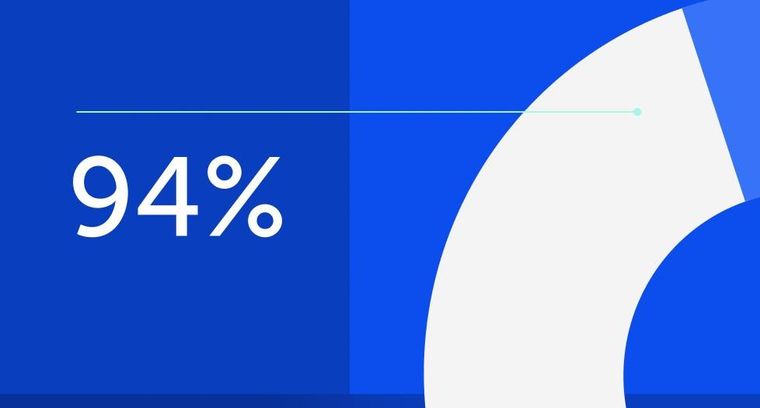
94% of researchers rate our articles as excellent or good
Learn more about the work of our research integrity team to safeguard the quality of each article we publish.
Find out more
ORIGINAL RESEARCH article
Front. Immunol., 13 October 2022
Sec. Microbial Immunology
Volume 13 - 2022 | https://doi.org/10.3389/fimmu.2022.882921
This article is part of the Research TopicImmune Interactions with Pathogenic and Commensal FungiView all 17 articles
Invasive mucormycosis (IM) is a life-threatening infection caused by the fungal order Mucorales, its diagnosis is often delayed, and mortality rates range from 40-80% due to its rapid progression. Individuals suffering from hematological malignancies, diabetes mellitus, organ transplantations, and most recently COVID-19 are particularly susceptible to infection by Mucorales. Given the increase in the occurrence of these diseases, mucormycosis has emerged as one of the most common fungal infections in the last years. However, little is known about the host immune response to Mucorales. Therefore, we characterized the interaction among L. corymbifera—one of the most common causative agents of IM—and human monocytes, which are specialized phagocytes that play an instrumental role in the modulation of the inflammatory response against several pathogenic fungi. This study covered four relevant aspects of the host-pathogen interaction: i) The recognition of L. corymbifera by human monocytes. ii) The intracellular fate of L. corymbifera. iii) The inflammatory response by human monocytes against the most common causative agents of mucormycosis. iv) The main activated Pattern-Recognition Receptors (PRRs) inflammatory signaling cascades in response to L. corymbifera. Here, we demonstrate that L. corymbifera exhibits resistance to intracellular killing over 24 hours, does not germinate, and inflicts minimal damage to the host cell. Nonetheless, viable fungal spores of L. corymbifera induced early production of the pro-inflammatory cytokine IL-1β, and late release of TNF-α and IL-6 by human monocytes. Moreover, we revealed that IL-1β production predominantly depends on Toll-like receptors (TLRs) priming, especially via TLR4, while TNF-α is secreted via C-type lectin receptors (CTLs), and IL-6 is produced by synergistic activation of TLRs and CTLs. All these signaling pathways lead to the activation of NF-kB, a transcription factor that not only regulates the inflammatory response but also the apoptotic fate of monocytes during infection with L. corymbifera. Collectively, our findings provide new insights into the host-pathogen interactions, which may serve for future therapies to enhance the host inflammatory response to L. corymbifera.
During the last years, the number of mucormycosis cases has increased (1–3) along with the predisposing risk factors such as hematological malignancies, diabetes, organ transplantations, and corticosteroid therapies (3–5). Mucormycosis, an infection caused by the fungal order of Mucorales, is characterized by a fast invasion of blood vessels, thrombosis, and tissue necrosis. The outcome of this infection commonly results in high mortality rates due to the rapid destruction of tissue, difficult early diagnosis, and resistance to antifungal agents (6–8). Within the Mucorales, Lichtheimia species are among the most important causative agents together with Rhizopus and Mucor species (3, 5, 9). These molds are currently emerging as opportunistic fungi and exhibit devastating results in immunocompromised patients (10), but also immunocompetent patients can suffer from Farmer’s lung disease, a form of hypersensitivity pneumonitis resulting from recurrent exposure to moldy plant materials (11). After inhalation by humans, the dormant sporangiospores (here assigned as resting spores) of Mucorales encounter several interactions with the host’s adaptive and innate immune system of which just a few have been studied as drivers of pathogenesis [as reviewed by (2, 12, 13)]. Hence, we found it highly relevant to understand their interaction with the host-immune cells and the mechanism that triggers antifungal alarm systems, such as the inflammatory response.
Antifungal immunity requires strict regulation among different cellular processes to eliminate the fungus and protect the organism from collateral damages (14). In that regard, specialized proteins known as cytokines and chemokines promote a balanced communication among several cellular types, modulate phagocyte recruitment, antigen presentation, activation, and immune cell maturation as well as activation and resolution of the inflammatory response, which includes clearance of the pathogen and tissue repair (14, 15). Besides other professional phagocytes, monocytes are instrumental in the modulation of the inflammatory response against several pathogenic fungi (16–18). These immune cells circulate in the bloodstream and migrate into infected tissue attracted by chemokines, where they differentiate into macrophages or dendritic cells (16, 19, 20). Furthermore, monocytes are the main source of IL-1β in humans, a cytokine involved in the initiation of immune responses, induction of further pro-inflammatory proteins, hematopoiesis, differentiation of TH17 cells, development of IL-10, and a typical marker of inflammasome activation (15, 21–23). Additionally, monocytes produce TNF-α and IL-6 which are pro-inflammatory cytokines that mediate host-apoptosis and inflammation as well as trafficking of leukocytes, production of acute-phase proteins, T-cell proliferation, B-cell differentiation, and survival (24, 25).
To initiate the inflammatory response against pathogenic fungi, immune cells recognize cell-wall constituents known as Pathogen-Associated Molecular Patterns (PAMPs), such as cell-wall proteins, glucans, chitin, and lipids, or endogenous signals known as damage-associated molecular patterns (DAMPs) (26, 27). This fungal recognition is mediated by Pattern-Recognition Receptors (PRRs), which are specialized cell-surface receptors triggering pathogen-killing and inflammatory signaling cascades upon stimulation (14, 15, 27). Furthermore, PRRs are classified into several families including Toll-like receptors (TLRs), C-type lectin receptors (CLRs), and NOD-like receptors (NLRs) which modulate several inflammatory signaling pathways resulting in cytokine production (28, 29). For this reason, unraveling the recognition mechanism and the modulation of the inflammatory response against Lichtheimia corymbifera could provide novel insights for diagnostics biomarkers and therapies. Therefore, in this study we focused on three relevant aspects of the host response against this fungus: i) characterization of L. corymbifera recognition by human monocytes. ii) Comparing the pro-inflammatory cytokine profile among the most causative agents of mucormycosis. iii) Determining predominant PRRs axes that prime the inflammatory response against L. corymbifera and its connection to the innate apoptotic immunity. We did not further characterize the host-pathogen interaction with Rhizopus and Mucor species since this subject has been previously described (30–34).
Human blood was obtained from the Institute for Transfusion Medicine, Jena University Hospital, after written informed consent of the healthy donors in accordance with ethics committee approval 4357-03/15 and with the Declaration of Helsinki 1975, as revised in 2008. Mice experiments were approved by Thueringer Landesamt für Verbraucherschutz (breeding license 02-052/16).
The most virulent strains of L. corymbifera JMRC: FSU:009682 (original strain nos.: CBS 429.75, ATCC 46771), and the least virulent JMRC: FSU:0010164, Mucor lusitanicus (formerly: M. circinelloides f. lusitanicus) JMRC: FSU:005859 (original strain no.: CBS 277.49), and Rhizopus arrhizus var. arrhizus (formerly: R. delemar) JMRC: FSU:008743 (original strain no.: 99-880) were grown in Potato Dextrose Agar for seven days at 37° C. Spores were harvested by using sterile phosphate-buffered saline (PBS, 137 mM NaCl, 10 mM Na2HPO4, 2.7 mM KCl, 1.76 mM KH2PO4, pH 7.4). To inactivate spores, 4% formaldehyde (PFA) was used for 30 min at a concentration of 1x107 spores/mL. Finally, spores were counted in a Thoma hemocytometer and adjusted to the desired concentration in RPMI 1640 supplemented with fetal bovine serum (FBS) (Gibco).
Peripheral Blood Mononuclear Cells (PBMC) were isolated from healthy donors’ buffy coats by a density gradient centrifugation using Histopaque-1077 (Sigma Aldrich). Consequently, human monocytes were positively selected from PBMC using CD14 MicroBeads coupled to a magnetic cell sorting system (Miltenyi Biotec). Isolated human monocytes were seeded into twenty-four-well plates at a density of 1x106 cells per well and cultured in RPMI-1640 (Lonza) supplemented with 10% heat-inactivated FBS (Gibco) for two hours at 37 °C and 5% CO2. Resting monocytes were infected with L. corymbifera spores at a multiplicity of infection (MOI) of 5 at the corresponding times.
