- 1Department of Pathology and Laboratory Medicine, Children’s Hospital of Philadelphia, Philadelphia, PA, United States
- 2Department of Pathology and Laboratory Medicine, University of Pennsylvania, Philadelphia, PA, United States
T-regulatory (Treg) cells display considerable heterogeneity in their responses to various cancers. The functional differences among this cell type are heavily influenced by multiprotein nuclear complexes that control their gene expression. Many such complexes act mechanistically by altering epigenetic profiles of genes important to Treg function, including the forkhead P3 (Foxp3) transcription factor. Complexes that form with certain members of the histone/protein deacetylase (HDAC) class of enzymes, like HDACs 1, 2, and 3, along with histone methyltransferase complexes, are important in the induction and stabilization of Foxp3 and Treg identity. The functional behavior of both circulating and intratumoral Tregs greatly impacts the antitumor immune response and can be predictive of patient outcome. Thus, targeting these regulatory complexes within Tregs may have therapeutic potential, especially in personalized immunotherapies.
Introduction
The immune system requires the cooperation of complex cellular and molecular signaling pathways to perform its functions and maintain homeostasis. Various immune cell types are specialized in the performance of these diverse functions, including T-regulatory (Treg) cells, which limit immune responses and help maintain self-tolerance (1–3). The function of Tregs depends on the regulation and manipulation of their gene expression. With few exceptions, the expression of forkhead P3 (Foxp3), CD25, CTLA4, HELIOS, and GITR defines the Treg lineage identity (4), although various subsets of Tregs display additional characteristics (5). The expression of Foxp3, the master regulator component of Treg transcription (6, 7), is controlled in multiple ways, including epigenetic mechanisms. Histone methylation, acetylation, ubiquitination, and DNA methylation all contribute to the epigenetic regulation of Foxp3+ Tregs, both by direct regulation of Foxp3 expression and by regulation of the total Foxp3+ Treg transcriptional identity (8). A consistent epigenetic feature of Tregs with stable Foxp3 expression is hypomethylation at the conserved non-coding sequence 2 (CNS2 or Treg-specific demethylation region, aka TSDR) site (9). Foxp3+ Tregs that lack a stable epigenetic profile can lose Foxp3 and gain proinflammatory IL-17 expression, and IL-17-producing Tregs contribute to the pathology of multiple inflammatory diseases (10, 11). Histone modification, contrary to DNA modification, tightly controls more transient regulation of gene expression.
Foxp3+ Tregs rely upon histone modification via acetylation, methylation, and ubiquitination to regulate and shift their acute transcriptional functions. Histone acetylation occurs on lysine residues of histones and leads to less condensed chromatin (euchromatin), thus allowing access of transcription factors to the DNA. Enzymes with opposing actions, histone/protein acetyltransferases (HATs) and histone/protein deacetylases (HDACs), are responsible for the addition or removal of acetyl groups on histones (12). Higher HAT activity and lower HDAC activity support transcriptional gene expression. Consistent with that, chromatin surrounding actively transcribed Foxp3 genes is hyperacetylated on histones H3 and H4 (13) (Figure 1). Histone acetylation has an important role in Foxp3 regulation mediated by three HATs and many HDACs (13, 14). These enzymes can also catalyze post-translational modifications of Foxp3. Thus, 3 HATs (CBP, p300 and TIP60, and p300/CBP) play critical roles in Foxp3 acetylation and promote Treg function (15–17), whereas at least 12 HDACs, (HDACs 1, 2, 3, 5, 6, 7, 8, 9, 10, and 11 and SIRT1 and SIRT3) can catalyze Foxp3 deacetylation with varying effects on Treg function (18–22) (Table 1).
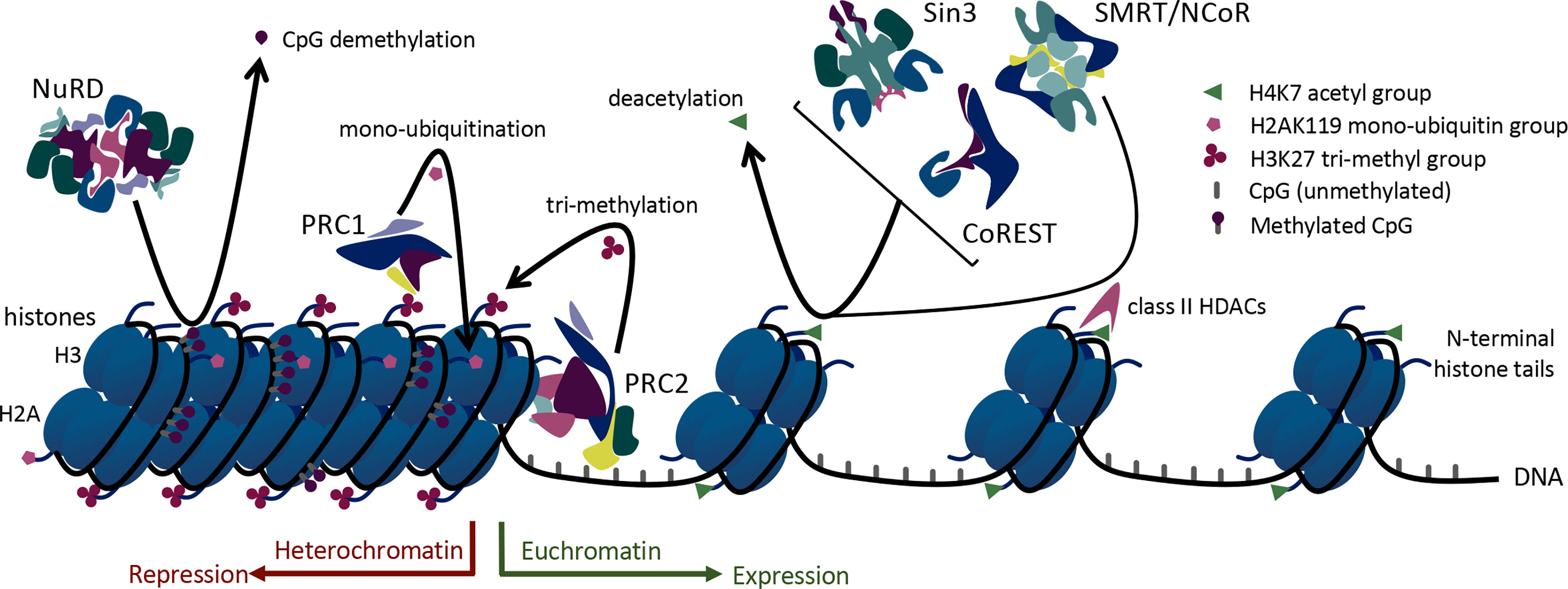
Figure 1 Mechanisms of epigenetic regulation utilized by large multiprotein complexes in Treg cells.
The 18 known HDACs are organized into 5 classes. HDACs involved in Foxp3 regulation come from all 5 HDAC classes: class I (HDACs 1, 2, 3, and 8), class IIa (HDACs 5, 7, and 9), class IIb (HDACs 6 and 10), class IV (HDAC11), and class III (SIRT1, 2 and 3). Class I, II, and IV HDACs have Zn2+-dependent catalytic activity (32), whereas class III sirtuins are NAD+-dependent and/or ADP ribosylase enzymes (33). Some HDACs, especially class I members, form large protein complexes that are recruited by methyl-binding domain proteins to selectively bind to methylated cytosines, which are frequently observed in the promoter regions of silenced genes (Figure 2). Such regulatory complexes include the switch independent 3 (Sin3), nucleosome remodeling and deacetylase (NuRD), mitotic deacetylase (MiDAC), a corepressor of REST (CoREST), and silencing mediator for retinoid or thyroid-hormone receptors/nuclear receptor corepressor (SMRT/NCoR). The Sin3, NuRD, MiDAC, and CoREST complexes include HDAC1 and HDAC2 as the core enzymatically active component(s), while SMRT/NCoR utilizes HDAC3.
