- 1State Key Laboratory of Silkworm Genome Biology, Southwest University, Chongqing, China
- 2School of Pharmaceutical Sciences, Wenzhou Medical University, Wenzhou, China
- 3Jiangsu Key Laboratory for Bioresources of Saline Soils, Jiangsu Synthetic Innovation Center for Coastal Bio-agriculture, Jiangsu Provincial Key Laboratory of Coastal Wetland Bioresources and Environmental Protection, School of Wetlands, Yancheng Teachers University, Yancheng, China
- 4Department of Zoology, Shaheed Benazir Bhutto Woman University, Peshawar, Pakistan
- 5Key Laboratory of Insect Developmental and Evolutionary Biology, Chinese Academy of Sciences (CAS) Center for Excellence in Molecular Plant Sciences, Shanghai Institute of Plant Physiology and Ecology, Chinese Academy of Sciences, Shanghai, China
Integrins are a large group of cell-surface proteins that are classified as transmembrane proteins. Integrins are classified into different types based on sequence variations, leading to structural and functional diversity. They are broadly distributed in animals and have a wide range of biological functions such as cell-to-cell communication, intracellular cytoskeleton organization, cellular signaling, immune responses, etc. Integrins are among the most abundant cell surface proteins in insects, exhibiting their indispensability in insect physiology. Because of their critical biological involvement in physiological processes, they appear to be a novel target for designing effective pest control strategies. In the current literature review, we first discuss the discovery and expression responses of integrins against various types of pathogens. Secondly, we examine the specific biological roles of integrins in controlling microbial pathogens, such as phagocytosis, encapsulation, nodulation, immune signaling, and so on. Finally, we describe the possible uses of integrins to control agricultural insect pests.
Introduction
Integrins are a family of cell surface adhesion receptors that were first discovered in 1986 and have been found in all metazoans. Since their discovery, a tremendous amount of work has been done that substantially improved our understanding. They are non-covalently coupled heterodimers made up of two subunits (α and β), each of which is a single-pass type I transmembrane protein (1–3). The extracellular domains of integrins interact with proteins of the extracellular matrix in a unique way, making them highly versatile. In some cases, these receptor proteins bind to adjacent cells, promoting cell adhesion, which is important for providing mechanical support to a membrane, tissue maintenance and repair, embryonic development, hemostasis, and host defense. These physiological processes rely on short cytoplasmic tails of integrin proteins interacting with the intracellular cytoskeleton, which facilitates bi-directional force transmission across the cell membrane (4, 5). Integrins also convey chemical signals into the cell (outside-in signaling), providing information on the cell’s location, adhesive state, local environment, and surrounding matrix (2, 6). In addition to controlling cellular responses such as survival, differentiation, migration, and motility, it also serves as a context for responding to other signals such as those transmitted by growth-factor or G protein-coupled receptors. Integrins have the ability to modulate their affinity for extracellular ligands in addition to signaling from outside-in. In order to do this, they undergo conformational changes in their extracellular domains in response to signals that impinge upon the integrin cytoplasmic tails. This process is named as inside-out signaling or activation (7).
Integrin signals thus regulate a wide range of physiological processes in living organisms. Despite the plethora of information on the immunological responses of insects against different types of pathogens (8–10), no systematic literature review on the biological involvement of integrins in insect immunity has been published. In this review article, we discuss an overview of the biological role of integrins in insects’ immunity. In particular, we focus on the brief discovery of integrins in insects and describe the molecular mechanism by which integrins control various immune responses such as nodule formation, encapsulation, phagocytosis, melanization, immune signaling pathways, and others. In addition, we examine the interaction of integrins with other proteins or molecules for the accomplishment of immune functions.
Discovery and Overall Structure of Integrins in Insects
Integrins are a large group of cell surface-adhesion receptors that are found in all metazoans. Integrins were discovered just over three decades ago and have since been extensively investigated in both invertebrates and vertebrates because they are indispensable for animal survival (11, 12). Integrins are transmembrane cell-surface glycoproteins of type I that are heterodimers made up of non-covalently linked α and β subunits (13). These subunits are constructed from different domains, with each subunit containing a large ectodomain that is important for ligand binding, a single transmembrane domain, and a cytoplasmic domain (cytoplasmic tail) that, in most cases, ranges in size from 20 to 70 amino acid residues (2, 14, 15).
Integrins have been reported in a variety of insect species, including Bombyx mori, Manduca sexta, and Drosophila melanogaster, etc. The number of integrins found in different species may vary. A recent study reported a total of eleven members, including α1-α3, αPS1- αPS3, and five β units (β1- β5) B. mori (16). While D. melanogaster possess Five α subunits (αPS1, αPS2, αPS3, αPS4, αPS5), and two β subunits (βPS and βν [beta-nu]) (17–20). Because Drosophila has tractable genetics, relatively much knowledge regarding how these integrin subunits are generated and how they play a biological role is available. The αPS1 subunit of Drosophila, which is encoded by the numerous edematous wings locus, is identical to the vertebrate subunits α3, α6, and α7, whereas αPS2, which is encoded by the inflated locus, is identical to α5, α8, αV, and αIIb (21, 22). In contrast, the remaining subunits (αPS3-αPS5) are not closely related to all of the vertebrate α subunits (18, 22). The αPS3 locus produces two transcripts and mutant alleles that induce phenotypic differences ranging from embryonic lethality with dorsal holes to adult viability (18). The putative ATG of αPS4 is only 259 bp downstream of the polyadenylation site of αPS3 transcripts, implying that it may depend on regulatory elements within the αPS3 gene and hence be part of the same complex gene. This could explain why apparent molecular null mutations in the αPS3 gen only cause a mild scab phenotype. It is unclear whether a strong scab affects both αPS3 and αPS4 subunits. In Drosophila, alternative splicing of the myospheroid transcripts encoding the βPS integrin subunit, a β integrin, leads to two distinct isoforms, each determined by the presence of one of two potential fourth exons (23, 24). The alternate exons, βps4A or βps4B, encode 29 amino acid residues in a region of the βPS subunit that has been involved in determining the ligand preferences of the human integrins. In the genome of C. capitata and other insect species, regions homologous to Drosophila βps4A and βps4B have also been reported (22). Furthermore, The expression of the second β subunit, βv, appears to be confined to gut endodermal cells (19). What α subunits form heterodimers with it is unknown. There are no known mutations in this gene, and embryo with a genetic defect in this region develops a morphologically normal gut (25).
Generally, animal genomes contain fewer β subunits compared to α subunits (26), suggesting that multiple α subunits can bind a single β subunit. For example, when one β subunit of Drosophila binds with three α subunits, three different functional integrin molecules are formed (20). The cytoplasmic domain of the β subunit is responsible for interacting with intracellular molecules that link integrins to the cytoskeleton and signaling molecules that transmit developmental signals (2). Thus, β subunits seem to play a crucial biological role in physiological processes, and their absence may result in functional abnormalities. The absence of β1 integrin subunits, for example, has been demonstrated to disrupt the organization of the mammalian embryo, mainly by inhibiting cell adhesion and spreading, resulting in cell death (27, 28).
Integrins are Components of Immune Cells
Integrins are proteins that have been shown to be essential components of immune cells. Immune cells in vertebrates are termed as leukocytes. They are divided into T cells and B cells, dendritic cells (DCs), neutrophils, and monocytes, which differentiate into tissue macrophages (29). Leukocytes have been shown to be activated in response to immune signals inducing cells to migrate rapidly to infected sites in order to effectively respond to damage or infection (29, 30). Integrins are essential components of these cells that assist them in carrying out immune reactions (31). In contrast, in insects, Integrins show tissular specificity and appear to be an essential component of hemocytes. For example, In P. includens and M. sexta, α1 and α3 are mainly produced in hemocytes, α2 is widely transcribed in fat bodies but lowly expressed in hemocytes, and β1 is specifically expressed only in hemocytes (32–34). β1, on the other hand, is strongly expressed in fat bodies (35). While β1 is predominantly expressed in hemocytes in O. furnacalis (36). In B. mori α1 is highly expressed in hemocytes, α3 is also greatly expressed in hemocytes, α2 has a modest degree of expression in hemocytes, and β2 is expressed explicitly in hemocytes (37). These pieces of evidence indicate that, like vertebrates, plasma membranes of immunes cells in insects also contain high concentrations of integrins, implying that integrins are essential components of immunes cells in both vertebrates and invertebrates.
The Biological Role of Integrins in Immunological Responses of Insects
Besides the development and growth, which is not the focus of the present review article, integrins have been known to be involved in insects’ immune defense. Since their discovery, integrins have been identified and characterized in a number of insect species. In addition, growing evidence indicates that they play an effective biological in the defense of insects. This section discusses the immunological importance of integrin (Figure 1).
