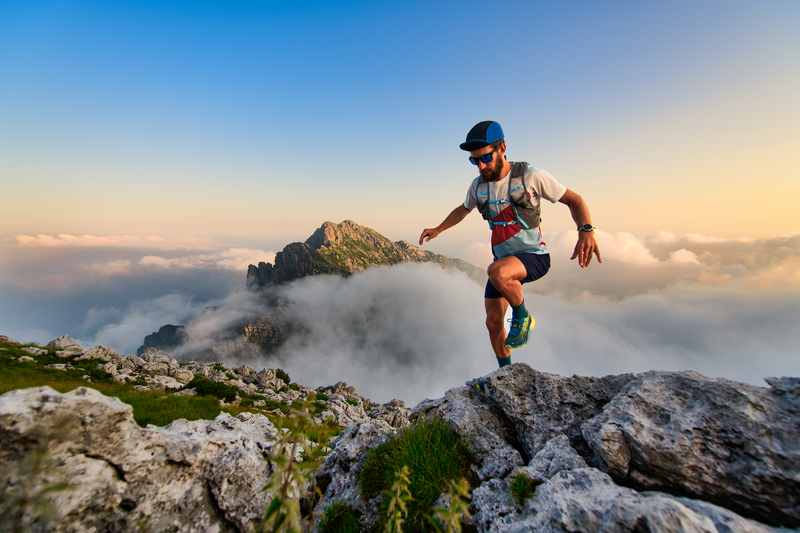
95% of researchers rate our articles as excellent or good
Learn more about the work of our research integrity team to safeguard the quality of each article we publish.
Find out more
ORIGINAL RESEARCH article
Front. Immunol. , 11 May 2022
Sec. Microbial Immunology
Volume 13 - 2022 | https://doi.org/10.3389/fimmu.2022.914010
This article is part of the Research Topic Probiotics-Modulated Intestinal Immunity against Infectious Diseases in Animals View all 21 articles
Grass carp haemorrhagic disease caused by grass carp reovirus II is a serious disease of the aquaculture industry and vaccination is the only effective method of GCRV protection. In this study, Lactococcus lactis was used as oral vaccine delivery to express the GCRV II VP6 protein. We evaluated the protective efficacy of the live vaccine strain to induce mucosal immune protection. After oral administration, the recombinant strains remained in the hindgut for antigen presentation and increased the survival rate 46.7% and the relative percent survival 42.9%, respectively versus control vaccination. Though L. lactis alone can induce the inflammatory response by stimulating the mucosal immune system, the recombinant L. lactis expressing VP6 greatly enhanced nonspecific immune responses via expression of immune related genes of the fish. Furthermore, both systemic and mucosal immunity was elicited following oral immunization with the recombinant strain and this strain also elicited an inflammatory response and cellular immunity to enhance the protective effect. L. lactis can therefore be utilized as a mucosal immune vector to trigger high levels of immune protection in fish at both the systemic and mucosal levels. L. lactis is a promising candidate for oral vaccine delivery.
Outbreaks of grass carp haemorrhagic disease (GCHD) caused by grass carp reovirus (GCRV), result in mass mortality of grass carp and serious economic losses for the aquaculture industry (1). GCRV can be classified into three different genotypes, and the results of epidemiological investigation showed that GCRV II is the major epidemic strain. An injectable vaccine has proven effective against GCRV II infections but is not an ideal immunization route due to its complex operations (2, 3). Oral immunization has many advantages such as easy operation and low labor input and can be applied to different culture conditions (4). However, environmental exposure of oral vaccines has led to antigen degradation that impeded the potency of oral vaccines (5).
Lactococcus lactis is a species of lactic acid bacteria and is a recognized safety grade microbe that has been used in oral immunization trials (6–8). L. lactis is Gram-positive bacterium and is therefore free of LPS (endotoxin) and can survive the intestinal tract and has been used as a tool for antigen presentation (9–11). Furthermore, L. lactis has multiple probiotic functions such as gut microecology regulation, improving feed utilization efficiency and enhancing innate and acquired immunity (12–14). These advantages of L. lactis may therefore allow its use as a carrier to express foreign antigenic proteins.
In order to develop an appropriate oral subunit vaccine, antigen choice is as important as the selection of a suitable delivery system (15). As with all reoviruses, GCRV II is organized as two concentric icosahedral layers: an inner core and an outer capsid layer (16). VP6 is one of the primary structural protein components of the GCRV II mature particle and is encoded by segment 9 and forms part of the viral core and plays an important role in replication (17). In particular, VP6 functions as a mediator that bridges the inner core and outer capsid and is directly inserted into position co-translationally without the involvement of ATP or chaperones. VP6 can directly bind target cell surface sialic acids (18). The VP6 protein has been shown to be immunogenic and elicit the production of neutralizing antibodies (19). Therefore, VP6 is a potential candidate protein for the development of a GCRV II oral vaccine.
GCHD is not confined to grass carp, and the rare minnow (Gobiocypris rarus) is as sensitive to GCRV II as grass carp (20). In addition, the use of the rare minnow as the model for evaluation of experimental vaccines against GCRV II infections has many advantages such as its small size, facile care, transparent egg membrane and annual spawning. These conditions are ideal for a model experimental aquatic animal. In addition, the immunogenicity and protective effects of vaccines for grass carp and rare minnow are consistent in their expression of immune-related genes, specific serum antibody titers and protection (21).
