- 1Laboratory of Clinical and Experimental Immunology, Health and Life Science School - Pontifical Catholic University of Rio Grande do Sul (PUCRS), Porto Alegre, Brazil
- 2Center for Transplantation Sciences, Department of Surgery, Massachusetts General Hospital, Harvard Medical School, Boston, MA, United States
This mini review describes the role of gut and lung microbiota during respiratory viral infection and discusses the implication of the microbiota composition on the immune responses generated by the vaccines designed to protect against these pathogens. This is a growing field and recent evidence supports that the composition and function of the microbiota can modulate the immune response of vaccination against respiratory viruses such as influenza and SARS-CoV-2. Recent studies have highlighted that molecules derived from the microbiome can have systemic effects, acting in distant organs. These molecules are recognized by the immune cells from the host and can trigger or modulate different responses, interfering with vaccination protection. Modulating the microbiota composition has been suggested as an approach to achieving more efficient protective immune responses. Studies in humans have reported associations between a better vaccine response and specific bacterial taxa. These associations vary among different vaccine strategies and are likely to be context-dependent. The use of prebiotics and probiotics in conjunction with vaccination demonstrated that bacterial components could act as adjuvants. Future microbiota-based interventions may potentially improve and optimize the responses of respiratory virus vaccines.
Introduction
Although it is a successful strategy, immune responses to vaccination can vary among individuals in the human population. One factor associated with this vaccine response variation is the microbiota composition. The human gut microbiota is composed of trillions of commensal microorganisms that can impact host physiology by metabolizing dietary components and synthesizing multiple metabolites that interact with host cells, including immune cells (1). The gut microbial community develops during early life together with the most extensive collection of immune cells in the body, the gut-associated lymphoid tissue (GALT), where they can influence the development of each other (2). However, the effects of the microbiome on the immune system go beyond acting locally in the intestine. Bacterial fragments and metabolites can translocate across the intestinal barrier, entering the circulation and reaching distant organs, including the lungs, where they can influence immune cells (3). Therefore, interventions targeting the gut microbiota may be one strategy to reduce disease burden and illness severity caused by respiratory tract infections (RTI) and increase vaccine responses against respiratory pathogens.
Most studies exploring the effects of the microbiota in immune responses focus on mucosal immunology in the gut, and responses to oral vaccination. However, the effect of the gut microbiota can go beyond intestinal tissues and influence responses to respiratory viruses’ vaccines. The lung microbiome is less diverse and contains smaller biomass than the gut (4, 5), but both present Bacteroidetes and Firmicutes as the dominant phyla (6–8). The colonization of the airways occurs shortly after birth, with the maturation of the microbiome occurring during the first 2-3 postnatal months in humans (9). The airway microbiota composition is mediated mainly by microbial immigration, microbial elimination, and the proliferation rate of bacteria (4, 5), with minimal contributions from selective pressures created by tissue growth conditions (7, 8). Thus, the gut and lung microbiota can have a role in the immune response during respiratory virus infection and the vaccine response against these pathogens. This mini review explores the current evidence relating microbiota composition, function, and its metabolites to the vaccine immune response against respiratory viruses, as well as the use of prebiotics and probiotics as natural adjuvants, describing the implication of this evidence on future research of vaccine development and potential interventions based on microbiota intervention.
Scientific Background
Microbiota, Respiratory Diseases and the Immune System
It is becoming more evident that changes in the gut microbiota composition are associated with respiratory diseases (10–12). Studies in mice using antibiotic treatment to deplete gut bacterial composition before airway infection challenge demonstrated that reducing intestinal microbiome diversity led to an increase in inflammation and mortality during the infection (13–15). An increase in Bacteroidetes and a decrease in Firmicutes phyla abundance in the gut was observed during respiratory syncytial virus (RSV) and influenza virus infections (16). In humans, alterations in alpha and beta diversity of gut microbial composition can also be observed during lung infections (17–19). In RSV infection, there was no significant difference in the abundance of microbes in the gut. However, the bacterial diversity had significantly changed, with an expansion of the families Clostridiales, Odoribacteraceae, Lactobacillacea and Actinomyces in infected patients compared to healthy controls (17). In COVID-19 patients, it was observed an increased abundance of Streptococcus, Clostridium, Lactobacillus and Bifidobacterium and a decrease of Bacteroides, Roseburia, Faecalibacterium, Coprococcus and Parabacteroides (18). Bacterial community richness and diversity were decreased in COVID-19 patients compared to healthy controls (19, 20). Certain intestinal commensal microorganisms associated with a healthy gut, such as Faecalibacterium prausnitzii and Eubacterium rectale and the butyrate-producing bacteria from the families Lachnospiraceae and Ruminoccocaceae were also decreased in COVID-19 and H1N1 patients (19, 21). Although the commonalities between the type of taxa that are changed during different diseases are present, most changes in microbial composition tend to be context-dependent and vary from one disease to another. The acknowledgment that multiple respiratory diseases present clinical intestinal manifestations (22, 23) indicates crosstalk at some level between these two mucosal surfaces. This crosstalk has been termed the gut-lung axis (3, 24–26).
The evolution of the immune system and the emergence of adaptive immunity coincided with the acquisition of a complex microbiota by the host (27), reinforcing the idea that the microbiome has a pivotal role in shaping the immune system. For example, leukocytes express a range of receptors that recognize microbial metabolites, conserved molecules associated with bacterial structural components (28) and other molecules synthesized by commensal bacteria. The disturbance of the normal microbiota, named dysbiosis, can dampen the host’s immune response, increasing susceptibility to complications during disease (29). Mice lacking microbiota (Germ-free [GF] mice) or antibiotic-treated mice have impaired responses to respiratory infections (30) and decreased IgA-producing plasma cells and the IgA repertoire (31, 32), confirming the idea that commensal bacteria can shape host immunity. Moreover, commensal bacteria regulate immune responses in the mucosal airways after influenza infection. Antibiotic treatment during respiratory infection in mice reduced the numbers of CD4+, CD8+ T cells, and IgA production. The number of dendritic cells (DCs) migrating from the lung to draining lymph nodes was also diminished. This effect was proposed to be mediated by a decreased production of inflammasome-related cytokines, such as IL-1b and IL-18, after antibiotic exposure (30). Interestingly, in the same study, it was reported that the administration of TLR agonists could rescue the immune responses against influenza infection after antibiotic treatment was also demonstrated by other groups (33).
The crosstalk between immune cells and commensal bacteria occurs mainly through cell adhesion contacts, especially in the mucosal sites, and through the interactions between microbial metabolites and receptors expressed by immune cells. One example of such metabolites is the short-chain fatty acids (SCFAs), a class of fatty acids produced by certain groups of bacteria after the metabolization of soluble and certain insoluble fibers ingested by the host (34). The SCFAs which have their physiologic role best described are acetic acid (acetate), butyric acid (butyrate), and propionic acid (propionate) (35). Through different mechanisms, these bacterial metabolites can influence different cellular processes, such as gene expression, proliferation, differentiation, and apoptosis (34, 35). One of these mechanisms is by acting on the cell surface, where the SCFAs can be sensed by G protein-coupled receptors (GPRs) expressed by several cell types, including immune cells. The GPR41 and GPR43 receptors can recognize all three SCFAs, whereas GPR109a can sense butyrate (35, 36). SCFAs can also enter cells via passive diffusion through the cell membrane or active transport by transmembrane proteins. Once inside, SCFAs can be metabolized by immune cells, increasing acetyl-CoA levels and subsequently fueling the TCA cycle and cell function (37). Intracellular SCFAs that are not metabolized can further migrate to the nuclei, acting as natural inhibitors of histone deacetylases (HDACs), leading to changes in gene expression patterns (36–38).
