- 1State Key Laboratory of Livestock and Poultry Breeding, Ministry of Agriculture Key Laboratory of Animal Nutrition and Feed Science in South China, Guangdong Key Laboratory of Animal Breeding and Nutrition, Maoming Branch, Guangdong Laboratory for Lingnan Modern Agriculture, Institute of Animal Science, Guangdong Academy of Agricultural Sciences, Guangzhou, China
- 2College of Animal Sciences and Technology, Zhongkai University of Agriculture and Engineering, Guangzhou, China
Intestinal epithelial barrier injury disrupts immune homeostasis and leads to many intestinal disorders. Lactobacillus reuteri (L. reuteri) strains can influence immune system development and intestinal function. However, the underlying mechanisms of L. reuteri LR1 that regulate inflammatory response and intestinal integrity are still unknown. The present study aimed to determine the effects of LR1 on the ETEC K88-induced intestinal epithelial injury on the inflammatory response, intestinal epithelial barrier function, and the MLCK signal pathway and its underlying mechanism. Here, we showed that the 1 × 109 cfu/ml LR1 treatment for 4 h dramatically decreased interleukin-8 (IL-8) and IL-6 expression. Then, the data indicated that the 1 × 108 cfu/ml ETEC K88 treatment for 4 h dramatically enhanced IL-8, IL-6, and tumor necrosis factor-α (TNF-α) expression. Furthermore, scanning electron microscope (SEM) data indicated that pretreatment with LR1 inhibited the ETEC K88 that adhered on IPEC-J2 and alleviated the scratch injury of IPEC J2 cells. Moreover, LR1 pretreatment significantly reversed the declined transepithelial electrical resistance (TER) and tight junction protein level, and enhanced the induction by ETEC K88 treatment. Additionally, LR1 pretreatment dramatically declined IL-8, IL-17A, IL-6, and TNF-α levels compared with the ETEC K88 group. Then, ETEC K88-treated IPEC-J2 cells had a higher level of myosin light-chain kinase (MLCK), higher MLC levels, and a lower Rho-associated kinase (ROCK) level than the control group, while LR1 pretreatment significantly declined the MLCK and MLC expression and enhanced ROCK level in the ETEC K88-challenged IPEC-J2 cells. Mechanistically, depletion of MLCK significantly declined MLC expression in IPEC-J2 challenged with ETEC K88 compared to the si NC+ETEC K88 group. On the other hand, the TER of the si MLCK+ETEC K88 group was higher and the FD4 flux in the si MLCK+ETEC K88 group was lower compared with the si NC+ETEC K88 group. In addition, depletion of MLCK significantly enhanced Claudin-1 level and declined IL-8 and TNF-α levels in IPEC-J2 pretreated with LR1 followed by challenging with ETEC K88. In conclusion, our work indicated that L. reuteri LR1 can decline inflammatory response and improve intestinal epithelial barrier function through suppressing the MLCK signal pathway in the ETEC K88-challenged IPEC-J2.
Introduction
Weaning stress is extremely serious during the initial post-weaning period, inducing alterations in intestinal morphology, disturbing intestinal microbiota, and inducing growth retardation, especially diarrhea brought by the invasion of pathogenic bacteria, particularly enterotoxigenic Escherichia coli (ETEC) (1–3). ETEC postweaning diarrhea, also known as postweaning enteric diarrhea, is a type of diarrhea that occurs during the weaned period (4, 5). Colibacillosis is a major cause of mortality and economic losses in global nursery pigs’ production (6). Thus, it is critical to identify a practical solution to alleviate the ETEC K88-induced intestinal disruption in weaned piglets.
Lactobacillus reuteri (L. reuteri) strains are critical parts of the commensal microbiota in the intestines of piglets that can influence the immune system development (7, 8). L. reuteri has been found to produce lactic acid, hydrogen peroxide (9, 10), and reutericyclin (11), which have antibacterial properties against some intestinal pathogens, such as E. coli. The intestinal immune system serves as an immunological barrier to improve mucosal barrier function (12). L. reuteri has been shown to regulate innate immune responses and intestinal barrier function (13, 14). A previous study reported that oral administration of L. reuteri I5007 enhanced T-cell differentiation and induced ileal cytokine production (15). Liu et al. found that L. reuteri DSM 17938 feeding of healthy newborn mice regulates immune responses while modulating gut microbiota and boosting beneficial metabolites (14). Previously, our group isolated L. reuteri LR1 from the feces of healthy weaned piglets, and its 16S rRNA sequence was submitted in the GenBank database (No. KT205306), and indicated that L. reuteri LR1 strain improved the intestinal epithelial barrier function (3, 16, 17). However, the underlying mechanisms of L. reuteri LR1 that regulate intestinal integrity and inflammatory response following challenge with ETEC K88 are still unknown. Myosin light chain kinase (MLCK) is a Ca2+ calmodulin-dependent serine/threonine kinase that constantly modulates actomyosin reorganization and cell contraction (18). Several studies reported that MLCK is involved in intestinal epithelial regulation, inflammation, and gastrointestinal disorders (18–20). It would be of great interest to focus on whether L. reuteri LR1 could prevent intestinal injury and inflammation induced by ETEC K88 via regulating the MLCK signaling pathway.
The present study aimed to determine the effects of LR1 on the ETEC K88-induced intestinal epithelial injury on the inflammatory response, intestinal epithelial barrier function, and the MLCK signal pathway and its underlying mechanism. The connection between the inflammatory response, intestinal epithelial barrier function, and the MLCK signal pathway provides insight into the development of nutritional strategies in the prevention of intestinal inflammation.
Material and Methods
Bacterial Strains and Growth Conditions
According to our previous reports (3, 16, 17), L. reuteri 1 (LR1) was isolated from the feces of a healthy 35-day-old weaned piglet and stored at our laboratory. The L. reuteri 1 strain was cultured overnight at 37°C in de Man Rogosa Sharpe (MRS) broth for 0 h, 4 h, 8 h, 12 h, 16 h, 20 h, and 24 h (Solarbio, Beijing, China). The ETEC K88 (ETEC) strain was cultivated in Luria-Bertani (LB) broth (Solarbio, Beijing, China) for 0 h, 4 h, 8 h, 12 h, 16 h, 20 h, and 24 h at 37°C with shaking at 170 rpm. The bacterial cultures were harvested by centrifugation for 5 min at 8,000 rpm and washed once with PBS. The bacteria were counted by dilution coating plate method and detected absorbance at OD600nm at 0 h, 4 h, 8 h, 12 h, 16 h, 20 h, and 24 h (Bio-Tek, Winooski, USA).
Cell Culture
IPEC-J2 was cultivated in DMEM/F-12 (Gibco) with 10% fetal bovine serum (Gibco) under a humidified atmosphere containing 5% CO2. Cells were tested negative for mycoplasma contamination before use. IPEC-J2 cells were treated with LR1 (1 × 109 CFU/ml) for 4 h followed by treatment with ETEC K88 (1 × 108 CFU/ml) groups for 4 h. To minimize the deviation, the control group and the other treatments had a consistent cell growth time. Briefly, as shown in Supplementary Figure S1, the IPEC-J2 cells (1×105 cells per well) were seeded in 12-well plates (Corning, New York, USA) for 24 h. The LR1+ETEC K88 group was pre-treated by LR1 (1 × 109 cfu/ml) for 4 h firstly, followed by ETEC K88 (1 × 108 cfu/ml) for 4 h. Then, simultaneously, the control group, LR1 group, and ETEC K88 group were treated accordingly for 4 h. To ensure the same CFU of bacteria, we calculated CFU by the dilution coating plate method in each experiment. To knock down the MLCK gene, the IPEC-J2 cells were transfected with MLCK small interfering RNA (siRNA) (50 nM) were performed by Lipofectamine™ 3000 Transfection Reagent (Thermo Fisher Scientific, USA) for 24 h. The si NC sequence is 5’-ACGUGACACGUUCGGAGAATT-3’, and the sequence of siRNA target MLCK 1 gene is 5’-AACCAGGGUGAACACGUCCTT-3’, and that of si MLCK 2 is 5’-UUGGUGCUCACCUUCUUGCTT-3’.
Cell Viability
The IPEC-J2 cells (1 × 104 cells per well) were seeded in 96-well plates (Corning, New York, USA) and treated with 1 × 105, 1 × 106, 1 × 107, 1 × 108, and 1 × 109 cfu/ml LR1 or ETEC K88. The cell viability was detected by CCK8 assay according to the manufactured protocol (Beyotime, Shanghai, China). Then, IPEC-J2 cells were incubated with 10 μl of CCK8 for 2 h (Beyotime, Shanghai, China). Absorbance at OD450 nm was detected by a fluorescence microplate reader (Bio-Tek, Winooski, USA).
Intestinal Epithelial Barrier Function
The intestinal epithelial barrier function assay was detected according to our previous reports (21, 22). Briefly, to determine the effect of LR1 and ETEC K88 on the integrity of the IPEC-J2 cell monolayers, the 1 × 105 IPEC-J2 cells/ml were seeded in the Transwell [12-mm-diameter inserts with a 0.4-μm pore size were from Corning, and the transepithelial electrical resistance (TER) was measured by the Millicell-ERS resistance system; Millipore, Bedford, MA]. Additionally, the 500 μg/ml FD4 was added to the upper layer of Transwell for 1.5 h and gathered 100 μl of cell culture medium to detect the FD4 fluorescence using a fluorescence microplate reader (Bio-Tek, Winooski, USA).
