- 1IRCCS Humanitas Research Hospital, Milan, Italy
- 2Milan Unit, CNR-IRGB, Milan, Italy
- 3Laboratory of Cancer Pharmacology, Department of Oncology, IRCCS Mario Negri Pharmacological Research Institute, Milan, Italy
The bone marrow (BM) niche is the spatial structure within the intra-trabecular spaces of spongious bones and of the cavity of long bones where adult haematopoietic stem cells (HSCs) maintain their undifferentiated and cellular self-renewal state through the intervention of vascular and nervous networks, metabolic pathways, transcriptional and epigenetic regulators, and humoral signals. Within the niche, HSCs interact with various cell types such as osteoblasts, endothelial cells, macrophages, and mesenchymal stromal cells (MSCs), which maintain HSCs in a quiescent state or sustain their proliferation, differentiation, and trafficking, depending on body needs. In physiological conditions, the BM niche permits the daily production of all the blood and immune cells and their admittance/ingress/progression into the bloodstream. However, disruption of this delicate microenvironment promotes the initiation and progression of malignancies such as those included in the spectrum of myeloid neoplasms, also favouring resistance to pharmacological therapies. Alterations in the MSC population and in the crosstalk with HSCs owing to tumour-derived factors contribute to the formation of a malignant niche. On the other hand, cells of the BM microenvironment cooperate in creating a unique milieu favouring metastasization of distant tumours into the bone. In this framework, the pro-tumorigenic role of MSCs is well-documented, and few evidence suggest also an anti-tumorigenic effect. Here we will review recent advances regarding the BM niche composition and functionality in normal and in malignant conditions, as well as the therapeutic implications of the interplay between its diverse cellular components and malignant cells.
Introduction
Bone marrow (BM) niches are specialized microenvironments within bones where supportive cells forming the cellular niche allow the maintenance and differentiation of haematopoietic and mesenchymal stem cells (HSCs and MSCs, respectively) (1, 2). The cellular characterization of these complex microenvironments has been achieved mainly by means of elaborated genetic approaches targeting selected candidate factors, despite limitations of specificity inherent to this strategy, resulting in controversial conclusions. More recently, the combined application of state-of-the-art technologies including high resolution imaging, single-cell RNA sequencing (scRNAseq) and spatially resolved transcriptomics has led to an unprecedented insight into the molecular, cellular, and spatial organization of BM niches, and hierarchical structures and differentiation trajectories therein (3–6). On this basis, sinusoidal, arteriolar and endosteal niches appear to be distinguished not only by their specific location, but also by their unique cellular composition and molecular requirements. Moreover, increasing evidence demonstrate that alterations at different levels in the niche composition are associated with malignancies including myelodysplastic syndromes (MDS) (7) and myeloproliferative neoplasms (MPN) (8), with an additional effect on the osteo-haematopoietic niche deriving from medical treatments (9, 10). Remarkable efforts have been devoted also to translating this basic knowledge, mainly derived from murine models, into the development of relevant in vitro platforms to study the human counterpart and to test drugs in a patient-specific setting (11, 12). As a perspective, these tools may also serve to test the inherent therapeutic potential of the various niche components.
This expanding field has been covered by several comprehensive reviews. Here we will give an overview of the interplay between BM niches and tumour cells (TCs), focus preferentially on very last papers, and highlight therapeutic implications and perspectives of this mutual relationship, for the benefit of a wide audience ranging from neophytes to experts.
Composition of BM Niches
MSC and HSC lineage cells are responsible for the high dynamism of the bone tissue, and shape the BM niches, spatially defined microenvironments classified as endosteal and subendosteal (based on the distance from the inner bone surface, particularly at the metaphyseal spongiosa), arteriolar (close to the main vessels carrying blood into the BM), sinusoidal (next to vessels carrying blood out of the BM and forming a wide network within the BMME) and non-vascular (6), based on their location. Overall, niche cell composition is varied, with a distinct array of components in a specific spatial location. Osteoblasts (OBs), chondrocytes and endosteal fibroblasts are present only in the endosteal niche; arteriolar endothelial cells (ECs), smooth muscle cells, arteriolar fibroblasts localize to the arteriolar niche; a newly identified subset of CXCL12-abundant reticular (CAR) cells called Osteo-CARs, displaying a high expression of CXCL12 and osteolineage genes such as osterix (SP7) and lower leptin receptor (LEPR), localize to arteriolar and non-vascular niches; sinusoidal ECs are found in the sinusoidal and in the (sub-)endosteal niches, in line with sinusoids’ elongation through the entire BM cavity. In the proximity of sinusoids, another CAR subpopulation called Adipo-CAR, characterized by high expression of adipogenic lineage genes, resides, too. Interestingly, this subtle dissection of the BM cellular niche has highlighted the heterogeneity of the CAR cell population, specialized MSCs essential for the HSC maintenance and control at many developmental stages, recently isolated also from human adult BM (13). On the other hand, consensus on the panel of protein markers unique for murine and human MSCs is still lacking (see section A Role for MSCs in MPN) and establishing the relationship between MSC subsets described by various recent works is not straightforward (14).
At steady state, HSCs are mostly quiescent and located in perisinusoidal niches, while periarteriolar niches are important mainly for lymphopoiesis (15–17). Committed progenitors likely also exploit dedicated sinusoidal niches, as revealed by elegant approaches of in situ mapping, delineating an atlas of spatially and functionally distinct niches (6). For what pertains to endosteal niches, and the contribution of fully differentiated skeletal cells to HSC maintenance, opposed pieces of evidence are present in the literature with respect to osteoclasts (OCs) (18, 19); OBs have been the first cell population reported to support HSCs (20, 21), but later studies have clearly demonstrated that the major role is played by CAR cells, not by OBs (22–24), so OB contribution has yet to be clarified. Finally, osteocytes might influence specifically myelopoiesis by means of secreted molecules (25).
A plethora of supportive factors are provided in the niches (Table 1). Recent evidence showed that the key niche factors SCF and CXCL12 can be modulated by the Caspase3/NLRP3 signaling, which extends understanding of regulatory mechanisms influencing haematopoiesis (40). Of note, the same molecular cue presented by diverse cells in BM niches may serve different functions in each compartment. For example, Himburg and colleagues recently demonstrated that the heparin-binding growth factor pleiotrophin, already known to promote HSC expansion in vitro and HSC regeneration in vivo, must be provided by LepR+ BM stromal cells for HSC maintenance during steady-state haematopoiesis, and by ECs for HSC regeneration after injury (irradiation) (32). Similar restrictions in growth factor provision apply to committed haematopoietic progenitors: for example, the maintenance of the pool of c-Kit+ haematopoietic progenitors requires (among other factors) SCF supply from LepR+ BM stromal cells and not from ECs (41). On the other hand, the same factor may elicit different effects on haematopoietic stem and progenitor cells (HSPCs) versus more downstream committed progenitors. For example, the secreted RNase angiogenin, expressed by BM osteolineage cells, has been recently demonstrated to restrict proliferation of primitive HSPCs, on one hand, and stimulate proliferation of myeloid-restricted progenitors, on the other, owing to a differential effect on RNA processing in the two subsets (42). Overall, this further demonstrates the cellular specialization within BM niches.
BM Niches as Tumour Cell Factory In Acquired Blood Disorders: A Focus on MPN and MDS
At the end of the 19th century, Stephen Paget postulated the “seed and soil” hypothesis that stated that TC (seeds) need a propitious medium (soil) to establish metastases. This concept can be applied also to malignant cells giving rise to haematological diseases. Most types of blood cancer, including acute and chronic leukaemia, myeloproliferative disorders and MDS, are primarily driven by accumulation of mutations in HSCs or in their progenitors. Growth and survival of the malignant clone is favoured by age-related or inflammation-driven changes in the BM microenvironment (BMME) (43, 44). On the other hand, BMME is affected by signals coming from mutated HSCs, in a bidirectional crosstalk (45).
Myeloproliferative neoplasms (MPN) and MDS are two paradigmatic and opposite examples of diseases caused by mutated HSCs that in turn can alter the surrounding microenvironment, although detailed mechanisms have not been completely defined. MPN include essential thrombocythemia, polycythaemia vera, and primary myelofibrosis, characterized by excess of platelets, erythrocytosis or myelofibrosis, respectively, with increased risk of thrombotic events and of leukemic transformation (46). They are due to mutations occurring at the HSC level in JAK2, MPL or CALR genes, all resulting in unregulated activation of the JAK/STAT pathway, although involvement of other genes (47) or microenvironmental factors contribute to disease initiation or progression.
