- 1State Key Laboratory of Ecological Pest Control for Fujian and Taiwan Crops, College of Life Sciences, Fujian Agriculture and Forestry University, Fuzhou, China
- 2Key Lab of Biopesticides and Chemical Biology, MOE, Fujian Agriculture and Forestry University, Fuzhou, China
- 3College of Life Sciences, South China Agricultural University, Guangzhou, China
- 4Nanjing Bioengineering (Gene) Technology Center for Medicines, Nanjing, China
Aedes aegypti is one of the world’s most dangerous mosquitoes, and a vector of diseases such as dengue fever, chikungunya virus, yellow fever, and Zika virus disease. Currently, a major global challenge is the scarcity of antiviral medicine and vaccine for arboviruses. Bacillus thuringiensis var israelensis (Bti) toxins are used as biological mosquito control agents. Endotoxins, including Cry4Aa, Cry4Ba, Cry10Aa, Cry11Aa, and Cyt1Aa, are toxic to mosquitoes. Insect eradication by Cry toxin relies primarily on the interaction of cry toxins with key toxin receptors, such as aminopeptidase (APN), alkaline phosphatase (ALP), cadherin (CAD), and ATP-binding cassette transporters. The carbohydrate recognition domains (CRDs) of lectins and domains II and III of Cry toxins share similar structural folds, suggesting that midgut proteins, such as C-type lectins (CTLs), may interfere with interactions among Cry toxins and receptors by binding to both and alter Cry toxicity. In the present review, we summarize the functional role of C-type lectins in Ae. aegypti mosquitoes and the mechanism underlying the alteration of Cry toxin activity by CTLs. Furthermore, we outline future research directions on elucidating the Bti resistance mechanism. This study provides a basis for understanding Bti resistance, which can be used to develop novel insecticides.
Introduction
The mosquito Aedes aegypti is one of the most important species responsible for transmitting viruses that cause life-threatening and epidemic human diseases worldwide, such as dengue virus (DENV), yellow fever virus (YFV), chikungunya virus (CHIKV) and, Zika virus (ZIKV), which drastically affect human populations (1). Dengue fever is a rapidly spreading arbovirus that has become a global health concern (2). The rapid expansion of CHIKV and ZIKV demands the identification of circulating lineages to design effective surveillance programs. The main vectors for the spread of these viruses in urban areas are Ae. aegypti (L.) and Ae. albopictus (Skuse), although other mosquito species have also been reported (3–5). To date, no efficient antiviral drugs or vaccines have been developed to control these viral diseases, with the exception of yellow fever. As a necessary consequence, efforts to control mosquito populations remain a critical strategy for reducing infection rates.
Chemical insecticides with active components, such as organophosphates, pyrethroids, organochlorines, and carbamates, have been used to control these disease vectors (6, 7). However, these chemicals are damaging to both the natural environment and human health. They cause depletion of natural enemies in the ecosystem and the development of insect resistance when used continuously (8–10). In recent years, chemical insecticides have been successfully replaced by eco-friendly biological control agents with high specificity, minimal influence on non-target organisms, and reduced insect resistance (11–13). Entomopathogenic bacteria, such as Bacillus thuringiensis (Bt), which produce different toxin spores, represent a promising substitute for mosquito control. These bacterial spores have a high potential to control insect pests (14–16) (17). Bt produces a number of crystal proteins that have insecticidal activity against over 3000 insect species, including Coleoptera, Lepidoptera, and Dipterans (18–20). These toxin proteins, including Cry4Aa, Cry4Ba, Cry10Aa, Cry11Aa, and Cyt1Aa, are toxic to mosquitoes (21–23). Cry toxin’s effectiveness against insect pests is dependent on their interactions with other receptors such as alkaline phosphatase (ALP), aminopeptidase-N (APN), cadherin (CAD), and ATP-binding cassette (ABC) transporters (24–31). For that reason, it is crucial to comprehend the interactions between Cry toxins and other midgut proteins. In addition to Cry toxins, Cyt toxins are important for inducing toxicity in some insect orders (23, 32, 33). For example, the Bt strain LLP29 produces the Cyt1Aa6 toxin, which is toxic to Ae. albopictus and Culex quinquefasciatus (34).
Lectins are a diverse group of ubiquitous carbohydrate-binding proteins found in all organisms that play an important role in self/non-self-immune recognition in insects (35–40). Lectins have a wide range of functional responses in symbiosis, host colonization by microbial pathogens, and host immune responses (41, 42). Genome-wide analyses have shown that C-type lectin (CTL) proteins are more abundant and distinct in invertebrates (43–47). Moreover, lectin proteins bind carbohydrates in the existence of Ca2+ ions via their C-type lectin-like domains (CTLD), containing the highly conserved motifs QPD (Gln-Pro-Asp) and, EPN (Glu-Pro-Asn) which are specific to mannose- and galactose-type carbohydrates (43). The Cry toxin domains II and III and carbohydrate-recognition domains (CRD) of lectins have similar structures (48–50), and because of these structural similarities, it is very important to further functionally investigate and comprehend the role and functional mechanism of lectins in Cry toxicity. Protein-protein interactions among lectin, Cry toxin, and related toxin receptors have been investigated to explore the function of lectin in Bt serovar israelensis (Bti) tolerance (51–54). Lectin binding research also showed the existence of numerous APN isoforms with O-linked carbohydrate structures known to bind with Cry1Ac toxin in Douglas fir tussock moth larvae (55). The lectin-like domain III of Cry toxins also known to involved in the interaction with the peritrophic membrane (PM) by attaching to PM chitin and GalNAc related numerous PM proteins (56–58), which may also contribute to the failure of some toxins to pass through the PM (59, 60). However, understanding the role of lectins in Cry toxicity is important, as it will not only broaden our understanding of the Bt mechanism but also aid in the implementation of new biocontrol strategies.
Ae. aegypti Invasion
Ae. aegypti is an important arthropod vector and model organism in invasion biology. Competition for the same available resources in the ecosystem disrupts and destabilizes the native population (61). nvasion results in the introduction of new diseases or the active spread of local diseases. Mosquitoes are important invaders due to a close relationship with human pathogens (62, 63). Human habitats are the most likely places for mosquitoes to live in and most mosquitoes change territories accordingly (64). Ae. aegypti survive worldwide in tropical and subtropical areas; however, populations vary in their capability (vector capacity) to transmit disease (65–70). Africa is considered the ancestral location of Ae. aegypti, which spread to other parts of the world probably by traveling on ships along trading routes (67, 69). Outside Africa, Ae. aegypti has a robust genetic inclination to enter homes and feed on humans’ blood, as well as the ability to survive and lay eggs in man-made water reservoirs in the human environment (66, 70). However, there is extensive variation in the appearance, ecology, and behavior among sub-Saharan African mosquito populations (6, 10, 71–73). Some populations are less contact with humans, live in forests, feed on other animals, and oviposit in tree holes (66, 67, 69, 70).
Origin of Ae. aegypti
There are two subspecies of Ae. aegypti (69), namely, Ae. aegypti formosus (Aaf) and Ae. aegypti aegypti (Aaa). Almost all populations of the African subspecies Ae. aegypti aegypti are strongly anthropophilic and light in color. However, in Africa, subspecies belonging to the Ae. aegypti formosus live in forests and are darker in color. Previously, the two subspecies were separated by coloration, with Aedes aegypti aegypti having pale scales on the first abdominal tergite (69). However, the populations of West Africa contain pale scales, on the other hand, appeared to be closer genetically to Aedes aegypti formosus populations than to Aedes aegypti aegypti populations from other parts of the tropics (10, 72, 73). Both species coexist in West Africa (Senegal) and East Africa (Kenya). Although they do not coexist in rural areas, they mate freely in urban environments. The combination of different factors, such as low migration, founder effects, and irregular habitats, makes populations more genetically structured (74). In earlier 16th to 18th centuries, trans-Atlantic shipping introduced Ae. aegypti to the recent world and in the late 19th century Ae. aegypti reached Asia (75–77). The mosquito exomes from five different populations of the globe were sequenced and compared them with those of the African populations of Ae. aegypti in West Africa (Senegal) and other regions (Mexico and Sri Lanka) (78).
Ae. aegypti Biology
Generally, plant nectar acts as a basic source of food for mosquitoes, but female mosquitoes require blood prior to laying eggs. Warm-blooded vertebrate host blood is a preferred nutrient source for adult female mosquitoes (79). Humans are the most stable hosts for sucking blood. Nutrients in the larval stages are stored and consumed during egg production (80). During its lifespan, an adult female can lay five batches of eggs, with a single batch containing up to 100-200 eggs. Eggs can resist drought conditions for a few months (81, 82). Most parts of the mosquito life cycle are in the aquatic phase, including the four larval stages and pupal stage. Larvae are fast growing, feeding completely on the water surface. The larval stages last for at least four days. At the end of the fourth instar, the larvae go through a non-feeding stage called the pupal phase, which lasts approximately two days. The lifespan of an adult mosquito changes according to environmental circumstances but generally ranges from two to four weeks (Figure 1) (81, 83, 84).
Global Burden of Mosquito-Borne Diseases
Vector-borne diseases affect two-thirds of the world’s population and cause the death of millions of people annually (66, 85, 86). Ae. aegypti is the main arboviruses vector (87–89). It is mainly linked with the spread of a many viral diseases in humans, including dengue fever, yellow fever, chikungunya and Zika virus disease. However, the world is less affected by yellow fever as a potent vaccine has been developed to control it, although it still exists (90, 91). Dengue viruses (DENVs) are the causal agents of dengue fever, a viral infectious mosquito-borne disease that spreads across the world’s tropics and subtropics (92). There are four DENV serotypes, namely, DENV-1, DENV-2, DENV-3, and DENV-4 (93, 94). Each year, approximately 390 million people worldwide become infected with the dengue virus (95). In 2014, the highest spread of dengue fever occurred in Taiwan with 15,732 reported cases, of which 136 were dengue hemorrhagic fever (96). From 1990 to 2019, the burden of dengue increased as most parts of the world experienced three decades of urbanization, global warming, and an increased population. Southeast Asia and South Asia remain areas of concern, especially as the burden of dengue fever in the Americas is rapidly increasing (97).
In 2007, the Zika virus (ZIKV) was detected in 55 countries in America, Oceania, Asia, and Africa. However, the first epidemic cases were recorded in Brazil in 2015 and approximately 1.5 million people were infected (98). Zika virus disease, which results in microcephaly in newborns, affects brain growth, and leads to the formation of cranial calcifications, is becoming increasingly prevalent in Brazil (99). An outbreak of Zika virus disease in South America, Central America, and the Caribbean was linked to prenatal brain dysfunction (100). The chikungunya virus (CHIKV) belongs to the Alphavirus genus, which is transmitted by both Ae. aegypti and Ae. albopictus, causing chikungunya fever with serious joint pain in infected patients for several years (101). In 1952-1953 the first CHIKV epidemic was reported in Tanzania (East Africa) (101) and considered as a leading reason of concern, causing epidemics in several Indian Ocean islands, Asia, as well as in America and Southern Europe. In 2005-2006, a CHIKV epidemic outbreak occurred in the Indian Ocean and 1.5 million people were infected. In 2010, an epidemic outbreak was reported in India, affecting more than one million people (102). However in 2013, CHIKV spread in the Western world and further spread in the Americas (46 countries) and 1.7 million suspected cases were reported (103). Existing data show that between 2010 and 2019, CHIKV and ZIKV caused average annual losses of more than 106,000 and 44,000 disability-adjusted life years (DALYs), respectively. The burden of these two viruses in the Americas far exceeds that of any other region of the World Health Organization (WHO) (104).
Biocontrol of Mosquitoes Using B. thuringiensis
The discovery of bacteria such as Bti are extremely toxic to Dipteran larvae, has opened the way to their usage as a possible bio-larvicide in mosquito eradication campaigns across the world (22, 105, 106). Bti toxin was initially found to be an excellent biological control agent for mosquito larvae and black flies (107). It can produce different toxins, such as Cry4Aa, Cry4Ba, Cry11Aa, Cyt1Aa, and Cyt2Ba crystal proteins (108, 109). Cry proteins are known to be very toxic against different insect orders, such as Coleopteran, Diptera, Lepidoptera, and Hymenoptera. In contrast, Cyt toxins are usually found in Bt strains that are active against Dipterans, with a few outliers of Cyt proteins that, are active against Coleopteran larvae have been documented (32, 110). However, Cry11Aa exhibited a high toxicity against Ae. aegypti (111). At present, Bti is largely used for mosquito control; therefore, improving the effectiveness of Bti products is a key issue that needs to be solved in the current development of Bti products. Biocontrol product limitations can be improved by enhancing the genetic and physiological mechanisms of biocontrol using a mixture of organisms as biocontrol agents (112, 113).