Scanning Electron Microscope (SEM)
We seeded 1 × 105 IPEC-J2 on sterile cover glass in a 12-well cell plate. After being treated with the corresponding treatments, the cells were washed gently with PBS, followed by adding glutaraldehyde in a 12-well cell plate. After fixing for 2 h at room temperature, transfer the petri dish to 4°C. Wash tissue blocks 3 times with 0.1 M PBS for 15 min each. Then, transfer tissue blocks into 1% OsO4 in 0.1 M PBS for 1–2 h at room temperature. After that, wash cell blocks 3 times in 0.1 M PBS for 15 min each. Furthermore, the cells were washed with 30% ethanol for 15 min, 50% ethanol for 15 min, 70% ethanol for 15 min, 80% ethanol for 15 min, 90% ethanol for 15 min, and 95% ethanol for 15 min. There were two changes of 100% ethanol for 15 min. Finally, the samples were transferred into isoamyl acetate for 15 min. Specimens are attached to metallic stubs using carbon stickers and sputter-coated with gold for 30 s. The samples were observed and images were taken with the scanning electron microscope.
Wound Healing Assay
The IPEC-J2 wound healing assay was conducted as previously described (23). Briefly, IPEC-J2 cells were grown in 6-well dishes and the IPEC-J2 monolayer was scratched in a straight line with a p200 pipet tip. The figures were captured with a phase-contrast microscope at the time of scraping and after the corresponding treatment. The percentage of wound closure was calculated using ImageJ. All experiments were repeated 3 times.
qPCR
Total RNA was extracted from the cell’s samples by Trizol reagent (Invitrogen, Carlsbad, CA). The amount of RNA extracted was determined, and its purity was verified using NanoDrop 1000 (Thermo Fisher Scientific). The cDNA was performed with reagents from EZB Biosystems following conventional protocols. Samples were normalized to GAPDH, and the relative changes of target gene expression were analyzed by the 2−ΔΔCt method. The primers are shown in Table S1.
Western Blot
IPEC-J2 cells were rinsed twice with ice-cold PBS and lysed by RIPA buffer (Invitrogen, USA) containing protease inhibitors, phosphatase inhibitor, and PMSF (Beyotime, Shanghai, China). The supernatant was collected, and a BCA protein assay kit (Beyotime, Shanghai, China) was used to quantify protein concentration. Cell supernatants were resolved on 10% SDS-PAGE gels (Beyotime, Shanghai, China), transferred on PVDF membranes (Millipore), and incubated with the corresponding primary antibodies overnight at 4°C (Claudin-1: ER1906-37, 1:1,000, Huabio; Occludin: ab31721, 1:1,000, Abcam; IL-17A: ER1902-37, 1:1,000, Huabio; beta-actin: 4967, 1:1,000, Cell Signaling). After multiple washing with TBST, the membranes were incubated with secondary antibodies (1:5,000, Huabio, Hangzhou, China) at room temperature for 1 h. Detection was performed by enhanced ECL chemiluminescence (Beyotime, Shanghai, China) and captured by Imaging System. The bands’ intensity was analyzed by ImageJ.
Data Analysis
Values are given as means ± SEM. Student’s unpaired t-test was used to determine statistical significance among each of the two groups. The difference was considered to be significant at p < 0.05.
Results
Effects of L. reuteri LR1 on Cell Viability and Inflammatory Cytokine Expression in IPEC-J2 Cells
The growth curve of LR1 is presented in Figure 1A. We optimized the growth condition of LR1 according to the logarithmic phase of LR1. The LR1 strain was cultured overnight at 37°C in MRS for 16 h and collected to treat cells. Figure 1B shows that 1 × 109 cfu/ml LR1 treatment for 3 h, 4 h, 5 h, and 6 h significantly enhanced IPEC-J2 cell viability compared with the control group (p < 0.05). Furthermore, compared with the control group, the 1 × 109 cfu/ml LR1 treatment for 4 h dramatically decreased the IL-8 and IL-6 expression (Figures 1C, D). Thus, in the subsequent experiments, we choose 1 × 109 cfu/ml LR1 pretreatment for 4 h to conduct further experiments.
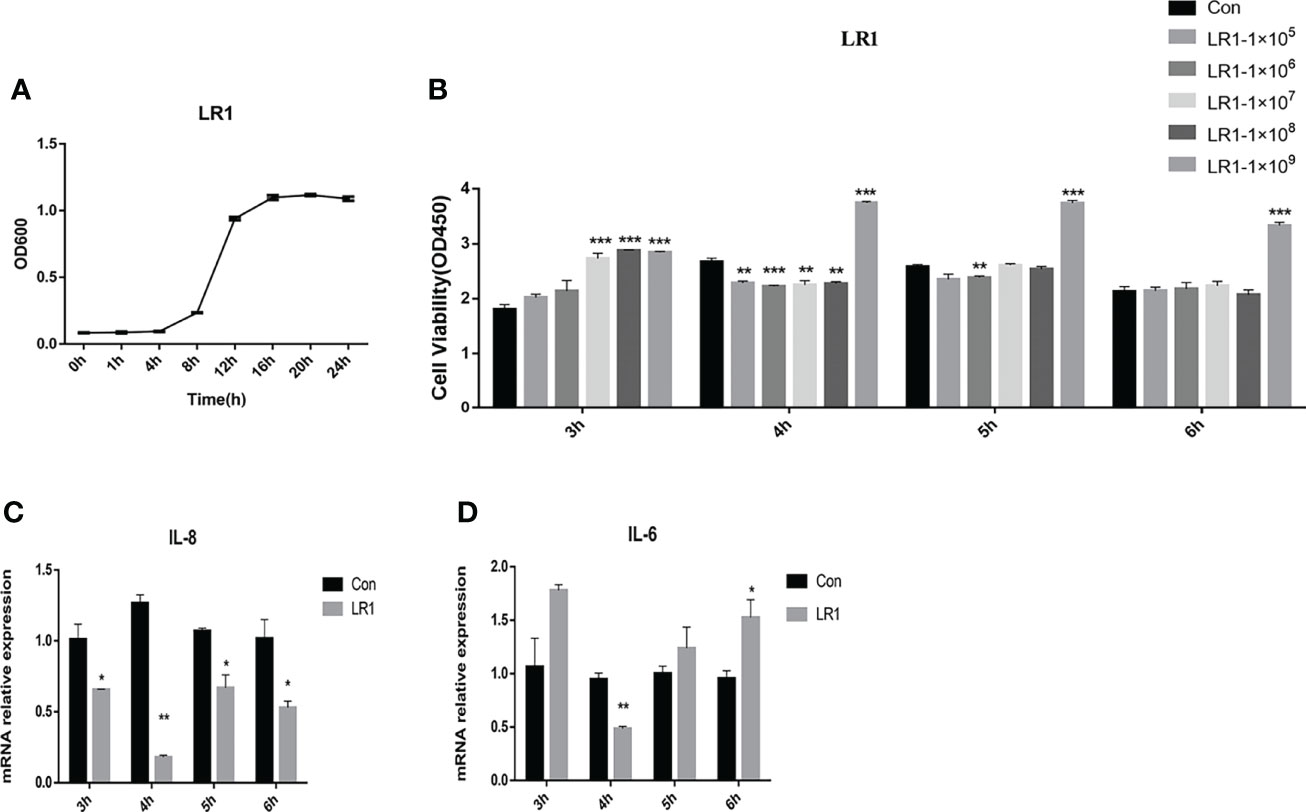
Figure 1 Effects of L. reuteri LR1 on cell viability and inflammatory cytokine expression in IPEC-J2 cells. (A) The growth curve of L. reuteri LR1. (B) The effects of different levels of L. reuteri LR1 on the viability of IPEC-J2 treated for 3 h, 4 h, 5 h, and 6 h. (C) The relative gene expression of IL-8 after being treated with 1 × 109 cfu/ml L. reuteri LR1 for 3 h, 4 h, 5 h, and 6 h. (D) The relative gene expression of IL-6 after being treated with 1 × 109 cfu/ml L. reuteri LR1 for 3 h, 4 h, 5 h, and 6 h. Data presented as mean ± SEM (n ≥ 3). *p < 0.05, **p < 0.01, and ***p < 0.001 vs. the control group.
Effects of ETEC K88 on Cell Viability and Inflammatory Cytokine Expression in IPEC-J2 Cells
The growth curve of ETEC K88 is presented in Figure 2A. We optimized the growth condition of ETEC K88 according to the logarithmic phase of ETEC K88. The ETEC K88 strain was cultured overnight at 37°C in LB for 8 h and collected to treat cells. Figure 2B shows that 1 × 108 cfu/ml ETEC K88 treatment for 4 h, 5 h, and 6 h significantly declined the IPEC-J2 cell viability compared to the control group (p < 0.05). Additionally, compared with the control group, the 1 × 108 cfu/ml ETEC K88 treatment for 4 h dramatically enhanced the IL-8, IL-6, and TNF-α expression (p < 0.05) (Figures 2C, D). Thus, in the subsequent experiments, we choose 1 × 108 cfu/ml ETEC K88 treatment for 4 h to perform further experiments.
