- 1Institute for Hygiene and Applied Immunology, Center for Pathophysiology, Infectiology and Immunology, Medical University of Vienna, Vienna, Austria
- 2Institute of Applied Physics, TU Wien, Vienna, Austria
Efficient scanning of tissue that T cells encounter during their migratory life is pivotal to protective adaptive immunity. In fact, T cells can detect even a single antigenic peptide/MHC complex (pMHC) among thousands of structurally similar yet non-stimulatory endogenous pMHCs on the surface of antigen-presenting cells (APCs) or target cells. Of note, the glycocalyx of target cells, being composed of proteoglycans and bulky proteins, is bound to affect and even modulate antigen recognition by posing as a physical barrier. T cell-resident microvilli are actin-rich membrane protrusions that puncture through such barriers and thereby actively place the considerably smaller T-cell antigen receptors (TCRs) in close enough proximity to APC-presented pMHCs so that productive interactions may occur efficiently yet under force. We here review our current understanding of how the plasticity of T-cell microvilli and physicochemical properties of the glycocalyx may affect early events in T-cell activation. We assess insights gained from studies on T-cell plasma membrane ultrastructure and provide an update on current efforts to integrate biophysical aspects such as the amplitude and directionality of TCR-imposed mechanical forces and the distribution and lateral mobility of plasma membrane-resident signaling molecules into a more comprehensive view on sensitized T-cell antigen recognition.
Introduction
The adaptive immune response is an extraordinarily complex process involving a multitude of different cell types, transmitters, and effector molecules performing their intended function in diverse tissue environments, some of which are altered by disease and infection. Slight deviations within the involved mechanisms can lead to severe medical complications, namely, allergies, autoimmune diseases, hypo- or hyper-reactions to invading pathogens, and the development of cancer. The degree of fine-tuning required for immune protection becomes apparent at multiple regulatory levels controlling T-cell activation. The pivotal event preceding many of the ensuing cellular interactions concerns the specific molecular recognition of processed pathogenic peptides displayed in the context of the major histocompatibility complex (MHC and peptide-presenting MHC; pMHC) by T cells via their clonotypic and genetically recombined T-cell antigen receptors (TCRs).
Interactions between stimulatory pMHCs and TCRs are highly specific and confer exquisite T-cell antigen sensitivity, a sine qua non considering the consequences of recognition failure. It is, however, not yet clear how such a level of specificity and sensitivity is maintained, since measured biochemical affinities between TCRs and pMHCs are astonishingly low. A number of models have been conceived and tested in the last two decades. Experimental efforts focused on revealing the molecular machinery involved in antigen recognition events and led to the formulation of concepts implicating kinetic segregation, kinetic proof-reading, co-receptor involvement, ligation-triggered conformational changes, serial engagement, and the contribution of mechanical forces in early recognition events [reviewed in (1)]. These models have their merits, and the ground truth will very likely be a combination of their aspects.
The complex interaction of naïve antigen-inexperienced T cells with antigen-presenting cells such as dendritic and B cells can be described on different spatial and temporal scales (see Figure 1 for an illustration of these processes). Highly dynamic cellular interactions involving massive cytoskeletal rearrangements transpire during the entirety of the cell–cell contact. Before antigen recognition, pre-existing membrane protrusions such as microvilli scan the surface of the target cell in search of their cognate antigen (2–5). As a result, physical barriers such as the glycocalyx of the target cell are overcome by the surveilling microvilli. During scanning, surface receptors at the tip of the microvilli are subject to a large range of mechanical forces such as tensile, compressive, and shear stresses (6). Once a specific recognition event is established, the T cell receives a movement arrest signal and the two interacting cells start reorganizing their conjugation plane, which involves massive membrane undulations and the formation of other membrane projections such as invadosome-like structures (2, 7–9). Proteins within the entire conjugation plane become spatially reorganized, forming the immunological synapse with a central and peripheral domain (10).
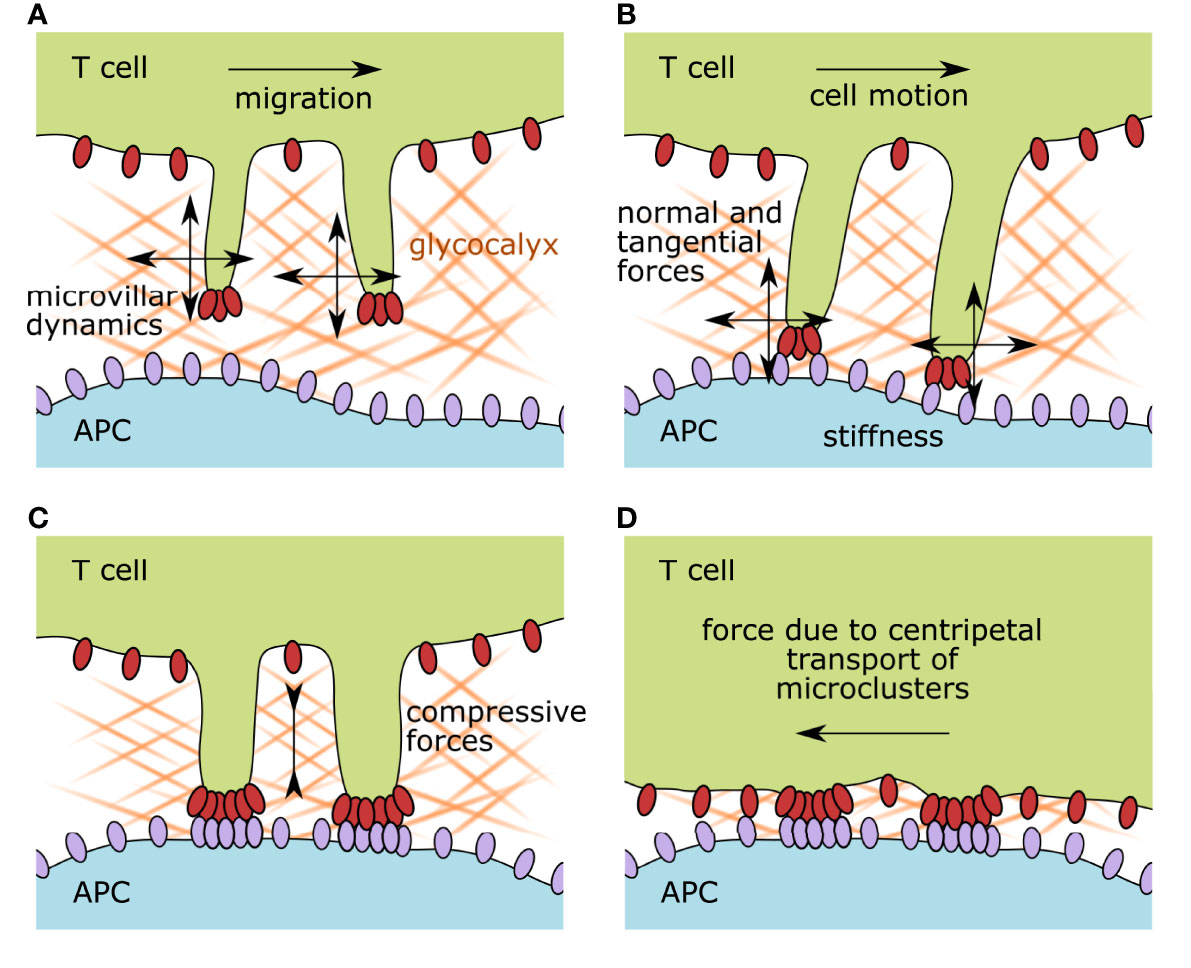
Figure 1 Illustration of the membrane organization of scanning and activated T cells and the accompanying possible mechanical forces affecting surface receptors. (A) During immune surveillance, T cells scan target cells via microvillar protrusions. The first physical barrier they encounter is the glycocalyx of the target cell. Antigen scanning speed is impacted by the glycocalyx physicochemical properties such as stiffness, density, and matrix composition, but also by the migrational speed of the T cell itself and its microvillar dynamics. (B) As soon as surface receptors on the microvillar tips interact with their ligands on the opposing membrane, the formed bonds experience a force vector with normal and tangential components. The surface stiffness of the target cell and the microvillar elasticity also influence the interacting receptor–ligand pairs. (C) Upon recognition of a cognate antigen, T-cell activation starts. Surface receptor molecules build signaling platforms while the two participating plasma membranes approach each other, compressing the remaining glycocalyx components. (D) After the initiation of TCR signaling, the zonal organization of the immunological synapse is established and signaling foci, called microclusters, are pulled by the actin cytoskeleton toward the center of the cell. This dragging motion is also causing mechanical strain on the involved receptor–ligand pairs. APC, antigen-presenting cell.
The unique two- and three-dimensional properties of immune synapses are likely to influence the dynamics of intrinsic receptor–ligand interactions (11). For example, massive membrane rearrangements ensue after initial contact and produce spatial constraints that eventually result in the molecular segregation of membrane receptors and ligands based on the size of their extracellular domains (12). Signal-maintaining microclusters are formed, containing ligated TCRs, which are subsequently dragged by the cytoskeleton toward the center of the synapse (13–17). This active process creates drag on other constituents, causing membrane undulations due to elastic deformation and relaxation (18). In this fashion, measurable pulling forces are exerted by the regulated, centripetal flow of the cortical actin cytoskeleton (19).
Considering the temporal flow of events, one must consider that signaling events during T-cell activation are divided into early recognition events, which eventually lead to motility arrest, and signal-maintaining events. Early recognition events fulfill the purpose of fast and efficient antigen screening (20, 21), whereas later events are needed to maintain a steady T-cell response at a continuous antigenic stimulus (14, 17, 22, 23).
In this review, we highlight the cell biological and biophysical features of T-cell microvilli, which act as antigen-sensing units and, in turn, reevaluate the impact of physical barriers and mechanical forces on immune surveillance.
Antigen Scanning Entities: Microvilli of T Cells
T cells are exceptionally motile as they roam a multitude of different environments during their life cycle (24, 25). After differentiation and selection in the thymus, naïve T cells move into the blood and lymphatic system to reach secondary lymphoid systems. In doing so, T cells are exposed to strong shear forces caused, for example, by the dynamics of the blood flow. To leave the blood stream, T cells perform a complex maneuver along the endothelial wall, comprising a selectin-mediated rolling and adhesion cycle that eventually leads to diapedesis. After entering a lymph node or tissue of interest, T cells start migrating through dense three-dimensional extracellular matrices while continuously screening antigen-presenting cells (APCs) or target cells, which they continuously encounter for cognate antigen. Upon stimulation, T cells switch their migration mode and form cell–cell interfaces, which, depending on tissue properties, are termed immunological kinapses or synapses. Subsequently, they start proliferating, and eventually start their surveilling migration anew throughout the body. Further triggering of an antigen-experienced T cell leads to the fulfillment of its effector function according to the subtype of the T cell.
During immune surveillance, the membrane ultrastructure of scanning T cells is especially dynamic in order to guarantee adaptation to different physical environments and functions. Prominent structures are the actin-rich membrane protrusions called microvilli, which play a central role in antigen surveillance.
Physical Barriers in Immune Surveillance
An interesting aspect of T-cell interactions concerns the biophysical environment T cells experience while they are scanning their surroundings. T cells are actively probing for pathogen-derived or otherwise atypical or non-endogenous peptides presented on the surface of cells that they encounter in their migratory life. However, cells are protected by the glycocalyx, a dense and wide coat of extracellular polysaccharides and proteins, which creates a physical barrier, preventing the close apposition of the cellular membranes and, consequently, any intercellular ligand–receptor interaction (26–28). To deal with this, T cells feature membrane protrusions that can puncture through the cell-resident glycocalyx to efficiently scan large portions of a large variety of cells and drive TCR-specific signaling (2, 4, 7, 29). The glycocalyx is a naturally occurring physical barrier made of extracellular branched carbohydrates, glycolipids, glycoproteins, and proteoglycans that covers the plasma membrane of cells (30). The involved carbohydrates (i) can be directly linked to their respective anchor molecules via N- or O-glycosidic bonds or are independent entities within the matrix, (ii) are continuously remodeled by cellular enzymes, (iii) are primarily negatively charged, and (iv) play an important role in cellular processes such as signal amplification, adhesion, migration, and cell death (31). The complexity and height of this dense, gel-like coating can vary from 250 to >500 nm (32) and it is filled with ions, growth factors, chemo- and cytokines.