In this study, we constructed a recombinant L. lactis displaying the VP6 protein and administered the recombinant strain to rare minnow cultures that were then challenged with GCRV II. The goal of this work was to investigate whether L. lactis expression of VP6 would stimulate mucosal immunity and protection.
Rare minnows approximately 4.0 ± 0.5 cm in length were obtained from the Institute of Hydrobiology, Chinese Academy of Sciences (Wuhan, China) and acclimatized in the laboratory for 2 weeks before experimental manipulations. Fish were fed a commercial diet daily. Water temperature was maintained at 28°C. Prior to experiments, fish were randomly selected and liver, kidney and spleens were examined for the presence of GCRV using RT-qPCR as described previously (21). All animal procedures were conducted according to animal welfare standards and approved by the Ethical Committee for Animal Experiments of Pearl River Fisheries Research Institute, Chinese Academy of Fishery Sciences, China. All animal experiments complied with the guidelines of the Animal Welfare Council of China.
The GCRV II (GCRV HuNan1307 strain) and L. lactis NZ9000 were kept in our laboratory. GCRV was propagated in cultured grass carp swim bladder cells at 28°C in M199 medium containing 5% fetal bovine serum. L. lactis NZ9000 was cultured at 30°C in M17 broth medium without shaking. LD50 determinations were performed according to the method of Reed-Muench (22). Viral titers were determined by the half lethal dose (LD50) method and was typically 10–5.25 LD50/20 μL for the rare minnow.
To determine a suitable segment of VP6 expressed by L. lactis NZ9000, a protein structure set was obtained from the SWISS-MODEL web server. The transmembrane area of VP6 was analyzed with TMHMM 2.0, the secondary structure was analyzed by the PSIpred-MEMSAT 3 web server and the functional domains was analyzed through the online software the PredictProtein server.
The designed gene construct was amplified by PCR using the primers GCRV VP6-F/R (Table 1). Amplicons were subcloned into plasmid pNZ8148 (Novagen, Madison, WI, USA) using Nco I and Xba I restriction enzymes resulting in recombinant pNZ8148-VP6. The recombinant plasmid was introduced into L. lactis NZ9000 by electroporation as previously described (23). The L. lactis transformants were cultured in M17 agar containing 5 μg/mL chloramphenicol and incubated at 30°C for 24 h. Positive clones were selected and confirmed by PCR using VP6 primers (Table 1).
The recombinant strain was cultured in M17 broth medium containing 10 μg/mL chloramphenicol 30 °C until log growth phase. Protein expression was induced by the addition of using nisin (Weijia, Guangzhou, China) for 4 h. L. lactis cells were then collected by centrifugation and the pellets were suspended in PBS. The cells were lysed with the addition of 10 mg lysozyme and incubation at 37°C for 1 h. The cell lysates were then further processed as described previously (23). Protein production was analyzed using 12% SDS-polyacrylamide gel electrophoresis (SDS-PAGE) and electrotransferred to polyvinylidene fluoride membranes (Millipore, Billerica, MA, USA). The membranes were then incubated with anti-rVP6 polyclonal antibody (24) at 1:1000 in PBS-Tween-20 (PBST) for 2 h at 37°C and followed by incubation with the secondary antibody HRP-conjugated goat anti-rabbit IgG (1:5000 diluted with PBS (Sigma-Aldrich, Pittsburg, PA, USA) and visualized with the addition of diaminobenzidine (Sigma-Aldrich).
Aliquots of protein (8 μg/mL) were added to 96-well plates and incubated at 4°C overnight and washed 3× with PBST and blocked with 5% skim milk in PBS for 2 h at 37°C. Anti-rVP6 polyclonal antibody (24) was then added at 1/1000 dilution in PBS for 2 h at 37°C and washed 3× with PBST and then incubated with HRP-conjugated goat anti-rabbit IgG (1:5000 Sigma-Aldrich) for 1 h at 37°C. OPD-H2O2 substrate (Beyotime, Canton, China) was then added and the plates were incubated for 10 min. The reaction was stopped by addition of 2M H2SO4 and absorbance was measured in a microplate reader (Biotek, Santa Clara, CA, USA) at 450 nm. Samples were assayed in triplicate.
Immunization trials were conducted on specific pathogen-free rare minnows (100 fish per group). On 1, 2, 3, 21, 22 and 23 days, the fish were orally immunized with 10 μL 2 × 109 CFU/mL/fish/d of the recombinant strain and control fish were exposed to with L. lactis NZ9000 or PBS as controls (25, 26). At 3-, 7-, 14- and 21-days post-vaccination (dpv), spleen, kidney and hindgut tissues were dissected for RNA extraction and stored at -80°C. During the immunization procedure, the fish were bled twice at weeks 3 and 6 using 10 fish per group. Samples were obtained by tail ablation and collected using heparinized microcapillaries and kept at -80°C for ELISA. At 42 dpv 30 fish from vaccinated and control groups were challenged by injection with GCRV HuNan1307. Fish mortality was monitored daily for 14 days and fish tissues were examined using RT-qPCR to quantify the viral loads using the newly established method in our laboratory. The relative percentage survival (RPS) (27) was calculated by the following formula: RPS = [1-(% mortality of vaccinated fish/% mortality of control fish)] × 100.
After oral immunization with 10 μL 2×109 CFU/mL/fish, the hindgut tissues were recovered from sacrificed fish at 3, 6, 12, 24, 48 and 72 h after the first immunization and homogenized in PBS before plating serial dilutions on M17 agar plates containing chloramphenicol (5 μg/mL). Several clones were picked up randomly and analyzed using PCR amplification to detect the presence of the VP6 gene (see above).