According to all the known Cry structures, activated Cry toxins have three individual functional domains consisting of α-helical bundles in domain-I, β-prism folds in domain-II, and a sandwich of αβ-sheets in domain-III. Domains I and II function in receptor recognition and membrane pore formation, respectively (114). Cry toxins interact with midgut receptors found in lipid rafts and this phase is necessary for oligomerization and toxin insertion into the membrane (115). Oligomerization is a complicated mechanism that involves toxin contact with receptors and subsequent proteolysis of the α-1 helix (116). Activated toxins bind to a wide range of receptors on midgut epithelial cells. The interaction of Cry toxin with its receptor results in toxin oligomerization and pore formation, eventually leading to cell death (117). Sequential binding of Cry1A toxins has been observed in lepidopteran insects. The binding mechanism may begin with alkaline phosphatase (ALP) and aminopeptidase-N (APN) receptors, followed by cadherin binding. Interaction with the cadherin receptor causes α-1 helix to be cleaved, resulting in the formation of oligomeric toxins (116). In case of Cry11Aa, it was reported that Cyt1Aa induce oligomerization of Cry11Aa resulting in membrane pore formation in Ae. aegypti (118). Cadherin receptor is important for the oligomerization of Cry11Aa but not for Cry4Ba (119).Cry toxins are very toxic to mosquito larvae. By binding to protein receptors on the gut epithelial cell membrane Cry toxins lead to pore formation and cell lysis (27, 120). Midgut proteins present in the brush border of larvae midgut bind to Cry toxins and facilitate events resulting in larval death (121–123). Many receptors have been reported in mosquitoes, including aminopeptidase (APN), alkaline phosphatase (ALP), cadherin (CAD) and ABC transporters, which are midgut receptors of Bti Cry4Ba, Cry11Aa, and Cry11Ba toxin in Ae. aegypti, respectively (30, 124–126).
Three conserved signaling pathways, including the Toll-like receptor pathway, immunodeficiency (IMD) pathway, and other Janus kinase-signal transducer and activator of transcription (JKT) pathways, participate in the mosquito defense mechanism (127, 128). The Toll pathway plays main role in the regulation of natural immunity. It is primarily responsible for the identification and protection of viruses and fungi. The IMD pathway can recognize and immunize gram-positive and -negative bacteria and can control antimicrobial peptides, such as Diptera and Drosophila peptides. Expression of AMP (129, 130) and the JKT pathway play important roles in the process of damage repair and tissue regeneration in the body.
Role of Lectins
Lectins are a class of multivalent proteins that specifically bind glycoproteins and are widely distributed in plants, animals, and microorganisms (35, 37). Lectins play important roles in cell signaling and photosynthesis, and many diverse lectin roles have been studied in the model plant Arabidopsis thaliana (131). Recently, plant lectins have been used in agricultural improvement, biomedical research, and glycobiology (132). In animals, they function as weapons to kill pathogens through aggregation and opsonization, and are present in all vertebrates and invertebrates (133, 134). C-type mannose-binding lectin (MBL) plays a key role in the immune system of vertebrates, and its deficit increases the chances of more infectious diseases to attack (41). The MBL in chickens can be activated when they are exposed to chicken diseases (135). Lectins are effective for invertebrate and vertebrate cancerous cells, prompting biochemists to use them in histochemical and cytochemical research (136, 137) as well as in human medicine (138)
Role of Lectins in Insects
Insects are a very abundant and miscellaneous phylum in the kingdom Animalia. They rely entirely on their innate immune system to prevent themselves from external environmental pathogens (42, 139, 140). When a harmful germ invades an insect body, it is recognized by a group of proteins recognized as pattern recognition receptors (PRRs). These PRRs can detect pathogens via the pathogen-associated molecular patterns (PAMPs) present on the pathogen surface (46). Invertebrates have seven groups of PRRs, namely, galactose-binding lectins (galectins), multi-domain scavenger receptors (SCRs), peptidoglycan recognition proteins (PGRPs), fibrinogen-related proteins (FREPs), gram-negative binding proteins (GNBPs), thioester-containing proteins (TEPs), and CTLs. More recently, Toll-like receptors and the mammalian Toll receptor family have been found to be more conserved and to function in innate immunity. Bombyx mori Toll9 acts as a PRR for lipopolysaccharide binding and Toll9 is more similar to the mammalian TLR4–MD-2–LPS pathway (141).
CTLs are a large family of proteins that are recognized by CTLDs and further classified into 17 different subgroups on the basis of structural domain and phylogeny (44). They bind carbohydrates in the presence of Ca2+ ions via their CTLD, containing the highly conserved motifs EPN (Glu-Pro-Asn) and QPD (Gln-Pro-Asp), which are specific to mannose-and galactose-type carbohydrates (43, 142). CTLs exhibit a wide range of functional responses in symbiosis, host colonization by infectious pathogens, and host immune responses (36, 41). Invertebrate CTLs have been shown to mediate immune responses and development (143, 144). Innate immunity is based on the secretion of different lectins that possess different functions, including nodule formation, Escherichia coli clearance, hemagglutination, encapsulation, melanization, the prophenoloxidase cascade, and phagocytosis (145, 146).
The novel CTLs TcCTL5 and TcCTL6 in the Coleopteran beetle (Tribolium castaneum) functioned against bacterial infection, whereas their silencing showed a significant decrease in four antimicrobial peptides (147, 148). A CTL in Plutella xylostella, PxIML, play a key role in the recognition of pathogen and the subsequent humoral and cellular immunity of the species (39). Similarly, the Mud Crab (Scylla paramamosain) CTL SpCTL6 plays an immune-protective role, and its expression level is significantly increased during the larval stages and after molting (149). A genome-wide comparative analysis of CTLs in seven insect species (Spodoptera litura, Helicoverpa armigera, Manduca sexta, B. mori, Drosophila melanogaster, Tribolium castaneum, and Ae. mellifera), showed interesting results. They observed that CTL-S1–S8 and CTL-X1–X4 ortholog groups were well conserved in seven species, whereas the CTL-X5 double CRD domain group, the three-CRD CTL-S11 group, the C-terminal long CTL-S9 group, and the CTL-Lepidopteran-specific S10 group were found to be not conserved (150). Furthermore, the CTL BrCTL10 induces multiple immune responses in silkworms (B. mori) (40). In addition, BmLBP in B. mori facilitates the clearance of E. coli (151). Most importantly, these insect CTLs can recognize dead cells as well as cancerous cells in invertebrates (152, 153). A total of 35 CTL genes were identified in the Oriental Armyworm (Mythimna separate) with a single and double CRD domain that roles in innate immune responses (154). M. sexta immulectins enable melanization and cellular encapsulation (155, 156). Furthermore, HaCTL3, a CTL gene in the cotton bollworm (H. armigera) plays a key role in development and larval growth (157).
Role of Lectins in Mosquitoes
The mosquito’s gut is responsible for pathogen entry and replication. The gut contains microbiome that interact with midgut cells and are essential for vector physiology (158, 159). Previous studies reported that the gut microbiome plays a vital role in vector competence (158, 160–162). CTLs in gut ecology play a vital role in immune activation and may serve as intervention targets for the control of vector-borne diseases in nature (36, 163). Ae. aegypti mosGCTL-3 regulates germline development and affects fertility, whereas knockout of mosGCTL-3 revealed a decrease in the number of gut microbiota, and GCTL-3 mutants showed a decrease in the dengue virus-2 infection rate (164). Modification of the mosquito’s immune system through expression of the human CTL CLEC18A gene can drastically reduce dengue virus infection. Transgenic mosquitoes showed significant differences in the midgut microbiota (165). Mosquito galectin, mosGCTL-1, interacts with the West Nile virus (WNV) and promotes mosquito infection (166) while mosGCTL-7 interacts with the Japanese encephalitis virus (JEV) in Ae. aegypti and facilitates virus entry (167).
The mosquito genomes of Ae. aegypti and Anopheles gambiae, and those of D. melanogaster and M. sexta, contain 39, 25, and 34 CTL genes, respectively (45, 127, 168, 169), whereas 183 CTL genes have been reported in Caenorhabditis elegans (168). Mosquito, shrimp, and Drosophila CTLs help these species defend themselves against bacterial infections. It has also been reported that silencing of CLTs causes rapid bacterial growth in cases of infection, which ultimately results in a short lifespan (170, 171). Furthermore, it has been described that mosquito CTLs play functions in the maintenance of homeostasis of the gut microbiome (36). CLTs play significant role in the activation of the melanization cascade in Ae. aegypti (172). Moreover, the CRDs of lectins and the Bti Cry toxin domains II and III adopt similar structures (48–50, 173). The tertiary structures of different Bti Cry toxins have determined through X-ray crystallography (27) (Figure 2). All of these structures are very similar to the three-domain organization, suggesting that all proteins in the Cry three-domain family share a similar mode of action. The N-terminal domain (domain I) consists of seven α helices, the central -α5 helix is hydrophobic and surrounded by six additional amphipathic helices; the helical domain is necessary for membrane insertion and pore formation. Domain II is made up of three anti-parallel β-sheets with exposed loop sections, while domain III is made up of a β-sandwich (174, 175). In domains II and III, exposed regions are required for receptor binding (27). Domain II shares structural resemblances with various carbohydrate-binding proteins, including lectin jacalin, lectin Mpa and vitelline (59, 176–179); Domain III is structurally identical to other carbohydrate-binding proteins like the cellulose binding domain of 1,4-β-glucanase C, β-glucoronidase, β-galactosidase, galactose oxidase, sialidase, and xylanase U (180). Because of these similarities, carbohydrate moieties may play a substantial part in the mechanism of three-domain Cry toxins.
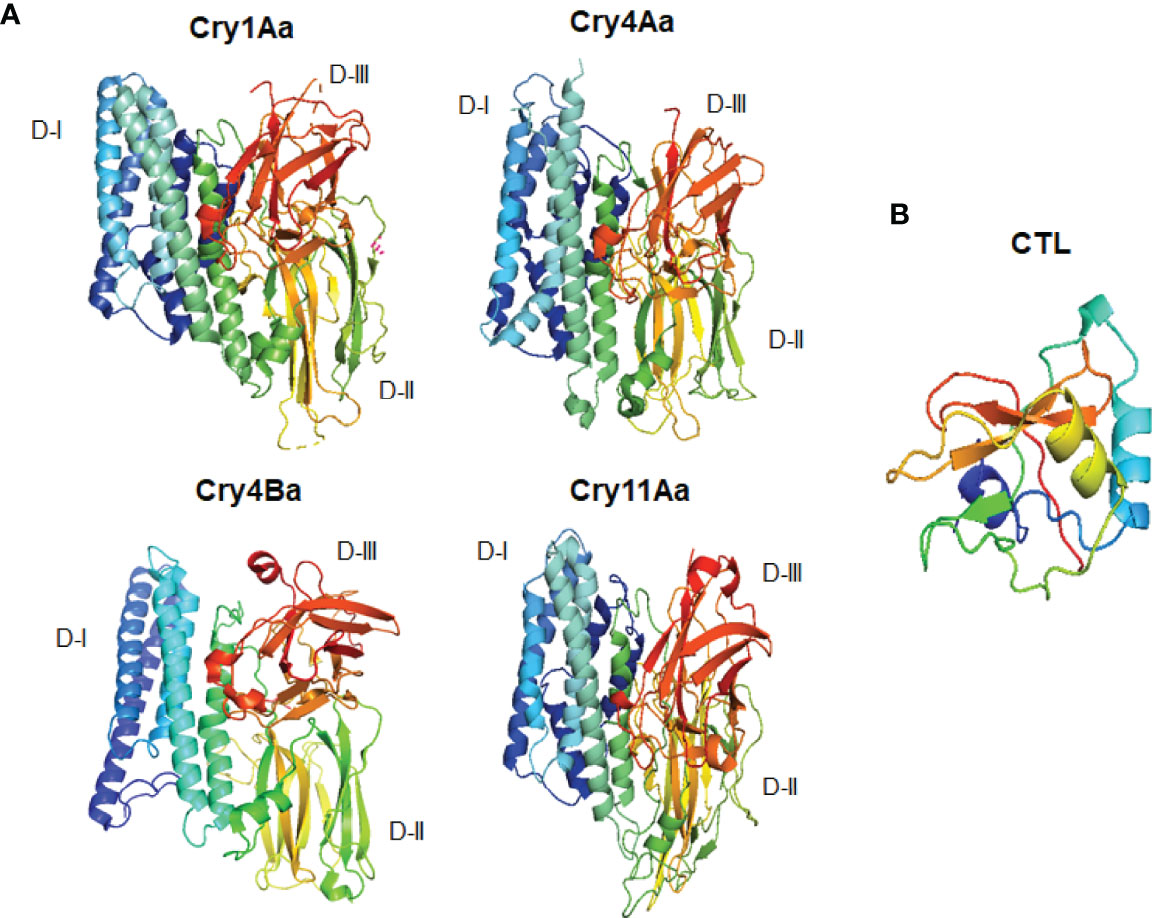
Figure 2 Three dimensional structural comparisons between different Cry toxins and CTL domain. (A) Cry1Aa (PDB: D6J4), Cry11Aa (PDB: 1DLC), Cry4Aa (PDB: 2C9K), Cry4Ba (PDB: 1W99); (B) CTL domain (PDB: 5E4L).
Due to various structural similarities, it is very important to further understand the function and molecular mechanism of mosquito lectin in Cry toxicity, protein-protein interactions among lectin, Cry toxin, and other important receptors (51, 52, 54, 125, 173).
