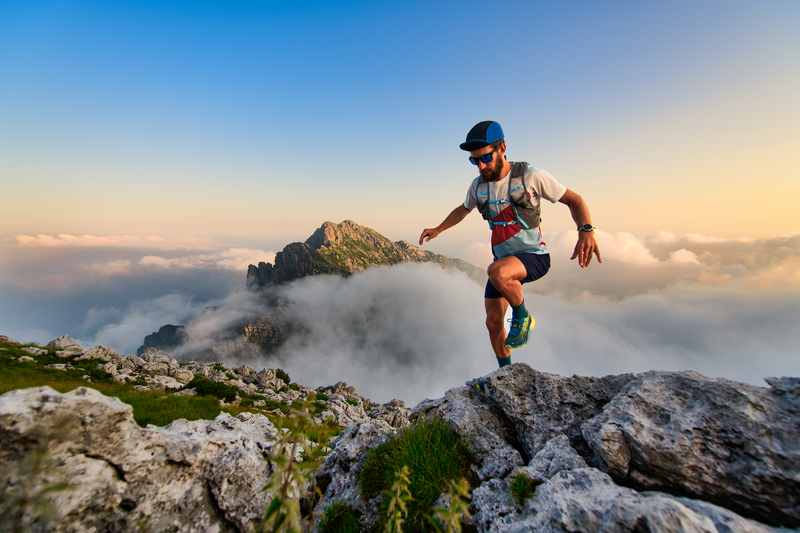
95% of researchers rate our articles as excellent or good
Learn more about the work of our research integrity team to safeguard the quality of each article we publish.
Find out more
ORIGINAL RESEARCH article
Front. Immunol. , 30 June 2022
Sec. Viral Immunology
Volume 13 - 2022 | https://doi.org/10.3389/fimmu.2022.884760
This article is part of the Research Topic Deleterious and Beneficial Humoral Immune Response in Viral Diseases: Two Sides of the Same Coin View all 9 articles
The SARS-CoV-2 pandemic has had a social and economic impact worldwide, and vaccination is an efficient strategy for diminishing those damages. New adjuvant formulations are required for the high vaccine demands, especially adjuvant formulations that induce a Th1 phenotype. Herein we assess a vaccination strategy using a combination of Alum and polyinosinic:polycytidylic acid [Poly(I:C)] adjuvants plus the SARS-CoV-2 spike protein in a prefusion trimeric conformation by an intradermal (ID) route. We found high levels of IgG anti-spike antibodies in the serum by enzyme linked immunosorbent assay (ELISA) and high neutralizing titers against SARS-CoV-2 in vitro by neutralization assay, after two or three immunizations. By evaluating the production of IgG subtypes, as expected, we found that formulations containing Poly(I:C) induced IgG2a whereas Alum did not. The combination of these two adjuvants induced high levels of both IgG1 and IgG2a. In addition, cellular immune responses of CD4+ and CD8+ T cells producing interferon-gamma were equivalent, demonstrating that the Alum + Poly(I:C) combination supported a Th1 profile. Based on the high neutralizing titers, we evaluated B cells in the germinal centers, which are specific for receptor-binding domain (RBD) and spike, and observed that more positive B cells were induced upon the Alum + Poly(I:C) combination. Moreover, these B cells produced antibodies against both RBD and non-RBD sites. We also studied the impact of this vaccination preparation [spike protein with Alum + Poly(I:C)] in the lungs of mice challenged with inactivated SARS-CoV-2 virus. We found a production of IgG, but not IgA, and a reduction in neutrophil recruitment in the bronchoalveolar lavage fluid (BALF) of mice, suggesting that our immunization scheme reduced lung inflammation. Altogether, our data suggest that Alum and Poly(I:C) together is a possible adjuvant combination for vaccines against SARS-CoV-2 by the intradermal route.
Intradermal (ID) vaccination has been shown to be a promising strategy to increase the immune response against pandemic viruses such as H5N1 (1), Ebola (2), Vaccinia (3), and SARS-CoV-2 (4). This strategy has already been tested for vaccines against other betacoronaviruses such as SARS-CoV (5, 6), and MERS-CoV (7). Furthermore, the ID route has also been used for clinical trials of MERS-CoV (NCT03721718) and SARS-CoV-2 vaccines (4, 8).
Currently, there are many mRNA and DNA vaccine candidates administered ID against SARS-CoV-2 in mice (9, 10), non-human primates (11) and rabbits (12). Covaxin, an inactivated vaccine developed by Indian pharmaceutical company, Bharat Biotech, was previously tested in mice, rats, and rabbits by the ID route, although the study had focused on the intramuscular (IM) route (13).
The advantages of the ID route include its easy administration through the use of painless microneedles that penetrate the skin, thus increasing vaccination acceptance and coverage, in addition to reducing errors in application and ensuring greater stability of the vaccine formulation (14). Besides, ID immunization has shown success to expand the germinal center (GC) (15), where plasma and memory B cells are regularly generated (16, 17). Potent elicitation of GC response has been demonstrated in experimental mice model of vaccination, which in its turn was highly related to the induction of specific antibodies against SARS-CoV-2, as well as neutralizing antibodies (10, 18, 19).
Furthermore, ID has already been shown to be a convenient route for the delivery of adjuvants (1). Polyinosinic:polycytidylic acid (Poly(I:C)) is a synthetic analogue of double-stranded (ds) RNA, a molecular pattern associated with viral infections (20). Poly(I:C) triggers a potent type 1 interferon (IFN) response through Toll-like receptor 3 (TLR3) and RIG-I-like receptor (RLR) (21). The activation of these receptors can induce expression of cytokines, chemokines, costimulatory factors, and other dsRNA-dependent systems, resulting in a cellular immune response for viral clearance (20).
A study comparing the role of Poly(I:C) and two derivatives as adjuvants in rhesus macaques demonstrated that treatment with all three Polys were able to induce T cell proliferation (22). Ampligen has been shown to enhance the immunogenicity of an H1N1 influenza vaccine in mice (23). PIKA, a stabilized derivative of Poly(I:C), was reported to be an adequate adjuvant candidate for an inactivated SARS-CoV vaccine, inducing strong anti-SARS-CoV mucosal and systemic humoral immune responses like IgA and IgG (24). Sun et al (25) reported that Ad5-hACE2-transduced mice, when treated intranasally with Poly(I:C), triggered weight loss control and led to greater viral clearance. Furthermore, Zhao, Wang and Wu showed that HLA-A*0201/Kb transgenic (Tg) mice injected subcutaneously (SC) with Poly(I:C) were able to induce a slight enhancement of SARS-CoV spike peptide-specific CD8+ T cells (26), demonstrating the potential use of Poly(I:C) as a vaccine candidate against SARS-CoV-2.
It was demonstrated that an inactivated vaccine candidate against MERS-CoV using Alum and MF59 adjuvants was able to induce neutralizing antibodies and reduce the viral load in the lungs of experimentally infected mice (22). On the other hand, increased lung immunopathology was observed which was associated with intense cell infiltration of eosinophils (27). However, to reduce eosinophilic infiltration in the lungs of mice, Iwata-Yoshikawa et al (28) used adjuvant containing Poly(I:C), which also resulted in lower levels of interleukin-4 (IL-4), IL-13, and eotaxin in the lungs. Moreover, Wang et al (29) demonstrated that the use of Poly(I:C) with other vaccine candidates was able to induce neutralizing and specific antibodies against MERS RBD in mice. Further to this, IFN-γ, IL-4, and IL-2 secreting cells were induced by the Poly(I:C) in comparison to the Alum adjuvant.
Data presented so far suggest that a vaccine given by the ID route using Poly(I:C) and Alum adjuvants could have great potential for a vaccination strategy against SARS-CoV-2. Here, we assess a vaccination strategy using SARS-CoV-2 spike protein in combination with Alum and Poly(I:C) adjuvants by an intradermal (ID) route in a mouse model. After two or three immunizations, we found high levels of IgG, IgG1 and IgG2a anti-spike serum antibodies, high neutralizing titers against SARS-CoV-2 and more Spike+RBD+ B cells in germinal center induced upon the Alum + Poly(I:C) combination. We also found the production of IgG, but not IgA, and a reduction in neutrophil recruitment in the BALF of mice in the challenge with inactivated SARS-CoV-2. Altogether, our data suggest that Alum and Poly(I:C) together is a great strategy for use in combination as adjuvants for vaccines against SARS-CoV-2.
Female BALB/c mice, 6–8 weeks old (n = 5 per group), were obtained from the breeding facility of UFRJ. All animals were kept in mini-isolators (Alesco, São Paulo, Brazil) and kept under controlled temperature and light/dark cycles of 12 h/12 h, in addition to receiving filtered water and commercial feed (Nuvilab, Curitiba, Paraná, Brazil). The experiments were carried out in accordance with the Ethics Committee on the Use of Animals of the Health Sciences Center of the Federal University of Rio de Janeiro (Comitê de Ética no Uso de Animais do Centro de Ciências da Saúde da Universidade Federal do Rio de Janeiro), under the protocol number: 074/20
The immunogen used is the whole soluble ectodomain (aminoacids 1-1208) of the spike (S) protein of SARS-CoV-2, containing mutations that stabilize it as a trimer in the prefusion conformation, as first proposed by Wrapp et al (30). The recombinant HEK293-derived affinity-purified S protein was obtained from the Cell Culture Engineering Laboratory of COPPE/UFRJ, and its purity and antigenicity have already been confirmed in previous works that used it to develop serological tests (31) and equine hyperimmune F(ab’)2 preparations (32). This protein was used in this work to immunize mice and as ELISA antigen to detect anti-SARS-CoV-2 antibodies in samples from immunized animals. Moreover, it was also labeled with Alexa-fluor-467 (similar to APC) and used in germinal center experiments.