RT-qPCR was used for relative quantitative analysis of the rare minnow cDNA obtained from spleen, kidney and hind gut tissues. The immune-related genes included Toll-like receptors 5 (TLR5), 3 (TLR3), myeloid differentiation primary response protein 88 (MyD88), nuclear factor kappa beta (NF-kB), interferon regulatory factor 7 (IRF7), Myxovirus resistance factor (Mx), interferon 2 (IFN2), major histocompatibility complex class II (MHC II) and interleukin-1β (IL-1β). β-actin was used as a reference gene for normalization (Table 1). RT-qPCR was performed using a SYBR Premix Ex Taq kit (Takara, Shiga, Japan) on an ABI 7500 fluorescence quantifier (Applied Biosystems, Foster City, CA, USA) with the following procedures: 95°C for 5 min and 35 cycles of 95°C for 15 s, 60°C for 45 s in triplicate. The expression level of immune genes was calculated using the 2-△△Ct method (21). All data were expressed as the mean ± SD.
To test the specific IgM levels against VP6 in the antisera of immunized fish, anti-IgM MAbs were prepared and kept in our laboratory. Serum antibody levels were determined by ELISA as described previously (23). Briefly, recombinant VP6 (rVP6) (24) (8 μg/mL in carbonate-bicarbonate buffer) was used as antigen to coat wells overnight at 4°C. After blocking with 5% skim milk at 37°C for 2 h, serum samples (1:50) were added and the plates were incubated for 2 h at 37°C and washed 3× with PBST and then incubated with rabbit anti-grass carp IgM (21) (1:1000) for 1 h at 37°C followed by incubation with HRP-conjugated goat anti-rabbit IgG (1:5000) for 1 h at 37°C. The wells were developed by reaction with tetramethylbenzidine substrate (Beyotime) for 10 min and the reaction was stopped by addition of 2 M H2SO4. OD values at 450 nm were taken as described above. Results of 10 samples were averaged to obtain the antibody titers of immunized fish at the different sampling times.
The experimental data was expressed as mean ± SD and the P values were calculated using one-way ANOVA with a Dunnett post-hoc test (SPSS Statistics, version 22.0, IBM, Chicago, Ill, USA). A P value of < 0.05 was considered significant, and < 0.01 was greatly significant.
The VP6 protein contained two functional domains; an N-terminal fibrous tail arranged as a triple coiled coil that serves as a virion anchor and a C-terminal globular head that interacts with the cellular receptor. These two parts form by separate trimerization events. The N-terminal fibrous tail forms on the polysome and post-translational assembly of the C-terminal globular head requires Hsp90 and binds target cell surface sialic acids and may promote apoptosis (Figure 1A). We examined the predicted structure of the N-terminus and identified 5 α-helices (residues 578-588, 596-613, 670-685, 721-737 and 740-775) connected by short aperiodic coils. The N-terminal of VP6 possessed no other complexities and was therefore suitable for the prokaryotic expression system (Figure 1B). GCRV II VP6 was amplified by PCR and cloned into the expression vector pNZ8148 and the recombinant plasmid was verified using double digestion (Figure 2A) and PCR (Figure 2B). Western blots following expression in L. lactis indicated the presence of 57.6 kDa bands from recombinant strains that were lacking in vector only controls (Figure 2C). In addition, indirect ELISA indicated a significant (p < 0.05) increase in the presence of the recombinant protein versus time of exposure to the inducer nisin (Figure 2D).
Figure 1 Function and structure predicted of the GCRV VP6. (A) Analysis of functional domains of the GCRV VP6. (B) Structure prediction of the N-terminal of the GCRV VP6.
Figure 2 Identification of the recombinant strain pNZ8148-VP6/L. lactis. (A) Identification by enzyme digestion. M: DNA maker DL5000; Lane 1: Nco I digestion; Lane 2: Xba I digestion; Lane 3: double digestion; Lane 4: pNZ8148-VP6 plasmid. (B) Identification of recombinant plasmids pNZ8148-VP6 by PCR. Lane M: DNA marker DL5000, Lanes 1–3: L. lactis pNZ8148-VP6. (C) Western blot analysis of pNZ8148-VP6 fusion protein expression, M: Low molecular weight protein maker. Lane 1: negative control L. lactis NZ9000. Lane 2: L. lactis pNZ8148-VP6. (D) Indirect ELISA analysis of pNZ8148-VP6 fusion protein expression. *p < 0.05.
We next evaluated the transit of pNZ8148-VP6/L. lactis in the rare minnow intestine. After exposure to the recombinant bacteria strain, hindgut samples at 3, 6, 12, 24, 48 and 72 h following the first immunization were collected and isolated chloramphenicol-resistant bacterial clones immediately (Figure 3A). The numbers of these colonies increased over time and reached a maximum at 6 h. The colony numbers then decreased but could still be detected and persisted at 72 h (Figure 3B). Isolated bacterial colonies also possessed the VP6 gene (Figure 3C) indicating the presence of the recombinant L. lactis in the intestines of immunized fish.