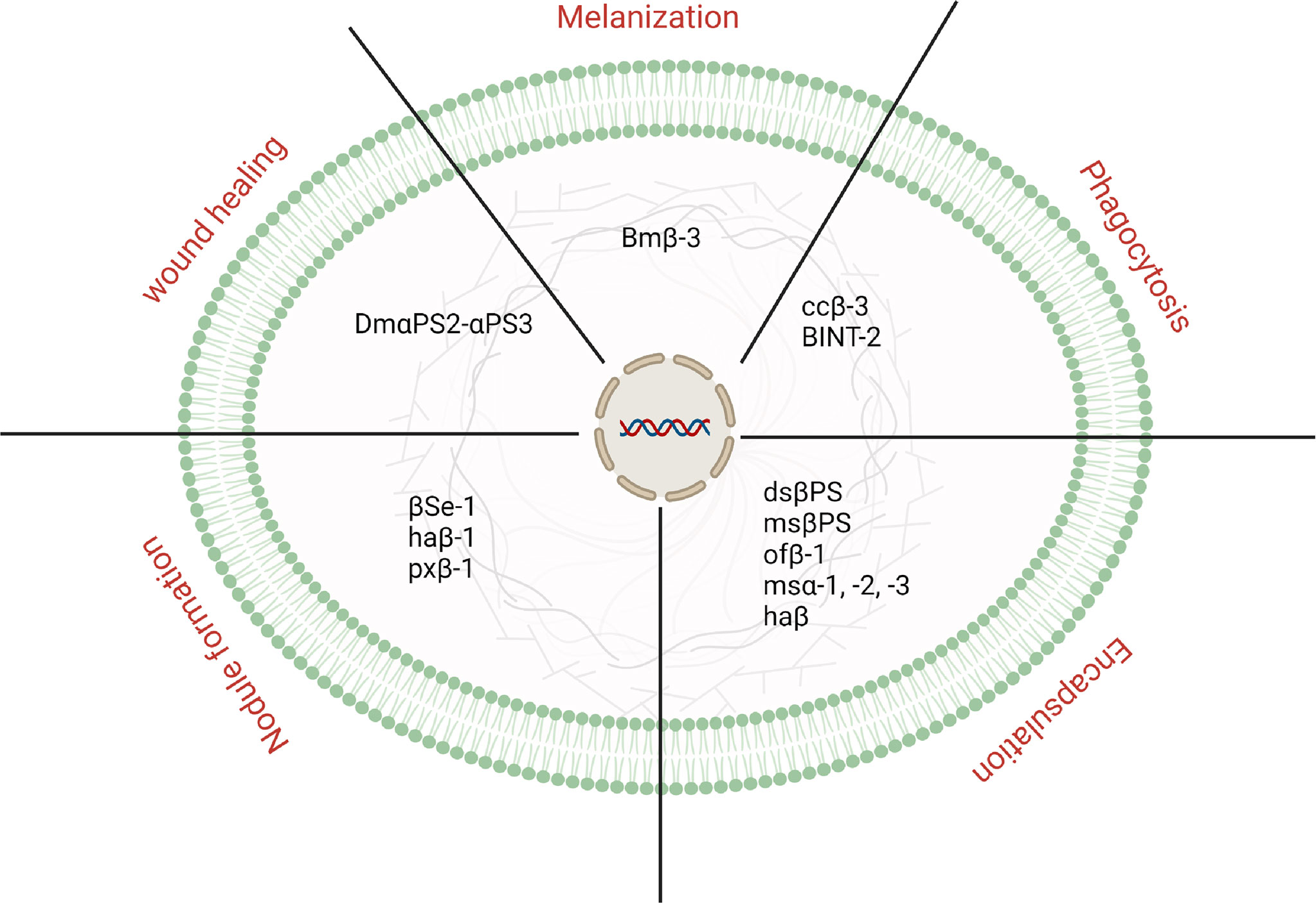
Figure 1 An overview is representing different physiological processes controlled by integrins in insects.
Integrins in Biotic Stress Responses
Growing evidence suggests that integrins production is increased in response to infection. In B. mori, β3 integrin has been shown to be extensively produced in response to bacterial and pathogen-associated molecular patterns (PAMPs), implying that these proteins are likely involved in hemocyte-mediated immunity (37). Another study recently observed comparable expression patterns of integrin proteins in hemocytes, confirming the hemocyte-associated biological roles of integrins (35).
Therefore, it was considered that integrins are only associated with hemocytes or blood cells in animals (29, 37). In contrast, a recent study on B. mori suggested that integrins are found in hemocytes and also expressed in other tissues, including fat bodies (35). The fat body is a multifunctional tissue that involves integrating signals, synthesizing immune system proteins, serving as a center of metabolism, regulating molting and metamorphosis, and synthesizing hormones that regulate body functions. Apart from their traditional roles in hemocytes-mediated immunity, integrin may also play a role in influencing the synthesis of immune proteins and hormones, hence regulating pathogen infection in insects. However, evidence is required to confirm their specific immune roles in the fat bodies (38). Zhang and his co-workers (2017) suggested that β3 integrin increases the expression of IMD, and Toll pathway-related genes in hemocytes, suggesting that likely these proteins may control the production of immune-associated factors. However, the authors failed to elaborate on the molecular mechanism by which integrin influences immune signaling pathways. In addition, Spodoptera exigua remarkably upregulates the expression of βSe1 during Gram-negative bacterial or fungal infection, and it shows a similar expression pattern after lipopolysaccharide or laminarin challenge; however, Gram-positive bacterial infection had no effect on expression levels (39). Another study used a different approach, challenged the Helicoverpa armigera with beads, and analyzed expression patterns of integrin α-PS1 at different time points. This study suggested that α-PS1 is highly induced after the bead challenge in hemocytes (40).
So far, only a few studies are available that directly discuss the responses of integrins against microbial pathogens. In vivo expression analysis indicates that integrins production is increased in the presence of various pathogens, such as Gram-positive bacteria, Gram-negative bacteria, or beads. The upregulation of these proteins is clear evidence that they have crucial biological roles in insect immune responses. Although the studies into the biological involvement of integrins in stress response are relatively restricted, these studies suggest that different integrins play a role in response to diverse stress conditions in insects.
Integrins in Phagocytosis
Phagocytosis is an evolutionarily conserved process in which hemocytes recognize, internalize and eventually eliminate apoptotic cells and invading microbial pathogens. In the hemolymph of insects, granulocytes or plasmatocytes are the only hemocytes responsible for phagocytosis (41–43). Various molecules and receptors residing on the surface of membranes, such as integrins, are involved in the binding and internalization of microbial pathogens.
Integrins are essential surface receptors that mediate signal transduction between the extracellular matrix and the cytoskeleton, influencing morphological changes in hemocytes, which are usually attributed to cytoskeletal rearrangements that occur during phagosome formation (Figure 2) (15, 44). Many members of the integrin family have been shown to have a role in the process of phagocytosis; however, their mechanisms of action may vary depending on the type of molecule. For example, in the Mediterranean fruit fly, Ceratitis copitata, integrin β3 serves as a receptor and stimulates cytoskeletal rearrangement for E. coli phagocytosis, and their binding activates integrin specific signal of pathogen internalization (45, 46). For the engulfment of E. coli, the β integrin subunit in the surface of medfly hemocytes transmits signals to focal adhesion kinase (FAK) and its downstream targets, including Src, Elk-1-like protein, and MAP kinases. The family of MAP kinase is an established intracellular evolutionary conserved phosphorylation cascade that regulates inflammatory responses, apoptosis, and phagocytosis (47, 48). In addition, these investigations suggested that integrins can only bind to gram-negative bacteria and cannot interact with gram-positive bacteria (45, 49).
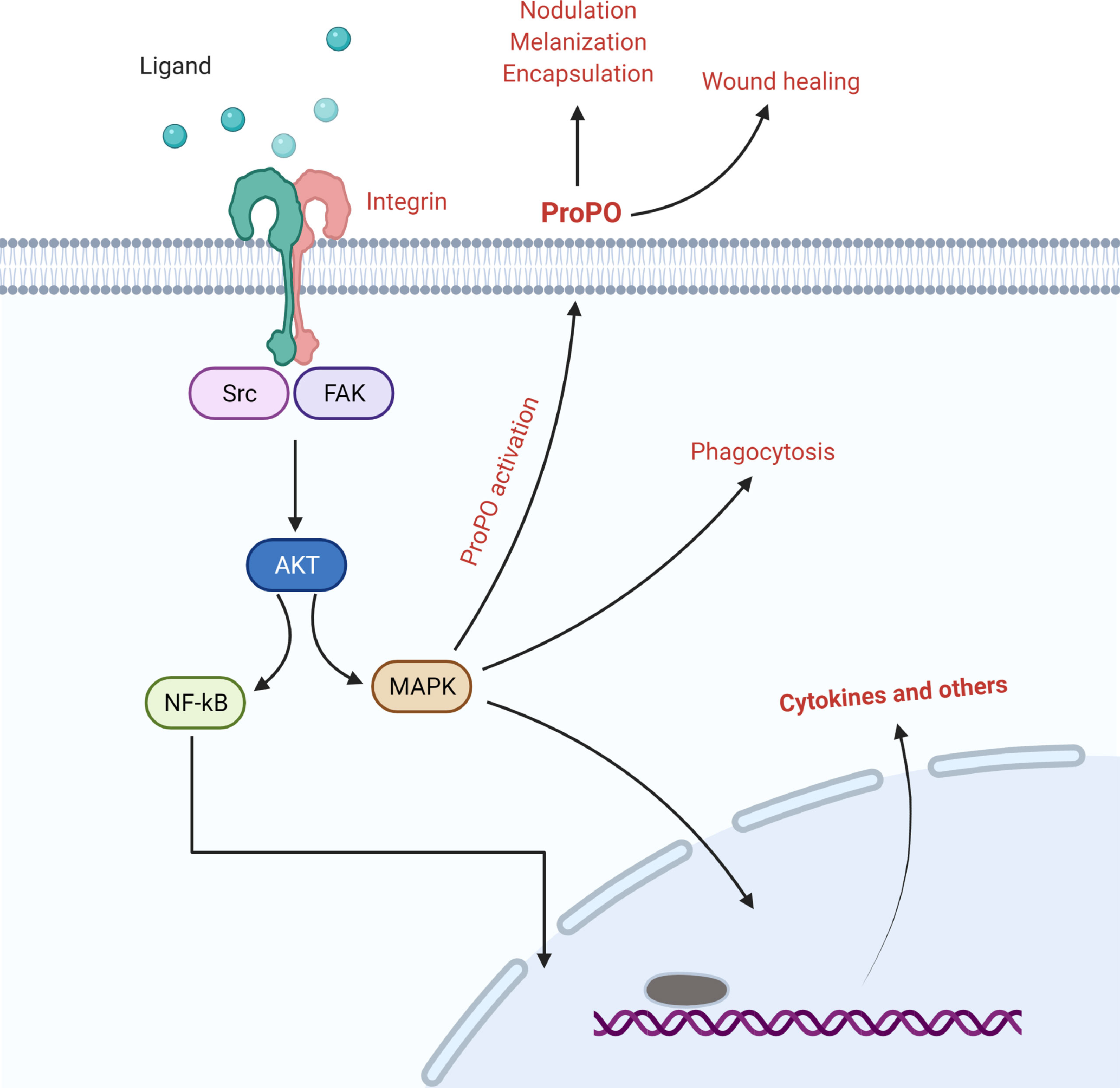
Figure 2 The involvement of integrins in the regulation of nodulation, melanization, encapsulation, and wound healing via focal adhesion kinase and its associated signaling.
