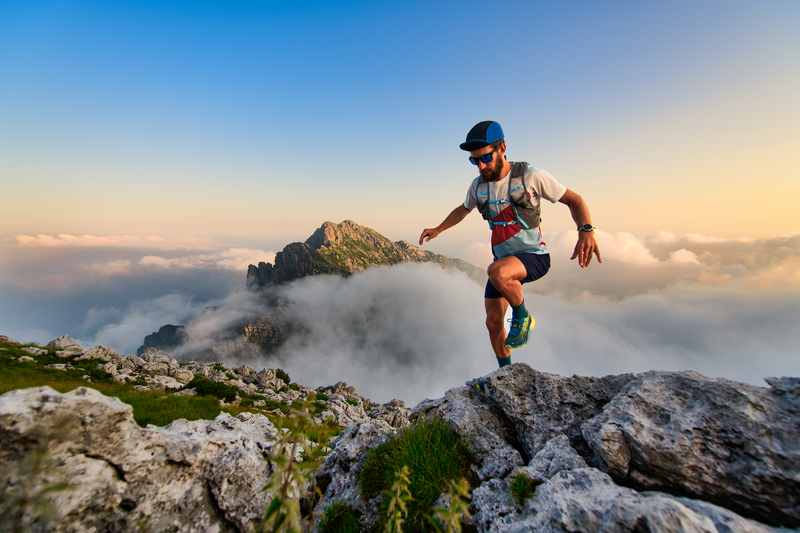
95% of researchers rate our articles as excellent or good
Learn more about the work of our research integrity team to safeguard the quality of each article we publish.
Find out more
MINI REVIEW article
Front. Immunol. , 24 June 2022
Sec. Microbial Immunology
Volume 13 - 2022 | https://doi.org/10.3389/fimmu.2022.878471
This article is part of the Research Topic Beyond Th1: Novel Concepts in tuberculosis vaccine immunology View all 9 articles
Tuberculosis (TB), caused by Mycobacterium tuberculosis is the world’s deadliest bacterial infection, resulting in more than 1.4 million deaths annually. The emergence of drug-resistance to first-line antibiotic therapy poses a threat to successful treatment, and novel therapeutic options are required, particularly for drug-resistant tuberculosis. One modality emerging for TB treatment is therapeutic vaccination. As opposed to preventative vaccination – the aim of which is to prevent getting infected by M. tuberculosis or developing active tuberculosis, the purpose of therapeutic vaccination is as adjunctive treatment of TB or to prevent relapse following cure. Several candidate therapeutic vaccines, using killed whole-cell or live attenuated mycobacteria, mycobacterial fragments and viral vectored vaccines are in current clinical trials. Other modes of passive immunization, including monoclonal antibodies directed against M. tuberculosis antigens are in various pre-clinical stages of development. Here, we will discuss these various therapeutics and their proposed mechanisms of action. Although the full clinical utility of therapeutic vaccination for the treatment of tuberculosis is yet to be established, they hold potential as useful adjunct therapies.
Until the Covid-19 pandemic, Tuberculosis (TB), caused by Mycobacterium tuberculosis (Mtb) was the leading cause of death from a single infectious agent, ranking above HIV or malaria. With the disruption to public health services as a result of the Covid-19 pandemic, the number of deaths due to TB rose for the first time in over 20 years (1, 2), setting back decades of albeit slow, progress. Further threats to eradication of TB as a major public health tragedy include the rise of drug-resistant tuberculosis (DR-TB). There are over half a million cases of rifampicin-resistant TB annually. Lack of access to, and the general lower efficacy of second-line therapeutic regimens results in a disproportionate contribute of DR-TB to poor outcomes (3), and as access to newer agents such bedaquiline and delaminid increases, it will inevitably be associated with a rise of resistance to these agents also (4). It is thought that, globally, there are 19 million individuals living with latent rifampicin-resistant tuberculosis further threatening future control strategies (5, 6). In addition to the specific concerns with respect to DR-TB, and despite the current lengthy standard regimen for drug-sensitive TB (~ 6 months of multiple drugs), approximately 2-10% of patients fail initial therapy or relapse (7), necessitating re-treatment. Treatment of HIV-TB co-infection also has specific considerations, with frequent drug-drug interactions, especially with the keystone rifamycin class and worse outcomes overall. All these considerations have renewed interest in non-antibiotic treatment modalities for tuberculosis.