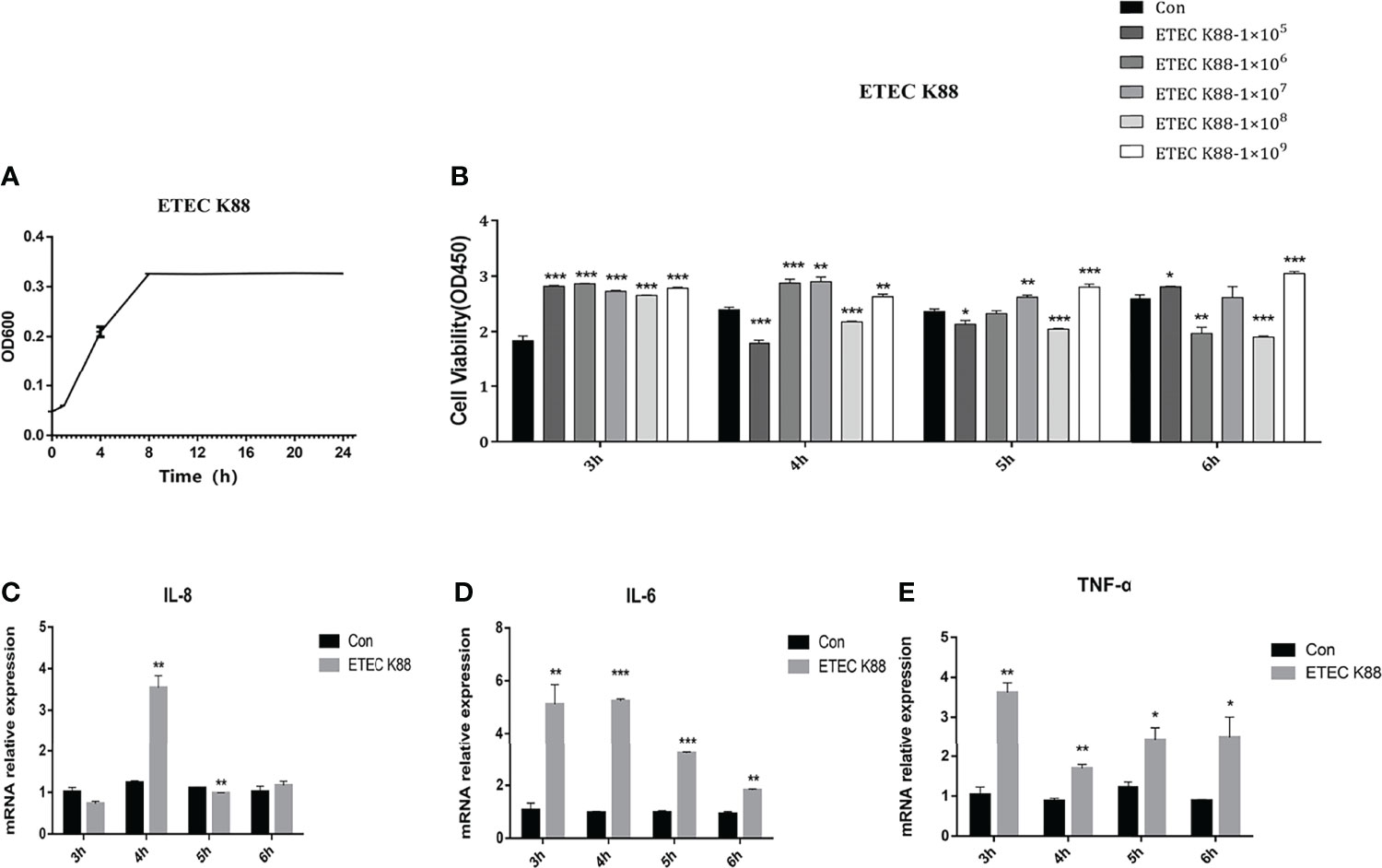
Figure 2 Effects of ETEC K88 on cell viability and inflammatory cytokine expression in IPEC-J2 cells. (A) The growth curve of ETEC K88. (B) The effects of different levels of ETEC K88 on the viability of IPEC-J2 treated for 3 h, 4 h, 5 h, and 6 h. (C) The relative gene expression of IL-8 after being treated with 1 × 108 cfu/ml ETEC K88 for 3 h, 4 h, 5 h, and 6 h. (D) The relative gene expression of IL-6 after being treated with 1 × 108 cfu/ml L. reuteri LR1 for 3 h, 4 h, 5 h, and 6 h. (E) The relative gene expression of TNF-α after being treated with 1 × 108 cfu/ml L. reuteri LR1 for 3 h, 4 h, 5 h, and 6 h. Data presented as mean ± SEM (n ≥ 3). *p < 0.05,**p < 0.01, and ***p < 0.001 vs. the control group.
Effects of L. reuteri LR1 on Cell Adherence and Wound Healing in IPEC-J2 Cells Treated With ETEC K88
We utilized the SEM to detect the adherence of LR1 and ETEC K88 on IPEC-J2. We found that pretreatment with LR1 inhibited the ETEC K88 that adhered on IPEC-J2 (Figure 3A). Furthermore, compared with the control group, the scratch edge of IPEC J2 cells retreated after ETEC K88 treatment. Compared with the ETEC K88 group, pretreatment with LR1 alleviated the scratch injury of IPEC J2 cells (p < 0.05) (Figure 3B).
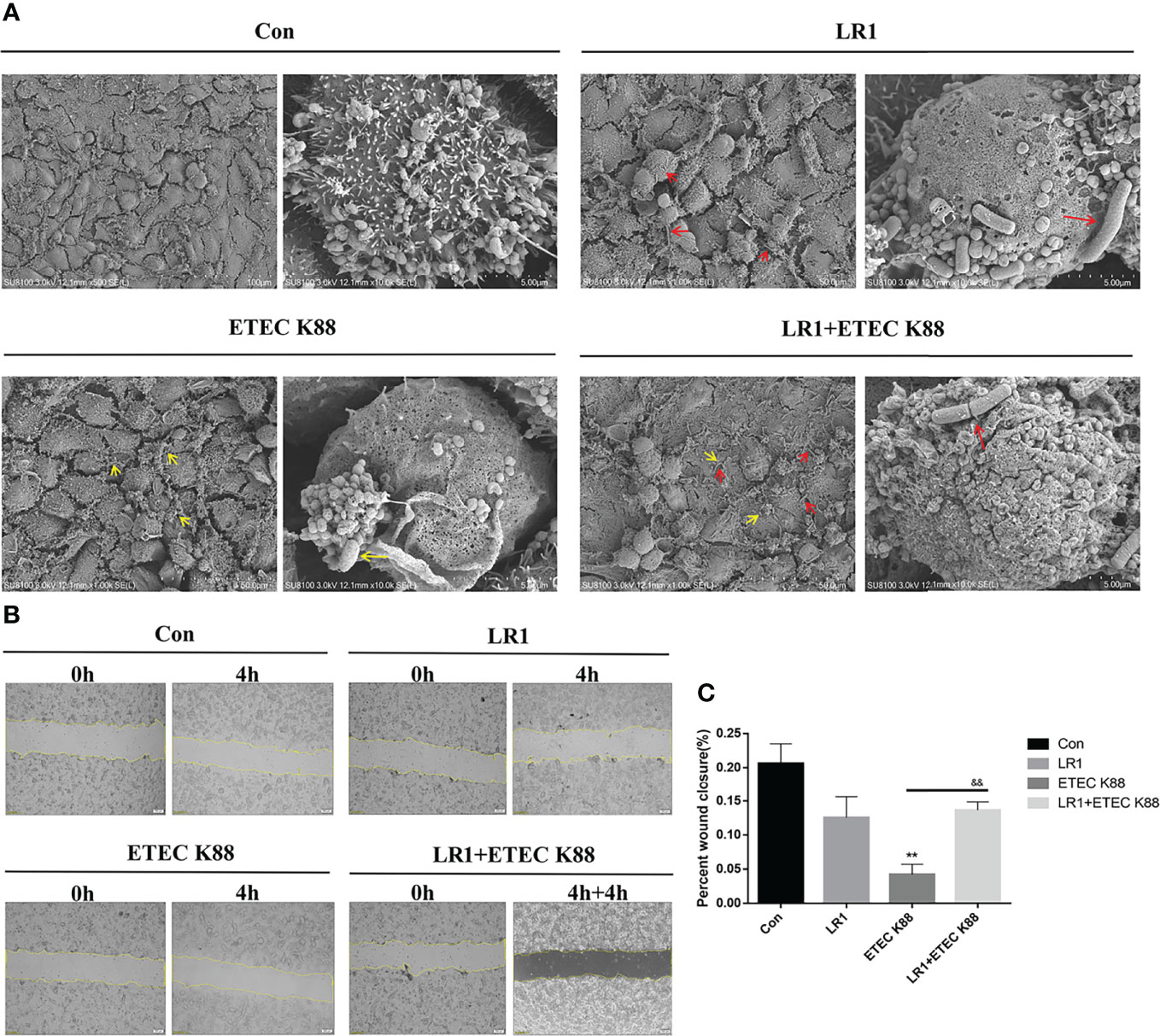
Figure 3 Effects of L. reuteri LR1 on cell adherence and wound healing in IPEC-J2 cells treated with ETEC K88. (A) The scanning electron microscope (SEM) of IEPC-J2 cells pretreated with L. reuteri LR1 (1 × 109 cfu/ml) for 4 h followed by ETEC K88 (1 × 108 cfu/ml) challenged for 4 h. (B) L. reuteri LR1 promotes cell motility as shown by in vitro scratch assays in IPEC-J2 cells challenged with ETEC K88. Live-cell images were taken right after the “scratches” were created (0 h) and at 8 h post-treatment (or not) of L. reuteri LR1 (1 × 109 cfu/ml) and ETEC K88 (1 × 108 cfu/ml). (C) Quantification of percent wound closure was performed by detecting the decline in the denuded area at 8 h. Cells were treated with L. reuteri LR1 (1 × 109 cfu/ml) for 4 h, and then followed by ETEC K88 (1 × 108 cfu/ml) for 4 h. Data presented as mean ± SEM (n = 3). **p < 0.01 vs. the control group, &&p < 0.01 vs. the ETEC K88 group. Red arrows in “LR1 and LR1+ETEC K88” represent Lactobacillus reuteri 1 (LR1), and yellow arrows in “ETEC K88 and LR1+ETEC K88” represent E. coli (ETEC K88).
Effects of L. reuteri LR1 on Intestinal Epithelial Barrier Function and Tight Junction Protein Expression in IPEC-J2 Cells Treated With ETEC K88
We measured the TER, FD4 flux, and tight junction protein expression by Western blotting to investigate the effects of LR1 on intestinal epithelial barrier function in IPEC-2 cells. As shown in Figure 4A, compared with the control group, ETEC K88 treatment significantly reduced the TER of IPEC J2 cells, and LR1 pretreatment significantly increased the TER after ETEC K88 treatment (p < 0.05), while, as presented in Figure 4B, in comparison with the control group, ETEC K88 treatment significantly enhanced the FD4 flux of IPEC J2 cells, and LR1 pretreatment significantly declined the FD4 flux after ETEC K88 treatment (p < 0.05). Furthermore, ETEC K88 treatment significantly declined the Claudin-1 expression compared to the control group. Additionally, LR1 pretreatment dramatically enhanced the Claudin-1 expression compared with the ETEC K88 group (p < 0.05) (Figures 4C, D). Consistently, LR1 pretreatment reversed the declined mRNA levels of Occludin and Claudin-1 induced by ETEC K88 challenge (p < 0.05) (Figures 4C, D).