The method was adapted from Hartung et al. (Cytometry A. 2019 Mar;95(3):332-338). Briefly, erythrocytes were depleted from healthy donors’ blood by osmotic shock using an Erythrocyte Lysis Buffer (0.15 M NH4Cl; 10 mM NH4Cl; 0.1 mM EDTA). Human leukocytes were seeded in a 24-well plate at a density of 1x106 cells per well and cultured in RPMI 1640 (Lonza) supplemented with 10% heat-inactivated FBS (Gibco). The obtained leukocytes were incubated with fluorescein isothiocyanate (FITC)-labeled (100µg/mL) spores at MOI 5 for 30, 60, and 120 minutes at 37 °C and 5% CO2. Consequently, leukocytes were harvested with PBS-2mM EDTA and stained with anti-CD45-BUV395 (Biosciences), anti-CD15-BV605 (Biolegend), anti-CD14-PECy7 (Biolegend), and anti-FITC-APC (Invitrogen) for 20 minutes at room temperature (RT). Phagocytosis was assessed by using an LSR Fortessa (Becton Dickinson) flow cytometer and data were analyzed with FlowJo v10.6.2 software.
Isolated CD14+ monocytes were incubated with L. corymbifera spores over 24 hours in a 24-well plate with RPMI-FBS supplemented medium at 37°C, 5% CO2. Non-phagocytosed spores were stained with Calcofluor-white (CFW) (10 mg/mL, Sigma) for 20 min. After harvesting the cells with PBS-2mM EDTA, the monocytes were lysed with radioimmunoprecipitation assay buffer (RIPA) (200 mM NaCl; 50 mM Tris-HCl, 4 mM EDTA, 1% Deoxychocolate, 1% Triton X-100 and 0.1% w/v SDS) for 40 min on ice. Phagocytosed and adherent spores were centrifuged at 3000 rpm, treated with DNAases (500 mg/mL) for 15 minutes at 37°C, and stained with Propidium Iodide (PI) (Biolegend) for viability measurements. Monocytes were assessed in a separate sample and stained with the Fixable Viability Dye - eFluor™ 780 APC-Cy7 (Thermo Scientific). Viability was quantified by using an LSR Fortessa (Becton Dickinson) flow cytometer and the data were analyzed with FlowJo v10.6.2 software.
Human monocytes (1x106/well) on a 12-mm-diameter coverslip were infected with FITC-labeled spores at MOI 5 for 2 hours. Non-phagocytosed spores were removed with PBS. To monitor the diameter of internalized spores, the cells were incubated for 1, 2, 4, 6, and 16 hours in RPMI medium (Lonza) supplemented with 10% heat-inactivated FBS (Gibco) at 37 °C and 5% CO2. Cells were carefully washed with PBS and counterstained with CFW (100 µg/mL, Thermo Scientific) for 20 minutes at RT. The interaction between monocytes and spores was fixed with 4% formaldehyde for 10 minutes and ProLong Gold Antifade Mountant (Thermo Scientific) was used for microscopy preparations. Microscopic observations were performed using a Zeiss LSM 780 confocal microscope, and the diameter of the internalized spores was measured by ImageJ software.
Human monocytes were positively selected by using CD14 microbeads coupled to a magnetic cell sorting system (Miltenyi Biotec). The isolated cells were incubated in a 24-well plate at 37 °C and 5% CO2, and stimulated for three hours with specific inhibitors reported in previous studies: 5 µM Interleukin-1 Receptor-Associated Kinase (IRAK) inhibitor (CAS 509093-47-4, Merck), 5 µM Spleen tyrosine kinase (Syk) inhibitor (ER 27319 maleate, R&D systems), 10 µM NF-KB inhibitor (Bay11-7082, In vivogen), 10 µg/mL human TLR2 Neutralizing antibody (Ab) (PAb-hTLR2, In vivogen), and 5 µM TLR4 inhibitor (CLI-095, In vivogen) (35–38). Inhibition of IRAK, Syk, and the nuclear factor kappa-light-chain-enhancer of activated B-cells (NF-κB) was performed at a density of 1x106 cells/well in 1 mL of RPMI medium supplemented with 10% v/v FBS, while TLRs at a density of 5x105 cells/well in 0,5 mL of total volume. Subsequently, the monocytes were infected with the mucoralean fungi at MOI 5 for sixteen hours. Then, the supernatants were collected and the secreted cytokines were determined by ELISA MAX™ Deluxe kit (Biolegend). PAM3CSKA (In vivoGen) and ultrapure Lipopolysaccharide (LPS) (In vivoGen) were used as agonists for TLR axis activation, while Mannan (Sigma Aldrich) was used for CTL activation.
Isolated monocytes were cultured in a 24-well plate at a density of 1x106 per well and incubated with freshly harvested spores at MOI 5 for 6 and 16 hours in 1mL of RPMI (Lonza) supplemented with 10% heat-inactivated FBS (Gibco) at 37 °C and 5% CO2. The percentage of damage in monocyte was measured by Lactate Dehydrogenase (LDH) activity following instructions from the cytotoxic detection kit plus LDH (Roche), whereas human IL-1β, TNF-α, IL-6, and IL-10 production were assessed by using ELISA MAX™ Deluxe kit according to the manufacturer’ instructions (Biolegend).
First, monocytes were infected for one hour as previously described. To induce apoptosis, monocytes were treated with staurosporine (STS) to a final concentration of 3.5 µM (Applichem) for three hours. Subsequently, cells were harvested and transferred into a cone-bottom 96-well plate and resuspended in PBS containing calcium and magnesium. To differentiate apoptotic stages and necrosis, monocytes were stained with 1:40 Annexin V conjugated with Pacific Blue (Invitrogen) and 1:2000 eBioscience™ Fixable Viability Dye eFluor™ 780 (Invitrogen) for 20 min at RT. Cells were analyzed using an LSR Fortessa BD flow cytometer and percentages of apoptosis were calculated with FlowJo v10.6.2 software.
Human monocytes were lysed using RIPA buffer (see 1.5) for 40 minutes to obtain whole-cell proteins. 50 µg of total proteins were run on an SDS PAGE gel, transferred to a polyvinylidene difluoride (PVDF) blotting membrane, and incubated with human Caspase-3 mouse mAb (3G2, Cell signaling technology) overnight, and BCL-2 mouse mAb (#15071, Cell signaling technology) for 16 hours. A goat anti-mouse horseradish peroxidase (HRP, Abcam) was then incubated at RT for one hour, followed by incubation with Immobilon Western HRP Substrate (Millipore), and detection by the chemiluminescence reader Octopus QPLEX from Dye AGNOSTICS NH.
Bone marrow from the femur of MyD88 -/- mice was isolated and differentiated into BMDMs according to (39). 7x105 differentiated monocytes were cultivated in RPMI-1640 supplemented with 10% heat-inactivated FBS in a 24-well plate and infected with 7-days old, harvested spores at MOI 5 for 16 hours at 37°C and 5% CO2. Post-infection, the supernatant was collected and the IL-1β production was assessed by using ELISA MAX™ Deluxe kit according to the manufacturer’s instructions (Biolegend).
D’Agostino & Pearson test was performed to determine normal distribution for samples with n ≥6, and Shapiro- Wilk test for n< 6. Values normally distributed were analyzed by using a one-way ANOVA with Sidak’s multiple comparisons test, and by Krustal-Wallis for non-normally distributed data, while differences among two different groups were assessed by paired t-test. All tests were performed in GraphPad Prism software version 6. A p-value <0.05 was considered significant and marked in graphs with the following symbols: *p, 0.05, **p, 0.01, and ***p, 0.001.
Phagocytosis evasion mechanisms contribute to fungal pathogenicity and prevent optimal host-immune response (40). Monocytes and neutrophils range among the professional phagocytes controlling the early stages of fungal infections (16, 41). Therefore, we evaluated the recognition efficacy of L. corymbifera spores by human monocytes and neutrophils, as well as the intracellular fate in human monocytes (Figure 1, S2). During the initial thirty minutes of infection (Figure 1A), we found (84,08 ± 5,48) phagocytosis percentage of L. corymbifera resting spores (alive) by human monocytes and (87,03 ± 7,54) percentage by neutrophils. Moreover, we killed L. corymbifera spores with formaldehyde to determine if fungal viability contribute to the recognition of L. corymbifera. Although phagocytosis was initially slightly decreased with killed spores (Figures 1B, C), we did not find significant differences compared to viable spores (Figure 1B, C). Independently of the treatment, all spores were similarly recognized at 120 minutes of infection and reach a phagocytic saturated stage (Figure 1B, C). These results indicate that monocytes and neutrophils efficiently recognize L. corymbifera spores.
Figure 1 Recognition and intracellular fate of L. corymbifera dormant sporangiospores, here designated as resting spores. (A) Phagocytosis of resting spores by primary monocytes and neutrophils. (B, C) Phagocytosis of alive and killed fungal spores by monocytes and neutrophils. (D) Intracellular growth of L. corymbifera after phagocytosis by primary human monocytes. Fungal spores were pre-stained with FITC and counterstained with calcofluor white (CFW) after phagocytosis to differentiate attached spores (blue) from internalized spores (green). (E) Diameter quantification of internalized spores (n=80/experiment). (F) Percentage of monocytes’ LDH release after infection with L. corymbifera. (G) Viability of phagocytosed spores. Symbols and bars represent the mean ± SD of at least three independent experiments. Differences among groups were assessed by unpaired t test or one-way ANOVA. *p < 0,05; ***p <0,001. ns indicates not significant.