The usual consideration for immunization is prevention of an infection or disease. Therapeutic vaccination is when an immunomodulatory agent (the vaccine) is administered in the context of already-established disease, either to improve outcomes, shorten treatment duration, or, in the case of TB, prevent relapse (8). In 2018, the WHO defined the target product profile for therapeutic vaccines for TB. They should: (1) reduce the rate of recurrence caused by drug-sensitive and resistant TB, following completion of a full course of drug therapy; (2) increase the proportion of cured patients, in particularly for rifampicin-resistant and extensively drug-resistant TB; (3) shorten therapy duration with the goal of improving compliance and reduce the likelihood of developing drug-resistance (9) and See Figure 1. A further possible definition of therapeutic vaccination in the context of tuberculosis is the administration of a vaccine given to people with evidence of M. tuberculosis exposure, e.g. by a positive interferon-gamma release assay (IGRA+ve), to prevent progression to active tuberculosis. The WHO states that the target population for therapeutic vaccines should include all TB patients, regardless of age, drug sensitivity and co-morbidities. We will survey therapeutic vaccines currently in clinical trials (Table 1), as well as some potential future treatment modalities. Therapeutic TB vaccines in current clinical development fall into several categories: whole killed - and fragmented-cell vaccines; live attenuated vaccines, adjuvanted protein subunit vaccines and viral vectored vaccines.
Figure 1 Overview of selected therapeutic TB vaccines in the clinical pipeline. The initial stages of TB infection involve inhalation of Mtb bacilli into the lung and phagocytosis by resident alveolar macrophages. The human immune system can contain or eliminate Mtb infection in the majority of cases, only a small proportion of exposed individuals go on to develop active tuberculosis. Therapeutic TB vaccines serve as immunotherapeutic adjuncts to chemotherapy and act through modulating host anti-TB immunity. These vaccines are either administrated to potentiate treatment during treatment of active disease (middle) or to prevent recurrence or relapse after standard treatment (right), or to prevent reactivation of latent tuberculosis to active tuberculosis (left). Vaccines that are being developed to improve treatment outcomes in active TB comprise M. vaccae, RUTI, MIP and AERAS-402. Vaccines that prevent relapse and reinfection include H56:IC31 and ID: GLA-SE subunit vaccines, RUTI, BCG, the recombinant BCG vaccine VPM1002 as well as MVA-85A. These candidates are currently in phase 2 or 3 clinical trials in TB patients during or after completion of treatment.
Mycobacterium vaccae (MV), originally discovered in milk and dung from cattle (hence vaccae, cow), is a rapidly growing environmental mycobacterium with poor pathogenic potential in humans. The vaccine was developed using a strain of M. vaccae isolated in a region of Uganda, where it was associated with enhanced protective efficacy of BCG immune responses to tuberculosis (27). Heat-killed preparations of M. vaccae (MV or SRL72) have been extensively studied as adjuncts to standard anti-tuberculous drug therapy for more than decade. It was hypothesized that inactivated MV works by stimulating the host immune responses to antigens shared with Mtb. Preclinical studies showed that mice injected with inactivated MV provoked either a predominantly Th1, or a mixed Th1/Th2 immune response, as well as potentially protective cytotoxic CD8+ T cells that could kill Mtb-infected macrophages (10, 12, 28). Administration of multiple doses series (2-5 doses) of MV, was shown to be safe and immunogenic in a Phase I and II clinical trials in HIV-infected children and adults primed or not with BCG vaccine conducted across multiple sites (29). Moreover, these studies showed that MV vaccination induced relevant immune responses in HIV-infected patients and that may be explained by the decrease of HIV loads in BCG primed and MV recipient individuals suggesting immunization with whole cell vaccine is a promising strategy for the prevention of HIV-associated TB. In an early small, randomized trial, 120 HIV seronegative newly diagnosed pulmonary TB patients were randomized to a single dose of MV versus placebo in addition to standard TB chemotherapy. MV therapy was associated with more rapid sputum culture conversion (i.e. clearance of viable Mtb), and improved radiological markers of disease (27). In addition to markers of cell-mediated immunostimulation, individuals treated with MV had increased antibody titers to the heat shock proteins HSP60 and HSP70 – which are known to be immunostimulatory (30) – in the first month of treatment, as well as raised levels of the cytokines IL-4, IL-10 and TNF-α from ex vivo stimulated PBMCs with inactivated Mtb (11). Of the therapeutic vaccines under consideration, MV is the most advanced in the clinical pipeline. After the initial set of small-scale studies, a single dose of intradermally delivered MV was felt to be insufficient for a robust clinical response, and trial protocols were modified to include multiple doses.