Phagocytosis has also been demonstrated in Anopheles gambiae and Ceratitis capitata (medfly), where the β subunit has been found to regulate the bacterial phagocytosis (49, 50). In Anopheles gambiae immune-responsive cell line, RGD ligand recognition receptors play a critical biological role in the phagocytic response, showing the overlap between molecular components participating in adhesion and phagocytosis. BINT2, a newly discovered integrin family member, has been shown to play a vital function in the phagocytosis of E. coli by a hemocyte-like cell line. Furthermore, in vivo suppression of BINT2 in mosquitoes (A. gambiae) results in a remarkable reduction (more than 70%) of E. coli phagocytosis (50, 51). It has been hypothesized that BINT2 protein can either directly bind to bacteria or indirectly recognize bacteria: BINT2 protein binds to an RGD containing lectin, which recognizes E. coli (50). Another study demonstrated an approximately similar phenomenon, in which this study identified globule-EGF-factor 8 (MFG-E8), which is produced by thioglycollate-elicited macrophages that links apoptotic cells to phagocytes. MFG-E8 specifically interacts with apoptotic cells by recognizing aminophospholipids such as phosphatidylserine. MFG-E8, when engaged by phospholipids, binds to cells via its RGD (arginine-glycine-aspartate) motif—it binds particularly strongly to cells expressing αvβ3 integrin. The NIH3T3 cell transformants that expressed a high level of αvβ3 integrin have been found to engulf apoptotic cells when MFG-E8 is added (52). Therefore, further research into the binding mechanism of this newly identified protein (BINT2) is needed.
In contrast, many recent studies argue that integrin β can bind to pathogen-associated molecular patterns (PAMPs) derived from Gram-positive and Gram-negative bacteria, such as LPS and PGN, implying that they are responsive to a wider spectrum of bacterial pathogens (37, 53–55). reported the ability of B. mori β3 integrin to bind with LPS in vivo. The interaction of β3 integrin with PAMPs, suggests that after binding, these proteins induce signals that cause the infectious particles to internalize, which is then responsible for phagocytosis. In comparison to PAMPs, the authors did not detect any interaction between recombinant β3 integrin protein and E. coli or P. aeruginosa bacteria in vitro. The possible reason for this weak interaction between them could be attributed to the weak binding of the recombinant protein to E. coli or P. aeruginosa. In addition, the other possible reason is that the recombinant integrin protein used in this study is likely not folded precisely in vitro (37). However, this phenomenon is of great interest to determine what biochemical changes occur in the structure of integrin β3 when it is expressed in the prokaryotic expression system, as these changes could limit the ability of the recombinant protein to bind to bacterial pathogens in vitro. Furthermore, these contrasting results suggest that integrin protein binding ability may vary in different species or some unknown mechanism controls their binding.
However, a recent study in the same species B. mori suggested that integrin β1 can interact with Gram-positive bacteria, S. aureus in the presence of CaCl2 in addition to Gram-negative bacteria, including E. coli, or P. aeruginosa (35). In another insect species (49), knockdown of βps4A and βps4B in medfly hemocytes reduce hemocytes capability by about 40% to uptake bacteria. In addition, the authors incubated hemocytes with gram-negative (E. coli) or gram-positive (S. aureus) bacteria in the presence of anti β1 and β3 human antibodies; subsequently, they found an almost 20% reduction in the uptake of bacteria by hemocytes.
Integrins have wide-spectrum activity against Gram-negative and Gram-positive bacteria, as evidenced by the examples above. The binding and elimination of bacterial pathogens by different types of integrins may differ. This ability may differ between species, implying that integrins may serve species-specific functions. Future studies on different insect species may help to clarify these inferences.
Integrins in Encapsulation
Encapsulation is the primary response of lepidopteran hemocytes against microbial pathogens, and hemocytes bind to non-self-molecules (e.g., microbial invaders) during this process. Hemocytes create a multilayered cellular sheath after attaching to microbial invaders, which is followed by melanization (42, 56). There are multiple lines of evidence that integrins are involved in insects’ cellular immune response by encapsulation (Figure 2) (32). The ability of lamellocytes to encapsulate has been shown to be disrupted in a βPS integrin loss of function mutant in D. melanogaster (57). Another study found that when integrins are suppressed by specific RNA interference, hemocyte-mediated encapsulation against microbial invaders in M. sexta is impaired (33, 34, 58). Integrin β1 from Ostrinia furnacalis has also been shown to influence the encapsulation process of plasmatocytes (36, 44). Additionally, although encapsulation and melanization are two distinct processes, melanization usually occurs together with encapsulation to remove foreign invaders (59). In Drosophila hemocytes, PS integrin is required for encapsulation reaction. Following encapsulation, Drosophila hemocytes induce the prophenoloxidase gene, which generates the enzymatic proteins essential for melanization of the capsule formed during encapsulation (57).
In Manduca sexta, α1, α2, and α3 integrins have also been reported to facilitate encapsulation, in particular α1 and α2. The α1 hemocyte-specific integrin contains a β subunit that has the capability to interact with the LEL domain of tetraspanin D76, suggesting that tetraspanin (Integral membrane proteins family with four transmembrane domains) on one cell surface interacts with hemocyte-specific integrin on another cell surface. This integrin-tetraspanin association is unique in insects and has not been reported in mammals (33, 60). In contrast, the α2 subunit is related to integrins with RGD-binding motifs and thus binds to substrates. However, all three α-integrin subunits are expressed on hemocytes during the immune response, and exposure of hemocytes in situ to dsRNAs that disrupt the expression of each of these α subunits also abolishes encapsulation (34). Overall, the individual α integrin subunits of M. sexta, like their mammalian immune system counterparts, have crucial, individual biological roles in cell-cell and cell-substrate interactions throughout cellular immune responses. Furthermore, activation of ligand-binding by the hemocyte-specific β1 integrin plays a critical biological role in inducing plasmatocyte adhesion leading to encapsulation. In vitro studies revealed that recombinant protein containing the I-like domain can bind to MS13 and MS34 (mAbs specific for M. sexta hemocytes), blocking their ligand-binding site and subsequently impairing the adhesion of plasmatocytes. In contrast, inhibiting integrin β1 in plasmatocytes resulted in a remarkable reduction in encapsulation (33).
Integrin has been proposed to serve as a C-type lectin receptor (CTL3) during the encapsulation reaction of hemocytes. This physical interaction between pattern recognition receptors and integrins promotes encapsulation reaction in insects, as it modulates the physiological modifications in cells throughout the encapsulation process (61). A recent study using in vitro and in vivo analysis demonstrated that CTL3 promotes encapsulation and melanization reactions in Helicoverpa armigera, while β integrin contributes to the encapsulation reaction. Co-immunoprecipitation analysis of CTL3 interacts with β-integrin, suggesting their strong interaction that simultaneously improves the encapsulation reaction. Further, this study observed that suppression of β integrin could reduce encapsulation reaction in CTL3-coated beads. Interestingly, the authors observed that 20-hydroxyecdysone (20E) administration could enhance CTL3 transcription and inferred that the interaction of pattern recognition receptors and integrin is governed by steroid hormone ecdysone and thereby it regulates the encapsulation process (62).
Integrins in Nodule Formation
Nodulation is a biphasic cellular immune response in insects that begins with hemocytes secreting adhesion molecules, followed by granulocytes entrapping microorganisms in clusters and forming aggregates with other granulocytes. Plasmatocytes eventually wall off these microaggregates, forming a nodule (63, 64). The attachment of hemocytes is mediated through cell surface adhesion molecules during nodulation and encapsulation. Hemocytes of lepidopteran insects contain cell surface integrin proteins that are involved in the adhesion of cells (34, 37, 65). Integrin proteins on plasmatocytes in M. sexta are derived in part via interactions with neuroglia and a tetraspanin on neighboring hemocytes (58).
Several studies have suggested that integrins play a biological role in immune responses by forming nodules in insects (Figure 2) (39, 66, 67). Surakasi et al., found that βSe1 stimulates the formation of nodules in S. exigua, which aids in the removal of pathogens (39). The authors also demonstrated that βSe1 suppression significantly decreases hemocyte-spreading behavior and nodule formation against bacterial challenges (39). Similar evidence has also been shown in P. xylostella and Helicoverpa assulta, where a deficiency of β1 reduced nodule formation following bacterial challenge (66, 67). Contacts with extracellular matrix or hemocyte-hemocyte interactions can cause hemocyte behavior to be aggregated. M. sexta secretes neuroglian, a cell adhesion molecule, on the cell surfaces of granular cells and a subset of large plasmatocytes (58). Hemocytes that are positive for neuroglian act as aggregation foci against foreign surfaces (68). Because some mammalian integrins can bind to at least three different members of the immunoglobulin superfamily: ICAM-1, ICXAM-2, and ICAM-3, the Immunoglobulin domain of neuroglian has been suggested to be a ligand of integrin system (69). Thus, it seems that β1 subunit is required to carry out the processes of hemocyte cellular immune responses. In insects, Cell-mediated responses of the immune system involve hemocytes quick transformation from their resting non-adherent states to their active adherent states (70). Hemocytes then form attachments with one another, allowing cell-cell interactions to form nodules (32). Foreign substrate recognition following immune challenge can induce β1 conformational change to transform hemocyte integrins into an active adhesive state to be involved in various cellular immune responses of S. exigua, based on a coupling role of integrins between the cell membrane and prediction of putative phosphorylation sites in βSe1 (39). Overall, the pieces of evidence indicate that integrins are an important component of plasma membranes that indirectly trigger adhesiveness in plasma membranes and ultimately cause nodule formation.
Integrins Modulate the Melanization Process
The Toll and IMD pathways drive the expression of antimicrobial peptides (AMPs) and other immune responsive genes in insects and hence play a crucial biological role in the innate immune system (8, 9, 71). However, these immune pathways take a few hours to a few days to induce their immune effectors. On the other hand, melanization is a more immediate immune response that occurs just a few minutes after infection. Melanization involves the oxidation of phenols to quinones, which then polymerize to produce melanin. Melanin is deposited around them to help sequester infiltrate microbial pathogens at wound sites. Microbial pathogens are thought to be directly harmful to the quinone substances and other reactive oxygen intermediates generated during melanization (8, 72). In addition, other processes in immune responses such as blood coagulation, wound healing, phagocytosis, and AMPs expression have been found to work together with the melanization reaction (8).