Figure 3 Colonization of strains in rare minnow intestinal tract. (A) Recombinant strain colonies in pNZ8148-VP6 group that grew on chloramphenicol M17 agar plates. (B) Colony counts obtained from hindgut tissues collected at the indicated time points following immunization. The data represent means ± SEM of 3 fish. (C) PCR specific amplification the fragment of pNZ8148-VP6 in colonies from L. lactis pNZ8148-VP6 group intestinal dilution contents. Lane M: DNA marker DL5000, Lanes 1–9: colonies picked from plates.
Immunized fish were challenged with 10 LD50 of GCRV HuNan1307 at 42 dpv and monitored for mortality and clinical symptoms for 14 d. All moribund fish presented typical clinical symptoms of GCHD including a deepening of body color, unresponsiveness and organ hemorrhaging. GCRV II could be detected in the tissues of all moribund fish. Fish mortality was first observed at 5 days post-infection in the PBS and L. lactis NZ9000 groups but 1 day later in the pNZ8148-VP6/L.lactis group. Mortality peaked for each group on day 8 following challenge. The cumulative mortality rate for the pNZ8148-VP6/L. lactis group was 53.3% and significantly (P<0.01) lower than the cumulative mortality for the PBS (93.3%) and the L. lactis NZ9000 (76.7%) groups. Hence, the protective efficacy of pNZ8148-VP6/L. lactis, compared with the PBS control yielded an RPS of 42.9%. These data indicated that the recombinant L. lactis greatly increased the survival of immunized fish against GCRV II infection (Figure 4).
Figure 4 Cumulative mortality curves of vaccinated fish exposed to GCRV-HuNan1307. Mortality was monitored daily for 14d post-challenge. *P < 0.05 between immunized and control groups at day 14.
The protective effects of the recombinant vaccine strains were then examined for their ability to induce immunity by measuring levels of specific anti-VP6 antibodies in the sera of immunized fish. Anti-VP6 levels were significantly elevated in the fish receiving the specific vaccine strain 21 days after the booster vaccination (p<0.05) and were even higher 42 days later (p<0.05). There were no significant differences in the level of specific serum antibody for the controls (Figure 5). These results indicated that the VP6 protein could be successfully delivered to the mucosal immune system of the fish by L. lactis and efficaciously induced the systemic immune response in the rare minnows.
Figure 5 Detection of serum specific antibody levels by ELISA. Levels are indicated using OD450 values and presented as mean ± SD. A comparison the L. lactis pNZ8148-VP6 group and the PBS group is shown. *p < 0.05.
Immune-related gene expression was then used to evaluate the immunized and control fish using RT-qPCR of cDNA derived from intestinal, spleen and kidney tissues. The relative expression levels of all the genes we used for screening with the exception of actin were significantly or greatly significantly up-regulated in the pNZ8148-VP6/L. lactis immunization group. This indicated that the oral immune recombinant strain induced both cellular and humoral immune responses in the fish. Specifically, intestinal levels of TLR3 were significantly up-regulated 7 dpv (p<0.05) and no significant changes in TLR5 expression were observed (p>0.05) (Figures 6A, B). Furthermore, although TLR3 expression was consistently increased 14 dpv in spleen and kidney (p<0.05), in the intestine it returned rapidly to basal levels. In the intestine, the expression level of genes downstream of TLR3 and TLR5 including NF-κB, IFN2 and Mx were also up-regulated significantly as in the spleen and kidney. In contrast, MyD88 and IRF7 in the intestine were not significantly altered (Figures 6C–G). The expression levels of MHCII in spleen, kidney and intestine were significantly increased on days 14 and 21 and returned to baseline levels at day 28 (Figure 6H). These results suggested that the pNZ8148-VP6/L. lactis strain could induce adaptive cellular immunity in both the mucosal and general immune systems. Additionally, the up-regulation of IL-1β expression could be observed in spleen, kidney and hind gut in the pNZ8148-VP6/L. lactis group indicating that the vaccine strain could cause an inflammatory response in the fish after oral immunization (Figure 6I).
Figure 6 RT-qPCR analysis of the expression of immune-related genes in different tissues of the rare minnow. (A) TLR3, (B) TLR5, (C) MyD88, (D) NF-κB, (E) Mx, (F) IFN2, (G) IRF7, (H) MHC II, (I) IL-1β. The mRNA level of each gene was normalized on the basis of β-actin gene expression. *p < 0.05, **p < 0.01 (versus PBS group).
Vaccination is considered the preferred method for reducing the severity of GCHD. Although attenuated vaccines are efficacious, they are limited because they must be physically injected in each fish and this is not compatible with the large sample sizes used in the fish culture industry. Oral vaccines are more convenient for farmers (28–31). In this study, we constructed a recombinant L. lactis strain that displayed the VP6 protein and exposure to this strain could effectively induce immune protection in the fish via oral administration.
In previous studies, VP4 (27), VP35 (28) and NS38 (29) have been tested as oral vaccine candidates. Although all these subunit vaccines generated certain levels of immune protection, the effectiveness was not as good as the injected live vaccine. The immunoprotective effects of these antigens are related to the robustness of the antigen. VP6 is a capsid component and our bioinformatics analysis indicated that the fibrous tail anchor also possessed a small region which could bind target cell surface sialic acids and may promote apoptosis. This region could elicit neutralizing antibodies and the structure was simple enough to be expressed in the prokaryote L. lactis.