The SCFA butyrate was shown to suppress immune responses by promoting the expansion of regulatory T cells (Tregs) (39). Butyrate’s ability to induce Treg differentiation is in part mediated by its function as an HDAC-inhibitor, which in turn increases histone acetylation in the Foxp3 and Il10 gene loci, supporting the generation of Foxp3+ T cells (40, 41). Butyrate can also decrease the expression of inflammatory genes in neutrophils and macrophages, affecting their migration and cytokine production (42–44). In contrast, other studies reported that butyrate could increase macrophage antimicrobial response and promote T cell memory development (45, 46). Interestingly, CD8+ T cells failed to differentiate into memory cells during infection in mice lacking microbiota, and deletion of GPR41 and GPR43 also impaired these memory recall responses (46). Acetate was also shown to modulate memory T cell responses (47, 48), promote IgA production in a mechanism dependent on GPR43 expression in dendritic cells (DCs) (49), and protect against respiratory pathogens in a mouse model of bacterial infection (50). Mice treatment with the SCFA propionate could modulate hematopoiesis and increase the generation of dendritic cell precursors with an enhanced phagocytic activity that protects against allergic inflammation (51). A recent study from the same group demonstrated that treatment with a high-fiber diet, which is known to increase the systemic levels of SCFAs, also modulated hematopoiesis during influenza infection in mice, promoting a population of monocyte-derived macrophages that protected the legs from tissue damage caused by excessive neutrophil infiltration during infection. It also increased T cell cytotoxic activity (52). Aside from SCFAs, other microbial metabolites have also been reported to affect host immune responses, as reviewed elsewhere (53–55). For example, some metabolites can bind to the aryl hydrocarbon receptor (AHR) and are termed AHR ligands. These metabolites were reported to be involved in developing of immune responses (56). Activation of AHR is necessary for the generation of innate lymphoid cell (ILC) populations, particularly IL-22-producing group 3 ILCs (ILC3s) (57). Indoles are a class of metabolites that can modulate ILC3s and promote IL-22 secretion (58). In addition, indoles also enhance epithelial barrier function (59).
Taken together, these studies show that the presence of a healthy microbiota is essential for the normal development of the immune system and control of respiratory diseases, including protection against respiratory viral infections. The concept that microbial components can induce different immune responses opens the possibility of developing strategies to harness this immunomodulatory effect of the microbiome and use it in combination with other interventions that seek to produce an appropriate immune response, for example, during vaccination.
Microbiota and Respiratory Virus Vaccine Responses
Vaccination is the most popular and cost-effective medical intervention, estimated to prevent more than 2 million deaths per year globally, and decrease disease severity (41, 60). However, the vaccinated individuals’ present variation in the immune response induced by vaccines. For example, antibody titers induced by yellow fever (61), seasonal trivalent inactivated influenza vaccine (TIV) (62) and hepatitis B (HepB) (63) vaccines were shown to vary more than 10-fold between individuals. The T cell response varied at similar levels to that of antibodies (64, 65). A better understanding of the mechanisms that lead to such variation is important to develop strategies that overcome these limitations.
The microbiome composition was associated with differences in responses to oral vaccination, including against respiratory pathogens. A seminal study in this field reported a correlation between the level of TLR5 expression and the antibody response against influenza hemagglutinin in humans after influenza vaccination (66). Subsequent work showing that TLR5-/- mice present an impaired capacity to mount antibody responses against TIV immunization confirmed the importance of this receptor for the generation of appropriate antibody responses after TIV immunization (67). The immune response was restored with the oral administration of flagellated, but not aflagellated strain of E. coli. Since flagellin is a well-known ligand for TLR5, this work indicates that PRRs derived from the gut microbiota can influence humoral responses to vaccinations against respiratory pathogens. Intriguingly, differences in intestinal microbiota composition did not affect responses against adjuvanted vaccines, demonstrating some limitations might exist in the extent to which commensal bacteria can modulate distal responses (67).
The use of antibiotics before or during vaccination was also demonstrated to modulate immune responses. In a randomized clinical trial, healthy individuals received a broad-spectrum antibiotic regimen (neomycin, vancomycin, and metronidazole) from three days before immunization until one day after (68). In this study, the lack of difference might be caused by pre-existing immunity to influenza, also known as immunological memory. The cells that mediate immune memory response were previously generated and present a more robust and rapid response during subsequent encounters with antigens. Thus, the authors suggested that this pre-defined immune memory may not be as susceptible as a primary response to the microbiota influence. To overcome this limitation, the authors conducted a second clinical trial only including individuals who had no exposure to influenza infection or vaccination in the preceding three years (68). These individuals had a marked decrease in the antibody titers before vaccination compared to the groups from the first study. The antibiotic-treated group had levels of IgG1 and IgA antibodies, and its avidity significantly decreased compared to the untreated group (68). These findings show that the impact of antibiotic exposure on the immune response during vaccination is limited by the presence of immunological memory, which seems to be more resilient to perturbations in the microbiota. In early life, when immunological memory is not well developed might be a better time for a microbiota intervention to increase vaccine response.
Since the emergence of COVID-19, a global effort has been made to try to understand how the disease develops and generate an effective vaccine. A portion of these studies focused on the associations between the vaccine response and the microbiota composition. One recent observational study reported that specific bacterial taxa were associated with the presence of higher neutralizing antibodies after vaccination. Interestingly, the microorganisms that positively correlated with neutralizing antibodies differed between the inactivated and the mRNA vaccines (69). This shows that the effects of different bacterial species in vaccine responses may be context-dependent. Also, the abundance of Prevotella copri and two species of Megamonas were reported to be increased in participants’ gut microbiota with fewer adverse effects to both vaccines (69). Further studies to understand the effects of gut commensal bacteria in COVID-19 vaccine responses are still ongoing. One ongoing observational study aims to analyze and compare the microbiome composition of subjects that received different COVID-19 vaccines and that recovered from the disease (70).
The findings discussed above show that the microbiota can modulate immune responses to vaccination. However, other factors known to influence these responses, such as route of administration, and genetic and environmental factors, may account for the variations observed. One limitation of these studies is that they are primarily cross-sectional, correlating the microbiome with vaccine responses at only one point in time. It is known that the microbiota composition can vary considerably over time depending on environmental exposure. For this reason, more longitudinal studies are needed to better evaluate the impact of the microbiota on vaccine responses.
The mechanisms associated with the effect of microbiota on vaccine response are not all described yet However, one explanation is that the microbiota constitutes a constant source of natural adjuvants that activate different pattern recognition receptors (PRRs) in host cells (71). One of these molecules, the muramyl dipeptide (MDP), present in the peptidoglycan of both Gram-positive and Gram-negative bacteria, is a ligand for the nucleotide-binding oligomerization domain containing 2 (Nod2) receptor and was implicated in enhancing antigen-specific IgG responses after intranasal immunization (72). Furthermore, Monophosphoryl lipid A (MPL), an agonist of TLR4 derived from Salmonella enterica endotoxin is already in clinical use as an adjuvant (73). Thus, alterations in the microbiota composition can change which PRRs and pathways are activated, leading to different innate and adaptive responses, potentially modulating vaccine responses.
The effect of microbiota on vaccine response and recent works showing how bacterial components can influence immunity led to increase in studies focusing on using prebiotic, probiotic and postbiotic treatments to increase vaccine responses (74). In the next section, we discuss some of the recent data regarding the effect of using these strategies in the context of vaccination against respiratory pathogens.
Use of Probiotics and Prebiotics to Improve Vaccine Responses
A probiotic is any live organism that confers a health benefit to the host when administered in adequate amounts (75). Here, we will limit our discussion of probiotics as beneficial bacteria introduced into the host. In that sense, daily probiotic administration was shown to reduce the incidence of pathogenic bacteria in the nasal cavity (76). On the one hand, two meta-analyses analyzing various randomized clinical trials using probiotics reported their capacity to reduce respiratory infection duration and incidence (77, 78). In mice, the administration of probiotic strains of bacteria, such as Lactobacillus, as a prophylactic treatment was shown to increase innate and adaptive response against influenza during infection (79–81). On the other hand, studies with healthy individuals showed that probiotics were ineffective for reducing the incidence of respiratory tract infections (RTIs) (82–85). However, two of these studies reported that although not changing the incidence, probiotics reduced the duration and severity of respiratory infections (82, 83).