Integrins have been implicated in melanization processes in some invertebrate species. For example, A recent study on Litopenaeus vannamei has suggested that integrin β plays an important role in proPO activation (73). However, in insects, only one study reported a relationship between integrin and melanization (Figure 2) (37). The authors suppressed integrin β3 in the larval stage of a lepidopteran model species, the silkworm, B. mori, and then challenged with bacterial pathogen (37). After reducing integrin β3, the authors reported that hemolymph melanization increased. They also reported an increase in the transcription of melanization-related genes (PPO1, PPO2, BAEE, SPH78, SPH125, and SPH127). Interestingly, these findings are in contrast with the previous study of Lin et al. on crustaceans, who reported a decrease in the PO activity after β integrin knockdown (73). So far, the molecular mechanism by which integrins participate to control negatively or positively has not been discovered in crustaceans and insects. In addition, it appears that proPO activation occurs in the presence of PAMPs, which are recognized and bound by PRPs like LGBP, and the PAMP–PRP complex reacts with integrin (74, 75), suggesting indispensability of integrins for the PO activation. However, this mechanism may vary in different groups of animals or may species specific. Future studies may highlight how integrin-govern melanization process.
Integrins Regulate Immune Pathways
Toll-like receptors (TLRs) and a growing number of non-TLR receptors are responsible for the detection of pathogens. For example, integrins have also been shown to act as pattern recognition receptors and modulate downstream signaling (e.g., Toll and IMD) in order to stimulate immune factors (Figure 3) (76). The first evidence in insects came from the study of Zhang and his colleagues, who discovered that RNAi knockdown integrin influence the innate immune responses of B. mori by affecting immune signaling. The authors noted that silencing of β3 in the presence of bacterial challenge induced Toll and IMD signaling. They found that loss of β3 integrin increased the expression of Toll (Relish and FADD), and IMD (TRAF2, Pelle, and Tube) pathway-associated genes, implying that integrin β3 could negatively modulate Toll and IMD signaling pathways in insect. However, the authors did not study the mechanism behind the upregulation of Toll and IMD pathway-associated genes in the absence of β3 integrin (37).
In contrast, in vertebrates, Gianni and his co-workers suggested that αvβ3 integrin is an important sensor and activator of specific components of the innate responses to herpes simplex virus-1. By loss- and gain-of-function assays, αvβ3 integrin has been shown to be crucial for the production of type 1-IFNs and of a specific set of cytokines, which is a major determinant in NF-κB activation and reduces viral growth in a single replication cycle. Mechanistically, the αvβ3 integrin relocates the herpes simplex virus-1 receptor nectin1, thus herpes simplex virus-1, to cholesterol-rich membrane microdomains, where the virus is endocytosed while also triggering the innate immune response (77, 78). For herpes simplex virus-1 and possibly for a number of other viral and bacterial pathogens, αvβ3 integrin seems to be a non-TLR pattern recognition receptor (76). In the case of HSV infection in vertebrates, αvβ3 detects and binds to virion glycoprotein, which in turn binds to TLR2 (79).
Collectively, only one study in insects identified the integrin as a pattern recognition receptor that can negatively regulate the expression of Toll and IMD pathway-associated genes, which seems to be in contrast with vertebrates. Future studies need to evaluate how integrins work differently in different taxons. If they do so, then how do insect integrins negatively modulate immune signaling pathways. More research on diverse insect species may further highlight how integrins govern Toll and IMD signaling pathway pathways. In addition, these may also analyze the molecular mechanisms by which integrins modulate these immune signaling pathways.
Integrins Involvement in Wound Healing
A skin wound exposes underlying tissues and the entire organism to further damage and infection, so it must be healed quickly. “Reep-ithelialization” (RE) is a vital step of wound healing in which sheets of skin cells migrate toward and reseal the wound. Our knowledge of reep-ithelialization mechanisms is informed by studies of single migratory cells that must polarize, generate functionally different front and rear sides, as well as engage contractile mechanisms to exert force. Finally, controlled adhesion and deadhesion enable movement across a substratum, typically the extracellular matrix. Small GTPases of the Rho family mediate front/rear polarization (80–82), and actomyosin supplies propulsive forces, with actin polymerization driving lamellipodia extension (82, 83). Integrins are the major receptors for the extracellular matrix and are essential for cell crawling (84, 85). Extensive but poorly understood mutual regulation among the Rho-GTPases, actomyosin, and integrins directs the forward migration of cells (80, 82, 86).
Integrins, heterodimers of α and β transmembrane glycoproteins that bind the extracellular matrix, are especially important in reep-ithelialization. Integrins nucleate large cytoplasmic complexes that not only tune adhesion in response to both intracellular and extracellular cues (87, 88) but also engage in bidirectional signaling, affecting cytoskeletal activities and gene expression inside the cell (89) and shaping extracellular matrix composition on the outside (90, 91). Integrin abundance and function are also regulated by transcriptional modulation (92), switching of different integrins (93, 94), protein clustering and localization (95), vesicle trafficking (96), and protein turnover (97, 98).
A recent study using in vivo analysis reported the involvement of αPS2-βPS and αPS3-βPS as the crucial integrin dimers and talin as the only integrin adhesion in the reepithelialization (99). The authors noted severe reepithelialization defects in βPS, PS, and talin (integrin adhesion component) deficient Drosophila larvae, notably in αPS2-αPS3 pair depletion, indicating that PS2 and PS3 integrins play a crucial role in larval epidermal wound closure. The expression of these proteins is enormously increased in the wound surrounding cells in a JNK-dependent manner (99, 100). After that, the integrins rapidly accumulate in a few rows of cells surrounding a wound. Intriguingly, the integrins localize to the distal margin in these cells instead of the frontal or lamellipodial distribution expected for proteins providing traction and recruiting non-muscle myosin II to the same location (99).
Another study analyzed the process of polyploidization, which is essential for wound healing. Polyploid cells appear in adult tissues, at least in part, to promote tissue repair and restore tissue mass. However, the signaling required for polyploid cells in response to injury in insects has recently been discovered by Besen-McNally et al. (101). The authors demonstrated that wound-induced polyploid cells are generated by cell fusion and endoreplication, resulting in a giant polyploid syncytium in the adult Drosophila epithelium. They further showed that the integrin focal adhesion complex is an activator of wound-induced polyploidization. Both integrin and focal adhesion kinase are upregulated in the wound-induced polyploid cells and are required for Yorkie-induced endoreplication and cell fusion, as evidenced by wound healing is perturbed when focal adhesion genes (Mys and Fak) are knocked down (101). However, the focal adhesion complex that regulates cell fusion and formation of syncytium other signaling mechanisms may also derive wound closure in insects that may also be connected with integrin proteins that are still unidentified.
Integrins as a Specific Target for Pest Control
Various insect groups, such as coleopterans, lepidopterans, and aphids, have a number of well-known pest species, the majority of which are voracious feeders of plant materials (102–104). So far, a variety of chemical and biological control strategies have been applied to keep their population well below a threshold level. On the other hand, insect pests have developed sophisticated defense tactics to adapt to various insecticidal materials (105). This scenario demands the development of a new targets arena and their effective regulators.
Integrins have been demonstrated to have a role in a variety of physiological processes in insects, making them suitable pest control targets. Integrin receptors mainly modulate immune responses, development, growth, molecular signaling, and others (35); for example, Mohamed et al. suggested that integrin may be used as a specific target to control the lepidopteran pest, P. xylostella (66). The authors noted that the suppression of βPx1 by double-stranded RNA has an impact on the developmental and immune activities of this species. They showed that larvae treated with dsβPx1 had a slower developing rate and that those that survived metamorphosed into relatively small pupae. This could be due to a lack of βPx1, which could cause multiple disruptions in different physiological processes in which integrins are involved in cell-cell interactions. In addition, silencing of integrin β1 increases the mortality of Plutella xylostella. This further showed that dsRNA has oral toxicity in young immature stages, with toxicity based on RNA interference (RNAi) being more specific to P. xylostella in a dose-dependent manner. When a transgenic plant producing dsRNA is used to manage insect pests in the field, this specificity is extremely important because transgenic plants can be exposed to a variety of plant herbivores. The oral toxicity of dsβPx1 implies a novel transgenic-plant-based strategy for controlling P. xylostella (66).
Spodoptera exigua is a polyphagous lepidopteran pest with a broad distribution that causes severe economic damage to agricultural crops (18). βSe1 has been shown as an important target for controlling S. exigua (39). Surakasi et al., synthesized double-stranded RNA against βSe1 (dsRNAβSe1) and administered it to larvae orally. The pupal weight was substantially reduced as a result of oral feeding. The dsβSe1 treatment also impaired innate immune responses of S. exigua in response to bacterial challenge. Furthermore, oral ingestion of dsβSe1 triggered reduction of βSe1 expression in the midgut and resulted in considerable mortality of S. exigua during immature development, implying that βSe1 could be an effective target for controlling this pest species (39). Another study further highlighted the use of βSe1 as a pest management target site (106). This study provides an efficient approach for using dsRNA specific to an integrin gene by mixing it with a biopesticide, Bacillus thuringiensis (Bt). Similar to Surakasi et al., this study noted that transformed E. coli expressing dsβSe1give has a strong oral insecticidal effpicacy against young larval instars and delays the larval development. Some of the larvae have a miniature body form, which was most likely due to malnutrition induced by dsβSe1 damage to the midgut (39, 106). Kim et al., further suggested that the transformed bacteria expressed the dsRNA, and the amount of dsRNA produced was proportional to the number of bacterial cells. It indicates that one recombinant E. coli can produce 2.8 ± 0.1 pg of dsRNA as the authors demonstrated based on the total dsRNA amount and the bacterial cell counts. In addition, the authors demonstrated that 350 ng of dsRNA to be effective to give a maximal insecticidal activity by feeding the transformed E. coli because they found the maximal mortality from the bacterial treatment at 107 cells per larva. This insecticidal activity of dsβSe1could be triggered by a transformed bacterial treatment producing dsβSe1 that induces a serious impairment of the midgut epithelium. Finally, the efficacy of Bt toxin in combination with bacteria expressing dsβSe1. For this purpose, Kim et al., suggested that for the effective combinational activity of the bacteria expressing dsβSe1 to Bt toxin, βSe1 should be suppressed before the Bt treatment because the Bt toxicity increased with the elapse time after feeding the dsINT-expressing bacteria (106). A similar bacterial treatment study in S. exigua showed that it took more than seven days after the feeding (107). However, Kim et al. found that the effective RNAi effect appeared at 3 days after the bacterial feeding treatment to S. exigua larvae, at which the Bt efficacy was significantly enhanced (106). Thus, this increased effeicacy combinational treatment is an important technique to enhance the insecticidal efficacy of current Bt crops by supplementation with expression of dsRNA specific to integrin (Figure 4).