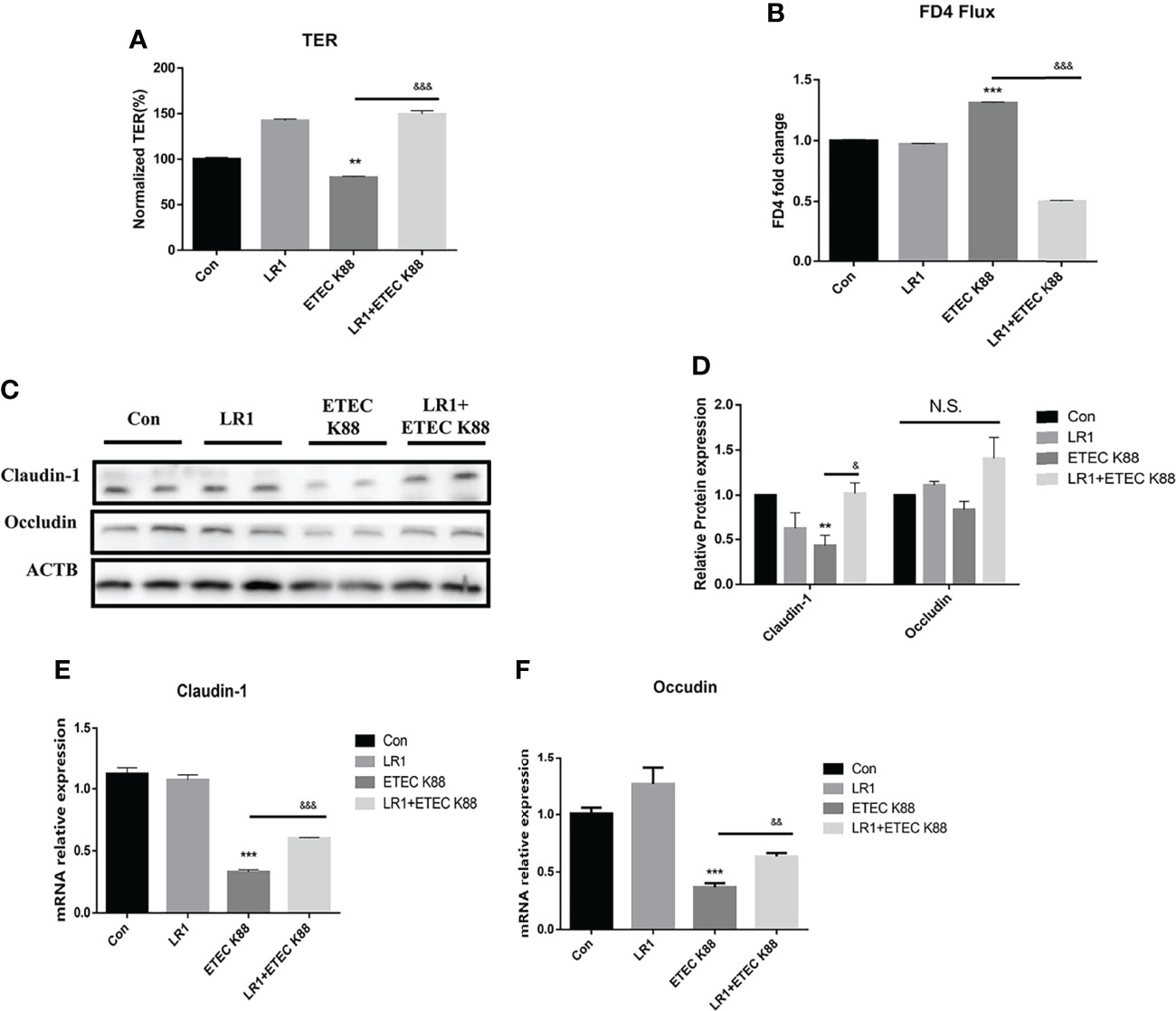
Figure 4 Effects of L. reuteri LR1 on intestinal epithelial barrier function and tight junction protein expression in IPEC-J2 cells treated with ETEC K88. (A) The effect of L. reuteri LR1 (1 × 109 cfu/ml) on transepithelial electrical resistance (TER) in IPEC-J2 cells treated with ETEC K88 (1 × 108 cfu/ml). (B) The effect of L. reuteri LR1(1 × 109 cfu/ml) on FD4 flux in IPEC-J2 cells treated with ETEC K88 (1 × 108 cfu/ml). (C) The effect of L. reuteri LR1 (1 × 109 cfu/ml) on tight junction protein expression in IPEC-J2 cells treated with ETEC K88 (1 × 108 cfu/ml). (D) Quantification of tight junction protein expression. (E) The effect of L. reuteri LR1 (1 × 109 cfu/ml) on Claudin-1 gene expression in IPEC-J2 cells treated with ETEC K88 (1 × 108 cfu/ml). (F) The effect of L. reuteri LR1 (1 × 109 cfu/ml) on Occludin gene expression in IPEC-J2 cells treated with ETEC K88 (1 × 108 cfu/ml). Cells were treated with L. reuteri LR1 (1 × 109 cfu/ml) for 4 h, and then followed by ETEC K88 (1 × 108 cfu/ml) for 4 h. Data presented as mean ± SEM (n = 3). **p < 0.01 and ***p < 0.001 vs. the control group, &&p < 0.01 and &&&p < 0.001 vs. the ETEC K88 group. N.S., not significant.
Effects of L. reuteri LR1 on Inflammatory Cytokine Expression and MLCK Signal Pathway Expression in IPEC-J2 Cells Treated With ETEC K88
We recollected the samples and measured the IL-6, IL-8, and TNF-α level to investigate the effects of LR1 on inflammatory cytokine expression. As shown in Figure 5A, compared with the control group, IL-6 and IL-8 levels were declined in IPEC-J2 after LR1 treatment, while IL-17A and TNF-α levels were not influenced by LR1 treatment in IPEC-J2 (p < 0.05) (Figure 5A). Furthermore, in comparison with the control group, ETEC K88 treatment significantly enhanced the IL-6, IL-8, IL-17A, and TNF-α expression in IPEC-J2 (p < 0.05) (Figure 5A). Meanwhile, compared with ETEC K88 group, LR1 pretreatment dramatically declined IL-6, IL-8, and TNF-α levels in IPEC-J2 treated with ETEC K88 (p < 0.05) (Figure 5A). Consistently, previous studies reported that LR alleviated inflammation by reducing the production of pro-inflammatory cytokines. Moreover, ETEC K88-treated IPEC-J2 cells had a higher MLCK level, higher MLC levels, and lower ROCK levels than the control group (p < 0.05) (Figure 5B), while LR1 pretreatment significantly declined MLCK and MLC expression and enhanced ROCK level, in comparison with the ETEC K88 group (p < 0.05) (Figure 5B).
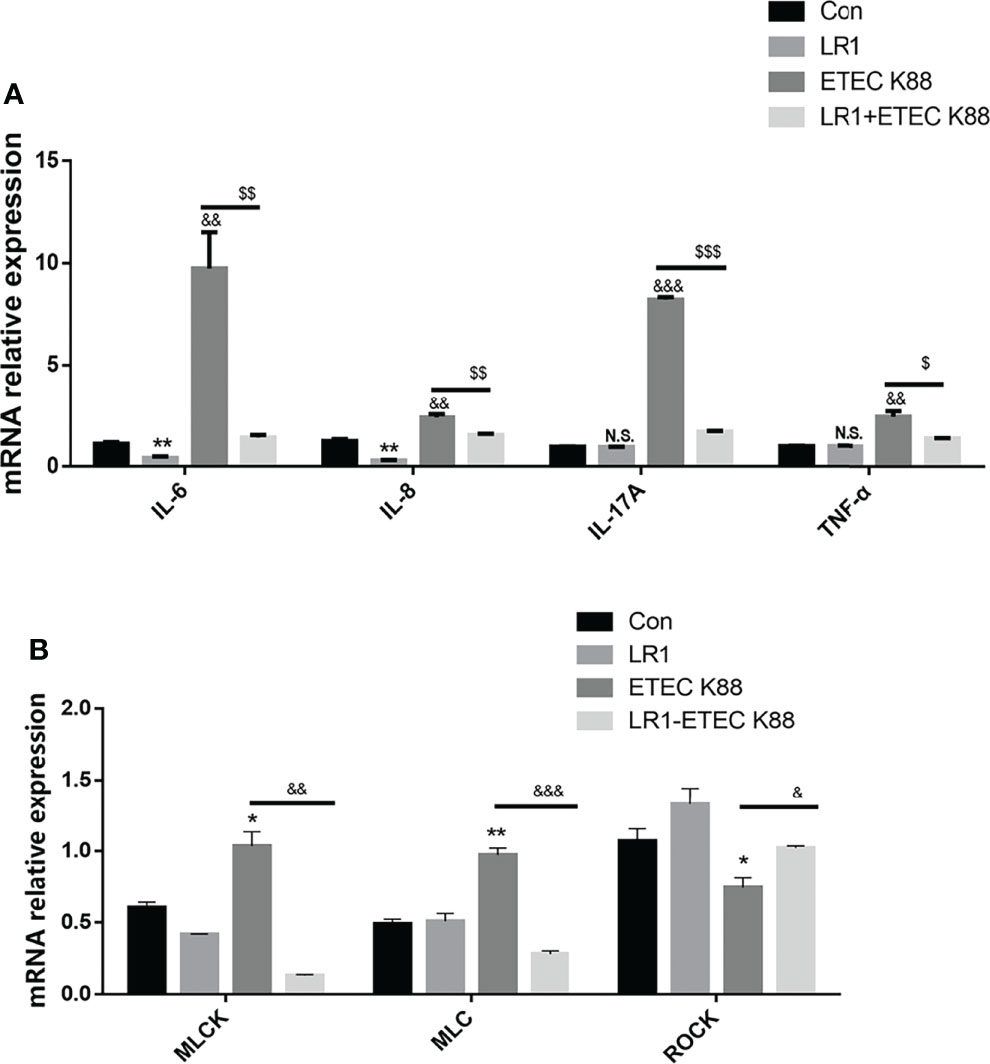
Figure 5 Effects of L. reuteri LR1 on inflammatory cytokine expression and MLCK signal pathway expression in IPEC-J2 cells treated with ETEC K88. (A) The effects of L. reuteri LR1 (1 × 109 cfu/ml) on IL-8, IL-17A, IL-6, and TNF-α gene expression in IPEC-J2 cells treated with ETEC K88 (1 × 108 cfu/ml). Cells were treated with L. reuteri LR1 (1 × 109 cfu/ml) for 4 h, and then followed by ETEC K88 (1 × 108 cfu/ml) for 4 h. Data presented as mean ± SEM (n = 3). (B) The effects of L. reuteri LR1 (1 × 109 cfu/ml) on MLCK, MLC, and ROCK gene expression in IPEC-J2 cells treated with ETEC K88 (1 × 108 cfu/ml). Cells were treated with L. reuteri LR1 (1 × 109 cfu/ml) for 4 h, and then followed by ETEC K88 (1 × 108 cfu/ml) for 4 h. Data presented as mean ± SEM (n = 3). *p < 0.05, **p < 0.01, and ***p < 0.001 vs. the control group; &p < 0.05, &&p < 0.01, and &&&p < 0.001 vs. the control group; $p < 0.05, $$p < 0.01, and $$$p < 0.001 vs. the ETEC K88 group. N.S., not significant.