MDS are a heterogeneous group of acquired clonal disorders of HSCs, characterized by ineffective haematopoiesis, peripheral cytopenia, genetic instability, and high risk of progression to acute myeloid leukaemia (AML) (48). MDS- and MPN-HSPCs display cell-intrinsic dysregulation of innate immune and inflammatory pathways, which in turn have an impact on the surrounding BMME. For example, MDS-HSPCs have aberrantly high expression of TLRs, which activate the adaptive immune system contributing to maintain an inflammatory environment that is detrimental for HSC function, characterized by increased local and systemic levels of IL-6, IL-1β, or type 1 IFN (49). The concomitant presence of chronic inflammation and of increased levels of anti-inflammatory proteins like TGF-β and TNF-α, contribute also to expand BM myeloid-derived suppressor cells (MDSCs), known to dampen T and natural killer (NK) cell anti-tumour activity (50). Other constituents of the BMME that are dysfunctional in MDS or MPN include ECs, within the vascular niche, and Schwann cells. MDS patients display increased BM microvascular density, likely due to the reported secretion of angiogenic growth factors from MDS cells. ECs from MDS patient manifest genetic, transcriptional and epigenetic modifications, along with secretion of supportive myeloid growth factors, further favouring the growth of the malignant clone (51). Regarding Schwann cells, their number is decreased in MPN compared to healthy donors (HDs), while it is markedly higher in MDS patients with severe fibrosis. Despite the relevance for the pathophysiology has not been demonstrated, one hypothesis is that Schwann cells enhance TGF-β activation, which contributes to the suppression of normal haematopoiesis as well as the promotion of BM fibrosis (52).
All the cell populations mentioned above cooperate in creating a unique milieu that favours TC immune evasion and promotes disease progression. Among all the different BMME components, here we will focus on MSCs, which can influence the malignant clone directly, through an altered HSC-supportive capacity, or by exerting immunosuppressive functions on innate and adaptive immunity cells, thus indirectly affecting malignant HSCs by favouring evasion from immunosurveillance.
A Role for MSCs in MPN
MSCs are multipotent cells able to differentiate into OBs, adipocytes, and chondrocytes. They are commonly characterized by spindle-shape morphology, plastic adherence, in vitro trilineage differentiation and expression of surface markers (comprising CD73, CD90, CD105, CD146, CD106, STRO‐1, SSEA‐4, CD49a, CD27, CD146, and LepR as positive markers, and CD45, CD34, CD19, CD14, CD11b, HLA II as negative ones) (53, 54), while tests for single-cell renewal and multipotency are usually omitted, which may raise some concern regarding the actual stemness of these cells. Even recent markers (e.g., LepR) are not specific and label also mature cell types (4). Lastly, Skeletal Stem Cells (SSCs), defined as bone-resident stem cells committed to skeletogenesis and able to recapitulate bone organogenesis in vivo, have been more reliably isolated from single-cell suspensions after bone enzymatic digestion (14). With this strategy, two spatially distinct SSC populations have been defined: osteochondral SSCs, giving rise to bone, cartilage and stromal lineage; and perivascular SSCs, displaying also adipogenic potential and HSC supportive capacity (55). This protocol is demanding and not yet routinely adopted. Human MSC characterization according to the criteria described above has highlighted some differences between HD- and MPN-derived MSCs. For example, increased expression of CD90, CD73, and CD44, and lower expression of CD105 has been reported in MPN-MSCs as compared to HD-MSCs, despite no difference in terms of morphology and cell differentiation capacity (56). Moreover, MPN-MSCs exhibit an altered expression of several genes involved in cell differentiation and migration (56), such as the MYADM and Angiopoietin-1. Differences between HD- and MPN-MSCs have been found also in the cytokine profile. In fact, MSCs secrete a wide range of soluble factors (VEGFA, CXCL12, ILs) to support regenerative processes and perform immunomodulatory properties on NK cells, lymphocytes and macrophages (57). For example, Activin A, a cytokine involved in inflammation and erythropoiesis, has been reported to induce high grade marrow fibrosis in some MPN patients (8). Another example is provided by secretion of TNF-α, IL-10, and TGF-β, known to reduce the number and function of anti-leukemic cytotoxic T lymphocytes. Moreover, cytokines such as ILs (IL-2, IL-4, IL-6, IL-8, IL-10), GM-CSF, and TGF-β released by MSCs alter the innate and adaptative immune cell activation status to favour tumour development, and progression (58).
MSCs exert a pro-tumorigenic function by favouring the survival and differentiation of mutated haematopoietic precursors (59) through poorly defined mechanisms. Among them, the increased release of extracellular vesicles (EVs) is associated with inflammation and thrombosis, and sustained malignant haematopoiesis (60, 61). Based on this, EVs could represent biomarkers of MPN onset (62), as crucial players in regulating tumour microenvironment through the education of key processes including vascular reactivity, angiogenesis, chemoresistance and immunity. Due to their biocompatibility, small size, ability to cross biological membranes and capacity to target specific cells, EVs also represent a promising new approach for drug delivery (63). Indeed, EVs have been studied as cargo of various oligonucleotides of natural and synthetic origin like Paclitaxel, Doxorubicin and phytochemicals (64). Another mechanism exploited by MPN-MSCs could be an exaggerated activation of the pro-inflammatory NF-kB pathway, leading to cytokine release, and proliferation and maintenance of the mutated HSCs and myeloid and lymphoid precursors (65, 66).
Interestingly, MSCs may also have an anti-tumorigenic function, as demonstrated by decreased in vitro TC proliferation and reduced in vivo tumour growth, through mechanisms including cell cycle arrest and inhibition of angiogenesis (67); this MSC behaviour has been better characterized in the framework of solid tumours like breast and lung cancers (68, 69). Additional evidence of MSC anti-tumorigenic function is the in vivo expansion of MPN-HSC and accelerated MPN progression observed in a murine model of the disease after Nestin+ MSC depletion and consequent reduction of MSC-derived CXCL12. On the contrary, prevention of Nestin+ MSC loss blocks MPN progression by indirectly reducing the number of leukemic stem cells (70).
Overall, this points to a delicate balance between opposite properties of the MSC population, with implications for therapy.
This “Janus” attitude of MSCs with respect to MPN may remind the well-known behaviour of M1/M2 macrophages in the framework of solid tumours (71). Further research will strengthen this intriguing parallelism.
A Role for MSCs in MDS
Most mutations found in MDS patients do not confer an obvious selective advantage to HSPCs that justify the clonal dominance. Indeed, MDS cells have been shown to alter the BMME and exploit cell extrinsic factors to maintain a selective advantage over non-mutated cells, as reviewed elsewhere (72, 73). Moreover, the altered microenvironment is harmful also to normal HSCs, thus negatively affecting the outcome of allogeneic HSPC transplantation.
Alterations of the BMME in MDS include disruption of the BM architecture and higher bone fragility. The mechanism underlying bone defects in MDS has not been fully clarified. For example, despite MSCs are altered in MDS and display recurrent mutations when expanded ex vivo, they are not clonally mutated in vivo (7). One intriguing explanation comes from an MDS murine model, in which delayed bone mineralization by OBs, due to increased levels of FGF-23, has been recently demonstrated (74). However, bone defects may also arise from functional impairment at the MSC level, including altered differentiation potential and cytokine production. Indeed, MSCs from MDS patients (MDS-MSCs) display increased in vitro adipogenic differentiation due to reduced DLK1 expression (75), likely at the expenses of the osteogenic potential. Functional inhibition of MSCs in MDS, leading to defective osteogenic differentiation capacity, is also mediated by TGF-β, present at increased levels in the MDS BMME (50), which cause abnormal gene expression of PITX2, HOXB6 and TBX15, leading to phenotypic and functional deficits (76).
In addition, MDS-MSCs have reduced HSC supporting capacity, as demonstrated by significantly lower chimerism in xenograft models when co-injecting HSPCs with MDS-MSCs as opposed to HD-MSCs (77). Further alterations in the osteo-haematopoietic niche may occur because of pharmacological therapies. For example, treatment with Rigosertib, a novel multi-kinase inhibitor anti-cancer drug currently tested in clinical trials for MDS, has been demonstrated to cause deterioration of the haematopoietic-supporting ability of MDS-MSCs, as shown by reduced number of colony-forming units, especially in the monocytic lineage, in a co-culture setting. In addition, Rigosertib impairs MDS-MSCs viability through microtubule destabilization and mitosis disruption, and decreases bone mass in a murine model of the disease (9).
An MDS-MSC driven mechanism has been shown to induce an immunosuppressive function in monocytes, which acquire an MDSC phenotype and suppress NK cell function (78). This in turn favours survival of MDS-HSPCs, although evidence in patients is lacking at present.
Increased amounts of BM Tregs, which have an immunosuppressive role, in high-risk compared to low-risk MDS patients has been recently described (79). BM Tregs directly affect the HSC supporting ability of BM MSCs. Whether Tregs from MDS patients affect MDS course by altering MSC function remains to be determined (80).
The Bone-BMME: A Tumour Cell Soil
In line with the “seed and soil” concept for metastases establishment, MSCs are an important “soil” component since they can enhance the metastatic ability of TC by strengthening their motility and invasiveness. Moreover, they create a metastatic niche at secondary tumour sites (81, 82). MSCs have been reported to promote gastric (83), lung (84) and breast cancer (BCa) (85) growth and metastasis via stimulation of epithelial to mesenchymal transition (EMT). For instance, OPN release by TC was found to induce MSC production of the chemokine CCL5, which in turn promoted CCR5-mediated BCa cell motility, invasiveness, and metastasis. CCL5 was also reported to be secreted in vitro by human BM MSCs in response to osteosarcoma (86) and BCa cells. MSCs release also factors such as TGF-β, IL-10, NO, PGE2, and IDO, implicated in immunomodulation and thus relevant in creating a TC favourable environment (87).
