33. doi: 10.3978/j.issn.2218-6751.2013.11.01
76. Bachmann MF, Oxenius A. Interleukin 2: From Immunostimulation to Immunoregulation and Back Again. EMBO Rep (2007) 8(12):1142–8. doi: 10.1038/sj.embor.7401099
77. Yui MA, Sharp LL, Havran WL, Rothenberg EV. Preferential Activation of an Il-2 Regulatory Sequence Transgene in Tcr Gamma Delta and Nkt Cells: Subset-Specific Differences in Il-2 Regulation. J Immunol (2004) 172(8):4691–9. doi: 10.4049/jimmunol.172.8.4691
78. Xiang Z, Liu Y, Zheng J, Liu M, Lv A, Gao Y, et al. Targeted Activation of Human Vgamma9vdelta2-T Cells Controls Epstein-Barr Virus-Induced B Cell Lymphoproliferative Disease. Cancer Cell (2014) 26(4):565–76. doi: 10.1016/j.ccr.2014.07.026
79. Zysk A, DeNichilo MO, Panagopoulos V, Zinonos I, Liapis V, Hay S, et al. Adoptive Transfer of Ex Vivo Expanded Vgamma9vdelta2 T Cells in Combination With Zoledronic Acid Inhibits Cancer Growth and Limits Osteolysis in a Murine Model of Osteolytic Breast Cancer. Cancer Lett (2017) 386:141–50. doi: 10.1016/j.canlet.2016.11.013
80. Wada I, Matsushita H, Noji S, Mori K, Yamashita H, Nomura S, et al. Intraperitoneal Injection of in Vitro Expanded Vgamma9vdelta2 T Cells Together With Zoledronate for the Treatment of Malignant Ascites Due to Gastric Cancer. Cancer Med (2014) 3(2):362–75. doi: 10.1002/cam4.196
81. Kondo M, Sakuta K, Noguchi A, Ariyoshi N, Sato K, Sato S, et al. Zoledronate Facilitates Large-Scale Ex Vivo Expansion of Functional Gammadelta T Cells From Cancer Patients for Use in Adoptive Immunotherapy. Cytotherapy (2008) 10(8):842–56. doi: 10.1080/14653240802419328
82. Pressey JG, Adams J, Harkins L, Kelly D, You Z, Lamb LS Jr. In Vivo Expansion and Activation of Gammadelta T Cells as Immunotherapy for Refractory Neuroblastoma: A Phase 1 Study. Med (Baltimore) (2016) 95(39):e4909. doi: 10.1097/MD.0000000000004909
83. Dieli F, Vermijlen D, Fulfaro F, Caccamo N, Meraviglia S, Cicero G, et al. Targeting Human {Gamma}Delta} T Cells With Zoledronate and Interleukin-2 for Immunotherapy of Hormone-Refractory Prostate Cancer. Cancer Res (2007) 67(15):7450–7. doi: 10.1158/0008-5472.CAN-07-0199
84. Wilhelm M, Kunzmann V, Eckstein S, Reimer P, Weissinger F, Ruediger T, et al. Gammadelta T Cells for Immune Therapy of Patients With Lymphoid Malignancies. Blood (2003) 102(1):200–6. doi: 10.1182/blood-2002-12-3665
85. Wang Y, Zhang Y, Yi P, Dong W, Nalin AP, Zhang J, et al. The Il-15-Akt-Xbp1s Signaling Pathway Contributes to Effector Functions and Survival in Human Nk Cells. Nat Immunol (2019) 20(1):10–7. doi: 10.1038/s41590-018-0265-1
86. Mikulak J, Oriolo F, Bruni E, Roberto A, FS C, Villa A, et al. Nkp46-Expressing Human Gut-Resident Intraepithelial Vdelta1 T Cell Subpopulation Exhibits High Antitumor Activity Against Colorectal Cancer. JCI Insight (2019) 4(24):1–19. doi: 10.1172/jci.insight.125884
87. Correia DV, Fogli M, Hudspeth K, da Silva MG, Mavilio D, Silva-Santos B. Differentiation of Human Peripheral Blood Vdelta1+ T Cells Expressing the Natural Cytotoxicity Receptor Nkp30 for Recognition of Lymphoid Leukemia Cells. Blood (2011) 118(4):992–1001. doi: 10.1182/blood-2011-02-339135