Knockdown of MLCK Promotes the Effects of L. reuteri LR1 on the Inflammatory Response and Barrier Function in IPEC-J2 Cells Treated With ETEC K88
To elucidate whether LR1 plays a protective role through the regulation of the MLCK signal pathway, we used the siRNA to knock down the MLCK gene in IPEC-J2 cells (Figure 6A). Interestingly, we found that depletion of MLCK significantly declined MLC expression in IPEC-J2 challenged with ETEC K88 than the si NC+ETEC K88 group (p < 0.05) (Figure 6B). Furthermore, in comparison with the si NC+LR1+ETEC K88 group, depletion of MLCK significantly declined MLCK and MLC expression (p < 0.05) (Figure 6B). The TER of IPEC-J2 in the si MLCK+ETEC K88 group was higher and the FD4 flux of IPEC-J2 in the si MLCK+ETEC K88 group was lower compared with the si NC+ETEC K88 group (p < 0.05) (Figures 6C, D), while there is no significant difference in TER and FD4 permeability between the si NC+LR1+ETEC K88 group and the si MLCK+LR1+ETEC K88 group (P > 0.05) (Figures 6C, D). Compared with the si NC+ETEC K88 group, the si MLCK+ETEC group had a higher expression of Claudin-1 and Occludin (p < 0.05) (Figures 6E, F). These data revealed that knockdown of the MLCK inhibited the intestinal barrier dysfunction induced by ETEC K88 challenge. Consistently, depletion of MLCK enhanced Claudin-1 level in comparison to the si NC+LR1+ETEC K88 group (p < 0.05) (Figures 6E, F). We added the inflammatory cytokine expression data in MLCK Knockdown IPEC-J2 (Supplementary Figure S3). We found that depletion of MLCK significantly declined IL-8 and TNF-α expression in IPEC-J2 challenged with ETEC K88 compared to the si NC+ETEC K88 group (p < 0.05) (Supplementary Figure S3). Furthermore, in comparison with the si NC+LR1+ETEC K88 group, depletion of MLCK significantly declined IL-8 and IL-6 expression (p < 0.05), suggesting that the MLCK signal pathway is involved in immune pathogenesis after being treated with ETEC K88 (Supplementary Figure S3).
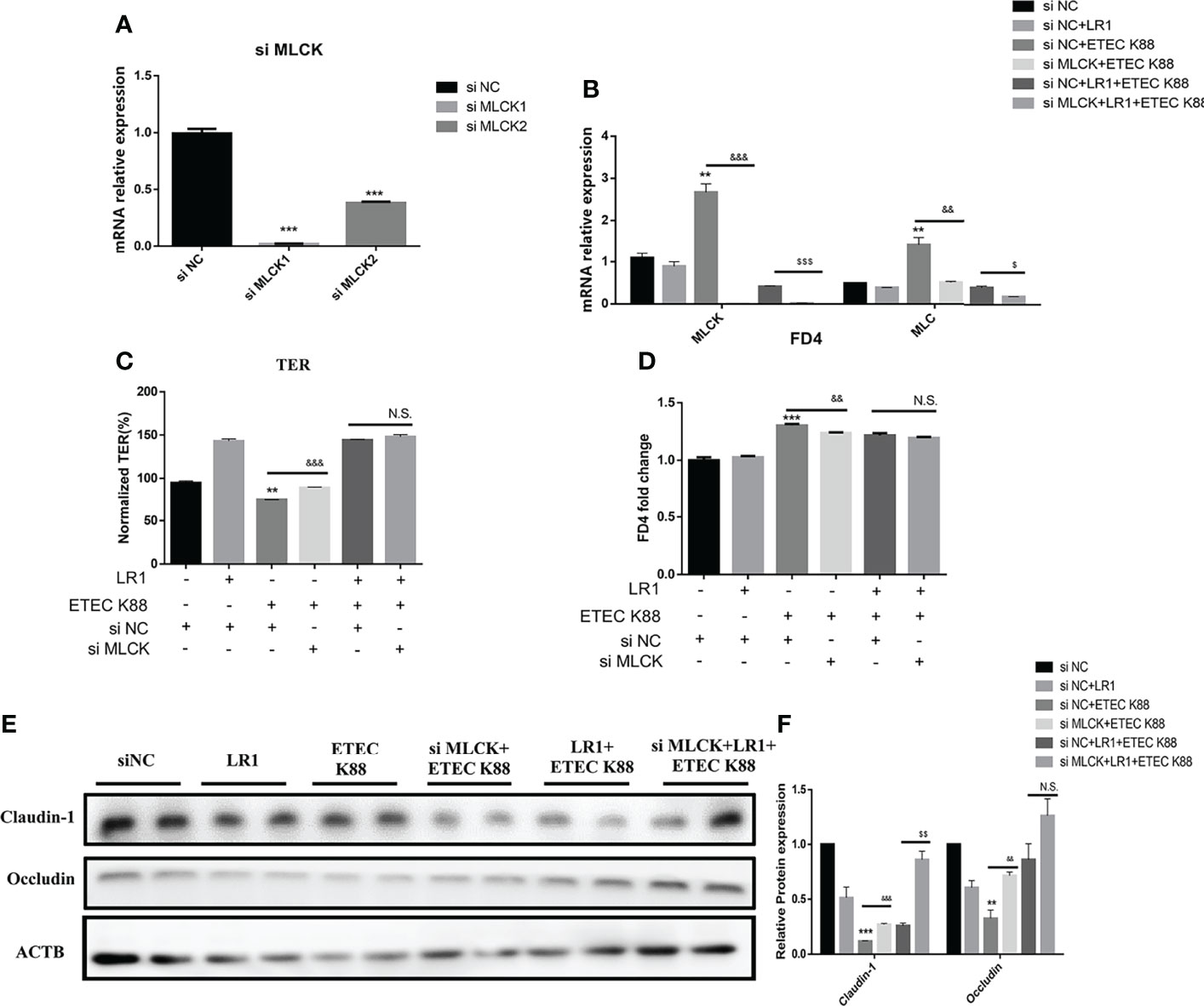
Figure 6 Knockdown MLCK promotes the effects of L. reuteri LR1 on the inflammatory response and barrier function in IPEC-J2 cells treated with ETEC K88. (A) Relative expression of MLCK in IPEC-J2 after being treated with si Negative Control (si NC), si MLCK 1, and si MLCK 2. (B) Gene expression of MLCK and MLC determined by qPCR in IPEC-J2 cells transfected with negative control siRNA or siRNA targeting MLCK. (C) TER of IPEC-J2 cells transfected with negative control siRNA or siRNA targeting MLCK. (D) FD4 flux of IPEC-J2 cells transfected with negative control siRNA or siRNA targeting MLCK. (E) Protein expression of Occludin and Claudin-1 determined by Western blotting in IPEC-J2 cells transfected with control siRNA or siRNA targeting MLCK. (F) Quantification of protein expression of Occludin and Claudin-1. Cells were treated with L. reuteri LR1 (1 × 109 cfu/ml) for 4 h, and then followed by ETEC K88 (1 × 108 cfu/ml) for 4 h. siRNA transfection for 24 h before all treatments. Data presented as mean ± SEM (n = 3). **p < 0.01 and ***p < 0.001 vs. the control group, &&p < 0.01 and &&&p < 0.001 vs. the ETEC K88 group, $$p < 0.01 vs. the siNC+LR1+ETEC K88 group. N.S., not significant.
Discussion
Intestinal epithelial barrier injury disrupts immune homeostasis and leads to many intestinal disorders (24–26). L. reuteri strains are important members of the commensal microbiota in the intestines of piglets, which can influence the immune system development and intestinal barrier (7, 8). However, the effects of L. reuteri LR1 on intestinal integrity and inflammatory response and the underlying mechanisms are yet unknown. Thus, in this study, we determined the effects of LR1 on the ETEC K88-induced intestinal epithelial injury on the inflammatory response, intestinal epithelial barrier function, and the MLCK signal pathway and its underlying mechanism.