Role of Lectins in the Ae. aegypti Response Against Cry Toxin
Cry toxin tolerance, especially Cry1A, has been extensively studied in Lepidoptera such as B. mori. Cry1A toxicity is altered in the presence of the midgut protein P252 and has antimicrobial activity against Bt, E. coli, and Serratia marcescens (181). These midgut membrane proteins also show low toxicity of Cry1Ac in H. armigera (182). In other Lepidopteran larvae, like Lymantria monacha, Thaumetopoea pityocampa, Heliothis virescens, M. sexta, and Spodoptera exigua, decrease Cry toxicity in late instars is associated with a decreased number of available binding sites (25, 183–186). Weaker interaction of Cry1A toxins was identified among the apical brush border of the midgut epithelium of Orgyia pseudotsugata and Cry1A toxins due to the presence of toxin-binding glycoproteins in the larval midgut (55). In M. sexta, Cry1Ac binding to the APN receptor is inhibited by the presence of N-acetylgalactosamine (GalNAc) on the receptor and decreases Cry toxicity. The Cry-domain III folds are involved in receptor recognition of carbohydrates, and GalNAc binds to Cry1Ac domain III positions and plays a competitive role like the lectin domain (56, 187).
Cry toxins bind to putative receptors, including ALP, APN, and CAD in the midgut epithelium of Ae. aegypti. ALP contains at least two Cry11Aa binding sites, such as residues R59-G102 interacting with loop α-8 from Cry11Aa domain II, and residues N257-I296 interacting with domain III of Cry11Aa (26, 124). The full-length AaeAPN2 region, including amino acids 569–641, has the highest binding activity to the Cry11Aa toxin and efficiently competes with the toxin binding to Aedes BBMV (54). The cadherin fragment, which contains CR7–11 (cadherin repeats 7–11) binds to Cry11Aa, primarily through loop α8 of domain II toxin, while Loop-3 of Cry11Aa binds to CR11 (cadherin repeats) of Ae. aegypti (51). Midgut proteins play an important role in this toxicity mechanism and alter the binding activity with receptors and Cry toxins. Previously, we identified highly expressed C - and G-type lectins in the Ae. aegypti midgut after treatment with the Bt LLP29 toxin (176). These midgut CTLs and galectins have been reported to inhibit Cry11Aa toxicity in Ae. aegypti by competing with Cry11Aa for binding to ALP and APN receptors (176–178) (Figure 3), but no evidence of binding competition was found in the case of CAD (177). Further silencing of these midgut proteins results in enhanced toxicity of Cry toxins (177). Moreover, the three-dimensional protein structures of the putative receptors ALP, APN, CAD, Cry11Aa toxin, and CTL were modeled in previously reported study (177–179) (Figures 4A, B). Molecular docking of ALP, APN, and CAD with both Cry11Aa and CTL showed that all receptors were docked to the CTL and Cry11Aa (178), and the residues (yellow colored) were the binding sites of the two proteins (Figures 5A–F). Even when these two proteins docked together with ALP and APN receptors, overlapping binding sites were found where residues in Cry11Aa and CTL were competing to bind with receptors (overlapping sites colored in yellow) (177, 178). Residues in red are the CTL binding sites, while the green smudge regions are the Cry11Aa binding sites in the ALP and APN receptors (Figures 6A, B) (178). However, no overlapping sites were found when CTLs and Cry11Aa were docked with the CAD receptor (179) (Figure 6C). It was suggested that these important proteins could sequester the toxin and interfere with the insecticidal process. Furthermore, the fact that these proteins are immune-related may suggest that Cry toxins may alter may alter insect’s immune responses. Such compounds or chemicals should be introduced to counteract the effect of CTLs in the midgut and improve the toxicity mechanism. These interesting ideas warrant future studies.
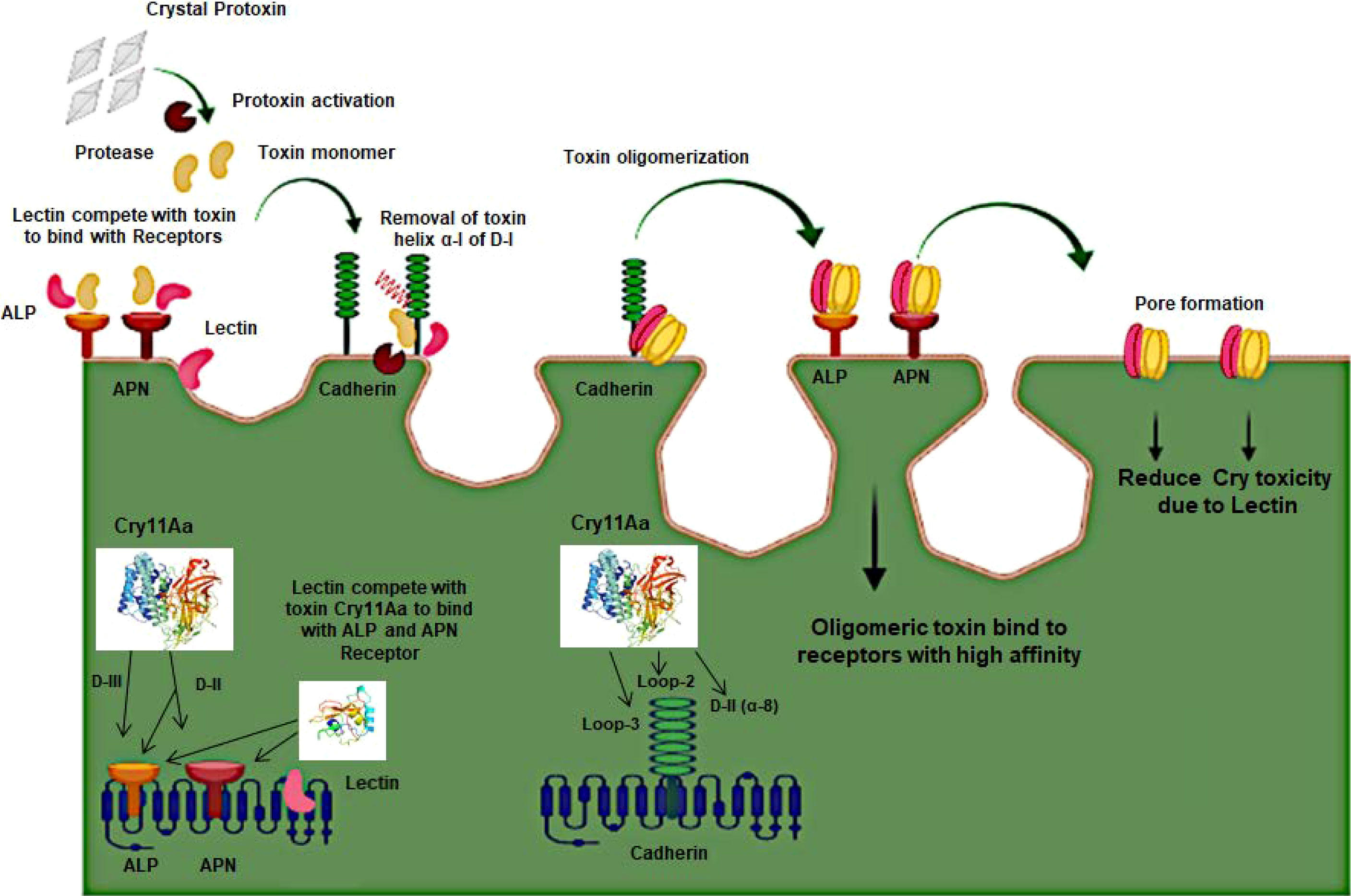
Figure 3 Schematic presentation of the 3D-Cry toxin mechanism with Receptors and Lectin in Mosquito.
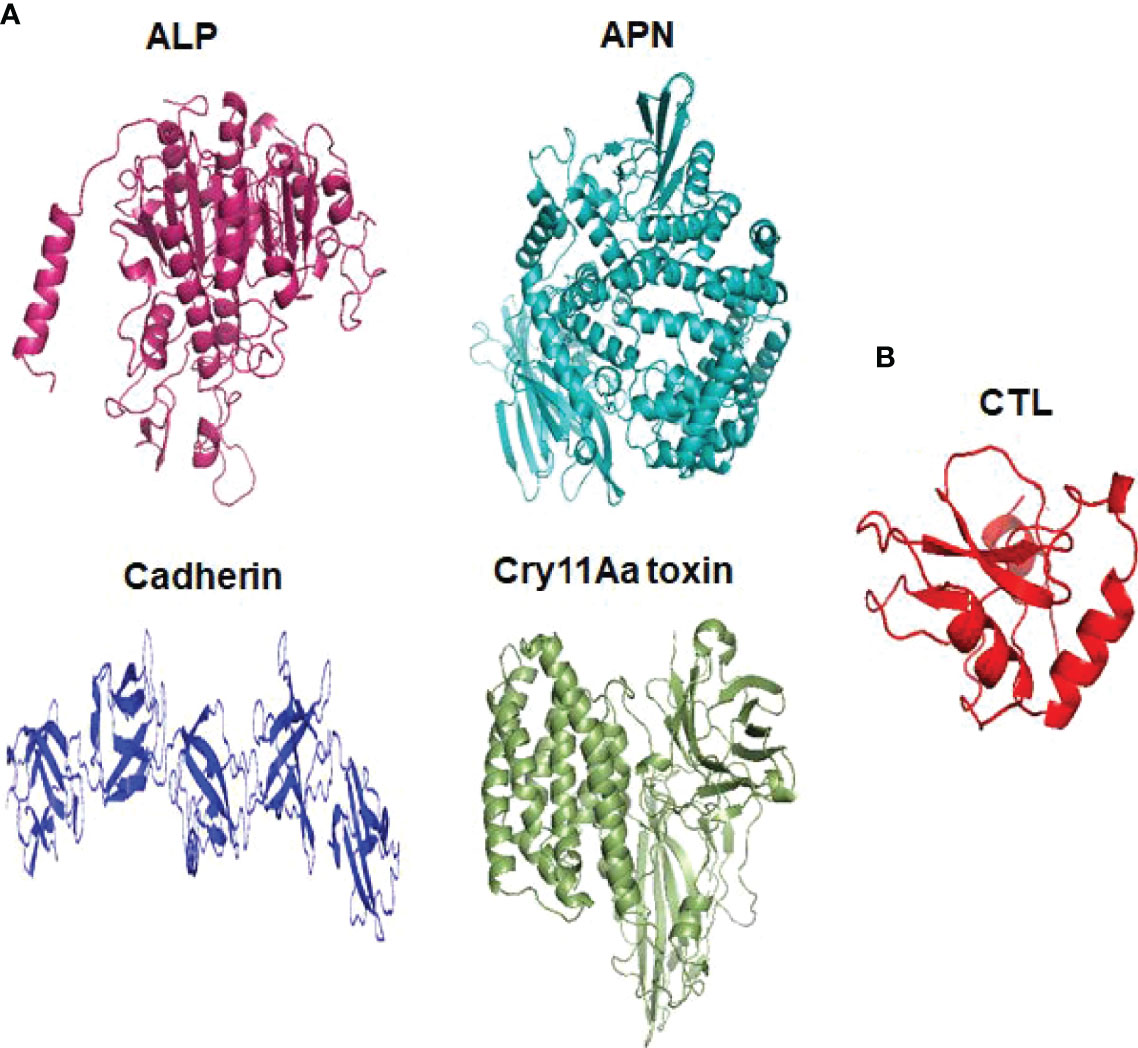
Figure 4 Three dimensional structural presentation of putative receptors, toxin and CTL domain in Ae. aegypti. (A) ALP (PDB: IK7H), APN (PDB: 4WZ9), Cadherin (PDB: 4UX8), Cry11Aa toxin (PDB: 1DLC) and (B) CTL domain (PDB: 5E4L).
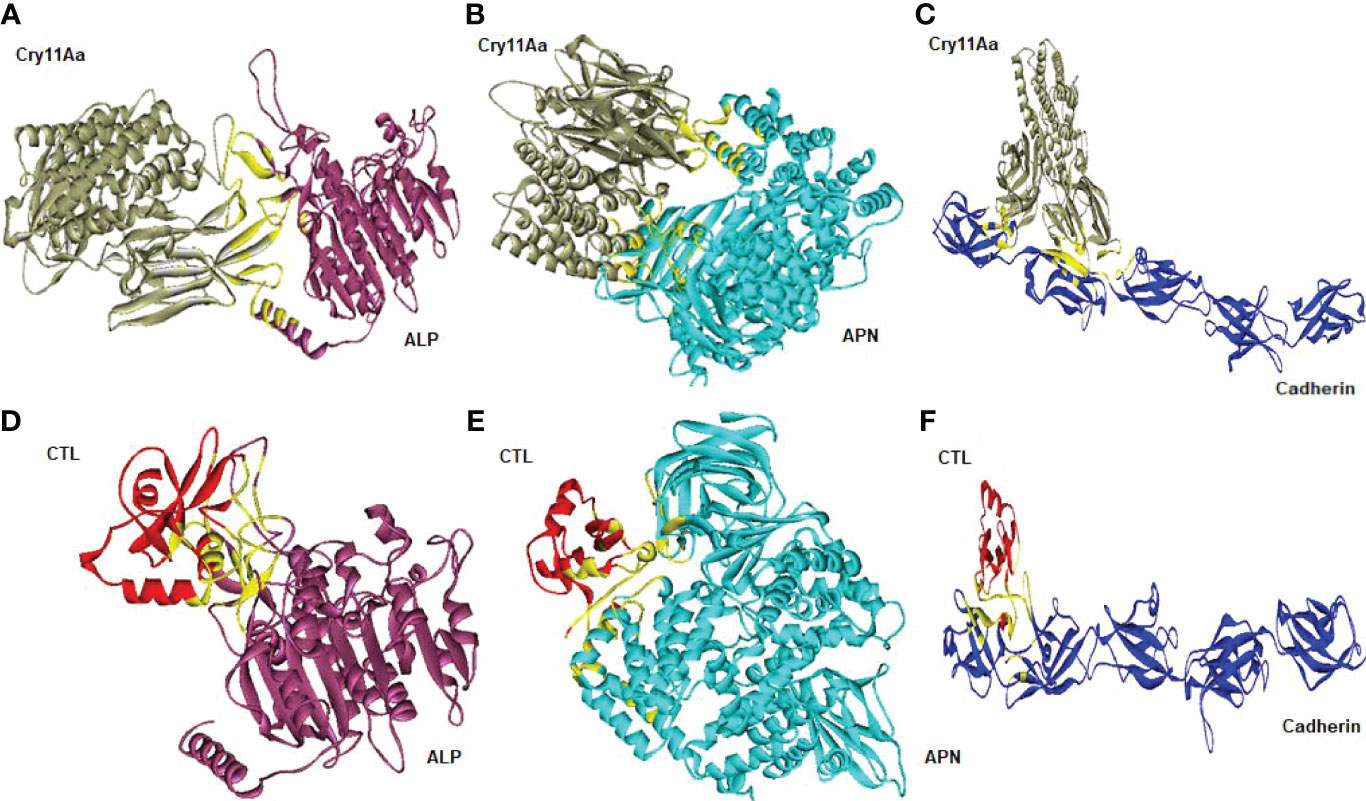
Figure 5 Molecular docking representation of receptors with Cry11Aa and CTL proteins. Cry11Aa binding with (A) ALP, (B) APN, and (C) Cadherin receptors. CTL binding with (D) ALP, (E) APN, and (F) Cadherin receptors. Yellow color showed the binding sites of two proteins.