The exact composition of the glycocalyx has been characterized in detail for endothelial cells: prominent membrane-anchored proteoglycan groups are syndecans and glypicans, whereas other proteoglycans such as mimecan, perlecan, and biglycan are actively secreted into the extracellular space and blood stream (33). These proteoglycans form a dense network by associating with glycosaminoglycans (GAGs) such as heparan sulfate, dermatan sulfate, chondroitin sulfate, and hyaluronic acid, to name a few ubiquitous GAGs. Apart from these components, a large variety of glycoproteins such as selectins, integrins, and immunoglobulin-like proteins also participate in forming a dense extracellular network (33). It is beyond the scope of this review to discuss all the participating glycocalyx players, but it is important to note the existence of a complex, multi-layered, and highly dynamic layer beyond the plasma membrane that impacts any cell–cell interaction.
The glycocalyx of the T cell consists mainly of a few prominent surface proteins with large extracellular domains, namely CD43 and CD44, and the protein tyrosine phosphatases CD45 and CD148. These proteins' extracellular domains are 3–4 fold larger in length than the physiological intermembrane distance, allowing for a pMHC–TCR bond (~15 nm) (26, 34), and resemble stiff rod-like structures that are unlikely to bend in response to external tensile forces (35). Similarly, CD148 and CD45 also carry large extracellular domains but fulfill additional signaling functions in the wake of TCR triggering. During the immune synapse formation, these proteins and integrin LFA-1 binding to ICAM-1 are positioned in distinct zones creating varying intermembrane distances. This zonal reorganization after TCR triggering leads to steep membrane curvatures accompanied by dynamic membrane tension profiles in the contacting APC and T cell membranes [reviewed in (34)]. Not much is yet known with regard to the presence of typical membrane-anchored and secreted proteoglycans and glycoproteins in the glycocalyx of T cells, yet one study investigated the upregulation of Mucin-1 (a very large glycocalyx-building proteoglycan) after mitogenic stimulus in activated T cells (36) and another study detected hyaluronan (a prominent GAG) on the surface of T cells in certain conditions (37). However, given their migratory lifestyle and the necessity to visit and scan a large variety of tissues, the presence of a bulky glycocalyx network seems disadvantageous and may only be upregulated in certain scenarios such as trafficking and homing. Also, T cells exhibit a stiffness modulus of only ~85 Pa, which is very soft in comparison to other cell systems (38). This has implications for cell–cell interactions, as the cell with the lower rigidity spreads at the interface. Recent rheological measurements of the viscoelastic properties of T cells during activation show a 2–3 fold increase in stiffness after stimulation with an activating microbead (39). The authors also performed AFM experiments with T cell–APC conjugates and concluded that mechanical changes occur within seconds of initial contact (39).
The glycocalic layers of professional APCs have so far been investigated to a much lesser extent, and given the migratory life of these cells, it is unclear if the glycocalyx layers are up or downregulated in their immature/mature states or the varying lymphoid environments they reside in. One study showed the presence of Mucin-1 on dendritic cells in vivo (40). Also, a recent publication investigated the contribution of hyaluronan (HA) to the glycocalyx layer of migratory DCs and discovered a 400–500 nm thick glycocalyx layer anchored and regulated by the HA-receptor CD44 (41). The study also showed that the HA content within the glycocalyx was upregulated for mature DCs compared to immature DCs, and consequently, that the presence of HA glycocalyx is necessary for trafficking over the lymphatic endothelium and allowing crawling along the endothelia (41, 42). This aspect becomes crucial considering that long-lived MHC-dependent T cell–DC interactions occur on the luminal side of afferent lymphatic capillaries (43, 44).
An interesting mechanism was recently uncovered in the work of Imbert et al., characterizing the immune-modulatory impact of the glycocalyx on the target cell during phagocytosis in in vitro and in vivo settings (45). The elegant experiments showed that the presence of a repulsive glycocalyx on target cells prevents phagocytosis, leading to effective immune evasion, and that, similarly the upregulation of glycocalyx layers on the phagocyte itself led to the same inhibition of phagocytosis. The study clearly showed that glycocalyx layers can actively prevent immune recognition and the triggering of surface receptors by restricting their accessibility. Along this line of thought, cancerous cells have been shown to alter their glycocalyx composition (46, 47), in that they vary its height and other biophysical parameters, thereby promoting their immune evasion capabilities (48, 49).
Biophysical parameters of the glycocalyx have been measured using AFM nano-indentation (50), resulting in an elastic modulus of around 0.39 kPa. The plasma membrane lacking a glycocalyx has been found to be less flexible with an elastic modulus of about 3 kPa. The corresponding stiffness of cells has been experimentally determined to be in the range of 10 Pa to 10 kPa, the variability resulting from different cell types and confounding parameters like intracellular pressure and actin–myosin contractility of the underlying cytoskeleton and differences in methodology (51). Interestingly, spread mesenchymal stem cells exhibit a larger stiffness modulus than rounded ones, with 3.2 and 2.5 kPa, respectively (52). The stiffness of monocyte-derived dendritic cells has been reported to be in the range of 0.5 kPa, with their stiffness changing upon inflammation (38), whereas another study reports a 2–3 fold increase in stiffness for maturing DCs (within the range of 2 to 8 kPa) (53).
All these aspects become relevant when considering the sensitivity of the T cell toward different substrate stiffnesses. The stiffness and porosity of the glycocalyx of both T cell and APC may hence be critical for antigen accessibility, whereas the APC cortex stiffness may be critical for antigen sensitivity and subsequent signaling (54). It has been comprehensively shown that the stiffness of the ligand-presenting surface impacts T cell signaling, proliferation, and differentiation (54–59). Some of these studies report a positive correlation between the substrate stiffness and T-cell activation (55–57), while others show an inverse correlation (54, 58). The investigated stiffness ranged from a few Pa to MPa, while most studies report a maximum cellular response at a substrate stiffness of 100 kPa. In a more physiological setting, Blumenthal et al. investigated the impact of the cortical stiffness of dendritic cells on the T-cell response (53): by varying the stiffness of stimulatory hydrogels in the physiological range of immature and mature DCs (2 to 8 kPa, respectively), the authors showed an increase in CD4+ T-cell antigen sensitivity and responses under stiffness conditions reflecting that of mature DCs. Strikingly and in contrast, CD8+ T cells only showed modest sensitivity toward stiffness. By substituting pMHC as a stimulatory ligand for activating antibodies, the authors revealed a dependence of the stiffness response on the type of TCR–ligand engagement. Within the measured physiological stiffness range (2–8 kPa), this limitation dramatically affected T-cell responsiveness, as only treatment with pMHCs but not with antibodies triggered T-cell activation under these conditions, except for very high ligand densities (53). Considering the effect of substrate stiffness on T-cell effector function, recent studies reporting on the vulnerability of cancer cells to T-cell cytolytic activity showed a positive correlation between immune response and cellular rigidity (60). As a consequence, cancer cells may actively evade antitumor immune responses by softening their cortical actin cytoskeleton.
Microvilli Dimensions and Localization
In the mid-1970s to 1980s, efforts were made to morphologically characterize lymphocytes using the then newly developed technique of scanning electron microscopy, and consequently, microvillar protrusions were discovered covering the surface of lymphocytes (61–63). These membrane structures were described as being dynamic and dependent on the cell cycle, temperature, inter-cell contact, and even antigenic stimulus (64). Twenty-five years later, it was possible to detect the presence of membrane protrusions on the surface of circulating T cells (65), and prior to engagement with antigen-presenting cells (2, 4, 66) and within lymph nodes (3). These studies demonstrated the existence of such membrane protrusions during initial contact formation. Cai et al. quantified the occurrence of microvillar protrusions via Lattice Light Sheet Microscopy: the cell membrane is densely packed with highly dynamic microvillar protrusions that cover 98% of the cellular surface over a time period of 1 min (2). After the formation of the immunological synapse, the membrane structure ultimately becomes more planar, creating wider close-contact zones between the participating cell membranes (66, 67). Leukocyte microvillar protrusions, used for initial tethering to and rolling along the endothelia within the high shear force conditions of the blood stream (9, 68, 69), will not be the focus of this review in view of their largely different functions and surface receptor composition.
Electron-microscopic snapshots of T cells interacting with antigen-presenting cells allow the characterization of the morphology of membrane protrusions on resting, scanning, and activating T cells. The protrusions are 300–400 nm long (median, up to 4 µm has been reported) and 70–350 nm in diameter with a density of 3–4 protrusions per µm2 on a resting T cell (3, 5, 7, 65, 70). So far, we are not aware of any comparative study quantifying the abundance of microvilli on circulating and scanning T cells. Interestingly, the dimensions of microvillar protrusions are similar when comparing murine and human blood-isolated lymphocytes, even though human lymphocytes are in general twice as large in cell diameter (65). This conserved feature size of the microvilli indicates the existence of a common physical parameter T cells must overcome during their life time, e.g., the thickness of the glycocalyx during immune surveillance or similar shear forces during rolling tethering in the blood stream. Upon contact formation with a professional antigen-presenting cell, the protrusion tips form close contacts with the opposing cellular membrane in an antigen-independent manner, i.e., the interaction frequency and protrusion density remain unchanged during surveillance and immune synapse formation (2). Upon TCR ligation, however, the ensuing antigen-specific interactions appear to lead to a longer dwell time of the microvilli tip in the synaptic area (2) and may even deform the target cell membrane, forming invadosome-like structures (4). It still remains to be investigated whether microvilli and invadosome-like structures are in fact morphologically and functionally similar protrusions. Recent studies from the Husson as well as the Hivroz group (71–73) have revealed the formation of large membrane protrusions after synapse formation, the physiological role of which remains unknown, but may not be confused with microvillar protrusions during diapedesis and immune surveillance.
Microvilli were described as continuously forming under the leading edge of the lamellipodium of migrating T cells. Upon antigen encounter and synapse formation, membrane protrusions can be preferentially observed forming at the synaptic periphery of the T cell–APC interface (4). Importantly, the transient interactions scanning microvilli form with their target surface do not cease after antigen-dependent triggering of the T cell (2), much in line with the findings that synapse maintenance depends on continuous recruitment of new TCR–pMHC interactions (14, 17, 74, 75).
Monitoring and characterizing of T cell microvilli during antigen scanning remains a big challenge within the otherwise extensively researched field of T-cell activation. Previous investigations are confounded by varying cell types, T-cell receptors, and presented ligands, but ultimately by the applied techniques and stimulation platforms. Studies were especially hindered by the lack of methods to investigate three-dimensional and highly dynamic nanostructures on living cells (7). A few research groups applied indirect observation methods to prove the existence of membrane protrusions within the immunological synapse, such as confocal microscopy (4), total internal reflection microscopy (TIRF) (5, 70, 76), and super-resolution techniques (3, 77). Other techniques like lattice light-sheet microscopy and synaptic contact mapping allow a more direct assessment of the membrane structure of activating T cells (2).
Distribution of Signaling Molecules on Microvillar Protrusions
As extensively reviewed by Orbach and Su (78), microvilli are well-equipped for antigen recognition, and recent studies show that microvillar protrusions are indeed the antigen-sensing entities driving immune surveillance (79–81). In short, TCRs and CD4 coreceptors, CD2 adhesion molecules and essential proteins for T-cell activation, such as Lck and LAT, have been found enriched in microvilli (80). Jung et al. investigated the impact of membrane ultrastructure on TCR distribution on T cells and observed TCR pre-clustering on resting T cells (3). Interestingly, no TCR clusters were observed when T cells were allowed to flatten out on non-activating surfaces (82). Recently, the Ley group observed CD45 exclusion from microvilli tips before antigen recognition (83), whereas Razvag et al. observed an exclusion of CD45 shortly after contact formation (77). Considering the kinetic segregation model, which postulates that T-cell activation is induced by the spatial separation of phosphatases such as CD45 from the phosphorylation sites of TCRs, this implies a high sensitivity of tip-resident TCRs toward antigenic pMHC. Indeed, contacts between microvilli and stimulating surfaces were found to be sufficient for T-cell activation (76, 84). What drives the organization of signaling molecules in microvilli has so far remained elusive, but it is speculated that the extreme membrane curvature and the lipid composition, in particular cholesterol content, may play a role (78). Furthermore, it is generally accepted that microvillar protrusions contain parallel bundles of actin filaments (2, 65) and may colocalize often, but not necessarily always, with TCR molecules [(2) and reviewed in (78)].