After engulfment, some pathogenic fungi use diverse mechanisms to avoid intracellular killing including inhibition of phagolysosome formation or lytic escape. This might result in further spread of the fungus and a worse outcome of the infection (30, 42). To evaluate the intracellular fate of L. corymbifera, we monitored its growth after phagocytosis by human monocytes (Figure 1D). Hence, we incubated FITC-labeled spores of L. corymbifera with monocytes, followed by counterstaining with CFW to distinguish adherent spores (blue) from internalized spores (green), which increased their diameter over sixteen hours of infection, but did not germinate (Figure 1E), contrary to viable spores cultured under the same conditions but in absence of the phagocytes (Figure S1, left). Moreover, to confirm the intracellular inhibition of L. corymbifera growth, we evaluated the host-cell membrane integrity. For that purpose, we measured the percentage of LDH released from primary monocytes and the monocytic cell line MM6 after exposure to the fungus (Figure 1F). After sixteen hours, we observed only a slight increase in LDH release from the infected primary monocytes (13,3 ± 14,37) and the monocytic cell line MM6 (5,74 ± 6,979), indicating that most monocytes remained viable after infection with L. corymbifera. To confirm these observations, we stained infected monocytes with Fixable Viability Dye eFluor™ 780 and observed that (91,13 ± 9,413) percent of the phagocytes were viable even after twenty-four hours of infection (Figure S3B). Finally, we evaluated the viability of phagocytosed spores by counterstaining non-phagocytosed spores with CFW and using PI to measure viability (Figure S3A). We observed that 91,52 ± 6,255 percent of phagocytosed spores were still alive after 24 hours of engulfment (Figure 1G). Altogether, our results indicate that internalized spores of L. corymbifera remain alive within human monocytes over 24 hours, do not germinate, and inflict minimal damage to the host cell.
Our previous results indicate that most monocytes remain viable during infection with L. corymbifera while restraining the intracellular growth of the fungus. Hence, we wondered whether monocytes could contain the fungal infection while alerting further immune cells via cytokine production. Therefore, we quantified the production of the pro-inflammatory cytokines IL-1β, TNF-α, IL-6, and the anti-inflammatory cytokine IL-10 during exposure to L. corymbifera (Figure 2). We observed that after sixteen hours of infection monocytes produced a significant amount of IL-1β, TNF-α, IL-6 and IL-10 in response to viable fungal spores (Figures 2B, D, F, H). While IL-1β, was the only cytokine significantly released after six hours of exposure to the pathogen, in comparison to monocytes without stimuli (Figure 2A). Then, we evaluated whether fungal viability could influence the production of these cytokines. For this purpose, we measured cytokine release after infection with PFA-inactivated spores. We observed that cytokine production was significantly impaired after incubation with killed spores, which suggests that activation of the monocytes’ inflammatory response to L. corymbifera requires metabolically active spores.
Figure 2 Alive resting spores of L corymbifera trigger early inflammatory responses by human monocytes. Pro-inflammatory cytokine release after six (A, C, E) and sixteen (B, D, F) hours of infection with L. corymbifera. (G, H) Anti-inflammatory cytokine production after infection with L. corymbifera. Bars represent means ± SD of data obtained from three independent experiments, each with one or two different donors, which are represented by dots. Differences among groups were assessed by one-way ANOVA for normally distributed data and Krustal-Wallis for non-normally distributed. *p< 0,05; ***p<0,001. S. proteins indicate surface proteins. ns indicates not significant.
Most of the reported cases of mucormycosis are caused by Rhizopus delemar, Mucor circinelloides, and Lichtheimia corymbifera species (5, 9). Although infection by these Mucorales shares similar characteristics, previous studies reported unique features that may determine the pathogenicity of each species (43). Hence, we evaluated whether monocytes produce a particular inflammatory response against the most virulent strain of L. corymbifera JMRC : FSU:09682 and the clinical reference strains of R. delemar 99-880 and M. circinelloides CBS277.49. For that purpose, we quantified the production of IL-1β, TNF-α, IL-6, and IL-10 after sixteen hours of exposure to the fungi (Figure 3). Despite a broad variation among the donors, we found that M. circinelloides and L. corymbifera induced significant production of IL-1β, contrary to R. delemar which triggered a non-significant but higher release of the cytokine compared to mock-treated monocytes (Figure 3A). Moreover, all mucoralean species produced a significant amount of TNF-α compared to the mock-treated monocytes, being M. circinelloides the lowest inducer of this cytokine (Figure 3B). L. corymbifera and M. circinelloides exerted production of the pro-inflammatory cytokine IL-6 (Figure 3C), and only L. corymbifera prompted a significant anti-inflammatory response via IL-10 production (Figure 3D). Altogether, these results suggest that the mucoralean strains used in this study promote a common signaling inflammatory cascade that results in TNF-α production. However, they may conserve particular traits, such as fungal cell-wall constituents (44), that promote differentcytokines profile. Nonetheless, to consider virulence variability within each species, more strains should be assessed in future studies.
Figure 3 Resting spores of the virulent strain of L corymbifera induce a higher production of TNF-α, IL-6, and IL-10 compared tothe clinical reference strains R. delemar and M. circinelloides. (A–C) Monocytes’ pro-inflammatory cytokine and (D) anti-inflammatory IL-10 release after sixteen hours of exposure to alive spores of the most common pathogenic Mucorales. Lines represent the mean of data obtained from six independent experiments, each with two or three different donors, which are represented by symbols. Differences among groups were assessed by one-way ANOVA for normally distributed data and Krustal-Wallis for non-normally distributed. *p< 0,05; **p<0,01; ***p<0,001. L corymbifera (L.cor), R. delemar (R. del), M. circinelloides (M.cir).
Cytokines are synthesized upon activation of PRRs through different downstream signaling cascades. These signaling pathways are determined by the type of fungal PAMPs that triggers either the CTLs, TLRs, or both of them for a cooperative amplification of fungal recognition and resolution of the infection (23, 28, 29, 45). Certainly, when evaluating pro-inflammatory cytokine production in response to mucoralean fungi, we observed that each downstream mediator, the Tyrosine-protein kinase (SYK)—a central mediator of the CTLs axis— and the Interleukin-1 Receptor-Associated-Kinase (IRAK), a central effector molecule of TLRs, regulate the inflammatory response by human monocytes. However, TNF-α production against R. delemar was reduced after SYK inhibition (Figure 4A) and increased after IRAK inhibition (Figure 4B), while TNF-α production against L. corymbifera was significantly impaired after SYK inhibition (Figure 4A) but not to IRAK inhibition. In the case IL-6 production in response to M. circillenoides, was significantly reduced to IRAK inhibition but not to SYK inhibition. Furthermore, IL-1β production in response to L. corymbifera significantly decreased only after the inhibition of IRAK (Figure 4B), but not to SYK (Figure 4A), These results suggest a predominant dependency on TLR priming to produce IL-1β in response to L. corymbifera infection. To confirm this, we infected BMDMs from mice lacking the adaptor protein MyD88, which regulates the downstream signaling cascade of TLRs, including IRAK (46). As a result, we observed that MyD88 -/- monocytes failed to induce IL-1β after infection with L. corymbifera in contrast to WT monocytes (Figure 5). Interestingly, our previous results showed that IL-1β was induced in response to L. corymbifera during the early and late stages of the infection (Figures 2A, B). Moreover, parallel testing of the inhibitors of TLRs, CTLs, and NF-KB revealed no increase in pro-inflammatory cytokine production which provides evidence for a working experimental system (Figure S4). Therefore, our results suggest that IL-1β production in response to L. corymbifera could be predominantly triggered by TLR priming.
Figure 4 IL-1β production in response to L corymbifera is predominantly primed via the IRAK signaling pathway in human monocytes. Downstream mediators of TLRs and CTLs were inhibited for three hours before infection with the mucoralean fungi. (A) Pro-inflammatory cytokines concentrations after inhibition of the Tyrosine-protein kinase (Syk) or (B) the Interleukin-1 Receptor-Associated-Kinase-1/4 (IRAK), During IRAK inhibition PAM3CSKA was used as a specific ligand, whereas mannan was used during Syk inhibition. Dots and lines represent paired values of every single donor. Data was obtained from three independent experiments, each with two or three different donors, and differences among groups were assessed by paired t-test. *p< 0,05; **p<0,01; ***p<0,001. ns indicates not significant. L corymbifera (L. cor), R. delemar (R. del), M. circinelloides (M. cir).
Figure 5 Bone marrow-derived monocytes (BMDM) from MyD88-/- mice failed to induce IL-1β release in response to L corymbifera. BMDM lacking the adaptor molecule MyD88 were incubated with the mucoralean fungi for sixteen hours. Subsequently, the production of IL-1β was quantified by ELISA. Lines represent the mean of data obtained in two independent experiments, each with three different mice, which are represented with symbols. Differences among groups were assessed by Krustal-Wallis as non-normally distributed data. **p<0,01.