88. Kouakanou L, Xu Y, Peters C, He J, Wu Y, Yin Z, et al. Vitamin C Promotes the Proliferation and Effector Functions of Human Gammadelta T Cells. Cell Mol Immunol (2020) 17(5):462–73. doi: 10.1038/s41423-019-0247-8
89. Xu Y, Xiang Z, Alnaggar M, Kouakanou L, Li J, He J, et al. Allogeneic Vgamma9vdelta2 T-Cell Immunotherapy Exhibits Promising Clinical Safety and Prolongs the Survival of Patients With Late-Stage Lung or Liver Cancer. Cell Mol Immunol (2021) 18(2):427–39. doi: 10.1038/s41423-020-0515-7
90. Colpitts SL, Puddington L, Lefrancois L. Il-15 Receptor Alpha Signaling Constrains the Development of Il-17-Producing Gammadelta T Cells. Proc Natl Acad Sci USA (2015) 112(31):9692–7. doi: 10.1073/pnas.1420741112
91. Mao Y, Yin S, Zhang J, Hu Y, Huang B, Cui L, et al. A New Effect of Il-4 on Human Gammadelta T Cells: Promoting Regulatory Vdelta1 T Cells Via Il-10 Production and Inhibiting Function of Vdelta2 T Cells. Cell Mol Immunol (2016) 13(2):217–28. doi: 10.1038/cmi.2015.07
92. Michel ML, Pang DJ, Haque SF, Potocnik AJ, Pennington DJ, Hayday AC. Interleukin 7 (Il-7) Selectively Promotes Mouse and Human Il-17-Producing Gammadelta Cells. Proc Natl Acad Sci USA (2012) 109(43):17549–54. doi: 10.1073/pnas.1204327109
93. Jiang H, Yang Z, Song Z, Green M, Song H, Shao Q. Gammadelta T Cells in Hepatocellular Carcinoma Patients Present Cytotoxic Activity But Are Reduced in Potency Due to Il-2 and Il-21 Pathways. Int Immunopharmacol (2019) 70:167–73. doi: 10.1016/j.intimp.2019.02.019
94. Bansal RR, Mackay CR, Moser B, Eberl M. Il-21 Enhances the Potential of Human Gammadelta T Cells to Provide B-Cell Help. Eur J Immunol (2012) 42(1):110–9. doi: 10.1002/eji.201142017
95. Barjon C, Michaud HA, Fages A, Dejou C, Zampieri A, They L, et al. Il-21 Promotes the Development of a Cd73-Positive Vgamma9vdelta2 T Cell Regulatory Population. Oncoimmunology (2017) 7(1):e1379642. doi: 10.1080/2162402X.2017.1379642
96. Song Y, Hu B, Liu Y, Jin Z, Zhang Y, Lin D, et al. Il-12/Il-18-Preactivated Donor Nk Cells Enhance Gvl Effects and Mitigate Gvhd After Allogeneic Hematopoietic Stem Cell Transplantation. Eur J Immunol (2018) 48(4):670–82. doi: 10.1002/eji.201747177
97. Romee R, Rosario M, Berrien-Elliott MM, Wagner JA, Jewell BA, Schappe T, et al. Cytokine-Induced Memory-Like Natural Killer Cells Exhibit Enhanced Responses Against Myeloid Leukemia. Sci Transl Med (2016) 8(357):357ra123. doi: 10.1126/scitranslmed.aaf2341
98. Romee R, Schneider SE, Leong JW, Chase JM, Keppel CR, Sullivan RP, et al. Cytokine Activation Induces Human Memory-Like Nk Cells. Blood (2012) 120(24):4751–60. doi: 10.1182/blood-2012-04-419283
99. Yoshimoto T, Takeda K, Tanaka T, Ohkusu K, Kashiwamura S, Okamura H, et al. Il-12 Up-Regulates Il-18 Receptor Expression on T Cells, Th1 Cells, and B Cells: Synergism With Il-18 for Ifn-Gamma Production. J Immunol (1998) 161(7):3400–7.