In summary, it is evident that microvilli form a restricted reaction volume harboring the necessary molecules for T-cell activation. An exciting observation was recently made by Klotzsch and colleagues, who demonstrated the ability of the T cell to reach into narrowly confined spaces and who showcased the very dynamic nature of their microvillar protrusions and their ability to scan for occluded antigens (85). Interestingly, when T cells reached into micropits below 200 nm in diameter, a slight yet quantifiable antigen-independent cytokine upregulation started. This observation indicates that the signaling molecules within the limited reaction volume of microvilli may be sufficient for cell-body independent signal amplification or, alternatively, that T cells may reach an even higher degree of antigen sensitivity when the dimensions of their microvilli are severely restricted. Effective T-cell activation may hence be aided by enforcing the spatial proximity of signaling molecules downstream of the TCR.
Microvilli Scanning Speed on Artificial and Natural APCs
The fractal arrangement of microvilli enables T cells to efficiently scan the surfaces of antigen-presenting cells (2). Scanning human CD4+ T cells move with a mean velocity of ~3 µm/min over cell surfaces (4). T cells perform immune surveillance in lymph nodes in the presence of antigen with a scanning speed of 2.6 to 5.4 µm/min in a random walk fashion (86, 87), and a dendritic cell typically interacts for ~3 min with individual T cells (88). In resting murine OT-1 TCR-transgenic T cells, microvilli were found to move at an average speed of 5.2 ± 0.4 μm/min, resulting in a 98% coverage of the T cell surface within 1 min. Similarly, the leading edge of the lamellipodium of migrating fibroblasts moves at a velocity of 6.3 µm/min (89). Note that comparable migration velocities have been observed for TCR microclusters in Jurkat T cells (8.4 ± 0.36 μm/min) (90). The mean velocity of actin retrograde flow in Jurkat T cells stimulated with a strong agonist has been recorded to be ~4.8–5.4 µm/min (91, 92), with faster velocities observed for weaker agonists (92).
Motion of any biological membrane in a perpendicular direction to the plane of the receptor–ligand interaction occurs at high frequencies and in the range of tens of nanometers due to thermal fluctuations or stochastic membrane displacement (reviewed in (34)). Perpendicular fluctuations of microvilli tips of about 67 nm were observed within 1 second-long observation windows (70). The lateral movement of microvilli within this experimental setup across a non-activating surface was however minimal, and only rarely spurts of tenths of a micrometer could be observed (70). These results indicate that microvilli move perpendicularly toward a cell surface and retract without much lateral movement to reappear at a different position to continue probing the APC surface. This observation of dynamic microvilli behavior is intriguing, considering that T cells must overcome the glycocalyx in order to probe for surface receptors. Lateral movement through the dense surface layer may be energetically disadvantageous. Also, the geometry of the antigen-presenting surface is likely to influence scanning membrane protrusions (4, 93).
In 2012, Sage et al. performed a series of experiments aimed at determining the depth and width of “invadosome-like” podosomes (ILPs). Although the depth of these structures was found to be stimulus-independent, the width showed a significant decrease in the presence of a specific antigen (4). The authors also showed that calcium flux is initiated ~25 s after the first appearance of an ILP. Interestingly, the presence of antigen caused a substantial stabilization of the ILP lifetime (4), i.e., the transient nature of the scanning ILP ceased to exist after contact with cognate antigen. Cai et al. observed rapid scanning of the opposing surface by microvillar structures and their subsequent arrest or stabilization upon encountering cognate antigen (2). This process seemed, however, to be independent of downstream signaling. A theoretical model has been proposed, attributing the microvilli contact stabilization to the formation of catch bonds (non-covalent bonds whose lifetime increases under force) between TCR and MHC loaded with stimulatory peptide (21), implying a critical role of mechanical forces exerted via microvilli in antigen discrimination. The suggested mechanism is in agreement with the finding that microvilli stabilization is independent of actin (2). Further theoretical work indicated that the antigen-dependent arrest of microvilli may indeed be essential for ligand discrimination (94): Within their framework, which was based on kinetic segregation, Fernandes and coworkers found specific TCR triggering if (i) close contact areas between T cells and APCs persisted for at least two seconds and (ii) the radius of the area was smaller than 220 nm.
Considering the mobility of pMHCs on APCs as an additional parameter for T-cell recognition, the field has not yet reached a consensus on to what extent the laterally immobile or the mobile fraction of pMHCs lead to efficient triggering in vivo (95–97). Several studies have identified the velocity and quantified the mobile fraction within the membranes of APC (98–100), but to our knowledge, none has shown the functional connection to T cell activation of either fraction. From our own experiments using activating adhesion-competent gel-phase and fluid-phase glass-supported lipid bilayers, we do know that T cells scan and activate efficiently on both surfaces (101), but we could observe a slight delay in response on laterally immobile surfaces, most likely due to a stalled microcluster formation. These results were corroborated by other studies (102, 103). A faster moving pMHC molecule would increase the likelihood of encountering the microvilli tips, which becomes important when contemplating scenarios with very low densities of cognate antigens (21). On the other hand, slower moving or immobilized pMHCs may facilitate rebinding events if microvilli tips stay in close proximity (21), which could influence antigen recognition thresholds (104).
Force Profile of Membrane Protrusions
As motile entities, T cells experience considerable strain. T-cell protrusions share many properties with filopodia, which are actively used by other motile cells when they screen the surroundings for biochemical and mechanical cues through environment-sensing receptors residing on their tips (105). Filopodia generation requires 5–30 pN (piconewton) of protrusion force (106). Once formed and anchored to cortical actin, they can exert extensive pulling forces on their own (79, 107). Podosome protrusion force was quantified using monocyte-derived cells spreading on a deformable artificial membrane: an average of 29 to 155 nN pushing force was measured, which was positively correlated with substrate rigidity (108). Interestingly, podosomes could adjust their core elasticity toward the substrate rigidity, maintaining a constant indentation depth. Similar results were obtained for podosomes of fibroblasts pushing against SLBs (109). The latter study quantified the molecular tension exerted by individual integrin molecules using DNA-based tension-gauge tethers, hence confirming the tendency of podosomes to predominantly exert perpendicular forces.
Via 3D traction force microscopy employing beads bound to an elastic hydrogel, forces of up to several hundred piconewtons exerted by single microvilli were observed (79). Force application via membrane protrusions was found to be crucial for the cytotoxic activity of CD8+ T cells (110): Knocking out the cytoskeletal regulator WASP not only led to diminished forces and deformation of target cells, but also to a 50% reduction in killing efficiency at low antigen levels.
Mechanical Forces in T-cell Antigen Recognition
For decades, scientists have attempted to tackle the mystery surrounding the high sensitivity and specificity of T cells for their cognate antigen and have naturally created a multitude of T-cell activation models, each with their own merits and weaknesses. These early models mainly investigated biochemical aspects of the TCR–pMHC interaction, such as the multimeric state of the ligands, the required ligand density for activation, or the influence of co-receptor binding on triggering potency. However, it quickly became apparent that all these models could not sufficiently explain the high sensitivity and specificity of the TCR–pMHC interaction. Eventually, a new parameter entered the field. In 2001, the Dustin group observed that different experimental methods led to marked differences in the measured kinetic parameters of the TCR–pMHC interaction. Consequently, it was hypothesized that the koff may increase due to mechanical force in a 2D setting, where settling T cells interact with immobilized ligands on a surface (111). The intuitive explanation for this phenomenon was the involvement of dynamic cellular processes in destabilizing the TCR–pMHC interaction. Indeed, ten years later, Huppa et al. observed a significant difference in 2D and 3D binding kinetics and, additionally, a pronounced increase in TCR–pMHC interaction lifetime upon the destabilization of the cortical cytoskeleton, indicating that mechanical forces impact T-cell antigen recognition and triggering (112).
The advancement of new techniques followed, with the purpose of identifying the impact of mechanical forces on/during T-cell activation (see Figure 2 for an overview of the most prominent methods). For this review, we will discriminate between the effects of externally applied mechanical forces and forces exerted through the TCR itself.
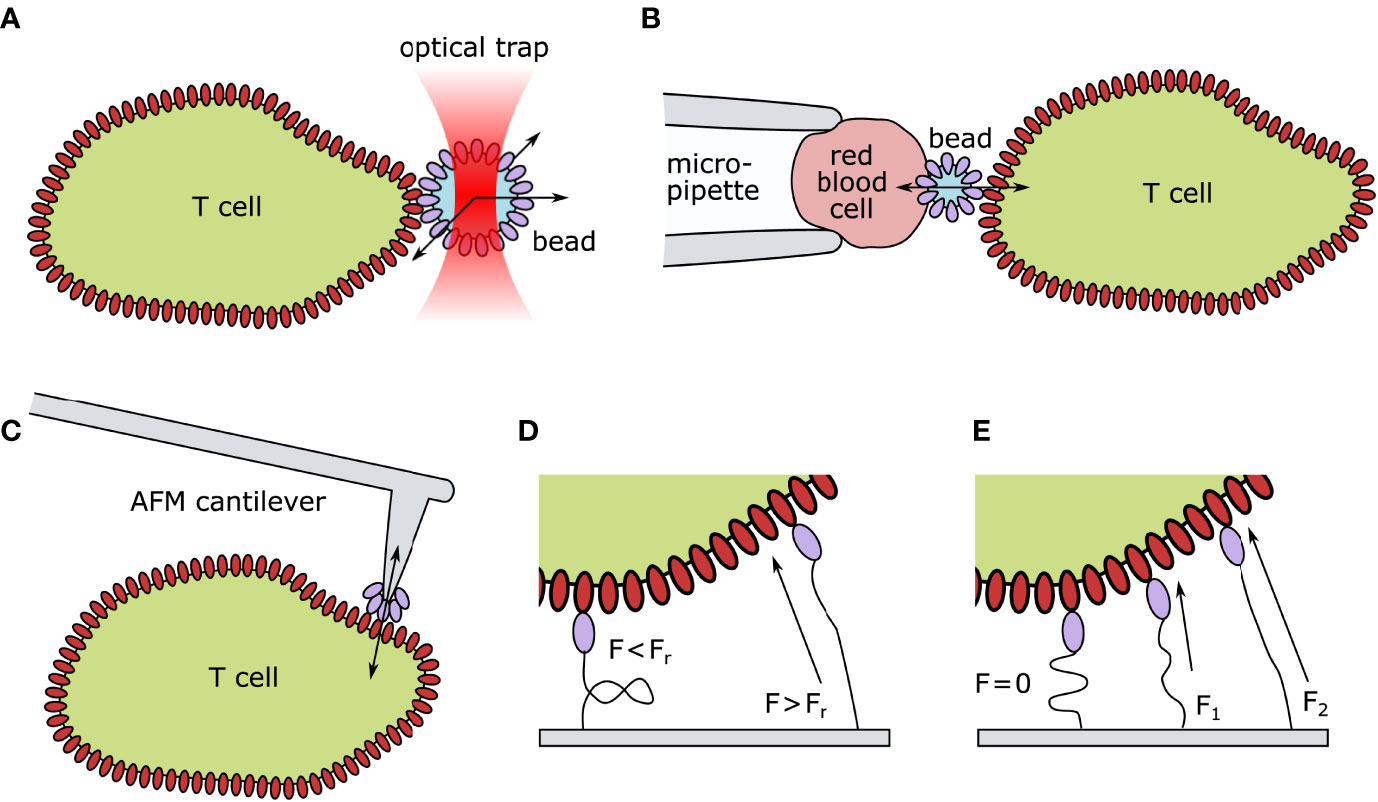
Figure 2 Technical approaches for quantifying mechanical forces exerted on TCR–pMHC pairs. (A) Optical tweezer setup: ligand-coated beads are spatially fixed by an optical trap. Upon TCR engagement, the bead is moved out of the laser focus. The deflection indicates the TCR-imposed mechanical force. (B) Biomembrane Force Probes: A T cell and a red blood cell are aspirated and held in place via a micropipette setup. A ligand-coated bead is attached to the surface of the red blood cells. Upon T-cell contact, altered thermal fluctuation of the bead indicates TCR–ligand engagement. Forces can be exerted by retracting the micropipette. (C) Atomic Force Microscopy: A ligand-coated cantilever tip is brought into close proximity of the T cell surface. Upon TCR engagement, the deflection of the cantilever indicates force generation. (D) Digital Molecular Force Sensors (MFS): A ligand is attached to a fluorescently labeled MFS unit. In their folded state, the fluorescence is entirely quenched. Such sensors can withstand a certain threshold of strain before (Fr) unfolding. Upon TCR engagement and force generation, the digital MFS unfolds, reducing the quencher efficiency and leading to a quantifiable increase in fluorescence. (E) Analog MFS: A ligand is attached to a fluorescently labeled spring unit framed with a FRET (Förster resonance energy transfer) pair. In its coiled state (F=0) the fluorophores are in close proximity and the FRET efficiency is high. Upon TCR engagement and force generation (F1<F2) the spring unit uncoils continuously decreasing the FRET efficiency between the two fluorophores. AFM, Atomic Force Microscopy; F, Force; Fr, hairpin rupture force.