Probiotics in both oral and intramuscular vaccine formulations against HA and M2e influenza proteins boosted cellular and humoral responses in mouse and chicken models (86, 87). One study explored the feasibility of a new vaccine strategy using Enterococcus faecium as a bacterial vector carrying influenza antigens given orally and reported that this strategy induced antigen-specific antibodies and protected mice against H1N1 lethal infection (86). The addition of Bacillus subtilis spores into an intramuscular vaccine formulation of inactivated avian influenza virus led to enhancement of H9N2 virus-specific antibody IgG responses compared to the standard vaccine group (87). Further, another study using Lactobacillus casei demonstrated that bacterial microorganisms could be used as a vector to deliver antigens against respiratory pathogens either orally or intranasally administered and induce protective responses (88). These findings demonstrate that probiotic strains can be incorporated into vaccine components to help increase their efficacy.
To evaluate the use of probiotics to increase vaccine responses against respiratory pathogens, most studies relied on randomized clinical trials using intramuscular influenza trivalent inactivated vaccines (TIV) as their immunization model. Three independent studies using L. paracasei as their probiotic strain reported no differences in antibody and cellular responses to influenza after TIV vaccination (89–91). In contrast, two studies using a model of influenza vaccination administered intramuscularly found increased IgA antibodies in the saliva, higher seroconversion, and IgG titers when L. paracasei was administered orally with other probiotic strains (92, 93). Using L. plantarum as a probiotic strain, one study reported an increase in influenza-specific IgA and IgG antibodies six months after vaccination with a trivalent influenza vaccine (94). Other studies involving probiotics and responses to TIV and other vaccines to non-respiratory pathogens can be found in a meta-analysis (95) and systematic reviews (96, 97). An interesting observation from these studies is that the probiotic treatments generally confer beneficial effects to only one or two of the influenza strains present in the vaccine. Changes in the probiotic composition also modify the specificity of the increased response. More recently, an experimental study in mice showed that oral administration of the probiotic strain Lactobacillus plantarum GUANKE increased neutralization antibody levels and cellular immune response after intramuscular DNA vaccination against SARS-CoV-2 (81). Currently, there are several ongoing clinical trials assessing the utility of probiotics in enhancing antiviral immune responses to multiple SARS-CoV-2 vaccines (98). Thus, these results suggest that different probiotic species can be harnessed to increase specific memory responses to different strains of pathogens, although further work is needed to confirm this hypothesis.
Another strategy to expand certain commensal bacteria in the gut is prebiotics. These molecules are characterized as substrates, or nutrients, that can be metabolized by beneficial bacteria present in the host and therefore expand this specific microbial population (75). After birth, maternal milk can be considered a prebiotic, as it presents many components that help shape a healthy microbiota, such as oligosaccharides, IgA antibodies and hormones (99–101). Notably, these milk substrates were shown to induce the expansion of Bifidobacterium species of bacteria in the gut, which increased mucosal barrier function and protection against infections (102, 103). Breast milk also contains bacterial cells that initially colonize the infant’s gut and continue to influence gut microbiota composition throughout life (104). Examples of bacterial genera found to be supported by breast milk are Veillonella and Rothia (104), which are known to increase protection against asthma (105).
The use of prebiotics derived from fermentable carbohydrates and plant-based compounds was shown to reduce the incidence, duration, and severity of RTIs (95, 106–108). These studies show that this beneficial effect is mediated by alterations in the microbiota composition and suggests that changes in the host’s nutrition could also lead to similar results. Regarding the use of prebiotics in vaccination against respiratory pathogens, it was demonstrated that they could increase seroprotection against H1N1, but not the H3N2 and B strains of influenza after vaccination with TIV (97). No difference was observed in seroconversion rates. Furthermore, many dietary components other than prebiotics have immunomodulatory functions, including certain vitamins and carbohydrates (109, 110). These components should be investigated for their potential ability to increase vaccine responses. Thus, it will be essential to perform further studies to evaluate how the increase in the abundance of probiotic strains can increase immunity and if the use of bacterial metabolites can bypass the need to alter microbiome composition and still present beneficial effects.
Conclusion and Perspectives
The microbiota composition is one of the factors that could contribute to some variability observed in vaccine responses. Recent studies demonstrated how microbiota could play a role in modulating the immune response elicited by vaccines. Those findings indicated that this effect is mediated by specific bacteria and depends on the context of the vaccine approach. Overall, these studies reinforce the idea that strategies to maintain a normal microbiota, including the use of pre- or probiotics, and the incorporation of bacterial molecules in the form of adjuvants into vaccine composition, can improve vaccine immune response (Figure 1). Since the beneficial effects of these bacterial metabolites come primarily from their interactions with cell surface receptors in host cells, the development and use of synthetic molecules that activate the same receptors with similar strength may be a feasible alternative to bypass the need to use bacterial cells to produce these components (111).
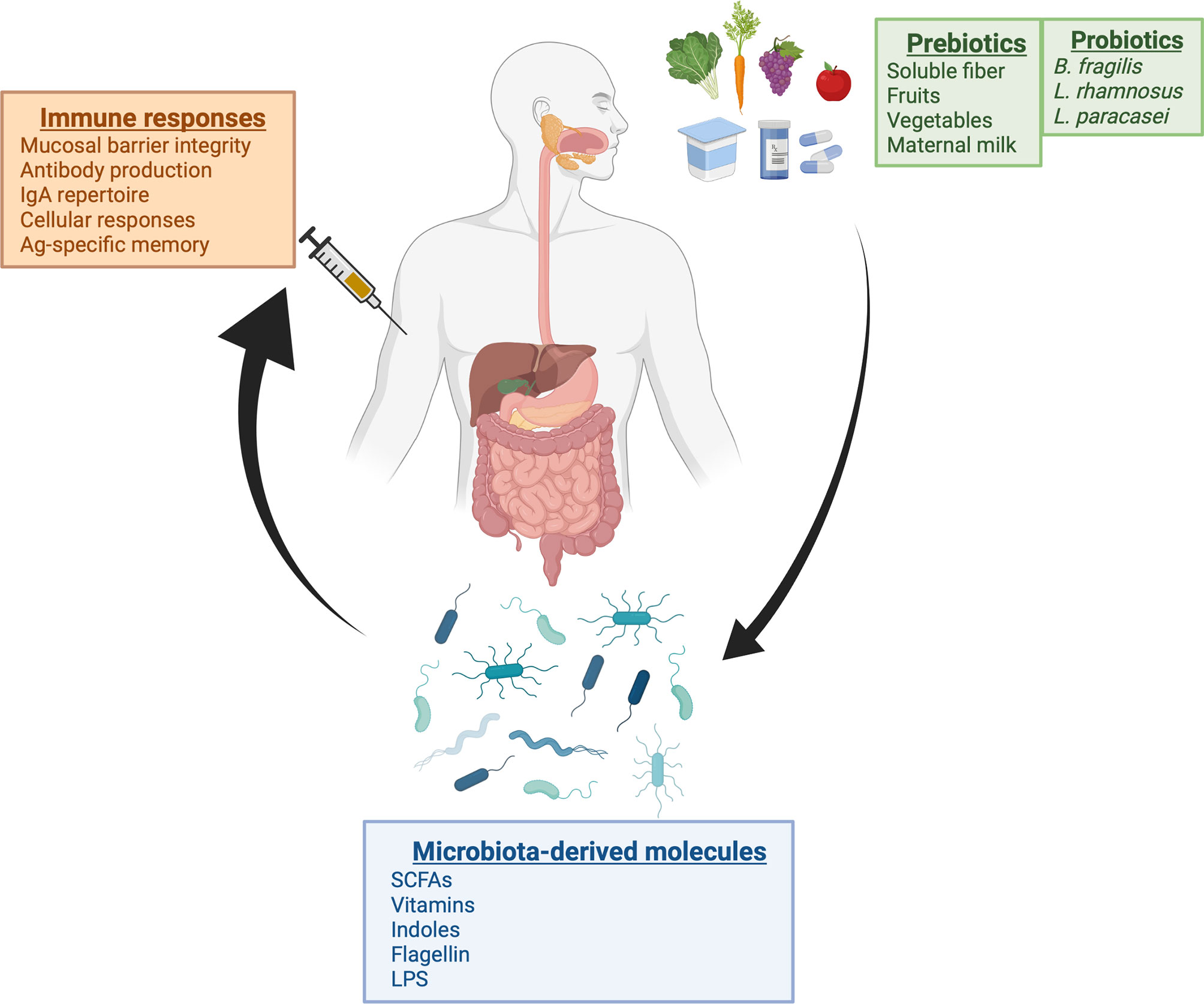
Figure 1 Schematic view of the microbiome-immunity relationship in the context of vaccination. Recent studies demonstrate that there is an association between microbiota and vaccine responses. Gut microbiome composition can be modulated through dietary intake and other environmental factors such as the use of antibiotics or probiotic consumption. With the discovery that abundance of specific bacterial taxa is correlated with increased immune responses after vaccination, therapeutic strategies using pre- or probiotics in combination with vaccination could represent an effective alternative to increase vaccine responses. Created with BioRender.com.