100. Schilbach K, Welker C, Krickeberg N, Kaisser C, Schleicher S, Hashimoto H. In the Absence of a Tcr Signal Il-2/Il-12/18-Stimulated Gammadelta T Cells Demonstrate Potent Anti-Tumoral Function Through Direct Killing and Senescence Induction in Cancer Cells. Cancers (Basel) (2020) 12(1):130. doi: 10.3390/cancers12010130
101. Domae E, Hirai Y, Ikeo T, Goda S, Shimizu Y. Cytokine-Mediated Activation of Human Ex Vivo-Expanded Vgamma9vdelta2 T Cells. Oncotarget (2017) 8(28):45928–42. doi: 10.18632/oncotarget.17498
102. Schofield L, Ioannidis LJ, Karl S, Robinson LJ, Tan QY, Poole DP, et al. Synergistic Effect of Il-12 and Il-18 Induces Tim3 Regulation of Gammadelta T Cell Function and Decreases the Risk of Clinical Malaria in Children Living in Papua New Guinea. BMC Med (2017) 15(1):114. doi: 10.1186/s12916-017-0883-8
103. Hibbert L, Pflanz S, De Waal Malefyt R, Kastelein RA. Il-27 and Ifn-Alpha Signal Via Stat1 and Stat3 and Induce T-Bet and Il-12rbeta2 in Naive T Cells. J Interferon Cytokine Res (2003) 23(9):513–22. doi: 10.1089/10799900360708632
104. Morandi F, Prigione I, Airoldi I. Human Tcrgammadelta+ T Cells Represent a Novel Target for Il-27 Activity. Eur J Immunol (2012) 42(6):1547–52. doi: 10.1002/eji.201142241
105. McGeachy MJ, Cua DJ. Th17 Cell Differentiation: The Long and Winding Road. Immunity (2008) 28(4):445–53. doi: 10.1016/j.immuni.2008.03.001
106. Revu S, Wu J, Henkel M, Rittenhouse N, Menk A, Delgoffe GM, et al. Il-23 and Il-1beta Drive Human Th17 Cell Differentiation and Metabolic Reprogramming in Absence of Cd28 Costimulation. Cell Rep (2018) 22(10):2642–53. doi: 10.1016/j.celrep.2018.02.044
107. Muschaweckh A, Petermann F, Korn T. Il-1beta and Il-23 Promote Extrathymic Commitment of Cd27(+)Cd122(-) Gammadelta T Cells to Gammadeltat17 Cells. J Immunol (2017) 199(8):2668–79. doi: 10.4049/jimmunol.1700287
108. Sutton CE, Lalor SJ, Sweeney CM, Brereton CF, Lavelle EC, Mills KH. Interleukin-1 and Il-23 Induce Innate Il-17 Production From Gammadelta T Cells, Amplifying Th17 Responses and Autoimmunity. Immunity (2009) 31(2):331–41. doi: 10.1016/j.immuni.2009.08.001
109. Do JS, PJ F, Li L, Spolski R, Robinson J, WJ L, et al. Cutting Edge: Spontaneous Development of Il-17-Producing Gamma Delta T Cells in the Thymus Occurs Via a Tgf-Beta 1-Dependent Mechanism. J Immunol (2010) 184(4):1675–9. doi: 10.4049/jimmunol.0903539
110. Ness-Schwickerath KJ, Jin C, Morita CT. Cytokine Requirements for the Differentiation and Expansion of Il-17a- and Il-22-Producing Human Vgamma2vdelta2 T Cells. J Immunol (2010) 184(12):7268–80. doi: 10.4049/jimmunol.1000600