Since the axis of TLRs seems to play an important role in the host-inflammatory response against Mucorales, we evaluated the particular contribution of the receptors TLR2 and TLR4 (Figure 6), which are known to mediate antifungal immunity against other fungi, such as C. albicans and C. neoformans (28, 29, 47, 48). In the case of Mucorales, we observed that TLR2 inhibition did not influence cytokine production in response to Mucorales fungi (Figure 6A). On the other hand, TLR4 inhibition promoted a significant reduction of IL-1β after infection with L. corymbifera and M. circinelloides and TNF-α in response to M. circinelloides. (Figure 6B), which suggests a predominant role of TLR4 in the activation of the monocyte inflammatory response against these Mucorales, more probably by recognition of a polysaccharide such as a glucan given that it is the major component of the spore cell wall in M. circinelloides and play an important role in the immune response against Rhizopus by dendritic cells (49, 50). Furthermore, we observed β-1,3-glucans in the early stages of L. corymbifera germination (Figure S5).
Figure 6 TLR4 contributes to the activation of the inflammatory response by primary human monocytes againstL corymbifera and M. circinelloides. Neutralizing TLR2-antibody and TLR4 inhibitor were incubated for three hours before infection with the Mucorales. (A) Pro-inflammatory cytokines concentrations after inhibition of TLR2 or (B) TLR4. PAM3CSKA was used as a specific ligand for TLR2 activation and LPS forTLR4 activation. Dots and lines represent paired values of every single donor. Data were obtained from three independent experiments, each with two or three different donors, and differences among groups were assessed by paired t-test. *p< 0,05; **p<0,01. ns indicates not significant. L corymbifera (L. cor), R. delemar (R. del), M. circinelloides (M. cir).
Previous studies described the transcription factor NF-kB as a pivotal mediator of the inflammatory response. During infection, immune cell recognition of diverse PAMPs and DAMPs by PRRs activates the NF-kB canonical pathway and induces the transcription of pro-inflammatory genes encoding inflammatory mediators (51). Thus, we evaluated the contribution of the NF-kB canonical signaling pathway to the induction of pro-inflammatory cytokines by preventing its translocation into the nucleus with the inhibitor BAY11-7082 (Figure 7, Figure S4). Certainly, IL-1β, TNF-α, and IL-6 production were significantly reduced after inhibition of NF-kB when infected with L. corymbifera (Figure 7), suggesting the existence of a downstream convergence for these cytokines signaling pathways. Furthermore, inhibition of the canonical NF-kB pathway did not completely abolish IL-1β release in contrast to TNF-α and IL-6 production (Figure 7), suggesting that inflammasome-independent mechanism, as previously described by (52) could be contributing to process pro-IL-1b into IL-1β by human monocytes in response to L. corymbifera.
Figure 7 NF-kB is a central mediator of the inflammatory response during infection with L. corymbifera. Monocytes were incubated for three hours with the NF-KB inhibitor (Bay11-7087), before infection with L. corymbifera for sixteen hours. Pro-inflammatory cytokines were measured by ELISA. Dots and lines represent paired values of every single donor. Data were obtained from three independent experiments, each with two or three different donors, and differences among groups were assessed by paired t-test. *p< 0,05; **p<0,01. L corymbifera (L. cor).
The downstream signaling and release of IL-1β by primary monocytes depend on the intensity of the pro-inflammatory stimuli. Whereas only TLR4 activation induces slow IL-1β release, simultaneous stimulation of several TLRs promotes faster IL-1β secretion and high levels of Reactive Oxygen Species (ROS), which are instrumental for antifungal immunity (23, 27, 40). Accordingly, single monocyte stimulation induces apoptosis, while diverse stimuli trigger pyroptosis (23). Our previous results suggest that L. corymbifera have a long-term intracellular survival (Figures 1F, H), do not cause significant damage in monocytes (Figure 1G and Figure S2C), and induce the early release of IL-1β (Figures 2A, B) predominantly primed by TLR4 activation (Figure 6B). All these findings led us to evaluate whether L. corymbifera could influence the monocyte’s apoptotic response. For that purpose, we infected human monocytes with spores and induced apoptosis with STS, a well-known chemical inducer of apoptosis by activation of caspase-3 (53, 54) (Figure 9). Surprisingly, L. corymbifera did not induce apoptosis in monocytes but did significantly reduce early apoptosis in the cells treated with STS when fully alive.(Figures 8A, B, Figure S6), similar to our results of IL-1β production (Figures 2A, B), suggesting that intracellular viability of L. corymbifera spores could have an influence on both, the inflammatory response and the apoptotic fate of monocytes. We further exposed human monocytes to the NF-kB inhibitor BAY11-7082, as well as L. corymbifera spores, and evaluated the expression of the pro-caspase 3, which is cleaved during apoptosis, and the anti-apoptotic protein BCL-2, which exerts a survival response to several apoptotic stimuli. As a result, the pro-caspase 3 protein expression decreased in the presence of the inhibitor but not of the spores (Figure 8C), whereas BCL-2 protein expression increased after exposure to spores, as well as BAY11-7082. These results together suggest that L. corymbifera spores do not induce apoptosis but a survival response in monocytes which might impact on the release of cytokines and the outcome of the infection.
Figure 8 Viable spores of L corymbifera reduce early apoptosis in monocytes and trigger survival response in human monocytes. (A, B). After phagocytosis of fungal spores, human monocytes were exposed to staurosporine (STS), a kinase inhibitor that induces apoptosis. Subsequently, monocyte apoptotic stages were evaluated by flow cytometry. (C) Detection of pro-caspase 3 (Pro-cas3) and the anti-apoptotic protein BCL-2 after exposure to Bay11-7087, an NF-KB inhibitor, and/or resting spores of L corymbifera. Bars represent means ± SD of data obtained from at least three independent experiments. Differences among groups were assessed by one-way ANOVA. *p< 0,05. ns indicates not significant.
Fungal clearance predominantly depends on the recognition and intracellular killing of pathogens by professional phagocytes. However, some pathogenic fungi such as A. fumigatus, C. neoformans, and C. albicans have developed different strategies to evade immune detection either via modification in their cell-wall constituents or secretion of inhibitory fungal molecules (28, 40, 55–59). In contrast to the previous pathogenic fungi, the most virulent strain of L. corymbifera (FSU: 009682) did not exhibit avoidance of immune detection by monocytes and neutrophils since its sporangiospores were rapidly and efficiently phagocytized by both cell types (Figure 1A), which suggests the presence of highly immunogenic molecules in its cell wall, such as β-1,3-glucans, as shown in L. corymbifera germlings (Figure S5), or unmasked chitosan after removal of glucans (60). However, recognition of an attenuated strain of L. corymbifera (FSU:10164) was reduced approximately by half when compared to the virulent strain (Figure S1B), indicating possible differences in the cell wall composition of each strain, which could influence on their virulence. Nonetheless, given the clinical relevance of L. corymbifera (FSU: 009682), we studied the monocyte response to this particular strain.
In contrast to several pathogenic fungi that avoid intracellular killing by lysing the host’s cells (28, 40), L. corymbifera did not cause significant damage in phagocytic monocytes (Figure 1F) and did not impair their viability over 24 hours of infection (Figure S3B), which was unexpected considering that several clinical cases of mucormycosis reported rapid destruction of tissue and necrosis, as well as the epithelial and endothelial damage caused by other mucoralean fungi upon intracellular growth (1, 6, 30, 61, 62). Moreover, L. corymbifera spores did not exhibit intracellular germination and remained alive after 24 hours of phagocytosis by human monocytes (Figures 1D, E, G), suggesting that the fungus inhibits the intracellular enzymatic degradation, which occurs in the phagolysosome after activation of specific PRRs and recruitment of specific phagosome proteins (28, 63).
In addition to pathogen recognition and clearance, the immune response also involves the production of cytokines (14, 15). These proteins induce inflammation as an “alarm system” to amplify the antifungal response and recruit several phagocytes to the site of infection, (14, 64). For instance, IL-1β promotes further inflammatory proteins, hematopoiesis, differentiation of TH cells as well as resolution of the inflammation via IL-10 induction (15, 21–23), whereas TNF-α mediates the host-apoptotic responses and the inflammatory response, and IL-6 promotes trafficking of leukocytes, production of acute-phase proteins, cell proliferation, and survival (24, 25). In this study, we observed that upon phagocytosis of L. corymbifera, human monocytes released IL-1β during the first hours of infection, while TNF-α and IL-6 were significantly released in later stages of the infection (Figure 2). Our observations suggest that IL-1β has an important role in antifungal immunity against L. corymbifera by promoting an early response in monocytes, which may alert other immune cells and induce further pro-inflammatory cytokines as observed in previous studies (22, 23, 36, 65). Interestingly, only viable spores of L. corymbifera induced pro-inflammatory cytokines compared to killed spores. This finding suggests that inflammatory response to the fungus may rely on a dynamic fungal cellular process, such as re-arrangement of the fungal cell wall or secretion of fungal molecules, as previously observed in R. delemar with the secretion of the ricin-like toxin Mucoricin (66).