Despite the increasing knowledge that there is an association between a healthy gut microbiome and more effective responses to vaccination against respiratory pathogens, possible limitations of the applications of this as therapeutics should be noted. It is known that most probiotic microorganisms and bacterial metabolites applied in these studies have immunomodulatory effects, leading to a reduction in the overall inflammatory response. This suppression of inflammation could potentially lead to inadequate vaccine response. This idea is highlighted in the context of oral vaccines from studies reporting that oral vaccines perform poorly in developing countries (112–114), where the population is reported to have an increased gut microbiome diversity (115). The potential limitations of probiotics and their immunomodulatory effects in systemic vaccination modes should be further explored in the future.
Moreover, a decrease in inflammatory mediators does not necessarily preclude the development of an effective memory response. As we discussed in this mini review, bacterial metabolites such as SCFAs, which have regulatory effects, could also support the development of memory CD8+ T cells (46) and increase the production of antibodies by B cells (49). Immunomodulatory molecules, such as IL-10, are relevant for the maturation of the memory T lymphocytes population (116). Therefore, further work is needed to validate the applicability of these approaches and identify the limits of this microbiota-mediated modulation of immunity.
Author Contributions
JG, TB, and AS wrote the paper. JG and TB did the figure. All authors contributed to the article and approved the submitted version.
Funding
This study received funds from Coordenação de Aperfeiçoamento de Pessoal de Nível Superior (CAPES), finance code 1 and CNPq. TB effort was supported by NIH R01AI143887-04 (to LVR).
Conflict of Interest
The authors declare that the research was conducted in the absence of any commercial or financial relationships that could be construed as a potential conflict of interest.
Publisher’s Note
All claims expressed in this article are solely those of the authors and do not necessarily represent those of their affiliated organizations, or those of the publisher, the editors and the reviewers. Any product that may be evaluated in this article, or claim that may be made by its manufacturer, is not guaranteed or endorsed by the publisher.
Acknowledgments
This is a short text to acknowledge the contributions of specific colleagues, institutions, or agencies that aided the efforts of the authors.
References
1. Cho I, Blaser MJ. The Human Microbiome: At the Interface of Health and Disease. Nat Rev Genet (2012) 13(4):260–70. doi: 10.1038/nrg3182
2. Tanaka M, Nakayama J. Development of the Gut Microbiota in Infancy and its Impact on Health in Later Life. Allergol Int (2017) 66(4):515–22. doi: 10.1016/j.alit.2017.07.010
3. Enaud R, Prevel R, Ciarlo E, Beaufils F, Wieërs G, Guery B, et al. The Gut-Lung Axis in Health and Respiratory Diseases: A Place for Inter-Organ and Inter-Kingdom Crosstalks. Front Cell Infect Microbiol (2020) 10:9. doi: 10.3389/fcimb.2020.00009
4. Mathieu E, Escribano-Vazquez U, Descamps D, Cherbuy C, Langella P, Riffault S, et al. Paradigms of Lung Microbiota Functions in Health and Disease, Particularly, in Asthma. Front Physiol (2018) 9:1168. doi: 10.3389/fphys.2018.01168
5. Sender R, Fuchs S, Milo R. Revised Estimates for the Number of Human and Bacteria Cells in the Body. PloS Biol (2016) 14(8):e1002533. doi: 10.1371/journal.pbio.1002533
6. Bassis CM, Erb-Downward JR, Dickson RP, Freeman CM, Schmidt TM, Young VB, et al. Analysis of the Upper Respiratory Tract Microbiotas as the Source of the Lung and Gastric Microbiotas in Healthy Individuals. MBio (2015) 6(2):e00037–15. doi: 10.1128/mbio.00037-15
7. Eckburg PB, Bik EM, Bernstein CN, Purdom E, Dethlefsen L, Sargent M, et al. Diversity of the Human Intestinal Microbial Flora. Science (2005) 308(5728):1635–8. doi: 10.1126/science.1110591
8. Dickson RP, Erb-Downward JR, Martinez FJ, Huffnagle GB. The Microbiome and the Respiratory Tract. Annu Rev Physiol (2016) 78(1):481–504. doi: 10.1146/annurev-physiol-021115-105238
9. Pattaroni C, Watzenboeck ML, Schneidegger S, Kieser S, Wong NC, Bernasconi E, et al. Early-Life Formation of the Microbial and Immunological Environment of the Human Airways. Cell Host Microbe (2018) 24(6):857–65.e4. doi: 10.1016/j.chom.2018.10.019
10. Muhlebach MS, Zorn BT, Esther CR, Hatch JE, Murray CP, Turkovic L, et al. Initial Acquisition and Succession of the Cystic Fibrosis Lung Microbiome is Associated With Disease Progression in Infants and Preschool Children. PloS Pathog (2018) 14(1):e1006798. doi: 10.1371/journal.ppat.1006798
11. Fujimura KE, Sitarik AR, Havstad S, Lin DL, Levan S, Fadrosh D, et al. Neonatal Gut Microbiota Associates With Childhood Multisensitized Atopy and T Cell Differentiation. Nat Med (2016) 22(10):1187–91. doi: 10.1038/nm.4176
12. Groves HT, Higham SL, Moffatt MF, Cox MJ, Tregoning JS. Respiratory Viral Infection Alters the Gut Microbiota by Inducing Inappetence. MBio (2020) 11(1):e03236–19. doi: 10.1128/mBio.03236-19
13. Chen L-W, Chen P-H, Hsu C-M. Commensal Microflora Contribute to Host Defense Against Escherichia Coli Pneumonia Through Toll-Like Receptors. Shock (2011) 36(1):67–75. doi: 10.1097/SHK.0b013e3182184ee7
14. Schuijt TJ, Lankelma JM, Scicluna BP, de Sousa e Melo F, Roelofs JJTH, de Boer JD, et al. The Gut Microbiota Plays a Protective Role in the Host Defence Against Pneumococcal Pneumonia. Gut (2016) 65(4):575–83. doi: 10.1136/gutjnl-2015-309728
15. Sencio V, Machado MG, Trottein F. The Lung-Gut Axis During Viral Respiratory Infections: The Impact of Gut Dysbiosis on Secondary Disease Outcomes. Mucosal Immunol (2021) 14(2):296–304. doi: 10.1038/s41385-020-00361-8