111. Caccamo N, La Mendola C, Orlando V, Meraviglia S, Todaro M, Stassi G, et al. Differentiation, Phenotype, and Function of Interleukin-17-Producing Human Vgamma9vdelta2 T Cells. Blood (2011) 118(1):129–38. doi: 10.1182/blood-2011-01-331298
112. Beatson RE, AC P-P, Halim L, Cozzetto D, Hull C, LM W, et al. Tgf-Beta1 Potentiates Vgamma9vdelta2 T Cell Adoptive Immunotherapy of Cancer. Cell Rep Med (2021) 2(12):100473. doi: 10.1016/j.xcrm.2021.100473
113. Peters C, Meyer A, Kouakanou L, Feder J, Schricker T, Lettau M, et al. Tgf-Beta Enhances the Cytotoxic Activity of Vdelta2 T Cells. Oncoimmunology (2019) 8(1):e1522471. doi: 10.1080/2162402X.2018.1522471
114. Zhou J, Kang N, Cui L, Ba D, He W. Anti-Gammadelta Tcr Antibody-Expanded Gammadelta T Cells: A Better Choice for the Adoptive Immunotherapy of Lymphoid Malignancies. Cell Mol Immunol (2012) 9(1):34–44. doi: 10.1038/cmi.2011.16
115. Kobayashi H, Tanaka Y, Yagi J, Minato N, Tanabe K. Phase I/Ii Study of Adoptive Transfer of Gammadelta T Cells in Combination With Zoledronic Acid and Il-2 to Patients With Advanced Renal Cell Carcinoma. Cancer Immunol Immunother (2011) 60(8):1075–84. doi: 10.1007/s00262-011-1021-7
116. Bennouna J, Levy V, Sicard H, Senellart H, Audrain M, Hiret S, et al. Phase I Study of Bromohydrin Pyrophosphate (Brhpp, Iph 1101), a Vgamma9vdelta2 T Lymphocyte Agonist in Patients With Solid Tumors. Cancer Immunol Immunother (2010) 59(10):1521–30. doi: 10.1007/s00262-010-0879-0
117. Bennouna J, Bompas E, Neidhardt EM, Rolland F, Philip I, Galea C, et al. Phase-I Study of Innacell Gammadelta, an Autologous Cell-Therapy Product Highly Enriched in Gamma9delta2 T Lymphocytes, in Combination With Il-2, in Patients With Metastatic Renal Cell Carcinoma. Cancer Immunol Immunother (2008) 57(11):1599–609. doi: 10.1007/s00262-008-0491-8
118. Kobayashi H, Tanaka Y, Yagi J, Osaka Y, Nakazawa H, Uchiyama T, et al. Safety Profile and Anti-Tumor Effects of Adoptive Immunotherapy Using Gamma-Delta T Cells Against Advanced Renal Cell Carcinoma: A Pilot Study. Cancer Immunol Immunother (2007) 56(4):469–76. doi: 10.1007/s00262-006-0199-6
119. Sugie T, Suzuki E, Yamauchi A, Yamagami K, Masuda N, Gondo N, et al. Combined Effects of Neoadjuvant Letrozole and Zoledronic Acid on Gammadeltat Cells in Postmenopausal Women With Early-Stage Breast Cancer. Breast (2018) 38:114–9. doi: 10.1016/j.breast.2017.12.017
120. Izumi T, Kondo M, Takahashi T, Fujieda N, Kondo A, Tamura N, et al. Ex Vivo Characterization of Gammadelta T-Cell Repertoire in Patients After Adoptive Transfer of Vgamma9vdelta2 T Cells Expressing the Interleukin-2 Receptor Beta-Chain and the Common Gamma-Chain. Cytotherapy (2013) 15(4):481–91. doi: 10.1016/j.jcyt.2012.12.004