RBD was produced in HEK293 cells and the production and purification was described previously (33). RBD was kindly donated by Dr. John Pak (Chan Zuckerberg Initiative Biohub).
To start labeling proteins with the Alexa kits from ThermoFisher, initially a dialysis was performed to transfer all proteins to PBS buffer. This procedure is recommended by the company to obtain results with a greater labeling efficiency. After this step, the proteins were quantified by Bradford method and then the standard protocol was continued (34). Then, 100 g of protein S and RBD were labeled with kits Alex-fluor-488 (494/519) and Alex-fluor-647 (650/688).
African green monkey kidney cells (Vero, subtype E6) were cultured in high glucose DMEM with 10% fetal bovine serum (FBS; HyClone, Logan, UT, USA), 100 U/mL penicillin and 100 μg/mL streptomycin (Pen/Strep; ThermoFisher, MA, USA) at 37°C in a humidified atmosphere with 5% CO2.
SARS-CoV-2 was prepared in Vero E6 cells. The isolates were originally obtained from a nasopharyngeal swab of a confirmed cases in Rio de Janeiro, Brazil (IRB approval, 30650420.4.1001.0008) and Delta variant B.1.617.2. All procedures related to virus culture were handled in a biosafety level 3 (BSL3) multiuser facility according to WHO guidelines. Virus titers were determined as plaque forming units (PFU)/mL. The virus strain was sequenced to confirm the identity and the complete genome is publicly available (https://www.ncbi.nlm.nih.gov/genbank/SARS-CoV-2/human/BRA/RJ01/2020 or MT710714). The virus stocks were kept in -80°C freezers.
The SARS-CoV particles were inactivated with β-propiolactone for 24 hours, followed by concentration in 30% sucrose cushion in 30000 RPM for 1:30 hour. The, the pellet was resuspended in phosphate buffer sodium and storege at -80°C.
Mice were anesthetized using an induction chamber with an atmosphere saturated with 5% Isoflurane (Cristália®, São Paulo, Brazil) in oxygen (Isoflurane Anesthesia Vaporizer System; Harvard Apparatus, MA, USA). Using a 300 µL 30 G syringe (BD Ultra-Fine™), immunization with S Ptn associated with the adjuvants Poly(I:C) HMW (VacciGrade™, 10 mg, batch #VPIC-34-05, In vivoGen, San Diego, CA, USA) and Alum/Alhydrogel 2% (lot #0001657855, In vivoGen, San Diego, CA, USA) was performed in the upper part of the right footpad, in the intradermal region, with visual confirmation of edema after the immunization. The animals received three immunizations, with the same dosage, with an interval of fourteen days between each dose (Supplementary Figure 7). Control animals received only the same volume of PBS. Administrations were as follows: 5 μg of S Ptn per dose (5 μL of a 1 mg/mL solution), 5 μg of Poly(I:C) (1 μL of a 5 mg/mL solution), and 50% of the final volume was Alhydrogel 2% (10 μL). For the combination of adjuvants with S Ptn, Poly(I:C) and Alum it was mixed before the immunization and co-administered. PBS was used to make up the final volume to 20 μL. For the challenge, mice received 20 μg of inactivated virus by intranasal route thirteen days after three immunizations.
Mice were immunized three times by intranasal route by installation of 5 µg of Spike protein alone associated with 5 µg Poly (I:C) as adjuvant.
Antigen-specific IgG (Cat. No. 1015-05, Southern Biotech, AL, USA), IgA (Cat. No.1040-05, Southern Biotech, AL, USA), IgG1 (Cat. No. 1071-01, Southern Biotech, AL, USA) and IgG2a (Cat. No.1101-01, Southern Biotech, AL, USA) levels were determined by enzyme linked immunosorbent assay (ELISA) using recombinant SARS-CoV-2 S Ptn as the capture antigen. ELISA plates (Corning, MA, USA) were coated with 4 μg/mL of S Ptn in PBS overnight at 4°C. The next morning, the coating solution was discarded and a blocking solution of PBS + 5% milk (Molico) was added for 1 h. The blocking solution from the ELISA plates was discarded and blood and BALF samples diluted in blocking solution were added for at least 2 h. After this incubation the ELISA plate was washed 5 times with a washing solution consisting of PBS + 0.05% Tween 20 and then the anti-mouse IgG, IgG1, IgG2a, and IgA-HRP detection antibodies (Southern Biotech, AL, USA) were added for another hour. The plate was washed 7 more times and TMB solution (Invitrogen, MA, USA) was added. The reaction was stopped with 1 N HCl and readed at 450nm. The normalized optical density (O.D.) was made by normalizing data from 4 different experiments using the control groups for that end. The O.D. summatory (Sum) was made by summing the values from normalized O.D. as previously described (25).
To assess the neutralization titer, we performed as previously described (35). Briefly, the serum samples were incubated with 100 PFU of SARS-CoV-2 (IRB approval, 30650420.4.1001.0008) and Delta variant B.1.617.2 with serial dilutions of mouse serum for 1 h at 37°C (to inactivate mouse serum, the samples were heated for 30 min at 56°C). Then, the samples were placed into 96-well plates with monolayers of Vero cells (2 x 104 cells/well) with supernatants for 1 h at 37°C. Cells were washed and fresh medium with 2% FBS and 2.4% carboxymethylcellulose was added. After 72 hours of infection, the monolayer was fixed with formalin 5% and stained with crystal violet dye solution. The cytopathic effect was scored by independent readers. The reader was blind in respect to the source of the supernatant.
Cells from lymph nodes (5 x 105) were washed with PBS by centrifugation at 400 g for 5 min at 4°C, blocked with 5 µL/well Human FcX (BioLegend) for 15 min, followed by 5 µL/well of the antibody cocktail and incubation for 30 min at 4°C in the dark. Cells were washed with a cytometry buffer solution (PBS with 5% FBS) then fixed with a 4% formaldehyde (Sigma-Aldrich) for 15 min at 4°C. Cells were washed, resuspended in cytometry buffer solution, and stored in the dark at 4°C until acquisition. The following antibodies were used: B220 (anti-B220-PerCP; eBiosciences), GL7 (anti-GL7-PE; BioLegend), CD38 (anti-CD38-PeCy7; BioLegend), RBD-conjugated with Alexa 488, S Ptn-conjugated with Alexa 647, TRCβ (anti-TRCβ-Pacific Blue; BioLegend), CD4 (anti-CD4-FITC; BioLegend), CD8 (anti-CD8-PeCy7; BioLegend), and IFN-γ (anti-IFN-γ-APC; BioLegend). To evaluated B cell, we analyzed B220+ cells. To look cell population inside germinal center we used the strategy (CD38- and GL7+) and outside GC (CD38+GL7-). After perform pre-gate on those population, we evaluated the binding with Spike and RBD (Strategy of gate in Supplementary Figure 2). Acquisition of events (100 thousand) was performed on a Becton-Dickinson LSR-II/Fortessa flow cytometer (BD Biosciences, San Diego, CA, USA). The gate strategy was performed based on the selection of cell size (FSC) and composition (SSC). After identifying the main population, a new gate was performed using the FSC-A (area) and FSC-H (weight), where cellular doublets were excluded. The data analyzes were performed using the FlowJo® software vX.0.7.
Immune cells were harvested from the airways via bronchoalveolar lavage (BAL). Briefly, mice were cannulated via a small tracheal incision and the lungs were flushed with 1 mL of sterile PBS. The collected BAL fluids were centrifuged at 1,500 rpm for 5 min at 4°C, and total cells were prepared for flow cytometry staining to determine the number and types of cells. Fc-blocked (1 μg/mL; eBiosciences) BALF cells were stained with anti-mouse SiglecF-PE (0.3 μg/mL; BD Pharmingen), CD11c-APC (0.3 μg/mL; eBiosciences), CD11b-PerCP (0.3 μg/mL; BioLegend), CD19-PECy5 (0.8 μg/mL; eBiosciences), Ly6G-PECy7 (0.8 μg/mL; BioLegend), Ly6C-APC-Cy7 (0.8 μg/mL; BD Pharmingen), and TCRβ-Pacific Blue (0.3 μg/mL; BioLegend). All samples were analyzed on a Becton-Dickinson LSR-II/Fortessa flow cytometer (BD Biosciences, San Diego, CA, USA) and analyzed by using FlowJo software (Tree Star Inc.).
Results are expressed as mean ± SEM with confidence level of p ≤ 0.05. For multiple comparisons, a one-way ANOVA followed by Tukey multiple comparison test was performed and a two-way ANOVA followed by Bonferroni post-test to compare replicate means by row. Data analysis was performed using GraphPad Prism® 8.0 software.
In order to understand whether the vaccine formulations were generating an effective B cell response, we evaluated the different antibody isotypes in the serum and BALF. Mice immunized with S Ptn + Alum, or S Ptn + Poly(I:C) + Alum produced higher total specific IgG levels than the group inoculated with S Ptn alone (Figures 1A–D). This occurred both at one week after two immunizations (Figures 1A, B) and three immunizations (Figures 1C, D). When we compared the adjuvant formulations combined with S Ptn between the two and three immunizations, we observed that three immunizations was enough to represent a higher O.D Sum than two immunizations (Supplementary Figure 1F). There were very low levels of detectable serum IgG 7 days after prime (Supplementary Figure 1A).