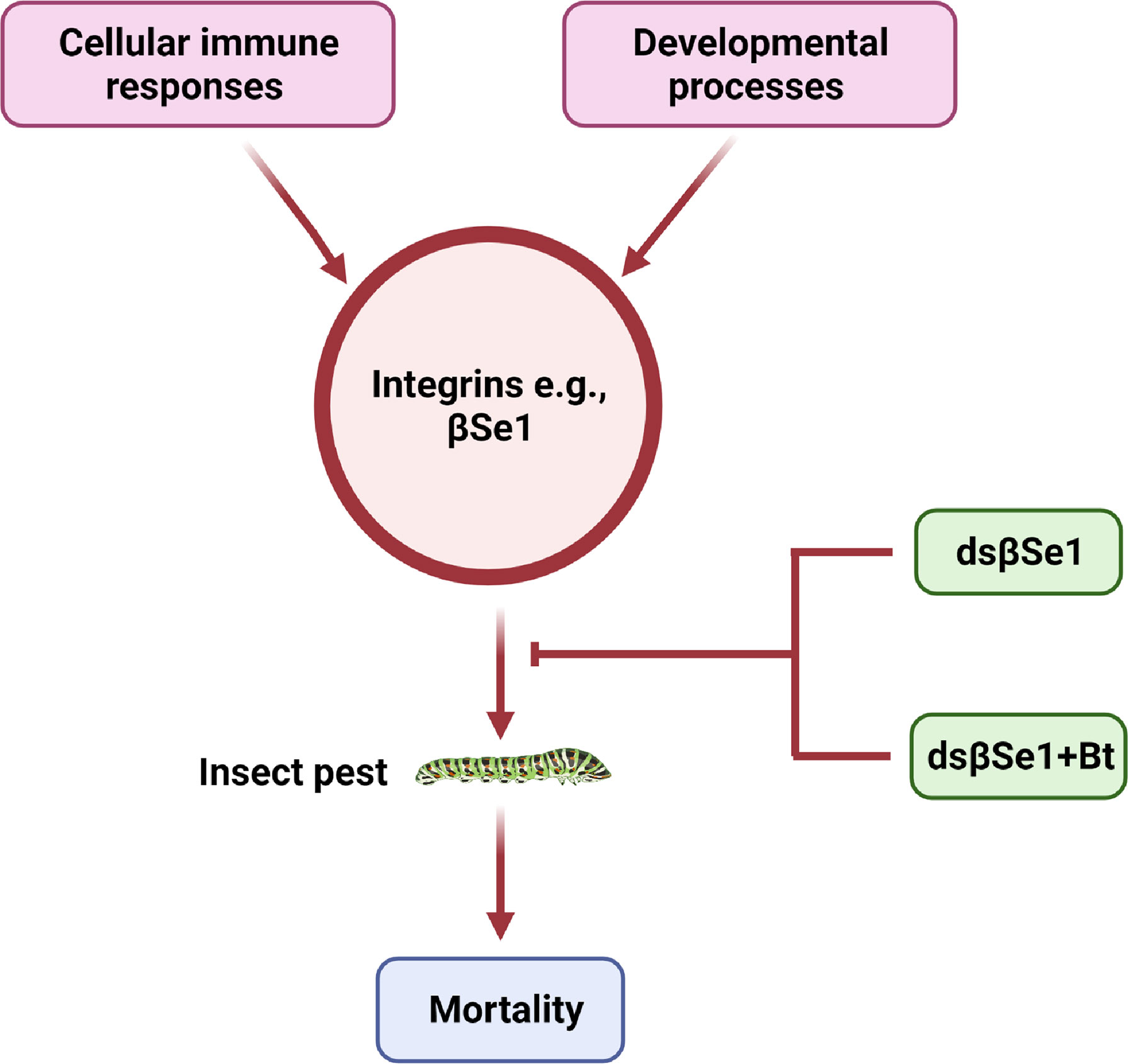
Figure 4 Integrins as a target for insect pest control, e.g., integrin βSe1 is vital for the development, cellular immune responses, and the survival of insects. A novel class of insecticidal compounds can be generated by using one more of the potential strategies.
The evidence provided above was sufficient to use βSe1 as a target sit for controlling S. exigua in the laboratory conditions. Later, Kim and Kim, developed a formulation technique of the dsRNA-expressing bacteria for applying the bacterial insecticide field populations (108). The authors formulated the recombinant bacteria by freeze-drying method and then tested its control efficacy against target insects. They found that the formulation had substantial insecticidal activity against the last instar larvae of S. exigua. In contrast, commercial Bt insecticide exhibits only about 60% insecticidal activity against S. exigua last instar. However, the authors did confirm that combining this formulation remarkably increased Bt insecticidal activity. They also suggested that integrin-expressing bacterial formulation exhibits relative selectivity to target insects depending on sequence similarity (108). These results suggest that βSe1 could be a key target to control pest species; however, insecticide products of this integrin seem to be species-specific or may be useful in species with integrin that have high similarity to βSe1.
Helicoverpa assulta, is an oligophagous insect that feeds on a wide range of commercially important plants (109). In H. assulta, the integrin β1 subunit is strongly produced in the gut and hemocytes, suggesting that it could be a promising target for insecticide development. Park et al. suggested that dsβ1 treatment increase H. assulta larval more susceptible to pathogenic Bt Cry toxins and X. nematophila (67). Thus, it seems that RNAi treatment of β1 integrin subunit in H. assulta may weaken the interaction of the resistant factor with Bt receptors and may enhance toxicity. These suggest that using dsRNA specific to the β1 subunit of integrin is a novel control strategy for H. assulta. Furthermore, the effects of RNAi on larval susceptibility to pathogens indicate that the combination of dsRNA and pathogenic bacteria to synergize the microbial pesticide control efficacy (67).
Overall, the researchers suggests that integrins could be a key target for developing effective insecticides against insect pests in the future. Although many integrins from different species have been shown to be target candidates for insecticides development, only the βSe1 integrin has been reported to be used as a formulation and its effectiveness (108). Therefore, future studies should focus on identifying more promising integrins candidates for insecticide synthesis in various insect species. The identified candidate then needs to use specific formulations under field conditions as well as evaluate their efficacy to cross-species.
Conclusion and Future Aspects
Integrins have been a topic of interest for several years due to their importance as a component of the cell membranes and their involvement in the development and the immune system. Insects and other invertebrates integrins seem to be structurally and functionally similar to vertebrate integrins, as indicated by significant progress in a functional study on insect integrins. Although, significant progress has been made on the biological roles of insect integrins over the last decades, but there is no comprehensive review so far on integrins regarding the immune system of insects. Integrins have been described in a variety of insect species, but their biological roles and molecular mechanisms are still unknown for most of the integrins. Additional studies on identification and functional characterization in insects are likely to be resolved remaining questions about the structural features and molecular mechanisms of integrin. With the complete sequencing of genomes, we will have access to the full complement of integrin subunits in organisms. Also, the further advancement of molecular genetic methods employed in the context of cell biology questions will permit a wider range of functional studies. Because integrin structure and function in invertebrates are similar to that of vertebrates, these studies will remain relevant and potentially may contribute substantially to our understanding of this remarkable group of receptors. Integrin negatively regulates the production of antimicrobial peptides; however, the mechanisms by which it mediates signaling are undiscovered. For example, beta integrin 3 of B. mori has been shown to control antimicrobial peptides, but how it controls this process is still unknown. In addition, to further ensure the regulatory role of the immune signaling cascade, a comparative study is needed. Our understanding of the molecular basis of these processes continues to advance, bringing with it the potential for strategies that modulate integrins and their associated signaling for the management of commercial insects and control of agricultural pests.
Author Contributions
The authors’ responsibilities were as follows: L-SD, Q-NL and IM designed this review article. L-SD, SK, MNA, IG, YL, and B-PT downloaded material and write down draft. L-SD, Q-NL and IM draw the digram and proofread the article. All authors contributed to the article and approved the submitted version.
Funding
This work was funded by the Natural Science Foundation of Zhejiang Province, China (No. LQ20C190009), the Natural Science Research General Program of Jiangsu Provincial Higher Education Institutions (21KJA240003), the Natural Science Foundation of Jiangsu Province (BE2020673), the Doctorial Start-up Fund of Southwest University (SWU020023), the National Key R&D Program of China (2019YFD0900404-05), the Industry-University-Research Cooperation Project of Jiangsu Province (BY2020644), the Open Funding Project of Anhui Province Key Laboratory of Aquaculture & Stock Enhancement (AHSC202001), the 16th Six Talents Peak Project of Jiangsu Province (NY-126), the National Natural Science Foundation of China (32070526), the Jiangsu Agriculture Science and Technology Innovation Fund (CX(18)3027). The study was sponsored by the Qing Lan Project of Jiangsu Province, and the ‘Outstanding Young Talents’ of YCTU.
Conflict of Interest
The authors declare that the research was conducted in the absence of any commercial or financial relationships that could be construed as a potential conflict of interest.
Publisher’s Note
All claims expressed in this article are solely those of the authors and do not necessarily represent those of their affiliated organizations, or those of the publisher, the editors and the reviewers. Any product that may be evaluated in this article, or claim that may be made by its manufacturer, is not guaranteed or endorsed by the publisher.