In addition to these regulatory complexes that modify histone acetylation, other epigenetic regulatory complexes influence histone methylation and ubiquitination. For example, members of the large multiprotein polycomb repressive complexes (PRCs) help control the methylation and ubiquitination of Foxp3. PRCs 1 and 2 are involved in epigenetic regulation of transcription via ubiquitin ligase and methyltransferase activities, respectively. Both histone methylation and ubiquitination via PRCs lead to chromatin condensation and transcriptional repression (34) (Figure 1). In addition, non-enzymatic chromatin remodeling functions of PRC1 can activate or repress genes, at least in Drosophila (35). Like the HDAC-associated multiprotein regulatory complexes, PRC2/1 influences the transcriptional availability of genes important to Tregs including Foxp3 itself, thus influencing the development and stability of Treg cells (Figure 2).
These large regulatory complexes contribute to a wide array of cellular functions and processes such as cell cycle, DNA repair and replication, embryo development, stem cell lineage determination, and cell lineage maintenance in diverse tissues and cell types. The activity of most of these epigenetic regulatory complexes typically results in transcriptional repression, and some complexes are exclusively considered corepressors. However, some are coregulators, as they influence both transcriptional activation and repression. Epigenetic coregulatory complexes contribute to genetic regulation within Foxp3+ Tregs both by direct epigenetic modification of Foxp3 promotor TSDR/CNS2 and by participating, in coordination with Foxp3 protein, in establishing and maintaining the Treg transcriptional program (8).
A primary function of Treg cells is to suppress the activity of effector immune cells. In the context of cancer pathology, increased activity of Tregs leads to increased tumor growth and poor patient outcomes (36). Thus, inhibition of Treg function is of interest with respect to developing new anticancer therapies, though options to do so using isoform-selective HDAC inhibitors are basically limited to HDAC3 and HDAC8. By contrast, HDACs 1 and 2, which share 83% identity, are key enzymatic components of several coregulator complexes, often in conjunction with additional enzymes. Within Tregs, histone-modifying, multiprotein regulatory complexes that contribute to controlling the Treg transcriptional program offer promising targets to inhibit suppressive function and will be reviewed.
CoREST
The CoREST complex is a chromatin-modifying transcriptional coregulator that contains two enzymes with different catalytic activities. In addition to the deacetylase activity of HDAC 1 or 2, the CoREST complex includes lysine-specific demethylase (LSD1). LSD1 targets mono- and di-methylated H3K4 and H3K9. Both catalytic functions can be executed by the CoREST complex, although not necessarily simultaneously (37, 38). HDAC1 or its close paralog HDAC2, together with LSD1 and the scaffolding protein, CoREST1/2/3 (aka RCOR1/2/3), form the overall CoREST complex (39, 40). Homology indicates that the ELM2-SANT domain of CoREST1/2/3 recruits HDAC1/2 and the SANT2 domains of CoREST1/2/3 are proposed to directly interact with DNA (40). The CoREST complex regulates gene expression in neuronal cells and dictates the fate of neuronal stem cells (41). It also has a well-established role in the epigenetic regulation of the hematopoietic system during embryonic development (42).
Transcriptional regulation within Tregs relies on CoREST-mediated repression of proinflammatory genes. Tregs that lack CoREST (Rcor1) undergo functional reprogramming through upregulation of proinflammatory transcription factors, cytokines, and chemokine receptors, including STAT1, T-bet, IL-2, IFN-γ, and CXCR3, mediated by increased H3K9-acetylation and H3K4-dimethylation through reduced recruitment of histone-modifying enzymes to the promotors of proinflammatory genes (43). Diminished suppressive and anti-inflammatory functions accompany the enhanced proinflammatory characteristics of Tregs lacking CoREST (44). Deletion of HDAC1 in Tregs leads to impaired function, while deletion of HDAC2 enhances function (27). HDAC inhibitors that bind both HDAC1 and HDAC2 with differing dissociation constants show relative HDAC2 selectivity (45, 46) and promote Treg functions in vitro, but their use has not been explored in vivo (23). In contrast, when the CoREST complex is inhibited via dual pharmacologic targeting of HDAC and LSD1 enzymes, Treg function is significantly curtailed in vitro and in vivo. Indeed, the use of corin (a dual-activity CoREST inhibitor) impaired Foxp3+ Treg function and promoted antitumor immunity in murine models (43, 44). A newly described non-canonical role of CoREST associating with RNA Polymerase II during transcription and deacetylating its carboxy-terminal domain at lysine 7 to inhibit productive elongation may also contribute to CoREST activity (47). Inhibition of the CoREST complex by various methods is a promising area of interest for cancer immunotherapy.
NuRD
The NuRD complex is an ATP-dependent chromatin remodeling large multiprotein complex involved in the regulation of gene transcription, genome stability, cell cycle progression, and DNA damage replication and repair response (48, 49). The core of the NuRD complex is composed of HDAC1/2, metastasis-associated proteins (MTA1/2/3), methyl-CpG binding domain protein 2/3 (MBD2/3), and retinoblastoma-binding proteins (RBBP4/7) in an elongated zigzag conformation (50). Two HDAC1/2 components form a dimer mediated by the ELM2-SANT domains of MTA1, and four RBBP4/7 proteins bind the C terminuses of the dimerized MTA1 proteins; two RBBP4/7 proteins bind one MTA1 protein (51). MBD2 and MTA1 subunits mediate NuRD complex binding to methylated DNA (52, 53). Other more transient components of the complex include p66alph/beta, deleted in oral cancer 1 (DOC1), and CHD3/4 (54–56). CHD3/4 subunits of the NuRD complex are responsible for its chromatin remodeling function via ATP-dependent helicase activity, while the HDAC1/2 subunits are responsible for its deacetylase activity (50). LSD1, a core component of the CoREST complex, has also been shown to associate with NuRD in some cell types (51), adding to the versatility and enzymatic potential of this regulatory complex.
The Mi-2/NuRD complex is an abundant deacetylase complex with a broad cellular and tissue distribution and is unique in that it couples histone deacetylation and chromatin-remodeling ATPase activities in the same complex (57). NuRD can perform its many functions using both catalytic and non-catalytic mechanisms. NuRD plus Bcl6 regulates the transcriptional program of both T follicular effector cells and follicular Tregs (54). In another study, the NuRD complex has displayed an unexpected function in its regulation of Foxp3 expression. The MBD2 component of NuRD, which typically mediates DNA-NuRD interaction leading to gene repression, instead promotes the demethylation of Foxp3 TSDR/CNS2 site by recruiting Tet enzymes and enhances Treg function (55). This is currently a mechanism utilized by MBD2/NuRD uniquely within Treg cells and provides a very useful therapeutic target.
Sin3
The switch-independent proteins, Sin3a and Sin3b, interact with HDACs 1 and/or 2, suppressors of defective silencing 3 (SDS3), sin3a-associated protein p30 (SAP30), FAM60, and RBBP4/7 to form the Sin3 coregulatory complex (58–60). The cofactors structure around Sin3a/b scaffolding proteins (61), which then form dimers through interaction between extended coiled-coil regions of the SDS3 proteins (62). While Sin3a and Sin3b are similar proteins, they can produce varying functions as part of the Sin3 complex (63). HDAC1/2 provides the sole enzymatic functionality of the Sin3 complex and is required for full deacetylase functionality, although its functions expand beyond acetyltransferase activities (64).
Originally described as a transcriptional corepressor (65, 66), the Sin3 complex is now appreciated to also function as a transcriptional activator (67, 68) and is therefore now considered a transcriptional coregulator. Sin3a was first identified in yeast and is highly conserved throughout mammalian species (69). Functions of the Sin3a complex are required for mammalian embryogenesis (70), T-cell lineage development (70), and transcriptional responses to hypoxia (71). In the case of Treg cells, the Sin3 complex interacts with and silences the transcriptional expression of Foxp3 and decreases Treg-suppressive function (72). In addition to silencing Foxp3, emerging research suggests Sin3a influences widespread transcriptional regulation within Tregs, with both inhibitory and enhancing actions (73, 74). The use of Sin3-specific peptide inhibitors in addition to avermectin is effective in impairing tumor growth in models of triple-negative breast cancer (75, 76), demonstrating that the Sin3 complex may be a beneficial target for therapeutic development.