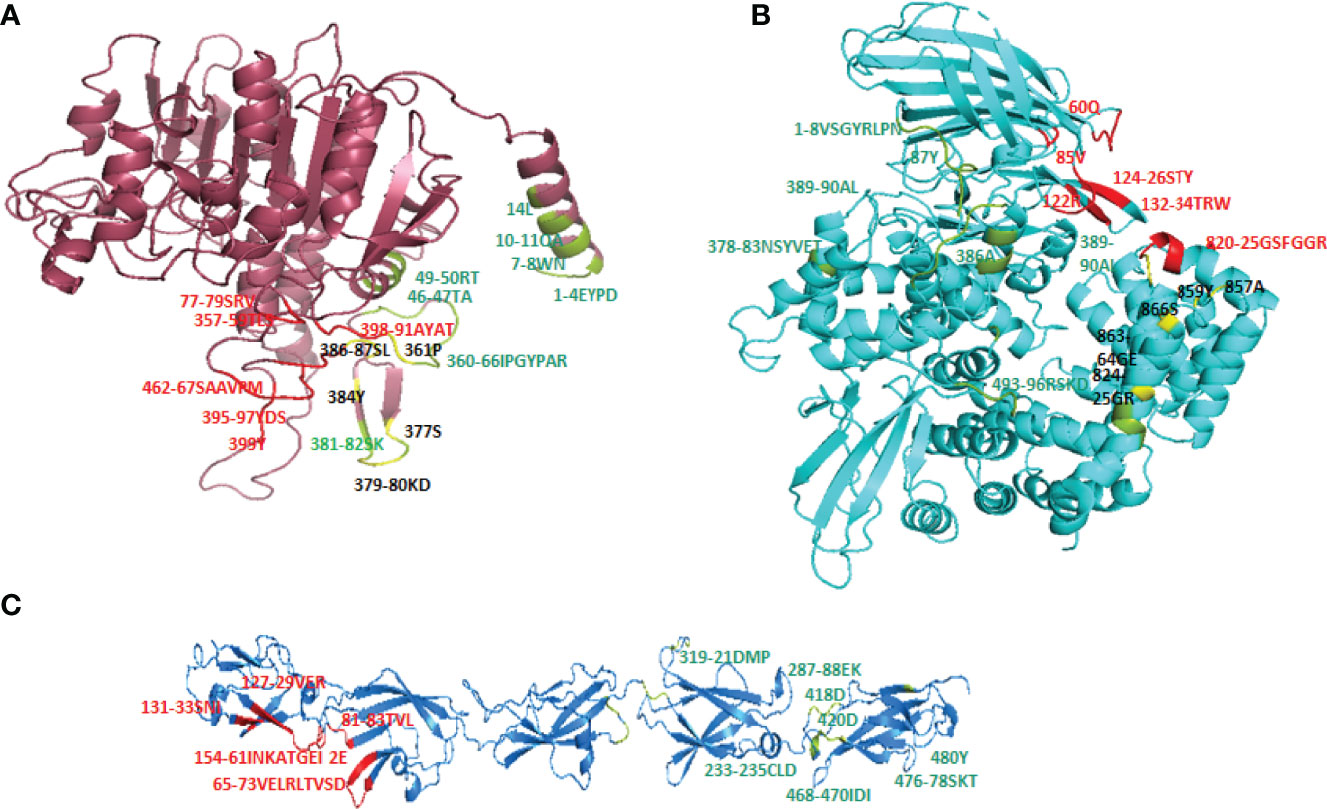
Figure 6 Overlapping binding sites in receptors interface. When both CTL and Cry11Aa proteins docked together in ALP, APN and Cadherin receptors overlapping binding sites (colored in yellow) were found in (A) ALP and (B) APN but none of residue in Cadherin receptor (C) found to be overlapped. Red colored residues are CTL binding sites while Cry11Aa binding sites are colored in green smudge.
Concluding Remarks and Future Perspectives
To date, many researchers have focused on the identification of different lectins and their further characterization in different organisms. Lectins have also been well studied in higher organisms, such as plants and animals, but limited literature is available on insects. Lectins play a crucial role in the innate immunity of insects. Both invertebrate and vertebrate CTLs contain specific CRDs. Nevertheless, research into the mechanisms and actions of insect CTLs in innate immunity will contribute to the protection of beneficial insects as well as the biological control of harmful vectors. Therefore, it is important to study the role of lectins in mosquitoes, especially in Ae. aegypti. Thus, if the major interaction among toxins and their receptors is reduced or eliminated, the toxicity of Bt will be greatly altered. Midgut protein engineering may also a considerable way to improve Cry toxicity. The expression of Ae. aegypti galection-14 was knocked down which resulted in increasing Cry toxicity (177). Still, the molecular studies in this domain are limited and need more experimental evidence in mosquitoes and other species. On another side, many reports published showed improving Cry toxins activity against mosquitoes and insects by using recombinant Cry toxins (188). Several reported studies have shown that midgut proteins may influence Cry toxin activity and have been studied in many other insect species, including P. xylostella (193), Trichoplusia ni (194), Leptinotarsa decemlineata (195), Cnaphalocrocis medinalis (196), Achaea janata (197), and the insect family Noctuidae (198). Therefore, the detection and identification of important midgut proteins that may interfere with this critical step may open a new avenue of research to fully understand the Bt mechanism and give a theoretical foundation for the development of new bioinsecticides for mosquito control.
Author Contributions
The review of literature, and manuscript writing were accomplished by IA and KB. WT, AI, XG and LZ revised the manuscript. XG and LZ provided technical support and vigorous guidance, and founded the research project. The authors read and approved the final manuscript.
Funding
This work was funded by the National Program of China (Grant Numbers 2017YFE0121700 and 2017YFE0122000); the United Fujian Provincial Health and Education Project for Tackling Key Research (Grant No. 2019-WJ-29); Natural Science Foundation of Fujian Province (Grant No. 2020J01550 and 2020I0031); the Special Fund for Scientific and Technological Innovation of Fujian Agriculture and Forestry University (KFA20124A).
Conflict of Interest
The authors declare that the research was conducted in the absence of any commercial or financial relationships that could be construed as a potential conflict of interest.
Publisher’s Note
All claims expressed in this article are solely those of the authors and do not necessarily represent those of their affiliated organizations, or those of the publisher, the editors and the reviewers. Any product that may be evaluated in this article, or claim that may be made by its manufacturer, is not guaranteed or endorsed by the publisher.
References
1. De Almeida JPP, Aguiar ERGR, Armache JN, Olmo RP, Marques JT. The Virome of Vector Mosquitoes. Curr Opin Virol (2021) 9:7–12. doi: 10.1016/j.coviro.2021.04.002
2. Sabir MJ, Al-Saud NBS, Hassan SM. Dengue and Human Health: A Global Scenario of Its Occurrence, Diagnosis and Therapeutics. Saudi J Biol Sci (2021) 28(9):5074–80. doi: 10.1016/j.sjbs.2021.05.023
3. Chouin-Carneiro T, Vega-Rua A, Vazeille M, Yebakima A, Girod R, Goindin D, et al. Differential Susceptibilities of Aedes Aegypti and Aedes Albopictus From the Americas to Zika Virus. PloS Negl Trop Dis (2016) 10(3):e0004543. doi: 10.1371/journal.pntd.0004543
4. Vorou R. Zika Virus, Vectors, Reservoirs, Amplifying Hosts, and Their Potential to Spread Worldwide: What We Know and What We Should Investigate Urgently. Int J Infect Dis (2016) 48:85–90. doi: 10.1016/j.ijid.2016.05.014
5. Gloria-Soria A, Payne AF, Bialosuknia SM, Stout J, Mathias N, Eastwood G, et al. Vector Competence of Aedes Albopictus Populations From the Northeastern United States for Chikungunya, Dengue, and Zika Viruses. Am J Trop Med (2021) 104(3):1123–30. doi: 10.4269/ajtmh.20-0874
6. Quimbayo M, Rúa-Uribe G, Parra-Henao G, Torres C. Evaluation of Lethal Ovitraps as a Strategy for Aedes Aegypti Control. Biomédica (2014) 34:473–82. doi: 10.7705/biomedica.v34i3.2146
7. Ritchie SA, Devine GJ, Vazquez-Prokopec GM, Lenhart AE, Manrique-Saide P, Scott TW. Insecticide-Based Approaches for Dengue Vector Control. In: Ecology and Control of Vector-Borne Diseases. Wageningen, Netherland: Wageningen Academic Publishers (2021). p. 59–89. doi: 10.3920/978-90-8686-895-7_4
8. Kefi M, Charamis J, Balabanidou V, Ioannidis P, Ranson H, Ingham VA, et al. Transcriptomic Analysis of Resistance and Short-Term Induction Response to Pyrethroids, in Anopheles Coluzzii Legs. J BMC Genomics (2021) 22:891. doi: 10.1186/s12864-021-08205-w
9. Pisa L, Goulson D, Yang E-C, Gibbons D, Sánchez-Bayo F, Mitchell E, et al. An Update of the Worldwide Integrated Assessment (WIA) on Systemic Insecticides. Part 2: Impacts on Organisms and Ecosystems. Environ Sci Pollut Res (2021) 28:11749–97. doi: 10.1007/s11356-017-0341-3
10. Braga IA, Valle D. Aedes Aegypti: Surveillance, Resistance Monitoring and Control Alternatives in Brazil. Epidemiol Health Serv (2007) 16:295–302. doi: 10.5123/S1679-49742007000400007
11. Guo S, Li X, He P, Ho H, Wu Y, He Y. Whole-Genome Sequencing of Bacillus Subtilis XF-1 Reveals Mechanisms for Biological Control and Multiple Beneficial Properties in Plants. J Ind Microbiol Biotechnol (2015) 42(6):925–37. doi: 10.1007/s10295-015-1612-y
12. Doloi D. A Study on Certain Biological Control Methods to Control and Manage Vector-Borne Diseases. Int J Mosq Res (2021) 8(1):31–4. doi: 10.22271/23487941.2021.v8.i1a.497
13. Lees R, Carvalho D, Bouyer J. Potential Impact of Integrating the Sterile Insect Technique Into the Fight Against Disease-Transmitting Mosquitoes. In: Sterile Insect Technique. Boca Raton, Florida: CRC Press (2021). p. 1081–118. doi: 10.1201/9781003035572-33
14. Carvalho KDS, Guedes DRD, Crespo MM, de Melo-Santos MAV, Silva-Filha MHNL. Aedes Aegypti Continuously Exposed to Bacillus Thuringiensis Svar. Israelensis Does Not Exhibit Changes in Life Traits But Displays Increased Susceptibility for Zika Virus. Parasites Vectors (2021) 14:379. doi: 10.1186/s13071-021-04880-6
15. Davis J, Bibbs CS, Müller GC, Xue R-D. Evaluation of Bacillus Thuringiensis Israelensis as Toxic Sugar Bait Against Adult Aedes Aegypti, Aedes Albopictus, and Culex Quinquefasciatus Mosquitoes. J Vector Ecol (2021) 46(1):30–3. doi: 10.52707/1081-1710-46.1.30
16. Nair K, Al-Thani R, Jaoua S. Bacillus Thuringiensis Strain QBT220 Pbtoxis Plasmid Structural Instability Enhances δ-Endotoxins Synthesis and Bioinsecticidal Activity. Ecotoxicol Environ Saf (2021) 228:112975. doi: 10.1016/j.ecoenv.2021.112975
17. Palma L, Muñoz D, Berry C, Murillo J, Caballero P. Bacillus Thuringiensis Toxins: An Overview of Their Biocidal Activity. Toxins (2014) 6(12):3296–325. doi: 10.3390/toxins6123296
18. Van Frankenhuyzen K. Insecticidal Activity of Bacillus Thuringiensis Crystal Proteins. J Invertebr Pathol (2009) 101(1):1–16. doi: 10.1016/j.jip.2009.02.009
19. Van Frankenhuyzen K. Cross-Order and Cross-Phylum Activity of Bacillus Thuringiensis Pesticidal Proteins. J Invertebr Pathol (2013) 114:76–85. doi: 10.1016/j.jip.2013.05.010
20. Rao P, Goswami D, Rawal R. Cry Toxins of Bacillus Thuringiensis: A Glimpse Into the Pandora’s Box for the Strategic Control of Vector Borne Diseases. Environ Sustain (2021) 4:23–37. doi: 10.1007/s42398-020-00151-9
21. Lee SB, Chen J, Aimanova KG, Gill SS. Aedes Cadherin Mediates the In Vivo Toxicity of the Cry11Aa Toxin to Aedes Aegypti. Peptides (2015) 68:140–7. doi: 10.1016/j.peptides.2014.07.015
22. Silva-Filha MHNL, Romão TP, Rezende TMT, Carvalho KDS, de Menezes HSG, Alexandre do Nascimento N, et al. Bacterial Toxins Active Against Mosquitoes: Mode of Action and Resistance. Toxins (2021) 13(8):523. doi: 10.3390/toxins13080523
23. Wu J, Wei L, He J, Fu K, Li X, Jia L, et al. Characterization of a Novel Bacillus Thuringiensis Toxin Active Against Aedes Aegypti Larvae. Acta Trop (2021) 223:106088. doi: 10.1016/j.actatropica.2021.106088
24. Knight PJ, Knowles BH, Ellar DJ. Molecular Cloning of an Insect Aminopeptidase N That Serves as a Receptor for Bacillus Thuringiensis CryIA (C) Toxin. J Biol Chem (1995) 270:17765–70. doi: 10.1074/jbc.270.30.17765
25. Jurat-Fuentes JL, Gahan LJ, Gould FL, Heckel DG, Adang MJ. The HevCaLP Protein Mediates Binding Specificity of the Cry1A Class of Bacillus Thuringiensis Toxins in Heliothis virescens. Biochem (2004) 43(44):14299–305. doi: 10.1021/bi048500i
26. Fernandez LE, Aimanova KG, Gill SS, Bravo A, Soberón M. A GPI-Anchored Alkaline Phosphatase Is a Functional Midgut Receptor of Cry11Aa Toxin in Aedes Aegypti Larvae. Biochem J (2006) 394(1):77–84. doi: 10.1042/BJ20051517
27. Bravo A, Gill SS, Soberon M. Mode of Action of Bacillus Thuringiensis Cry and Cyt Toxins and Their Potential for Insect Control. Toxicon (2007) 49(4):423–35. doi: 10.1016/j.toxicon.2006.11.022