Impact of Externally Applied Mechanical Forces on T-Cell Activation
In a multitude of studies, mechanical forces were applied to the TCR–pMHC bond to characterize the TCR as a mechanosensor. Different approaches were devised to stretch the bond in a defined manner (see overview in Table 1):
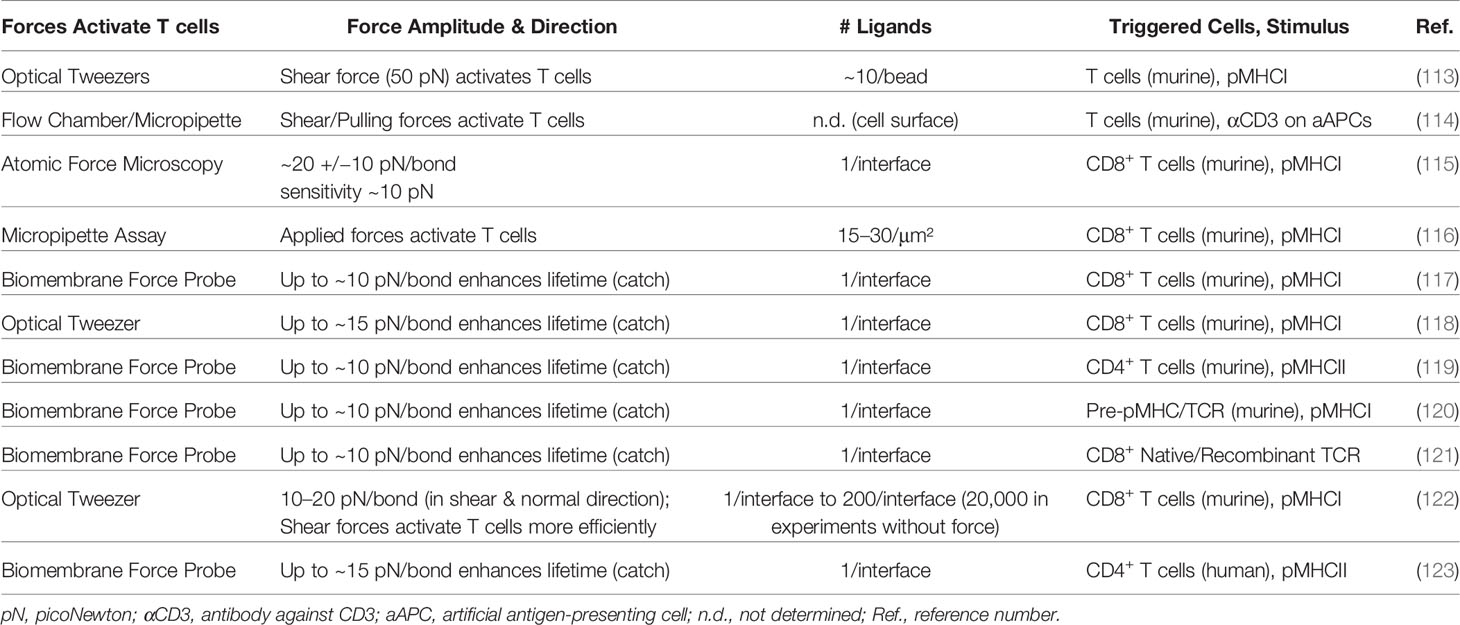
Table 1 Overview of published articles investigating the impact of mechanical forces on T-cell activation.
One of the first experimental strategies was to confront T cells with a bead coated with a defined number of TCR–ligands, followed by targeted force application and simultaneous recording of TCR-downstream signaling. A force of as little as 50 pN applied to the pMHC-coated bead in a tangential but not normal orientation with regard to the T cell surface turned out to be sufficient to activate T cells (113). A subsequent study determined the effect of applied shear and pulling forces on the TCR–pMHC bond using artificial APCs and demonstrated that mechanical forces can activate T cells as well in a cell–cell conjugate (114). Forces of as little as 10 pN were found sufficient to induce signaling when imposed through pMHCs or TCR/CD3-specific antibodies (122). Combined, these studies are consistent with the notion that triggering thresholds are not only defined by the intrinsic biochemical properties of TCRs and pMHC but also by force load and directionality. Interestingly, the latter study revealed that forces applied to no more than 1 molecule at the bead–cell interface could not trigger a robust T-cell response, whereas forces applied over 2 bonds resulted in T-cell activation. Given that the physiological concentration of presented antigen via pMHCI is estimated to be around 10–100 per interface (124), and as few as 3–10 bonds are sufficient to trigger cytotoxicity (125), these insights let mechanical forces in the context of scanning microvilli tips shine in a new light.
Adding to the multitude of unique insights, the study by Feng et al. provided the first clear evidence that forces applied over multiple bonds at the bead–cell interface are being distributed (122). A prerequisite to load distribution would be the physical coupling of the involved surface area. Along this line of reasoning, the biophysical parameters of membrane curvature and tension recently emerged as a possible mechanism influencing T-cell motility, protrusion, and immune synapse formation (reviewed in (126)). The decisive physical parameter in this model is the modulation of membrane tension and tension decay at the triggering point, which could even be used to explain certain aspects of ligand discrimination (127) and synapse breaking (128).
By measuring TCR–pMHC dissociation kinetics under load with the use of a biomembrane force probe (BFP), Zhu and colleagues found a correlation between the bond lifetime and stimulatory potency (116, 117, 119, 129). BFP probes were used to apply mechanical forces to CD8+ (117) and CD4+ T cells (119, 123), and showed that for agonistic antigens, forces up to 10 pN prolong the interaction lifetime, forming ‘catch bonds,’ while non-stimulatory pMHCs give rise to much reduced lifetimes under load (‘slip bonds’). By using an optical tweezer setup (118), catch-bond characteristics of stimulatory TCR–pMHC interactions were also observed by others. Furthermore, the directionality of externally applied forces probing the TCR–pMHC interaction impacts the antigen sensitivity of the T cell (122). Therefore, one prevalent model in the field describing antigen discrimination concerns the mechanical probing of each TCR–pMHC interaction. The TCR-imposed molecular forces exerted by the structural dynamics of the cellular membrane help in probing the ligand–receptor interaction, and only strong agonists allow the necessary resistance (life-time) to trigger activation (117, 122). It seems, therefore, plausible that linking forces to synaptic lifetime and the stimulatory potency of a given TCR–pMHC pair manifests as a major principle underlying antigen discrimination in the physiological context of T-cell antigen recognition. However, this has been contested by the recent observation that even agonist pMHCs coated on beads and interacting with a TCR-coated surface exhibited a clear slip bond behaviour behavior under defined flow-generated force in an in vitro experiment without cells (130).
Force Amplitude of TCR-Imposed Mechanical Forces
Adhesion-related forces of about 1–2 nN have been reported within cell–cell contacts. However, measured values varied significantly depending on the cell line under investigation (131, 132). Moreover, net adhesion forces recorded between the conjugated cells correlated with the stimulatory potency of the pMHC (132). Several attempts have since been made to assess forces imposed on a truly molecular level (see Table 2).
Husson et al. (71) adapted the BFP technology with single-molecule detection to visualize the temporal response of single T cells to glass beads functionalized with anti-CD3 and covalently attached to the surface of a red blood cell. Force generation was monitored by the elongation of the red blood cell. In this fashion, distinct pulling and pushing phases could be observed in receptor-engaged T cells.
Traction force microscopy (TFM) has been applied to assess forces related to T-cell adhesion and activation. This methodology involves tracking the force-induced displacement of fluorescent beads embedded in a matrix of defined stiffness resulting from cells crawling or spreading thereon. From such studies, it was concluded that T cells adapt their signaling response according to substrate stiffness. TFM provided the net amplitude and directionality of averaged cellular mechanical forces. With the use of elastic pillars coated with pMHC or activating antibodies, Bashour et al. visualized and quantified TCR-imposed forces (133): T cells deflected pillars with approximately 200 pN per pillar in an antigen-dependent manner. However, the exact number of participating molecular bonds could not be determined. A similar TFM experiment conducted with Jurkat T cells revealed that activating surface conditions (anti-CD3 antibodies) produce higher deforming forces than non-activating conditions (56).
Atomic force microscopy (AFM) using pMHC-coated cantilevers confronting the T cell surface showed distinct pushing and pulling phases without further application of external force (19). The net pulling forces of activating T cells were stronger for high affinity antibodies than for pMHC and absent for control antibodies. Similarly, T cells immobilized on AFM cantilevers exhibited both pushing and pulling forces when contacting activating supported lipid bilayers (76).
Intra- and extracellular molecular force sensors (MFSs) are recent technological additions to the field of nano-mechanosensors and can either have an analog (for peptide/PEG-based sensors) or digital readout (for DNA-based sensors). By defining the mechanical force necessary to unzip a DNA hairpin spanned between a fluorescent dye and a quenching gold particle, Salaita and colleagues successfully measured the range of forces exerted by a defined number of TCR. CD8+ T cells were shown to unzip pMHC-carrying hairpins of 12 pN but not of 19 pN resistance, and CD4+ T cells unzipped hairpins of 4.7 pN resistance (134, 135). In this follow-up study by the same group, force probe-decorated gold particles were anchored to a fluid lipid bilayer (135). Although this system can be considered mobile, the force probe itself was still immobilized on a rigid surface, resulting in a high counter force. Furthermore, the same technique was applied to gain insights into the mechanical sampling of antigenic peptides of varying potency. The authors reported a correlation between tension, potency, and successful TCR triggering (136).
The shortcomings of digital MFSs can be mitigated by using peptide-based analog MFSs. These contain a flexible peptide whose extension is a continuous function of the applied force. Like a macroscopic spring, the higher the pulling force is exerted, the larger the end-to-end distance of the peptide becomes, allowing for direct measurement of the force. This approach has been showcased by the quantification of forces exerted by single integrins by Morimatsu et al. in 2013 (137). Reasonable estimates of single integrin forces were between 2 and 40 pN (AFM rupture forces), and maximal transmitted forces were measured by a digital DNA-based force sensor to be 20–30 pN. However, peptide-based analog force sensors reported only 1–5 pN (extrapolated from bulk measurements) (137) and 1–3 pN (determined by single-molecule FRET measurements) (138) for individual integrins. There is a considerable difference between the results of these studies depending on the acquisition method. Given the more direct method of data acquisition with single-molecule resolution, the latter studies likely approach the ground truth.