Figure 1 Formulations containing adjuvants induce strong IgG response in the serum. Antigen-specific antibody levels were determined by ELISA and normalized using the control groups. Serum IgG levels were evaluated after two (A, B) or three immunizations (C, D). The summatory of all dilutions are represented in (B, D). Data in this figure consists 4 independent experiments normalized using the control groups and shown as mean ± S.D. Groups: Naïve (n=10); PBS (n=15); S Ptn (n=15); Alum + Poly(I:C) (n=11); S Ptn + Poly(I:C) (n=15); S Ptn + Alum (n=15); S Ptn + Poly(I:C) + Alum (n=20). * - represents differences between S Ptn and S Ptn + Poly(I:C) groups; + - represents differences between S Ptn and S Ptn + Alum groups; § - represents differences between S Ptn and S Ptn + Poly(I:C) + Alum groups. (A, C) was performed two-way ANOVA followed by Bonferroni post-test and (B, D) was analyzed by one-way ANOVA with Tukey’s post hoc test. ****p<0.0001.
Since the first interaction between the host and SARS-CoV-2 happens in the lungs, we challenged mice intranasally with inactivated SARS-CoV-2 13 days after three immunizations. Twenty-four hours after the viral challenge we euthanized mice and evaluated the antibody levels in the BALF. Our results showed that S Ptn + Poly(I:C), S Ptn + Alum, and S Ptn + Poly(I:C) + Alum formulations induced higher IgG levels in the BALF (Figures 2A, B). There was no increase in BALF IgA levels between the groups (Figures 2C, D), indicating no specific IgA response. Furthermore, we observed very low levels of detectable serum IgA even after two and three immunizations in all groups (Supplementary Figures 1B, C, respectively).
Figure 2 Formulations containing adjuvants induce a strong IgG, but not IgA, response in the BALF. Antigen-specific antibody levels in the BALF were determined by ELISA and normalized using the control groups. Twenty-four hours after the viral challenge we euthanized mice and evaluated in the BALF the IgG (A, B) and IgA levels (C, D) were determined. The summatory of all dilutions are represented in (B, D). Data in this figure consists 4 independent experiments normalized using the control groups and shown as mean ± S.D. Groups: Naïve (n=10); PBS (n=15); S Ptn (n=15); Alum + Poly(I:C) (n=11); S Ptn + Poly(I:C) (n=15); S Ptn + Alum (n=15); S Ptn + Poly(I:C) + Alum (n=20). # - represents differences between PBS and S Ptn groups; @ - represents differences between S Ptn and Alum + Poly(I:C) groups; * - represents differences between S Ptn and S Ptn + Poly(I:C) groups; + - represents differences between S Ptn and S Ptn + Alum groups; § - represents differences between S Ptn and S Ptn + Poly(I:C) + Alum groups; $ - represents differences between S Ptn + Poly(I:C) and S Ptn + Poly(I:C) + Alum groups; & - represents differences between S Ptn + Poly(I:C) and S Ptn + Alum groups; ‘ - represents differences between S Ptn + Alum and S Ptn + Poly(I:C) + Alum groups; ♪ - represents differences between Naïve and S Ptn groups. (A, C) was performed two-way ANOVA followed by Bonferroni post-test and (B, D) was analyzed by one-way ANOVA with Tukey’s post hoc test. *p<0.05, ****p<0.0001.
In order to check whether the lack of a specific antibody response in the BALF was due to the immunization route, we performed an intranasal immunization. This route appeared to efficiently increase the amount of IgA (Supplementary Figure 1D) and IgG (Supplementary Figure 1E) in the groups vaccinated with S Ptn + Poly(I:C) in comparison to S Ptn.
The presence of IgG1 is usually related to a type 2 immune response while IgG2a is related to a type 1 response. To understand which response was being induced by the immunizations, we evaluated these IgG subtype (IgG1 and IgG2a) levels in the serum. We found that mice immunized with S Ptn + Poly(I:C), S Ptn + Alum, or S Ptn + Poly(I:C) + Alum produced higher levels of IgG1 compared to mice immunized with S Ptn alone after two (Figures 3A, B) and three immunizations (Figures 3C, D). Moreover, the S Ptn group presented higher levels of IgG1 than the PBS and Alum + Poly(I:C) groups after the two (Figures 3A, B) and three immunizations (Figures 3C, D), but there were no differences in the levels of IgG2a between those groups after either immunizations (Figures 3E, G, H). Interestingly, after only two immunizations, the S Ptn group presented a lower O.D. Sum level than the Alum + Poly(I:C) group (Figure 3F). S Ptn + Poly(I:C) and S Ptn + Poly(I:C) + Alum groups presented higher levels of IgG2a than the S Ptn and S Ptn + Alum groups after two (Figures 3E, F) and three immunizations (Figures 3G, H). Furthermore, mice immunized with S Ptn + Alum had a higher level of O.D. Sum than the S Ptn group after two immunizations (Figure 3F).
Figure 3 Formulations containing Poly(I:C) were able to induce type 1 response serum antibodies. Antigen-specific antibody levels were determined by ELISA and normalized using the control groups. Serum IgG1 (A–D) and IgG2a (E–H) levels were evaluated after two (A, B, E, F) or three immunizations (C, D, G, H). The summatory of all dilutions are represented in (B, D, F, H). Data in this figure consists 4 independent experiments normalized using the control groups and shown as mean ± S.D. Groups: PBS (n=10); S Ptn (n=15); Alum + Poly(I:C) (n=11); S Ptn + Poly(I:C) (n=15); S Ptn + Alum (n=15); S Ptn + Poly(I:C) + Alum (n=20). # - represents differences between PBS and S Ptn groups; @ - represents differences between S Ptn and Alum + Poly(I:C) groups; * - represents differences between S Ptn and S Ptn + Poly(I:C) groups; + - represents differences between S Ptn and S Ptn + Alum groups; § - represents differences between S Ptn and S Ptn + Poly(I:C) + Alum groups; $ - represents differences between S Ptn + Poly(I:C) and S Ptn + Poly(I:C) + Alum groups; & - represents differences between S Ptn + Poly(I:C) and S Ptn + Alum groups; ‘ - represents differences between S Ptn + Alum and S Ptn + Poly(I:C) + Alum groups. (A, C, E, G) was performed two-way ANOVA followed by Bonferroni post-test and (B, D, F, H) was analyzed by one-way ANOVA with Tukey’s post hoc test. *p<0.05, ****p<0.0001.
Together, these data suggest that all adjuvant-containing formulations induce IgG in the serum, but the ones containing Poly(I:C) are able to induce a strong type 1 antibody response against SARS-CoV-2 spike protein, while the ones containing Alum were able to induce slightly higher IgG levels in the BALF (Figure 2B). Immunization with S Ptn alone generated more type2 antibodies in the serum than the groups containing adjuvants (Figure 3).
We assessed the in vitro neutralizing activity against SARS-CoV-2 in mouse sera collected one week after two and three immunizations (days 21 after first immunization) (Figure 4). We did not observe neutralizing antibodies in the sera of mice immunized with S Ptn, as measured by the neutralizing titers of PRNT50 and PRNT90. Similarly, naïve and PBS-receiving mice were not able to induce neutralizing antibodies. However, mice immunized with S Ptn associated with the adjuvants Poly(I:C), Alum, or Poly(I:C) + Alum were capable of inducing neutralizing antibodies. The average neutralizing titers of the formulations containing S Ptn + Poly(I:C) + Alum (PRNT50 titer of 512 and PRNT90 titer of 230.4) were higher than that of S Ptn + Alum (PRNT50 titer of 204.8 and PRNT90 titer of 96) and S Ptn + Poly(I:C) (PRNT50 titer of 108.5 and PRNT90 titer of 32). Mice immunized with the adjuvants Poly(I:C) + Alum without S Ptn weren’t able to induce neutralizing antibodies. Moreover, the average of S Ptn + Alum it was superior to the S Ptn + Poly(I:C). These data show that two immunizations are already enough to trigger neutralizing antibodies when S Ptn is combined with the adjuvants Poly(I:C) or Alum, although the mixture of Poly(I:C) + Alum was more efficient.
Figure 4 Spike protein associated to Poly(I:C) plus Alum co-administrated induced high titers of neutralizing antibodies. Titers of neutralizing antibodies were determined in vitro by neutralization assay after two and three immunizations. PRNT50 (A, C) and PRNT90 (B, D) for mice plasma collected 7 days after two and three immunization. Figure representative of 4 independent experiments and shown as mean ± S.D. and analyzed by one-way ANOVA with Tukey’s post hoc test. *p<0.05, ***p<0.001, ****p<0.0001.
Afterwards, we decided to analyze the neutralizing titers then three immunizations. It was observed that naïve, PBS-receiving mice and mice immunized with the adjuvants Poly(I:C) + Alum without S Ptn weren’t able to induce neutralizing antibodies.
However, mice immunized with S Ptn associated with the adjuvants Poly(I:C), Alum, or Poly(I:C) + Alum were capable of inducing neutralizing antibodies. The average neutralizing titers of the formulations containing S Ptn + Poly(I:C) + Alum (PRNT50 titer of 3600) were higher than that of S Ptn + Alum (PRNT50 titer of 1600) and S Ptn + Poly(I:C) (PRNT50 titer of 576). Moreover, the average of S Ptn + Alum it was superior to the S Ptn + Poly(I:C). Regarding to neutralizing titers with PRNT90, the average neutralizing titers of the formulations containing S Ptn + Poly(I:C) + Alum (PRNT90 titer of 1102) were higher than that of S Ptn + Poly(I:C) (PRNT90 titer of 256) and S Ptn + Alum (PRNT90 titer of 456).