MiDAC
Mitotic deacetylase complex (MiDAC) is a chromatin remodeling corepressor complex recently identified in affinity chromatography studies of extracts of dividing cells exposed to HDAC inhibitors (77). The MiDAC complex is composed of HDAC1/2, deoxynucleotidyltransferase terminal-interacting protein 1 (DNTTIP1), and mitotic deacetylase-associated SANT domain (MIDEAS) protein and associates with cyclin A2 (CCNA2) and cyclin-dependent kinase (CDK2) (77–79). There are four copies of each protein component of MiDAC within a single complex, where they form a dimer of dimers completing a tetrameric structure. Dimerization of this complex is mediated by the ELM2-SANT domains of MIDEAS along with the N-terminal regions of DNTTIP1 (40). The C-terminal of DNTTIP1 has been suggested to interact directly with DNA and nucleosomes due to its structural relation to SKI/SNO/HDAC (80). MiDAC can modulate gene expression by negatively regulating the repressive histone mark H4K20ac or the active histone mark H3K27ac, respectively, leading to roles as an activator or repressor of different gene sets (81). MiDAC is required for late embryogenesis since deletion of MIDEAS or DNTTIP1 impairs cardiac development and hematopoiesis (82). However, no role for MiDAC in the regulation of immune functions has yet been reported.
SMRT/NCoR
SMRT and NCoR are homologous non-redundant corepressor proteins that interact with transcriptional repressors and hormone receptors (unbound to ligand). SMRT and NCoR together with transducin beta-like protein 1 (TBL1), G protein pathway suppressor 2 (GPS2), and HDAC3 form the SMRT/NCoR complex (83). The N-termini of TBL1 proteins bind as a tetramer that interacts with the remaining complex proteins SMRT/NCoR, HDAC3, and GPS2 (40). HDAC3 deacetylase activity requires binding to SMRT/NCoR (84). The complex also interacts with class IIa HDACs 4, 5, 7, and 9 (85). Class IIa HDACs have relatively low catalytic activity and act primarily via protein–protein interactions and/or participation in large multiprotein complexes such as SMRT/NCoR (86–88). Indeed, the primary role of class IIa HDACs may be to function as acetyl-lysine binding proteins that recruit HDAC3/SMRT/NCoR (89). The SMRT/NCoR repressor complex has significant roles in cardiac and neuronal development and the maintenance of metabolic and immune homeostasis (90). HDAC3 deletion in Tregs derepresses Treg production of IL-2 and is associated with the rapid development of lethal autoimmunity (24). The HDAC3/SMRT/NCoR complex activity is essential for the development and suppressive functions of Foxp3+ Tregs via direct Foxp3-HDAC3 interaction (24). Hence, targeting this complex, its interaction with Foxp3, and/or its interaction with class II HDACs is a promising therapeutic strategy for disrupting Treg function for anticancer immunity.
PRC1/2
PRC1 and PRC2 are epigenetic regulators originally identified in Drosophila as important to cell lineage determination and maintenance through transcriptional repression (35). Recent studies have shown PRC1 functioning to anchor activating and enhancing loops (91), broadening understanding of the functions of these complexes. PRC2 binds unmethylated CpG islands of repressed genes, resulting in trimethylation of surrounding histones on H3K27 residue. Canonical PRC1 then recognizes the trimethylated H3K27 (H3K27me3) residues and further represses the gene by monoubiquitination of H3AK119 or by promoting non-enzymatic chromatin condensation (92).
Components of the canonical PRC1 complex include obligate heterodimer ubiquitin ligase ring finger protein 1 (RING1A/B) and nucleosome binding subunit chromo box (CBX), together with polyhomeotic homolog (PHC1, PHC2, and PHC3) and polycomb group ring finger protein (PCGF2, 3, and 4) subunits (40). PRC1 represses gene transcription through enzymatic histone ubiquitination and non-enzymatic chromatin re-structuring and condensation mechanisms (34). RING1A/B catalyzes the ubiquitination of histones H2A on lysine 119 (H2AK119). Core components of PRC2 include enhancer of zeste homolog 1 (EZH1) or EZH2, embryotic ectoderm development (EED), and suppressor of zeste 12 (SUZ12) together with the more transiently associated subunits of AE binding protein 2 (AEBP2), Jumonji and AT-rich interaction domain containing 2 (JARID2), and RBAP46 (aka RBBP7) or RBAP48 (aka RBBP4). Active PRC2 represses gene transcription via histone di- or tri-methylation of H3K27. The enzymatically active component of the PRC2 complex consists of the histone methyltransferases, EZH1 or EZH2, which facilitate the addition of methyl groups to histones (92). While PRC1 and PRC2 are often expressed coordinately, they can also function independently in certain cell types.
The Treg master transcription factor, Foxp3, is subject to epigenetic repression by PRC1 and 2 in the classical PRC2/1 repression and maintenance model. PRC-associated elements recruit PRC2 to unmethylated CpG regions of the Foxp3 promoter, resulting in histone methylation of the region and therefore transcriptional repression of Foxp3 (93). PRC1 complex containing the heterodimeric RING1A/B and PCGF4 homolog (BMI1) recognizes PRC2-mediated H3K27me3 of the Foxp3 promoter(s) and functions to maintain its inactivated state via ubiquitination of H3AK119 (94). The EZH2 methyltransferase helps maintain Treg identity and function following activation by being recruited to the Foxp3 protein and leading to repression of genes within the Foxp3 transcriptional program (93–96).
PRC subunits tend to be upregulated in cancers such as melanomas, lymphomas, and prostate and breast cancers (41). Mutations, both gain-of-function and loss-of-function, in PRC2 components especially EZH2 can lead to various cancer manifestations. EZH2 dysregulation specifically has been associated with particularly aggressive cancers and malignancies (92). PRC2 complex activity has exhibited oncogenic and tumor-suppressive functions (35, 92). Interestingly, perturbations in PRC2 and/or H3K27me3, which occur in various hematopoietic malignancies, also render the cancerous cells susceptible to PRC2/1 complex inhibition (97). PRC2 could potentially make a very effective target for Treg inhibition because of its role in maintaining the Foxp3-lead transcriptional profile during Treg activation. For the same reason, it would most likely provide a useful therapeutic used in combination with a different functional target.
Discussion
While Treg cells play an essential role in maintaining a homeostatic balance within the immune system, their suppressive activity impedes the effector immune response to tumors. When Treg function is abrogated, the effector immune response is unrestrained, and anticancer immunity increases. For these reasons, Tregs provide a valuable target for immunotherapies, especially as part of combination therapy. The 5 large epigenetic regulatory complexes discussed in this review provide targets for inhibition within Tregs, given their involvement in the maintenance and stability of Foxp3+ Treg function. The complexes function both by epigenetic modification of the TSDR/CNS2 promotor of Foxp3 and by direct interaction with the Foxp3 protein. Despite only limited evidence about the contributions of these multiprotein complexes in Treg biology, the available data suggest the potential to exploit one or more of these complexes to promote antitumor immunity.