Probiotics have been previously used as live vector vaccine systems and can be an effective antigen delivery system (32). L. lactis can symbiose in the gut and is safe for aquaculture and terrestrial animals to promote intestinal health (33–35). In this study, L. lactis was used to deliver the oral vaccine displaying the N-terminus of VP6. The recombinant strain could be continuously isolated and detected in the intestinal tract over 72 h indicating a compatibility with the fish gut. This also resulted in stimulation of mucosal immunity. These results were consistent with the uses of L. lactis in colonization of the intestinal tracts of terrestrial animals (26).
In order to explore the mucosal immune effect and mechanism for the generation of immunity with the recombinant L. lactis, the rare minnow was used as the model for evaluation of the oral vaccine. We assessed the cumulative mortality rate of vaccinated fish challenged with GCRV. Compared with the naive (PBS) group, the survival rate and the relative percent survival were 46.7% and 42.9%, respectively. Additionally, compared with the oral vaccines using Bacillus subtilis as the delivery system, the L. lactis presented better immunoprotective effects in fish (26). The same antigen and different inoculation routes and methods may lead a different effects for different immune activation pathways (28, 36) and immunoprotection of VP6 by injection was better than by the oral methods (37). The focus of future research will be the choosing of a proper immunopotentiator to improve the immune protection effect.
Our L. lactis vaccine generated specific IgM production in serum that was significantly (p<0.05) elevated over controls. IgM is the most important Ig isotype for teleosts and the most abundant immunoglobulin in blood and in mucosal-associated lymphoid tissue (MALT). IgM therefore plays a key role in mucosal immunity (38). Our results indicated that oral pNZ8148-VP6/L. lactis could effectively induced systemic humoral immune response in the fish.
Recombinant probiotic vaccine strains may also generate similar immunoprotective effects through different mechanisms. Oral administration of antigens can elicit an effective immune response by regulation of cytokines or other factors (39). For instance, MHC II levels on the surface of antigen-presenting cells (APC) are rapidly and significantly up-regulated in spleen, kidney and intestine. Our results indicated that the antigen carried by L. lactis was also taken up by professional APCs in the gut. These APCs can interact directly with CD4+ T helper cells to stimulate fish immunity (40–42).
The innate immunity of fish is also activated via pattern recognition receptors (PRR) (43) that are responsible for sensing the presence of pathogen-associated molecular patterns. TLR3 and TLR5 as the classical PRRs and are important sensors for the presence of invading microorganisms and play important roles in antiviral immunity (44, 45) These function via MyD88-dependent and MyD88-independent signaling pathways (46). We found that TLR3 expression first increased in intestinal tissues and 7 days later was increased in the spleen and kidney. TLR5 and MyD88 were up-regulated in the spleen and kidney 14 dpv. These results indicated that the APCs have the ability to directly activate innate immune signaling pathways in the immune tissue of the intestine. The recombinant vaccine strain also induced expression of inflammatory and cytokine related genes such as NF-κB, Mx, IRF7 and IFN2. Elevation of NF-κB was followed by Mx in spleen and kidney as well as the intestine. Additionally, vaccination induced a large-scale amplification of the signaling cascade that activates IFNs via IFN regulatory factors in the spleen and kidney. Although the expression level of IRF7 in the intestine was not up-regulated, some of the downstream genes including IFN2 were up-regulated. This was most likely a consequence of compensatory regulation from the spleen or kidney.
In summary, we constructed a recombinant L. lactis vaccine strain that displayed the GCRV VP6 protein. After oral immunization, the strain could reside in the hindgut of the fish at least 72 h to induce mucosal as well as systemic immunity. Fish survival of a GCRV challenge was also significantly enhanced by vaccination. This work highlights the use of L. lactis as a powerful oral vaccine delivery system in aquatic systems and may be used for other oral vaccines.
The original contributions presented in the study are included in the article/supplementary material. Further inquiries can be directed to the corresponding authors.
The animal study was reviewed and approved by the Ethical Committee for Animal Experiments of Pearl River Fisheries Research Institute, Chinese Academy of Fishery Sciences, China. All animal experiments complied with the guidelines of the Animal Welfare Council of China.
All authors listed have made a substantial, direct, and intellectual contribution to the work and approved it for publication.
This work was supported by National Key R&D Program of China [grant numbers 2019YFD0900103], the China Agriculture Research System of MOF and MARA [grant numbers CARS-45], Central Public-interest Scientific Institution Basal Research Fund (CAFS 2020TD45), Central Central Public-interest Scientific Institution Basal Research Fund, CAFS [grant number 2021SJ-XK1], Guangdong Provincial Special Fund For Modern Agriculture Industry Technology Innovation Teams [grant numbers 2021 KJ150], and National Freshwater Genetic Resource Center [grant number FGRC-18537].
The authors declare that the research was conducted in the absence of any commercial or financial relationships that could be construed as a potential conflict of interest.
All claims expressed in this article are solely those of the authors and do not necessarily represent those of their affiliated organizations, or those of the publisher, the editors and the reviewers. Any product that may be evaluated in this article, or claim that may be made by its manufacturer, is not guaranteed or endorsed by the publisher.
We are grateful to everyone who helped us, our institutions and our country.