Taken together, our data show that two immunizations with S Ptn plus Poly(I:C), Alum, or a mixture of Poly(I:C) + Alum is enough to induce neutralizing antibodies, although the mixture presented the highest titers. Moreover, neutralizing titers were higher with three immunizations than those of only two immunizations, therefore having greater potential as a strategy to trigger neutralizing antibodies against SARS-CoV-2.
Besides, we evaluated in vitro neutralizing activity against SARS-CoV-2 Delta variant in mouse sera collected one week after three immunizations. Mice immunized with S Ptn + Poly(I:C) + Alum (PRNT50 titer of 588,8 and PRNT90 titer of 473,6); was capable of inducing more neutralizing antibodies than mice that received S Ptn + Alum (PRNT50 titer of 83,2 and PRNT90 titer of 41,6)and no difference in comparison of S Ptn + Poly(I:C) (PRNT50 titer of 326,4 and PRNT90 titer of 217,6) (Supplementary Figure 6) indicating the capacity of the formulation to produce high titers of neutralizing antibodies against variants of concern.
We performed the analysis of lymph node cells draining from the immunization site then three immnizations of the groups that received S Ptn alone or S Ptn together with adjuvants. Our results showed that the group that received S Ptn + Poly(I:C) + Alum had an increase in the number of total cells when compared to the group that received only S Ptn (Figure 5A; Supplementary Figure 2). Next, we evaluated the response profile of B cells and observed that there were no differences in the frequency and number of cells between the groups studied (Figures 5B, C; Supplementary Figure 3). Within the germinal center, there was an increase both in the percentage and number of cells that were CD38-GL7+ in the group that received S Ptn + Poly(I:C) + Alum, when compared to the other groups (Figures 5D, E). In this cell population, there was an increase in the frequency of RBD+S Ptn+ cells in the group that received S Ptn + Alum, when compared to the group that received S Ptn + Poly(I:C) and the control groups (Figure 5F). However, we saw that there was a greater number of these cells in the group that received S Ptn + Poly(I:C) + Alum, when compared either to the control groups, the group that received only S Ptn, and the group that received S Ptn + Poly(I:C) (Figure 5G). We also evaluated cells that were RBD-S Ptn+ and did not observe differences between the groups (Figures 5H, I).
Figure 5 B cell response within the germinal center after immunization. Lymphocytes from the draining popliteal lymph node were analyzed after three immunizations intradermal immunization with S protein alone or with adjuvants [Poly(I:C); Alum; Poly(I:C) + Alum]. Controls were performed with PBS or Poly(I:C) + Alum. (A) Number of total cells. (B) Percentage of B220+ cells. (C) Number of B220+ cells. (D) Percentage of CD38+GL7- cells. (E) Number of CD38+GL7- cells. (F) Percentage of RBD+S Ptn+ cells. (G) Number of RBD+S Ptn+ cells. (H) Percentage of RBD-S Ptn+ cells. (I) Number of RBD-S Ptn+ cells. Figure representative of 4 independent experiments and shown as mean ± S.D. and was performed by one-way ANOVA with Tukey’s post hoc test. *p<0.03, **p<0.005, ***p<0.0002, ****p<0.0001. (SEM; n=4-13).
We also continued with the analysis of cells outside the germinal center and noticed that there was a reduction in the frequency of CD38+GL7- cells in the group that received S Ptn + Poly(I:C) + Alum in relation to the other groups (Figure 6A). The same was not observed for the number of cells though (Figure 6B). In this cell population, we also observed that the S Ptn + Alum group had an increase in the percentage and number of RBD+S Ptn+ cells when compared to the other groups (Figures 6C, D).
Figure 6 B cell profile outside the germinal center after immunization. After three immunizations, lymphocytes from the draining popliteal lymph node macerated after intradermal immunization with S protein alone or with adjuvants [Poly(I:C); Alum; Poly(I:C) + Alum]. Controls were performed with PBS or Poly(I:C) + Alum. (A) Percentage of CD38+GL7- cells. (B) Number of CD38+GL7- cells. (C) Percentage of RBD+S Ptn+ cells. (D) Number of RBD+S Ptn+ cells. Figure representative of 4 independent experiments and shown as mean ± S.D. and was performed by one-way ANOVA with Tukey’s post hoc test. *p<0.05, **p<0.005, ***p<0.0005. (SEM; n=4-13).
The T cell response was observed for the groups that received S Ptn + Poly(I:C) and S Ptn + Poly(I:C) + Alum and the control group was performed with PBS. In relation to CD4+ T cells, our data revealed that there was no difference in the frequency and the number of these cells between the groups (Supplementary Figures 4A, B). We also observed the production of IFNγ by these cells and there were no differences regarding the frequency and number of these cells (Supplementary Figures 4C, D). Next, we analyzed CD8+ T cells and the IFNγ production by these cells. Our results showed no differences regarding the frequency and the number of these cells between groups (Supplementary Figures 4E–H).
In order to better understand the inflammatory cellular infiltration in the BALF of mice immunized with the different vaccine formulations based on SARS-CoV-2 S Ptn, we performed flow cytometry to distinguish alveolar macrophages (AMs) (SiglecF+CD11c+), neutrophils (SiglecF-CD11b+Ly6G+), and T cells (SiglecF-CD11b-TCRβ+), 24 hours after challenge with inactivated SARS-CoV-2 (Supplementary Figure 5). We found a pronounced decrease in the percentage (Figure 7A) and absolute numbers (Figure 7B) of AMs in the BALF from non-immunized (PBS) and S Ptn-immunized mice with different combination of adjuvants compared to naïve mice (non-immunized and non-challenged).
Figure 7 Analysis of BALF immune cell content in mice immunized with S Ptn together with different adjuvants following challenge with inactivated SARS-CoV-2 virus. BALF cells were collected 24 hours after inactivated SARS-CoV-2 (iSARS-CoV-2) virus challenge. The percentage and number of AMs, neutrophils, and T cells from naïve and mice immunized with S Ptn and different adjuvant combinations as gated on flow cytometry plots using markers including SiglecF, CD11c, CD11b, Ly6G, Ly6C, and TCRβ. Percentage of (A) AMs (CD11b+CD11c-Ly6chiF4/80+), (C) Neutrophils (CD11b+CD11c-Ly6c+F4/80-), and (E) T cells (CD11b+CD11c-Ly6c+F4/80-) in the BALFs. Absolute numbers of (B) AMs, (D) Neutrophils, and (F) T cells in the BALFs. Data are presented as the mean ± SEM of three pooled experiments and analyzed by one-way ANOVA with Tukey’s post hoc test, *p<0.05, **p<0.01, ***p<0.001, ****p<0.0001.
COVID-19 severity is marked by neutrophil and T cell imbalance in blood samples of patients (36, 37). In addition, elevated numbers of neutrophils are observed in the nasopharyngeal epithelium and later in the more distal parts of the lungs upon SARS-CoV-2 infection (38, 39). In our model, we found a higher percentage of neutrophils (Figure 7C) and lower percentage of T cells (Figure 7E) in the BALF of mice immunized with S Ptn together with the different combinations of adjuvants compared to naïve mice. Interestingly, we also observed that injection itself with either PBS or S Ptn alone increased the percentage of neutrophils and decreased the percentage of T cells in the BALF of challenged mice compared to the percentages found in naïve mice (Figures 7C, E). On the other hand, the absolute numbers of neutrophils were quite variable among non-immunized and S Ptn-immunized mice. Specifically, we found that S Ptn alone and S Ptn + Poly(I:C) immunization induced an enhancement in the numbers of neutrophils when compared to non-immunized BALF of SARS-CoV-2 challenged mice. In addition, S Ptn without adjuvant increased the numbers of neutrophils when compared to the S Ptn + Poly(I:C) + Alum group. Finally, S Ptn + Poly(I:C) induced an enhancement in the numbers of neutrophils when compared to the S Ptn + Alum and S Ptn + Poly(I:C) + Alum groups (Figure 7D). No statistically significant increases were found for the absolute numbers of T cells among groups of non-immunized and S Ptn-immunized mice with different combinations of adjuvants. However, we found a tendency of increasing numbers of T cells in mice immunized with S Ptn together with different combinations of adjuvants compared to naïve and non-immunized mice (PBS) (Figure 7F).
The ID route has recently been shown to be an optimal immunization strategy against SARS-CoV-2 due to its ability to stimulate Antigen Presenting Cells (APCs), such as Dendritic cells (DCs) and macrophages, in the dermis, which presents high vascularization, facilitating the migration of cells to secondary lymphoid organs for T and B cell activation (40). One of the main advantages of the ID route is that, due to the presence of a large number of APCs, it allows the administration of a lower dose of antigens and adjuvants is required to generate immune responses, unlike those necessary for the IM and SC routes (41–43).
To this day, there have been two vaccines approved for use in humans against SARS-CoV-2 that use the adjuvant Alum in the formulation together with purified virus inactivated by β-propiolactone, PiCoVacc (44) and BBIBP-CorV (45). Zhang et al. (44) observed that various doses of PiCoVacc mixed with Alum (0, 1.5, 3, or 6 μg per dose) in BALB/c mice induced neutralizing antibodies against S Ptn after two immunizations by the IM route (7, 14, 21, 28, and 35 days). The animals immunized with Alum only did not induce neutralizing antibodies. In the experimental trials of BBIBP-CorV, Wang et al. (46) evaluated the immunization of 0.5 mL of vaccine (2, 4, or 8 μg per total dose) containing Alum (0.45 mg/mL) following the protocol with one-dose (D0), two-dose (D0/D21), and three-dose (D0/D7/D14) in BALB/c mice administered intraperitoneally. They showed that 28 days after the first immunization the three-dose immunization program led to higher levels of neutralizing antibodies than both the one- and two-dose protocols. In addition, they demonstrated that the two-dose immunization protocol was also able to induce neutralizing antibodies at 7 days after the second immunization (day 21). Our data demonstrated similar results, showing that the second immunization via the ID route with S Ptn associated to Alum, Poly(I:C), and Alum plus Poly(I:C) is already sufficient to induce neutralizing antibodies and three immunizations are capable of inducing even more mainly for the combination with Alum plus Poly(I:C).