121. Fazzi R, Petrini I, Giuliani N, Morganti R, Carulli G, Dalla Palma B, et al. Phase Ii Trial of Maintenance Treatment With Il2 and Zoledronate in Multiple Myeloma After Bone Marrow Transplantation: Biological and Clinical Results. Front Immunol (2020) 11:573156. doi: 10.3389/fimmu.2020.573156
122. Wilhelm M, Smetak M, Schaefer-Eckart K, Kimmel B, Birkmann J, Einsele H, et al. Successful Adoptive Transfer and in Vivo Expansion of Haploidentical Gammadelta T Cells. J Transl Med (2014) 12:45. doi: 10.1186/1479-5876-12-45
123. Kunzmann V, Smetak M, Kimmel B, Weigang-Koehler K, Goebeler M, Birkmann J, et al. Tumor-Promoting Versus Tumor-Antagonizing Roles of Gammadelta T Cells in Cancer Immunotherapy: Results From a Prospective Phase I/Ii Trial. J Immunother (2012) 35(2):205–13. doi: 10.1097/CJI.0b013e318245bb1e
124. Lang JM, Kaikobad MR, Wallace M, Staab MJ, Horvath DL, Wilding G, et al. Pilot Trial of Interleukin-2 and Zoledronic Acid to Augment Gammadelta T Cells as Treatment for Patients With Refractory Renal Cell Carcinoma. Cancer Immunol Immunother (2011) 60(10):1447–60. doi: 10.1007/s00262-011-1049-8
125. Sumi E, Sugie T, Yoshimura K, Tada H, Ikeda T, Suzuki E, et al. Effects of Zoledronic Acid and the Association Between Its Efficacy and Gammadeltat Cells in Postmenopausal Women With Breast Cancer Treated With Preoperative Hormonal Therapy: A Study Protocol. J Transl Med (2014) 12:310. doi: 10.1186/s12967-014-0310-2
126. Sakamoto M, Nakajima J, Murakawa T, Fukami T, Yoshida Y, Murayama T, et al. Adoptive Immunotherapy for Advanced Non-Small Cell Lung Cancer Using Zoledronate-Expanded Gammadeltatcells: A Phase I Clinical Study. J Immunother (2011) 34(2):202–11. doi: 10.1097/CJI.0b013e318207ecfb
127. Nakajima J, Murakawa T, Fukami T, Goto S, Kaneko T, Yoshida Y, et al. A Phase I Study of Adoptive Immunotherapy for Recurrent Non-Small-Cell Lung Cancer Patients With Autologous Gammadelta T Cells. Eur J Cardiothorac Surg (2010) 37(5):1191–7. doi: 10.1016/j.ejcts.2009.11.051
128. Meraviglia S, Eberl M, Vermijlen D, Todaro M, Buccheri S, Cicero G, et al. In Vivo Manipulation of Vgamma9vdelta2 T Cells With Zoledronate and Low-Dose Interleukin-2 for Immunotherapy of Advanced Breast Cancer Patients. Clin Exp Immunol (2010) 161(2):290–7. doi: 10.1111/j.1365-2249.2010.04167.x