28. Gahan LJ, Pauchet Y, Vogel H, Heckel DG. An ABC Transporter Mutation Is Correlated With Insect Resistance to Bacillus Thuringiensis Cry1Ac Toxin. PloS Genet (2010) 6(12):e1001248. doi: 10.1371/journal.pgen.1001248
29. Park Y, González-Martínez RM, Navarro-Cerrillo G, Chakroun M, Kim Y, Ziarsolo P, et al. ABCC Transporters Mediate Insect Resistance to Multiple Bt Toxins Revealed by Bulk Segregant Analysis. BMC Biol (2014) 12:46–6. doi: 10.1186/1741-7007-12-46
30. Chen J, Aimanova KG, Gill SS. Aedes Cadherin Receptor That Mediates Bacillus Thuringiensis Cry11A Toxicity Is Essential for Mosquito Development. PloS Negl Trop Dis (2020) 14(2):e0007948. doi: 10.1371/journal.pntd.0007948
31. Wang J, Yang X, He H, Chen J, Liu Y, Huang W, et al. Knockout of Two Cry-Binding Aminopeptidase N Isoforms Does Not Change Susceptibility of Aedes Aegypti Larvae to Bacillus Thuringiensis Subsp. Israelensis Cry4Ba and Cry11Aa Toxins. Insects (2021) 12(3):223. doi: 10.3390/insects12030223
32. Federici BA, Bauer LS. Cyt1Aa Protein of Bacillus thuringiensisIs Toxic to the Cottonwood Leaf Beetle, Chrysomela Scripta, and Suppresses High Levels of Resistance to Cry3Aa. Appl Environ Microbiol (1998) 64(11):4368–71. doi: 10.1128/AEM.64.11.4368-4371.1998
33. Sieiro C, Pichardo-Gallardo Á., Areal-Hermida L, Almuiña-González R, Villa TG. Parasporal Crystal Toxins in Bacillus Thuringiensis. In: Developmental Biology in Prokaryotes and Lower Eukaryotes. Springer Nature Switzerland AG: Springer (2021). p. 125–48. doi: 10.1007/978-3-030-77595-7_4
34. Zhang L, Tang B, Huang E, Huang Z, Liu Z, Huang T, et al. Different Toxicity of the Novel Bacillus Thuringiensis (Bacillales: Bacillaceae) Strain LLP29 Against Aedes Albopictus and Culex Quinquefasciatus (Diptera: Culicidae). J Econ Entomol (2013) 106:1098–102. doi: 10.1603/EC12308
35. Mcconnell MT, Lisgarten DR, Byrne LJ, Harvey SC, Bertolo E. Winter Aconite (Eranthis Hyemalis) Lectin as a Cytotoxic Effector in the Lifecycle of Caenorhabditis Elegans. PeerJ (2015) 3:e1206. doi: 10.7717/peerj.1206
36. Pang X, Xiao X, Liu Y, Zhang R, Liu J, Liu Q, et al. Mosquito C-Type Lectins Maintain Gut Microbiome Homeostasis. Nat Microbiol (2016) 1:1–11. doi: 10.1038/nmicrobiol.2016.23
37. Fiorentino MA, Paolicchi FA, Campero CM, Barbeito CG. Lectin Binding Patterns and Immunohistochemical Antigen Detection in Placenta and Lungs of Brucella Abortus-Bovine Infected Fetuses. Open Vet J (2018) 8(1):57–63. doi: 10.4314/ovj.v8i1.10
38. Dorsch MA, De Yaniz MG, Fiorani F, Hecker YP, Odeon AC, Morrell EL, et al. A Descriptive Study of Lectin Histochemistry of the Placenta in Cattle Following Inoculation of Neospora Caninum. J Comp Pathol (2019) 166:45–53. doi: 10.1016/j.jcpa.2018.10.172
39. Li J-Y, Lin J-H, Fernandez-Grandon GM, Zhang J-Y, You M-S, Xia X-F. Functional Identification of C-Type Lectin in the Diamondback Moth, Plutella Xylostella (L.) Innate Immunity. J Integr Agric (2021) 20(12):3240–55. doi: 10.1016/S2095-3119(21)63650-X
40. Liu F-F, Liu Z, Li H, Zhang W-T, Wang Q, Zhang B-X, et al. CTL10 has Multiple Functions in the Innate Immune Responses of the Silkworm, Bombyx Mori. Dev Comp Immunol (2021) 127:104309. doi: 10.1016/j.dci.2021.104309
41. Ahmed H. Animal Lectins: A Functional View. Boca Raton, Florida: Crc Press (2008). doi: 10.1201/9781420006971
42. Eleftherianos I, Zhang W, Heryanto C, Mohamed A, Contreras G, Tettamanti G, et al. Diversity of Insect Antimicrobial Peptides and Proteins-A Functional Perspective: A Review. Int J Biol Macromol (2021) 191:277–87. doi: 10.1016/j.ijbiomac.2021.09.082
43. Weis WI, Taylor ME, Drickamer K. The C-Type Lectin Superfamily in the Immune System. Immunol Rev (1998) 163:19–34. doi: 10.1111/j.1600-065X.1998.tb01185.x
44. Zelensky AN, Gready JE. The C-Type Lectin-Like Domain Superfamily. FEBS J (2005) 272:6179–217. doi: 10.1111/j.1742-4658.2005.05031.x
45. Waterhouse RM, Kriventseva EV, Meister S, Xi Z, Alvarez KS, Bartholomay LC, et al. Evolutionary Dynamics of Immune-Related Genes and Pathways in Disease-Vector Mosquitoes. science (2007) 316:1738–43. doi: 10.1126/science.1139862
46. Zhang X, He Y, Cao X, Gunaratna RT, Chen Y-R, Blissard G, et al. Phylogenetic Analysis and Expression Profiling of the Pattern Recognition Receptors: Insights Into Molecular Recognition of Invading Pathogens in Manduca Sexta. Insect Biochem Mol Biol (2015) 62:38–50. doi: 10.1016/j.ibmb.2015.02.001
47. Chen H, Cai X, Qiu H, Fang J, Wu X. A Novel C-Type Lectin From Crassostrea Gigas Involved in the Innate Defense Against Vibrio Alginolyticus. Biochem Biophys Res Commun (2021) 566:155–63. doi: 10.1016/j.bbrc.2021.05.092
48. Bourne Y, Roig-Zamboni V, Barre A, Peumans WJ, Astoul CH, Van Damme EJ, et al. The Crystal Structure of the Calystegia Sepium Agglutinin Reveals a Novel Quaternary Arrangement of Lectin Subunits With a β-Prism Fold. J Biol Chem (2004) 279(1):527–33. doi: 10.1074/jbc.M308218200
49. Meagher JL, Winter HC, Ezell P, Goldstein IJ, Stuckey JA. Crystal Structure of Banana Lectin Reveals a Novel Second Sugar Binding Site. Glycobiology (2005) 15:1033–42. doi: 10.1093/glycob/cwi088
50. Lee S-B, Aimanova KG, Gill SS. Alkaline Phosphatases and Aminopeptidases Are Altered in a Cry11Aa Resistant Strain of Aedes Aegypti. Insect Biochem Mol Biol (2014) 54:112–21. doi: 10.1016/j.ibmb.2014.09.004
51. Chen J, Aimanova KG, Fernandez LE, Bravo A, Soberon M, Gill SS. Aedes Aegypti Cadherin Serves as a Putative Receptor of the Cry11Aa Toxin From Bacillus Thuringiensis Subsp. Israelensis. Biochem J (2009) 424(2):191–200. doi: 10.1042/BJ20090730
52. Chen J, Aimanova KG, Pan S, Gill SS. Identification and Characterization of Aedes Aegypti Aminopeptidase N as a Putative Receptor of Bacillus Thuringiensis Cry11A Toxin. Insect Biochem Mol Biol (2009) 39(10):688–96. doi: 10.1016/j.ibmb.2009.08.003
53. Likitvivatanavong S, Chen J, Evans AM, Bravo A, Soberon M, Gill SS. Multiple Receptors as Targets of Cry Toxins in Mosquitoes. JAFC (2011) 59:2829–38. doi: 10.1021/jf1036189
54. Chen J, Likitvivatanavong S, Aimanova KG, Gill SS. A 104 kDa Aedes Aegypti Aminopeptidase N Is a Putative Receptor for the Cry11Aa Toxin From Bacillus Thuringiensis Subsp. Israelensis. Insect Biochem Mol Biol (2013) 43(12):1201–8. doi: 10.1016/j.ibmb.2013.09.007
55. Valaitis AP, Podgwaite JD. Bacillus Thuringiensis Cry1A Toxin-Binding Glycoconjugates Present on the Brush Border Membrane and in the Peritrophic Membrane of the Douglas-Fir Tussock Moth Are Peritrophins. J Invertebr Pathol (2013) 112(1):1–8. doi: 10.1016/j.jip.2012.10.002
56. Burton SL, Ellar DJ, Li J, Derbyshire DJ. N-Acetylgalactosamine on the Putative Insect Receptor Aminopeptidase N Is Recognised by a Site on the Domain III Lectin-Like Fold of aBacillus Thuringiensis Insecticidal Toxin. J Mol Biol (1999) 287(5):1011–22. doi: 10.1006/jmbi.1999.2649
57. Jenkins JL, Lee MK, Sangadala S, Adang MJ, Dean DH. Binding of Bacillus Thuringiensis Cry1Ac Toxin to Manduca Sexta Aminopeptidase-N Receptor Is Not Directly Related to Toxicity. FEBS Lett (1999) 462:373–6. doi: 10.1016/S0014-5793(99)01559-8
58. Feng D, Chen Z, Wang Z, Zhang C, He K, Guo S. Domain III of Bacillus Thuringiensis Cry1Ie Toxin Plays an Important Role in Binding to Peritrophic Membrane of Asian Corn Borer. PloS One (2015) 10(8):e0136430. doi: 10.1371/journal.pone.0136430
59. De Maagd RA, Bravo A, Berry C, Crickmore N, Schnepf HE. Structure, Diversity, and Evolution of Protein Toxins From Spore-Forming Entomopathogenic Bacteria. Annu Rev Genet (2003) 37:409–33. doi: 10.1146/annurev.genet.37.110801.143042
60. Rees JS, Jarrett P, Ellar DJ. Peritrophic Membrane Contribution to Bt Cry δ-Endotoxin Susceptibility in Lepidoptera and the Effect of Calcofluor. J Invertebrate Pathol (2009) 100(3):139–46. doi: 10.1016/j.jip.2009.01.002
61. Mack RN, Simberloff D, Mark Lonsdale W, Evans H, Clout M, Bazzaz FA. Biotic Invasions: Causes, Epidemiology, Global Consequences, and Control. Ecol Appl (2000) 10:689–710. doi: 10.1890/1051-0761(2000)010[0689:BICEGC]2.0.CO;2
62. Lounibos LP. Invasions by Insect Vectors of Human Disease. Annu Rev Entomol (2002) 47:233–66. doi: 10.1146/annurev.ento.47.091201.145206
63. Vilà M, Dunn AM, Essl F, Gómez-Díaz E, Hulme PE, Jeschke JM, et al. Viewing Emerging Human Infectious Epidemics Through the Lens of Invasion Biology. BioScience (2021) 71(7):722–40. doi: 10.1093/biosci/biab047
64. Rose NH, Sylla M, Badolo A, Lutomiah J, Ayala D, Aribodor OB, et al. Climate and Urbanization Drive Mosquito Preference for Humans. Curr Biol (2020) 30(18):3570–9:e3576. doi: 10.1016/j.cub.2020.06.092
65. Gubler DJ. Dengue and Dengue Hemorrhagic Fever. Clin Microbiol Rev (1998) 11(3):480–96. doi: 10.1128/CMR.11.3.480
66. Gubler DJ. Resurgent Vector-Borne Diseases as a Global Health Problem. Emerg Infect Dis (1998) 4(3):442–50. doi: 10.3201/eid0403.980326
67. Gubler DJ, Reiter P, Ebi KL, Yap W, Nasci R, Patz JA. Climate Variability and Change in the United States: Potential Impacts on Vector-and Rodent-Borne Diseases. Environ Health Perspect (2001) 109:223. doi: 10.1289/ehp.109-1240669
68. Gubler DJ. Epidemic Dengue/Dengue Hemorrhagic Fever as a Public Health, Social and Economic Problem in the 21st Century. Trends Microbiol (2002) 10(2):100–3. doi: 10.1016/S0966-842X(01)02288-0
69. Vasconcelos PFDC. Doença Pelo Vírus Zika: A New Emerging Problem in the Americas? Pan-Amazonian Health Magazine (2015) 6:9–10. doi: 10.5123/S2176-62232015000200001
70. WHO. Alert Global Response (GAR): Impact of Dengue. World Health Organization (2013). Available at: http://www.who.int/csr/disease/dengue/impact/en.