During fungal infection, any delay in the activation of the inflammatory response and recruitment of phagocytes to the site of infection could promote faster fungal dissemination and destruction of the tissue (67, 68). Following this, previous studies reported that patients with invasive pulmonary mucormycosis presented extensive angioinvasion but a limited or moderate inflammatory response (69–71). Therefore, we investigated if the production of inflammatory cytokine was repressed in human monocytes exposed to common pathogenic mucoralean fungi. Interestingly, we observed that virulent strain of L. corymbifera induced a broader inflammatory response in human monocytes compared to the reference strains, Rhizopus delemar 99-880 and Mucor circinelloides CBS277.49, which are the most reported strains in previous host-pathogen interaction studies (30, 34, 72–75)(Figure 3). For this reason, we postulate that the virulent strain of L. corymbifera is a good candidate to elucidate molecular mechanisms, such as priming of PRRs and cytokine signaling pathways activated during infection with Mucorales, since it may possess unique traits, either cell-wall constituents or secreted fungal molecules that promote a faster and broader inflammatory response compared to the other strains.
In this study, we provide new insights into candidate signaling pathways involved in the inflammatory response against Mucorales by evaluating the production of common pro-inflammatory cytokines upon inhibition of the main PRRs’ downstream mediators, such as the Tyrosine-protein kinase (SYK) that modulates the CTLs signaling pathway, or the Interleukin-1 Receptor-Associated Kinase (IRAK) that mediates signaling from most of the TLRs (26, 28, 29). We observed that both, SYK and IRAK inhibition resulted in a significant reduction of IL-6 and TNF-α release during infection in response to the mucoralean species (Figures 4A, B), which is in agreement with previous studies that reported cooperation between CTLs and TLRs to amplify the inflammatory immune response (28, 45, 76). However, we observed that production of IL-6 and TNF-α depending on a single-axis activation via CTLs, or TLRs relies on the type of mucoralean fungi. As an example, we observed a predominant modulation of IL-6 via TLRs after infection with M. circinelloides, and TNF-α production via CTLs after infection with R. delemar (Figure 4A). Curiously, TNF-α expression in response to R. delemar was increased after IRAK inhibition. Given that the inhibitor alone did not influence its production (Figure S4B), we hypothesize that TNF-α is overproduced via CTLs as a compensatory effect. Interestingly, the use of TNF antagonists as treatment for autoimmune diseases has been associated with an increased incidence of fungal infections (77, 78). IL-1β production in response to L. corymbifera was significantly reduced only after IRAK inhibition (Figure 4B), suggesting a specific route to produce this particular cytokine. This finding may provide new insights for future therapies since IL-1β is produced early in response to L. corymbifera. Thus, a balanced enhancement of this cytokine may promote higher phagocyte recruitment and prevent further fungal invasion. Nonetheless, potential benefits must be weighed against possible complications to avoid detrimental hyperinflammatory responses.
Among the TLRs, TLR2 and TLR4 have been extensively studied in the immune response against other pathogenic fungi. These receptors recognize specific fungal PAMPs, such as α-1,4-glucans, Glucuronoxylomannans, and Phospholipomannans in the case of the TLR2; and O-linked mannans and Rhamnomannansin in the case of TLR4 (28, 29, 47, 48), which later on leads to the nuclear translocation of the central regulator NF-κB (51, 79). In that regard, we observed that TLR4 inhibition reduced the production of TNF-α in response to M. circinelloides and IL-1β secretion after exposure to L. corymbifera and M. circinelloides (Figure 6B), suggesting that TLR4 has a predominant role in the activation of these signaling pathways. Furthermore, TLR2 inhibition did not affect the pro-inflammatory cytokine production after exposure to the Mucorales. A previous study by Chamilos et al. showed that exposure to R. delemar induced tlr2 expression (80). However, the authors measured the mRNA levels in human polymorphonuclear cells instead of total proteins in monocytes as we described here, potentially explaining the discrepancy between studies. Moreover, we observed that NF-κB inhibition reduced IL-1β production and completely abolished TNF-α and IL-6 (Figure 7), suggesting that IL-1β production partially depends on the activation of the transcription factor, while TNF-α and IL-6 pathways converge downstream in NF-κB. Altogether, our findings suggest that each mucoralean species possesses unique cell-wall constituents that may activate different TLRs and CLRs and induce the production of particular pro-inflammatory cytokines, most of them regulated by NF-κB. Hence, future studies should focus on the identification of these specific receptors and their ligands.
Previous studies described a close connection between cell death mechanisms and inflammation during infection, in which the type of activated caspases, produced cytokines, and intensity of the initial pro-inflammatory stimuli determine the nature of the subsequent inflammatory mechanism and the types of programmed cell death (79, 81). As an example, apoptotic responses induce the production of IL-1β, while necroptosis causes the release of IL-1α, in both cases, the cytokines lead to the activation of downstream inflammatory central regulators, such as the transcription factor NF-κB (23, 51, 79). In that regard, we observed that alive spores of L. corymbifera reduced early apoptosis in human monocytes contrary to killed spores (Figure 8A), suggesting that the apoptotic fate of monocytes could depend on a dynamic fungal process, similar to our previous observations of IL-1β secretion in response to L. corymbifera (Figure 2B). Furthermore, we confirmed a connection between the apoptotic and inflammatory responses after detecting pro-caspase 3 expression—a hallmark of apoptosis—in response to the fungus and the NF-KB inhibitor, Bay11-7087. Here, exposure to the fungal spores did not reduce the expression of pro-caspase 3 contrary to the Bay11-7087 treatment that completely abolished the signal (Figure 8C). Moreover, monocytes infected with the fungus increased their expression of the anti-apoptotic protein BCL-2 similarly to those exposed to Bay11-7087, suggesting that L. corymbifera may influence the apoptotic fate of monocytes by promoting their survival via NF-KB modulation. Therefore, we hypothesize that several immune responses to L. corymbifera such as cell death and inflammation could be modulated by the family of inducible transcription factors NF-κB.
Collectively our findings highlight a long-term survival of L. corymbifera within human monocytes, as well as resistance to intracellular killing. This trait may confer the fungus a temporal niche to escape the host immune response. Hence, we believe that further immunotherapy-based strategies are needed to enhance the intracellular degradation of the pathogen. In this study, we found that IL-1β plays an instrumental role in antifungal immunity against L. corymbifera by promoting an early inflammatory response by monocytes (Figure 9). Interestingly, IL-1β is well-known for its key role in the activation and amplification of inflammatory signaling cascades, recruitment of other immune cells to the site of infection, and enhancement of intracellular killing. Here, we provide a predominant route for the production of IL-1β via activation of the TLRs-IRAK-NF-κB axis in response to L. corymbifera, from which TLR4 is a major PRR involved in the recognition of the fungus. Furthermore, we describe the regulation of TNF-α by the CTLs axis, and IL-6 modulation by both, TLRs and CTLs (Figure 9). Altogether, our findings may serve as background to further studies on the inflammatory response driven by the interaction among specific mucoralean ligands and TLR4.
Figure 9 Mechanism of the monocyte inflammatory response against the pathogenic fungus L. corymbifera. (Left panel) Main findings of the initial host-pathogen interactions between human monocytes and L. corymbifera spores over infection. Clockwise, 1) Recognition of the fungal spores within the first hours of infection. 2,3) intracellular growth inhibition and fungal survival after 24 hours post-infection. 4) Minimal damage to the host by the fungus. (Right panel) Monocytic pro-inflammatory cytokine activation in response to L. corymbifera. The production of the pro-inflammatory cytokine IL-1β is predominantly induced by TLRs (blue arrows), especially via TLR4. Whereas, TNF-α release is mediated by CTLs (red arrows), and IL-6 by synergistic activation of CTLs and TLRs (blue arrows). These signaling pathways are regulated by the transcription factor NF-κB, which also modulates the apoptotic fate of infected human monocytes by promoting the expression of the anti-apoptotic protein BCL-2 (green arrow).
The raw data supporting the conclusions of this article will be made available by the authors, without undue reservation.
The studies involving human participants were reviewed and approved by the Institute for Transfusion Medicine, Jena University Hospital, after written informed consent of the healthy donors in accordance with ethics committee approval 4357-03/15 and with the Declaration of Helsinki 1975, as revised in 2008. The patients/participants provided their written informed consent to participate in this study. The animal study was reviewed and approved by Thueringer Landesamt für Verbraucherschutz (breeding license 02-052/16). Written informed consent was obtained from the owners for the participation of their animals in this study.
DM designed and executed the experiments for this study, analyzed the obtained data, and wrote the manuscript. KV conceptualized and supervised the study as well as reviewed and edited the manuscript. SH and ML-T designed the phagocytosis quantification method, provided scientific advice on the study, and supplied human biological material. MW, RA, and BJ designed and performed Murine Bone Marrow-Derived Monocytes co-infection experiments. All authors contributed to the article and approved the submitted version.
This work was supported by the German Research Foundation (DFG) through the CRC/TRR 124 Pathogenic fungi and their human host: Networks of interaction (FungiNet), DFG project number 210879364, Projects A1, A6 and C4 to MvLT, KV and BJ, respectively. We thank financial support also from the Leibniz Institute for Natural Product Research and Infection Biology Jena – Hans Knöll Institute (HKI) and the Friedrich-Schiller University (FSU) of Jena.