BCa cells entry into the BM may be facilitated by MSCs through Tac-1 regulation of SDF-1α and CXCR4 (88). The subsequent establishment of TC within the BM results in a pathological cellular crosstalk disrupting bone homeostasis which is mainly controlled by the RANKL/RANK axis (89–91). Whether and how OCs contribute to the pre-metastatic niche and TC bone tropism is largely unknown. RSPO2 and RANKL, secreted by BCa cells as recruiting factors for OC precursors, have been demonstrated to bind their receptor LGR4 through an autocrine/paracrine loop and stimulate the production of DKK1, which acts on OC precursors to promote OC differentiation and pre-metastatic niche formation (92). RANKL signalling is harnessed also in other contexts. For example, in Multiple Myeloma (MM), a plasma cell malignancy developing in the BM, TCs have increased RANKL and decreased OPG expression, resulting in enhanced OC bone resorption and the development of lytic bone lesions (93). Moreover, CXCL12-expressing fibroblasts have been associated with a cancer-promoting phenotype in BCa and aggressive solid tumours (94), pointing to a role in bone metastases (95).
Not only solid, but also haematological cancers remodel the bone microenvironment and generate bone metastases. For example, patients with Adult T-cell leukaemia/lymphoma (ATL) may have widespread osteolytic lesions and hypercalcemia, and a novel ATL mouse model has been recently generated to dissect disease mechanisms and heterogeneity (96). Osteolytic and/or osteosclerotic lesions are present in Hodgkin’s Lymphoma patients, too (97). Moreover, scRNAseq analysis of the BM stroma in a murine model of AML has demonstrated that TCs impair mesenchymal osteogenic differentiation and deregulate the expression of CXCL12 and KITL (3). As another example, MM hijacks the BM niche through direct cell-cell interaction and MM-derived EV-mediated signalling; in this respect, the oncogenic NOTCH receptors have been recently identified as part of the MM-EV cargo with pro-tumorigenic effect (98).
Intercellular communication, even on the long range, has been demonstrated to occur also through diverse types of EVs including exosomes (99). Exosome-mediated PKM2 transfer from prostate cancer (PCa) cells into BMSCs has been shown to promote premetastatic niche formation. Specifically, exosome-derived PKM2 increased CXCL12 production by BMSC in a HIF-1α-dependent fashion, which in turn enhanced PCa seeding and growth in the BM. Accordingly, targeting this axis diminished exosome-mediated bone metastasis (100). Using a bone metastatic model of enzalutamide-resistant PCa, Henrich et al. demonstrated that BM myeloid cells in vitro and in vivo did uptake the EVs released by PCa, leading to activation of NF-κB signalling, enhancing OC differentiation, and decreasing myeloid TSP-1 expression. Reducing BM myeloid cell cholesterol, through systemic administration of nanoparticle mimic of native HDL, prevented the uptake of PCa EVs and, consequently, reduced metastatic burden by 77% (101).
RUNX2 and its regulated genes have been shown to facilitate the acquisition of osteomimetic features and enhance the bone metastatic potential of BCa cells, when overexpressed. Different EV proteins were identified mediating the specific recognition of tumour-derived EVs by OBs (CDH11) and the induction of the osteogenic premetastatic niche (ITGA5). These new markers were demonstrated to be responsible for the formation of a premetastatic niche, revealing a potential EV-based premetastatic niche blockage strategy (102).
Therapeutic Implications
Myelodisplastic and myeloproliferative disorders are characterized by vivid interactions with the osteo-haematopoietic niche. Thus, treatment strategies targeting not only malignant cells, but also the signalling pathways connecting both sides need to be developed to provide a more effective approach (Figure 1). The importance of the BMME is highlighted by the high rates of graft failure and relapses as well as the prolonged time to stabilize the engraftment in MDS patients after allogeneic HSPC transplantation; in fact, the prerequisite of a success stands within the appropriate milieu provided by the BMME and the crosstalk that must be re-built with the haematopoietic cells. In this perspective, for example, the hypomethylating agents Azacytidine and Decitabine are effective not only on the leukemic clone through Wnt signalling inhibition, but also on bone cells improving bone metabolism and favouring bone formation (103, 104). Additionally, Azacitidine in combination with Magrolimab (anti-CD47 antibody) and APR-246 (Eprenetapopt) are exploited in high-risk MDS patients including those with TP53 mutations, which have a complete remission rate lower than 20% with the standard-of-care Azacitidine therapy and poor prognosis. Mechanistically, APR-246 covalently binds to mutant p53 leading to its thermodynamic stabilization, thus shifting the equilibrium toward a functional conformation restoring its activity (105). In a phase Ib/II study, combination treatment with Eprenetapopt and Azacitidine is well-tolerated yielding high rates of clinical response and molecular remissions in patients with TP53-mutant MDS and oligoblastic AML (106).
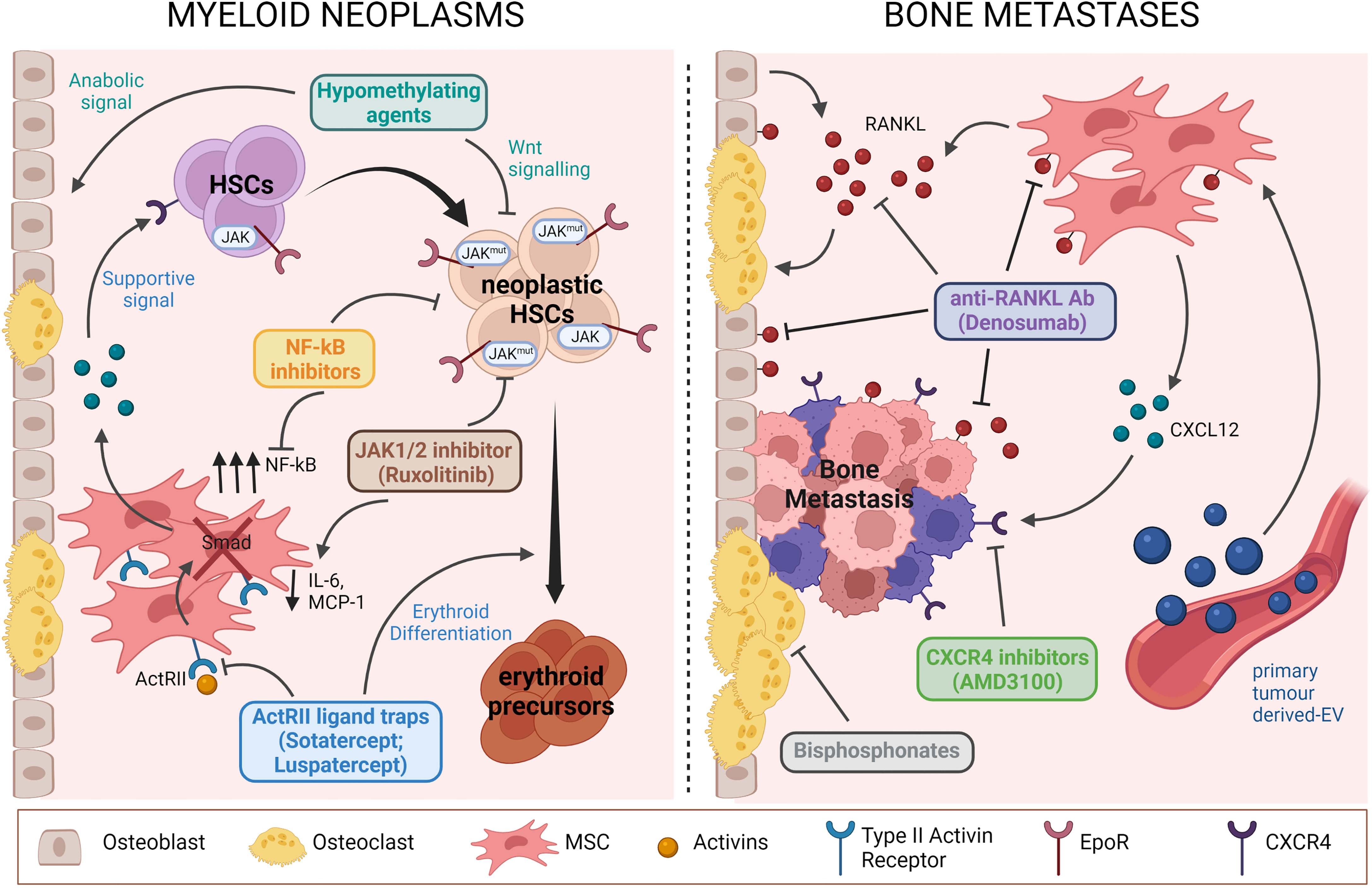
Figure 1 Schematic representation of pathological mechanisms involving BM niche components targeted by current therapies for myeloid neoplasms (we show the example of MPN) and bone metastases. Drugs acting both on BM niche cells and TCs are highlighted. Figure was created with BioRender.com.