Using an analog peptide-based MFS, we recently quantified the mechanical forces exerted by single TCRs and found a striking difference between activating and scanning conditions (101). We developed a quantitative FRET-based force sensor for application within the immunological synapse, which operates at the single-molecule level (101). As a spring element, we employed a peptide derived from the flagelliform spider silk protein with known elastic properties (139, 140). The biotinylated spring peptide was conjugated to either (i) a stimulating single-chain antibody fragment derived from the TCRβ-reactive H57 monoclonal antibody or (ii) the natural ligand, an MCC-presenting MHC protein. The sensor fits well within the immunological synapse, as it only spans 8 nm in its collapsed configuration (2, 141). We observed 5 to 8 pN force-peaks per TCR for T cells engaging gel-phase and ~2 pN for fluid-phase glass-supported lipid bilayers using the H57-derived MFS, and T cells stimulated with the natural ligand exerted an average force of ~2 pN on gel-phase surfaces. The functional consequences of mechanical forces along the bond axis remain to be investigated, but this study suggests that perpendicular endogenous forces seem negligible. Additionally, temporal single-molecule force profiles revealed a strong molecular force peak of 7.5 pN in early contact situations while T cells scan for antigen and a late arising force peak of 5.6 pN after T-cell activation (101). These results clearly show that force profiles experienced by individual TCR–ligand pairs differ during antigen surveillance and synapse formation. The analog MFS further enabled us to record the time course of force application. We observed linearly increasing forces at a rate of 1.5 pN/s for 2–3 s, followed by a sudden drop to zero, which we speculate is due to TCRs losing their frictional coupling to the actin cytoskeleton.
Force Orientation During TCR Triggering
The impact of the orientation of the force vector on mechanotransduction is another hotly debated topic in the immune surveillance and T-cell triggering fields. The aforementioned experiments using optical tweezers to pull a bead tangentially or perpendicularly with respect to the T cell surface (113) yielded the first insights into a differential response toward the directionality of externally applied forces. Here, non-agonistic antibodies binding to the CD3ϵγ subunit of the TCR could be used for triggering if pulled tangentially, but not normally with respect to the cell surface. A recent study by the Salaita group yielded the latest insights into the force vector directionality for triggering of TCRs by applying a newly developed technique named SIM-MFM, in which polarization-modulated structured illumination is combined with DNA-based membrane force sensor technology (142). Using an activating antibody against CD3ϵ, they found no preferred direction of TCR-imposed forces. Although these results do not answer the question of force orientation during immune surveillance, the reported method provides a first step toward resolving the force direction required for TCR triggering.
Impact of Mechanical Forces After Immunological Synapse Formation
Investigating mechanical forces during T-cell activation lays bare numerous differences in the mechanisms employed by various T cell lineages and subtypes. Adhesion cascades, cytoskeletal rearrangement, and, consequently, synapse shape and dynamics are very much dependent on the encountered cellular target. LFA-1 conformation and function clearly depend on the retrograde flow of the actin cytoskeleton and impacts T-cell activation via co-stimulation (143, 144). Naïve T cells scanning DCs within the lymphoid tissue experience a rapid LFA-1 maturation cascade in view of the ligand rigidification on the DC membrane (53, 98). The latter study showed that for a DC subset, MHC mobility remained unchanged upon DC cell differentiation (98). Whether MHC molecules also experience mobility changes during or as a result of certain signaling events or synapse formation remains to be addressed (143). Another prominent example of how mechanical forces act during T-cell activation concerns target cell killing by CD8+ T cells. As shown by Huse and colleagues, antigen-experienced cytolytic T cells massively strain and deform the target cell membrane surface and promote in this fashion perforin function (145).
Concluding Remarks
T cells feature specialized membrane protrusions in varying environmental contexts. In the last few years, membrane protrusions have received much attention for their active role during immune surveillance. Microvilli provide the platform for exerting forces based on their cytoskeletal core and their dynamic nature. So far, the community has gained the following insights into the topic:
(i) Microvilli are important for fast and efficient antigen scanning and sampling.
(ii) Microvilli carry all molecules necessary for adhesion and initiating TCR-proximal signaling.
(iii) Microvilli form limited reaction volumes, which may sensitize T cells for antigen.
(iv) Microvilli may not only be involved in the biochemical probing of the environment but may also be necessary for testing the biomechanical properties of the target cells and tissues.
(v) Glycocalyx and cellular stiffness parameters affect TCR triggering.
Considering the dynamic cellular processes surrounding mechanosurveillance, a precise temporal control of the triggering event is a sine qua non for further insights in the field, and based on the aforementioned studies, a number of conclusions can already be drawn:
(i) Tensile forces do affect early T cell activation.
(ii) Force transduction is TCR- and peptide-dependent.
(iii) Shear forces or torque affect T cell activation more strongly than normal forces.
(iv) Generated tensile forces are invariably tied to physiological T cell recognition and precede T cell activation.
(v) Individual molecules at protrusion tips are subject to pulling and pushing forces in the pN range.
(vi) Tensile forces are generated internally by rearrangements of the actin cytoskeleton and do not involve the action of acto-myosin motor proteins.
(vii) Immune synapse formation generates mechanical forces that activate ligand-bound integrins.
(viii) T cell-imposed forces deform target cells and promote their killing.
Open Questions
(i) Are there any lateral movements of antigen-bound microvilli tips? What resistance does the glycocalyx pose to the lateral movement of microvilli? Can the microvillar scanning process be imagined more like a “dragging through the waves” or more like a repeated “poking through the barrier”?
(ii) There is conflicting evidence for increased microvilli life- or dwell times upon antigen encounter. Does antigen binding lead to an arrest of microvilli dynamics? Are such arrests only happening for triggering interactions or also for probing events?
(iii) Is the glycocalyx posing a resistance to microvilli penetration?
(iv) At what point is the force vector reversed from pushing to pulling? How are adhesive interactions influencing this process? Is this process used for ligand discrimination by mechanical probing?
(v) What is the fate of microvillar protrusions after TCR triggering? Are the protein platforms at the microvillar tips evolving to microclusters? What is the impact of LFA-1 maturation on signaling within microvillar protrusions?
(vi) Is the mobility of the pMHCs decisive for the interaction probability and/or rebinding in the context of microvillar scanning? Are target cells mechanically altering their presenting surfaces to allow or inhibit efficient scanning of the surface?
(vii) How are microvilli recognition events coupled to the movement of the entire cell body? How are these signals communicated within the T cell?
Author Contributions
JG conceived and drafted the manuscript. JG and LS conducted literature review and wrote the manuscript. LS designed the figures. JG, LS, GS, and JH revised the manuscript. All authors listed have made a substantial, direct, and intellectual contribution to the work and approved it for publication.
Funding
This work was supported by the Austrian Science Fund (FWF) project P32307-B (JG, LS, and GS), and I5056-B (LS and GS), and by the Vienna Science and Technology Fund (WWTF) project LS13-030 (JG, LS, JH, and GS).
Conflict of Interest
The authors declare that the research was conducted in the absence of any commercial or financial relationships that could be construed as a potential conflict of interest.
Publisher’s Note
All claims expressed in this article are solely those of the authors and do not necessarily represent those of their affiliated organizations, or those of the publisher, the editors and the reviewers. Any product that may be evaluated in this article, or claim that may be made by its manufacturer, is not guaranteed or endorsed by the publisher.
References
1. Malissen B, Bongrand P. Early T Cell Activation: Integrating Biochemical, Structural, and Biophysical Cues. Annu Rev Immunol (2015) 33(1):539–61. doi: 10.1146/annurev-immunol-032414-112158
2. Cai E, Marchuk K, Beemiller P, Beppler C, Rubashkin MG, Weaver VM, et al. Visualizing Dynamic Microvillar Search and Stabilization During Ligand Detection by T Cells. Science (2017) 356(6338):eaal3118. doi: 10.1126/science.aal3118
3. Jung Y, Riven I, Feigelson SW, Kartvelishvily E, Tohya K, Miyasaka M, et al. Three-Dimensional Localization of T-cell Receptors in Relation to Microvilli Using a Combination of Superresolution Microscopies. Proc Natl Acad Sci USA (2016) 113(40):E5916–24. doi: 10.1073/pnas.1605399113
4. Sage PT, Varghese LM, Martinelli R, Sciuto TE, Kamei M, Dvorak AM, et al. Antigen Recognition is Facilitated by Invadosome-like Protrusions Formed by Memory/Effector T Cells. J Immunol (2012) 188(8):3686–99. doi: 10.4049/jimmunol.1102594
5. Brodovitch A, Bongrand P, Pierres A. T Lymphocytes Sense Antigens Within Seconds and Make a Decision Within One Minute. J Immunol (2013) 191(5):2064–71. doi: 10.4049/jimmunol.1300523
6. Ji L, Lim J, Danuser G. Fluctuations of Intracellular Forces During Cell Protrusion. Nat Cell Biol (2008) 10(12):1393–400. doi: 10.1038/ncb1797
7. Pettmann J, Santos AM, Dushek O, Davis SJ. Membrane Ultrastructure and T Cell Activation. Front Immunol (2018) 9:2152. doi: 10.3389/fimmu.2018.02152
8. Stinchcombe JC, Bossi G, Booth S, Griffiths GM. The Immunological Synapse of CTL Contains a Secretory Domain and Membrane Bridges. Immunity (2001) 15(5):751–61. doi: 10.1016/S1074-7613(01)00234-5
9. Carman CV, Sage PT, Sciuto TE, de la Fuente MA, Geha RS, Ochs HD, et al. Transcellular Diapedesis Is Initiated by Invasive Podosomes. Immunity (2007) 26(6):784–97. doi: 10.1016/j.immuni.2007.04.015