16. Groves HT, Cuthbertson L, James P, Moffatt MF, Cox MJ, Tregoning JS. Respiratory Disease Following Viral Lung Infection Alters the Murine Gut Microbiota. Front Immunol (2018) 9:182. doi: 10.3389/fimmu.2018.00182
17. Harding JN, Siefker D, Vu L, You D, DeVincenzo J, Pierre JF, et al. Altered Gut Microbiota in Infants is Associated With Respiratory Syncytial Virus Disease Severity. BMC Microbiol (2020) 20(1):140. doi: 10.1186/s12866-020-01816-5
18. Tao W, Zhang G, Wang X, Guo M, Zeng W, Xu Z, et al. Analysis of the Intestinal Microbiota in COVID-19 Patients and its Correlation With the Inflammatory Factor IL-18. Med Microecol (2020) 5(100023):100023. doi: 10.1016/j.medmic.2020.100023
19. Zuo T, Zhang F, Lui GCY, Yeoh YK, Li AYL, Zhan H, et al. Alterations in Gut Microbiota of Patients With COVID-19 During Time of Hospitalization. Gastroenterology (2020) 159(3):944–55.e8. doi: 10.1053/j.gastro.2020.05.048
20. Yeoh YK, Zuo T, Lui GC-Y, Zhang F, Liu Q, Li AYL, et al. Gut Microbiota Composition Reflects Disease Severity and Dysfunctional Immune Responses in Patients With COVID-19. Gut (2021) 70(4):698–706. doi: 10.1136/gutjnl-2020-323020
21. Gu S, Chen Y, Wu Z, Chen Y, Gao H, Lv L, et al. Alterations of the Gut Microbiota in Patients With Coronavirus Disease 2019 or H1N1 Influenza. Clin Infect Dis (2020) 71(10):2669–78. doi: 10.1093/cid/ciaa709
22. Dilantika C, Sedyaningsih ER, Kasper MR, Agtini M, Listiyaningsih E, Uyeki TM, et al. Influenza Virus Infection Among Pediatric Patients Reporting Diarrhea and Influenza-Like Illness. BMC Infect Dis (2010) 10(1):3. doi: 10.1186/1471-2334-10-3
23. Russell SL, Gold MJ, Hartmann M, Willing BP, Thorson L, Wlodarska M, et al. Early Life Antibiotic-Driven Changes in Microbiota Enhance Susceptibility to Allergic Asthma. EMBO Rep (2012) 13(5):440–7. doi: 10.1038/embor.2012.32
24. Wypych TP, Wickramasinghe LC, Marsland BJ. The Influence of the Microbiome on Respiratory Health. Nat Immunol (2019) 20(10):1279–90. doi: 10.1038/s41590-019-0451-9
25. Dang AT, Marsland BJ. Microbes, Metabolites, and the Gut-Lung Axis. Mucosal Immunol (2019) 12(4):843–50. doi: 10.1038/s41385-019-0160-6
26. de Oliveira GLV, Oliveira CNS, Pinzan CF, de Salis LVV, Cardoso CR deB. Microbiota Modulation of the Gut-Lung Axis in COVID-19. Front Immunol (2021) 12:635471. doi: 10.3389/fimmu.2021.635471
27. Ansaldo E, Farley TK, Belkaid Y. Control of Immunity by the Microbiota. Annu Rev Immunol (2021) 39(1):449–79. doi: 10.1146/annurev-immunol-093019-112348
28. Medzhitov R. Recognition of Microorganisms and Activation of the Immune Response. Nature (2007) 449(7164):819–26. doi: 10.1038/nature06246
29. Levy M, Kolodziejczyk AA, Thaiss CA, Elinav E. Dysbiosis and the Immune System. Nat Rev Immunol (2017) 17(4):219–32. doi: 10.1038/nri.2017.7
30. Ichinohe T, Pang IK, Kumamoto Y, Peaper DR, Ho JH, Murray TS, et al. Microbiota Regulates Immune Defense Against Respiratory Tract Influenza A Virus Infection. Proc Natl Acad Sci USA (2011) 108(13):5354–9. doi: 10.1073/pnas.1019378108
31. Wei B, Su TT, Dalwadi H, Stephan RP, Fujiwara D, Huang TT, et al. Resident Enteric Microbiota and CD8+ T Cells Shape the Abundance of Marginal Zone B Cells. Eur J Immunol (2008) 38(12):3411–25. doi: 10.1002/eji.200838432
32. Lindner C, Wahl B, Föhse L, Suerbaum S, Macpherson AJ, Prinz I, et al. Age, Microbiota, and T Cells Shape Diverse Individual IgA Repertoires in the Intestine. J Exp Med (2012) 209(2):365–77. doi: 10.1084/jem.20111980
33. Wu S, Jiang Z-Y, Sun Y-F, Yu B, Chen J, Dai C-Q, et al. Microbiota Regulates the TLR7 Signaling Pathway Against Respiratory Tract Influenza A Virus Infection. Curr Microbiol (2013) 67(4):414–22. doi: 10.1007/s00284-013-0380-z
34. Tan J, McKenzie C, Potamitis M, Thorburn AN, Mackay CR, Macia L. The Role of Short-Chain Fatty Acids in Health and Disease. Adv Immunol (2014) 121:91–119. doi: 10.1016/B978-0-12-800100-4.00003-9
35. Corrêa-Oliveira R, Fachi JL, Vieira A, Sato FT, Vinolo MAR. Regulation of Immune Cell Function by Short-Chain Fatty Acids. Clin Transl Immunol (2016) 5(4):e73. doi: 10.1038/cti.2016.17
36. Blacher E, Levy M, Tatirovsky E, Elinav E. Microbiome-Modulated Metabolites at the Interface of Host Immunity. J Immunol (2017) 198(2):572–80. doi: 10.4049/jimmunol.1601247
37. Kim CH. Control of Lymphocyte Functions by Gut Microbiota-Derived Short-Chain Fatty Acids. Cell Mol Immunol (2021) 18(5):1161–71. doi: 10.1038/s41423-020-00625-0
38. Sepahi A, Liu Q, Friesen L, Kim CH. Dietary Fiber Metabolites Regulate Innate Lymphoid Cell Responses. Mucosal Immunol (2021) 14(2):317–30. doi: 10.1038/s41385-020-0312-8
39. Smith PM, Howitt MR, Panikov N, Michaud M, Gallini CA, Bohlooly-Y M, et al. The Microbial Metabolites, Short-Chain Fatty Acids, Regulate Colonic Treg Cell Homeostasis. Science (2013) 341(6145):569–73. doi: 10.1126/science.1241165
40. Arpaia N, Campbell C, Fan X, Dikiy S, van der Veeken J, deRoos P, et al. Metabolites Produced by Commensal Bacteria Promote Peripheral Regulatory T-Cell Generation. Nature (2013) 504(7480):451–5. doi: 10.1038/nature12726
41. Zimmermann P, Curtis N. Factors That Influence the Immune Response to Vaccination. Clin Microbiol Rev (2019) 32(2):e00084–18. doi: 10.1128/CMR.00084-18
42. Vinolo MAR, Rodrigues HG, Hatanaka E, Sato FT, Sampaio SC, Curi R. Suppressive Effect of Short-Chain Fatty Acids on Production of Proinflammatory Mediators by Neutrophils. J Nutr Biochem (2011) 22(9):849–55. doi: 10.1016/j.jnutbio.2010.07.009
43. Chang PV, Hao L, Offermanns S, Medzhitov R. The Microbial Metabolite Butyrate Regulates Intestinal Macrophage Function via Histone Deacetylase Inhibition. Proc Natl Acad Sci USA (2014) 111(6):2247–52. doi: 10.1073/pnas.1322269111
44. Furusawa Y, Obata Y, Fukuda S, Endo TA, Nakato G, Takahashi D, et al. Commensal Microbe-Derived Butyrate Induces the Differentiation of Colonic Regulatory T Cells. Nature (2013) 504(7480):446–50. doi: 10.1038/nature12721