HDAC family members are often overexpressed in human cancers (98), histone H4 is commonly deacetylated in human primary malignancies (99), and low acetylation of histone H3 is a predictor of poor outcomes in pancreatic, breast, gastric, ovarian, prostate, and lung cancers (100, 101). Because of this and their roles in transcriptional regulation, HDAC inhibitors have been tested in various cancer models and clinical trials (Table 2), though with widely varying results (101). HDAC inhibitors are effective against the progression of various cancers, especially hematological malignancies such as cutaneous T-cell lymphoma (99). HDAC inhibitors have varying effects on Foxp3 expression and Treg-suppressive functions (Table 1) (27, 102). An optimal strategy would be to specifically target individual HDACs within Tregs for anticancer therapeutics. In particular, HDACs 1 and 2 are interchangeably present within NuRD, CoREST, MiDAC, and Sin3 complexes, and when knocked out of Tregs, these two HDACs have opposing effects on Treg function. The lack of HDAC1 decreases Treg-suppressive functions, while HDAC2 increases Treg-suppressive functions (Table 1). Thus, a specific inhibitor of HDAC2 would be a promising pharmacologic tool for Treg repression in cancer therapy. Unfortunately, such compounds are yet to be available and have been difficult to generate (40). Efforts to overcome this obstacle have included targeting multiple members of a given complex or blocking interactions between complex members to disrupt their formation. An example of the former is the dual-inhibitor corin, which targets both the HDAC and LSD1 components of the CoREST complex (44). When used to treat mice with TC1 lung tumors, corin decreased Foxp3+ Treg function and promoted antitumor immunity (43, 44). This method of dual-target inhibition could be effective on the NuRD complex by targeting the active domains of the HDAC and MBD2 components. The MBD2 component of NuRD is of particular interest in that the mechanism of activating genetic expression by MBD2/NuRD is unique within Tregs (55).
Complex inhibition by blocking interactions/binding between complex members to disrupt complex formation is another strategy being explored to decrease Treg function. In the case of targeting components of the PRC2/1 complexes to increase anticancer immunity, inhibition of EZH2 has been effective against rare sarcomas and follicular lymphomas (Table 2). The expression of EZH2 has been associated with poor clinical outcomes in cancer patients, contributing to metastasis, metabolism, drug resistance, and angiogenesis (103), while conversely displaying tumor-suppressive functions (104). Cancer cells that express EZH2 have reduced CXCR9 expression (105) and decreased effector T-cell infiltration of tumors (106). Treatment of solid tumors with EZH2 inhibitors increases the recruitment and function of CD4+ and CD8+ effector T cells by induction of an inflammatory phenotype within tumor-infiltrating Tregs (107). Promising advances for this class of cancer therapeutics disrupt the complex formation of PRC2 rather than EZH2 enzymatic activity. Compounds A769662 (108) and MAK-683 (109, 110) accomplish this by blocking EZH2 interaction with PRC2 components SUZ12 and EED, respectively. MAK-683 is currently undergoing clinical trials for the treatment of diffuse large B-cell lymphoma (Table 2).
Other approaches in targeting Tregs for cancer interventions include activation of epigenetic regulatory complexes that function to repress Foxp3 and Treg function. For example, inositol phosphates activate the deacetylase activity of SMRT/NCoR, NuRD, and MiDAC (80, 84). Considering that NuRD and SMRT/NCoR have been found to induce Foxp3 expression in Tregs, the use of inositol phosphates could be particularly useful for the anticancer treatment or as part of a combination regimen (84).
Anticancer therapies targeting HDACs and EZH2 within epigenetic regulatory complexes have been individually effective at treating various cancer types and have received Food and Drug Administration (FDA) approval (Table 2). However, such therapeutics may potentially perform better in combination with drugs targeting separate cellular mechanisms. HDAC inhibitors used in combination with checkpoint inhibitors that target proteins such as PD-1/PDL1 or CTLA4 show therapeutic promise. Clinical trials for cervical cancer and cervical neoplasm with HDAC inhibitor toripalimab and PD-1 inhibitor chidamide. Such HDAC inhibitors can function to prime or sensitize cells for the checkpoint inhibitors to induce anticancer immunity. Another strategy for combination therapies involves the EZH2 inhibitor, SHR2554, currently in clinical trials as both an independent therapy and in combination with anti-PD-L1/TGFβ antibody, SHR1701. Further, in preclinical studies, EZH2 inhibition in Ewing sarcoma induces the expression of ganglioside G-D2, which is then targeted with gene-modified T cells, resulting in tumor regression (111). This is just one example of regulatory complex inhibition in combination with adoptive T-cell therapy, albeit a strategy gaining in popularity.
Anticancer therapeutics that target epigenetic regulation within Tregs have been effective clinically. While this has not been universally the case, our understanding, development, and employment of such therapeutics continue to progress, providing for more precise and effective therapeutic methods. Modulation of the epigenetic state of immune cells through targeting multiprotein regulatory complexes can involve new approaches. For example, in chimeric antigen receptor T (CAR-T) cell therapy, CAR-T cells could potentially be treated ex vivo with HDAC- or EZH2 complex-targeted inhibitors to stabilize their functional identity. A better understanding of the roles and mechanisms of large epigenetic regulatory complexes will advance the development of both conventional and innovative strategies for cancer immunotherapies.
While anticancer therapies continue to improve in efficacy and precision, obstacles persist. Collectively, these regulatory complexes are essential in establishing and maintaining cell lineage identity and therefore could be expected to be dysregulated in cancers. Indeed, this is usually the case; furthermore, mutations in the genes causing dysfunction often induce cancer development and contribute to cancer pathology (98). Dysregulation of the complexes and individual complex components, within cancer cells and tumor microenvironments, could result in altered effects of therapeutic inhibitors. In addition, the functions performed by these complexes are dynamic in that they vary in differing environments with and in response to specific regulatory landscapes. Functional variations are influenced by physical conformation, chemical modifications, and the availability and/or incorporation of cofactors to the complexes. The described plasticity of Foxp3 transcriptional regulation presents a barrier with respect to the prospect of Treg-specific therapeutic interventions (112), and designing and generating compounds to target large multiprotein complexes are inherently challenging (40). In addition, having various transcriptional functions in alternate cell types of these complexes, off-target effects provide additional obstacles to be considered. Indeed, there exists much room for improvement, and many obstacles persist, yet modulation of Treg function for therapeutic intervention against cancer is effective and continuously improving.
Conclusion
As with other cell types, large multiprotein complexes are involved in the regulation of Foxp3+ Treg development, stability, and function. Except for MiDAC, about which little is known in terms of its immune functions, these complexes directly influence the transcription of Foxp3 gene. As coregulators, histone modification represses Foxp3 when facilitated by CoREST, Sin3a, and PRC2/1, while SMRT/NCoR and NuRD complexes enhance Foxp3 expression. The variation in function among the complexes deepens when individual subunits are taken into consideration, as shown by the opposing effects of HDAC1 and HDAC2 on Foxp3 expression in Tregs. Some of the complexes demonstrate multiple functions within Tregs. In addition to direct regulation of Foxp3 gene, CoREST, SMRT/NCoR, and PRC2 interact with the Foxp3 protein and contribute to the regulation of the Treg transcriptional program. These epigenetic regulatory complexes play important roles in the control of the transcriptional activity of Treg cells, which make them promising targets for the development of future anticancer therapeutic strategies.
Author Contributions
LC drafted the review. LC and WH prepared the final version of the text. LC prepared the graphics. All authors listed have made a substantial, direct, and intellectual contribution to the work and approved it for publication.
Funding
This work was supported in part by the National Institutes of Health (1R01CA253320).
Conflict of Interest
The authors declare that the research was conducted in the absence of any commercial or financial relationships that could be construed as a potential conflict of interest.
Publisher’s Note
All claims expressed in this article are solely those of the authors and do not necessarily represent those of their affiliated organizations, or those of the publisher, the editors and the reviewers. Any product that may be evaluated in this article, or claim that may be made by its manufacturer, is not guaranteed or endorsed by the publisher.