We are thankful to the International Leibniz Research School (ILRS) and Dr. Christine Vogler for administrative support. to Dr. Hans-Martin Dahse for providing the human monocytes Mono Mac 6 (MM6) cell-line and cell culture material. We are also grateful to Wolfgang Vivas for the critical reading of the manuscript. We thank Caroline Semm (FSU Jena) for technical assistance with strain maintenance. We also express our gratitude to Silke Steinbach for technical support with Western blotting.
The authors declare that the research was conducted in the absence of any commercial or financial relationships that could be construed as a potential conflict of interest.
All claims expressed in this article are solely those of the authors and do not necessarily represent those of their affiliated organizations, or those of the publisher, the editors and the reviewers. Any product that may be evaluated in this article, or claim that may be made by its manufacturer, is not guaranteed or endorsed by the publisher.
The Supplementary Material for this article can be found online at: https://www.frontiersin.org/articles/10.3389/fimmu.2022.882921/full#supplementary-material
Supplementary Figure 1 | L. corymbifera spores do not germinate after fixation with paraformaldehyde (PF) (A). L. corymbifera treated-spores after sixteen hours of incubation in RPMI-FCS supplemented medium. Scale bar 20 µm. (B) Phagocytosis percentages by primary human monocytes and neutrophils of a virulent (FSU: 9682) and an attenuated (FSU: 10164) strain of L. corymbifera. Symbols and bars represent means ± SD of four independent experiments.
Supplementary Figure 2 | Gating strategy to quantify percentages of phagocytosis. Isolated human leukocytes were incubated with FITC-labeled spores over 180 minutes, followed by identification with CD45+, while monocytes were gated from CD14+ and neutrophils from CD15+ populations. Phagocytosed spores were identified as FITC+/APC-anti FITC- and adherent spores as FITC+/APC-anti FITC+. Numbers in the gating represent percentages of each population from one typical experiment.
Supplementary Figure 3 | Cell viability during fungal infection. (A) Gating strategy to determine the viability of phagocytosed spores after 24 hours of co-infection. Calcofluor white (CFW) was used to discriminate between phagocytosed and adherent spores, and Propidium Iodide (PI) for their viability. (B) Viability of human monocytes over 24 hours of infection with L corymbifera spores. Percentages of viability were determined by staining with Fixable Viability Dye - eFluor™ 780 APC-Cy7 and analyzed by flow cytometry. Bars represent means ± SD of at least three independent experiments.
Supplementary Figure 4 | Inhibitors of TLRs, CLRs, and NF-KB do not increase pro-inflammatory cytokine production in absence of spores. Human monocytes were incubated for sixteen hours with the Interleukin-1 Receptor-Associated-Kinase-1/4 inhibitor (IRAK inh.), the Tyrosine-protein kinase inhibitor (Syk inh.), and the NF-KB inhibitor (Bay11-7087). (A) IL-1β, (B) TNF-α, and (C) IL-6 production after incubation with the inhibitors were measured by ELISA. Bars represent means ± SD of data obtained from at least three independent experiments, each with two or three different donors.
Supplementary Figure 5 | The initial stage of germination exposed 1,3-β-glucans of L. corymbifera. Resting spores of L. corymbifera were grown in RPMI cell-culture medium for four hours at 37°C. Germlings and resting spores of L. corymbifera were incubated with 1,3- β- glucans, anti-mouse IgG: Alexa 488, and visualized by Confocal Laser Scanning Microscopy. Scale bar represents 10µm distance.
Supplementary Figure 6 | Gating strategy to determine apoptosis in human primary monocytes. Annexin V conjugated to Pacific Blue was used to identify apoptotic monocytes and Fixable Viability Dye eFluor™ 780-APC-Cy7 to discriminate between late apoptotic and necrotic cells. Viable cells were identified as Pacific Blue -/APC-Cy7 -, early apoptotic cells as Pacific Blue +/APC-Cy7 -, late apoptotic cells as Pacific Blue +/APC-Cy7 +, and necrotic cells as Pacific Blue -/APC-Cy7 +. Numbers in the gating represent percentages of each population from one typical experiment.
Pathogen-Associated Molecular Patterns (PAMPs), damage-associated molecular patterns (DAMPs), Pattern-Recognition Receptors (PRRs), Toll-like receptors (TLRs), C-type lectin receptors (CTLs), NOD-like receptors (NLRs), phosphate-buffered saline (PBS), para-formaldehyde (PFA), fetal bovine serum (FBS), Peripheral Blood Mononuclear Cells (PBMC), Calcofluor-white (CFW), Fluorescein Isothiocyanate (FITC), Staurosporine (STS).
1. Cornely OA, Alastruey-Izquierdo A, Arenz D, Chen SCA, Dannaoui E, Hochhegger B, et al. Global guideline for the diagnosis and management of mucormycosis: an initiative of the European confederation of medical mycology in cooperation with the mycoses study group education and research consortium. Lancet Infect Dis (2019) 19(12):e405–21. doi: 10.1016/s1473-3099(19)30312-3
2. Hassan MIA, Voigt K. Pathogenicity patterns of mucormycosis: epidemiology, interaction with immune cells and virulence factors. Med Mycol (2019) 57(Supplement_2):S245–s256. doi: 10.1093/mmy/myz011
3. Prakash H, Chakrabarti A. Global epidemiology of mucormycosis. J fungi (Basel Switzerland) (2019) 5(1):26. doi: 10.3390/jof5010026
4. Roden MM, Zaoutis TE, Buchanan WL, Knudsen TA, Sarkisova TA, Schaufele RL, et al. Epidemiology and outcome of zygomycosis: a review of 929 reported cases. Clin Infect Dis (2005) 41(5):634–53. doi: 10.1086/432579
5. Guinea J, Escribano P, Vena A, Muñoz P, Martínez-Jiménez M, Padilla B, et al. Increasing incidence of mucormycosis in a large Spanish hospital from 2007 to 2015: Epidemiology and microbiological characterization of the isolates. PLoS One (2017) 12(6):e0179136. doi: 10.1371/journal.pone.0179136
6. Eucker J, Sezer O, Lehmann R, Weber JR, Graf B, Denkert C, et al. Disseminated mucormycosis caused by Absidia corymbifera leading to cerebral vasculitis. Infection (2000) 28(4):246–50. doi: 10.1007/s150100070047
7. Ribes JA, Vanover-Sams CL, Baker DJ. Zygomycetes in human disease. Clin Microbiol Rev (2000) 13(2):236–301. doi: 10.1128/cmr.13.2.236-301.2000
8. Hoffmann K, Pawlowska J, Walther G, Wrzosek M, de Hoog GS, Benny GL, et al. The family structure of the mucorales: a synoptic revision based on comprehensive multigene-genealogies. Persoonia (2013) 30:57–76. doi: 10.3767/003158513x666259
9. Spellberg B, Edwards J Jr., Ibrahim A. Novel perspectives on mucormycosis: pathophysiology, presentation, and management. Clin Microbiol Rev (2005) 18(3):556–69. doi: 10.1128/cmr.18.3.556-569.2005
10. Schwartze VU, Jacobsen ID. Mucormycoses caused by lichtheimia species. Mycoses (2014) 57 Suppl 3:73–8. doi: 10.1111/myc.12239
11. Bellanger AP, Reboux G, Botterel F, Candido C, Roussel S, Rognon B, et al. New evidence of the involvement of lichtheimia corymbifera in farmer's lung disease. Med Mycol (2010) 48(7):981–7. doi: 10.3109/13693781003713711
12. Ibrahim AS, Kontoyiannis DP. Update on mucormycosis pathogenesis. Curr Opin Infect Dis (2013) 26(6):508–15. doi: 10.1097/qco.0000000000000008
13. Petrikkos G, Tsioutis C. Recent advances in the pathogenesis of mucormycoses. Clin Ther (2018) 40(6):894–902. doi: 10.1016/j.clinthera.2018.03.009
14. Netea MG, Balkwill F, Chonchol M, Cominelli F, Donath MY, Giamarellos-Bourboulis EJ, et al. A guiding map for inflammation. Nat Immunol (2017) 18(8):826–31. doi: 10.1038/ni.3790
15. Akdis M, Aab A, Altunbulakli C, Azkur K, Costa RA, Crameri R, et al. Interleukins (from IL-1 to IL-38), interferons, transforming growth factor beta, and TNF-alpha: Receptors, functions, and roles in diseases. J Allergy Clin Immunol (2016) 138(4):984–1010. doi: 10.1016/j.jaci.2016.06.033