References
1. Humphries JD, Byron A, Humphries MJ. Integrin Ligands at a Glance. J Cell Sci (2006) 119(Pt 19):3901–3. doi: 10.1242/jcs.03098
2. Hynes RO. Integrins: Bidirectional, Allosteric Signaling Machines. Cell (2002) 110(6):673–87. doi: 10.1016/S0092-8674(02)00971-6
3. Tamkun JW, DeSimone DW, Fonda D, Patel RS, Buck C, Horwitz AF, et al. Structure of Integrin, a Glycoprotein Involved in the Transmembrane Linkage Between Fibronectin and Actin. Cell (1986) 46(2):271–82. doi: 10.1016/0092-8674(86)90744-0
4. Calderwood DA, Shattil SJ, Ginsberg MH. Integrins and Actin Filaments: Reciprocal Regulation of Cell Adhesion and Signaling. J Biol Chem (2000) 275(30):22607–10. doi: 10.1074/jbc.R900037199
5. Evans EA, Calderwood DA. Forces and Bond Dynamics in Cell Adhesion. Science (2007) 316(5828):1148–53. doi: 10.1126/science.1137592
6. Miranti CK, Brugge JS. Sensing the Environment: A Historical Perspective on Integrin Signal Transduction. Nat Cell Biol (2002) 4(4):E83–90. doi: 10.1038/ncb0402-e83
8. Kausar S, Abbas MN, Zhao Y, Cui H. Immune Strategies of Silkworm, Bombyx Mori Against Microbial Infections. ISJ Invertebrate Survival J (2019) p:130–40. doi: 10.25431/1824-307X/isj.v0i0.130-140
9. Abbas MN, Kausar S, Zhao E, Cui H. Suppressors of Cytokine Signaling Proteins as Modulators of Development and Innate Immunity of Insects. Dev Comp Immunol (2020) 104:103561. doi: 10.1016/j.dci.2019.103561
10. Kausar S, Abbas MN, Cui H. A Review on the DNA Methyltransferase Family of Insects: Aspect and Prospects. Int J Biol Macromol (2021) 186:289–302. doi: 10.1016/j.ijbiomac.2021.06.205
11. Stepp MA. Corneal Integrins and Their Functions. Exp Eye Res (2006) 83(1):3–15. doi: 10.1016/j.exer.2006.01.010
12. Campbell ID, Humphries MJ. Integrin Structure, Activation, and Interactions. Cold Spring Harbor Perspect Biol (2011) 3(3):a004994. doi: 10.1101/cshperspect.a004994
13. Hughes AL. Evolution of the Integrin α and β Protein Families. J Mol Evol (2001) 52(1):63–72. doi: 10.1007/s002390010134
14. Humphries MJ, Travis MA, Clark K, Mould AP. Mechanisms of Integration of Cells and Extracellular Matrices by Integrins. Biochem Soc Trans (2004) 32(Pt 5):822–5. doi: 10.1042/BST0320822
15. Giancotti FG. A Structural View of Integrin Activation and Signaling. Dev Cell (2003) 4(2):149–51. doi: 10.1016/S1534-5807(03)00034-0
16. Zhang K, Tan J, Xu M, Su J, Hu R, Chen Y, et al. A Novel Granulocyte-Specific α Integrin is Essential for Cellular Immunity in the Silkworm Bombyx Mori. J Insect Physiol (2014) 71:61–7. doi: 10.1016/j.jinsphys.2014.10.007
17. Brown NH. Integrins Hold Drosophila Together. Bioessays (1993) 15(6):383–90. doi: 10.1002/bies.950150604
18. Stark KA, Yee GH, Roote CE, Williams EL, Zusman S, Hynes RO. A Novel Alpha Integrin Subunit Associates With betaPS and Functions in Tissue Morphogenesis and Movement During Drosophila Development. Development (1997) 124(22):4583–94. doi: 10.1242/dev.124.22.4583
19. Yee GH, Hynes RO. A Novel, Tissue-Specific Integrin Subunit, Beta Nu, Expressed in the Midgut of Drosophila Melanogaster. Dev (Cambridge England) (1993) 118(3):845–58. doi: 10.1242/dev.118.3.845
20. Hynes RO, Zhao Q. The Evolution of Cell Adhesion. J Cell Biol (2000) 150(2):F89–96. doi: 10.1083/jcb.150.2.F89
21. Martin-Bermudo MD, Dunin-Borkowski OM, Brown NH. Specificity of PS Integrin Function During Embryogenesis Resides in the Alpha Subunit Extracellular Domain. EMBO J (1997) 16(14):4184–93. doi: 10.1093/emboj/16.14.4184
22. Bunch TA, Miller SW, Brower DL. Analysis of the Drosophila betaPS Subunit Indicates That Regulation of Integrin Activity is a Primal Function of the C8-C9 Loop. Exp Cell Res (2004) 294(1):118–29. doi: 10.1016/j.yexcr.2003.11.002
23. Graner MW, Bunch TA, Baumgartner S, Kerschen A, Brower DL. Splice Variants of the Drosophila PS2 Integrins Differentially Interact With RGD-Containing Fragments of the Extracellular Proteins Tiggrin, Ten-M, and D-Laminin α2*. J Biol Chem (1998) 273(29):18235–41. doi: 10.1074/jbc.273.29.18235
24. Zusman S, Grinblat Y, Yee G, Kafatos FC, Hynes RO. Analyses of PS Integrin Functions During Drosophila Development. Development (1993) 118(3):737–50. doi: 10.1242/dev.118.3.737
25. Hayashi Y, Hayashi M, Kobayashi S. Nanos Suppresses Somatic Cell Fate in Drosophila Germ Line. Proc Natl Acad Sci USA (2004) 101(28):10338–42. doi: 10.1073/pnas.0401647101
26. Takada Y, Ye X, Simon S. The Integrins. Genome Biol (2007) 8(5):215. doi: 10.1186/gb-2007-8-5-215
27. Giancotti FG, Ruoslahti E. Integrin Signaling. Science (1999) 285(5430):1028–32. doi: 10.1126/science.285.5430.1028
28. Zervas CG, Gregory SL, Brown NH. Drosophila Integrin-Linked Kinase Is Required at Sites of Integrin Adhesion to Link the Cytoskeleton to the Plasma Membrane. J Cell Biol (2001) 152(5):1007–18. doi: 10.1083/jcb.152.5.1007
29. Lämmermann T, Sixt M. The Microanatomy of T-Cell Responses. Immunol Rev (2008) 221:26–43. doi: 10.1111/j.1600-065X.2008.00592.x
30. Chtanova T, Schaeffer M, Han SJ, van Dooren GG, Nollmann M, Herzmark P, et al. Dynamics of Neutrophil Migration in Lymph Nodes During Infection. Immunity (2008) 29(3):487–96. doi: 10.1016/j.immuni.2008.07.012
31. Abadie V, Badell E, Douillard P, Ensergueix D, Leenen PJM, Tanguy M, et al. Neutrophils Rapidly Migrate via Lymphatics After Mycobacterium Bovis BCG Intradermal Vaccination and Shuttle Live Bacilli to the Draining Lymph Nodes. Blood (2005) 106(5):1843–50. doi: 10.1182/blood-2005-03-1281
32. Lavine MD, Strand MR. Haemocytes From Pseudoplusia Includens Express Multiple Alpha and Beta Integrin Subunits. Insect Mol Biol (2003) 12(5):441–52. doi: 10.1046/j.1365-2583.2003.00428.x
33. Levin DM, Breuer LN, Zhuang S, Anderson SA, Nardi JB, Kanost MR. A Hemocyte-Specific Integrin Required for Hemocytic Encapsulation in the Tobacco Hornworm, Manduca Sexta. Insect Biochem Mol Biol (2005) 35(5):369–80. doi: 10.1016/j.ibmb.2005.01.003
34. Zhuang S, Kelo L, Nardi JB, Kanost MR. Multiple α Subunits of Integrin are Involved in Cell-Mediated Responses of the Manduca Immune System. Dev Comp Immunol (2008) 32(4):365–79. doi: 10.1016/j.dci.2007.07.007
35. Li C, Zhang K, Pan G, Zhang L, Hu X, Zhao G, et al. Bmintegrin β1: A Broadly Expressed Molecule Modulates the Innate Immune Response of Bombyx Mori. Dev Comp Immunol (2021) 114:103869. doi: 10.1016/j.dci.2020.103869
36. Hu J, Zhao H, Yu X, Liu J, Wang P, Chen J, et al. Integrin β1 Subunit From Ostrinia Furnacalis Hemocytes: Molecular Characterization, Expression, and Effects on the Spreading of Plasmatocytes. J Insect Physiol (2010) 56(12):1846–56. doi: 10.1016/j.jinsphys.2010.08.001
37. Zhang K, Tan J, Su J, Liang H, Shen L, Li C, Pan G, et al. Integrin β3 Plays a Novel Role in Innate Immunity in Silkworm, Bombyx Mori. Dev Comp Immunol (2017) 77:307–17. doi: 10.1016/j.dci.2017.08.009
38. Skowronek P, Wójcik Ł, Strachecka A. Fat Body-Multifunctional Insect Tissue. (2021) 12(6):547. doi: 10.3390/insects12060547
39. Surakasi VP, Mohamed AA, Kim Y. RNA Interference of β1 Integrin Subunit Impairs Development and Immune Responses of the Beet Armyworm, Spodoptera Exigua. J Insect Physiol (2011) 57(11):1537–44. doi: 10.1016/j.jinsphys.2011.08.006
40. Zhang MM, Ling E, Yu XQ. Calcium and Integrin-Binding Protein 1-Like Interacting With an Integrin α-Cytoplasmic Domain Facilitates Cellular Immunity in Helicoverpa Armigera. Dev Comp Immunol (2022) 131:104379. doi: 10.1016/j.dci.2022.104379
41. Ling E, Yu X-Q. Prophenoloxidase Binds to the Surface of Hemocytes and is Involved in Hemocyte Melanization in Manduca Sexta. Insect Biochem Mol Biol (2005) 35(12):1356–66. doi: 10.1016/j.ibmb.2005.08.007
42. Marmaras VJ, Lampropoulou M. Regulators and Signalling in Insect Haemocyte Immunity. Cell Signal (2009) 21(2):186–95. doi: 10.1016/j.cellsig.2008.08.014
43. Nazario-Toole AE, Wu LP. Chapter Two - Phagocytosis in Insect Immunity. In: Ligoxygakis P, editor. Advances in Insect Physiology. Academic Press (2017). p. 35–82. doi: 10.1016/bs.aiip.2016.12.001