References
1. Sakaguchi S, Wing K, Miyara M. Regulatory T Cells - a Brief History and Perspective. Eur J Immunol (2007) 37(Suppl 1)::S116–123. doi: 10.1002/eji.200737593
2. Sakaguchi S, Miyara M, Costantino CM, Hafler DA. FOXP3+ Regulatory T Cells in the Human Immune System. Nat Rev Immunol (2010) 10:490–500. doi: 10.1038/nri2785
3. Rudensky AY. Regulatory T Cells and Foxp3. Immunol Rev (2011) 241:260–8. doi: 10.1111/j.1600-065X.2011.01018.x
4. von Knethen A, Heinicke U, Weigert A, Zacharowski K, Brune B. Histone Deacetylation Inhibitors as Modulators of Regulatory T Cells. Int J Mol Sci (2020) 21:2356. doi: 10.3390/ijms21072356
5. Kohne M, Beyer M. ATAC-Ing Human Tissue Treg Cells. Immunity (2021) 54:605–7. doi: 10.1016/j.immuni.2021.03.014
6. Li B, Samanta A, Song X, Iacono KT, Brennan P, Chatila TA, et al. FOXP3 Is a Homo-Oligomer and a Component of a Supramolecular Regulatory Complex Disabled in the Human XLAAD/IPEX Autoimmune Disease. Int Immunol (2007) 19:825–35. doi: 10.1093/intimm/dxm043
7. Khattri R, Cox T, Yasayko SA, Ramsdell F. An Essential Role for Scurfin in CD4+CD25+ T Regulatory Cells. Nat Immunol (2003) 4:337–42. doi: 10.1038/ni909
8. Rudra D, deRoos P, Chaudhry A, Niec RE, Arvey A, Samstein RM, et al. Transcription Factor Foxp3 and Its Protein Partners Form a Complex Regulatory Network. Nat Immunol (2012) 13:1010–9. doi: 10.1038/ni.2402
9. Helmin KA, Morales-Nebreda L, Torres Acosta MA, Anekalla KR, Chen SY, Abdala-Valencia H, et al. Maintenance DNA Methylation Is Essential for Regulatory T Cell Development and Stability of Suppressive Function. J Clin Invest (2020) 130:6571–87. doi: 10.1172/JCI137712
10. Voo KS, Wang YH, Santori FR, Boggiano C, Wang YH, Arima K, et al. Identification of IL-17-Producing FOXP3+ Regulatory T Cells in Humans. Proc Natl Acad Sci USA (2009) 106:4793–8. doi: 10.1073/pnas.0900408106
11. Jung MK, Kwak JE, Shin EC. IL-17a-Producing Foxp3(+) Regulatory T Cells and Human Diseases. Immune Netw (2017) 17:276–86. doi: 10.4110/in.2017.17.5.276
12. Yang XJ, Seto E. HATs and HDACs: From Structure, Function and Regulation to Novel Strategies for Therapy and Prevention. Oncogene (2007) 26:5310–8. doi: 10.1038/sj.onc.1210599
13. Xiao Y, Li B, Zhou Z, Hancock WW, Zhang H, Greene MI. Histone Acetyltransferase Mediated Regulation of FOXP3 Acetylation and Treg Function. Curr Opin Immunol (2010) 22:583–91. doi: 10.1016/j.coi.2010.08.013
14. Dong Y, Yang C, Pan F. Post-Translational Regulations of Foxp3 in Treg Cells and Their Therapeutic Applications. Front Immunol (2021) 12:626172. doi: 10.3389/fimmu.2021.626172
15. Liu Y, Wang L, Han R, Beier UH, Akimova T, Bhatti T, et al. Two Histone/Protein Acetyltransferases, CBP and P300, Are Indispensable for Foxp3+ T-Regulatory Cell Development and Function. Mol Cell Biol (2014) 34:3993–4007. doi: 10.1128/MCB.00919-14
16. Xiao Y, Nagai Y, Deng G, Ohtani T, Zhu Z, Zhou Z, et al. Dynamic Interactions Between TIP60 and P300 Regulate FOXP3 Function Through a Structural Switch Defined by a Single Lysine on TIP60. Cell Rep (2014) 7:1471–80. doi: 10.1016/j.celrep.2014.04.021
17. Bin Dhuban K, d'Hennezel E, Nagai Y, Xiao Y, Shao S, Istomine R, et al. Suppression by Human FOXP3(+) Regulatory T Cells Requires FOXP3-TIP60 Interactions. Sci Immunol (2017) 2:eaai9297. doi: 10.1126/sciimmunol.aai9297
18. Li B, Samanta A, Song X, Iacono KT, Bembas K, Tao R, et al. FOXP3 Interactions With Histone Acetyltransferase and Class II Histone Deacetylases are Required for Repression. Proc Natl Acad Sci USA (2007) 104:4571–6. doi: 10.1073/pnas.0700298104
19. de Zoeten EF, Wang L, Butler K, Beier UH, Akimova T, Sai H, et al. Histone Deacetylase 6 and Heat Shock Protein 90 Control the Functions of Foxp3(+) T-Regulatory Cells. Mol Cell Biol (2011) 31:2066–78. doi: 10.1128/MCB.05155-11
20. Beier UH, Wang L, Bhatti TR, Liu Y, Han R, Ge G, et al. Sirtuin-1 Targeting Promotes Foxp3+ T-Regulatory Cell Function and Prolongs Allograft Survival. Mol Cell Biol (2011) 31:1022–9. doi: 10.1128/MCB.01206-10
21. Huang J, Wang L, Dahiya S, Beier UH, Han R, Samanta A, et al. Histone/protein Deacetylase 11 Targeting Promotes Foxp3+ Treg Function. Sci Rep (2017) 7:8626. doi: 10.1038/s41598-017-09211-3
22. Dahiya S, Beier UH, Wang L, Han R, Jiao J, Akimova T, et al. HDAC10 Deletion Promotes Foxp3(+) T-Regulatory Cell Function. Sci Rep (2020) 10:424. doi: 10.1038/s41598-019-57294-x
23. Wang L, Samanta A, Levine MH, Beier UH, Han R, Kalin J, et al. Vital Role of the CoREST Complex as a Master Regulator of Foxp3+ T-Regulatory Cell Gene Expression and Suppressive Function. Am J Transplant (2017) 17(Suppl 3):342.
24. Wang L, Liu Y, Han R, Beier UH, Bhatti TR, Akimova T, et al. FOXP3+ Regulatory T Cell Development and Function Require Histone/Protein Deacetylase 3. J Clin Invest (2015) 125:1111–23. doi: 10.1172/JCI77088
25. Xiao H, Jiao J, Wang L, O'Brien S, Newick K, Wang LC, et al. HDAC5 Controls the Functions of Foxp3(+) T-Regulatory and CD8(+) T Cells. Int J Cancer (2016) 138:2477–86. doi: 10.1002/ijc.29979
26. Tao R, Hancock WW. Resistance of Foxp3+ Regulatory T Cells to Nur77-Induced Apoptosis Promotes Allograft Survival. PLos One (2008) 3:e2321. doi: 10.1371/journal.pone.0002321
27. Wang L, Beier UH, Akimova T, Dahiya S, Han R, Samanta A, et al. Histone/protein Deacetylase Inhibitor Therapy for Enhancement of Foxp3+ T-Regulatory Cell Function Posttransplantation. Am J Transplant (2018) 18:1596–603. doi: 10.1111/ajt.14749
28. Tao R, de Zoeten EF, Ozkaynak E, Chen C, Wang L, Porrett PM, et al. Deacetylase Inhibition Promotes the Generation and Function of Regulatory T Cells. Nat Med (2007) 13:1299–307. doi: 10.1038/nm1652
29. Woods DM, Woan KV, Cheng F, Sodre AL, Wang D, Wu Y, et al. T Cells Lacking HDAC11 Have Increased Effector Functions and Mediate Enhanced Alloreactivity in a Murine Model. Blood (2017) 130:146–55. doi: 10.1182/blood-2016-08-731505
30. Chadha S, Wang L, Hancock WW, Beier UH. Sirtuin-1 in Immunotherapy: A Janus-Headed Target. J Leukoc Biol (2019) 106:337–43. doi: 10.1002/JLB.2RU1118-422R
31. Beier UH, Angelin A, Akimova T, Wang L, Liu Y, Xiao H, et al. Essential Role of Mitochondrial Energy Metabolism in Foxp3(+) T-Regulatory Cell Function and Allograft Survival. FASEB J (2015) 29:2315–26. doi: 10.1096/fj.14-268409