We also tested the capacity of mice immunized with S Ptn from Wuhan combined with adjuvants to induce neutralizing antibodies against Delta variant. In our analysis, as we observed antibodies to the regions within and outside the RBD, we were able to verify the neutralization capacity for both regions. Therefore, even if modifications occur in the RBD, giving rise to variants, we can analyze the neutralization in regions that are outside the RBD. Overall, a reduction in the ability to induce neutralizing antibodies against the Delta variant is observed in SARS-CoV-2 vaccine (47). We also observed a reduction of at least 1/3 of the neutralizing capacity in the Wuhan virus assay compared to the Delta variant on mice that received S Ptn + Alum + Poly(I:C). But it is worth mentioning that are titles of considerable importance.
Although Alum is acknowledged for its ability to induce a Th2 response (48), many studies regarding SARS-CoV-2 vaccine development have reported a Th1 response directed by Alum (49–51), which was associated to the TLR9 adjuvant CpG + Alum coupled activity in some cases (52–54). Also, trimeric RBD adjuvanted with Alum adsorbed 3M-052 (TLR7/8 agonist) induced high neutralizing antibodies and protection in mice (55). Formulation using AS03 or CPG plus Alum as adjuvants were safe and immunogenic in humans (56). These results together demonstrated the potential of combination usage of adjuvants with Alum. Other platforms using scalene based adjuvants as AddaVax have been used and also demonstrated potential against SARS-Cov-2 ((57, 58), when comparing Alum versus AddaVax, the results for AddaVax were superior (57) indicating the necessity the use of Alum associated to TLR agonist.
Poly(I:C) is a TLR3 agonist and formulations of mixed adjuvant containing Alum + Poly(I:C) have been shown to elicit a T cell immune response. This response was observed by the increase of antigen-specific IgG1 and IgG2a levels, related as well to maturation and activation of dendritic cells. The same study observed a similar response for a formulation of Alum + CpG (59). Chuai et al (60 also demonstrated that immunization of mice with Alum + Poly(I:C) formulation in conjunction with the S protein from Hepatitis B virus induced increased levels of IgG, as well as IFN-γ, and IL-2, which are related to a Th1 immune response. Along with that, researchers have studied a derivative of Poly(I:C), poly-ICLC (Hiltonol), which is already being tested in clinical trials (NCT04672291), and has shown to be efficient in protecting BALB/c mice in a lethal SARS-CoV infection model (61). Moreover, Smith et al. (62) demonstrated that immunization of BALB/c mice with synthesized vaccine peptides was capable of inducing IFN-γ release in response to predicted T cell epitopes in mice vaccinated with peptides + Poly(I:C) rather than Poly(I:C) alone.
We therefore suggest our formulation of S Ptn + Alum + Poly(I:C) is capable of stimulating Pattern Recognition Receptors, leading to activation of the innate immune response and an antiviral response, such as induced IFN-γ production and increased T cell activation. An adaptive immune response was also observed, with high production of antigen-specific IgG, as well as higher levels of GC B cells specific for both RBD and S Ptn. Our formulation of S Ptn + Alum + Poly(I:C) was capable of driving a sustained type 1 immune response, with the production IFN-γ and IgG2a, as well as inducing higher levels of type 2 immune response antibodies like IgG1 compared to the group immunized with S Ptn alone.
Nanishi et al. (63) analyzed an immunization strategy against SARS containing Alum mixed with other adjuvants by the IM route in BALB/c mice. They noted that the formulation containing Alum (100 μg) + Poly(I:C) (50 μg) was able to induce more neutralizing antibodies than a formulation containing either only Poly(I:C) or no adjuvants at 28 days after immunization in old and young mice. This response was still observed at 210 days post-immunization with the formulation containing Alum + Poly(I:C). The use of Alum (50 μg) and Poly(I:C) (50 μg) has also been proven to induce neutralizing antibodies against MERS-CoV (29).
It was demonstrated by Lederer K, et al. (64) that after mRNA immunization in humans induced B cells that recognize Spike+ RBD+ (at the same time) and Spike+ RBD- in the germinal centers of the draining lymph nodes. We also observed after immunization using spike proteins associated to adjuvants Alum, or Poly(I:C) or the combination with alum:Poly(I:C) high levels of B cells in GC that recognize Spike+ RBD- and double positives Spike+ RBD+ were found. We also observed that B cells outside GC can recognize Spike+ RBD+ and Spike+ RBD-, however, with fewer recognition in comparison to cells from GC. Taken together, these data may lead to the implication that reactions on the GC are highly required for the formation of neutralizing antibodies. However, more studies to evaluate the importance of somatic hypermutation to generate high-affinity GC B cell clones are required to confirm the role of GC to formation of neutralizing antibodies.
Understanding the efficacy of a vaccine also requires a comprehensive assessment of cellular events that occur after its administration. The literature on COVID-19 vaccine efficacy is focused on the important parameters of antibody production and T cell activation. However, one of the hallmarks of COVID-19 severity is the elevated numbers of neutrophils in the blood samples (36, 37) as well as in the nasopharyngeal epithelium and later in the more distal parts of the lungs upon SARS-CoV-2 infection (37, 38). In addition, a recent study reinforced the importance of reducing neutrophil recruitment to the lungs to prevent severe forms of COVID-19 (65). Here, we found a higher recruitment of neutrophils to the lungs of S Ptn-immunized mice following SARS-CoV-2 challenge. However, when S Ptn was combined with Poly(I:C) + Alum adjuvants, we found lower numbers of neutrophils in the BAL of these immunized mice compared to all the adjuvants combination tested. Based on the reduction of neutrophils we wonder the possibility of the impact of vaccination using the combination of S Ptn + Poly(I:C) + Alum could reduce inflammatory response caused by neutrophils, which may represent an advantage in preventing the worsening of lung damage due to inactivated or live SARS-CoV-2 challenge. The use of inactivated virus is a limitation in our study, futures experiments using challenge with SARS-CoV-2 can confirm this hypothesis.
Alveolar macrophages are also involved with the severity of SARS-CoV-2 infection (66). We decided to investigate whether these cells would be altered and we found a pronounced decrease in the percentage and absolute numbers. AMs are very sensitive to respiratory microorganisms (67, 68), and their decreasing could be explained by induction of their cell death following the challenge with inactivated virus. Besides, we did not find an increasing of eosinophils and monocytes with the gate strategy applied in these studies.
In our study, we begin the evaluation of the immunogenicity of Spike protein associated with Alum plus Poly(I:C) co-administrated by intradermal route in mice model. More studies in other models as hamster and non-human primates and also clinical studies are necessaries to prove the efficacy of this formulation. Taking these data together, we suggest our formulation of S Ptn + Alum + Poly(I:C) is capable of inducing neutralizing antibodies against SARS-CoV-2 at rates higher than formulations with single adjuvant or no adjuvants at 21 days and 35 days after the two and three immunizations, respectively. The combination of both Alum and Poly(I:C) adjuvants together with S Ptn as antigen are good candidates for a vaccine against COVID-19.
The raw data supporting the conclusions of this article will be made available by the authors, without undue reservation.
The animal study was reviewed and approved by CEUA UFRJ.
HLMG conception of study. HLMG, ACO, AMO, ADF and JLS designed the study. JSS, LFC, AMFM, DOM, GGP, VARP, ADF and HLMG wrote the manuscript. JSS, LFC, AMFM, DOM, GGP, VARP and CHR performed the experiments. Assistance in experiments: FHGS, ACVS, MSL, JRMF, KGP and BRB. JSS, LFC, AMFM and ADF analyzed the data. JSS and DOM – Immunization and Challenge. JSS, LFC and AMFM - Flow cytometry. LFC - ELISA. ADF, MSL, JRMF and KGF - BALF. LC, DASR, MVMS, OF, RSMB and AMO - S Pnt labeling, RBD. RGA, TML, FFM and DPA - S Ptn production. Final review of the text by HLMG, JLS, ACO, AMO and ADF. Scientific discussion performed by JSS, LFC, AMFM, DOM, GGP, JLS, ACO, AMO, ADF, AMV, BRB and HLMG. All authors contributed tothe article and approved the submitted version.
This work was supported by CNPq: PQ-2 (308012/2019-4), CAPES: Finance code 001, FAPERJ: JCNE (E-26/202.674/2018) and (E-26/210.237/2020 (258135).
The authors declare that the research was conducted in the absence of any commercial or financial relationships that could be construed as a potential conflict of interest.
All claims expressed in this article are solely those of the authors and do not necessarily represent those of their affiliated organizations, or those of the publisher, the editors and the reviewers. Any product that may be evaluated in this article, or claim that may be made by its manufacturer, is not guaranteed or endorsed by the publisher.
We thank Professor Leda Castilho from COPPE/UFRJ to donate Spike protein for this study. We thank Dr. Jon Park (Chan Zuckerberg Initiative Biohub) for kindly donation of RBD. We also thank Serrapilheira, FAPERJ and CNPq for financial support.