71. Oliveira RMD. Dengue in Rio De Janeiro: Rethinking Popular Participation in Health. Cad Public Health (1998) 14:S69–78. doi: 10.1590/S0102-311X1998000600006
72. Vilarinhos P, Dias J, Andrade C, Araújo-Coutinho C. Use of Bacteria for the Control of Culicidae and Simuliidae. Alves SB. Microbial Control of Insects. Piracicaba: Luiz Queiroz Agrarian Stud Foundation (1998) 20:447–80. doi: 10.1016/j.rbe.2017.11.004
73. Rebêlo JMM, Costa JML, Silva FS, Pereira YNO, Silva JMD. Distribution of Aedes Aegypti and Dengue in the State of Maranhão, Brasil. Cadernos Saúde Pública (1999) 15:477–86. doi: 10.1590/S0102-311X1999000300004
74. Gloria-Soria A, Ayala D, Bheecarry A, Calderon-Arguedas O, Chadee DD, Chiappero M, et al. Global Genetic Diversity of Aedes Aegypti. Mol Ecol (2016) 25(21):5377–95. doi: 10.1111/mec.13866
75. Brown JE, Mcbride CS, Johnson P, Ritchie S, Paupy C, Bossin H, et al. Worldwide Patterns of Genetic Differentiation Imply Multiple ‘Domestications’ of Aedes Aegypti, a Major Vector of Human Diseases. Proc R Soc B (2011) 278(1717):2446–54. doi: 10.1098/rspb.2010.2469
76. Brown JE, Evans BR, Zheng W, Obas V, Barrera-Martinez L, Egizi A, et al. Human Impacts Have Shaped Historical and Recent Evolution in Aedes Aegypti, the Dengue and Yellow Fever Mosquito. Evolution (2014) 68(2):514–25. doi: 10.1111/evo.12281
77. Bennett KL, Shija F, Linton YM, Misinzo G, Kaddumukasa M, Djouaka R, et al. Historical Environmental Change in Africa Drives Divergence and Admixture of Aedes Aegypti Mosquitoes: A Precursor to Successful Worldwide Colonization? Mol Ecol (2016) 25:4337–54. doi: 10.1111/mec.13762
78. Crawford JE, Alves JM, Palmer WJ, Day JP, Sylla M, Ramasamy R, et al. Population Genomics Reveals That an Anthropophilic Population of Aedes Aegypti Mosquitoes in West Africa Recently Gave Rise to American and Asian Populations of This Major Disease Vector. BMC Biol (2017) 15:16. doi: 10.1186/s12915-017-0351-0
80. Clements A. The Biology of Mosquitoes: Development, Nutrition and Reproduction Vol. 1. Wallingford: CAB International publisher (1992). p. 1978613.
81. Clements AN. The Biology of Mosquitoes. Volume 2: Sensory Reception and Behaviour. Wallingford. Localização: CABI publishing (1999). BR1719.1;595.77 LI, C626b, 1999 v.
82. Hawkes FM, Hopkins RJ. The Mosquito: An Introduction. In: Mosquitopia. London, United Kingdom: Routledge (2021). p. 16–31. doi: 10.4324/9781003056034-2
83. Christophers S. Aedes Aegypti (L.) the Yellow Fever Mosquito: Its Life History, Bionomics and Structure. London: Cambridge University Press (1960).
84. Foster W, Walker E. Mosquitoes (Culicidae). In: Mullen G, Durden L, editors. Med. Vet. Entomol. San Diego, CA: Academic (2002). p. 203–62.
85. Kraemer MU, Reiner RC, Brady OJ, Messina JP, Gilbert M, Pigott DM, et al. Past and Future Spread of the Arbovirus Vectors Aedes Aegypti and Aedes Albopictus. Nat Microbiol (2019) 4:854–63. doi: 10.1038/s41564-019-0376-y
86. Morand S, Lajaunie C. Outbreaks of Vector-Borne and Zoonotic Diseases Are Associated With Changes in Forest Cover and Oil Palm Expansion at Global Scale. Front Vet Sci (2021) 8:661063. doi: 10.3389/fvets.2021.661063
87. Chen R, Vasilakis N. Dengue— Where Are You and Where Are You Going? Viruses (2011) 3(9):1562–608. doi: 10.3390/v3091562
88. Burt FJ, Rolph MS, Rulli NE, Mahalingam S, Heise MT. Chikungunya: A Re-Emerging Virus. Lancet (2012) 379(9816):662–71. doi: 10.1016/S0140-6736(11)60281-X
89. Calvez E, Guillaumot L, Millet L, Marie J, Bossin H, Rama V, et al. Genetic Diversity and Phylogeny ofAedes Aegypti, the Main Arbovirus Vector in the Pacific. PloS Negl Trop Dis (2016) 10(1):e0004374. doi: 10.1371/journal.pntd.0004374
90. Barrett AD, Higgs S. Yellow Fever: A Disease That has Yet to be Conquered. Annu Rev Entomol (2007) 52:209–29. doi: 10.1146/annurev.ento.52.110405.091454
91. Bovay A, Fuertes Marraco SA, Speiser DE. Yellow Fever Virus Vaccination: An Emblematic Model to Elucidate Robust Human Immune Responses. Hum Vaccines Immunother (2021) 17(8):2471–81. doi: 10.1080/21645515.2021.1891752
92. Guzman MG, Halstead SB, Artsob H, Buchy P, Farrar J, Gubler DJ, et al. Dengue: A Continuing Global Threat. Nat Rev Microbiol (2010) 8:S7–S16. doi: 10.1038/nrmicro2460
93. Pinheiro FP, Corber SJ. Global Situation of Dengue and Dengue Haemorrhagic Fever, and Its Emergence in the Americas. World Health Stat (1997) 50(3-4):161–9.
94. Abd-Jamil J, Ngui R, Nellis S, Fauzi R, Lim ALY, Chinna K, et al. Possible Factors Influencing the Seroprevalence of Dengue Among Residents of the Forest Fringe Areas of Peninsular Malaysia. J Trop Med (2020) 2020:1019238. doi: 10.1155/2020/1019238
95. Bhatt S, Gething PW, Brady OJ, Messina JP, Farlow AW, Moyes CL, et al. The Global Distribution and Burden of Dengue. Nature (2013) 496:504–7. doi: 10.1038/nature12060
96. Wang S-F, Chang K, Loh E-W, Wang W-H, Tseng S-P, Lu P-L, et al. Consecutive Large Dengue Outbreaks in Taiwan in 2014–2015. Emerg Microbes Infect (2016) 5:e123. doi: 10.1038/emi.2016.124
97. Yang X, Quam MBM, Zhang T, Sang S. Global Burden for Dengue and the Evolving Pattern in the Past 30 Years. J Travel Med (2021) 28(8):1–11. doi: 10.1093/jtm/taab146
98. Carod-Artal FJ. Epidemiology and Neurological Complications of Infection by the Zika Virus: A New Emerging Neurotropic Virus. Reply. Rev Neurol (2016) 62(7):317–28.
99. Teixeira MG, da Conceição N Costa M, de Oliveira WK, Nunes ML, Rodrigues LC. The Epidemic of Zika Virus–Related Microcephaly in Brazil: Detection, Control, Etiology, and Future Scenarios. Am J Public Health (2016) 106:601–5. doi: 10.2105/ajph.2016.303113
100. Fauci AS, Morens DM. Zika Virus in the Americas—yet Another Arbovirus Threat. NEJM (2016) 374(7):601–4. doi: 10.1056/nejmp1600297
101. Robinson MC. An Epidemic of Virus Disease in Southern Province, Tanganyika Territory, in 1952-53. I. Clinical Features. Trans R Soc Trop Med Hyg (1955) 49:28–32. doi: 10.1016/0035-9203(55)90080-8
102. Shrinet J, Jain S, Sharma A, Singh SS, Mathur K, Rana V, et al. Genetic Characterization of Chikungunya Virus From New Delhi Reveal Emergence of a New Molecular Signature in Indian Isolates. Virol J (2012) 9:100. doi: 10.1186/1743-422X-9-100
103. Wimalasiri-Yapa BR, Stassen L, Huang X, Hafner LM, Hu W, Devine GJ, et al. Chikungunya Virus in Asia–Pacific: A Systematic Review. Emerg Microbes Infect (2019) 8:70–9. doi: 10.1080/22221751.2018.1559708
104. Puntasecca CJ, King CH, Labeaud AD. Measuring the Global Burden of Chikungunya and Zika Viruses: A Systematic Review. PloS Negl Trop Dis (2021) 15:e0009055. doi: 10.1371/journal.pntd.0009055
105. Poopathi S, Charles J-F, Nielsen-Leroux C. Alternative Method for Preservation of Mosquito Larvae to Study Binding Mechanisms of Bacillus Sphaericus Binary Toxin. J Invertebr Pathol (2002) 79:132–4. doi: 10.1016/s0022-2011(02)00017-4
106. Stalinski R, Laporte F, Després L, Tetreau G. Alkaline Phosphatases Are Involved in the Response of A Edes Aegypti Larvae to Intoxication With B Acillus Thuringiensis Subsp. Israelensis C Ry Toxins. Environ Microbiol (2016) 18:1022–36. doi: 10.1111/1462-2920.13186
107. Ben-Dov E. Bacillus Thuringiensis Subsp. Israelensis and Its Dipteran-Specific Toxins. Toxins (2014) 6(4):1222–43. doi: 10.3390/toxins6041222
108. Berry C, O'neil S, Ben-Dov E, Jones AF, Murphy L, Quail MA, et al. Complete Sequence and Organization of Pbtoxis, the Toxin-Coding Plasmid of Bacillus Thuringiensis Subsp. Israelensis. Appl Environ Microbiol (2002) 68:5082–95. doi: 10.1128/AEM.68.10.5082-5095.2002
109. Xiong G. Progress in the Studies and Application of Bacillus Thuringiensis. Rev China Agric Sci Technol (2006) 8(6):5.