129. Noguchi A, Kaneko T, Kamigaki T, Fujimoto K, Ozawa M, Saito M, et al. Zoledronate-Activated Vgamma9gammadelta T Cell-Based Immunotherapy Is Feasible and Restores the Impairment of Gammadelta T Cells in Patients With Solid Tumors. Cytotherapy (2011) 13(1):92–7. doi: 10.3109/14653249.2010.515581
130. Santini D, Martini F, Fratto ME, Galluzzo S, Vincenzi B, Agrati C, et al. In Vivo Effects of Zoledronic Acid on Peripheral Gammadelta T Lymphocytes in Early Breast Cancer Patients. Cancer Immunol Immunother (2009) 58(1):31–8. doi: 10.1007/s00262-008-0521-6
131. Abe Y, Muto M, Nieda M, Nakagawa Y, Nicol A, Kaneko T, et al. Clinical and Immunological Evaluation of Zoledronate-Activated Vgamma9gammadelta T-Cell-Based Immunotherapy for Patients With Multiple Myeloma. Exp Hematol (2009) 37(8):956–68. doi: 10.1016/j.exphem.2009.04.008
132. Aoki T, Matsushita H, Hoshikawa M, Hasegawa K, Kokudo N, Kakimi K. Adjuvant Combination Therapy With Gemcitabine and Autologous Gammadelta T-Cell Transfer in Patients With Curatively Resected Pancreatic Cancer. Cytotherapy (2017) 19(4):473–85. doi: 10.1016/j.jcyt.2017.01.002
133. Lin M, Zhang X, Liang S, Luo H, Alnaggar M, Liu A, et al. Irreversible Electroporation Plus Allogenic Vgamma9vdelta2 T Cells Enhances Antitumor Effect for Locally Advanced Pancreatic Cancer Patients. Signal Transduct Target Ther (2020) 5(1):215. doi: 10.1038/s41392-020-00260-1
134. Zhang T, Chen J, Niu L, Liu Y, Ye G, Jiang M, et al. Clinical Safety and Efficacy of Locoregional Therapy Combined With Adoptive Transfer of Allogeneic Gammadelta T Cells for Advanced Hepatocellular Carcinoma and Intrahepatic Cholangiocarcinoma. J Vasc Interv Radiol (2022) 33(1):19–27.e3. doi: 10.1016/j.jvir.2021.09.012
135. Ji N, Mukherjee N, Reyes RM, Gelfond J, Javors M, Meeks JJ, et al. Rapamycin Enhances Bcg-Specific Gammadelta T Cells During Intravesical Bcg Therapy for Non-Muscle Invasive Bladder Cancer: A Random Double-Blind Study. J Immunother Cancer (2021) 9(3):e001941. doi: 10.1136/jitc-2020-001941
136. Nada MH, Wang H, Workalemahu G, Tanaka Y, Morita CT. Enhancing Adoptive Cancer Immunotherapy With Vgamma2vdelta2 T Cells Through Pulse Zoledronate Stimulation. J Immunother Cancer (2017) 5:9. doi: 10.1186/s40425-017-0209-6
137. Nishimoto KP, Barca T, Azameera A, Makkouk A, Romero JM, Bai L, et al. Allogeneic Cd20-Targeted Gammadelta T Cells Exhibit Innate and Adaptive Antitumor Activities in Preclinical B-Cell Lymphoma Models. Clin Transl Immunol (2022) 11(2):e1373. doi: 10.1002/cti2.1373
138. Zhai X, You F, Xiang S, Jiang L, Chen D, Li Y, et al. Muc1-Tn-Targeting Chimeric Antigen Receptor-Modified Vgamma9vdelta2 T Cells With Enhanced Antigen-Specific Anti-Tumor Activity. Am J Cancer Res (2021) 11(1):79–91.