45. Schulthess J, Pandey S, Capitani M, Rue-Albrecht KC, Arnold I, Franchini F, et al. The Short Chain Fatty Acid Butyrate Imprints an Antimicrobial Program in Macrophages. Immunity (2019) 50(2):432–45.e7. doi: 10.1016/j.immuni.2018.12.018
46. Bachem A, Makhlouf C, Binger KJ, de Souza DP, Tull D, Hochheiser K, et al. Microbiota-Derived Short-Chain Fatty Acids Promote the Memory Potential of Antigen-Activated CD8+ T Cells. Immunity (2019) 51(2):285–97.e5. doi: 10.1016/j.immuni.2019.06.002
47. Balmer ML, Ma EH, Bantug GR, Grählert J, Pfister S, Glatter T, et al. Memory CD8(+) T Cells Require Increased Concentrations of Acetate Induced by Stress for Optimal Function. Immunity (2016) 44(6):1312–24. doi: 10.1016/j.immuni.2016.03.016
48. Balmer ML, Ma EH, Thompson AJ, Epple R, Unterstab G, Lötscher J, et al. Memory CD8+ T Cells Balance Pro- and Anti-Inflammatory Activity by Reprogramming Cellular Acetate Handling at Sites of Infection. Cell Metab (2020) 32(3):457–67.e5. doi: 10.1016/j.cmet.2020.07.004
49. Wu W, Sun M, Chen F, Cao AT, Liu H, Zhao Y, et al. Microbiota Metabolite Short-Chain Fatty Acid Acetate Promotes Intestinal IgA Response to Microbiota Which is Mediated by GPR43. Mucosal Immunol (2017) 10(4):946–56. doi: 10.1038/mi.2016.114
50. Machado MG, Patente TA, Rouillé Y, Heumel S, Melo EM, Deruyter L, et al. Acetate Improves the Killing of Streptococcus Pneumoniae by Alveolar Macrophages via NLRP3 Inflammasome and Glycolysis-HIF-1α Axis. Front Immunol (2022) 13:773261. doi: 10.3389/fimmu.2022.773261
51. Trompette A, Gollwitzer ES, Yadava K, Sichelstiel AK, Sprenger N, Ngom-Bru C, et al. Gut Microbiota Metabolism of Dietary Fiber Influences Allergic Airway Disease and Hematopoiesis. Nat Med (2014) 20(2):159–66. doi: 10.1038/nm.3444
52. Trompette A, Gollwitzer ES, Pattaroni C, Lopez-Mejia IC, Riva E, Pernot J, et al. Dietary Fiber Confers Protection Against Flu by Shaping Ly6c- Patrolling Monocyte Hematopoiesis and CD8+ T Cell Metabolism. Immunity (2018) 48(5):992–1005.e8. doi: 10.1016/j.immuni.2018.04.022
53. Skelly AN, Sato Y, Kearney S, Honda K. Mining the Microbiota for Microbial and Metabolite-Based Immunotherapies. Nat Rev Immunol (2019) 19(5):305–23. doi: 10.1038/s41577-019-0144-5
54. Ruff WE, Greiling TM, Kriegel MA. Host-Microbiota Interactions in Immune-Mediated Diseases. Nat Rev Microbiol (2020) 18(9):521–38. doi: 10.1038/s41579-020-0367-2
55. Lavelle A, Sokol H. Gut Microbiota-Derived Metabolites as Key Actors in Inflammatory Bowel Disease. Nat Rev Gastroenterol Hepatol (2020) 17(4):223–37. doi: 10.1038/s41575-019-0258-z
56. Dong F, Perdew GH. The Aryl Hydrocarbon Receptor as a Mediator of Host-Microbiota Interplay. Gut Microbes (2020) 12(1):1859812. doi: 10.1080/19490976.2020.1859812
57. Lee JS, Cella M, McDonald KG, Garlanda C, Kennedy GD, Nukaya M, et al. AHR Drives the Development of Gut ILC22 Cells and Postnatal Lymphoid Tissues via Pathways Dependent on and Independent of Notch. Nat Immunol (2011) 13(2):144–51. doi: 10.1038/ni.2187
58. Zelante T, Iannitti RG, Cunha C, De Luca A, Giovannini G, Pieraccini G, et al. Tryptophan Catabolites From Microbiota Engage Aryl Hydrocarbon Receptor and Balance Mucosal Reactivity via Interleukin-22. Immunity (2013) 39(2):372–85. doi: 10.1016/j.immuni.2013.08.003
59. Bansal T, Alaniz RC, Wood TK, Jayaraman A. The Bacterial Signal Indole Increases Epithelial-Cell Tight-Junction Resistance and Attenuates Indicators of Inflammation. Proc Natl Acad Sci USA (2010) 107(1):228–33. doi: 10.1073/pnas.0906112107
60. Centers for Disease Control and Prevention (CDC). Impact of Vaccines Universally Recommended for Children–United States, 1990-1998. MMWR Morb Mortal Wkly Rep (1999) 48(12):243–8. doi: doi:10.1001/jama.281.16.1482-JWR0428-2-1
61. Querec TD, Akondy RS, Lee EK, Cao W, Nakaya HI, Teuwen D, et al. Systems Biology Approach Predicts Immunogenicity of the Yellow Fever Vaccine in Humans. Nat Immunol (2009) 10(1):116–25. doi: 10.1038/ni.1688
62. Kurupati R, Kossenkov A, Haut L, Kannan S, Xiang Z, Li Y, et al. Race-Related Differences in Antibody Responses to the Inactivated Influenza Vaccine are Linked to Distinct Pre-Vaccination Gene Expression Profiles in Blood. Oncotarget (2016) 7(39):62898–911. doi: 10.18632/oncotarget.11704
63. Doi H, Kanto T. Factors Influencing the Durability of Hepatitis B Vaccine Responses. Vaccine (2021) 39(36):5224–30. doi: 10.1016/j.vaccine.2021.07.017
64. McElhaney J. Responses to Influenza Vaccination in Different T-Cell Subsets: A Comparison of Healthy Young and Older Adults. Vaccine (1998) 16(18):1742–7. doi: 10.1016/s0264-410x(98)00133-9
65. McElhaney JE, Meneilly GS, Beattie BL, Helgason CD, Lee S-F, Devine RDO, et al. The Effect of Influenza Vaccination on IL2 Production in Healthy Elderly: Implications for Current Vaccination Practices. J Gerontol (1992) 47(1):M3–8. doi: 10.1093/geronj/47.1.m3
66. Nakaya HI, Hagan T, Duraisingham SS, Lee EK, Kwissa M, Rouphael N, et al. Systems Analysis of Immunity to Influenza Vaccination Across Multiple Years and in Diverse Populations Reveals Shared Molecular Signatures. Immunity (2015) 43(6):1186–98. doi: 10.1016/j.immuni.2015.11.012
67. Oh JZ, Ravindran R, Chassaing B, Carvalho FA, Maddur MS, Bower M, et al. TLR5-Mediated Sensing of Gut Microbiota is Necessary for Antibody Responses to Seasonal Influenza Vaccination. Immunity (2014) 41(3):478–92. doi: 10.1016/j.immuni.2014.08.009
68. Hagan T, Cortese M, Rouphael N, Boudreau C, Linde C, Maddur MS, et al. Antibiotics-Driven Gut Microbiome Perturbation Alters Immunity to Vaccines in Humans. Cell (2019) 178(6):1313–28.e13. doi: 10.1016/j.cell.2019.08.010
69. Ng SC, Peng Y, Zhang L, Mok CK, Zhao S, Li A, et al. Gut Microbiota Composition is Associated With SARS-CoV-2 Vaccine Immunogenicity and Adverse Events. Gut (2022), gutjnl–2021-326563. doi: 10.1136/gutjnl-2021-326563
70. Gut Microbiota Profile and Its Impact on Immunity Status in COVID-19 Vaccinated Cohorts (2022). Available at: https://clinicaltrials.gov/ct2/show/NCT04980560.