10. Dustin ML, Chakraborty AK, Shaw AS. Understanding the Structure and Function of the Immunological Synapse. Cold Spring Harb Perspect Biol (2010) 2(10):a002311. doi: 10.1101/cshperspect.a002311
11. Ma Z, Janmey PA, Finkel TH. The Receptor Deformation Model of TCR Triggering. FASEB J (2008) 22(4):1002–8. doi: 10.1096/fj.07-9331hyp
12. Davis SJ, Merwe PA. The Kinetic-Segregation Model: TCR Triggering and Beyond. Nat Immunol (2006) 7(8):803–9. doi: 10.1038/ni1369
13. Bunnell SC, Hong DI, Kardon JR, Yamazaki T, McGlade CJ, Barr VA, et al. T Cell Receptor Ligation Induces the Formation of Dynamically Regulated Signaling Assemblies. J Cell Biol (2002) 158(7):1263–75. doi: 10.1083/jcb.200203043
14. Campi G, Varma R, Dustin ML. Actin and Agonist MHC-peptide Complex-Dependent T Cell Receptor Microclusters as Scaffolds for Signaling. J Exp Med (2005) 202(8):1031–6. doi: 10.1084/jem.20051182
15. Yi J, Wu XS, Crites T, Hammer JA. Actin Retrograde Flow and Actomyosin II Arc Contraction Drive Receptor Cluster Dynamics at the Immunological Synapse in Jurkat T Cells. Mol Biol Cell (2012) 23(5):834–52. doi: 10.1091/mbc.e11-08-0731
16. Lee K-H, Holdorf AD, Dustin ML, Chan AC, Allen PM, Shaw AS. T Cell Receptor Signaling Precedes Immunological Synapse Formation. Science (2002) 295(5559):1539–42. doi: 10.1126/science.1067710
17. Yokosuka T, Sakata-Sogawa K, Kobayashi W, Hiroshima M, Hashimoto-Tane A, Tokunaga M, et al. Newly Generated T Cell Receptor Microclusters Initiate and Sustain T Cell Activation by Recruitment of Zap70 and SLP-76. Nat Immunol (2005) 6(12):1253–62. doi: 10.1038/ni1272
18. Hivroz C, Saitakis M. Biophysical Aspects of T Lymphocyte Activation at the Immune Synapse. Front Immunol (2016) 7:46. doi: 10.3389/fimmu.2016.00046
19. Hu KH, Butte MJ. T Cell Activation Requires Force Generation. J Cell Biol (2016) 213(5):535–42. doi: 10.1083/jcb.201511053
20. Pielak RM, O’Donoghue GP, Lin JJ, Alfieri KN, Fay NC, Low-Nam ST, et al. Early T Cell Receptor Signals Globally Modulate Ligand:Receptor Affinities During Antigen Discrimination. Proc Natl Acad Sci USA (2017) 114(46):12190–5. doi: 10.1073/pnas.1613140114
21. Pullen RH, Abel SM. Mechanical Feedback Enables Catch Bonds to Selectively Stabilize Scanning Microvilli at T-Cell Surfaces. Mol Biol Cell (2019) 30(16):2087–95. doi: 10.1091/mbc.E19-01-0048
22. Huppa JB, Gleimer M, Sumen C, Davis MM. Continuous T Cell Receptor Signaling Required for Synapse Maintenance and Full Effector Potential. Nat Immunol (2003) 4(8):749–55. doi: 10.1038/ni951
23. Kuhns MS, Davis MM. Tcr Signaling Emerges From the Sum of Many Parts. Front Immunol (2012) 3:159. doi: 10.3389/fimmu.2012.00159
24. Moreau JM, Gouirand V, Rosenblum MD. T-Cell Adhesion in Healthy and Inflamed Skin. JID Innov (2021) 1(2):100014. doi: 10.1016/j.xjidi.2021.100014
25. Hor JL, Whitney PG, Zaid A, Brooks AG, Heath WR, Mueller SN. Spatiotemporally Distinct Interactions With Dendritic Cell Subsets Facilitates CD4+ and CD8+ T Cell Activation to Localized Viral Infection. Immunity (2015) 43(3):554–65. doi: 10.1016/j.immuni.2015.07.020
26. Springer TA. Adhesion Receptors of the Immune System. Nature (1990) 346(6283):425–34. doi: 10.1038/346425a0
27. Bell GI, Dembo M, Bongrand P. Cell Adhesion. Competition Between Nonspecific Repulsion and Specific Bonding. Biophys J (1984) 45(6):1051–64. doi: 10.1016/S0006-3495(84)84252-6
28. Ardman B, Sikorski MA, Staunton DE. CD43 Interferes With T-lymphocyte Adhesion. Proc Natl Acad Sci USA. (1992) 89(11):5001–5. doi: 10.1073/pnas.89.11.5001
29. Zhao Y, Chien S, Weinbaum S. Dynamic Contact Forces on Leukocyte Microvilli and Their Penetration of the Endothelial Glycocalyx. Biophys J (2001) 80(3):1124–40. doi: 10.1016/S0006-3495(01)76090-0
30. Tarbell JM, Pahakis MY. Mechanotransduction and the Glycocalyx. J Internal Med (2006) 259(4):339–50. doi: 10.1111/j.1365-2796.2006.01620.x
31. Bi S, Baum LG. Sialic Acids in T Cell Development and Function. Biochim Biophys Acta (BBA) - Gen Subjects (2009) 1790(12):1599–610. doi: 10.1016/j.bbagen.2009.07.027
32. Weinbaum S, Tarbell JM, Damiano ER. The Structure and Function of the Endothelial Glycocalyx Layer. Annu Rev BioMed Eng (2007) 9:121–67. doi: 10.1146/annurev.bioeng.9.060906.151959
33. Reitsma S, Slaaf DW, Vink H, van Zandvoort MAMJ, oude Egbrink MGA. The Endothelial Glycocalyx: Composition, Functions, and Visualization. Pflugers Arch (2007) 454(3):345–59. doi: 10.1007/s00424-007-0212-8
34. Al-Aghbar MA, Jainarayanan AK, Dustin ML, Roffler SR. The Interplay Between Membrane Topology and Mechanical Forces in Regulating T Cell Receptor Activity. Commun Biol (2022) 5(1):1–16. doi: 10.1038/s42003-021-02995-1
35. Chang VT, Fernandes RA, Ganzinger KA, Lee SF, Siebold C, McColl J, et al. Initiation of T Cell Signaling by CD45 Segregation at ‘Close Contacts’. Nat Immunol (2016) 17(5):574–82. doi: 10.1038/ni.3392
36. Agrawal B, Krantz MJ, Parker J, Longenecker BM. Expression of MUC1 Mucin on Activated Human T Cells: Implications for a Role of MUC1 in Normal Immune Regulation. Cancer Res (1998) 58(18):4079–81.
37. Mummert ME, Mummert D, Edelbaum D, Hui F, Matsue H, Takashima A. Synthesis and Surface Expression of Hyaluronan by Dendritic Cells and Its Potential Role in Antigen Presentation. J Immunol (2002) 169(8):4322–31. doi: 10.4049/jimmunol.169.8.4322
38. Bufi N, Saitakis M, Dogniaux S, Buschinger O, Bohineust A, Richert A, et al. Human Primary Immune Cells Exhibit Distinct Mechanical Properties That Are Modified by Inflammation. Biophys J (2015) 108(9):2181–90. doi: 10.1016/j.bpj.2015.03.047
39. Zak A, Merino-Cortés SV, Sadoun A, Mustapha F, Babataheri A, Dogniaux S, et al. Rapid Viscoelastic Changes Are a Hallmark of Early Leukocyte Activation. Biophys J (2021) 120(9):1692–704. doi: 10.1016/j.bpj.2021.02.042
40. Cloosen S, Thio M, Vanclée A, van Leeuwen EBM, Senden-Gijsbers BLMG, Oving EBH, et al. Mucin-1 Is Expressed on Dendritic Cells, Both In Vitro and In Vivo. Int Immunol (2004) 16(11):1561–71. doi: 10.1093/intimm/dxh157
41. Johnson LA, Banerji S, Lagerholm BC, Jackson DG. Dendritic Cell Entry to Lymphatic Capillaries Is Orchestrated by CD44 and the Hyaluronan Glycocalyx. Life Sci Alliance (2021) 4(5):e202000908. doi: 10.26508/lsa.202000908
42. Teijeira A, Hunter MC, Russo E, Proulx ST, Frei T, Debes GF, et al. T Cell Migration From Inflamed Skin to Draining Lymph Nodes Requires Intralymphatic Crawling Supported by ICAM-1/LFA-1 Interactions. Cell Rep (2017) 18(4):857–65. doi: 10.1016/j.celrep.2016.12.078
43. Hunter MC, Teijeira A, Montecchi R, Russo E, Runge P, Kiefer F, et al. Dendritic Cells and T Cells Interact Within Murine Afferent Lymphatic Capillaries. Front Immunol (2019) 10:520. doi: 10.3389/fimmu.2019.00520
44. Arasa J, Collado-Diaz V, Halin C. Structure and Immune Function of Afferent Lymphatics and Their Mechanistic Contribution to Dendritic Cell and T Cell Trafficking. Cells (2021) 10(5):1269. doi: 10.3390/cells10051269
45. Imbert PRC, Saric A, Pedram K, Bertozzi CR, Grinstein S, Freeman SA. An Acquired and Endogenous Glycocalyx Forms a Bidirectional “Don’t Eat” and “Don’t Eat Me” Barrier to Phagocytosis. Curr Biol (2021) 31(1):77–89. doi: 10.1016/j.cub.2020.09.082
46. Paszek MJ, DuFort CC, Rossier O, Bainer R, Mouw JK, Godula K, et al. The Cancer Glycocalyx Mechanically Primes Integrin-Mediated Growth and Survival. Nature (2014) 511(7509):319–25. doi: 10.1038/nature13535
47. Ghasempour S, Freeman SA. The Glycocalyx and Immune Evasion in Cancer. FEBS J (2022). doi: 10.1111/febs.16236
48. Möckl L, Pedram K, Roy AR, Krishnan V, Gustavsson A-K, Dorigo O, et al. Quantitative Super-Resolution Microscopy of the Mammalian Glycocalyx. Dev Cell (2019) 50(1):57–72.e6. doi: 10.1016/j.devcel.2019.04.035
49. Möckl L. The Emerging Role of the Mammalian Glycocalyx in Functional Membrane Organization and Immune System Regulation. Front Cell Dev Biol (2020) 8:253/full. doi: 10.3389/fcell.2020.00253/full
50. Bai K, Wang W. Spatio-Temporal Development of the Endothelial Glycocalyx Layer and Its Mechanical Property In Vitro. J R Soc Interface (2012) 9(74):2290–8. doi: 10.1098/rsif.2011.0901
51. Bao G, Suresh S. Cell and Molecular Mechanics of Biological Materials. Nat Mater (2003) 2(11):715–25. doi: 10.1038/nmat1001
52. Guimarães CF, Gasperini L, Marques AP, Reis RL. The Stiffness of Living Tissues and Its Implications for Tissue Engineering. Nat Rev Mater (2020) 5(5):351–70. doi: 10.1038/s41578-019-0169-1
53. Blumenthal D, Chandra V, Avery L, Burkhardt JK. Mouse T Cell Priming is Enhanced by Maturation-Dependent Stiffening of the Dendritic Cell Cortex. eLife (2020) 9:e55995. Akhmanova A, Dustin ML, Hivroz C, Huse M. doi: 10.7554/eLife.55995
54. O’Connor RS, Hao X, Shen K, Bashour K, Akimova T, Hancock WW, et al. Substrate Rigidity Regulates Human T Cell Activation and Proliferation. J Immunol (2012) 189(3):1330–9. doi: 10.4049/jimmunol.1102757
55. Judokusumo E, Tabdanov E, Kumari S, Dustin ML, Kam LC. Mechanosensing in T Lymphocyte Activation. Biophys J (2012) 102(2):L5–7. doi: 10.1016/j.bpj.2011.12.011
56. Hui KL, Balagopalan L, Samelson LE, Upadhyaya A. Cytoskeletal Forces During Signaling Activation in Jurkat T-Cells. Mol Biol Cell (2015) 26(4):685–95. doi: 10.1091/mbc.E14-03-0830
57. Saitakis M, Dogniaux S, Goudot C, Bufi N, Asnacios S, Maurin M, et al. Different TCR-Induced T Lymphocyte Responses Are Potentiated by Stiffness With Variable Sensitivity. eLife (2017) 6:e23190. Dustin ML, editor. doi: 10.7554/eLife.23190
58. Nataraj NM, Dang AP, Kam LC, Lee JH. Ex Vivo Induction of Regulatory T Cells From Conventional CD4+ T Cells Is Sensitive to Substrate Rigidity. J Biomed Materials Res Part A. (2018) 106(12):3001–8. doi: 10.1002/jbm.a.36489
59. Alatoom A, Sapudom J, Soni P, Mohamed WKE, Garcia-Sabaté A, Teo J. Artificial Biosystem for Modulation of Interactions Between Antigen-Presenting Cells and T Cells. Adv Biosyst (2020) 4(7):e2000039. doi: 10.1002/adbi.202000039
60. Tello-Lafoz M, Srpan K, Sanchez EE, Hu J, Remsik J, Romin Y, et al. Cytotoxic Lymphocytes Target Characteristic Biophysical Vulnerabilities in Cancer. Immunity (2021) 54(5):1037–1054.e7. doi: 10.1016/j.immuni.2021.02.020
61. van Ewijk W. Immunoelectron-Microscopic Characterization of Lymphoid Microenvironments in the Lymph Node and Thymus. Ciba Found Symp (1980) 71:21–37. doi: 10.1002/9780470720547.ch3
62. Polliack A, Lampen N, Clarkson BD, de Harven E, Bentwich Z, Siegal FP, et al. Identification of Human B and T Lymphocytes by Scanning Electron Microscopy. J Exp Med (1973) 138(3):607–24. doi: 10.1084/jem.138.3.607
63. Sanderson CJ, Glauert AM. The Mechanism of T-cell Mediated Cytotoxicity. VI. T-Cell Projections and Their Role in Target Cell Killing. Immunology (1979) 36(1):119–29.