Another example of treatment affecting both compartments is ACE-11 (Sotatercept), an activin receptor IIA (ActRIIA) ligand trap, which binds activin and other ligand of the TGF-β family thus interfering with the SMAD pathway (107). Sotatercept influences differentiation of erythroid progenitors or precursors probably by modulating factors of the BM niche (108). Indeed, stromal cells showed alterations in the expression of various important genes and cytokines in response to the drug (109). For example, several secreted proteins with relevance for the regulation of erythropoiesis were upregulated (e.g., IGFBP2, angiotensin II, BMP6, TSP1) or suppressed (e.g., VEGFA, OSM, BMP2) in response to ACE-011 treatment. The analogous ACE-536 (Luspatercept) targets preferentially GDF-8 and GDF-11, corrects the anaemia associated with ineffective erythropoiesis in the NUP98-HOXD13 murine model (110) and has been recently shown to reinstate SDF-1-mediated haematopoietic support by MSCs, thus restoring ineffective haematopoiesis (10). Importantly, both drugs promote maturation of late-stage Epo-independent erythroid precursors and co-treatment with Epo-induced synergistic responses suggesting their use for the treatment of MDS-related anaemia (108), as well as for concomitant alterations of the osteo-haematopoietic niche. In fact, Luspatercept was recently approved for the treatment of β-thalassemia (111) and for MDS low-risk patients with ring sideroblasts who have failed or are ineligible for erythropoiesis-stimulating agents (112).
An alternative strategy to interfere with the niche in MDS could be to counteract the iron overload by means of exogenous hepcidin, transferrin, hepcidin analogues and signalling agonists, since evidence in patients suggested that iron chelation could improve erythropoiesis (113).
The MSC population could be also influenced by Ruxolitinib, a drug used to treat MPN. Ruxolitinib is a JAK1/2 inhibitor that reduces JAK-STAT signalling, which is altered in MPN cells due to the presence of somatic mutations in JAK2 (JAK2V617F), CALR or MPL. The drug acts also on MSCs, by altering the expression level of fibrosis- and HSC maintenance-associated genes (such as LOXL2, SPARC and ADAMTS4, on one hand; and CDH2, CXCL12 and ANGPT1, on the other) and by modifying the cytokine profile, reducing MCP-1 and IL-6 secretion (114).
Moreover, based on the reported hyperactive NF-kB signalling in MPN-MSCs, this pathway could serve as a target in a combined therapeutic approach against haematological malignancies, using for example a NF-kB inhibitor such as Bortezomib or Carfilzomib (66).
For what pertains to bone metastases, treatment is aimed at preventing disease progression and alleviating symptoms and may vary depending on the disease. The classical bone-targeting agents bisphosphonates and Denosumab (anti-RANKL antibody) have been shown to decrease the incidence of skeletal-related events in patients with MM and in those with bone metastases (regardless of the tumour type), but they do not replace the missing bone and, therefore, patients remain at risk of developing fractures, while the use of bone anabolic agents is not yet approved for routine clinical practice (115).
A wide range of agents studied in the last decade block OC bone resorption (116), including Everolimus (mTOR inhibitor), cathepsin K (a protease that degrades collagen during bone resorption) inhibitors (117), SRC tyrosine kinase inhibitors (118) and Cabozantinib (an inhibitor of receptor tyrosine kinases including VEGFR2 and MET) (119).
Emerging targets are the RSPO2/RANKL-LGR4 axis for inhibiting BCa bone metastasis (92), as well as metabolic factors like cholesterol homeostasis (101), that plays critical gate-keeping roles in regulating pro-metastatic signals by target cells at distant sites; both would motivate strategic diagnostic and therapeutic interventions aimed at preventing metastasis. Moreover, the exosome-induced CXCL12 axis could be another actionable pathway, based on the promising results in diminishing exosome-mediated bone metastasis (100). Notably, preventing CXCL12-CXCR4 interaction with the CXCR4 inhibitor Plerixafor (AMD3100) disrupted MM cell contacts with the BMME, thus leading to MM cell mobilization into the circulation (120).
Conclusions and Perspectives
In conclusion, up-to-date extremely powerful technologies have been increasingly unveiling the kaleidoscopic nature of BM niches and their changes in pathologic conditions. In this microenvironment, MSCs are a key component and attract specific interest for therapeutic purposes, even though thus far successful applications in the clinic are limited, while strategies to exploit their plasticity in situ could be explored to bring results closer to expectations. In the tumour setting, future research should better dissect the mechanisms underlying altered MSC function. A pro- and anti-tumorigenic function has been demonstrated for MSCs with respect to MPN cells. To the best of our knowledge, no evidence of a similar mechanism in MDS cells is present in the literature, while it would be worth investigating, particularly for therapeutic purposes.
In the framework of bone metastases, whether TC infiltration elicits long lasting effects on the BM resident cells and whether the BMME remains dysfunctional even after depletion of TC from the metastatic site, are open questions. In a translational perspective, a better understanding of the impact of TC infiltration on the BM milieu could reveal better therapeutic targets. A mechanism of cell-cell communication raising much interest lastly is the crosstalk mediated by EVs. Tumour-derived EV are potent mediators of pre-metastatic niche formation due to their pro-malignant molecular cargo and their propensity to target specific cell types, thus engineering EVs as drug carriers for targeted therapy is an attractive option. Last, combination of the most effective therapies that address different mechanisms, depending on the disease, is likely to be superior to any single therapy.
Author Contributions
VG wrote the Abstract and the “A role for MSCs in MPN” chapter. LC and FF wrote the “BM Niches as Tumour Cell Factory in Acquired Blood Disorders: A Focus on MPN and MDS" and “A role for MSCs in MDS” chapters. And also: All the authors contributed to the “Conclusions and Perspectives” chapter. CN wrote the “The Bone-BMME: a tumour cell soil” and “Therapeutic implications” chapters. CS wrote the “Introduction” and “Composition of BM niches” chapters. All the authors contributed to the “Discussion and perspectives” chapter. LC generated the figure. All the authors contributed to the “Conclusions and perspectives” chapter. All authors contributed to the article and approved the submitted version.
Funding
This work was partially supported by the Italian Ministry of Health (grant RF-2018-12367680 to CS).
Conflict of Interest
The authors declare that the research was conducted in the absence of any commercial or financial relationships that could be construed as a potential conflict of interest.
Publisher’s Note
All claims expressed in this article are solely those of the authors and do not necessarily represent those of their affiliated organizations, or those of the publisher, the editors and the reviewers. Any product that may be evaluated in this article, or claim that may be made by its manufacturer, is not guaranteed or endorsed by the publisher.
Acknowledgments
VG and CN are supported by Fondazione Beppe e Nuccy Angiolini Onlus. We gratefully acknowledge their generous contribution to our research.
Abbreviations
CXCL12, C-X-C Motif Chemokine Ligand 12; SP7, Osterix; LepR, Leptin Receptor; SCF, Stem Cell Factor; IL-6, Interleukin-6; FGF2, Fibroblast Growth Factor 2; NLRP3, NLR Family Pyrin Domain Containing 3; JAK2, Janus Kinase 2; MPL, Myeloproliferative Leukemia, Thrombopoietin Receptor; CALR, Calreticulin; STAT, Signal Transducer and Activator of Transcription; TLR, Toll Like Receptor; IL-1β, Interleukin-1β; IFN, Interferon; VEGFA, Vascular Endothelial Growth Factor A; MYADM, Myeloid Associated Differentiation Marker; GM-CSF, Granulocyte-Macrophage Colony-Stimulating Factor; TGF-β, Transforming Growth Factor Beta; NF-kB, Nuclear Factor Kappa B; WNT, Wingless-type; AKT, Serine/Threonine Kinase 1; FGF-23, Fibroblast Growth Factor 23; DLK1, Delta Like Non-Canonical Notch Ligand 1; CCR5, C-C chemokine receptor type 5; CCL5, C-C Motif Chemokine Ligand 5; IL10, Interleukin-10; NO, Nitric Oxide; PGE2, Prostaglandin E2; IDO, Indoleamine 2,3-dioxygenase; SDF-1α, Stromal cell-Derived Factor 1α; CXCR4, C-X-C Motif Chemokine Receptor 4; RANKL, Receptor Activator of Nuclear Factor kappa B Ligand; RANK, Receptor Activator of Nuclear Factor kappa B; OPG, Osteoprotegerin; RSPO2, R-spondin 2; LGR4, Leucine Rich Repeat Containing G Protein-Coupled Receptor 4; DKK1, Dickkopf 1; PKM2, Pyruvate Kinase M2; TSP-1, Thrombospondin-1; RUNX2, Runt-related transcription factor 2; CDH11, Cadherin 11; ITGA5, integrin subunit alpha 5; FBXW7, F-Box And WD Repeat Domain Containing 7; PTEN, Phosphatase And Tensin Homolog; GSK3, Glycogen Synthase Kinase 3; IGFBP2, Insulin Like Growth Factor Binding Protein 2; BMP6, Bone Morphogenetic Protein 6; TSP1, Thrombospondin 1; OSM, Oncostatin M; BMP2, Bone Morphogenetic Protein 2; GDF-8, Growth/Differentiation Factor 8; GDF-11, Growth/Differentiation Factor 11; MCP-1, Monocyte Chemoattractant Protein-1; LOXL2, Lysyl-Oxidase 2; SPARC, Secreted Protein Acidic and Cysteine Rich; ADAMTS4, ADAM Metallopeptidase with Thrombospondin type 1 motif 4; CDH2, Cadherin 2; MET, Mesenchymal Epithelial Transition; TPO, thrombopoietin; PTN, pleyotropin; ANGPT, Angiopoietin; JAG1, Jagged-1; PGI2, Prostacyclin/prostaglandin I2; OPN, Osteopontin; VCAM1, vascular cell adhesion molecule 1.