110. Guerchicoff A, Delécluse A, Rubinstein CP. The Bacillus Thuringiensis Cyt Genes for Hemolytic Endotoxins Constitute a Gene Family. Appl Environ Microbiol (2001) 67(3):1090–6. doi: 10.1128/AEM.67.3.1090-1096.2001
111. Chilcott CN, Ellar DJ. Comparative Toxicity of Bacillus Thuringiensis Var. Israelensis Crystal Proteins In Vivo and In Vitro. Microbiology (1988) 134(9):2551–8. doi: 10.1099/00221287-134-9-2551
112. Janisiewicz WJ, Korsten L. Biological Control of Postharvest Diseases of Fruits. Annu Rev Phytopathol (2002) 40:411–41. doi: 10.1146/annurev.phyto.40.120401.130158
113. Do Nascimento J, Goncalves KC, Dias NP, De Oliveira JL, Bravo A, Polanczyk RA. Adoption of Bacillus Thuringiensis-Based Biopesticides in Agricultural Systems and New Approaches to Improve Their Use in Brazil. Biol Control (2021) 165:104792. doi: 10.1016/j.biocontrol.2021.104792
114. Boonserm P, Davis P, Ellar DJ, Li J. Crystal Structure of the Mosquito-Larvicidal Toxin Cry4Ba and Its Biological Implications. J Mol Biol (2005) 348(2):363–82. doi: 10.1016/j.jmb.2005.02.013
115. Cabiaux V, Wolff C, Ruysschaert J-M. Interaction With a Lipid Membrane: A Key Step in Bacterial Toxins Virulence. Int J Biol Macromol (1997) 21(4):285–98. doi: 10.1016/S0141-8130(97)00078-0
116. Gómez I, Sánchez J, Miranda R, Bravo A, Soberón M. Cadherin-Like Receptor Binding Facilitates Proteolytic Cleavage of Helix α-1 in Domain I and Oligomer Pre-Pore Formation of Bacillus Thuringiensis Cry1Ab Toxin. FEBS Lett (2002) 513(3):242–6. doi: 10.1016/S0014-5793(02)02321-9
117. Pigott CR, Ellar DJ. Role of Receptors in Bacillus Thuringiensis Crystal Toxin Activity. Microbiol Mol Biol Rev (2007) 71:255–81. doi: 10.1128/MMBR.00034-06
118. Cantón PE, Reyes EZ, De Escudero IR, Bravo A, Soberón M. Binding of Bacillus Thuringiensis Subsp. Israelensis Cry4Ba to Cyt1Aa has an Important Role in Synergism. Peptides (2011) 32(3):595–600. doi: 10.1016/j.peptides.2010.06.005
119. Rodríguez-Almazán C, Reyes EZ, Zúñiga-Navarrete F, Muñoz-Garay C, Gómez I, Evans AM, et al. Cadherin Binding Is Not a Limiting Step for Bacillus Thuringiensis Subsp. Israelensis Cry4Ba Toxicity to Aedes Aegypti Larvae. Biochem J (2012) 443:711–7. doi: 10.1042/BJ20111579
120. Gómez I, Pardo-López L, Munoz-Garay C, Fernandez L, Pérez C, Sánchez J, et al. Role of Receptor Interaction in the Mode of Action of Insecticidal Cry and Cyt Toxins Produced by Bacillus Thuringiensis. Peptides (2007) 28(1):169–73. doi: 10.1016/j.peptides.2006.06.013
121. Vachon V, Laprade R, Schwartz J-L. Current Models of the Mode of Action of Bacillus Thuringiensis Insecticidal Crystal Proteins: A Critical Review. J Invertebr Pathol (2012) 111(1):1–12. doi: 10.1016/j.jip.2012.05.001
122. Pardo-Lopez L, Soberon M, Bravo A. Bacillus Thuringiensis Insecticidal Three-Domain Cry Toxins: Mode of Action, Insect Resistance and Consequences for Crop Protection. FEMS Microbiol Rev (2013) 37:3–22. doi: 10.1111/j.1574-6976.2012.00341.x
123. Adang MJ, Crickmore N, Jurat-Fuentes JL. Diversity of Bacillus Thuringiensis Crystal Toxins and Mechanism of Action. In: Adv In Insect Phys. Iowa, United States: Elsevier (2014). p. 39–87. doi: 10.1016/B978-0-12-800197-4.00002-6
124. Fernandez LE, Martinez-Anaya C, Lira E, Chen J, Evans A, Hernández-Martínez S, et al. Cloning and Epitope Mapping of Cry11Aa-Binding Sites in the Cry11Aa-Receptor Alkaline Phosphatase From Aedes Aegypti. Biochemistry (2009) 48(37):8899–907. doi: 10.1021/bi900979b
125. Likitvivatanavong S, Chen J, Bravo A, Soberón M, Gill SS. Cadherin, Alkaline Phosphatase, and Aminopeptidase N as Receptors of Cry11Ba Toxin From Bacillus Thuringiensis Subsp. Jegathesan in Aedes Aegypti. Appl Environ Microbiol (2011) 77:24–31. doi: 10.1128/AEM.01852-10
126. Jiménez AI, Reyes EZ, Cancino-Rodezno A, Bedoya-Pérez LP, Caballero-Flores GG, Muriel-Millan LF, et al. Aedes Aegypti Alkaline Phosphatase ALP1 Is a Functional Receptor of Bacillus Thuringiensis Cry4Ba and Cry11Aa Toxins. Insect Biochem Mol Biol (2012) 42(9):683–9. doi: 10.1016/j.ibmb.2012.06.001
127. Christophides GK, Zdobnov E, Barillas-Mury C, Birney E, Blandin S, Blass C, et al. Immunity-Related Genes and Gene Families in Anopheles Gambiae. Science (2002) 298(5591):159–65. doi: 10.1126/science.1077136
128. Daborn PJ, Lumb C, Boey A, Wong W, Batterham P. Evaluating the Insecticide Resistance Potential of Eight Drosophila Melanogaster Cytochrome P450 Genes by Transgenic Over-Expression. Insect Biochem Mol Biol (2007) 37(5):512–9. doi: 10.1016/j.ibmb.2007.02.008
129. Shin SW, Kokoza V, Lobkov I, Raikhel AS. Relish-Mediated Immune Deficiency in the Transgenic Mosquito Aedes Aegypti. PNAS (2003) 100:2616–21. doi: 10.1073/pnas.0537347100
130. Antonova Y, Alvarez KS, Kim YJ, Kokoza V, Raikhel AS. The Role of NF-κb Factor REL2 in the Aedes Aegypti Immune Response. Insect Biochem Mol Biol (2009) 39:303–14. doi: 10.1016/j.ibmb.2009.01.007
131. Naithani S, Komath SS, Nonomura A, Govindjee G. Plant Lectins and Their Many Roles: Carbohydrate-Binding and Beyond. J Plant Physiol (2021) 266:153531. doi: 10.1016/j.jplph.2021.153531
132. Van Damme EJ. 35 Years in Plant Lectin Research: A Journey From Basic Science to Applications in Agriculture and Medicine. Glycoconjugate J (2021) 39:1–15. doi: 10.1007/s10719-021-10015-x
133. Ahmad S, Singh S, Wasim S, Naziya S. Role of Glycan-Lectin Interaction in Diseases: A Review. Saudi J BioMed Res (2021) 6:85–94. doi: 10.36348/sjbr.2021.v06i05.002
134. Carneiro DC, Fernandez LG, Monteiro-Cunha JP, Benevides RG, Cunha Lima ST. A Patent Review of the Antimicrobial Applications of Lectins: Perspectives on Therapy of Infectious Diseases. J Appl Microbiol (2021) 132(2):841–54. doi: 10.1111/jam.15263
135. Idowu PA, Idowu AP, Zishiri OT, Mpofu TJ, Veldhuizen EJA, Nephawe KA, et al. Activity of Mannose-Binding Lectin on Bacterial-Infected Chickens—A Review. Animals (2021) 11(3):787. doi: 10.3390/ani11030787
136. Kamei R, Devi OS, Singh SJ, Singh SS. Roles and Biomedical Applications of Haemolymph Lectin. Curr Pharm Biotechnol (2020) 21(14):1444–50. doi: 10.2174/1389201021666200730123330
137. Lindenwald DL, Lepenies B. C-Type Lectins in Veterinary Species: Recent Advancements and Applications. IJMS (2020) 21(14):5122. doi: 10.3390/ijms21145122
138. Speakman EA, Dambuza IM, Salazar F, Brown GD. T Cell Antifungal Immunity and the Role of C-Type Lectin Receptors. Trends Immunol (2020) 41:61–76. doi: 10.1016/j.it.2019.11.007
139. Lemaitre B, Hoffmann J. The Host Defense of Drosophila Melanogaster. Annu Rev Immunol (2007) 25:697–743. doi: 10.1146/annurev.immunol.25.022106.141615
140. Nunes C, Sucena E, Koyama T. Endocrine Regulation of Immunity in Insects. FEBS J (2021) 288:3928–47. doi: 10.1111/febs.15581
141. Zhang R, Li X, Zhang J, Li Y, Wang Y, Song Y, et al. Toll9 From Bombyx Mori Functions as a Pattern Recognition Receptor That Shares Features With Toll-Like Receptor 4 From Mammals. PNAS (2021) 118:e2103021118. doi: 10.1073/pnas.2103021118
142. Xia X, You M, Rao X-J, Yu X-Q. Insect C-Type Lectins in Innate Immunity. Dev Comp Immunol (2018) 83:70–9. doi: 10.1016/j.dci.2017.11.020
143. Ling E, Ao J, Yu X-Q. Nuclear Translocation of Immulectin-3 Stimulates Hemocyte Proliferation. Mol Immunol (2008) 45(9):2598–606. doi: 10.1016/j.molimm.2007.12.021
144. Li M, Li C, Ma C, Li H, Zuo H, Weng S, et al. Identification of a C-Type Lectin With Antiviral and Antibacterial Activity From Pacific White Shrimp Litopenaeus Vannamei. Dev Comp Immunol (2014) 46(2):231–40. doi: 10.1016/j.dci.2014.04.014
145. Yu X-Q, Zhu Y-F, Ma C, Fabrick J, Kanost M. Pattern Recognition Proteins in Manduca Sexta Plasma. Insect Biochem Mol Biol (2002) 32:1287–93. doi: 10.1016/S0965-1748(02)00091-7
146. Yu X-Q, Kanost MR. Manduca Sexta Lipopolysaccharide-Specific Immulectin-2 Protects Larvae From Bacterial Infection. Dev Comp Immunol (2003) 27(3):189–96. doi: 10.1016/S0145-305X(02)00099-X
147. Bi J, Feng F, Li J, Mao J, Ning M, Song X, et al. AC-Type Lectin With a Single Carbohydrate-Recognition Domain Involved in the Innate Immune Response of Tribolium Castaneum. Insect Mol Biol (2019) 28:649–61. doi: 10.1111/imb.12582
148. Li J, Bi J, Zhang P, Wang Z, Zhong Y, Xu S, et al. Functions of a C-Type Lectin With a Single Carbohydrate-Recognition Domain in the Innate Immunity and Movement of the Red Flour Beetle, Tribolium Castaneum. Insect Mol Biol (2021) 30:90–101. doi: 10.1111/imb.12680
149. Qiu W, Chen F, Chen R, Li S, Zhu X, Xiong M, et al. A New C-Type Lectin Homolog SpCTL6 Exerting Immunoprotective Effect and Regulatory Role in Mud Crab Scylla Paramamosain. Front Immunol (2021) 12:661823. doi: 10.3389/fimmu.2021.661823
150. Lu Y, Su F, Zhu K, Zhu M, Li Q, Hu Q, et al. Comparative Genomic Analysis of C-Type Lectin-Domain Genes in Seven Holometabolous Insect Species. Insect Biochem Mol Biol (2020) 126:103451. doi: 10.1016/j.ibmb.2020.103451
151. Koizumi N, Imamura M, Kadotani T, Yaoi K, Iwahana H, Sato R. The Lipopolysaccharide-Binding Protein Participating in Hemocyte Nodule Formation in the Silkworm Bombyx Mori Is a Novel Member of the C-Type Lectin Superfamily With Two Different Tandem Carbohydrate-Recognition Domains 1. FEBS Lett (1999) 443:139–43. doi: 10.1016/S0014-5793(98)01701-3
152. Dambuza IM, Brown GD. C-Type Lectins in Immunity: Recent Developments. Curr Opin Immunol (2015) 32:21–7. doi: 10.1016/j.coi.2014.12.002
153. Iborra S, Sancho D. Signalling Versatility Following Self and Non-Self Sensing by Myeloid C-Type Lectin Receptors. Immunobiology (2015) 220(2):175–84. doi: 10.1016/j.imbio.2014.09.013
154. Li H, Liu F-F, Fu L-Q, Liu Z, Zhang W-T, Wang Q, et al. Identification of 35 C-Type Lectins in the Oriental Armyworm, Mythimna Separata (Walker). Insects (2021) 12(6):559. doi: 10.3390/insects12060559
155. Yu X-Q, Gan H, Kanost MR. Immulectin, an Inducible C-Type Lectin From an Insect, Manduca Sexta, Stimulates Activation of Plasma Prophenol Oxidase. Insect Biochem Mol Biol (1999) 29(7):585–97. doi: 10.1016/S0965-1748(99)00036-3
156. Yu X-Q, Kanost MR. Immulectin-2, a Pattern Recognition Receptor That Stimulates Hemocyte Encapsulation and Melanization in the Tobacco Hornworm, Manduca Sexta. Dev Comp Immunol (2004) 28(9):891–900. doi: 10.1016/j.dci.2004.02.005
157. Wang W, Wang G, Zhuo X, Liu Y, Tang L, Liu X, et al. C-Type Lectin-Mediated Microbial Homeostasis Is Critical for Helicoverpa Armigera Larval Growth and Development. PloS Pathog (2020) 16:e1008901. doi: 10.1371/journal.ppat.1008901
158. Dillon R, Dillon V. The Gut Bacteria of Insects: Nonpathogenic Interactions. Annu Rev Entomol (2004) 49:71–92. doi: 10.1146/annurev.ento.49.061802.123416