64. Polliack A. The Contribution of Scanning Electron Microscopy in Haematology: Its Role in Defining Leucocyte and Erythrocyte Disorders. J Microscopy (1981) 123(2):177–87. doi: 10.1111/j.1365-2818.1981.tb01293.x
65. Majstoravich S, Zhang J, Nicholson-Dykstra S, Linder S, Friedrich W, Siminovitch KA, et al. Lymphocyte Microvilli Are Dynamic, Actin-Dependent Structures That Do Not Require Wiskott-Aldrich Syndrome Protein (Wasp) for Their Morphology. Blood (2004) 104(5):1396–403. doi: 10.1182/blood-2004-02-0437
66. Brossard C, Feuillet V, Schmitt A, Randriamampita C, Romao M, Raposo G, et al. Multifocal Structure of the T Cell - Dendritic Cell Synapse. Eur J Immunol (2005) 35(6):1741–53. doi: 10.1002/eji.200425857
67. Ueda H, Zhou J, Xie J, Davis MM. Distinct Roles of Cytoskeletal Components in Immunological Synapse Formation and Directed Secretion. J Immunol (2015) 195(9):4117–25. doi: 10.4049/jimmunol.1402175
68. Robert P, Touchard D, Bongrand P, Pierres A. Biophysical Description of Multiple Events Contributing Blood Leukocyte Arrest on Endothelium. Front Immunol (2013) 4:108. doi: 10.3389/fimmu.2013.00108
69. Abadier M, Pramod AB, McArdle S, Marki A, Fan Z, Gutierrez E, et al. Effector and Regulatory T Cells Roll at High Shear Stress by Inducible Tether and Sling Formation. Cell Rep (2017) 21(13):3885–99. doi: 10.1016/j.celrep.2017.11.099
70. Brodovitch A, Limozin L, Bongrand P, Pierres A. Use of TIRF to Monitor T-Lymphocyte Membrane Dynamics With Submicrometer and Subsecond Resolution. Cell Mol Bioeng (2015) 8(1):178–86. doi: 10.1007/s12195-014-0361-8
71. Husson J, Chemin K, Bohineust A, Hivroz C, Henry N. Force Generation Upon T Cell Receptor Engagement. PloS One (2011) 6(5):e19680. doi: 10.1371/journal.pone.0019680
72. Sawicka A, Babataheri A, Dogniaux S, Barakat AI, Gonzalez-Rodriguez D, Hivroz C, et al. Micropipette Force Probe to Quantify Single-Cell Force Generation: Application to T-cell Activation. MBoC (2017) 28(23):3229–39. Théry M, editor. doi: 10.1091/mbc.e17-06-0385
73. Zucchetti AE, Paillon N, Markova O, Dogniaux S, Hivroz C, Husson J. Influence of External Forces on Actin-Dependent T Cell Protrusions During Immune Synapse Formation. Biol Cell (2021) 113(5):250–63. doi: 10.1111/boc.202000133
74. DeMond AL, Mossman KD, Starr T, Dustin ML, Groves JT. T Cell Receptor Microcluster Transport Through Molecular Mazes Reveals Mechanism of Translocation. Biophys J (2008) 94(8):3286–92. doi: 10.1529/biophysj.107.119099
75. Varma R, Campi G, Yokosuka T, Saito T, Dustin ML. T Cell Receptor-Proximal Signals Are Sustained in Peripheral Microclusters and Terminated in the Central Supramolecular Activation Cluster. Immunity (2006) 25(1):117–27. doi: 10.1016/j.immuni.2006.04.010
76. Fölser M, Motsch V, Platzer R, Huppa JB, Schütz GJ. A Multimodal Platform for Simultaneous T-Cell Imaging, Defined Activation, and Mechanobiological Characterization. Cells (2021) 10(2):235. doi: 10.3390/cells10020235
77. Razvag Y, Neve-Oz Y, Sajman J, Reches M, Sherman E. Nanoscale Kinetic Segregation of TCR and CD45 in Engaged Microvilli Facilitates Early T Cell Activation. Nat Commun (2018) 9(1):732. doi: 10.1038/s41467-018-03127-w
78. Orbach R, Su X. Surfing on Membrane Waves: Microvilli, Curved Membranes, and Immune Signaling. Front Immunol (2020) 11:2187/full. doi: 10.3389/fimmu.2020.02187/full
79. Aramesh M, Mergenthal S, Issler M, Plochberger B, Weber F, Qin X-H, et al. Functionalized Bead Assay to Measure Three-Dimensional Traction Forces During T-cell Activation. Nano Lett (2021) 21(1):507–14. doi: 10.1021/acs.nanolett.0c03964
80. Ghosh S, Di Bartolo V, Tubul L, Shimoni E, Kartvelishvily E, Dadosh T, et al. Erm-Dependent Assembly of T Cell Receptor Signaling and Co-stimulatory Molecules on Microvilli Prior to Activation. Cell Rep (2020) 30(10):3434–3447.e6. doi: 10.1016/j.celrep.2020.02.069
81. Farrell MV, Webster S, Gaus K, Goyette J. T Cell Membrane Heterogeneity Aids Antigen Recognition and T Cell Activation. Front Cell Dev Biol (2020) 8:609. doi: 10.3389/fcell.2020.00609
82. Rossboth B, Arnold AM, Ta H, Platzer R, Kellner F, Huppa JB, et al. Tcrs Are Randomly Distributed on the Plasma Membrane of Resting Antigen-Experienced T Cells. Nat Immunol (2018) 19(8):821–7. doi: 10.1038/s41590-018-0162-7
83. Jung Y, Wen L, Altman A, Ley K. CD45 Pre-Exclusion From the Tips of T Cell Microvilli Prior to Antigen Recognition. Nat Commun (2021) 12(1):3872. doi: 10.1038/s41467-021-23792-8
84. Razvag Y, Neve-Oz Y, Sajman J, Yakovian O, Reches M, Sherman E. T Cell Activation Through Isolated Tight Contacts. Cell Rep (2019) 29(11):3506–3521.e6. doi: 10.1016/j.celrep.2019.11.022
85. Aramesh M, Stoycheva D, Sandu I, Ihle SJ, Zünd T, Shiu J-Y, et al. Nanoconfinement of Microvilli Alters Gene Expression and Boosts T Cell Activation. Proc Natl Acad Sci USA (2021) 118(40):e2107535118. doi: 10.1073/pnas.2107535118
86. Miller MJ, Safrina O, Parker I, Cahalan MD. Imaging the Single Cell Dynamics of CD4+ T Cell Activation by Dendritic Cells in Lymph Nodes. J Exp Med (2004) 200(7):847–56. doi: 10.1084/jem.20041236
87. Mempel TR, Henrickson SE, Von Andrian UH. T-Cell Priming by Dendritic Cells in Lymph Nodes Occurs in Three Distinct Phases. Nature (2004) 427(6970):154–9. doi: 10.1038/nature02238
88. Miller MJ, Hejazi AS, Wei SH, Cahalan MD, Parker I. T Cell Repertoire Scanning Is Promoted by Dynamic Dendritic Cell Behavior and Random T Cell Motility in the Lymph Node. Proc Natl Acad Sci USA (2004) 101(4):998–1003. doi: 10.1073/pnas.0306407101
89. Giannone G, Dubin-Thaler BJ, Döbereiner H-G, Kieffer N, Bresnick AR, Sheetz MP. Periodic Lamellipodial Contractions Correlate With Rearward Actin Waves. Cell (2004) 116(3):431–43. doi: 10.1016/S0092-8674(04)00058-3
90. Kaizuka Y, Douglass AD, Varma R, Dustin ML, Vale RD. Mechanisms for Segregating T Cell Receptor and Adhesion Molecules During Immunological Synapse Formation in Jurkat T Cells. PNAS (2007) 104(51):20296–301. doi: 10.1073/pnas.0710258105
91. Murugesan S, Hong J, Yi J, Li D, Beach JR, Shao L, et al. Formin-Generated Actomyosin Arcs Propel T Cell Receptor Microcluster Movement at the Immune Synapse. J Cell Biol (2016) 215(3):383–99. doi: 10.1083/jcb.201603080
92. Colin-York H, Javanmardi Y, Skamrahl M, Kumari S, Chang VT, Khuon S, et al. Cytoskeletal Control of Antigen-Dependent T Cell Activation. Cell Rep (2019) 26(12):3369–79. doi: 10.1016/j.celrep.2019.02.074
93. Ueda H, Morphew MK, McIntosh JR, Davis MM. Cd4+ T-cell Synapses Involve Multiple Distinct Stages. Proc Natl Acad Sci USA (2011) 108(41):17099–104. doi: 10.1073/pnas.1113703108
94. Fernandes RA, Ganzinger KA, Tzou JC, Jönsson P, Lee SF, Palayret M, et al. A Cell Topography-Based Mechanism for Ligand Discrimination by the T Cell Receptor. PNAS (2019) 116(28):14002–10. doi: 10.1073/pnas.1817255116
95. Aleksic M, Dushek O, Zhang H, Shenderov E, Chen J-L, Cerundolo V, et al. Dependence of T Cell Antigen Recognition on T Cell Receptor-Peptide Mhc Confinement Time. Immunity (2010) 32(2):163–74. doi: 10.1016/j.immuni.2009.11.013
96. Luxembourg AT, Brunmark A, Kong Y, Jackson MR, Peterson PA, Sprent J, et al. Requirements for Stimulating Naive Cd8+ T Cells Via Signal 1 Alone. J Immunol (1998) 161(10):5226–35.
97. Segura J-M, Guillaume P, Mark S, Dojcinovic D, Johannsen A, Bosshard G, et al. Increased Mobility of Major Histocompatibility Complex I-Peptide Complexes Decreases the Sensitivity of Antigen Recognition. J Biol Chem (2008) 283(35):24254–63. doi: 10.1074/jbc.M803549200
98. Comrie WA, Li S, Boyle S, Burkhardt JK. The Dendritic Cell Cytoskeleton Promotes T Cell Adhesion and Activation by Constraining ICAM-1 Mobility. J Cell Biol (2015) 208(4):457–73. doi: 10.1083/jcb.201406120
99. Mecheri S, Edidin M, Dannecker G, Mittler RS, Hoffmann MK. Immunogenic Ia-binding Peptides Immobilize the Ia Molecule and Facilitate Its Aggregation on the B Cell Membrane. Control by the M1s-1 Gene. J Immunol (1990) 144(4):1361–8.
100. Treanor B, Harwood NE, Batista FD. Microsignalosomes: Spatially Resolved Receptor Signalling. Biochem Soc Trans (2009) 37(5):1014–8. doi: 10.1042/BST0371014
101. Göhring J, Kellner F, Schrangl L, Platzer R, Klotzsch E, Stockinger H, et al. Temporal Analysis of T-Cell Receptor-Imposed Forces Via Quantitative Single Molecule Fret Measurements. Nat Commun (2021) 12(1):2502. doi: 10.1038/s41467-021-22775-z.