Glossary
References
1. Morrison SJ, Scadden DT. The Bone Marrow Niche for Haematopoietic Stem Cells. Nature (2014) 505(7483):327–34. doi: 10.1038/nature12984
2. Mesnieres M, Bohm AM, Peredo N, Trompet D, Valle-Tenney R, Bajaj M, et al. Fetal Hematopoietic Stem Cell Homing Is Controlled by VEGF Regulating the Integrity and Oxidative Status of the Stromal-Vascular Bone Marrow Niches. Cell Rep (2021) 36(8):109618. doi: 10.1016/j.celrep.2021.109618
3. Baryawno N, Przybylski D, Kowalczyk MS, Kfoury Y, Severe N, Gustafsson K, et al. A Cellular Taxonomy of the Bone Marrow Stroma in Homeostasis and Leukemia. Cell (2019) 177(7):1915–32.e16. doi: 10.1016/j.cell.2019.04.040
4. Tikhonova AN, Dolgalev I, Hu H, Sivaraj KK, Hoxha E, Cuesta-Dominguez A, et al. The Bone Marrow Microenvironment at Single-Cell Resolution. Nature (2019) 569(7755):222–8. doi: 10.1038/s41586-019-1104-8
5. Wolock SL, Krishnan I, Tenen DE, Matkins V, Camacho V, Patel S, et al. Mapping Distinct Bone Marrow Niche Populations and Their Differentiation Paths. Cell Rep (2019) 28(2):302–11.e5. doi: 10.1016/j.celrep.2019.06.031
6. Baccin C, Al-Sabah J, Velten L, Helbling PM, Grünschläger F, Hernández-Malmierca P, et al. Combined Single-Cell and Spatial Transcriptomics Reveals the Molecular, Cellular and 2 Spatial Bone Marrow Niche Organization. Nat Cell Biol (2020) 22(1):38–48. doi: 10.1101/718395
7. Jann JC, Mossner M, Riabov V, Altrock E, Schmitt N, Flach J, et al. Bone Marrow Derived Stromal Cells From Myelodysplastic Syndromes are Altered But Not Clonally Mutated In Vivo. Nat Commun (2021) 12(1):6170. doi: 10.1038/s41467-021-26424-3
8. Rambaldi B, Diral E, Donsante S, Di Marzo N, Mottadelli F, Cardinale L, et al. Heterogeneity of the Bone Marrow Niche in Patients With Myeloproliferative Neoplasms: ActivinA Secretion by Mesenchymal Stromal Cells Correlates With the Degree of Marrow Fibrosis. Ann Hematol (2021) 100(1):105–16. doi: 10.1007/s00277-020-04306-w
9. Balaian E, Weidner H, Wobus M, Baschant U, Jacobi A, Mies A, et al. Effects of Rigosertib on the Osteo-Hematopoietic Niche in Myelodysplastic Syndromes. Ann Hematol (2019) 98(9):2063–72. doi: 10.1007/s00277-019-03756-1
10. Wobus M, Mies A, Asokan N, Oelschlägel U, Möbus K, Winter S, et al. Luspatercept Restores SDF-1-Mediated Hematopoietic Support by MDS-Derived Mesenchymal Stromal Cells. Leukemia (2021) 35:2936–47. doi: 10.1038/s41375-021-01275-5
11. Born G, Nikolova M, Scherberich A, Treutlein B, Garcia-Garcia A, Martin I. Engineering of Fully Humanized and Vascularized 3D Bone Marrow Niches Sustaining Undifferentiated Human Cord Blood Hematopoietic Stem and Progenitor Cells. J Tissue Eng (2021) 12:20417314211044855. doi: 10.1177/20417314211044855
12. Garcia-Garcia A, Klein T, Born G, Hilpert M, Scherberich A, Lengerke C, et al. Culturing Patient-Derived Malignant Hematopoietic Stem Cells in Engineered and Fully Humanized 3D Niches. Proc Natl Acad Sci USA (2021) 118(40):e2114227118. doi: 10.1073/pnas.2114227118
13. Aoki K, Kurashige M, Ichii M, Higaki K, Sugiyama T, Kaito T, et al. Identification of CXCL12-Abundant Reticular Cells in Human Adult Bone Marrow. Br J Haematol (2021) 193(3):659–68. doi: 10.1111/bjh.17396
14. Chan CKF, Gulati GS, Sinha R, Tompkins JV, Lopez M, Carter AC, et al. Identification of the Human Skeletal Stem Cell. Cell (2018) 175(1):43–56.e21. doi: 10.1016/j.cell.2018.07.029
15. Pinho S, Marchand T, Yang E, Wei Q, Nerlov C, Frenette PS. Lineage-Biased Hematopoietic Stem Cells Are Regulated by Distinct Niches. Dev Cell (2018) 44(5):634–41.e4. doi: 10.1016/j.devcel.2018.01.016
16. Christodoulou C, Spencer JA, Yeh SA, Turcotte R, Kokkaliaris KD, Panero R, et al. Live-Animal Imaging of Native Haematopoietic Stem and Progenitor Cells. Nature (2020) 578(7794):278–83. doi: 10.1038/s41586-020-1971-z
17. Shen B, Tasdogan A, Ubellacker JM, Zhang J, Nosyreva ED, Du L, et al. A Mechanosensitive Peri-Arteriolar Niche for Osteogenesis and Lymphopoiesis. Nature (2021) 591(7850):438–44. doi: 10.1038/s41586-021-03298-5
18. Lymperi S, Ersek A, Ferraro F, Dazzi F, Horwood NJ. Inhibition of Osteoclast Function Reduces Hematopoietic Stem Cell Numbers In Vivo. Blood (2011) 117(5):1540–9. doi: 10.1182/blood-2010-05-282855
19. Miyamoto K, Yoshida S, Kawasumi M, Hashimoto K, Kimura T, Sato Y, et al. Osteoclasts are Dispensable for Hematopoietic Stem Cell Maintenance and Mobilization. J Exp Med (2011) 208(11):2175–81. doi: 10.1084/jem.20101890
20. Calvi LM, Adams GB, Weibrecht KW, Weber JM, Olson DP, Knight MC, et al. Osteoblastic Cells Regulate the Haematopoietic Stem Cell Niche. Nature (2003) 425(6960):841–6. doi: 10.1038/nature02040
21. Zhang J, Niu C, Ye L, Huang H, He X, Tong W-G, et al. Identification of the Haematopoietic Stem Cell Niche and Control of the Niche Size. Nature (2003) 425(6960):836–41. doi: 10.1038/nature02041
22. Sugiyama T, Kohara H, Noda M, Nagasawa T. Maintenance of the Hematopoietic Stem Cell Pool by CXCL12-CXCR4 Chemokine Signaling in Bone Marrow Stromal Cell Niches. Immunity (2006) 25(6):977–88. doi: 10.1016/j.immuni.2006.10.016
23. Ding L, Saunders TL, Enikolopov G, Morrison SJ. Endothelial and Perivascular Cells Maintain Haematopoietic Stem Cells. Nature (2012) 481(7382):457–62. doi: 10.1038/nature10783
24. Omatsu Y, Seike M, Sugiyama T, Kume T, Nagasawa T. Foxc1 Is a Critical Regulator of Haematopoietic Stem/Progenitor Cell Niche Formation. Nature (2014) 508(7497):536–40. doi: 10.1038/nature13071
25. Azab E, Chandler KB, Uda Y, Sun N, Hussein A, Shuwaikan R, et al. Osteocytes Control Myeloid Cell Proliferation and Differentiation Through Gsalpha-Dependent and -Independent Mechanisms. FASEB J Off Publ Fed Am Soc Exp Biol (2020) 34(8):10191–211. doi: 10.1096/fj.202000366R
26. Greenbaum A, Hsu YM, Day RB, Schuettpelz LG, Christopher MJ, Borgerding JN, et al. CXCL12 in Early Mesenchymal Progenitors is Required for Haematopoietic Stem-Cell Maintenance. Nature (2013) 495(7440):227–30. doi: 10.1038/nature11926
27. Shah M, Li H, Harris M, He J, Paterson AJ, Bhatia R. Role of SCF-Expressing Bone Marrow Populations in Hematopoietic and Leukemic Stem Cell Regulation. Blood (2017) 130(Supplement 1):2439. doi: 10.1182/blood.V130.Suppl_1.2439.2439
28. Tie R, Li H, Cai S, Liang Z, Shan W, Wang B, et al. Interleukin-6 Signaling Regulates Hematopoietic Stem Cell Emergence. Exp Mol Med (2019) 51(10):1–12. doi: 10.1038/s12276-019-0320-5
29. Bisht K, McGirr C, Lee SY, Tseng HW, Fleming W, Alexander KA, et al. Oncostatin M Regulates Hematopoietic Stem Cell (HSC) Niches in the Bone Marrow to Restrict HSC Mobilization. Leukemia (2022) 36(2):333–47. doi: 10.1038/s41375-021-01413-z