32. Porter NJ, Christianson DW. Structure, Mechanism, and Inhibition of the Zinc-Dependent Histone Deacetylases. Curr Opin Struct Biol (2019) 59:9–18. doi: 10.1016/j.sbi.2019.01.004
33. Sauve AA. Sirtuin Chemical Mechanisms. Biochim Biophys Acta (2010) 1804:1591–603. doi: 10.1016/j.bbapap.2010.01.021
34. Yang Y, Li G. Post-Translational Modifications of PRC2: Signals Directing its Activity. Epigenet Chromatin (2020) 13:47. doi: 10.1186/s13072-020-00369-1
35. Margueron R, Reinberg D. The Polycomb Complex PRC2 and Its Mark in Life. Nature (2011) 469:343–9. doi: 10.1038/nature09784
36. Cavassani KA, Carson W, Moreira AP, Wen H, Schaller MA, Ishii M, et al. The Post Sepsis-Induced Expansion and Enhanced Function of Regulatory T Cells Create an Environment to Potentiate Tumor Growth. Blood (2010) 115:4403–11. doi: 10.1182/blood-2009-09-241083
37. Song Y, Dagil L, Fairall L, Robertson N, Wu M, Ragan TJ, et al. Mechanism of Crosstalk Between the LSD1 Demethylase and HDAC1 Deacetylase in the CoREST Complex. Cell Rep (2020) 30:2699–711.e2698. doi: 10.1016/j.celrep.2020.01.091
38. Yang M, Gocke CB, Luo X, Borek D, Tomchick DR, Machius M, et al. Structural Basis for CoREST-Dependent Demethylation of Nucleosomes by the Human LSD1 Histone Demethylase. Mol Cell (2006) 23:377–87. doi: 10.1016/j.molcel.2006.07.012
39. You A, Tong JK, Grozinger CM, Schreiber SL. CoREST is an Integral Component of the CoREST- Human Histone Deacetylase Complex. Proc Natl Acad Sci USA (2001) 98:1454–8. doi: 10.1073/pnas.98.4.1454
40. Millard CJ, Watson PJ, Fairall L, Schwabe JWR. Targeting Class I Histone Deacetylases in a “Complex" Environment. Trends Pharmacol Sci (2017) 38:363–77. doi: 10.1016/j.tips.2016.12.006
41. Laugesen A, Helin K. Chromatin Repressive Complexes in Stem Cells, Development, and Cancer. Cell Stem Cell (2014) 14:735–51. doi: 10.1016/j.stem.2014.05.006
42. Foster CT, Dovey OM, Lezina L, Luo JL, Gant TW, Barlev N, et al. Lysine-Specific Demethylase 1 Regulates the Embryonic Transcriptome and CoREST Stability. Mol Cell Biol (2010) 30:4851–63. doi: 10.1128/MCB.00521-10
43. Xiong Y, Wang L, Di Giorgio E, Akimova T, Beier UH, Han R, et al. Inhibiting the Coregulator CoREST Impairs Foxp3+ Treg Function and Promotes Antitumor Immunity. J Clin Invest (2020) 130:1830–42. doi: 10.1172/JCI131375
44. Kalin JH, Wu M, Gomez AV, Song Y, Das J, Hayward D, et al. Targeting the CoREST Complex With Dual Histone Deacetylase and Demethylase Inhibitors. Nat Commun (2018) 9:53. doi: 10.1038/s41467-017-02242-4
45. Wagner FF, Zhang YL, Fass DM, Joseph N, Gale JP, Weiwer M, et al. Kinetically Selective Inhibitors of Histone Deacetylase 2 (HDAC2) as Cognition Enhancers. Chem Sci (2015) 6:804–15. doi: 10.1039/C4SC02130D
46. Zhou J, Li M, Chen N, Wang S, Luo HB, Zhang Y, et al. Computational Design of a Time-Dependent Histone Deacetylase 2 Selective Inhibitor. ACS Chem Biol (2015) 10:687–92. doi: 10.1021/cb500767c
47. Rivera C, Lee HG, Lappala A, Wang D, Noches V, Olivares-Costa M, et al. Unveiling RCOR1 as a Rheostat at Transcriptionally Permissive Chromatin. Nat Commun (2022) 13:1550. doi: 10.1038/s41467-022-29261-0
48. Zhang Y, Ng HH, Erdjument-Bromage H, Tempst P, Bird A, Reinberg D. Analysis of the NuRD Subunits Reveals a Histone Deacetylase Core Complex and a Connection With DNA Methylation. Genes Dev (1999) 13:1924–35. doi: 10.1101/gad.13.15.1924
49. Schmidberger JW, Sharifi Tabar M, Torrado M, Silva AP, Landsberg MJ, Brillault L, et al. The MTA1 Subunit of the Nucleosome Remodeling and Deacetylase Complex can Recruit Two Copies of RBBP4/7. Protein Sci (2016) 25:1472–82. doi: 10.1002/pro.2943
50. Leighton G, Williams DC Jr. The Methyl-CpG-Binding Domain 2 and 3 Proteins and Formation of the Nucleosome Remodeling and Deacetylase Complex. J Mol Biol (2020) 432:1624–39. doi: 10.1016/j.jmb.2019.10.007
51. Wang Y, Zhang H, Chen Y, Sun Y, Yang F, Yu W, et al. LSD1 is a Subunit of the NuRD Complex and Targets the Metastasis Programs in Breast Cancer. Cell (2009) 138:660–72. doi: 10.1016/j.cell.2009.05.050
52. Reynolds N, Latos P, Hynes-Allen A, Loos R, Leaford D, O'Shaughnessy A, et al. NuRD Suppresses Pluripotency Gene Expression to Promote Transcriptional Heterogeneity and Lineage Commitment. Cell Stem Cell (2012) 10:583–94. doi: 10.1016/j.stem.2012.02.020
53. Lai AY, Wade PA. Cancer Biology and NuRD: A Multifaceted Chromatin Remodelling Complex. Nat Rev Cancer (2011) 11:588–96. doi: 10.1038/nrc3091
54. Shen E, Wang Q, Rabe H, Liu W, Cantor H, Leavenworth JW. Chromatin Remodeling by the NuRD Complex Regulates Development of Follicular Helper and Regulatory T Cells. Proc Natl Acad Sci USA (2018) 115:6780–5. doi: 10.1073/pnas.1805239115
55. Wang L, Liu Y, Han R, Beier UH, Thomas RM, Wells AD, et al. Mbd2 Promotes Foxp3 Demethylation and T-Regulatory-Cell Function. Mol Cell Biol (2013) 33:4106–15. doi: 10.1128/MCB.00144-13
56. Millard CJ, Varma N, Saleh A, Morris K, Watson PJ, Bottrill AR, et al. The Structure of the Core NuRD Repression Complex Provides Insights Into its Interaction With Chromatin. Elife (2016) 5:e13941. doi: 10.7554/eLife.13941
57. Denslow SA, Wade PA. The Human Mi-2/NuRD Complex and Gene Regulation. Oncogene (2007) 26:5433–8. doi: 10.1038/sj.onc.1210611
58. Fleischer TC, Yun UJ, Ayer DE. Identification and Characterization of Three New Components of the Msin3a Corepressor Complex. Mol Cell Biol (2003) 23:3456–67. doi: 10.1128/MCB.23.10.3456-3467.2003
59. Smith KT, Sardiu ME, Martin-Brown SA, Seidel C, Mushegian A, Egidy R, et al. Human Family With Sequence Similarity 60 Member A (FAM60A) Protein: A New Subunit of the Sin3 Deacetylase Complex. Mol Cell Proteomics (2012) 11:1815–28. doi: 10.1074/mcp.M112.020255
60. Banks CAS, Zhang Y, Miah S, Hao Y, Adams MK, Wen Z, et al. Integrative Modeling of a Sin3/HDAC Complex Sub-Structure. Cell Rep (2020) 31:107516. doi: 10.1016/j.celrep.2020.03.080
61. Grzenda A, Lomberk G, Zhang JS, Urrutia R. Sin3: Master Scaffold and Transcriptional Corepressor. Biochim Biophys Acta (2009) 1789:443–50. doi: 10.1016/j.bbagrm.2009.05.007