44. Xu Q, Yu X, Liu J, Zhao H, Wang P, Hu S, et al. Ostrinia Furnacalis Integrin β1 may be Involved in Polymerization of Actin to Modulate Spreading and Encapsulation of Plasmatocytes. Dev Comp Immunol (2012) 37(3-4):438–45. doi: 10.1016/j.dci.2012.02.003
45. Foukas LC, Katsoulas HL, Paraskevopoulou N, Metheniti A, Lambropoulou M, Marmaras VJ, et al. Phagocytosis of Escherichia Coli by Insect Hemocytes Requires Both Activation of the Ras/mitogen-Activated Protein Kinase Signal Transduction Pathway for Attachment and Beta3 Integrin for Internalization. J Biol Chem (1998) 273(24):14813–8. doi: 10.1074/jbc.273.24.14813
46. Mamali I, Kotsantis P, Lampropoulou M, Marmaras VJ. Elk-1 is a Novel Protein-Binding Partner for FAK, Regulating Phagocytosis in Medfly Hemocytes. J Cell Biochem (2008) 103(6):1895–911. doi: 10.1002/jcb.21580
47. Johnson GL, Lapadat R. Mitogen-Activated Protein Kinase Pathways Mediated by ERK, JNK, and P38 Protein Kinases. Science (2002) 298(5600):1911–2. doi: 10.1126/science.1072682
48. Mamali I, Kotsantis P, Lampropoulou M, Marmaras VJ. Apoptosis in Medfly Hemocytes Is Regulated During Pupariation Through FAK, Src, ERK, PI-3K P85a, and Akt Survival Signaling. J Cell Biochem (2007) 101(2):331–47. doi: 10.1002/jcb.21175
49. Mamali I, Lamprou I, Karagiannis F, Karakantza M, Lampropoulou M, Marmaras VJ, et al. A β Integrin Subunit Regulates Bacterial Phagocytosis in Medfly Haemocytes. Dev Comp Immunol (2009) 33(7):858–66. doi: 10.1016/j.dci.2009.02.004
50. Moita LF, Vriend G, Mahairaki V, Louis C, Kafatos FC. Integrins of Anopheles Gambiae and a Putative Role of a New Beta Integrin, BINT2, in Phagocytosis of E. Coli. Insect Biochem Mol Biol (2006) 36(4):282–90. doi: 10.1016/j.ibmb.2006.01.004
51. Moita LF, Wang-Sattler R, Michel K, Zimmermann T, Blandin S, Levashina EA, et al. In Vivo Identification of Novel Regulators and Conserved Pathways of Phagocytosis in A. Gambiae. Immunity (2005) 23(1):65–73. doi: 10.1016/j.immuni.2005.05.006
52. Hanayama R, Tanaka M, Miwa K, Shinohara A, Iwamatsu A, Nagata S, et al. Identification of a Factor That Links Apoptotic Cells to Phagocytes. Nature (2002) 417(6885):182–7. doi: 10.1038/417182a
53. Jia Z, Zhang T, Jiang S, Wang M, Cheng Q, Sun M, et al. An Integrin From Oyster Crassostrea Gigas Mediates the Phagocytosis Toward Vibrio Splendidus Through LPS Binding Activity. Dev Comp Immunol (2015) 53(1):253–64. doi: 10.1016/j.dci.2015.07.014
54. Huang Y, Zhao LL, Feng JL, Zhu HX, Huang X, Ren Q, et al. A Novel Integrin Function in Innate Immunity From Chinese Mitten Crab (Eriocheir Sinensis). Dev Comp Immunol (2015) 52(2):155–65. doi: 10.1016/j.dci.2015.05.005
55. Wang Z, Shao Y, Li C, Lv Z, Wang H, Zhang W, et al. A β-Integrin From Sea Cucumber Apostichopus Japonicus Exhibits LPS Binding Activity and Negatively Regulates Coelomocyte Apoptosis. Fish Shellfish Immunol (2016) 52:103–10. doi: 10.1016/j.fsi.2016.03.031
56. Ling E, Yu XQ. Cellular Encapsulation and Melanization are Enhanced by Immulectins, Pattern Recognition Receptors From the Tobacco Hornworm Manduca Sexta. Dev Comp Immunol (2006) 30(3):289–99. doi: 10.1016/j.dci.2005.05.005
57. Irving P, Ubeda JM, Doucet D, Troxler L, Lagueux M, Zachary D, et al. New Insights Into Drosophila Larval Haemocyte Functions Through Genome-Wide Analysis. Cell Microbiol (2005) 7(3):335–50. doi: 10.1111/j.1462-5822.2004.00462.x
58. Zhang K, Su J, Hu X, Yan X, Chen S, Li C, G, et al. Integrin β2 and β3: Two Plasmatocytes Markers Deepen Our Understanding of the Development of Plasmatocytes in the Silkworm Bombyx Mori. Insect Science (2022) 0:1–13. doi: 10.1111/1744-7917.13045
59. Wang X, et al. Mosquito Innate Immunity: Involvement of Beta 1,3-Glucan Recognition Protein in Melanotic Encapsulation Immune Responses in Armigeres Subalbatus. Mol Biochem Parasitol (2005) 139(1):65–73. doi: 10.1016/j.molbiopara.2004.09.009
60. Zhuang S, Kelo L, Nardi JB, Kanost MR. An Integrin-Tetraspanin Interaction Required for Cellular Innate Immune Responses of an Insect, Manduca Sexta*. J Biol Chem (2007) 282(31):22563–72. doi: 10.1074/jbc.M700341200
61. Wang XW, Xu JD, Zhao XF, Vasta GR, Wang JX. A Shrimp C-Type Lectin Inhibits Proliferation of the Hemolymph Microbiota by Maintaining the Expression of Antimicrobial Peptides. J Biol Chem (2014) 289(17):11779–90. doi: 10.1074/jbc.M114.552307
62. Wang P, Zhuo XR, Tang L, Liu XS, Wang YF, Wang G.X., et al. C-Type Lectin Interacting With β-Integrin Enhances Hemocytic Encapsulation in the Cotton Bollworm, Helicoverpa Armigera. Insect Biochem Mol Biol (2017) 86:29–40. doi: 10.1016/j.ibmb.2017.05.005
63. Ratcliffe NA, Gagen SJ. Studies on the In Vivo Cellular Reactions of Insects: An Ultrastructural Analysis of Nodule Formation in Galleria Mellonella. Tissue Cell (1977) 9(1):73–85. doi: 10.1016/0040-8166(77)90050-7
64. Lapointe JF, Dunphy GB, Mandato CA. Hemocyte–hemocyte Adhesion and Nodulation Reactions of the Greater Wax Moth, Galleria Mellonella A\re Influenced by Cholera Toxin and its B-Subunit. Results Immunol (2012) 2:54–65. doi: 10.1016/j.rinim.2012.02.002
65. Zhang Q, Huang J, Li F, Liu S, Liu Q, Wei J, et al. Molecular Characterization, Immune Response Against White Spot Syndrome Virus Infection of Peroxiredoxin 4 in Fenneropenaeus Chinensis and its Antioxidant Activity. Fish Shellfish Immunol (2014) 37(1):38–45. doi: 10.1016/j.fsi.2013.12.026
66. Mohamed AAM, Kim Y. A Target-Specific Feeding Toxicity of ²(1) Integrin dsRNA Against Diamondback Moth, Plutella Xylostella. Arch Insect Biochem Physiol (201) 78 4:216–30. doi: 10.1002/arch.20455
67. Park Y, Ahn SJ, Vogel H, Kim Y. Integrin β Subunit and its RNA Interference in Immune and Developmental Processes of the Oriental Tobacco Budworm, Helicoverpa Assulta. Dev Comp Immunol (2014) 47(1):59–67. doi: 10.1016/j.dci.2014.06.017
68. Nardi JB, Pilas B, Bee CM, Zhuang S, Garsha K, Kanost MR, et al. Neuroglian-Positive Plasmatocytes of Manduca Sexta and the Initiation of Hemocyte Attachment to Foreign Surfaces. Dev Comp Immunol (2006) 30(5):447–62. doi: 10.1016/j.dci.2005.06.026
69. Bouti P, Webbers SDS, Fagerholm SC, Alon R, Moser M, Matlung HL, et al. β2 Integrin Signaling Cascade in Neutrophils: More Than a Single Function. Front Immunol (2021) 11:619925–5. doi: 10.3389/fimmu.2020.619925
70. Gillespie JP, Kanost MR, Trenczek T. Biological Mediators of Insect Immunity. Annu Rev Entomol (1997) 42(1):611–43. doi: 10.1146/annurev.ento.42.1.611
71. Ferrandon D, Imler JL, Hetru C, Hoffmann JA. The Drosophila Systemic Immune Response: Sensing and Signalling During Bacterial and Fungal Infections. Nat Rev Immunol (2007) 7(11):862–74. doi: 10.1038/nri2194
72. Christensen BM, Li J, Chen CC, Nappi AJ. Melanization Immune Responses in Mosquito Vectors. Trends Parasitol (2005) 21(4):192–9. doi: 10.1016/j.pt.2005.02.007
73. Lin YC, Chen JC, Chen YY, Liu CH, Cheng W, Hsu CH, et al. Characterization of White Shrimp Litopenaeus Vannamei Integrin β and its Role in Immunomodulation by dsRNA-Mediated Gene Silencing. Dev Comp Immunol (2013) 40(2):167–79. doi: 10.1016/j.dci.2013.01.001
74. Jiravanichpaisal P, Lee BL, Söderhäll K. Cell-Mediated Immunity in Arthropods: Hematopoiesis, Coagulation, Melanization and Opsonization. Immunobiology (2006) 211(4):213–36. doi: 10.1016/j.imbio.2005.10.015
75. Kausar S, Abbas MN, Qian C, Zhu B, Sun Y, Sun Y, et al. Serpin-14 Negatively Regulates Prophenoloxidase Activation and Expression of Antimicrobial Peptides in Chinese Oak Silkworm Antheraea Pernyi. Dev Comp Immunol (2017) 76:45–55. doi: 10.1016/j.dci.2017.05.017
76. Gianni T, Campadelli-Fiume G. αvβ3-Integrin Relocalizes Nectin1 and Routes Herpes Simplex Virus to Lipid Rafts. J Virol (2012) 86(5):2850–5. doi: 10.1128/JVI.06689-11
77. Gianni T, Leoni V, Chesnokova LS, Hutt-Fletcher LM, Campadelli-Fiume G. αvβ3-Integrin is a Major Sensor and Activator of Innate Immunity to Herpes Simplex Virus-1. Proc Natl Acad Sci USA. (2012) 109(48):19792–7. doi: 10.1073/pnas.1212597109