The Supplementary Material for this article can be found online at: https://www.frontiersin.org/articles/10.3389/fimmu.2022.884760/full#supplementary-material
Supplementary Figure 1 | The prime was not a good inducer of IgG, and IgA levels were very low after immunizations. Antigen-specific antibody levels were determined by ELISA and normalized using the control groups. Serum IgG levels (A) was evaluated 1 week after prime. Serum IgA levels (B, C) were evaluated after two (B) or three immunizations (C). BALF IgA (D) and IgG (E) levels. Data in (A–C) are representative of 4 independent experiments and are shown as mean ± S.D. Data in (D, E) are from a single experiment and are shown as mean ± S.D. Groups: PBS (n=5); S Ptn (n=5); Alum + Poly(I:C) (n=6); S Ptn + Poly(I:C) (n=5); S Ptn + Alum (n=5); S Ptn + Poly(I:C) + Alum (n=5). * - represents differences between S Ptn + Poly(I:C) (intradermal) and S Ptn + Poly(I:C) (intranasal) groups (D, E) and analyzed by two-way ANOVA followed by Bonferroni post-test and (F) was analyzed by one-way ANOVA with Tukey’s post hoc test. *p<0.05, **p<0.01, ***p<0.001, ****p<0.0001.
Supplementary Figure 2 | Gating strategy of B cells in draining lymph nodes. Lymphocytes from the draining popliteal lymph node were analyzed after three immunizations as follows: (A) Cells FSC x SSC. (B) Single cells - FSC-A x FSC-H. (C) B220+ (PerCP x FSC-A). (D) CD38-GL7+ (PE-Cy7 x PE). (E, F) RBD+S Ptn+ (FITC x APC). (G) FMO GL7. (H) FMO RBD. (I) FMO S Ptn.
Supplementary Figure 3 | Dot plot of B220+ cells. Lymphocytes isolated from the draining popliteal lymph node after three immunizations by intradermal immunization with S Ptn alone or with adjuvants (Poly(I:C); Alum; Poly(I:C) + Alum). Controls were performed with PBS or Poly(I:C) + Alum. Dot plot of B220+ cells (PerCP x FSC-A).
Supplementary Figure 4 | Profile of CD4+ and CD8+ T cell response after immunization. Lymphocytes isolated from the draining popliteal lymph node after three immunizations by intradermal immunization with S Ptn plus Poly(I:C) or Poly(I:C) + Alum. Controls were performed with PBS. (A) Percentage of CD4+ T cells. (B) Number of CD4+ T cells. (C) Percentage of IFN-γ+CD4+ T cells. (D) Number of IFN-γ+CD4+ T cells. (E) Percentage of CD8+ T cells. (F) Number of CD8+ T cells. (G) Percentage of IFN-γ+CD8+ T cells. (H) Number of IFN-γ+CD8+ T cells. (SEM; n=5-15).
Supplementary Figure 5 | Gating strategy for the flow cytometric detection of macrophages, eosinophils, T cells, and neutrophils in BALF. BALF cells were first gated on a forward scatter/side scatter (FSC-A/SSC-A) and then on single cells using FSC-A/FSC-H. A sequential gating strategy was used to identify cellular populations expressing specific markers: alveolar macrophages (AMs) (SiglecF+CD11c+), eosinophils (SiglecF+CD11c-), T cells (CD11b-TCRβ+), and neutrophils (CD11b+Ly6C-Ly6G+).
Supplementary Figure 6 | Spike protein + Poly(I:C) + Alum and Spike protein + Poly(I:C) induced high titers of neutralizing antibodies against Delta variant. Titers of neutralizing antibodies were determined in vitro by neutralization assay after three immunizations and PRNT50 and PRNT90 for mice plasma collected 7 days after third immunization. Figure representative of 2 experiments and shown as mean ± S.D. and analyzed by one-way ANOVA with Tukey’s post hoc test. *p<0.05 (SD; n=5-10).
Supplementary Figure 7 | Protocol of Immunization. The animals received three immunizations, with the same dosage, with an interval of fourteen days between each dose. Blood samples was collected after two and three immunizations. Then, mice were challenge with inactivated SARS-CoV-2 and 24h later the mice were euthanized.
1. Carter D, van Hoeven N, Baldwin S, Levin Y, Kochba E, Magill A, et al. The Adjuvant GLA-AF Enhances Human Intradermal Vaccine Responses. Sci Adv (2018) 4(9):eaas9930. doi: 10.1126/sciadv.aas9930
2. Tebas P, Kimberly AK, Ami P, Joel NM, Matthew PM, Albert JS, et al. Intradermal Syncon® Ebola GP DNA Vaccine Is Temperature Stable And Safely Demonstrates Cellular And Humoral Immunogenicity Advantages In Healthy Volunteers. J Of Infect Dis (2019) 220(3):400–10. doi: 10.1093/infdis/jiz132
3. Frey SE, Anna W, Srilatha E, Lisa AJ, Jack TS, Hana ES, et al. Comparison Of Lyophilized Versus Liquid Modified Vaccinia Ankara (Mva) Formulations and Subcutaneous Versus Intradermal Routes Of Administration In Healthy Vaccinia-Naïve Subjects”. Vaccine (2015) 33(39):5225–34. doi: 10.1016/j.vaccine.2015.06.075
4. Tebas P, ShuPing Y, Jean DB, Emma LR, Ami P, Aaron CQ, et al. Safety and Immunogenicity of INO-4800 DNA Vaccine Against SARS-Cov-2: A Preliminary Report of an Open-Label, Phase 1 Clinical Trial. EClinicalMedicine (2021) 31:100689. doi: 10.1016/j.eclinm.2020.100689
5. Zeng F, Chow KY, Hon CC, Law KM, Yip CW, Chan KH, et al. Characterization of Humoral Responses in Mice Immunized With Plasmid DNAs Encoding SARS-CoV Spike Gene Fragments. Biochem Biophys Res Commun (2004) 315(4):1134–9. doi: 10.1016/j.bbrc.2004.01.166
6. Zakhartchouk AN, Viswanathan S, Moshynskyy I, Petric M, Babiuk LA. Optimization of a DNA Vaccine Against SARS. DNA Cell Biol (2007) 26(10):721–6. doi: 10.1089/dna.2007.0616
7. Kim HJ, Kwak HW, Kang KW, Bang YJ, Lee YS, Park HJ, et al. MERS-Cov Spike Protein Vaccine and Inactivated Influenza Vaccine Formulated With Single Strand RNA Adjuvant Induce T-Cell Activation Through Intranasal Immunization in Mice. Pharmaceutics (2020) 12(5):441. doi: 10.3390/pharmaceutics12050441
8. Taufik M, Kevinkumar K, Hardik P, Sunil S, Bhumika S, Jatin P, et al. Safety and Immunogenicity of a DNA Sars-CoV-2 Vaccine (Zycov-D): Results of an Open-Label, Non-Randomized Phase I Part of Phase I/II Clinical Study by Intradermal Route in Healthy Subjects in India. EClinicalMedicine (2021) 38:101020. doi: 10.1016/j.eclinm.2021.101020
9. Smith TRF, Patel A, Ramos S, Elwood D, Zhu X, Yan J, et al. Immunogenicity of a DNA Vaccine Candidate for COVID-19. Nat Commun (2020) 11(1):2601. doi: 10.1038/s41467-020-16505-0
10. Tai W, Zhang X, Drelich A, Shi J, Hsu JC, Luchsinger L, et al. A Novel Receptor-Binding Domain (RBD)-Based mRNA Vaccine Against SARS-Cov-2. Cell Res (2020) 30(10):932–35. doi: 10.1038/s41422-020-0387-5
11. Patel A, Walters J, Reuschel EL, Schultheis K, Parzych E, Gary EN, et al. Intradermal-Delivered DNA Vaccine Induces Durable Immunity Mediating a Reduction in Viral Load in a Rhesus Macaque SARS-CoV-2 Challenge Model. Cell Rep Med (2021) 2(10):100420. doi: 10.1016/j.xcrm.2021.100420
12. Kitabatake M, Inoue S, Yasui F, Yokochi S, Arai M, Morita K, et al. Sars-CoV Spike Protein-Expressing Recombinant Vaccinia Virus Efficiently Induces Neutralizing Antibodies in Rabbits Pre-Immunized With Vaccinia Virus. Vaccine (2007) 25(4):630–7. doi: 10.1016/j.vaccine.2006.08.039
13. Ganneru B, Jogdand H, Dharam VK, Molugu NR, Prasad SD, Vellimudu S, et al. TH-1 Skewed Immune Response of Whole Virion Inactivated SARS-CoV-2 Vaccine and Its Safety Evaluation. iScience (2021) 24(4):102298. doi: 10.1016/j.isci.2021.102298
14. Ita K. Transdermal Delivery of Vaccines - Recent Progress and Critical Issues. Biomed Pharmacother (2016) 83:1080–8. doi: 10.1016/j.biopha.2016.08.026
15. Pardi N, Hogan MJ, Naradikian MS, Parkhouse K, Cain DW, Jones L, et al. Nucleoside-modified mRNA Vaccines Induce Potent T Follicular Helper and Germinal Center B Cell Responses. J Exp Med (2018) 215(6):1571–88. doi: 10.1084/jem.20171450
16. Allen CDC, Okada T, Cyster JG. Germinal-Center Organization and Cellular Dynamics. Immunity (2007) 27:190–202. doi: 10.1016/j.immuni.2007.07.009