159. Gabrieli P, Caccia S, Varotto-Boccazzi I, Arnoldi I, Barbieri G, Comandatore F, et al. Mosquito Trilogy: Microbiota, Immunity and Pathogens, and Their Implications for the Control of Disease Transmission. Front Microbiol (2021) 12:630438. doi: 10.3389/fmicb.2021.630438
160. Dong Y, Manfredini F, Dimopoulos G. Implication of the Mosquito Midgut Microbiota in the Defense Against Malaria Parasites. PloS Pathog (2009) 5(5):e1000423. doi: 10.1371/journal.ppat.1000423
161. Meister S, Agianian B, Turlure F, Relógio A, Morlais I, Kafatos FC, et al. Anopheles Gambiae PGRPLC-Mediated Defense Against Bacteria Modulates Infections With Malaria Parasites. PloS Pathog (2009) 5:e1000542. doi: 10.1371/journal.ppat.1000542
162. Ramirez JL, Souza-Neto J, Cosme RT, Rovira J, Ortiz A, Pascale JM, et al. Reciprocal Tripartite Interactions Between the Aedes Aegypti Midgut Microbiota, Innate Immune System and Dengue Virus Influences Vector Competence. PloS Negl Trop Dis (2012) 6:e1561. doi: 10.1371/journal.pntd.0001561
163. Adelman ZN, Myles KM. The C-Type Lectin Domain Gene Family in Aedes Aegypti and Their Role in Arbovirus Infection. Viruses (2018) 10:367. doi: 10.3390/v10070367
164. Li H-H, Cai Y, Li J-C, Su MP, Liu W-L, Cheng L, et al. C-Type Lectins Link Immunological and Reproductive Processes in Aedes Aegypti. Iscience (2020) 23(9):101486. doi: 10.1016/j.isci.2020.101486
165. Cheng L, Liu W-L, Tsou Y-T, Li J-C, Chien C-H, Su MP, et al. Transgenic Expression of Human C-Type Lectin Protein Clec18a Reduces Dengue Virus Type 2 Infectivity in Aedes Aegypti. Front Immunol (2021) 12:640367. doi: 10.3389/fimmu.2021.640367
166. Cheng G, Cox J, Wang P, Krishnan MN, Dai J, Qian F, et al. A C-Type Lectin Collaborates With a CD45 Phosphatase Homolog to Facilitate West Nile Virus Infection of Mosquitoes. Cell (2010) 142(5):714–25. doi: 10.1016/j.cell.2010.07.038
167. Liu K, Qian Y, Jung Y-S, Zhou B, Cao R, Shen T, et al. mosGCTL-7, a C-Type Lectin Protein, Mediates Japanese Encephalitis Virus Infection in Mosquitoes. J Virol (2017) 91:e01348-01316. doi: 10.1128/JVI.01348-16
168. Dodd RB, Drickamer K. Lectin-Like Proteins in Model Organisms: Implications for Evolution of Carbohydrate-Binding Activity. Glycobiology (2001) 11(5):71R–9R. doi: 10.1093/glycob/11.5.71R
169. Rao X-J, Cao X, He Y, Hu Y, Zhang X, Chen Y-R, et al. Structural Features, Evolutionary Relationships, and Transcriptional Regulation of C-Type Lectin-Domain Proteins in Manduca Sexta. Insect Biochem Mol Biol (2015) 62:75–85. doi: 10.1016/j.ibmb.2014.12.006
170. Schnitger AK, Yassine H, Kafatos FC, Osta MA. Two C-Type Lectins Cooperate to Defend Anopheles Gambiae Against Gram-Negative Bacteria. J Biol Chem (2009) 284:17616–24. doi: 10.1074/jbc.M808298200
171. Wang X-W, Xu J-D, Zhao X-F, Vasta GR, Wang J-X. A Shrimp C-Type Lectin Inhibits Proliferation of the Hemolymph Microbiota by Maintaining the Expression of Antimicrobial Peptides. J Biol Chem (2014) 289:11779–90. doi: 10.1074/jbc.M114.552307
172. Ferreira PG, Tesla B, Horácio ECA, Nahum LA, Brindley MA, De Oliveira Mendes TA, et al. Temperature Dramatically Shapes Mosquito Gene Expression With Consequences for Mosquito-Zika Virus Interactions. Front Microbiol (2020) 11:901. doi: 10.3389/fmicb.2020.00901
173. Kanagawa M, Satoh T, Ikeda A, Nakano Y, Yagi H, Kato K, et al. Crystal Structures of Human Secretory Proteins ZG16p and ZG16b Reveal a Jacalin-Related β-Prism Fold. Biochem Biophys Res Commun (2011) 404(1):201–5. doi: 10.1016/j.bbrc.2010.11.093
174. Li J, Carroll J, Ellar DJ. Crystal Structure of Insecticidal δ-Endotoxin From Bacillus Thuringiensis at 2.5 Å Resolution. Nature (1991) 353:815–21. doi: 10.1038/353815a0
175. Grochulski P, Masson L, Borisova S, Pusztai-Carey M, Schwartz J-L, Brousseau R, et al. Bacillus Thuringiensis CrylA (a) Insecticidal Toxin: Crystal Structure and Channel Formation. J Mol Biol (1995) 254(3):447–64. doi: 10.1006/jmbi.1995.0630
176. Batool K, Alam I, Zhao G, Wang J, Xu J, Yu X, et al. C-Type Lectin-20 Interacts With ALP1 Receptor to Reduce Cry Toxicity inAedes Aegypti. Toxins (2018) 10(10):390. doi: 10.3390/toxins10100390
177. Zhang L-L, Hu X-H, Wu S-Q, Batool K, Chowdhury M, Lin Y, et al. Aedes Aegypti Galectin Competes With Cry11Aa for Binding to ALP1 To Modulate Cry Toxicity. JAFC (2018) 66:13435–43. doi: 10.1021/acs.jafc.8b04665
178. Batool K, Alam I, Jin L, Xu J, Wu C, Wang J, et al. CTLGA9 Interacts With ALP1 and APN Receptors To Modulate Cry11Aa Toxicity in Aedes Aegypti. JAFC (2019) 67:8896–904. doi: 10.1021/acs.jafc.9b01840
179. Hu X, Chen H, Xu J, Zhao G, Huang X, Liu J, et al. Function of Aedes Aegypti Galectin-6 in Modulation of Cry11Aa Toxicity. Pesticide Biochem Physiol (2019) 162:96–104. doi: 10.1016/j.pestbp.2019.09.010
180. Griffitts JS, Haslam SM, Yang T, Garczynski SF, Mulloy B, Morris H, et al. Glycolipids as Receptors for Bacillus Thuringiensis Crystal Toxin. Science (2005) 307:922–5. doi: 10.1126/science.1104444
181. Pandian GN, Ishikawa T, Togashi M, Shitomi Y, Haginoya K, Yamamoto S, et al. Bombyx Mori Midgut Membrane Protein P252, Which Binds to Bacillus Thuringiensis Cry1A, Is a Chlorophyllide-Binding Protein, and the Resulting Complex has Antimicrobial Activity. Appl Environ Microbiol (2008) 74:1324–31. doi: 10.1128/AEM.01901-07
182. Da Silva IHS, Goméz I, Sánchez J, De Castro DLM, Valicente FH, Soberón M, et al. Identification of Midgut Membrane Proteins From Different Instars of Helicoverpa Armigera (Lepidoptera: Noctuidae) That Bind to Cry1Ac Toxin. PloS One (2018) 13:e0207789. doi: 10.1371/journal.pone.0207789
183. Rausell C, Martínez-Ramírez AC, García-Robles I, Real MD. A Binding Site for Bacillus Thuringiensis Cry1Ab Toxin Is Lost During Larval Development in Two Forest Pests. Appl Environ Microbiol (2000) 66:1553–8. doi: 10.1128/AEM.66.4.1553-1558.2000
184. Jurat-Fuentes JL, Adang MJ. Characterization of a Cry1Ac-Receptor Alkaline Phosphatase in Susceptible and Resistant Heliothis Virescens Larvae. Eur J Chem (2004) 271:3127–35. doi: 10.1111/j.1432-1033.2004.04238.x
185. Herrero S, Gechev T, Bakker PL, Moar WJ, De Maagd RA. Bacillus Thuringiensis Cry1Ca-Resistant Spodoptera Exigua Lacks Expression of One of Four Aminopeptidase N Genes. BMC Genomics (2005) 6:96. doi: 10.1186/1471-2164-6-96
186. Flores-Escobar B, Rodríguez-Magadan H, Bravo A, Soberón M, Gómez I. Differential Role of Manduca Sexta Aminopeptidase-N and Alkaline Phosphatase in the Mode of Action of Cry1Aa, Cry1Ab, and Cry1Ac Toxins From Bacillus Thuringiensis. Appl Environ Microbiol (2013) 79(15):4543–50. doi: 10.1128/AEM.01062-13
187. Knowles BH, Knight PJ, Ellar DJ. N-Acetyl Galactosamine Is Part of the Receptor in Insect Gut Epithelia That Recognizes an Insecticidal Protein From Bacillus Thuringiensis. Proc R Soc Lond Ser B: Biol Sci (1991) 245:31–5. doi: 10.1098/rspb.1991.0084
188. Wirth MC. Mosquito Resistance to Bacterial Larvicidal Toxins. Open Toxinol J (2010) 3:126–40. doi: 10.2174/1875414701003010126
189. Shao E, Lin L, Chen C, Chen H, Zhuang H, Wu S, et al. Loop Replacements With Gut-Binding Peptides in Cry1Ab Domain II Enhanced Toxicity Against the Brown Planthopper, Nilaparvata Lugens (Stål). Sci Rep (2016) 6:20106. doi: 10.1038/srep20106
190. Torres-Quintero M-C, Gómez I, Pacheco S, Sánchez J, Flores H, Osuna J, et al. Engineering Bacillus Thuringiensis Cyt1Aa Toxin Specificity From Dipteran to Lepidopteran Toxicity. Sci Rep (2018) 8:1–12. doi: 10.1038/s41598-018-22740-9
191. Valtierra-De-Luis D, Villanueva M, Lai L, Williams T, Caballero P. Potential of Cry10Aa and Cyt2Ba, Two Minority δ-Endotoxins Produced by Bacillus Thuringiensis Ser. Israelensis, for the Control of Aedes Aegypti Larvae. Toxins (2020) 12(6):355. doi: 10.3390/toxins12060355
192. López-Molina S, Do Nascimento NA, Silva-Filha MHNL, Guerrero A, Sánchez J, Pacheco S, et al. In Vivo Nanoscale Analysis of the Dynamic Synergistic Interaction of Bacillus Thuringiensis Cry11Aa and Cyt1Aa Toxins inAedes Aegypti. PloS Pathog (2021) 17:e1009199. doi: 10.1371/journal.ppat.1009199
193. Guo Z, Kang S, Wu Q, Wang S, Crickmore N, Zhou X, et al. The Regulation Landscape of MAPK Signaling Cascade for Thwarting Bacillus Thuringiensis Infection in an Insect Host. PloS Pathog (2021) 17:e1009917. doi: 10.1371/journal.ppat.1009917
194. Ma X, Shao E, Chen W, Cotto-Rivera RO, Yang X, Kain W, et al. Bt Cry1Ac Resistance in Trichoplusia Ni Is Conferred by Multi-Gene Mutations. Insect Biochem Mol Biol (2022) 140:103678. doi: 10.1016/j.ibmb.2021.103678
195. Güney G, Cedden D, Hänniger S, Heckel DG, Coutu C, Hegedus DD, et al. Silencing of an ABC Transporter, But Not a Cadherin, Decreases the Susceptibility of Colorado Potato Beetle Larvae to Bacillus Thuringiensis Ssp. Tenebrionis Cry3Aa Toxin. Arch Insect Biochem Physiol (2021) 108:e21834. doi: 10.1002/arch.21834
196. Yang Y-J, Xu H-X, Wu Z-H, Lu Z-X. Effects of Inhibitors on the Protease Profiles and Degradation of Activated Cry Toxins in Larval Midgut Juices of Cnaphalocrocis Medinalis (Lepidoptera: Pyralidae). J Integr Agric (2021) 20(8):2195–203. doi: 10.1016/S2095-3119(20)63316-0
197. Dhania NK, Chauhan VK, Abhilash D, Thakur V, Chaitanya R, Dutta-Gupta S, et al. Gut-Specific Arylphorin Mediates Midgut Regenerative Response Against Cry-Induced Damage in Achaea Janata. Comp Biochem Physiol Part B: Biochem Mol Biol (2021) 255:110600. doi: 10.1016/j.cbpb.2021.110600
Keywords: Bacillus thuringiensis, Aedes aegypti, lectin, toxicity, mechanism
Citation: Alam I, Batool K, Idris AL, Tan W, Guan X and Zhang L (2022) Role of Lectin in the Response of Aedes aegypti Against Bt Toxin. Front. Immunol. 13:898198. doi: 10.3389/fimmu.2022.898198
Received: 17 March 2022; Accepted: 19 April 2022;
Published: 13 May 2022.
Edited by:
Fengliang Jin, South China Agricultural University, ChinaReviewed by:
Bernardo Franco, University of Guanajuato, MexicoZhiqing Li, Southwest University, China
Copyright © 2022 Alam, Batool, Idris, Tan, Guan and Zhang. This is an open-access article distributed under the terms of the Creative Commons Attribution License (CC BY). The use, distribution or reproduction in other forums is permitted, provided the original author(s) and the copyright owner(s) are credited and that the original publication in this journal is cited, in accordance with accepted academic practice. No use, distribution or reproduction is permitted which does not comply with these terms.
*Correspondence: Lingling Zhang, bGluZ2xpbmcwMDI2NEAxNjMuY29t
†These authors have contributed equally to this work