Several lines of evidence revealed that L. reuteri has the potential to reduce mucosal leakage and protect the cell against death induced by stressors (27, 28). Xie et al. reported that L. reuteri stimulates intestinal epithelial proliferation and induces differentiation into goblet cells in young chickens (27). Wu et al. found that L. reuteri helps to maintain intestinal epithelium renewal and homeostasis and heals pathological damage (28). Our results showed that 1 × 109 cfu/ml LR1 treatment significantly enhanced IPEC-J2 cell viability compared with the control group. Cytokines, which modulate intestinal mucosal inflammation and epithelial integrity, are known to be involved in the pathology development of inflammatory bowel disease (29–31). Wang et al. reported that L. reuteri can ameliorate intestinal inflammation in dextran sulfate sodium-induced colitis in mice, indicated by decreased levels of the proinflammatory cytokines IFN-γ, TNF-α, IL-1β, IL-6, and IL-17A in the colon tissue and serum of mice (32). The present study demonstrated that the 1 × 109 cfu/ml LR1 treatment for 4 h dramatically decreased the IL-8 and IL-6 expression.
Many studies reported that L. reuteri had a strong pathogen inhibition effect through microbe interactions, inhibiting the adhesion of pathogenic bacteria, competition for nutrients, and binding sites (33, 34). Zhang et al. reported that L. reuteri adhered to IPEC-J2 and Caco-2 cells via glyceraldehyde-3-phosphate dehydrogenase (35). Walsham et al. indicated that L. reuteri can bind to the intestinal layer that led to declined enteropathogenic E. coli adherence to small intestinal biopsy epithelium (34). Consistently, we utilized the SEM to detect the adherence of LR1 and ETEC K88 on IPEC-J2, and we found that pretreatment with LR1 inhibited the ETEC K88 that adhered on IPEC-J2. Some studies reported that L. reuteri could promote cell renewal and wound healing (36, 37). In this study, the function of IPEC-J2 cell migration and the ability of wound healing were estimated by the scratch-simulated wound migration assay. Our data indicated that compared with the ETEC K88 group, pretreatment with LR1 alleviated the scratch injury of IPEC J2 cells. These data suggested that LR1 pretreatment could inhibit ETEC K88 adhesion and promote cell migration in the ETEC K88-challenged model.
The gut epithelium serves as the most important barrier to prevent endogenous and exogenous harmful antigens and pathogens, and the tight junction is a basis for intestinal barrier function (26, 38). In this study, the tight junction of intestinal epithelial cells and the paracellular permeability of the gut were indirectly reflected by the TER and FD4 flux (21, 22). The data indicated that LR1 pretreatment significantly reversed the declined TER and tight junction protein level and enhanced the induction by ETEC K88 treatment. Our previous study reported that LR1 supplementation at 5 × 1010 cfu/kg could improve the growth performance, intestinal morphology, and intestinal barrier function in weaned pigs (17). Similarly, Li et al. indicated that L. reuteri improves gut barrier function and affects the diurnal variation of the gut microbiota in mice fed a high-fat diet (39). Cui et al. reported that L. reuteri ZJ617 maintains intestinal integrity via regulating tight junction, autophagy, and apoptosis in mice challenged with lipopolysaccharide (40). Several studies reported that there is a strong connection between the enhanced proinflammatory cytokine level and gut integrity damage (25, 41). Several lines of evidence revealed that the inflammatory response of intestinal epithelial cells is closely related to the expression of inflammatory cytokines (42, 43). Our data found that LR1 pretreatment dramatically declined the proinflammatory cytokines IL-8, IL-17A, IL-6, and TNF-α levels compared with the ETEC K88 group. Consistently, previous studies reported that LR alleviated inflammation by reducing the production of pro-inflammatory cytokines. Tang et al. found that LR treatment significantly decreased IL-1b, IL-6, TNF-α, and IFN-γ expression in the jejunum of weaning piglets (44). Hsieh et al. reported that L. reuteri GMNL-263 decreased serum TNF-α and IL-6 levels in mice fed with a high-fat diet (45). Karimi et al. pointed out that pretreatment with L. reuteri decreased IL-6 and TNF-α levels, and enhanced a longer isoform of ZO-1 and maintained E-cadherin expression. The interleukin-17 (IL-17) family consists of a subset of cytokines that participate in both acute and chronic intestinal inflammatory responses (46). Though Th17 cells were thought as a major source of IL-17A, IL-17A can also be produced by other cell types (47). We detected the IL-17A protein levels in IPEC-J2 and used the mouse colonic tissue protein results as a positive control. As shown in Supplementary Figure S2A, we confirmed that IL-17A can be expressed in IPEC J2 cells (Supplementary Figure S2A). Consistently, Zhang et al. found that IL-17 can express in IPEC-J2 cells (48). Furthermore, Yu et al. found that TLR5-mediated IL-17C expression in IPEC-J2 enhances immune responses in the intestinal epithelium against ETEC infection (49). These data revealed that LR1 pretreatment could reverse the disrupted intestinal barrier function and inflammatory response induced by ETEC K88 challenge.
The MLCK signal pathway has been widely reported to play a critical function in regulating the tight junction proteins’ reorganization, via promoting the enhanced contraction and tonicity of actomyosin before tight junction (50, 51). Then, the activated MLCK signal pathway led to the tight junction disrupted arrangement and enhanced the intestinal paracellular permeability (20, 52, 53). Moreover, the MLCK signal pathway is involved in mediating pro-inflammatory cytokine expressions, such as IL-β, IL-6, and IL-8 (54, 55). Several studies reported that there is a strong connection between the enhanced proinflammatory cytokines’ level and the immune pathogenesis of intestinal inflammatory (29). Several lines of evidence revealed that E. coli is an important pathogenic bacteria to induce intestinal inflammatory diseases, indicated by the enhanced proinflammatory cytokine expression and cell damage (56). Su et al. found that targeted inhibition of intestinal epithelial MLCK may be therapeutically effective in immune-mediated inflammatory bowel disease, particularly in preventing reactivation of quiescent inflammatory bowel disease (57). Our data suggested that LR1 pretreatment reversed the enhanced MLCK and MLC expression and decreased ROCK level induced by ETEC K88-challenged IPEC-J2 cells. In addition, we also found that depletion of MLCK significantly enhanced Claudin-1 level and declined IL-8 and TNF-α level in IPEC-J2 pretreated with LR1 followed by challenging with ETEC K88. These results suggest that the inhibited MLCK signal pathway may be responsible for L. reuteri intestinal protection functions and alleviating inflammatory response in IPEC-J2 cells.
In conclusion, our work proposes that L. reuteri LR1 can improve intestinal epithelial barrier function and declined inflammatory response through suppressing the MLCK signal pathway. These results provide us with new insights into the protective effect of L. reuteri on the gut epithelial integrity and inflammation response, giving us a potential therapeutic function for the therapy of intestinal inflammatory diseases.
Data Availability Statement
The datasets presented in this study can be found in online repositories. The names of the repository/repositories and accession number(s) can be found below: Scan electron microscope (SEM) data has been uploaded to FigShare. The DOI number is https://doi.org/10.6084/m9.figshare.19880665.v1.
Author Contributions
Conceptualization: ZJ, SC, and LW. Methodology: JG and SC. Software: JG. Validation: HX, SH, KY, and KH. Formal analysis, SC and JG. Data curation: JG. Writing—original draft preparation: SC and JG. Writing—review and editing: LW. Visualization: SC. Supervision: ZJ and LW. Funding acquisition: ZJ, LW, and SC. All authors have reviewed and approved the final manuscript.
Funding
This study was supported by the National Natural Science Foundation of China (32172777), the China Postdoctoral Science Foundation (2021M700894), the Guangdong Basic and Applied Basic Research Foundation (2022A1515011406, 2021A1515110636), and the Science and Technology Program of Guangdong Academy of Agricultural Sciences (R2020PY-JG009, 202106TD).
Conflict of Interest
The authors declare that the research was conducted in the absence of any commercial or financial relationships that could be construed as a potential conflict of interest.
Publisher’s Note
All claims expressed in this article are solely those of the authors and do not necessarily represent those of their affiliated organizations, or those of the publisher, the editors and the reviewers. Any product that may be evaluated in this article, or claim that may be made by its manufacturer, is not guaranteed or endorsed by the publisher.
Acknowledgments
We thank the staff and postgraduate students of the Institute of Animal Science of Guangdong Academy of Agricultural Sciences for providing technical assistance.
Supplementary Material
The Supplementary Material for this article can be found online at: https://www.frontiersin.org/articles/10.3389/fimmu.2022.897395/full#supplementary-material
Supplementary Figure S1 | Diagram for cell treatments. The IPEC-J2 cells (1×105 cells per well) were seeded in 12-well plates (Corning, New York, USA) for 24h. And the LR1 + ETEC K88 group was pre-treated by LR1 (1 × 109 cfu/mL) for 4h firstly, followed by ETEC K88 (1 × 108 cfu/mL) for 4h. And then, simultaneously, the control group, LR1 group and ETEC K88 group were treated accordingly for 4h. Four independent experiments were conducted to verify the results and three replicates per group in every independent test.
Supplementary Figure S2 | IL-17A protein expression. (A) IL-17A proteins expression in mouse colon and IPEC-J2 cells.
Supplementary Figure S3 | Knockdown MLCK inhibition the effects of L. reuteri LR1 on the inflammatory response in IPEC-J2 Cells treated with ETEC K88. (A) Gene expression of IL-8 determined by qPCR in IPEC-J2 cells transfected with negative control siRNA or siRNA targeting MLCK. (B) Gene expression of TNF-α determined by qPCR in IPEC-J2 cells transfected with negative control siRNA or siRNA targeting MLCK. Data presented as mean ± SEM (n=3). ***p < 0.001 vs. Control group, ##p < 0.01 and ###p < 0.001 vs. ETEC K88 group, &p < 0.05 and &&p < 0.01 vs. siNC+LR1+ETEC K88 group.