17. Mesin L, Ersching J, Victora GD. Germinal Center B Cell Dynamics. Immunity (2016) 45:471–82. doi: 10.1016/j.immuni.2016.09.001
18. Lederer K, Castaño D, Atria DG, Oguin TH, Wang S, Manzoni TB, et al. Sars-CoV-2 mRNA Vaccines Foster Potent Antigen-Specific Germinal Center Responses Associated With Neutralizing Antibody Generation. Immunity (2020) 53(6):1281–1295.e5. doi: 10.1016/j.immuni.2020.11.009
19. Vogel AB, Kanevsky I, Che Y, Swanson KA, Muik A, Vormehr M, et al. BNT162b Vaccines Protect Rhesus Macaques From SARS-Cov-2. Nature (2021) 592:283–9. doi: 10.1038/s41586-021-03275-y
20. Martins KA, Bavari S, Salazar AM. Vaccine Adjuvant Uses of poly-IC and Derivatives. Expert Rev Vaccines (2015) 14(3):447–59. doi: 10.1586/14760584.2015.966085
21. Chattopadhyay S, Sen GC. dsRNA-Activation of TLR3 and RLR Signaling: Gene Induction-Dependent and Independent Effects. J Interferon Cytokine Res (2014) 34:427–36. doi: 10.1089/jir.2014.0034
22. Stahl-Hennig C, Eisenblatter M, Jasny E, Rzehak T, Tenner-Racz K, Trumpfheller C, et al. Synthetic Double-Stranded RNAs Are Adjuvants for the Induction of T Helper 1 and Humoral Immune Responses to Human Papillomavirus in Rhesus Macaques. PloS Pathog (2009) 5(4):e1000373. doi: 10.1371/journal.ppat.1000373
23. Ichinohe T, Ainai A, Tashiro M, Sata T, Hasegawa H. PolyI: polyC12U Adjuvant-Combined Intranasal Vaccine Protects Mice Against Highly Pathogenic H5N1 Influenza Virus Variants. Vaccine (2009) 27:6276–9. doi: 10.1016/j.vaccine.2009.04.074
24. Gai WW, Zhang Y, Zhou DH, Chen YQ, Yang JY, Yan HM. PIKA Provides an Adjuvant Effect to Induce Strong Mucosal and Systemic Humoral Immunity Against SARS-Cov. Virol Sin (2011) 26(2):81–94. doi: 10.1007/s12250-011-3183-z
25. Hartman H, Wang Y, Schroeder HW Jr, Cui X. Absorbance Summation: A Novel Approach for Analyzing High-Throughput ELISA Data in the Absence of a Standard. PloS One (2018) 13(6):e0198528. doi: 10.1371/journal.pone.0198528
26. Zhao K, Wang H, Wu C. The Immune Responses of HLA-A*0201 Restricted SARS-Cov S Peptide-Specific CD8+ T Cells Are Augmented in Varying Degrees by CpG Odn, PolyI:C and R848. Vaccine (2011) 29(38):6670–8. doi: 10.1016/j.vaccine.2011.06.100
27. Agrawal AS, Tao X, Algaissi A, Garron T, Narayanan K, Peng BH, et al. Immunization With Inactivated Middle East Respiratory Syndrome Coronavirus Vaccine Leads to Lung Immunopathology on Challenge With Live Virus. Hum Vaccin Immunother (2016) 12(9):2351–6. doi: 10.1080/21645515.2016.1177688
28. Iwata-Yoshikawa N, Uda A, Suzuki T, Tsunetsugu-Yokota Y, Sato Y, Morikawa S, et al. Effects of Toll-like Receptor Stimulation on Eosinophilic Infiltration in Lungs of BALB/c Mice Immunized With UV-inactivated Severe Acute Respiratory Syndrome-Related Coronavirus Vaccine. J Virol (2014) 88(15):8597–614. doi: 10.1128/JVI.00983-14
29. Wang C, Zheng X, Gai W, Wong G, Wang H, Jin H, et al. Novel Chimeric Virus-Like Particles Vaccine Displaying MERS-CoV Receptor-Binding Domain Induce Specific Humoral and Cellular Immune Response in Mice. Antiviral Res (2017) 140:55–61. doi: 10.1016/j.antiviral.2016.12.019
30. Wrapp D, Wang N, Corbett KS, Goldsmith JA, Hsieh CL, Abiona O, et al. Cryo-EM Structure of the 2019-nCoV Spike in the Prefusion Conformation. Science (2020) 367:1260–3. doi: 10.1126/science.abb2507
31. Alvim RGF, Lima TM, Rodrigues DAS, Marsili FF, Bozza VBT, Higa LM, et al. Development and Large-Scale Validation of a Highly Accurate SARS-COV-2 Serological Test Using Regular Test Strips for Autonomous and Affordable Finger-Prick Sample Collection, Transportation, and Storage. (2021). doi: 10.21203/rs.3.rs-668316/v1
32. Cunha LER, Stolet AA, Strauch MA, Pereira VAR, Dumard CH, Gomes AMO. Polyclonal F(ab')2 Fragments of Equine Antibodies Raised Against the Spike Protein Neutralize SARS-CoV-2 Variants With High Potency. iScience (2021) 24(11):103315. doi: 10.1016/j.isci.2021.103315
33. Byrum JR, Waltari E, Janson O, Guo SM, Folkesson J, Chhun BB, et al. multiSero: Open multiplex-ELISA Platform for Analyzing Antibody Responses to SARS-CoV-2 Infection. medRxiv (2021). doi: 10.1101/2021.05.07.21249238
35. Bewley K. R., Coombes N. S., Gagnon L., McInroy L., Baker N., Shaik I., et al Quantification of SARS-CoV-2 Neutralizing Antibody by Wild-type Plaque Reduction Neutralization, Microneutralization and Pseudotyped Virus Neutralization Assays. Nat Protoc (2021) 16, 3114–40. doi: 10.1038/s41596-021-00536-y
36. Meizlish ML, Pine AB, Bishai JD, Goshua G, Nadelmann ER, Simonov M, et al. A Neutrophil Activation Signature Predicts Critical Illness and Mortality in COVID-19. Blood Adv (2021) 5(5):1164–77. doi: 10.1182/bloodadvances.2020003568
37. Lagunas-Rangel FA. Neutrophil-to-Lymphocyte Ratio and lymphocyte-to-C-reactive Protein Ratio in Patients With Severe Coronavirus Disease 2019 (COVID-19): A Meta-Analysis. J Med Virol (2020) 92:1733–4. doi: 10.1002/jmv.25819
38. Chua RL, Lukassen S, Trump S, Hennig BP, Wendisch D, Pott F, et al. Covid-19 Severity Correlates With Airway Epithelium-Immune Cell Interactions Identified by Single-Cell Analysis. Nat Biotechnol (2020) 38:970–9. doi: 10.1038/s41587-020-0602-4
39. Liao M, Liu Y, Yuan J, Wen Y, Xu G, Zhao J, et al. Single-Cell Landscape of Bronchoalveolar Immune Cells in Patients With COVID-19. Nat Med (2020) 26:842–4. doi: 10.1038/s41591-020-0901-9
40. Hettinga J, Carlisle R. Vaccination Into the Dermal Compartment: Techniques, Challenges, and Prospects. Vaccines (Basel) (2020) 8(3):534. doi: 10.3390/vaccines8030534
41. Schaumburg F, De Pijper CA, Grobusch MP. Intradermal Travel Vaccinations When Less Means More. Trav Med Infect Dis (2019) 28:3–5. doi: 10.1016/j.tmaid.2019.03.007
42. Programme for Appropriate Technology in Health and World Health Organization. Intradermal Delivery of Vaccines: A Review of the Literature and the Potential for Development for Use in Low-And Middle-Income Countries (2009). Available at: https://www.path.org/resources/intradermal-delivery-of-vaccines-a-review-of-the-literature-and-the-potential-for-development-for-use-in-low-and-middle-income-countries/ (Accessed September 22, 2021).
43. Migliore A, Gigliucci G, Di Marzo R, Russo D, Mammucari M. Intradermal Vaccination: A Potential Tool in the Battle Against the COVID-19 Pandemic? Risk Manage Healthc Policy (2021) 14:2079–87. doi: 10.2147/RMHP.S309707
44. Zhang Y, Zeng G, Pan H, Li PC, Hu Y, Chu K. Safety, Tolerability, and Immunogenicity of an Inactivated SARS-CoV-2 Vaccine in Healthy Adults Aged 18-59 Years: A Randomised, Double-Blind, Placebo-Controlled, Phase 1/2 Clinical Trial. Lancet Infect Dis (2020) 21(2):181–92. doi: 10.1016/S1473-3099(20)30843-4
45. Xia S, Zhang Y, Wang Y, Wang H, Yang Y, Gao PGF. Safety and Immunogenicity of an Inactivated SARS-CoV-2 Vaccine, BBIBP-CorV: A Randomised, Double-Blind, Placebo-Controlled, Phase 1/2 Trial. Lancet Infect Dis (2021) 21(1):39–51. doi: 10.1016/S1473-3099(20)30831-8
46. Wang H, Zhang Y, Huang B, Deng W, Quan Y, Wang W, et al. Development of an Inactivated Vaccine Candidate, Bbibp-CorV, With Potent Protection Against SARS-Cov-2. Cell (2020) 182(3):713–721.e9. doi: 10.1016/j.cell.2020.06.008
47. Planas D, Veyer D, Baidaliuk A, Staropoli I, Guivel-Benhassine F, Rajah MM, et al. Reduced Sensitivity of SARS-CoV-2 Variant Delta to Antibody Neutralization. Nature (2021) 596:276–80. doi: 10.1038/s41586-021-03777-9
48. Brewer JM, Conacher M, Hunter CA, Mohrs M, Brombacher F, Alexander J. Aluminium Hydroxide Adjuvant Initiates Strong Antigen-Specific Th2 Responses in the Absence of IL-4- or IL- 13-Mediated Signaling. J Immunol (1999) 163(12):6448–54.