71. Collins N, Belkaid Y. Do the Microbiota Influence Vaccines and Protective Immunity to Pathogens?: Engaging Our Endogenous Adjuvants. Cold Spring Harb Perspect Biol (2018) 10(2):a028860. doi: 10.1101/cshperspect.a028860
72. Kim D, Kim Y-G, Seo S-U, Kim D-J, Kamada N, Prescott D, et al. Nod2-Mediated Recognition of the Microbiota Is Critical for Mucosal Adjuvant Activity of Cholera Toxin. Nat Med (2016) 22(5):524–30. doi: 10.1038/nm.4075
73. Lousada-Dietrich S, Jogdand PS, Jepsen S, Pinto VV, Ditlev SB, Christiansen M, et al. A Synthetic TLR4 Agonist Formulated in an Emulsion Enhances Humoral and Type 1 Cellular Immune Responses Against GMZ2–a GLURP-MSP3 Fusion Protein Malaria Vaccine Candidate. Vaccine (2011) 29(17):3284–92. doi: 10.1016/j.vaccine.2011.02.022
74. Lynn DJ, Benson SC, Lynn MA, Pulendran B. Modulation of Immune Responses to Vaccination by the Microbiota: Implications and Potential Mechanisms. Nat Rev Immunol (2022) 22(1):33–46. doi: 10.1038/s41577-021-00554-7
75. Hill C, Guarner F, Reid G, Gibson GR, Merenstein DJ, Pot B, et al. Expert Consensus Document. The International Scientific Association for Probiotics and Prebiotics Consensus Statement on the Scope and Appropriate Use of the Term Probiotic: Expert Consensus Document. Nat Rev Gastroenterol Hepatol (2014) 11(8):506–14. doi: 10.1038/nrgastro.2014.66
76. Glück U, Gebbers J-O. Ingested Probiotics Reduce Nasal Colonization With Pathogenic Bacteria (Staphylococcus Aureus, Streptococcus Pneumoniae, and Beta-Hemolytic Streptococci). Am J Clin Nutr (2003) 77(2):517–20. doi: 10.1093/ajcn/77.2.517
77. Hao Q, Dong BR, Wu T. Probiotics for Preventing Acute Upper Respiratory Tract Infections. Cochrane Database Syst Rev (2015) 2):CD006895. doi: 10.1002/14651858.CD006895.pub3
78. King S, Glanville J, Sanders ME, Fitzgerald A, Varley D. Effectiveness of Probiotics on the Duration of Illness in Healthy Children and Adults Who Develop Common Acute Respiratory Infectious Conditions: A Systematic Review and Meta-Analysis. Br J Nutr (2014) 112(1):41–54. doi: 10.1017/S0007114514000075
79. Jung Y-J, Lee Y-T, Ngo VL, Cho Y-H, Ko E-J, Hong S-M, et al. Heat-Killed Lactobacillus Casei Confers Broad Protection Against Influenza A Virus Primary Infection and Develops Heterosubtypic Immunity Against Future Secondary Infection. Sci Rep (2017) 7(1):41598-017-17487-8. doi: 10.1038/s41598-017-17487-8
80. Villena J, Li C, Vizoso-Pinto MG, Sacur J, Ren L, Kitazawa H. Lactiplantibacillus Plantarum as a Potential Adjuvant and Delivery System for the Development of SARS-CoV-2 Oral Vaccines. Microorganisms (2021) 9(4):683. doi: 10.3390/microorganisms9040683
81. Xu J, Ren Z, Cao K, Li X, Yang J, Luo X, et al. Boosting Vaccine-Elicited Respiratory Mucosal and Systemic COVID-19 Immunity in Mice With the Oral Lactobacillus Plantarum. Front Nutr (2021) 8:789242. doi: 10.3389/fnut.2021.789242
82. Cox AJ, Pyne DB, Saunders PU, Fricker PA. Oral Administration of the Probiotic Lactobacillus Fermentum VRI-003 and Mucosal Immunity in Endurance Athletes. Br J Sports Med (2010) 44(4):222–6. doi: 10.1136/bjsm.2007.044628
83. de Vrese M, Winkler P, Rautenberg P, Harder T, Noah C, Laue C, et al. Effect of Lactobacillus Gasseri PA 16/8, Bifidobacterium Longum SP 07/3, B. bifidum MF 20/5 on common cold episodes: a double blind, randomized, controlled trial. Clin Nutr (2005) 24(4):481–91. doi: 10.1016/j.clnu.2005.02.006
84. Kekkonen RA, Vasankari TJ, Vuorimaa T, Haahtela T, Julkunen I, Korpela R. The Effect of Probiotics on Respiratory Infections and Gastrointestinal Symptoms During Training in Marathon Runners. Int J Sport Nutr Exerc Metab (2007) 17(4):352–63. doi: 10.1123/ijsnem.17.4.352
85. Tiollier E, Chennaoui M, Gomez-Merino D, Drogou C, Filaire E, Guezennec CY. Effect of a Probiotics Supplementation on Respiratory Infections and Immune and Hormonal Parameters During Intense Military Training. Mil Med (2007) 172(9):1006–11. doi: 10.7205/milmed.172.9.1006
86. Mezhenskaya D, Isakova-Sivak I, Gupalova T, Bormotova E, Kuleshevich E, Kramskaya T, et al. A Live Probiotic Vaccine Prototype Based on Conserved Influenza a Virus Antigens Protect Mice Against Lethal Influenza Virus Infection. Biomedicines (2021) 9(11):1515. doi: 10.3390/biomedicines9111515
87. Lee JE, Kye Y-C, Park S-M, Shim B-S, Yoo S, Hwang E, et al. Bacillus Subtilis Spores as Adjuvants Against Avian Influenza H9N2 Induce Antigen-Specific Antibody and T Cell Responses in White Leghorn Chickens. Vet Res (2020) 51(1):68. doi: 10.1186/s13567-020-00788-8
88. Lee J-S, Poo H, Han DP, Hong S-P, Kim K, Cho MW, et al. Mucosal Immunization With Surface-Displayed Severe Acute Respiratory Syndrome Coronavirus Spike Protein on Lactobacillus Casei Induces Neutralizing Antibodies in Mice. J Virol (2006) 80(8):4079–87. doi: 10.1128/JVI.80.8.4079-4087.2006
89. Jespersen L, Tarnow I, Eskesen D, Morberg CM, Michelsen B, Bügel S, et al. Effect of Lactobacillus Paracasei Subsp. Paracasei, L. Casei 431 on Immune Response to Influenza Vaccination and Upper Respiratory Tract Infections in Healthy Adult Volunteers: A Randomized, Double-Blind, Placebo-Controlled, Parallel-Group Study. Am J Clin Nutr (2015) 101(6):1188–96. doi: 10.3945/ajcn.114.103531
90. Maruyama M, Abe R, Shimono T, Iwabuchi N, Abe F, Xiao J-Z. The Effects of non-Viable Lactobacillus on Immune Function in the Elderly: A Randomised, Double-Blind, Placebo-Controlled Study. Int J Food Sci Nutr (2016) 67(1):67–73. doi: 10.3109/09637486.2015.1126564
91. Van Puyenbroeck K, Hens N, Coenen S, Michiels B, Beunckens C, Molenberghs G, et al. Efficacy of Daily Intake of Lactobacillus Casei Shirota on Respiratory Symptoms and Influenza Vaccination Immune Response: A Randomized, Double-Blind, Placebo-Controlled Trial in Healthy Elderly Nursing Home Residents. Am J Clin Nutr (2012) 95(5):1165–71. doi: 10.3945/ajcn.111.026831
92. Rizzardini G, Eskesen D, Calder PC, Capetti A, Jespersen L, Clerici M. Evaluation of the Immune Benefits of Two Probiotic Strains Bifidobacterium Animalis Ssp. Lactis, BB-12® and Lactobacillus Paracasei Ssp. Paracasei, L. Casei 431® in an Influenza Vaccination Model: A Randomised, Double-Blind, Placebo-Controlled Study. Br J Nutr (2012) 107(6):876–84. doi: 10.1017/S000711451100420X
93. Boge T, Rémigy M, Vaudaine S, Tanguy J, Bourdet-Sicard R, van der Werf S. A Probiotic Fermented Dairy Drink Improves Antibody Response to Influenza Vaccination in the Elderly in Two Randomised Controlled Trials. Vaccine (2009) 27(41):5677–84. doi: 10.1016/j.vaccine.2009.06.094