30. Rongvaux A, Willinger T, Takizawa H, Rathinam C, Auerbach W, Murphy AJ, et al. Human Thrombopoietin Knockin Mice Efficiently Support Human Hematopoiesis In Vivo. Proc Natl Acad Sci USA (2011) 108(6):2378–83. doi: 10.1073/pnas.1019524108
31. Winkler IG, Wiercinska E, Barbier V, Nowlan B, Bonig H, Levesque JP. Mobilization of Hematopoietic Stem Cells With Highest Self-Renewal by G-CSF Precedes Clonogenic Cell Mobilization Peak. Exp Hematol (2016) 44(4):303–14.e1. doi: 10.1016/j.exphem.2016.01.001
32. Himburg HA, Termini CM, Schlussel L, Kan J, Li M, Zhao L, et al. Distinct Bone Marrow Sources of Pleiotrophin Control Hematopoietic Stem Cell Maintenance and Regeneration. Cell Stem Cell (2018) 23(3):370–81.e5. doi: 10.1016/j.stem.2018.07.003
33. Yoon KA, Son Y, Choi YJ, Kim JH, Cho JY. Fibroblast Growth Factor 2 Supports Osteoblastic Niche Cells During Hematopoietic Homeostasis Recovery After Bone Marrow Suppression. Cell Commun Signal CCS (2017) 15(1):25. doi: 10.1186/s12964-017-0181-2
34. Zhou BO DL, Morrison SJ. Hematopoietic Stem and Progenitor Cells Regulate the Regeneration of Their Niche by Secreting Angiopoietin-1. eLife (2015) 4:e05521. doi: 10.7554/eLife.05521.020
35. Zhao M, Perry JM, Marshall H, Venkatraman A, Qian P, He XC, et al. Megakaryocytes Maintain Homeostatic Quiescence and Promote Post-Injury Regeneration of Hematopoietic Stem Cells. Nat Med (2014) 20(11):1321–6. doi: 10.1038/nm.3706
36. Azizidoost S, Bavarsad MS, Bavarsad MS, Shahrabi S, Jaseb K, Rahim F, et al. The Role of Notch Signaling in Bone Marrow Niche. Hematology (2015) 20(2):93–103. doi: 10.1179/1607845414Y.0000000167
37. Tay J, Barbier V, Helwani FM, Price GR, Levesque JP, Winkler IG. Prostacyclin is an Endosteal Bone Marrow Niche Component and its Clinical Analog Iloprost Protects Hematopoietic Stem Cell Potential During Stress. Stem Cells (2021) 39(11):1532–45. doi: 10.1002/stem.3438
38. Boyerinas B, Zafrir M, Yesilkanal AE, Price TT, Hyjek EM, Sipkins DA. Adhesion to Osteopontin in the Bone Marrow Niche Regulates Lymphoblastic Leukemia Cell Dormancy. Blood (2013) 121(24):4821–31. doi: 10.1182/blood-2012-12-475483
39. Li D, Xue W, Li M, Dong M, Wang J, Wang X, et al. VCAM-1(+) Macrophages Guide the Homing of HSPCs to a Vascular Niche. Nature (2018) 564(7734):119–24. doi: 10.1038/s41586-018-0709-7
40. Zhang J, Chen Y, Yin D, Feng F, An Q, Liu Z, et al. Caspase-3/NLRP3 Signaling in the Mesenchymal Stromal Niche Regulates Myeloid-Biased Hematopoiesis. Stem Cell Res Ther (2021) 12(1):579. doi: 10.1186/s13287-021-02640-y
41. Comazzetto S, Murphy MM, Berto S, Jeffery E, Zhao Z, Morrison SJ. Restricted Hematopoietic Progenitors and Erythropoiesis Require SCF From Leptin Receptor+ Niche Cells in the Bone Marrow. Cell Stem Cell (2019) 24(3):477–86.e6. doi: 10.1016/j.stem.2018.11.022
42. Goncalves KA, Silberstein L, Li S, Severe N, Hu MG, Yang H, et al. Angiogenin Promotes Hematopoietic Regeneration by Dichotomously Regulating Quiescence of Stem and Progenitor Cells. Cell (2016) 166(4):894–906. doi: 10.1016/j.cell.2016.06.042
43. Kokkaliaris KD, Scadden DT. Cell Interactions in the Bone Marrow Microenvironment Affecting Myeloid Malignancies. Blood Adv (2020) 4(15):3795–803. doi: 10.1182/bloodadvances.2020002127
44. Tosato G, Feng J-X, Ohnuki H, Sim M. Bone Marrow Niches in Myelodysplastic Syndromes. J Cancer Metastasis Treat (2021) 7:52. doi: 10.20517/2394-4722.2021.120
45. Soto CA, Lo Celso C, Purton LE, Frisch BJ. From the Niche to Malignant Hematopoiesis and Back: Reciprocal Interactions Between Leukemia and the Bone Marrow Microenvironment. JBMR Plus (2021) 5(10):e10516. doi: 10.1002/jbm4.10516
46. Baumeister J, Chatain N, Sofias AM, Lammers T, Koschmieder S. Progression of Myeloproliferative Neoplasms (MPN): Diagnostic and Therapeutic Perspectives. Cells (2021) 10(12):3551. doi: 10.3390/cells10123551
47. Muggeo S, Crisafulli L, Uva P, Fontana E, Ubezio M, Morenghi E, et al. PBX1-Directed Stem Cell Transcriptional Program Drives Tumor Progression in Myeloproliferative Neoplasm. Stem Cell Rep (2021) 16(11):2607–16. doi: 10.1016/j.stemcr.2021.09.016
48. Menssen AJ, Walter MJ. Genetics of Progression From MDS to Secondary Leukemia. Blood (2020) 136(1):50–60. doi: 10.1182/blood.2019000942
49. Sallman DA, List A. The Central Role of Inflammatory Signaling in the Pathogenesis of Myelodysplastic Syndromes. Blood (2019) 133(10):1039–48. doi: 10.1182/blood-2018-10-844654
50. Kapor S, Santibanez JF. Myeloid-Derived Suppressor Cells and Mesenchymal Stem/Stromal Cells in Myeloid Malignancies. J Clin Med (2021) 10(13):2788. doi: 10.3390/jcm10132788
51. Mosteo L, Storer J, Batta K, Searle EJ, Duarte D, Wiseman DH. The Dynamic Interface Between the Bone Marrow Vascular Niche and Hematopoietic Stem Cells in Myeloid Malignancy. Front Cell Dev Biol (2021) 9:635189. doi: 10.3389/fcell.2021.635189
52. Cao-Sy L, Obara N, Sakamoto T, Kato T, Hattori K, Sakashita S, et al. Prominence of Nestin-Expressing Schwann Cells in Bone Marrow of Patients With Myelodysplastic Syndromes With Severe Fibrosis. Int J Hematol (2019) 109(3):309–18. doi: 10.1007/s12185-018-02576-9
53. Wright A, Arthaud-Day ML, Weiss ML. Therapeutic Use of Mesenchymal Stromal Cells: The Need for Inclusive Characterization Guidelines to Accommodate All Tissue Sources and Species. Front Cell Dev Biol (2021) 9:632717. doi: 10.3389/fcell.2021.632717
54. Samsonraj RM, Rai B, Sathiyanathan P, Puan KJ, Rötzschke O, Hui JH, et al. Establishing Criteria for Human Mesenchymal Stem Cell Potency. Stem Cells (Dayton Ohio) (2015) 33(6):1878–91. doi: 10.1002/stem.1982
55. Ambrosi TH, Sinha R, Steininger HM, Hoover MY, Murphy MP, Koepke LS, et al. Distinct Skeletal Stem Cell Types Orchestrate Long Bone Skeletogenesis. eLife (2021) 10:e66063. doi: 10.7554/eLife.66063
56. Ramos TL, Sanchez-Abarca LI, Roson-Burgo B, Redondo A, Rico A, Preciado S, et al. Mesenchymal Stromal Cells (MSC) From JAK2+ Myeloproliferative Neoplasms Differ From Normal MSC and Contribute to the Maintenance of Neoplastic Hematopoiesis. PloS One (2017) 12(8):e0182470. doi: 10.1371/journal.pone.0182470
57. Andrzejewska A, Lukomska B, Janowski M. Concise Review: Mesenchymal Stem Cells: From Roots to Boost. Stem Cells (2019) 37(7):855–64. doi: 10.1002/stem.3016
58. Joel MDM, Yuan J, Wang J, Yan Y, Qian H, Zhang X, et al. MSC: Immunoregulatory Effects, Roles on Neutrophils and Evolving Clinical Potentials. Am J Transl Res (2019) 11(6):3890–904.