49. Chen WH, Tao X, Agrawal A, Algaissi A, Peng BH, Pollet J, et al. Yeast-Expressed SARS-CoV Recombinant Receptor-Binding Domain (Rbd219-N1) Formulated With Aluminum Hydroxide Induces Protective Immunity and Reduces Immune Enhancement. Vaccine (2020) 38(47):7533–41. doi: 10.1016/j.vaccine.2020.09.061
50. An Y, Li S, Jin X, Han JB, Xu K, Han Y, et al. A Tandem-Repeat Dimeric RBD Protein-Based COVID-19 Vaccine ZF2001 Protects Mice and Nonhuman Primates. Emerg Microbes Infect (2022) 11(1):1058–71. doi: 10.1101/2021.03.11.434928
51. Yang J, Wang W, Chen Z, Lu S, Yang F, Bi Z, et al. A Vaccine Targeting the RBD of the S Protein of SARS-CoV-2 Induces Protective Immunity. Nature (2020) 586:572–7. doi: 10.1038/s41586-020-2599-8
52. Danmei S, Li X, He C, Huang X, Chen M, Wang Q, et al. Broad Neutralization Against SARS-CoV-2 Variants Induced by a Modified B.1.351 Protein-Based COVID-19 Vaccine Candidate. bioRxiv (2021). doi: 10.1101/2021.05.16.444369
53. Kuo TY, Lin MY, Coffman RL, Campbell JD, Traquina P, Lin YJ, et al. Development of CpG-adjuvanted Stable Prefusion SARS-CoV-2 Spike Antigen as a Subunit Vaccine Against COVID-19. Sci Rep (2020) 10:20085. doi: 10.1038/s41598-020-77077-z
54. Guirakhoo F, Kuo L, Peng J, Huang JH, Kuo B, Lin F, et al. A Novel SARS-Cov-2 Multitope Protein/Peptide Vaccine Candidate Is Highly Immunogenic and Prevents Lung Infection in an Adeno Associated Virus Human Angiotensin-Converting Enzyme 2 (AAV Hace2) Mouse Model. bioRxiv (2020). doi: 10.1101/2020.11.30.399154
55. Routhu NK, Cheedarla N, Bollimpelli VS, Gangadhara S, Edara VV, Lai L, et al. SARS-CoV-2 RBD Trimer Protein Adjuvanted With Alum-3M-052 Protects From SARS-CoV-2 Infection and Immune Pathology in the Lung. Nat Commun (2021) 12:3587. doi: 10.1038/s41467-021-23942-y
56. Richmond P, Hatchuel L, Dong M, Ma B, Hu B, Smolenov I, et al. Safety and Immunogenicity of S-Trimer (Scb-2019), a Protein Subunit Vaccine Candidate for COVID-19 in Healthy Adults: A Phase 1, Randomised, Double-Blind, Placebo-Controlled Trial. Lancet (2021) 397(10275):682–94. doi: 10.1016/S0140-6736(21)00241-5
57. Dai L, Zheng T, Xu K, Han Y, Xu L, Huang E, et al. A Universal Design of Betacoronavirus Vaccines Against COVID-19, MERS, and SARS. Cell (2020) 82(3):722–733.e11. doi: 10.1016/j.xinn.2021.100140
58. Yang L, Tian D, Han JB, Fan W, Zhang Y, Li Y, et al. A Recombinant Receptor-Binding Domain in Trimeric Form Generates Protective Immunity Against SARS-CoV-2 Infection in Nonhuman Primates. Innovation (2021) 2(3):100140. doi: 10.1016/j.xinn.2021.100140
59. Lu F, Mosley YC, Carmichael B, Brown DD, HogenEsch H. Formulation of Aluminum Hydroxide Adjuvant With TLR Agonists Poly(I:C) and CpG Enhances the Magnitude and Avidity of the Humoral Immune Response. Vaccine (2019) 37(14):1945–53. doi: 10.1016/j.vaccine.2019.02.033
60. Chuai X, Chen H, Wang W, Deng Y, Wen B, Ruan L, et al. Poly(I:C)/Alum Mixed Adjuvant Priming Enhances HBV Subunit Vaccine-Induced Immunity in Mice When Combined With Recombinant Adenoviral-Based HBV Vaccine Boosting. PloS One (2013) 8(1):e54126. doi: 10.1371/journal.pone.0054126
61. Kumaki Y, Salazar AM, Wandersee MK, Barnard DL. Prophylactic and Therapeutic Intranasal Administration With an Immunomodulator, Hiltonol ® (Poly IC: LC), in a Lethal SARS-CoV-infected BALB/C Mouse Model. Antiviral Res (2017) 139:1–12. doi: 10.1016/j.antiviral.2016.12.007
62. Smith CC, Olsen KS, Gentry KM, Sambade M, Beck W, Garness J, et al. Landscape and Selection of Vaccine Epitopes in SARS-Cov-2. Genome Med (2021) 13:101. doi: 10.1186/s13073-021-00910-1
63. Nanishi E, Borriello F, O’Meara TR, McGrath ME, Saito Y, Haupt RE, et al. An Aluminum hydroxide:CpG Adjuvant Enhances Protection Elicited by a SARS-CoV-2 Receptor Binding Domain Vaccine in Aged Mice. Sci Transl Med (2021) 14(629):eabj5305. doi: 10.1126/scitranslmed.abj5305
64. Lederer K, Parvathaneni K, Painter MM, Bettini E, Agarwal D, Lundgreen KA, et al. Germinal Center Responses to SARS-CoV-2 mRNA Vaccines in Healthy and Immunocompromised Individuals. Cell (2021) 185:1008–24. doi: 10.1016/j.cell.2022.01.027
65. Hoang TN, Pino M, Boddapati AK, Viox EG, Starke CE, Upadhyay AA, et al. Baricitinib Treatment Resolves Lower-Airway Macrophage Inflammation and Neutrophil Recruitment in SARS-CoV-2-infected Rhesus Macaques. Cell (2021) 184(2):460–475.e21. doi: 10.1016/j.cell.2020.11.007
66. Wang C, Xie J, Zhao L, Fei X, Zhang H, Tan Y, et al. Alveolar Macrophage Dysfunction and Cytokine Storm in the Pathogenesis of Two Severe COVID-19 Patients. EBioMedicine (2020) 57:102833. doi: 10.1016/j.ebiom.2020.102833
67. Pribul PK, Harker J, Wang B, Wang H, Tregoning JS, Schwarz J, et al. Alveolar Macrophages Are a Major Determinant of Early Responses to Viral Lung Infection But Do Not Influence Subsequent Disease Development. J Virol (2008) 82(9):4441–8. doi: 10.1128/JVI.02541-07
Keywords: poly (I:C), alum, adjuvants, intradermal route, spike protein, vaccine, SARS-CoV-2
Citation: dos-Santos JS, Firmino-Cruz L, da Fonseca-Martins AM, Oliveira-Maciel D, Perez GG, Roncaglia-Pereira VA, Dumard CH, Guedes-da-Silva FH, Santos ACV, Leandro MdS, Ferreira JRM, Guimarães-Pinto K, Conde L, Rodrigues DAS, Silva MVdM, Alvim RGF, Lima TM, Marsili FF, Abreu DPB, Ferreira Jr. OC, Mohana Borges RdS, Tanuri A, Souza TML, Rossi-Bergmann B, Vale AM, Silva JL, de Oliveira AC, Filardy AD’A, Gomes AMO and de Matos Guedes HL (2022) Immunogenicity of SARS-CoV-2 Trimeric Spike Protein Associated to Poly(I:C) Plus Alum. Front. Immunol. 13:884760. doi: 10.3389/fimmu.2022.884760
Received: 27 February 2022; Accepted: 15 April 2022;
Published: 30 June 2022.
Edited by:
Pengfei Wang, Fudan University, ChinaReviewed by:
Gerco den Hartog, National Institute for Public Health and the Environment (Netherlands), NetherlandsCopyright © 2022 dos-Santos, Firmino-Cruz, da Fonseca-Martins, Oliveira-Maciel, Perez, Roncaglia-Pereira, Dumard, Guedes-da-Silva, Santos, Leandro, Ferreira, Guimarães-Pinto, Conde, Rodrigues, Silva, Alvim, Lima, Marsili, Abreu, Ferreira Jr., Mohana Borges, Tanuri, Souza, Rossi-Bergmann, Vale, Silva, de Oliveira, Filardy, Gomes and de Matos Guedes. This is an open-access article distributed under the terms of the Creative Commons Attribution License (CC BY). The use, distribution or reproduction in other forums is permitted, provided the original author(s) and the copyright owner(s) are credited and that the original publication in this journal is cited, in accordance with accepted academic practice. No use, distribution or reproduction is permitted which does not comply with these terms.
*Correspondence: Herbert Leonel de Matos Guedes, aGVyYmVydEBtaWNyby51ZnJqLmJy; aGVyYmVydEBpb2MuZmlvY3J1ei5icg==
†These authors have contributed equally to this work
‡These authors share senior authorship
Disclaimer: All claims expressed in this article are solely those of the authors and do not necessarily represent those of their affiliated organizations, or those of the publisher, the editors and the reviewers. Any product that may be evaluated in this article or claim that may be made by its manufacturer is not guaranteed or endorsed by the publisher.
Research integrity at Frontiers
Learn more about the work of our research integrity team to safeguard the quality of each article we publish.