16. Espinosa V, Jhingran A, Dutta O, Kasahara S, Donnelly R, Du P, et al. Inflammatory monocytes orchestrate innate antifungal immunity in the lung. PLoS Pathog (2014) 10(2):e1003940. doi: 10.1371/journal.ppat.1003940
17. Ngo LY, Kasahara S, Kumasaka DK, Knoblaugh SE, Jhingran A, Hohl TM. Inflammatory monocytes mediate early and organ-specific innate defense during systemic candidiasis. J Infect Dis (2014) 209(1):109–19. doi: 10.1093/infdis/jit413
18. Lauvau G, Loke P, Hohl TM. Monocyte-mediated defense against bacteria, fungi, and parasites. Semin Immunol (2015) 27(6):397–409. doi: 10.1016/j.smim.2016.03.014
19. Appay V, Rowland-Jones SL. RANTES: a versatile and controversial chemokine. Trends Immunol (2001) 22(2):83–7. doi: 10.1016/S1471-4906(00)01812-3
20. Autissier P, Soulas C, Burdo TH, Williams KC. Evaluation of a 12-color flow cytometry panel to study lymphocyte, monocyte, and dendritic cell subsets in humans. Cytometry A (2010) 77A(5):410–9. doi: 10.1002/cyto.a.20859
21. Rubartelli A, Cozzolino F, Talio M, Sitia R. A novel secretory pathway for interleukin-1 beta, a protein lacking a signal sequence. EMBO J (1990) 9(5):1503–10. doi: 10.1002/j.1460-2075.1990.tb08268.x
22. Caffrey AK, Obar JJ. Alarming the innate immune system to invasive fungal infections. Curr Opin Microbiol (2016) 32:135–43. doi: 10.1016/j.mib.2016.06.002
23. Semino C, Carta S, Gattorno M, Sitia R, Rubartelli A. Progressive waves of IL-1β release by primary human monocytes via sequential activation of vesicular and gasdermin d-mediated secretory pathways. Cell Death Dis (2018) 9(11):1088. doi: 10.1038/s41419-018-1121-9
24. Idriss HT, Naismith JH. TNFα and the TNF receptor superfamily: Structure-function relationship(s). Microsc Res Tech. (2000) 50(3):184–95. doi: 10.1002/1097-0029(20000801)50:3<184::Aid-jemt2>3.0.Co;2-h
25. Rose-John S, Winthrop K, Calabrese L. The role of IL-6 in host defence against infections: immunobiology and clinical implications. Nat Rev Rheumatol (2017) 13(7):399–409. doi: 10.1038/nrrheum.2017.83
26. Akira S, Uematsu S, Takeuchi O. Pathogen recognition and innate immunity. Cell (2006) 124(4):783–801. doi: 10.1016/j.cell.2006.02.015
27. Lavieri R, Piccioli P, Carta S, Delfino L, Castellani P, Rubartelli A. TLR costimulation causes oxidative stress with unbalance of proinflammatory and anti-inflammatory cytokine production. J Immunol (2014) 192(11):5373–81. doi: 10.4049/jimmunol.1303480
28. Erwig LP, Gow NA. Interactions of fungal pathogens with phagocytes. Nat Rev Microbiol (2016) 14(3):163–76. doi: 10.1038/nrmicro.2015.21
29. Salazar F, Brown GD. Antifungal innate immunity: A perspective from the last 10 years. J Innate Immun (2018) 10(5-6):373–97. doi: 10.1159/000488539
30. Ibrahim AS, Spellberg B, Avanessian V, Fu Y, Edwards JE Jr. Rhizopus oryzae adheres to, is phagocytosed by, and damages endothelial cells in vitro. Infect Immun (2005) 73(2):778–83. doi: 10.1128/IAI.73.2.778-783.2005
31. Schmidt S, Tramsen L, Perkhofer S, Lass-Flörl C, Hanisch M, Röger F, et al. Rhizopus oryzae hyphae are damaged by human natural killer (NK) cells, but suppress NK cell mediated immunity. Immunobiology (2013) 218(7):939–44. doi: 10.1016/j.imbio.2012.10.013
32. Andrianaki AM, Kyrmizi I, Thanopoulou K, Baldin C, Drakos E, Soliman SSM, et al. Iron restriction inside macrophages regulates pulmonary host defense against Rhizopus species. Nat Commun (2018) 9(1):3333. doi: 10.1038/s41467-018-05820-2
33. Lopez-Munoz A, Nicolas FE, Garcia-Moreno D, Perez-Oliva AB, Navarro-Mendoza MI, Hernandez-Onate MA, et al. An adult zebrafish model reveals that mucormycosis induces apoptosis of infected macrophages. Sci Rep (2018) 8(1):12802. doi: 10.1038/s41598-018-30754-6
34. Pérez-Arques C, Navarro-Mendoza MI, Murcia L, Lax C, Martínez-García P, Heitman J, et al. Mucor circinelloides thrives inside the phagosome through an atf-mediated germination pathway. J mBio (2019) 10(1):e02765–02718. doi: 10.1128/mBio.02765-18
35. Kawamoto T, Ii M, Kitazaki T, Iizawa Y, Kimura H. TAK-242 selectively suppresses toll-like receptor 4-signaling mediated by the intracellular domain. Eur J Pharmacol (2008) 584(1):40–8. doi: 10.1016/j.ejphar.2008.01.026
36. Toth A, Zajta E, Csonka K, Vagvolgyi C, Netea MG, Gacser A. Specific pathways mediating inflammasome activation by Candida parapsilosis. Sci Rep (2017) 7:43129. doi: 10.1038/srep43129
37. Briard B, Karki R, Malireddi RKS, Bhattacharya A, Place DE, Mavuluri J, et al. Fungal ligands released by innate immune effectors promote inflammasome activation during Aspergillus fumigatus infection. Nat Microbiol (2019) 4(2):316–27. doi: 10.1038/s41564-018-0298-0
38. Irmscher S, Brix SR, Zipfel SLH, Halder LD, Mutlutürk S, Wulf S, et al. Serum FHR1 binding to necrotic-type cells activates monocytic inflammasome and marks necrotic sites in vasculopathies. Nat Commun (2019) 10(1):2961. doi: 10.1038/s41467-019-10766-0
39. Wagner M, Koester H, Deffge C, Weinert S, Lauf J, Francke A, et al. Isolation and intravenous injection of murine bone marrow derived monocytes. J Vis Exp (2014) 94:1–8. doi: 10.3791/52347
40. Hunniger K, Kurzai O. Phagocytes as central players in the defence against invasive fungal infection. Semin Cell Dev Biol (2019) 89:3–15. doi: 10.1016/j.semcdb.2018.03.021
41. Hohl TM, Rivera A, Lipuma L, Gallegos A, Shi C, Mack M, et al. Inflammatory monocytes facilitate adaptive CD4 T cell responses during respiratory fungal infection. Cell Host Microbe (2009) 6(5):470–81. doi: 10.1016/j.chom.2009.10.007
42. Casadevall A, Coelho C, Alanio A. Mechanisms of cryptococcus neoformans-mediated host damage. Front Immunol (2018) 9:855. doi: 10.3389/fimmu.2018.00855
43. Schwartze VU, Winter S, Shelest E, Marcet-Houben M, Horn F, Wehner S, et al. Gene expansion shapes genome architecture in the human pathogen lichtheimia corymbifera: an evolutionary genomics analysis in the ancient terrestrial mucorales (Mucoromycotina). PLoS Genet (2014) 10(8):e1004496. doi: 10.1371/journal.pgen.1004496
44. Lecointe K, Cornu M, Leroy J, Coulon P, Sendid B. Polysaccharides cell wall architecture of mucorales. Front Microbiol (2019) 10:469. doi: 10.3389/fmicb.2019.00469
45. Netea MG, Joosten LAB, van der Meer JWM, Kullberg B-J, van de Veerdonk FL. Immune defence against candida fungal infections. Nat Rev Immunol (2015) 15(10):630–42. doi: 10.1038/nri3897
46. Cohen P. The TLR and IL-1 signalling network at a glance. J Cell Sci (2014) 127(Pt 11):2383–90. doi: 10.1242/jcs.149831
47. van der Graaf CAA, Netea MG, Verschueren I, van der Meer JWM, Kullberg BJ. Differential cytokine production and toll-like receptor signaling pathways by candida albicans blastoconidia and hyphae. Infection Immun (2005) 73(11):7458–64. doi: 10.1128/IAI.73.11.7458-7464.2005
48. Lionakis MS, Levitz SM. Host control of fungal infections: Lessons from basic studies and human cohorts. Annu Rev Immuno (2018) 36(1):157–91. doi: 10.1146/annurev-immunol-042617-053318
49. Bartnicki-Garcia S, Lindberg B. Partial characterization of mucoran: the glucuronomannan component. Carbohydr Res (1972) 23(1):75–85. doi: 10.1016/s0008-6215(00)81579-7
50. Chamilos G, Ganguly D, Lande R, Gregorio J, Meller S, Goldman WE, et al. Generation of IL-23 producing dendritic cells (DCs) by airborne fungi regulates fungal pathogenicity via the induction of TH-17 responses. PLoS One (2010) 5(9):e12955. doi: 10.1371/journal.pone.0012955
51. Liu T, Zhang L, Joo D, Sun SC. NF-kappaB signaling in inflammation. Signal Transduct Target Ther (2017) 2:1–9. doi: 10.1038/sigtrans.2017.23
52. Crişan TO, Plantinga TS, van de Veerdonk FL, Farcaş MF, Stoffels M, Kullberg B-J, et al. Inflammasome-independent modulation of cytokine response by autophagy in human cells. PLoS One (2011) 6(4):e18666. doi: 10.1371/journal.pone.0018666
53. Chae HJ, Kang JS, Byun JO, Han KS, Kim DU, Oh SM, et al. Molecular mechanism of staurosporine-induced apoptosis in osteoblasts. Pharmacol Res (2000) 42(4):373–81. doi: 10.1006/phrs.2000.0700