1. Brudeseth BE, Wiulsrød R, Fredriksen BN, Lindmo K, Løkling KE, Bordevik M, et al. Status and Future Perspectives of Vaccines for Industrialised Fin-Fish Farming. Fish Shellfish Immunol (2013) 35(6):1759–68. doi: 10.1016/j.fsi.2013.05.029
2. Zheng YY, Qiu DK, Guo ZR, Gong YM, Wang GX, Zhu B. Evaluation of SWCNTs-Loaded DNA Vaccine Encoding Predominant Antigen Epitope VP4-3 Against Type II GCRV. Aquaculture (2021) 534:1–10. doi: 10.1016/j.aquaculture.2020.736197
3. Qiu DK, Xiong Y, Gong YM, Zheng YY, Ma R, Zhang C, et al. Dominant Antigen of Grass Carp Reovirus and Immunity Assessment With DNA Vaccine for Grass Carp. Aquaculture (2021) 530:9–7. doi: 10.1016/j.aquaculture.2020.735948
4. Cai YZ, Liu ZG, Lu MX, Ke XL, Zhang DF, Gao FY, et al. Oral Immunization With Surface Immunogenic Protein From Streptococcus Agalactiae Expressed in Lactococcus Lactis Induces Protective Immune Responses of Tilapia (Oreochromis Niloticus). Aquac Rep (2020) 18:100538. doi: 10.1016/j.aqrep.2020.100538
5. Cao P, Xu ZP, Li L. Tailoring Functional Nanoparticles for Oral Vaccine Delivery: Recent Advances and Future Perspectives. Compos Part B Eng (2022) 236:109826. doi: 10.1016/j.compositesb.2022.109826
6. Liu JK, Hou XL, Wei CH, Yu LY, He XJ, Wang GH, et al. Induction of Immune Responses in Mice After Oral Immunization With Recombinant Lactobacillus Casei Strains Expressing Enterotoxigenic Escherichia Coli F41 Fimbrial Protein. Appl Environ Microbiol (2009) 75(13):4491–7. doi: 10.1128/AEM.02672-08
7. Sáez D, Fernández P, Rivera A, Andrews E, Oñate A. Oral Immunization of Mice With Recombinant Lactococcus Lactis Expressing Cu, Zn Superoxide Dismutase of Brucella Abortus Triggers Protective Immunity. Vaccine (2012) 30(7):1283–90. doi: 10.1016/j.vaccine.2011.12.088
8. Wegmann U, O’Connell-Motherway M, Zomer A, Buist G, Shearman C, Canchaya C, et al. Complete Genome Sequence of the Prototype Lactic Acid Bacterium Lactococcus Lactis Subsp. Cremoris MG1363. J Bacteriol (2007) 189(8):3256–70. doi: 10.1128/JB.01768-06
9. Ribeiro LA, Azevedo V, Le Loir Y, Oliveira SC, Dieye Y, Piard JC, et al. Production and Targeting of the Brucella Abortus Antigen L7/L12 in Lactococcus Lactis: A First Step Towards Food-Grade Live Vaccines Against Brucellosis. Appl Environ Microbiol (2002) 68(2):910–6. doi: 10.1128/AEM.68.2.910-916.2002
10. Bahey-El-Din M, Gahan CG. Lactococcus Lactis-Based Vaccines: Current Status and Future Perspectives. Hum Vaccin (2011) 7(1):106–9. doi: 10.4161/hv.7.1.13631
11. Bahey-El-Din M. Lactococcus Lactis-Based Vaccines From Laboratory Bench to Human Use: An Overview. Vaccine (2012) 30(4):685–90. doi: 10.1016/j.vaccine.2011.11.098
12. Qiao YT, Qiu ZC, Tian FW, Yu LL, Zhao JX, Zhang H, et al. Effect of Bacteriocin-Producing Pediococcus Acidilactici Strains on the Immune System and Intestinal Flora of Normal Mice. Food Sci Hum Wellness (2022) 11(2):238–46. doi: 10.1016/j.fshw.2021.11.008
13. Obst M, Hehn R, Vogel RF, Hammes WP. Lactose Metabolism in Lactobacillus Curvatus and Lactobacillus Sake. FEMS Microbiol Lett (1992) 97(3):209–14. doi: 10.1016/0378-1097(92)90338-O
14. Qin S, Huang Z, Wang Y, Pei L, Shen Y. Probiotic Potential of Lactobacillus Isolated From Horses and Its Therapeutic Effect on DSS-Induced Colitis in Mice. Microb Pathog (2021), 105216. doi: 10.1016/j.micpath.2021.105216
15. Nazifi N, Tahmoorespur M, Sekhavati MH, Haghparast A, Behroozikhah AM. In Vivo Immunogenicity Assessment and Vaccine Efficacy Evaluation of a Chimeric Tandem Repeat of Epitopic Region of OMP31 Antigen Fused to Interleukin 2 (IL-2) Against Brucella Melitensis in BALB/c Mice. BMC Vet Res (2019) 15(1):402. doi: 10.1186/s12917-019-2074-7
16. Wang Q, Zeng W, Liu C, Zhang C, Wang Y, Shi C, et al. Complete Genome Sequence of a Reovirus Isolated From Grass Carp, Indicating Different Genotypes of GCRV in China. J Virol (2012) 86(22):12466. doi: 10.1128/JVI.02333-12
17. Yan XY, Xiong LF, Li J, Wang Y, Wu ZH, Jian JC, et al. GCRV 096 VP6 Protein and Its Impacts on GCRV Replication With Different Genotypes in CIK Cells. Aquac Fish (2018) 3(5):184–90. doi: 10.1016/j.aaf.2018.07.005
18. Marchler-Bauer A, Bo Y, Han L, He J, Lanczycki CJ, Lu S, et al. CDD/SPARCLE: Functional Classification of Proteins via Subfamily Domain Architectures. Nucleic Acids Res (2017) 45(D1):D200–D03. doi: 10.1093/nar/gkw1129