102. Hsu C-J, Hsieh W-T, Waldman A, Clarke F, Huseby ES, Burkhardt JK, et al. Ligand Mobility Modulates Immunological Synapse Formation and T Cell Activation. PloS One (2012) 7(2):e32398. doi: 10.1371/journal.pone.0032398
103. Dillard P, Varma R, Sengupta K, Limozin L. Ligand-Mediated Friction Determines Morphodynamics of Spreading T Cells. Biophys J (2014) 107(11):2629–38. doi: 10.1016/j.bpj.2014.10.044
104. Hellmeier JP, Platzer R, Karner A, Motsch V, Bamieh V, Preiner J, et al. Spatial Requirements for T-Cell Receptor Triggering Probed Via Functionalized Dna Origami Platforms. Biophys J (2020) 118(3):245a. doi: 10.1016/j.bpj.2019.11.1437
105. Mattila PK, Lappalainen P. Filopodia: Molecular Architecture and Cellular Functions. Nat Rev Mol Cell Biol (2008) 9(6):446–54. doi: 10.1038/nrm2406
106. Dai J, Sheetz MP. Mechanical Properties of Neuronal Growth Cone Membranes Studied by Tether Formation With Laser Optical Tweezers. Biophys J (1995) 68(3):988–96. doi: 10.1016/S0006-3495(95)80274-2
107. Schwarz US, Gardel ML. United We Stand: Integrating the Actin Cytoskeleton and Cell-Matrix Adhesions in Cellular Mechanotransduction. J Cell Sci (2012) 125(Pt 13):3051–60. doi: 10.1242/jcs.093716
108. Labernadie A, Bouissou A, Delobelle P, Balor S, Voituriez R, Proag A, et al. Protrusion Force Microscopy Reveals Oscillatory Force Generation and Mechanosensing Activity of Human Macrophage Podosomes. Nat Commun (2014) 5(1):5343. doi: 10.1038/ncomms6343
109. Glazier R, Salaita K. Supported Lipid Bilayer Platforms to Probe Cell Mechanobiology. Biochim Biophys Acta (2017) 1859(9 Pt A):1465–82. doi: 10.1016/j.bbamem.2017.05.005
110. Tamzalit F, Wang MS, Jin W, Tello-Lafoz M, Boyko V, Heddleston JM, et al. Interfacial Actin Protrusions Mechanically Enhance Killing by Cytotoxic T Cells. Sci Immunol (2019) 4(33):22. doi: 10.1126/sciimmunol.aav5445
111. Dustin ML, Bromley SK, Davis MM, Zhu C. Identification of Self Through Two-Dimensional Chemistry and Synapses. Annu Rev Cell Dev Biol (2001) 17:133–57. doi: 10.1146/annurev.cellbio.17.1.133
112. Huppa JB, Axmann M, Mörtelmaier MA, Lillemeier BF, Newell EW, Brameshuber M, et al. Tcr-peptide-MHC Interactions In Situ Show Accelerated Kinetics and Increased Affinity. Nature (2010) 463(7283):963–7. doi: 10.1038/nature08746
113. Kim ST, Takeuchi K, Sun Z-YJ, Touma M, Castro CE, Fahmy A, et al. The αβ T Cell Receptor Is an Anisotropic Mechanosensor. J Biol Chem (2009) 284(45):31028–37. doi: 10.1074/jbc.M109.052712
114. Li Y-C, Chen B-M, Wu P-C, Cheng T-L, Kao L-S, Tao M-H, et al. Cutting Edge: Mechanical Forces Acting on T Cells Immobilized Via the TCR Complex Can Trigger TCR Signaling. J Immunol (2010) 184(11):5959–63. doi: 10.4049/jimmunol.0900775
115. Puech P-H, Nevoltris D, Robert P, Limozin L, Boyer C, Bongrand P. Force Measurements of TCR/pMHC Recognition at T Cell Surface. PloS One (2011) 6(7):e22344. doi: 10.1371/journal.pone.0022344
116. Pryshchep S, Zarnitsyna VI, Hong J, Evavold BD, Zhu C. Accumulation of Serial Forces on TCR and CD8 Frequently Applied by Agonist Antigenic Peptides Embedded in MHC Molecules Triggers Calcium in T Cells. J Immunol (2014) 193(1):68–76. doi: 10.4049/jimmunol.1303436
117. Liu B, Chen W, Evavold BD, Zhu C. Accumulation of Dynamic Catch Bonds Between TCR and Agonist Peptide-MHC Triggers T Cell Signaling. Cell (2014) 157(2):357–68. doi: 10.1016/j.cell.2014.02.053
118. Das DK, Feng Y, Mallis RJ, Li X, Keskin DB, Hussey RE, et al. Force-Dependent Transition in the T-Cell Receptor β-Subunit Allosterically Regulates Peptide Discrimination and pMHC Bond Lifetime. Proc Natl Acad Sci USA (2015) 112(5):1517–22. doi: 10.1073/pnas.1424829112
119. Hong J, Persaud SP, Horvath S, Allen PM, Evavold BD, Zhu C. Force-Regulated In Situ TCR-Peptide-Bound Mhc Class Ii Kinetics Determine Functions of CD4+ T Cells. J Immunol (2015) 195(8):3557–64. doi: 10.4049/jimmunol.1501407
120. Mallis RJ, Bai K, Arthanari H, Hussey RE, Handley M, Li Z, et al. Pre-TCR Ligand Binding Impacts Thymocyte Development Before αβtcr Expression. Proc Natl Acad Sci USA (2015) 112(27):8373–8. doi: 10.1073/pnas.1504971112
121. Liu B, Chen W, Natarajan K, Li Z, Margulies DH, Zhu C. The Cellular Environment Regulates In Situ Kinetics of T-cell Receptor Interaction With Peptide Major Histocompatibility Complex. Eur J Immunol (2015) 45(7):2099–110. doi: 10.1002/eji.201445358
122. Feng Y, Brazin KN, Kobayashi E, Mallis RJ, Reinherz EL, Lang MJ. Mechanosensing Drives Acuity of αβ T-cell Recognition. Proc Natl Acad Sci USA (2017) 114(39):E8204–13. doi: 10.1073/pnas.1703559114
123. Sibener LV, Fernandes RA, Kolawole EM, Carbone CB, Liu F, McAffee D, et al. Isolation of a Structural Mechanism for Uncoupling T Cell Receptor Signaling From Peptide-MHC Binding. Cell (2018) 174(3):672–687.e27. doi: 10.1016/j.cell.2018.06.017
124. Bernardeau K, Gouard S, David G, Ruellan A-L, Devys A, Barbet J, et al. Assessment of CD8 Involvement in T Cell Clone Avidity by Direct Measurement of HLA-A2/Mage3 Complex Density Using a High-Affinity TCR Like Monoclonal Antibody. Eur J Immunol (2005) 35(10):2864–75. doi: 10.1002/eji.200526307
125. Purbhoo MA, Irvine DJ, Huppa JB, Davis MM. T Cell Killing Does Not Require the Formation of a Stable Mature Immunological Synapse. Nat Immunol (2004) 5(5):524–30. doi: 10.1038/ni1058
126. Chabaud M, Paillon N, Gaus K, Hivroz C. Mechanobiology of Antigen-Induced T Cell Arrest. Biol Cell (2020) 112(7):196–212. doi: 10.1111/boc.201900093
127. Allard JF, Dushek O, Coombs D, van der Merwe PA. Mechanical Modulation of Receptor-Ligand Interactions at Cell-Cell Interfaces. Biophys J (2012) 102(6):1265–73. doi: 10.1016/j.bpj.2012.02.006
128. Kumari S, Mak M, Poh Y, Tohme M, Watson N, Melo M, et al. Cytoskeletal Tension Actively Sustains the Migratory T-Cell Synaptic Contact. EMBO J (2020) 39(5):e102783. doi: 10.15252/embj.2019102783
129. Zhu C, Chen Y, Ju LA. Dynamic Bonds and Their Roles in Mechanosensing. Curr Opin Chem Biol (2019) 53:88–97. doi: 10.1016/j.cbpa.2019.08.005
130. Limozin L, Bridge M, Bongrand P, Dushek O, van der Merwe PA, Robert P. Tcr–pMHC Kinetics Under Force in a Cell-Free System Show No Intrinsic Catch Bond, But a Minimal Encounter Duration Before Binding. Proc Natl Acad Sci USA (2019) 116(34):16943–8. doi: 10.1073/pnas.1902141116
131. Hosseini BH, Louban I, Djandji D, Wabnitz GH, Deeg J, Bulbuc N, et al. Immune Synapse Formation Determines Interaction Forces Between T Cells and Antigen-Presenting Cells Measured by Atomic Force Microscopy. Proc Natl Acad Sci USA 106(42):17852–7. doi: 10.1073/pnas.0905384106
132. Lim TS, Mortellaro A, Lim CT, Hämmerling GJ, Ricciardi-Castagnoli P. Mechanical Interactions Between Dendritic Cells and T Cells Correlate With T Cell Responsiveness. J Immunol (2011) 187(1):258–65. doi: 10.4049/jimmunol.1100267
133. Bashour KT, Gondarenko A, Chen H, Shen K, Liu X, Huse M, et al. CD28 and CD3 Have Complementary Roles in T-Cell Traction Forces. PNAS (2014) 111(6):2241–6. doi: 10.1073/pnas.1315606111
134. Liu Y, Blanchfield L, Ma VP-Y, Andargachew R, Galior K, Liu Z, et al. DNA-Based Nanoparticle Tension Sensors Reveal That T-cell Receptors Transmit Defined Pn Forces to Their Antigens for Enhanced Fidelity. PNAS (2016) 113(20):5610–5. doi: 10.1073/pnas.1600163113
135. Ma VP-Y, Liu Y, Blanchfield L, Su H, Evavold BD, Salaita K. Ratiometric Tension Probes for Mapping Receptor Forces and Clustering at Intermembrane Junctions. Nano Lett (2016) 16(7):4552–9. doi: 10.1021/acs.nanolett.6b01817
136. Ma R, Kellner AV, Ma VP-Y, Su H, Deal BR, Brockman JM, et al. DNA Probes That Store Mechanical Information Reveal Transient Piconewton Forces Applied by T Cells. Proc Natl Acad Sci USA (2019) 116(34):16949–54. doi: 10.1073/pnas.1904034116
137. Morimatsu M, Mekhdjian AH, Adhikari AS, Dunn AR. Molecular Tension Sensors Report Forces Generated by Single Integrin Molecules in Living Cells. Nano Lett (2013) 13(9):3985–9. doi: 10.1021/nl4005145
138. Chang AC, Mekhdjian AH, Morimatsu M, Denisin AK, Pruitt BL, Dunn AR. Single Molecule Force Measurements in Living Cells Reveal a Minimally Tensioned Integrin State. ACS Nano (2016) 10(12):10745–52. doi: 10.1021/acsnano.6b03314
139. Becker N, Oroudjev E, Mutz SJ, Cleveland JP, Hansma PK, Hayashi CY, et al. Molecular Nanosprings in Spider Capture-Silk Threads. Nat Materials (2003) 2(4):278–83. doi: 10.1038/nmat858
140. Brenner MD, Zhou R, Conway DE, Lanzano L, Gratton E, Schwartz MA, et al. Spider Silk Peptide Is a Compact, Linear Nanospring Ideal for Intracellular Tension Sensing. Nano Lett (2016) 16(3):2096–102. doi: 10.1021/acs.nanolett.6b00305
141. Choudhuri K, Wiseman D, Brown MH, Gould K, van der Merwe PA. T-Cell Receptor Triggering Is Critically Dependent on the Dimensions of Its peptide-MHC Ligand. Nature (2005) 436(7050):578–82. doi: 10.1038/nature03843
142. Blanchard A, Combs JD, Brockman JM, Kellner AV, Glazier R, Su H, et al. Turn-Key Mapping of Cell Receptor Force Orientation and Magnitude Using a Commercial Structured Illumination Microscope. Nat Commun (2021) 12:4693. doi: 10.1038/s41467-021-24602-x
143. Basu R, Huse M. Mechanical Communication at the Immunological Synapse. Trends Cell Biol (2017) 27(4):241–54. doi: 10.1016/j.tcb.2016.10.005
144. Comrie WA, Burkhardt JK. Action and Traction: Cytoskeletal Control of Receptor Triggering at the Immunological Synapse. Front Immunol (2016) 7:68. doi: 10.3389/fimmu.2016.00068
Keywords: immune surveillance, mechanical force, T-cell antigen recognition, glycocalyx, physical barriers, microvilli, membrane ultrastructure
Citation: Göhring J, Schrangl L, Schütz GJ and Huppa JB (2022) Mechanosurveillance: Tiptoeing T Cells. Front. Immunol. 13:886328. doi: 10.3389/fimmu.2022.886328
Received: 28 February 2022; Accepted: 19 April 2022;
Published: 26 May 2022.
Edited by:
Jerome Delon, INSERM U1016 Institut Cochin, FranceReviewed by:
Claire Hivroz, Maria Sklodowska-Curie National Research Institute of Oncology, PolandJanis K. Burkhardt, University of Pennsylvania, United States
Kheya Sengupta, Centre Interdisciplinaire de Nanoscience de Marseille (CINaM)/CNRS, France
Copyright © 2022 Göhring, Schrangl, Schütz and Huppa. This is an open-access article distributed under the terms of the Creative Commons Attribution License (CC BY). The use, distribution or reproduction in other forums is permitted, provided the original author(s) and the copyright owner(s) are credited and that the original publication in this journal is cited, in accordance with accepted academic practice. No use, distribution or reproduction is permitted which does not comply with these terms.
*Correspondence: Janett Göhring, amFuZXR0LmdvZWhyaW5nQG1lZHVuaXdpZW4uYWMuYXQ=