139. Juraske C, Wipa P, Morath A, Hidalgo JV, Hartl FA, Raute K, et al. Anti-Cd3 Fab Fragments Enhance Tumor Killing by Human Gammadelta T Cells Independent of Nck Recruitment to the Gammadelta T Cell Antigen Receptor. Front Immunol (2018) 9:1579. doi: 10.3389/fimmu.2018.01579
140. De Gassart A, KS Le, Brune P, Agaugue S, Sims J, Goubard A, et al. Development of Ict01, a First-In-Class, Anti-Btn3a Antibody for Activating Vgamma9vdelta2 T Cell-Mediated Antitumor Immune Response. Sci Transl Med (2021) 13(616):eabj0835. doi: 10.1126/scitranslmed.abj0835
141. Ganesan R, Chennupati V, Ramachandran B, Hansen MR, Singh S, Grewal IS. Selective Recruitment of Gammadelta T Cells by a Bispecific Antibody for the Treatment of Acute Myeloid Leukemia. Leukemia (2021) 35(8):2274–84. doi: 10.1038/s41375-021-01122-7
142. Oberg HH, Kellner C, Gonnermann D, Sebens S, Bauerschlag D, Gramatzki M, et al. Tribody [(Her2)2xcd16] Is More Effective Than Trastuzumab in Enhancing Gammadelta T Cell and Natural Killer Cell Cytotoxicity Against Her2-Expressing Cancer Cells. Front Immunol (2018) 9:814. doi: 10.3389/fimmu.2018.00814
143. Oberg HH, Kellner C, Gonnermann D, Peipp M, Peters C, Sebens S, et al. Gammadelta T Cell Activation by Bispecific Antibodies. Cell Immunol (2015) 296(1):41–9. doi: 10.1016/j.cellimm.2015.04.009
144. Hoeres T, Pretscher D, Holzmann E, Smetak M, Birkmann J, Triebel J, et al. Improving Immunotherapy Against B-Cell Malignancies Using Gammadelta T-Cell-Specific Stimulation and Therapeutic Monoclonal Antibodies. J Immunother (2019) 42(9):331–44. doi: 10.1097/CJI.0000000000000289
145. Pei Y, Wen K, Xiang Z, Huang C, Wang X, Mu X, et al. Cd137 Costimulation Enhances the Antiviral Activity of Vgamma9vdelta2-T Cells Against Influenza Virus. Signal Transduct Target Ther (2020) 5(1):74. doi: 10.1038/s41392-020-0174-2
146. Pan Y, Chiu YH, Chiu SC, Cho DY, Lee LM, Wen YC, et al. Gamma/Delta T-Cells Enhance Carboplatin-Induced Cytotoxicity Towards Advanced Bladder Cancer Cells. Anticancer Res (2020) 40(9):5221–7. doi: 10.21873/anticanres.14525
147. Chitadze G, Lettau M, Luecke S, Wang T, Janssen O, Furst D, et al. Nkg2d- and T-Cell Receptor-Dependent Lysis of Malignant Glioma Cell Lines by Human Gammadelta T Cells: Modulation by Temozolomide and a Disintegrin and Metalloproteases 10 and 17 Inhibitors. Oncoimmunology (2016) 5(4):e1093276. doi: 10.1080/2162402X.2015.1093276
148. Hu Y, Liu T, Li J, Mai F, Li J, Chen Y, et al. Selenium Nanoparticles as New Strategy to Potentiate Gammadelta T Cell Anti-Tumor Cytotoxicity Through Upregulation of Tubulin-Alpha Acetylation. Biomaterials (2019) 222:119397. doi: 10.1016/j.biomaterials.2019.119397
149. Lin L, He J, Li J, Xu Y, Li J, Wu Y. Chitosan Nanoparticles Strengthen Vgamma9vdelta2 T-Cell Cytotoxicity Through Upregulation of Killing Molecules and Cytoskeleton Polarization. Int J Nanomedicine (2019) 14:9325–36. doi: 10.2147/IJN.S212898
150. Nada MH, Wang H, Hussein AJ, Tanaka Y, Morita CT. Pd-1 Checkpoint Blockade Enhances Adoptive Immunotherapy by Human Vgamma2vdelta2 T Cells Against Human Prostate Cancer. Oncoimmunology (2021) 10(1):1989789. doi: 10.1080/2162402X.2021.1989789