54. Volling K, Thywissen A, Brakhage AA, Saluz HP. Phagocytosis of melanized aspergillus conidia by macrophages exerts cytoprotective effects by sustained PI3K/Akt signalling. Cell Microbiol (2011) 13(8):1130–48. doi: 10.1111/j.1462-5822.2011.01605.x
55. Cross CE, Bancroft GJ. Ingestion of acapsular cryptococcus neoformans occurs via mannose and beta-glucan receptors, resulting in cytokine production and increased phagocytosis of the encapsulated form. Infect Immun (1995) 63(7):2604–11. doi: 10.1128/iai.63.7.2604-2611.1995
56. Aimanianda V, Bayry J, Bozza S, Kniemeyer O, Perruccio K, Elluru SR, et al. Surface hydrophobin prevents immune recognition of airborne fungal spores. Nature (2009) 460(7259):1117–21. doi: 10.1038/nature08264
57. Luo S, Poltermann S, Kunert A, Rupp S, Zipfel PF. Immune evasion of the human pathogenic yeast candida albicans: Pra1 is a factor h, FHL-1 and plasminogen binding surface protein. Mol Immunol (2009) 47(2-3):541–50. doi: 10.1016/j.molimm.2009.07.017
58. Voelz K, May RC. Cryptococcal interactions with the host immune system. Eukaryot Cell (2010) 9(6):835–46. doi: 10.1128/ec.00039-10
59. Vivas W, Leonhardt I, Hünniger K, Häder A, Marolda A, Kurzai O. Multiple signaling pathways involved in human dendritic cell maturation are affected by the fungal quorum-sensing molecule farnesol. J Immunol (2019) 203(11):2959–69. doi: 10.4049/jimmunol.1900431
60. Hassan MIA, Keller M, Hillger M, Binder U, Reuter S, Herold K, et al. The impact of episporic modification of lichtheimia corymbifera on virulence and interaction with phagocytes. Comput Struct Biotechnol J (2021) 19:880–96. doi: 10.1016/j.csbj.2021.01.023
61. Ibrahim AS, Spellberg B, Walsh TJ, Kontoyiannis DP. Pathogenesis of mucormycosis. Clin Infect Dis (2012) 54(suppl_1):S16–22. doi: 10.1093/cid/cir865
62. Belic S, Page L, Lazariotou M, Waaga-Gasser AM, Dragan M, Springer J, et al. Comparative analysis of inflammatory cytokine release and alveolar epithelial barrier invasion in a transwell bilayer model of mucormycosis. Front Microbiol (2018) 9:3204. doi: 10.3389/fmicb.2018.03204
63. Feldman MB, Vyas JM, Mansour MK. It takes a village: Phagocytes play a central role in fungal immunity. Semin Cell Dev Biol (2019) 89:16–23. doi: 10.1016/j.semcdb.2018.04.008
64. Romani L. Immunity to fungal infections. Nat Rev Immunol (2011) 11(4):275–88. doi: 10.1038/nri2939
65. Zielinski CE, Mele F, Aschenbrenner D, Jarrossay D, Ronchi F, Gattorno M, et al. Pathogen-induced human TH17 cells produce IFN-γ or IL-10 and are regulated by IL-1β. Nature (2012) 484(7395):514–8. doi: 10.1038/nature10957
66. Soliman SSM, Baldin C, Gu Y, Singh S, Gebremariam T, Swidergall M, et al. Mucoricin is a ricin-like toxin that is critical for the pathogenesis of mucormycosis. Nat Microbiol (2021) 6(3):313–26. doi: 10.1038/s41564-020-00837-0
67. van de Veerdonk FL, Gresnigt MS, Oosting M, van der Meer JWM, Joosten LAB, Netea MG, et al. Protective host defense against disseminated candidiasis is impaired in mice expressing human interleukin-37. Front Microbiol (2015) 5(762):1–6. doi: 10.3389/fmicb.2014.00762
68. Alvarez-Rueda N, Rouges C, Touahri A, Misme-Aucouturier B, Albassier M, Pape PL. In vitro immune responses of human PBMCs against candida albicans reveals fungal and leucocyte phenotypes associated with fungal persistence. Sci Rep (2020) 10(1):6211. doi: 10.1038/s41598-020-63344-6
69. Ben-Ami R, Luna M, Lewis RE, Walsh TJ, Kontoyiannis DP. A clinicopathological study of pulmonary mucormycosis in cancer patients: Extensive angioinvasion but limited inflammatory response. J Infect (2009) 59(2):134–8. doi: 10.1016/j.jinf.2009.06.002
70. Luo L-C, Cheng D-Y, Zhu H, Shu X, Chen W-B. Inflammatory pseudotumoural endotracheal mucormycosis with cartilage damage. Eur Respir Rev (2009) 18(113):186–9. doi: 10.1183/09059180.00000709
71. Mekki SO, Hassan AA, Falemban A, Alkotani N, Alsharif SM, Haron A, et al. Pulmonary mucormycosis: A case report of a rare infection with potential diagnostic problems. Case Rep Pathol (2020) 2020:5845394. doi: 10.1155/2020/5845394
72. Liu M, Spellberg B, Phan QT, Fu Y, Fu Y, Lee AS, et al. The endothelial cell receptor GRP78 is required for mucormycosis pathogenesis in diabetic mice. J Clin Invest (2010) 120(6):1914–24. doi: 10.1172/JCI42164
73. Gebremariam T, Liu M, Luo G, Bruno V, Phan QT, Waring AJ, et al. CotH3 mediates fungal invasion of host cells during mucormycosis. J Clin Invest (2014) 124(1):237–50. doi: 10.1172/JCI71349
74. Lee SC, Li A, Calo S, Inoue M, Tonthat NK, Bain JM, et al. Calcineurin orchestrates dimorphic transitions, antifungal drug responses and host-pathogen interactions of the pathogenic mucoralean fungus mucor circinelloides. Mol Microbiol (2015) 97(5):844–65. doi: 10.1111/mmi.13071
75. Lopez-Fernandez L, Sanchis M, Navarro-Rodriguez P, Nicolas FE, Silva-Franco F, Guarro J, et al. Understanding mucor circinelloides pathogenesis by comparative genomics and phenotypical studies. Virulence (2018) 9(1):707–20. doi: 10.1080/21505594.2018.1435249
76. Lionakis MS, Netea MG. Candida and host determinants of susceptibility to invasive candidiasis. PLoS Pathog (2013) 9(1):e1003079. doi: 10.1371/journal.ppat.1003079
77. Filler SG, Yeaman MR, Sheppard DC. Tumor necrosis factor inhibition and invasive fungal infections. Clin Infect Dis (2005) 41(Supplement_3):S208–12. doi: 10.1086/430000
78. Ali T, Kaitha S, Mahmood S, Ftesi A, Stone J, Bronze MS. Clinical use of anti-TNF therapy and increased risk of infections. Drug Healthc Patient Saf (2013) 5:79–99. doi: 10.2147/dhps.S28801
79. England H, Summersgill HR, Edye ME, Rothwell NJ, Brough D. Release of interleukin-1α or interleukin-1β depends on mechanism of cell death*. J Biol Chem (2014) 289(23):15942–50. doi: 10.1074/jbc.M114.557561
80. Chamilos G, Lewis RE, Lamaris G, Walsh TJ, Kontoyiannis DP. Zygomycetes hyphae trigger an early, robust proinflammatory response in human polymorphonuclear neutrophils through toll-like receptor 2 induction but display relative resistance to oxidative damage. Antimicrob Agents Chemother (2008) 52(2):722–4. doi: 10.1128/aac.01136-07
Keywords: Mucorales, mucormycosis, phagocytosis, inflammatory response, receptors, cytokines, cell damage, apoptosis
Citation: Montaño DE, Hartung S, Wich M, Ali R, Jungnickel B, von Lilienfeld-Toal M and Voigt K (2022) The TLR-NF-kB axis contributes to the monocytic inflammatory response against a virulent strain of Lichtheimia corymbifera, a causative agent of invasive mucormycosis. Front. Immunol. 13:882921. doi: 10.3389/fimmu.2022.882921
Received: 24 February 2022; Accepted: 20 September 2022;
Published: 13 October 2022.
Edited by:
Carolina Coelho, University of Exeter, United KingdomReviewed by:
Aldo Henrique Tavares, University of Brasilia, BrazilCopyright © 2022 Montaño, Hartung, Wich, Ali, Jungnickel, von Lilienfeld-Toal and Voigt. This is an open-access article distributed under the terms of the Creative Commons Attribution License (CC BY). The use, distribution or reproduction in other forums is permitted, provided the original author(s) and the copyright owner(s) are credited and that the original publication in this journal is cited, in accordance with accepted academic practice. No use, distribution or reproduction is permitted which does not comply with these terms.
*Correspondence: Kerstin Voigt, a2Vyc3Rpbi52b2lndEBsZWlibml6LWhraS5kZQ==
Disclaimer: All claims expressed in this article are solely those of the authors and do not necessarily represent those of their affiliated organizations, or those of the publisher, the editors and the reviewers. Any product that may be evaluated in this article or claim that may be made by its manufacturer is not guaranteed or endorsed by the publisher.
Research integrity at Frontiers
Learn more about the work of our research integrity team to safeguard the quality of each article we publish.