19. Xue R, Liu L, Cao G, Xu S, Li J, Zou Y, et al. Oral Vaccination of BacFish-Vp6 Against Grass Carp Reovirus Evoking Antibody Response in Grass Carp. Fish Shellfish Immunol (2013) 34(1):348–55. doi: 10.1016/j.fsi.2012.11.024
20. Chen JM, Chang OQ, Li YY, Wang YY, Liu C, Yin JY, et al. Establishment of a Rare Minnow (Gobiocypris Rarus) Disease Model for Grass Carp Reovirus Genotype II. Aquaculture (2021) 533:1–8. doi: 10.1016/j.aquaculture.2020.736133
21. Chen JM, Li YY, Wang YY, Wu SY, Chang OQ, Yin JY, et al. Establishment of a Rare Minnow (Gobiocypris Rarus) Model for Evaluation of Experimental Vaccines Against a Disease Induced by Grass Carp Reovirus Genotype II. Fish Shellfish Immunol (2021) 117:53–61. doi: 10.1016/j.fsi.2021.07.014
22. Reed LJ, Muench H. A Simple Method of Estimating Fifty Per Cent Endpoints. Am J Epidemiol (1938) 27(3):493–97. doi: 10.1093/oxfordjournals.aje.a118408
23. Yin JY, Guo CQ, Wang Z, Yu ML, Gao S, Bukhari SM, et al. Directed Chromosomal Integration and Expression of Porcine Rotavirus Outer Capsid Protein VP4 in Lactobacillus Casei ATCC393. Appl Microbiol Biotechnol (2016) 100(22):9593–604. doi: 10.1007/s00253-016-7779-y
24. Tang YF, Zeng WW, Wang Q, Wang YY, Shi CB, R F, et al. Preparation and Specification Analysis of Polyclonal Antibody Against Grass Carp Reovirus Type II VP6 Protein. Freshw Fish (2018) 48(1):9–14. doi: 10.13721/j.cnki.dsyy.2018.01.002
25. Wang N, Yin J, Wang Y, Li Y, Wu S, Shi C, et al. Isolation, Identification and Biological Characteristics of Lactobacillus From Grass Carp. South China Fish Sci (2021) 17(6):74–84. doi: 10.12131/20210039
26. Chen DD, Yao YY, Cui ZW, Zhang XY, Guo X, Zhou YY, et al. Comparative Study of the Immunoprotective Effect of Two Grass Carp-Sourced Bacillus Subtilis Spore-Based Vaccines Against Grass Carp Reovirus. Aquaculture (2019) 504:88–95. doi: 10.1016/j.aquaculture.2019.01.055
27. Sholichah L, Yuhana M, Lusiastuti AM, Prihadi TH. Potency and Efficacy Test of a Vaccine in Addition With Adjuvant Against Koi Herpesvirus in Koi (Cypronus carpio). Indonesian Aquaculture J (2016) 11:41–47. doi: 10.15578/iaj.11.1.2016.41-47
28. Hao K, Chen XH, Qi XZ, Zhu B, Wang GX, Ling F. Display of GCRV Vp7 Protein on the Surface of Escherichia Coli and Its Immunoprotective Effects in Grass Carp (Ctenopharyngodon Idella). Fish Shellfish Immunol (2018) 72:199–209. doi: 10.1016/j.fsi.2017.10.060
29. Tian Y, Ye X, Zhang L, Deng G, Bai Y. Development of a Novel Candidate Subunit Vaccine Against Grass Carp Reovirus Guangdong Strain (GCRV-Gd108). Fish Shellfish Immunol (2013) 35(2):351–6. doi: 10.1016/j.fsi.2013.04.022
30. Qiu DK, Jia YJ, Gong YM, Zheng YY, Wang GX, Zhu B. Optimizing the Immunization Procedure of Single-Walled Carbon Nanotubes Based Vaccine Against Grass Carp Reovirus for Grass Carp. Aquaculture (2021) 533:1–8. doi: 10.1016/j.aquaculture.2020.736152
31. Zeng W, Wang Q, Wang Y, Zhao C, Li Y, Shi C, et al. Immunogenicity of a Cell Culture-Derived Inactivated Vaccine Against a Common Virulent Isolate of Grass Carp Reovirus. Fish Shellfish Immunol (2016) 54:473–80. doi: 10.1016/j.fsi.2016.04.133
32. Chen XP, Ishfaq M, Wang J. Effects of Lactobacillus Salivarius Supplementation on the Growth Performance, Liver Function, Meat Quality, Immune Responses and Salmonella Pullorum Infection Resistance of Broilers Challenged With Aflatoxin B1. Poult Sci (2022) 101(3):101651. doi: 10.1016/j.psj.2021.101651
33. Cano-Lozano JA, Villamil Diaz LM, Melo Bolivar JF, Hume ME, Ruiz Pardo RY. Probiotics in Tilapia (Oreochromis Niloticus) Culture: Potential Probiotic Lactococcus Lactis Culture Conditions. J Biosci Bioeng (2022) 133(3):187–94. doi: 10.1016/j.jbiosc.2021.11.004
34. Song S, Li P, Zhang R, Chen J, Lan J, Lin S, et al. Oral Vaccine of Recombinant Lactococcus Lactis Expressing the VP1 Protein of Duck Hepatitis A Virus Type 3 Induces Mucosal and Systemic Immune Responses. Vaccine (2019) 37(31):4364–69. doi: 10.1016/j.vaccine.2019.06.026