59. Corradi G, Baldazzi C, Ocadlikova D, Marconi G, Parisi S, Testoni N, et al. Mesenchymal Stromal Cells From Myelodysplastic and Acute Myeloid Leukemia Patients Display In Vitro Reduced Proliferative Potential and Similar Capacity to Support Leukemia Cell Survival. Stem Cell Res Ther (2018) 9(1):271. doi: 10.1186/s13287-018-1013-z
60. Barone M, Catani L, Ricci F, Romano M, Forte D, Auteri G, et al. The Role of Circulating Monocytes and JAK Inhibition in the Infectious-Driven Inflammatory Response of Myelofibrosis. Oncoimmunology (2020) 9(1):1782575. doi: 10.1080/2162402X.2020.1782575
61. Forte D, Barone M, Morsiani C, Simonetti G, Fabbri F, Bruno S, et al. Distinct Profile of CD34(+) Cells and Plasma-Derived Extracellular Vesicles From Triple-Negative Patients With Myelofibrosis Reveals Potential Markers of Aggressive Disease. J Exp Clin Cancer Res CR (2021) 40(1):49. doi: 10.1186/s13046-020-01776-8
62. Catani L, Cavo M, Palandri F. The Power of Extracellular Vesicles in Myeloproliferative Neoplasms: "Crafting" a Microenvironment That Matters. Cells (2021) 10(9):2316. doi: 10.3390/cells10092316
63. Claridge B, Lozano J, Poh QH, Greening DW. Development of Extracellular Vesicle Therapeutics: Challenges, Considerations, and Opportunities. Front Cell Dev Biol (2021) 9. doi: 10.3389/fcell.2021.734720
64. Pirisinu M, Pham TC, Zhang DX, Hong TN, Nguyen LT, Le MTN. Extracellular Vesicles as Natural Therapeutic Agents and Innate Drug Delivery Systems for Cancer Treatment: Recent Advances, Current Obstacles, and Challenges for Clinical Translation. Semin Cancer Biol (2022) 80:340–55. doi: 10.1016/j.semcancer.2020.08.007
65. Fisher DAC, Fowles JS, Zhou A, Oh ST. Inflammatory Pathophysiology as a Contributor to Myeloproliferative Neoplasms. Front Immunol (2021) 12:683401. doi: 10.3389/fimmu.2021.683401
66. Sherman LS, Patel SA, Castillo MD, Unkovic R, Taborga M, Gergues M, et al. NFkB Targeting in Bone Marrow Mesenchymal Stem Cell-Mediated Support of Age-Linked Hematological Malignancies. Stem Cell Rev Rep (2021) 17(6):2178–92. doi: 10.1007/s12015-021-10235-6
67. Lee MW, Ryu S, Kim DS, Lee JW, Sung KW, Koo HH, et al. Mesenchymal Stem Cells in Suppression or Progression of Hematologic Malignancy: Current Status and Challenges. Leukemia (2019) 33(3):597–611. doi: 10.1038/s41375-018-0373-9
68. He N, Kong Y, Lei X, Liu Y, Wang J, Xu C, et al. MSCs Inhibit Tumor Progression and Enhance Radiosensitivity of Breast Cancer Cells by Down-Regulating Stat3 Signaling Pathway. Cell Death Dis (2018) 9(10):1026. doi: 10.1038/s41419-018-0949-3
69. Liu T, Zhu K, Ke C, Yang S, Yang F, Li Z, et al. Mesenchymal Stem Cells Inhibited Development of Lung Cancer Induced by Chemical Carcinogens in a Rat Model. Am J Transl Res (2017) 9(6):2891–900.
70. Arranz L, Sanchez-Aguilera A, Martin-Perez D, Isern J, Langa X, Tzankov A, et al. Neuropathy of Haematopoietic Stem Cell Niche Is Essential for Myeloproliferative Neoplasms. Nature (2014) 512(7512):78–81. doi: 10.1038/nature13383
71. Cendrowicz E, Sas Z, Bremer E, Rygiel TP. The Role of Macrophages in Cancer Development and Therapy. Cancers (2021) 13(8):1946. doi: 10.3390/cancers13081946
72. Teodorescu P, Pasca S, Dima D, Tomuleasa C, Ghiaur G. Targeting the Microenvironment in MDS: The Final Frontier. Front Pharmacol (2020) 11:1044. doi: 10.3389/fphar.2020.01044
73. Mian SA, Bonnet D. Nature or Nurture? Role of the Bone Marrow Microenvironment in the Genesis and Maintenance of Myelodysplastic Syndromes. Cancers (2021) 13(16):4116. doi: 10.3390/cancers13164116
74. Weidner H, Baschant U, Lademann F, Ledesma Colunga MG, Balaian E, Hofbauer C, et al. Increased FGF-23 Levels are Linked to Ineffective Erythropoiesis and Impaired Bone Mineralization in Myelodysplastic Syndromes. JCI Insight (2020) 5(15):e137062. doi: 10.1172/jci.insight.137062
75. Weickert MT, Hecker JS, Buck MC, Schreck C, Riviere J, Schiemann M, et al. Bone Marrow Stromal Cells From MDS and AML Patients Show Increased Adipogenic Potential With Reduced Delta-Like-1 Expression. Sci Rep (2021) 11(1):5944. doi: 10.1038/s41598-021-85122-8
76. Geyh S, Rodriguez-Paredes M, Jager P, Koch A, Bormann F, Gutekunst J, et al. Transforming Growth Factor Beta1-Mediated Functional Inhibition of Mesenchymal Stromal Cells in Myelodysplastic Syndromes and Acute Myeloid Leukemia. Haematologica (2018) 103(9):1462–71. doi: 10.3324/haematol.2017.186734
77. Mian SA, Abarrategi A, Kong KL, Rouault-Pierre K, Wood H, Oedekoven CA, et al. Ectopic Humanized Mesenchymal Niche in Mice Enables Robust Engraftment of Myelodysplastic Stem Cells. Blood Cancer Discov (2021) 2(2):135–45. doi: 10.1158/2643-3230.BCD-20-0161
78. Sarhan D, Wang J, Sunil Arvindam U, Hallstron C, Verneris MR, Grzywacz B, et al. Mesenchymal Stromal Cells Shape the MDS Microenvironment by Inducing Suppressive Monocytes That Dampen NK Cell Function. JCI Insight (2020) 5(5):e130155. doi: 10.1172/jci.insight.130155
79. Sallman DA, McLemore AF, Aldrich AL, Komrokji RS, McGraw KL, Dhawan A, et al. TP53 Mutations in Myelodysplastic Syndromes and Secondary AML Confer an Immunosuppressive Phenotype. Blood (2020) 136(24):2812–23. doi: 10.1182/blood.2020006158
80. Camacho V, Matkins VR, Patel SB, Lever JM, Yang Z, Ying L, et al. Bone Marrow Tregs Mediate Stromal Cell Function and Support Hematopoiesis via IL-10. JCI Insight (2020) 5(22):e135681. doi: 10.1172/jci.insight.135681
81. Liang W, Chen X, Zhang S, Fang J, Chen M, Xu Y, et al. Mesenchymal Stem Cells as a Double-Edged Sword in Tumor Growth: Focusing on MSC-Derived Cytokines. Cell Mol Biol Lett (2021) 26(1):3. doi: 10.1186/s11658-020-00246-5
82. Johnson RW, Suva LJ. Hallmarks of Bone Metastasis. Calcified Tissue Int (2018) 102(2):141–51. doi: 10.1007/s00223-017-0362-4
83. Chen B, Yu J, Wang Q, Zhao Y, Sun L, Xu C, et al. Human Bone Marrow Mesenchymal Stem Cells Promote Gastric Cancer Growth via Regulating C-Myc. Stem Cells Int (2018) 2018:9501747. doi: 10.1155/2018/9501747
84. Gu JJ, Hoj J, Rouse C, Pendergast AM. Mesenchymal Stem Cells Promote Metastasis Through Activation of an ABL-MMP9 Signaling Axis in Lung Cancer Cells. PloS One (2020) 15(10):e0241423. doi: 10.1371/journal.pone.0241423
85. Ahn SY. The Role of MSCs in the Tumor Microenvironment and Tumor Progression. Anticancer Res (2020) 40(6):3039–47. doi: 10.21873/anticanres.14284
86. Avnet S, Di Pompo G, Chano T, Errani C, Ibrahim-Hashim A, Gillies RJ, et al. Cancer-Associated Mesenchymal Stroma Fosters the Stemness of Osteosarcoma Cells in Response to Intratumoral Acidosis via NF-kappaB Activation. Int J Cancer (2017) 140(6):1331–45. doi: 10.1002/ijc.30540
87. Weiss ARR, Dahlke MH. Immunomodulation by Mesenchymal Stem Cells (MSCs): Mechanisms of Action of Living, Apoptotic, and Dead MSCs. Front Immunol (2019) 10:1191. doi: 10.3389/fimmu.2019.01191
88. Corcoran KE, Trzaska KA, Fernandes H, Bryan M, Taborga M, Srinivas V, et al. Mesenchymal Stem Cells in Early Entry of Breast Cancer Into Bone Marrow. PloS One (2008) 3(6):e2563. doi: 10.1371/journal.pone.0002563
89. Sobacchi C, Palagano E, Villa A, Menale C. Soluble Factors on Stage to Direct Mesenchymal Stem Cells Fate. Front Bioeng Biotechnol (2017) 5:32. doi: 10.3389/fbioe.2017.00032
90. Kim J-M, Lin C, Stavre Z, Greenblatt MB, Shim J-H. Osteoblast-Osteoclast Communication and Bone Homeostasis. Cells (2020) 9(9):2073. doi: 10.3390/cells9092073
91. Carminati L, Taraboletti G. Thrombospondins in Bone Remodeling and Metastatic Bone Disease. Am J Physiol Cell Physiol (2020) 319(6):C980–C90. doi: 10.1152/ajpcell.00383.2020