151. Guo Q, Zhao P, Zhang Z, Zhang J, Zhang Z, Hua Y, et al. Tim-3 Blockade Combined With Bispecific Antibody Mt110 Enhances the Anti-Tumor Effect of Gammadelta T Cells. Cancer Immunol Immunother (2020) 69(12):2571–87. doi: 10.1007/s00262-020-02638-0
152. Van Acker HH, Anguille S, Willemen Y, Van den Bergh JM, Berneman ZN, Lion E, et al. Interleukin-15 Enhances the Proliferation, Stimulatory Phenotype, and Antitumor Effector Functions of Human Gamma Delta T Cells. J Hematol Oncol (2016) 9(1):101. doi: 10.1186/s13045-016-0329-3
153. Makkouk A, Yang XC, Barca T, Lucas A, Turkoz M, Wong JTS, et al. Off-The-Shelf Vdelta1 Gamma Delta T Cells Engineered With Glypican-3 (Gpc-3)-Specific Chimeric Antigen Receptor (Car) and Soluble Il-15 Display Robust Antitumor Efficacy Against Hepatocellular Carcinoma. J Immunother Cancer (2021) 9(12):e003411. doi: 10.1136/jitc-2021-003441
154. Oberg HH, Kellner C, Peipp M, Sebens S, Adam-Klages S, Gramatzki M, et al. Monitoring Circulating Gammadelta T Cells in Cancer Patients to Optimize Gammadelta T Cell-Based Immunotherapy. Front Immunol (2014) 5:643. doi: 10.3389/fimmu.2014.00643
155. Liu Y, Song Y, Lin D, Lei L, Mei Y, Jin Z, et al. Ncr(-) Group 3 Innate Lymphoid Cells Orchestrate Il-23/Il-17 Axis to Promote Hepatocellular Carcinoma Development. EBioMedicine (2019) 41:333–44. doi: 10.1016/j.ebiom.2019.02.050
156. Yang L, Pang Y, Moses HL. Tgf-Beta and Immune Cells: An Important Regulatory Axis in the Tumor Microenvironment and Progression. Trends Immunol (2010) 31(6):220–7. doi: 10.1016/j.it.2010.04.002
157. Kawamoto K, Pahuja A, Hering BJ, Bansal-Pakala P. Transforming Growth Factor Beta 1 (Tgf-Beta1) and Rapamycin Synergize to Effectively Suppress Human T Cell Responses Via Upregulation of Foxp3+ Tregs. Transpl Immunol (2010) 23(1-2):28–33. doi: 10.1016/j.trim.2010.03.004
158. Zaharoff DA, Hoffman BS, Hooper HB, Benjamin CJ Jr., Khurana KK, Hance KW, et al. Intravesical Immunotherapy of Superficial Bladder Cancer With Chitosan/Interleukin-12. Cancer Res (2009) 69(15):6192–9. doi: 10.1158/0008-5472.CAN-09-1114
159. Cohen J. Il-12 Deaths: Explanation and a Puzzle. Science (1995) 270(5238):908. doi: 10.1126/science.270.5238.908a
Keywords: γδT cell, cytokine, cancer, immunotherapy, cellular therapy
Citation: Song Y, Liu Y, Teo HY and Liu H (2022) Targeting Cytokine Signals to Enhance γδT Cell-Based Cancer Immunotherapy. Front. Immunol. 13:914839. doi: 10.3389/fimmu.2022.914839
Received: 07 April 2022; Accepted: 09 May 2022;
Published: 07 June 2022.
Edited by:
Andy Hee-Meng Tan, Bioprocessing Technology Institute (A*STAR), SingaporeReviewed by:
Wenwei Tu, The University of Hong Kong, Hong Kong SAR, ChinaJaydeep Bhat, Ruhr University Bochum, Germany
Copyright © 2022 Song, Liu, Teo and Liu. This is an open-access article distributed under the terms of the Creative Commons Attribution License (CC BY). The use, distribution or reproduction in other forums is permitted, provided the original author(s) and the copyright owner(s) are credited and that the original publication in this journal is cited, in accordance with accepted academic practice. No use, distribution or reproduction is permitted which does not comply with these terms.
*Correspondence: Haiyan Liu, bWljbGl1aEBudXMuZWR1LnNn