References
1. Wang W, Ma H, Yu H, Qin G, Tan Z, Wang Y, et al. Screening of Lactobacillus Plantarum Subsp. Plantarum With Potential Probiotic Activities for Inhibiting ETEC K88 in Weaned Piglets. Molecules (2020) 25(19):4481. doi: 10.3390/molecules25194481
2. Liu G, Gu K, Wang F, Jia G, Zhao H, Chen X, et al. Tryptophan Ameliorates Barrier Integrity and Alleviates the Inflammatory Response to Enterotoxigenic Escherichia Coli K88 Through the CaSR/Rac1/PLC-Gamma1 Signaling Pathway in Porcine Intestinal Epithelial Cells. Front Immunol (2021) 12:748497. doi: 10.3389/fimmu.2021.748497
3. Yi H, Wang L, Xiong Y, Wang Z, Qiu Y, Wen X, et al. Lactobacillus Reuteri LR1 Improved Expression of Genes of Tight Junction Proteins via the MLCK Pathway in IPEC-1 Cells During Infection With Enterotoxigenic Escherichia Coli K88. Mediators Inflammation (2018) 2018:(6434910). doi: 10.1155/2018/6434910
4. Yang KM, Jiang ZY, Zheng CT, Wang L, Yang XF. Effect of Lactobacillus Plantarum on Diarrhea and Intestinal Barrier Function of Young Piglets Challenged With Enterotoxigenic Escherichia Coli K88. J Anim Sci (2014) 92(4):1496–503. doi: 10.2527/jas.2013-6619
5. Gonzalez-Ortiz G, Perez JF, Hermes RG, Molist F, Jimenez-Diaz R, Martin-Orue SM. Screening the Ability of Natural Feed Ingredients to Interfere With the Adherence of Enterotoxigenic Escherichia Coli (ETEC) K88 to the Porcine Intestinal Mucus. Br J Nutr (2014) 111(4):633–42. doi: 10.1017/S0007114513003024
6. Sun Y, Kim SW. Intestinal Challenge With Enterotoxigenic Escherichia Coli in Pigs, and Nutritional Intervention to Prevent Postweaning Diarrhea. Anim Nutr (2017) 3(4):322–30. doi: 10.1016/j.aninu.2017.10.001
7. Coccorullo P, Strisciuglio C, Martinelli M, Miele E, Greco L, Staiano A. Lactobacillus Reuteri (DSM 17938) in Infants With Functional Chronic Constipation: A Double-Blind, Randomized, Placebo-Controlled Study. J Pediatr (2010) 157(4):598–602. doi: 10.1016/j.jpeds.2010.04.066
8. Francavilla R, Lionetti E, Castellaneta S, Ciruzzi F, Indrio F, Masciale A, et al. Randomised Clinical Trial: Lactobacillus Reuteri DSM 17938 vs. Placebo in Children With Acute Diarrhoea–a Double-Blind Study. Aliment Pharmacol Ther (2012) 36(4):363–9. doi: 10.1111/j.1365-2036.2012.05180.x
9. Martinez RC, Seney SL, Summers KL, Nomizo A, De Martinis EC, Reid G. Effect of Lactobacillus Rhamnosus GR-1 and Lactobacillus Reuteri RC-14 on the Ability of Candida Albicans to Infect Cells and Induce Inflammation. Microbiol Immunol (2009) 53(9):487–95. doi: 10.1111/j.1348-0421.2009.00154.x
10. Sabet-Azad R, Sardari RR, Linares-Pasten JA, Hatti-Kaul R. Production of 3-Hydroxypropionic Acid From 3-Hydroxypropionaldehyde by Recombinant Escherichia Coli Co-Expressing Lactobacillus Reuteri Propanediol Utilization Enzymes. Bioresour Technol (2015) 180:(214–21). doi: 10.1016/j.biortech.2014.12.109
11. Hou C, Zeng X, Yang F, Liu H, Qiao S. Study and Use of the Probiotic Lactobacillus Reuteri in Pigs: A Review. J Anim Sci Biotechnol (2015) 6(1):14. doi: 10.1186/s40104-015-0014-3
12. Karimi S, Jonsson H, Lundh T, Roos S.. Lactobacillus Reuteri Strains Protect Epithelial Barrier Integrity of IPEC-J2 Monolayers From the Detrimental Effect of Enterotoxigenic Escherichia Coli. Physiol Rep (2018) 6(2):e13514. doi: 10.14814/phy2.13514
13. Wang T, Zheng N, Luo Q, Jiang L, He B, Yuan X, et al. Probiotics Lactobacillus Reuteri Abrogates Immune Checkpoint Blockade-Associated Colitis by Inhibiting Group 3 Innate Lymphoid Cells. Front Immunol (2019) 10:1235. doi: 10.3389/fimmu.2019.01235
14. Liu Y, Tian X, He B, Hoang TK, Taylor CM, Blanchard E, et al. Lactobacillus Reuteri DSM 17938 Feeding of Healthy Newborn Mice Regulates Immune Responses While Modulating Gut Microbiota and Boosting Beneficial Metabolites. Am J Physiol Gastrointest Liver Physiol (2019) 317(6):G824–G38. doi: 10.1152/ajpgi.00107.2019
15. Wang A, Yu H, Gao X, Li X, Qiao S. Influence of Lactobacillus Fermentum I5007 on the Intestinal and Systemic Immune Responses of Healthy and E. Coli Challenged Piglets Antonie Van Leeuwenhoek (2009) 96(1):89–98. doi: 10.1007/s10482-009-9339-2
16. Wang Z, Wang L, Chen Z, Ma X, Yang X, Zhang J, et al. In Vitro Evaluation of Swine-Derived Lactobacillus Reuteri: Probiotic Properties and Effects on Intestinal Porcine Epithelial Cells Challenged With Enterotoxigenic Escherichia Coli K88. J Microbiol Biotechnol (2016) 26(6):1018–25. doi: 10.4014/jmb.1510.10089
17. Yi H, Wang L, Xiong Y, Wen X, Wang Z, Yang X, et al. Effects of Lactobacillus Reuteri LR1 on the Growth Performance, Intestinal Morphology, and Intestinal Barrier Function in Weaned Pigs. J Anim Sci (2018) 96(6):2342–51. doi: 10.1093/jas/sky129
18. Du L, Kim JJ, Shen J, Dai N. Crosstalk Between Inflammation and ROCK/MLCK Signaling Pathways in Gastrointestinal Disorders With Intestinal Hyperpermeability. Gastroenterol Res Pract (2016),2016:7374197. doi: 10.1155/2016/7374197
19. Cheng Y, Wu T, Tang S, Liang F, Fang Y, Cao W, et al. Fermented Blueberry Pomace Ameliorates Intestinal Barrier Function Through the NF-kappaB-MLCK Signaling Pathway in High-Fat Diet Mice. Food Funct (2020) 11(4):3167–79. doi: 10.1039/c9fo02517k
20. Li W, Gao M, Han T. Lycium Barbarum Polysaccharides Ameliorate Intestinal Barrier Dysfunction and Inflammation Through the MLCK-MLC Signaling Pathway in Caco-2 Cells. Food Funct (2020) 11(4):3741–8. doi: 10.1039/d0fo00030b
21. Cao S, Wang C, Yan J, Li X, Wen J, Hu C. Curcumin Ameliorates Oxidative Stress-Induced Intestinal Barrier Injury and Mitochondrial Damage by Promoting Parkin Dependent Mitophagy Through AMPK-TFEB Signal Pathway. Free Radic Biol Med (2020) 147:(8–22). doi: 10.1016/j.freeradbiomed.2019.12.004
22. Cao S, Xiao H, Li X, Zhu J, Gao J, Wang L, et al. AMPK-PINK1/Parkin Mediated Mitophagy Is Necessary for Alleviating Oxidative Stress-Induced Intestinal Epithelial Barrier Damage and Mitochondrial Energy Metabolism Dysfunction in IPEC-J2. Antioxid (Basel) (2021) 10(12):2021. doi: 10.3390/antiox10122010
23. Lin HH, Chung Y, Cheng CT, Ouyang C, Fu Y, Kuo CY, et al. Autophagic Reliance Promotes Metabolic Reprogramming in Oncogenic KRAS-Driven Tumorigenesis. Autophagy (2018) 14(9):1481–98. doi: 10.1080/15548627.2018.1450708
24. Wang C, Li Q, Ren J. Microbiota-Immune Interaction in the Pathogenesis of Gut-Derived Infection. Front Immunol (2019) 10:1873. doi: 10.3389/fimmu.2019.01873
25. Schoultz I, Keita AV. Cellular and Molecular Therapeutic Targets in Inflammatory Bowel Disease-Focusing on Intestinal Barrier Function. Cells (2019) 8(2):193. doi: 10.3390/cells8020193
26. Sina C, Kemper C, Derer S. The Intestinal Complement System in Inflammatory Bowel Disease: Shaping Intestinal Barrier Function. Semin Immunol (2018) 37:(66–73). doi: 10.1016/j.smim.2018.02.008
27. Xie S, Zhao S, Jiang L, Lu L, Yang Q, Yu Q. Lactobacillus Reuteri Stimulates Intestinal Epithelial Proliferation and Induces Differentiation Into Goblet Cells in Young Chickens. J Agric Food Chem (2019) 67(49):13758–66. doi: 10.1021/acs.jafc.9b06256
28. Wu H, Xie S, Miao J, Li Y, Wang Z, Wang M, et al. Lactobacillus Reuteri Maintains Intestinal Epithelial Regeneration and Repairs Damaged Intestinal Mucosa. Gut Microbes (2020) 11(4):997–1014. doi: 10.1080/19490976.2020.1734423
29. Friedrich M, Pohin M, Powrie F. Cytokine Networks in the Pathophysiology of Inflammatory Bowel Disease. Immunity (2019) 50(4):992–1006. doi: 10.1016/j.immuni.2019.03.017
30. Korolkova OY, Myers JN, Pellom ST, Wang L, M'koma AE. Characterization of Serum Cytokine Profile in Predominantly Colonic Inflammatory Bowel Disease to Delineate Ulcerative and Crohn’s Colitides. Clin Med Insights Gastroenterol (2015) 8:(29–44). doi: 10.4137/CGast.S20612