35. Lee SH, Beck BR, Hwang SH, Song SK. Feeding Olive Flounder (Paralichthys Olivaceus) With Lactococcus Lactis BFE920 Expressing the Fusion Antigen of Vibrio OmpK and FlaB Provides Protection Against Multiple Vibrio Pathogens: A Universal Vaccine Effect. Fish Shellfish Immunol (2021) 114:253–62. doi: 10.1016/j.fsi.2021.05.007
36. Zhu B, Liu GL, Gong YX, Ling F, Wang GX. Protective Immunity of Grass Carp Immunized With DNA Vaccine Encoding the Vp7 Gene of Grass Carp Reovirus Using Carbon Nanotubes as a Carrier Molecule. Fish Shellfish Immunol (2015) 42(2):325–34. doi: 10.1016/j.fsi.2014.11.026
37. Utke K, Kock H, Schuetze H, Bergmann SM, Lorenzen N, Einer-Jensen K, et al. Cell-Mediated Immune Responses in Rainbow Trout After DNA Immunization Against the Viral Hemorrhagic Septicemia Virus. Dev Comp Immunol (2008) 32(3):239–52. doi: 10.1016/j.dci.2007.05.010
38. Magadan S, Sunyer OJ, Boudinot P. Unique Features of Fish Immune Repertoires: Particularities of Adaptive Immunity Within the Largest Group of Vertebrates. Results Probl Cell Differ (2015) 57:235–64. doi: 10.1007/978-3-319-20819-0_10
39. Sigh J, Lindenstrom T, Buchmann K. Expression of Pro-Inflammatory Cytokines in Rainbow Trout (Oncorhynchus Mykiss) During an Infection With Ichthyophthirius Multifiliis. Fish Shellfish Immunol (2004) 17(1):75–86. doi: 10.1016/j.fsi.2003.12.005
40. Barrera C, Espejo R, Reyes VE. Differential Glycosylation of MHC Class II Molecules on Gastric Epithelial Cells: Implications in Local Immune Responses. Hum Immunol (2002) 63(5):384–93. doi: 10.1016/S0198-8859(02)00386-5
41. Abualrous ET, Sticht J, Freund C. Major Histocompatibility Complex (MHC) Class I and Class II Proteins: Impact of Polymorphism on Antigen Presentation. Curr Opin Immunol (2021) 70:95–104. doi: 10.1016/j.coi.2021.04.009
42. Li XY, Du HJ, Liu L, You XL, Wu MJ, Liao ZY. MHC Class II Alpha, Beta and MHC Class II-Associated Invariant Chains From Chinese Sturgeon (Acipenser Sinensis) and Their Response to Immune Stimulation. Fish Shellfish Immunol (2017) 70:1–12. doi: 10.1016/j.fsi.2017.08.042
43. Aoki T, Hikima J-i, Hwang SD, Jung TS. Innate Immunity of Finfish: Primordial Conservation and Function of Viral RNA Sensors in Teleosts. Fish Shellfish Immunol (2013) 35(6):1689–702. doi: 10.1016/j.fsi.2013.02.005
44. Takeuchi O, Akira S. Pattern Recognition Receptors and Inflammation. Cell (2010) 140(6):805–20. doi: 10.1016/j.cell.2010.01.022
45. Cao LP, Du JL, Jia R, Ding WD, Xu P, Yin GJ, et al. Effects of Cyclophosphamide on Antioxidative and Immune Functions of Nile Tilapia (Oreochromis Niloticus) via the TLR-NF-κB Signaling Pathway. Aquat Toxicol (2021) 239:105956. doi: 10.1016/j.aquatox.2021.105956
Keywords: grass carp reovirus, Lactococcus lactis, oral vaccine, VP6, mucosal immune protection
Citation: Wang N, Li J, Wang Y, Wang Y, Zhang D, Shi C, Li Y, Bergmann SM, Mo X, Yin J and Wang Q (2022) Recombinant Lactococcus lactis Expressing Grass Carp Reovirus VP6 Induces Mucosal Immunity Against Grass Carp Reovirus Infection. Front. Immunol. 13:914010. doi: 10.3389/fimmu.2022.914010
Received: 06 April 2022; Accepted: 19 April 2022;
Published: 11 May 2022.
Edited by:
Yigang Xu, Zhejiang A&F University, ChinaReviewed by:
Hao Wang, Shanghai Ocean University, ChinaCopyright © 2022 Wang, Li, Wang, Wang, Zhang, Shi, Li, Bergmann, Mo, Yin and Wang. This is an open-access article distributed under the terms of the Creative Commons Attribution License (CC BY). The use, distribution or reproduction in other forums is permitted, provided the original author(s) and the copyright owner(s) are credited and that the original publication in this journal is cited, in accordance with accepted academic practice. No use, distribution or reproduction is permitted which does not comply with these terms.
*Correspondence: Qing Wang, c3VubnlfOTI5QDE2My5jb20=; Jiyuan Yin, eWluaml5dWFuQHByZnJpLmFjLmNu
Disclaimer: All claims expressed in this article are solely those of the authors and do not necessarily represent those of their affiliated organizations, or those of the publisher, the editors and the reviewers. Any product that may be evaluated in this article or claim that may be made by its manufacturer is not guaranteed or endorsed by the publisher.
Research integrity at Frontiers
Learn more about the work of our research integrity team to safeguard the quality of each article we publish.