94. Bosch M, Méndez M, Pérez M, Farran A, Fuentes MC, Cuñé J. Lactobacillus Plantarum CECT7315 and CECT7316 Stimulate Immunoglobulin Production After Influenza Vaccination in Elderly. Nutr Hosp (2012) 27(2):504–9. doi: 10.1590/S0212-16112012000200023
95. Lei W-T, Shih P-C, Liu S-J, Lin C-Y, Yeh T-L. Effect of Probiotics and Prebiotics on Immune Response to Influenza Vaccination in Adults: A Systematic Review and Meta-Analysis of Randomized Controlled Trials. Nutrients (2017) 9(11):1175. doi: 10.3390/nu9111175
96. Karl JP. Gut Microbiota-Targeted Interventions for Reducing the Incidence, Duration, and Severity of Respiratory Tract Infections in Healthy non-Elderly Adults. Mil Med (2021) 186(3–4):e310–8. doi: 10.1093/milmed/usaa261
97. Yeh T-L, Shih P-C, Liu S-J, Lin C-H, Liu J-M, Lei W-T, et al. The Influence of Prebiotic or Probiotic Supplementation on Antibody Titers After Influenza Vaccination: A Systematic Review and Meta-Analysis of Randomized Controlled Trials. Drug Des Devel Ther (2018) 12:217–30. doi: 10.2147/dddt.s155110
98. Xavier-Santos D, Padilha M, Fabiano GA, Vinderola G, Gomes Cruz A, Sivieri K, et al. Evidences and Perspectives of the Use of Probiotics, Prebiotics, Synbiotics, and Postbiotics as Adjuvants for Prevention and Treatment of COVID-19: A Bibliometric Analysis and Systematic Review. Trends Food Sci Technol (2022) 120:174–92. doi: 10.1016/j.tifs.2021.12.033
99. van Best N, Hornef MW, Savelkoul PHM, Penders J. On the Origin of Species: Factors Shaping the Establishment of Infant’s Gut Microbiota: ESTABLISHMENT OF Infant’s GUT MICROBIOTA. Birth Defects Res C Embryo Today (2015) 105(4):240–51. doi: 10.1002/bdrc.21113
100. Charbonneau MR, O’Donnell D, Blanton LV, Totten SM, Davis JCC, Barratt MJ, et al. Sialylated Milk Oligosaccharides Promote Microbiota-Dependent Growth in Models of Infant Undernutrition. Cell (2016) 164(5):859–71. doi: 10.1016/j.cell.2016.01.024
101. Penders J, Thijs C, Vink C, Stelma FF, Snijders B, Kummeling I, et al. Factors Influencing the Composition of the Intestinal Microbiota in Early Infancy. Pediatrics (2006) 118(2):511–21. doi: 10.1542/peds.2005-2824
102. Sela DA, Mills DA. Nursing Our Microbiota: Molecular Linkages Between Bifidobacteria and Milk Oligosaccharides. Trends Microbiol (2010) 18(7):298–307. doi: 10.1016/j.tim.2010.03.008
103. Ewaschuk JB, Diaz H, Meddings L, Diederichs B, Dmytrash A, Backer J, et al. Secreted Bioactive Factors From Bifidobacterium Infantis Enhance Epithelial Cell Barrier Function. Am J Physiol Gastrointest Liver Physiol (2008) 295(5):G1025–34. doi: 10.1152/ajpgi.90227.2008
104. Pannaraj PS, Li F, Cerini C, Bender JM, Yang S, Rollie A, et al. Association Between Breast Milk Bacterial Communities and Establishment and Development of the Infant Gut Microbiome. JAMA Pediatr (2017) 171(7):647. doi: 10.1001/jamapediatrics.2017.0378
105. Arrieta M-C, Stiemsma LT, Dimitriu PA, Thorson L, Russell S, Yurist-Doutsch S, et al. Early Infancy Microbial and Metabolic Alterations Affect Risk of Childhood Asthma. Sci Transl Med (2015) 7(307):307ra152. doi: 10.1126/scitranslmed.aab2271
106. Riede L, Grube B, Gruenwald J. Larch Arabinogalactan Effects on Reducing Incidence of Upper Respiratory Infections. Curr Med Res Opin (2013) 29(3):251–8. doi: 10.1185/03007995.2013.765837
107. Langkamp-Henken B, Bender BS, Gardner EM, Herrlinger-Garcia KA, Kelley MJ, Murasko DM, et al. Nutritional Formula Enhanced Immune Function and Reduced Days of Symptoms of Upper Respiratory Tract Infection in Seniors: Nutritional Formula Influences Immunity. J Am Geriatr Soc (2004) 52(1):3–12. doi: 10.1111/j.1532-5415.2004.52003.x
108. Nagafuchi S, Yamaji T, Kawashima A, Saito Y, Takahashi T, Yamamoto T, et al. Effects of a Formula Containing Two Types of Prebiotics, Bifidogenic Growth Stimulator and Galacto-Oligosaccharide, and Fermented Milk Products on Intestinal Microbiota and Antibody Response to Influenza Vaccine in Elderly Patients: A Randomized Controlled Trial. Pharmaceuticals (Basel) (2015) 8(2):351–65. doi: 10.3390/ph8020351
109. Rooks MG, Garrett WS. Gut Microbiota, Metabolites and Host Immunity. Nat Rev Immunol (2016) 16(6):341–52. doi: 10.1038/nri.2016.42
110. Levy M, Blacher E, Elinav E. Microbiome, Metabolites and Host Immunity. Curr Opin Microbiol (2017) 35:8–15. doi: 10.1016/j.mib.2016.10.003
111. Kim S, Kim J-H, Park BO, Kwak YS. Perspectives on the Therapeutic Potential of Short-Chain Fatty Acid Receptors. BMB Rep (2014) 47(3):173–8. doi: 10.5483/bmbrep.2014.47.3.272
112. Zaman K, Dang DA, Victor JC, Shin S, Yunus M, Dallas MJ, et al. Efficacy of Pentavalent Rotavirus Vaccine Against Severe Rotavirus Gastroenteritis in Infants in Developing Countries in Asia: A Randomised, Double-Blind, Placebo-Controlled Trial. Lancet (2010) 376(9741):615–23. doi: 10.1016/S0140-6736(10)60755-6
113. John TJ. Antibody Response of Infants in Tropics to Five Doses of Oral Polio Vaccine. Br Med J (1976) 1(6013):812. doi: 10.1136/bmj.1.6013.812
114. John TJ, Jayabal P. Oral Polio Vaccination of Children in the Tropics. I. The Poor Seroconversion Rates and the Absence of Viral Interference. Am J Epidemiol (1972) 96(4):263–9. doi: 10.1093/oxfordjournals.aje.a121457
115. De Filippo C, Cavalieri D, Di Paola M, Ramazzotti M, Poullet JB, Massart S, et al. Impact of Diet in Shaping Gut Microbiota Revealed by a Comparative Study in Children From Europe and Rural Africa. Proc Natl Acad Sci USA (2010) 107(33):14691–6. doi: 10.1073/pnas.1005963107
Keywords: virus, microbiota, vaccine, respiratory virus, immune response
Citation: Gonçalves JB, Borges TJ and de Souza APD (2022) Microbiota and the Response to Vaccines Against Respiratory Virus. Front. Immunol. 13:889945. doi: 10.3389/fimmu.2022.889945
Received: 04 March 2022; Accepted: 12 April 2022;
Published: 06 May 2022.
Edited by:
John Willaim Sleasman, Duke University, United StatesReviewed by:
Jian Tan, The University of Sydney, AustraliaCopyright © 2022 Gonçalves, Borges and de Souza. This is an open-access article distributed under the terms of the Creative Commons Attribution License (CC BY). The use, distribution or reproduction in other forums is permitted, provided the original author(s) and the copyright owner(s) are credited and that the original publication in this journal is cited, in accordance with accepted academic practice. No use, distribution or reproduction is permitted which does not comply with these terms.
*Correspondence: Ana Paula Duarte de Souza, YW5hLmR1YXJ0ZUBwdWNycy5icg==