31. Singh UP, Singh NP, Murphy EA, Price RL, Fayad R, Nagarkatti M, et al. Chemokine and Cytokine Levels in Inflammatory Bowel Disease Patients. Cytokine (2016) 77:(44–9). doi: 10.1016/j.cyto.2015.10.008
32. Wang G, Huang S, Cai S, Yu H, Wang Y, Zeng X, et al. Lactobacillus Reuteri Ameliorates Intestinal Inflammation and Modulates Gut Microbiota and Metabolic Disorders in Dextran Sulfate Sodium-Induced Colitis in Mice. Nutrients (2020) 12(8):2298. doi: 10.3390/nu12082298
33. Navabi N, Mcguckin MA, Linden SK. Gastrointestinal Cell Lines Form Polarized Epithelia With an Adherent Mucus Layer When Cultured in Semi-Wet Interfaces With Mechanical Stimulation. PLoS One (2013) 8(7):e68761. doi: 10.1371/journal.pone.0068761
34. Walsham AD, Mackenzie DA, Cook V, Wemyss-Holden S, Hews CL, Juge N, et al. Lactobacillus Reuteri Inhibition of Enteropathogenic Escherichia Coli Adherence to Human Intestinal Epithelium. Front Microbiol (2016) 7:244. doi: 10.3389/fmicb.2016.00244
35. Zhang WM, Wang HF, Gao K, Wang C, Liu L, Liu JX. Lactobacillus Reuteri Glyceraldehyde-3-Phosphate Dehydrogenase Functions in Adhesion to Intestinal Epithelial Cells. Can J Microbiol (2015) 61(5):373–80. doi: 10.1139/cjm-2014-0734
36. Han N, Jia L, Su Y, Du J, Guo L, Luo Z, et al. Lactobacillus Reuteri Extracts Promoted Wound Healing via PI3K/AKT/beta-Catenin/TGFbeta1 Pathway. Stem Cell Res Ther (2019) 10(1):243. doi: 10.1186/s13287-019-1324-8
37. Halper J, Leshin LS, Lewis SJ, Li WI. Wound Healing and Angiogenic Properties of Supernatants From Lactobacillus Cultures. Exp Biol Med (Maywood) (2003) 228(11):1329–37. doi: 10.1177/153537020322801111
38. Mccarty MF, Lerner A. Perspective: Prospects for Nutraceutical Support of Intestinal Barrier Function. Adv Nutr (2021) 12(2):316–24. doi: 10.1093/advances/nmaa139
39. Li S, Qi C, Zhu H, Yu R, Xie C, Peng Y, et al. Lactobacillus Reuteri Improves Gut Barrier Function and Affects Diurnal Variation of the Gut Microbiota in Mice Fed a High-Fat Diet. Food Funct (2019) 10(8):4705–15. doi: 10.1039/c9fo00417c
40. Cui Y, Liu L, Dou X, Wang C, Zhang W, Gao K, et al. Lactobacillus Reuteri ZJ617 Maintains Intestinal Integrity via Regulating Tight Junction, Autophagy and Apoptosis in Mice Challenged With Lipopolysaccharide. Oncotarget (2017) 8(44):77489–99. doi: 10.18632/oncotarget.20536
41. Eftychi C, Schwarzer R, Vlantis K, Wachsmuth L, Basic M, Wagle P, et al. Temporally Distinct Functions of the Cytokines IL-12 and IL-23 Drive Chronic Colon Inflammation in Response to Intestinal Barrier Impairment. Immunity (2019) 51(2):367–80.e4. doi: 10.1016/j.immuni.2019.06.008
42. Fiocchi C. Inflammatory Bowel Disease: Etiology and Pathogenesis. Gastroenterology (1998) 115(1):182–205. doi: 10.1016/s0016-5085(98)70381-6
43. Stengel ST, Fazio A, Lipinski S, Jahn MT, Aden K, Ito G, et al. Activating Transcription Factor 6 Mediates Inflammatory Signals in Intestinal Epithelial Cells Upon Endoplasmic Reticulum Stress. Gastroenterology (2020) 159(4):1357–74.e10. doi: 10.1053/j.gastro.2020.06.088
44. Tang Q, Yi H, Hong W, Wu Q, Yang X, Hu S, et al. Comparative Effects of L. Plantarum CGMCC 1258 and L. Reuteri LR1 on Growth Performance, Antioxidant Function, and Intestinal Immunity in Weaned Pigs. Front Veterinary Sci (2021) 8:728849. doi: 10.3389/fvets.2021.728849
45. Hsieh FC, Lan CC, Huang TY, Chen KW, Chai CY, Chen WT, et al. Heat-Killed and Live Lactobacillus Reuteri GMNL-263 Exhibit Similar Effects on Improving Metabolic Functions in High-Fat Diet-Induced Obese Rats. Food Funct (2016) 7(5):2374–88. doi: 10.1039/c5fo01396h
46. Gu C, Wu L, Li X. IL-17 Family: Cytokines, Receptors and Signaling. Cytokine (2013) 64(2):477–85. doi: 10.1016/j.cyto.2013.07.022
47. Yang JY, Jie Z, Mathews A, Zhou X, Li Y, Gu M, et al. Intestinal Epithelial TBK1 Prevents Differentiation of T-Helper 17 Cells and Tumorigenesis in Mice. Gastroenterology (2020) 159(5):1793–806. doi: 10.1053/j.gastro.2020.07.047
48. Zhang S, Wu L, Chen J, Wei J, Cai H, Ma M, et al. Effects of Porcine IL-17B and IL-17E Against Intestinal Pathogenic Microorganism. Mol Immunol (2019) 116:(151–9). doi: 10.1016/j.molimm.2019.10.011
49. Luo Y, Xu J, Zhang C, Jiang C, Ma Y, He H, et al. Toll-Like Receptor 5-Mediated IL-17C Expression in Intestinal Epithelial Cells Enhances Epithelial Host Defense Against F4(+) ETEC Infection. Veterinary Res (2019) 50(1):48. doi: 10.1186/s13567-019-0665-8
50. Guo S, Chen S, Ma J, Ma Y, Zhu J, Ma Y, et al. Escherichia Coli Nissle 1917 Protects Intestinal Barrier Function by Inhibiting NF-kappaB-Mediated Activation of the MLCK-P-MLC Signaling Pathway. Mediators Inflammation (2019) 2019:(5796491). doi: 10.1155/2019/5796491
51. Zhou HY, Zhu H, Yao XM, Qian JP, Yang J, Pan XD, et al. Metformin Regulates Tight Junction of Intestinal Epithelial Cells via MLCK-MLC Signaling Pathway. Eur Rev Med Pharmacol Sci (2017) 21(22):5239–46. doi: 10.26355/eurrev_201711_13847
52. Wang H, Zhai N, Chen Y, Fu C, Huang K. OTA Induces Intestinal Epithelial Barrier Dysfunction and Tight Junction Disruption in IPEC-J2 Cells Through ROS/Ca(2+)-Mediated MLCK Activation. Environ pollut (2018) 242(Pt A):106–12. doi: 10.1016/j.envpol.2018.06.062
53. Xie Y, Zhan X, Tu J, Xu K, Sun X, Liu C, et al. Atractylodes Oil Alleviates Diarrhea-Predominant Irritable Bowel Syndrome by Regulating Intestinal Inflammation and Intestinal Barrier via SCF/c-Kit and MLCK/MLC2 Pathways. J Ethnopharmacol (2021) 272:(113925). doi: 10.1016/j.jep.2021.113925
54. Long Y, Du L, Kim JJ, Chen B, Zhu Y, Zhang Y, et al. MLCK-Mediated Intestinal Permeability Promotes Immune Activation and Visceral Hypersensitivity in PI-IBS Mice. Neurogastroenterol Motil (2018) 30(9):e13348. doi: 10.1111/nmo.13348
55. Chen S, Zhu J, Chen G, Zuo S, Zhang J, Chen Z, et al. 1,25-Dihydroxyvitamin D3 Preserves Intestinal Epithelial Barrier Function From TNF-Alpha Induced Injury via Suppression of NF-kB P65 Mediated MLCK-P-MLC Signaling Pathway. Biochem Biophys Res Commun (2015) 460(3):873–8. doi: 10.1016/j.bbrc.2015.03.125
56. Roussel C, De Paepe K, Galia W, De Bodt J, Chalancon S, Denis S, et al. Multi-Targeted Properties of the Probiotic Saccharomyces Cerevisiae CNCM I-3856 Against Enterotoxigenic Escherichia Coli (ETEC) H10407 Pathogenesis Across Human Gut Models. Gut Microbes (2021) 13(1):953246. doi: 10.1080/19490976.2021.1953246
Keywords: Lactobacillus reuteri, enterotoxigenic Escherichia coli K88, inflammatory response, intestinal epithelial barrier, MLCK signaling pathway
Citation: Gao J, Cao S, Xiao H, Hu S, Yao K, Huang K, Jiang Z and Wang L (2022) Lactobacillus reuteri 1 Enhances Intestinal Epithelial Barrier Function and Alleviates the Inflammatory Response Induced by Enterotoxigenic Escherichia coli K88 via Suppressing the MLCK Signaling Pathway in IPEC-J2 Cells. Front. Immunol. 13:897395. doi: 10.3389/fimmu.2022.897395
Received: 16 March 2022; Accepted: 13 June 2022;
Published: 14 July 2022.
Edited by:
Peng Ji, University of California, Davis, United StatesReviewed by:
Jinfeng Miao, Nanjing Agricultural University, ChinaManuela Buettner, Hannover Medical School, Germany
Copyright © 2022 Gao, Cao, Xiao, Hu, Yao, Huang, Jiang and Wang. This is an open-access article distributed under the terms of the Creative Commons Attribution License (CC BY). The use, distribution or reproduction in other forums is permitted, provided the original author(s) and the copyright owner(s) are credited and that the original publication in this journal is cited, in accordance with accepted academic practice. No use, distribution or reproduction is permitted which does not comply with these terms.
*Correspondence: Li Wang, d2FuZ2xpMUBnZGFhcy5jbg==
†These authors have contributed equally to this work