78. Gianni T, Gatta V, Campadelli-Fiume G. αvβ3-Integrin Routes Herpes Simplex Virus to an Entry Pathway Dependent on Cholesterol-Rich Lipid Rafts and Dynamin2. Proc Natl Acad Sci USA. (2010) 107(51):22260–5. doi: 10.1073/pnas.1014923108
79. Leoni V, Gianni T, Salvioli S, Campadelli-Fiume G. Herpes Simplex Virus Glycoproteins Gh/gL and gB Bind Toll-Like Receptor 2, and Soluble Gh/gL is Sufficient to Activate NF-κb. J Virol (2012) 86(12):6555–62. doi: 10.1128/JVI.00295-12
80. Ridley AJ, Schwartz MA, Burridge K, Firtel RA, Ginsberg MH, Borisy G, et al. Cell Migration: Integrating Signals From Front to Back. Science (2003) 302(5651):1704–9. doi: 10.1126/science.1092053
81. Charest PG, Firtel RA. Big Roles for Small GTPases in the Control of Directed Cell Movement. Biochem J (2007) 401(2):377–90. doi: 10.1042/BJ20061432
82. Parsons JT, Horwitz AR, Schwartz MA. Cell Adhesion: Integrating Cytoskeletal Dynamics and Cellular Tension. Nat Rev Mol Cell Biol (2010) 11(9):633–43. doi: 10.1038/nrm2957
83. Vicente-Manzanares M, Ma X, Adelstein RS, Horwitz AR. Non-Muscle Myosin II Takes Centre Stage in Cell Adhesion and Migration. Nat Rev Mol Cell Biol (2009) 10(11):778–90. doi: 10.1038/nrm2786
84. Scales TM, Parsons M. Spatial and Temporal Regulation of Integrin Signalling During Cell Migration. Curr Opin Cell Biol (2011) 23(5):562–8. doi: 10.1016/j.ceb.2011.05.008
85. Maartens AP, Brown NH. Anchors and Signals: The Diverse Roles of Integrins in Development. Curr Top Dev Biol (2015) 112:233–72. doi: 10.1016/bs.ctdb.2014.11.020
86. Gupton SL, Waterman-Storer CM. Spatiotemporal Feedback Between Actomyosin and Focal-Adhesion Systems Optimizes Rapid Cell Migration. Cell (2006) 125(7):1361–74. doi: 10.1016/j.cell.2006.05.029
87. Horton ER, Byron A, Askari JA, Ng DHJ, Millon-Frémillon A, Robertson J, et al. Definition of a Consensus Integrin Adhesome and its Dynamics During Adhesion Complex Assembly and Disassembly. Nat Cell Biol (2015) 17(12):1577–87. doi: 10.1038/ncb3257
88. Iwamoto DV, Calderwood DA. Regulation of Integrin-Mediated Adhesions. Curr Opin Cell Biol (2015) 36:41–7. doi: 10.1016/j.ceb.2015.06.009
89. Legate KR, Wickström SA, Fässler R. Genetic and Cell Biological Analysis of Integrin Outside-in Signaling. Genes Dev (2009) 23(4):397–418. doi: 10.1101/gad.1758709
90. deHart GW, Healy KE, Jones JC. The Role of Alpha3beta1 Integrin in Determining the Supramolecular Organization of Laminin-5 in the Extracellular Matrix of Keratinocytes. Exp Cell Res (2003) 283(1):67–79. doi: 10.1016/S0014-4827(02)00028-9
91. Humphrey JD, Dufresne ER, Schwartz MA. Mechanotransduction and Extracellular Matrix Homeostasis. Nat Rev Mol Cell Biol (2014) 15(12):802–12. doi: 10.1038/nrm3896
92. Homsy JG, Jasper H, Peralta XG, Wu H, Kiehart DP, Bohmann D, et al. JNK Signaling Coordinates Integrin and Actin Functions During Drosophila Embryogenesis. Dev Dyn (2006) 235(2):427–34. doi: 10.1002/dvdy.20649
93. Truong H, Danen EHJ. Integrin Switching Modulates Adhesion Dynamics and Cell Migration. Cell Adhesion Migration (2009) 3(2):179–81. doi: 10.4161/cam.3.2.8036
94. Elosegui-Artola A, Bazellières E, Allen MD, Andreu I, Oria R, Sunyer R, et al. Rigidity Sensing and Adaptation Through Regulation of Integrin Types. Nat Mater (2014) 13(6):631–7. doi: 10.1038/nmat3960
95. Yamaguchi N, Mizutani T, Kawabata K, Haga H. Leader Cells Regulate Collective Cell Migration via Rac Activation in the Downstream Signaling of Integrin β1 and PI3K. Sci Rep (2015) 5:7656. doi: 10.1038/srep07656
96. Paul S, Willmes S, Hoppmann M, Hunkeler PA, Wesche C, Nicolaus M, et al. Snow Grain Size and Type Determination on Atka Bay Landfast Sea Ice During ANT-Land 2012/2013 Season. In: In Supplement to: Paul, S Et al. (2015): The Impact of Early-Summer Snow Properties on Antarctic Landfast Sea-Ice X-Band Backscatter. Annals of Glaciology, vol. 56. PANGAEA (2015). p. 263–73. doi: 10.3189/2015AoG69A715
97. Webb DJ, Parsons JT, Horwitz AF. Adhesion Assembly, Disassembly and Turnover in Migrating Cells – Over and Over and Over Again. Nat Cell Biol (2002) 4(4):E97–100. doi: 10.1038/ncb0402-e97
98. López-Ceballos P, Herrera-Reyes AD, Coombs DX, Tanentzapf G. In Vivo Regulation of Integrin Turnover by Outside-in Activation. J Cell Sci (2016) 129(15):2912–24. doi; 10.1242/jcs.190256
99. Park SH, Lee CW, Lee JH, Park JY, Roshandell M, Brennan CA, et al. Requirement for and Polarized Localization of Integrin Proteins During Drosophila Wound Closure. Mol Biol Cell (2018) 29(18):2137–47. doi: 10.1091/mbc.E17-11-0635
100. Wang Y, Antunes M, Anderson AE, Kadrmas JL, Jacinto A, Galko MJ, et al. Integrin Adhesions Suppress Syncytium Formation in the Drosophila Larval Epidermis. Curr Biol (2015) 25(17):2215–27. doi: 10.1016/j.cub.2015.07.031
101. Besen-McNally R, Gjelsvik KJ, Losick VP. Wound-Induced Polyploidization is Dependent on Integrin-Yki Signaling. Biol Open (2021) 10(1):bio055996. doi: 10.1242/bio.055996.
102. Sun YX, Wang L, Wei GQ, Qian C, Dai LS. Characterization of the Complete Mitochondrial Genome of Leucoma Salicis (Lepidoptera: Lymantriidae) and Comparison With Other Lepidopteran Insects. Sci Rep (2016) 6(1):39153. doi: 10.1038/srep39153
103. Dai LS, Kausar S, Abbas MN, Wang TT. Complete Sequence and Characterization of the Ectropis Oblique Mitochondrial Genome and its Phylogenetic Implications. Int J Biol Macromol (2018) 107(Pt A):1142–50. doi: 10.1016/j.ijbiomac.2017.09.093
104. Zheng N, Sun YX, Yang LL, Wuc L, Abbas MN, Chen C, et al. Characterization of the Complete Mitochondrial Genome of Biston Marginata (Lepidoptera: Geometridae) and Phylogenetic Analysis Among Lepidopteran Insects. Int J Biol Macromol (2018) 113:961–70. doi: 10.1016/j.ijbiomac.2018.02.110
105. Zhu F, Lavine L, O'Neal S, Lavine M, Foss C, Walsh D, et al. Insecticide Resistance and Management Strategies in Urban Ecosystems. Insects (2016) 7(1):2. doi: 10.3390/insects7010002
106. Kim E, Park Y, Kim Y. A Transformed Bacterium Expressing Double-Stranded RNA Specific to Integrin β1 Enhances Bt Toxin Efficacy Against a Polyphagous Insect Pest, Spodoptera Exigua. PloS One (2015) 10(7):e0132631–e0132631. doi: 10.1371/journal.pone.0132631
107. Tian H, Peng H, Yao Q, Chen H, Xie Q, Tang B, et al. Developmental Control of a Lepidopteran Pest Spodoptera Exigua by Ingestion of Bacteria Expressing dsRNA of a non-Midgut Gene. PloS One (2009) 4(7):e6225. doi: 10.1371/journal.pone.0006225
108. Kim E, Kim Y. A Freeze-Drying Formulation and Target Specificity of Double-Stranded RNA-Expressing Bacteria to Control Insect Pests. Korean J Appl Entomol (2016) p:81–9. doi: 10.5656/KSAE.2016.02.0.004
Keywords: insects, hemocytes, integrins, innate immunity, target for pest control
Citation: Kausar S, Abbas MN, Gul I, Liu Y, Tang B-P, Maqsood I, Liu Q-N and Dai L-S (2022) Integrins in the Immunity of Insects: A Review. Front. Immunol. 13:906294. doi: 10.3389/fimmu.2022.906294
Received: 28 March 2022; Accepted: 02 May 2022;
Published: 09 June 2022.
Edited by:
Zhen Zou, Chinese Academy of Sciences (CAS), ChinaReviewed by:
Yeon Soo Han, Chonnam National University, South KoreaLingling Zhang, Fujian Agriculture and Forestry University, China
Copyright © 2022 Kausar, Abbas, Gul, Liu, Tang, Maqsood, Liu and Dai. This is an open-access article distributed under the terms of the Creative Commons Attribution License (CC BY). The use, distribution or reproduction in other forums is permitted, provided the original author(s) and the copyright owner(s) are credited and that the original publication in this journal is cited, in accordance with accepted academic practice. No use, distribution or reproduction is permitted which does not comply with these terms.
*Correspondence: Bo-Ping Tang, Ym9wdGFuZ0AxNjMuY29t; Iram Maqsood, ZHIuaXJhbW1hcXNvb2RAc2Jid3UuZWR1LnBr; Qiu-Ning Liu, bGl1cW5AeWN0dS5lZHUuY24=; Li-Shang Dai, bGlzaGFuZzIwMTZAd211LmVkdS5jbg==
†These authors have contributed equally to this work