92. Yue Z, Niu X, Yuan Z, Qin Q, Jiang W, He L, et al. RSPO2 and RANKL Signal Through LGR4 to Regulate Osteoclastic Premetastatic Niche Formation and Bone Metastasis. J Clin Invest (2022) 132(2):e144579. doi: 10.1172/JCI144579
93. Parrondo RD, Sher T. Prevention Of Skeletal Related Events In Multiple Myeloma: Focus On The RANK-L Pathway In The Treatment Of Multiple Myeloma. OncoTargets Ther (2019) 12:8467–78. doi: 10.2147/OTT.S192490
94. Ahirwar DK, Nasser MW, Ouseph MM, Elbaz M, Cuitino MC, Kladney RD, et al. Fibroblast-Derived CXCL12 Promotes Breast Cancer Metastasis by Facilitating Tumor Cell Intravasation. Oncogene (2018) 37(32):4428–42. doi: 10.1038/s41388-018-0263-7
95. Costa A, Kieffer Y, Scholer-Dahirel A, Pelon F, Bourachot B, Cardon M, et al. Fibroblast Heterogeneity and Immunosuppressive Environment in Human Breast Cancer. Cancer Cell (2018) 33(3):463–79.e10. doi: 10.1016/j.ccell.2018.01.011
96. Kohart NA, Elshafae SM, Supsahvad W, Alasonyalilar-Demirer A, Panfil AR, Xiang J, et al. Mouse Model Recapitulates the Phenotypic Heterogeneity of Human Adult T-Cell Leukemia/Lymphoma in Bone. J Bone Oncol (2019) 19:100257. doi: 10.1016/j.jbo.2019.100257
97. Gaudio F, Pedote P, Niccoli Asabella A, Ingravallo G, Sindaco P, Alberotanza V, et al. Bone Involvement in Hodgkin’s Lymphoma: Clinical Features and Outcome. Acta Haematol (2018) 140(3):178–82. doi: 10.1159/000490489
98. Giannandrea D, Platonova N, Colombo M, Mazzola M, Citro V, Adami R, et al. Extracellular Vesicles Mediate the Communication Between Multiple Myeloma and Bone Marrow Microenvironment in a NOTCH Dependent Way. Haematologica (2022). doi: 10.3324/haematol.2021.279716
99. Cappariello A, Rucci N. Extracellular Vesicles in Bone Tumors: How to Seed in the Surroundings Molecular Information for Malignant Transformation and Progression. Front Oncol (2021) 11:722922. doi: 10.3389/fonc.2021.722922
100. Dai J, Escara-Wilke J, Keller JM, Jung Y, Taichman RS, Pienta KJ, et al. Primary Prostate Cancer Educates Bone Stroma Through Exosomal Pyruvate Kinase M2 to Promote Bone Metastasis. J Exp Med (2019) 216(12):2883–99. doi: 10.1084/jem.20190158
101. Henrich SE, McMahon KM, Plebanek MP, Calvert AE, Feliciano TJ, Parrish S, et al. Prostate Cancer Extracellular Vesicles Mediate Intercellular Communication With Bone Marrow Cells and Promote Metastasis in a Cholesterol-Dependent Manner. J Extracell Vesicles (2020) 10(2):e12042. doi: 10.1002/jev2.12042
102. Li XQ, Zhang R, Lu H, Yue XM, Huang YF. Extracellular Vesicle-Packaged CDH11 and ITGA5 Induce the Premetastatic Niche for Bone Colonization of Breast Cancer Cells. Cancer Res (2022) 82(8):1560–74. doi: 10.1158/0008-5472.CAN-21-1331
103. Boada M, Echarte L, Guillermo C, Diaz L, Tourino C, Grille S. 5-Azacytidine Restores Interleukin 6-Increased Production in Mesenchymal Stromal Cells From Myelodysplastic Patients. Hematol Transfus Cell Ther (2021) 43(1):35–42. doi: 10.1016/j.htct.2019.12.002
104. Poon Z, Dighe N, Venkatesan SS, Cheung AMS, Fan X, Bari S, et al. Bone Marrow MSCs in MDS: Contribution Towards Dysfunctional Hematopoiesis and Potential Targets for Disease Response to Hypomethylating Therapy. Leukemia (2019) 33(6):1487–500. doi: 10.1038/s41375-018-0310-y
105. Feld J, Belasen A, Navada SC. Myelodysplastic Syndromes: A Review of Therapeutic Progress Over the Past 10 Years. Expert Rev Anticancer Ther (2020) 20(6):465–82. doi: 10.1080/14737140.2020.1770088
106. Sallman DA, DeZern AE, Garcia-Manero G, Steensma DP, Roboz GJ, Sekeres MA, et al. Eprenetapopt (APR-246) and Azacitidine in TP53-Mutant Myelodysplastic Syndromes. J Clin Oncol (2021) 39(14):1584–94. doi: 10.1200/JCO.20.02341
107. Dussiot M, Maciel TT, Fricot A, Chartier C, Negre O, Veiga J, et al. An Activin Receptor IIA Ligand Trap Corrects Ineffective Erythropoiesis in Beta-Thalassemia. Nat Med (2014) 20(4):398–407. doi: 10.1038/nm.3468
108. Verma A, Suragani RN, Aluri S, Shah N, Bhagat TD, Alexander MJ, et al. Biological Basis for Efficacy of Activin Receptor Ligand Traps in Myelodysplastic Syndromes. J Clin Invest (2020) 130(2):582–9. doi: 10.1172/JCI133678
109. Iancu-Rubin C, Mosoyan G, Wang J, Kraus T, Sung V, Hoffman R. Stromal Cell-Mediated Inhibition of Erythropoiesis can be Attenuated by Sotatercept (ACE-011), an Activin Receptor Type II Ligand Trap. Exp Hematol (2013) 41(2):155–66.e17. doi: 10.1016/j.exphem.2012.12.002
110. Suragani RN, Cadena SM, Cawley SM, Sako D, Mitchell D, Li R, et al. Transforming Growth Factor-Beta Superfamily Ligand Trap ACE-536 Corrects Anemia by Promoting Late-Stage Erythropoiesis. Nat Med (2014) 20(4):408–14. doi: 10.1038/nm.3512
111. Cappellini MD, Taher AT. The Use of Luspatercept for Thalassemia in Adults. Blood Adv (2021) 5(1):326–33. doi: 10.1182/bloodadvances.2020002725
112. Kubasch AS, Fenaux P, Platzbecker U. Development of Luspatercept to Treat Ineffective Erythropoiesis. Blood Adv (2021) 5(5):1565–75. doi: 10.1182/bloodadvances.2020002177
113. Parisi S, Finelli C. Prognostic Factors and Clinical Considerations for Iron Chelation Therapy in Myelodysplastic Syndrome Patients. J Blood Med (2021) 12:1019–30. doi: 10.2147/JBM.S287876
114. Zacharaki D, Ghazanfari R, Li H, Lim HC, Scheding S. Effects of JAK1/2 Inhibition on Bone Marrow Stromal Cells of Myeloproliferative Neoplasm (MPN) Patients and Healthy Individuals. Eur J Haematol (2018) 101(1):57–67. doi: 10.1111/ejh.13079
115. Coleman R, Hadji P, Body JJ, Santini D, Chow E, Terpos E, et al. Bone Health in Cancer: ESMO Clinical Practice Guidelines. Ann Oncol Off J Eur Soc Med Oncol (2020) 31(12):1650–63. doi: 10.1016/j.annonc.2020.07.019
116. Sousa S, Clezardin P. Bone-Targeted Therapies in Cancer-Induced Bone Disease. Calcified Tissue Int (2018) 102(2):227–50. doi: 10.1007/s00223-017-0353-5
117. Liang W, Wang F, Chen Q, Dai J, Escara-Wilke J, Keller ET, et al. Targeting Cathepsin K Diminishes Prostate Cancer Establishment and Growth in Murine Bone. J Cancer Res Clin Oncol (2019) 145(8):1999–2012. doi: 10.1007/s00432-019-02950-y
118. Uehara S, Udagawa N, Kobayashi Y. Regulation of Osteoclast Function via Rho-Pkn3-C-Src Pathways. J Oral Biosci (2019) 61(3):135–40. doi: 10.1016/j.job.2019.07.002
119. Escudier B PT, Motzer RJ, Olencki T, Arén Frontera O, Oudard S, Rolland F, et al. Cabozantinib, a New Standard of Care for Patients With Advanced Renal Cell Carcinoma and Bone Metastases? Subgroup Analysis of the METEOR Trial. J Clin Oncol (2018) 36(8):765–72. doi: 10.1200/JCO.2017.74.7352
Keywords: bone marrow niches, hematopoietic stem cells (HSCs), MSCs, myeloid neoplasms, metastasis, targeted therapy, RANKL, JAK2
Citation: Granata V, Crisafulli L, Nastasi C, Ficara F and Sobacchi C (2022) Bone Marrow Niches and Tumour Cells: Lights and Shadows of a Mutual Relationship. Front. Immunol. 13:884024. doi: 10.3389/fimmu.2022.884024
Received: 25 February 2022; Accepted: 12 April 2022;
Published: 06 May 2022.
Edited by:
Antonio Maurizi, University of L’Aquila, ItalyReviewed by:
Giovanna D’Amico, Fondazione Matilde Tettamanti Menotti De Marchi, ItalyCopyright © 2022 Granata, Crisafulli, Nastasi, Ficara and Sobacchi. This is an open-access article distributed under the terms of the Creative Commons Attribution License (CC BY). The use, distribution or reproduction in other forums is permitted, provided the original author(s) and the copyright owner(s) are credited and that the original publication in this journal is cited, in accordance with accepted academic practice. No use, distribution or reproduction is permitted which does not comply with these terms.
*Correspondence: Cristina Sobacchi, Y3Jpc3RpbmEuc29iYWNjaGlAaHVtYW5pdGFzcmVzZWFyY2guaXQ=
†These authors share first authorship
‡These authors share last authorship