62. Clark MD, Marcum R, Graveline R, Chan CW, Xie T, Chen Z, et al. Structural Insights Into the Assembly of the Histone Deacetylase-Associated Sin3L/Rpd3L Corepressor Complex. Proc Natl Acad Sci USA (2015) 112:E3669–3678. doi: 10.1073/pnas.1504021112
63. Lewis MJ, Liu J, Libby EF, Lee M, Crawford NP, Hurst DR. SIN3A and SIN3B Differentially Regulate Breast Cancer Metastasis. Oncotarget (2016) 7:78713–25. doi: 10.18632/oncotarget.12805
64. Hassig CA, Fleischer TC, Billin AN, Schreiber SL, Ayer DE. Histone Deacetylase Activity is Required for Full Transcriptional Repression by Msin3a. Cell (1997) 89:341–7. doi: 10.1016/S0092-8674(00)80214-7
65. Laherty CD, Yang WM, Sun JM, Davie JR, Seto E, Eisenman RN. Histone Deacetylases Associated With the Msin3 Corepressor Mediate Mad Transcriptional Repression. Cell (1997) 89:349–56. doi: 10.1016/s0092-8674(00)80215-9
66. Adams GE, Chandru A, Cowley SM. Co-Repressor, Co-Activator and General Transcription Factor: The Many Faces of the Sin3 Histone Deacetylase (HDAC) Complex. Biochem J (2018) 475:3921–32. doi: 10.1042/BCJ20170314
67. Silverstein RA, Ekwall K. Sin3: A Flexible Regulator of Global Gene Expression and Genome Stability. Curr Genet (2005) 47:1–17. doi: 10.1007/s00294-004-0541-5
68. Williams K, Christensen J, Pedersen MT, Johansen JV, Cloos PA, Rappsilber J, et al. TET1 and Hydroxymethylcytosine in Transcription and DNA Methylation Fidelity. Nature (2011) 473:343–8. doi: 10.1038/nature10066
69. Vidal M, Strich R, Esposito RE, Gaber RF. RPD1 (SIN3/UME4) is Required for Maximal Activation and Repression of Diverse Yeast Genes. Mol Cell Biol (1991) 11:6306–16. doi: 10.1128/mcb.11.12.6306-6316.1991
70. Cowley SM, Iritani BM, Mendrysa SM, Xu T, Cheng PF, Yada J, et al. The Msin3a Chromatin-Modifying Complex is Essential for Embryogenesis and T-Cell Development. Mol Cell Biol (2005) 25:6990–7004. doi: 10.1128/MCB.25.16.6990-7004.2005
71. Tiana M, Acosta-Iborra B, Puente-Santamaria L, Hernansanz-Agustin P, Worsley-Hunt R, Masson N, et al. The SIN3A Histone Deacetylase Complex is Required for a Complete Transcriptional Response to Hypoxia. Nucleic Acids Res (2018) 46:120–33. doi: 10.1093/nar/gkx951
72. Xiong Y, Svingen PA, Sarmento OO, Smyrk TC, Dave M, Khanna S, et al. Differential Coupling of KLF10 to Sin3-HDAC and PCAF Regulates the Inducibility of the FOXP3 Gene. Am J Physiol Regul Integr Comp Physiol (2014) 307:R608–620. doi: 10.1152/ajpregu.00085.2014
73. Christensen LM, Akimova T, Han R, Wang L, Samanta A, Hancock WW. Unexpected Regulation of Foxp3+ Treg Production, Stability and Function by the Nuclear Co-Repressor, Sin3A. Am J Transplant (2018) 20:302.
74. Christensen LM, Akimova T, Han R, Wang L, Samanta A, Hancock WW. Regulation of Foxp3+ Treg Production, Stability and Function by the Nuclear Co-Repressor, Sin3A. Am J Pathol (2020) 190:S19–20.
75. Farias EF, Petrie K, Leibovitch B, Murtagh J, Chornet MB, Schenk T, et al. Interference With Sin3 Function Induces Epigenetic Reprogramming and Differentiation in Breast Cancer Cells. Proc Natl Acad Sci U S A (2010) 107:11811–6. doi: 10.1073/pnas.1006737107
76. Kwon YJ, Petrie K, Leibovitch BA, Zeng L, Mezei M, Howell L, et al. Selective Inhibition of SIN3 Corepressor With Avermectins as a Novel Therapeutic Strategy in Triple-Negative Breast Cancer. Mol Cancer Ther (2015) 14:1824–36. doi: 10.1158/1535-7163.MCT-14-0980-T
77. Bantscheff M, Hopf C, Savitski MM, Dittmann A, Grandi P, Michon AM, et al. Chemoproteomics Profiling of HDAC Inhibitors Reveals Selective Targeting of HDAC Complexes. Nat Biotechnol (2011) 29:255–65. doi: 10.1038/nbt.1759
78. Hein MY, Hubner NC, Poser I, Cox J, Nagaraj N, Toyoda Y, et al. A Human Interactome in Three Quantitative Dimensions Organized by Stoichiometries and Abundances. Cell (2015) 163:712–23. doi: 10.1016/j.cell.2015.09.053
79. Huttlin EL, Ting L, Bruckner RJ, Gebreab F, Gygi MP, Szpyt J, et al. The BioPlex Network: A Systematic Exploration of the Human Interactome. Cell (2015) 162:425–40. doi: 10.1016/j.cell.2015.06.043
80. Itoh T, Fairall L, Muskett FW, Milano CP, Watson PJ, Arnaudo N, et al. Structural and Functional Characterization of a Cell Cycle Associated HDAC1/2 Complex Reveals the Structural Basis for Complex Assembly and Nucleosome Targeting. Nucleic Acids Res (2015) 43:2033–44. doi: 10.1093/nar/gkv068
81. Mondal B, Jin H, Kallappagoudar S, Sedkov Y, Martinez T, Sentmanat MF, et al. The Histone Deacetylase Complex MiDAC Regulates a Neurodevelopmental Gene Expression Program to Control Neurite Outgrowth. Elife (2020) 9:e57519. doi: 10.7554/eLife.57519
82. Turnbull RE, Fairall L, Saleh A, Kelsall E, Morris KL, Ragan TJ, et al. The MiDAC Histone Deacetylase Complex Is Essential for Embryonic Development and has a Unique Multivalent Structure. Nat Commun (2020) 11:3252. doi: 10.1038/s41467-020-17078-8
83. Guenther MG, Barak O, Lazar MA. The SMRT and N-CoR Corepressors Are Activating Cofactors for Histone Deacetylase 3. Mol Cell Biol (2001) 21:6091–101. doi: 10.1128/mcb.21.18.6091-6101.2001
84. Millard CJ, Watson PJ, Celardo I, Gordiyenko Y, Cowley SM, Robinson CV, et al. Class I HDACs Share a Common Mechanism of Regulation by Inositol Phosphates. Mol Cell (2013) 51:57–67. doi: 10.1016/j.molcel.2013.05.020
85. Hudson GM, Watson PJ, Fairall L, Jamieson AG, Schwabe JWR. Insights Into the Recruitment of Class IIa Histone Deacetylases (HDACs) to the SMRT/NCoR Transcriptional Repression Complex. J Biol Chem (2015) 290:18237–44. doi: 10.1074/jbc.M115.661058
86. Fischle W, Dequiedt F, Fillion M, Hendzel MJ, Voelter W, Verdin E. Human HDAC7 Histone Deacetylase Activity is Associated With HDAC3 In Vivo. J Biol Chem (2001) 276:35826–35. doi: 10.1074/jbc.M104935200
87. Fischle W, Dequiedt F, Hendzel MJ, Guenther MG, Lazar MA, Voelter W, et al. Enzymatic Activity Associated With Class II HDACs Is Dependent on a Multiprotein Complex Containing HDAC3 and SMRT/N-CoR. Mol Cell (2002) 9:45–57. doi: 10.1016/S1097-2765(01)00429-4
88. Lahm A, Paolini C, Pallaoro M, Nardi MC, Jones P, Neddermann P, et al. Unraveling the Hidden Catalytic Activity of Vertebrate Class IIa Histone Deacetylases. Proc Natl Acad Sci USA (2007) 104:17335–40. doi: 10.1073/pnas.0706487104
89. Bradner JE, Mak R, Tanguturi SK, Mazitschek R, Haggarty SJ, Ross K, et al. Chemical Genetic Strategy Identifies Histone Deacetylase 1 (HDAC1) and HDAC2 as Therapeutic Targets in Sickle Cell Disease. Proc Natl Acad Sci U S A (2010) 107:12617–22. doi: 10.1073/pnas.1006774107
90. Seto E, Yoshida M. Erasers of Histone Acetylation: The Histone Deacetylase Enzymes. Cold Spring Harb Perspect Biol (2014) 6:a018713. doi: 10.1101/cshperspect.a018713
91. Skourti-Stathaki K, Torlai Triglia E, Warburton M, Voigt P, Bird A, Pombo A. R-Loops Enhance Polycomb Repression at a Subset of Developmental Regulator Genes. Mol Cell (2019) 73:930–45.e934. doi: 10.1016/j.molcel.2018.12.016
92. Comet I, Riising EM, Leblanc B, Helin K. Maintaining Cell Identity: PRC2-Mediated Regulation of Transcription and Cancer. Nat Rev Cancer (2016) 16:803–10. doi: 10.1038/nrc.2016.83
93. Arvey A, van der Veeken J, Samstein RM, Feng Y, Stamatoyannopoulos JA, Rudensky AY. Inflammation-Induced Repression of Chromatin Bound by the Transcription Factor Foxp3 in Regulatory T Cells. Nat Immunol (2014) 15:580–7. doi: 10.1038/ni.2868
94. Gonzalez MM, Bamidele AO, Svingen PA, Sagstetter MR, Smyrk TC, Gaballa JM, et al. BMI1 Maintains the Treg Epigenomic Landscape to Prevent Inflammatory Bowel Disease. J Clin Invest (2021) 131:e140755. doi: 10.1172/JCI140755
95. DuPage M, Chopra G, Quiros J, Rosenthal WL, Morar MM, Holohan D, et al. The Chromatin-Modifying Enzyme Ezh2 Is Critical for the Maintenance of Regulatory T Cell Identity After Activation. Immunity (2015) 42:227–38. doi: 10.1016/j.immuni.2015.01.007
96. Bamidele AO, Svingen PA, Sagstetter MR, Sarmento OF, Gonzalez M, Braga Neto MB, et al. Disruption of FOXP3-EZH2 Interaction Represents a Pathobiological Mechanism in Intestinal Inflammation. Cell Mol Gastroenterol Hepatol (2019) 7:55–71. doi: 10.1016/j.jcmgh.2018.08.009
97. Xu B, Konze KD, Jin J, Wang GG. Targeting EZH2 and PRC2 Dependence as Novel Anticancer Therapy. Exp Hematol (2015) 43:698–712. doi: 10.1016/j.exphem.2015.05.001
98. Jenke R, Ressing N, Hansen FK, Aigner A, Buch T. Anticancer Therapy With HDAC Inhibitors: Mechanism-Based Combination Strategies and Future Perspectives. Cancers (Basel) (2021) 13:634. doi: 10.3390/cancers13040634
99. Wang P, Wang Z, Liu J. Role of HDACs in Normal and Malignant Hematopoiesis. Mol Cancer (2020) 19:5. doi: 10.1186/s12943-019-1127-7
100. Weichert W, Roske A, Gekeler V, Beckers T, Stephan C, Jung K, et al. Histone Deacetylases 1, 2 and 3 Are Highly Expressed in Prostate Cancer and HDAC2 Expression is Associated With Shorter PSA Relapse Time After Radical Prostatectomy. Br J Cancer (2008) 98:604–10. doi: 10.1038/sj.bjc.6604199
101. Li Y, Seto E. HDACs and HDAC Inhibitors in Cancer Development and Therapy. Cold Spring Harb Perspect Med (2016) 6:a026831. doi: 10.1101/cshperspect.a026831
102. Wang L, de Zoeten EF, Greene MI, Hancock WW. Immunomodulatory Effects of Deacetylase Inhibitors: Therapeutic Targeting of FOXP3+ Regulatory T Cells. Nat Rev Drug Discovery (2009) 8:969–81. doi: 10.1038/nrd3031
103. Varambally S, Dhanasekaran SM, Zhou M, Barrette TR, Kumar-Sinha C, Sanda MG, et al. The Polycomb Group Protein EZH2 Is Involved in Progression of Prostate Cancer. Nature (2002) 419:624–9. doi: 10.1038/nature01075
104. Shimizu T, Kubovcakova L, Nienhold R, Zmajkovic J, Meyer SC, Hao-Shen H, et al. Loss of Ezh2 Synergizes With JAK2-V617F in Initiating Myeloproliferative Neoplasms and Promoting Myelofibrosis. J Exp Med (2016) 213:1479–96. doi: 10.1084/jem.20151136
105. Peng D, Kryczek I, Nagarsheth N, Zhao L, Wei S, Wang W, et al. Epigenetic Silencing of TH1-Type Chemokines Shapes Tumour Immunity and Immunotherapy. Nature (2015) 527:249–53. doi: 10.1038/nature15520
106. Dangaj D, Bruand M, Grimm AJ, Ronet C, Barras D, Duttagupta PA, et al. Cooperation Between Constitutive and Inducible Chemokines Enables T Cell Engraftment and Immune Attack in Solid Tumors. Cancer Cell (2019) 35:885–900.e810. doi: 10.1016/j.ccell.2019.05.004
107. Wang D, Quiros J, Mahuron K, Pai CC, Ranzani V, Young A, et al. Targeting EZH2 Reprograms Intratumoral Regulatory T Cells to Enhance Cancer Immunity. Cell Rep (2018) 23:3262–74. doi: 10.1016/j.celrep.2018.05.050
108. Wan L, Xu K, Wei Y, Zhang J, Han T, Fry C, et al. Phosphorylation of EZH2 by AMPK Suppresses PRC2 Methyltransferase Activity and Oncogenic Function. Mol Cell (2018) 69:279–91.e275. doi: 10.1016/j.molcel.2017.12.024
109. Qi W, Zhao K, Gu J, Huang Y, Wang Y, Zhang H, et al. An Allosteric PRC2 Inhibitor Targeting the H3K27me3 Binding Pocket of EED. Nat Chem Biol (2017) 13:381–8. doi: 10.1038/nchembio.2304
110. Duan R, Du W, Guo W. EZH2: A Novel Target for Cancer Treatment. J Hematol Oncol (2020) 13:104. doi: 10.1186/s13045-020-00937-8
111. Kailayangiri S, Altvater B, Lesch S, Balbach S, Gottlich C, Kuhnemundt J, et al. EZH2 Inhibition in Ewing Sarcoma Upregulates GD2 Expression for Targeting With Gene-Modified T Cells. Mol Ther (2019) 27:933–46. doi: 10.1016/j.ymthe.2019.02.014
Keywords: histone/protein deacetylases, inhibitors, tumor immunity, T-regulatory cells, SIN3A
Citation: Christensen LM and Hancock WW (2022) Nuclear Coregulatory Complexes in Tregs as Targets to Promote Anticancer Immune Responses. Front. Immunol. 13:909816. doi: 10.3389/fimmu.2022.909816
Received: 31 March 2022; Accepted: 18 May 2022;
Published: 20 June 2022.
Edited by:
Joseph Barbi, University at Buffalo, United StatesReviewed by:
Ashutosh Chaudhry, Memorial Sloan Kettering Cancer Center, United StatesJianxun J. Song, Texas A&M Health Science Center, United States
Copyright © 2022 Christensen and Hancock. This is an open-access article distributed under the terms of the Creative Commons Attribution License (CC BY). The use, distribution or reproduction in other forums is permitted, provided the original author(s) and the copyright owner(s) are credited and that the original publication in this journal is cited, in accordance with accepted academic practice. No use, distribution or reproduction is permitted which does not comply with these terms.
*Correspondence: Wayne W. Hancock, d2hhbmNvY2tAbWFpbC5tZWQudXBlbm4uZWR1