To facilitate delivery, a tablet form of heat-killed MV (termed V7) was formulated and evaluated in a small (but designated phase III) placebo-controlled efficacy trial. Patients with either drug-sensitive or drug-resistant TB were randomized to V7 or placebo daily for the first month of therapy. MV therapy was again associated with rapid sputum culture clearance of Mtb. Notably, MV therapy was also associated with weight gain in patients, and improved markers for drug-induced hepatoxicity. Given that both TB-related weight loss (31) and hepatoxicity (32) are thought to have inflammatory components, these findings are further support of the immunomodulatory effect of MV. The largest study of MV: a phase III trial conducted in China with 10,000 tuberculin skin test positive enrollees to measure efficacy in preventing progression to active tuberculosis completed enrollment in 2017 (NCT01979900) but is yet to report its findings.
In summary, MV has proven immunomodulatory activity in both animal preclinical studies as well as multiple, albeit small, clinical studies. The observed effect on decreasing time to sputum culture clearance appears robust, in multiple studies in many different patient populations. However, definitive evidence for MV in treatment outcome in large-scale studies is still awaited.
MIP comprises killed M. indicus pranii, previously known as Mw – a non-pathogenic mycobacterium closely related to M. avium (33). MIP has been studied as an adjunct therapy for leprosy and has been shown to have both immunoprophylactic and immunotherapeutic effects and the duration of multi-drug therapy in leprosy patients by improving the immune responses to M. leprae (34). In keeping with other whole-cell mycobacterial vaccines, preclinical evaluation was confirmed to reduce organ Mtb bacillary burden in small animal models, associated with increased early cell-mediated immunity, including, notably, increased cytotoxic T cells and followed by a balanced Th1/Th2 responses in later part of the chemotherapy (14).
Clinical studies have focused on “category II TB”, i.e. patients that have either failed therapy, relapsed, or otherwise been lost to follow-up. These patients have high rates of treatment failure, as well as risk to progression to drug-resistant TB (15). In a phase II study of 890 sputum smear positive category II patients, patients were randomized to 6 injections of MIP or placebo. Unfortunately, 248 patients (equally distributed between the intervention and control arms) failed to complete the treatment regimen and were excluded from per protocol analysis. There was a subtle decrease in time to sputum culture conversion in the MIP arm. However, there was no statistically significant different in cure rates – possibly because the high rates of cure (94% in MIP arm and 90% in placebo arm) meant the study was underpowered to detect a small difference. In the two years of follow-up, a total of 34 patients in the MIP arm and 26 in the placebo arm relapsed, which again, was not statistically significant (15). There is an ongoing phase III efficacy and safety trial for preventing pulmonary TB among healthy-household contacts of sputum positive TB patients in India and first results are expected soon (18). In summary, MIP has shown robust evidence of immunostimulation. Evidence of efficacy, however, is still lacking or awaited in either treatment, or prevention of active TB following high risk exposure.
RUTI is composed of liposomes containing detoxified fragments of Mtb that was cultured under conditions of hypoxia/stress, which are known to induce expression of a wide-range of stress-related proteins (35). The rationale is that by inducing an immune response to these antigens, RUTI will have improved efficacy against non-replicating bacteria (NRB), that are hypothesized to contribute to the requirement of prolonged treatment, as well as relapse (36).
In endemic settings, populations at increased risk for latent TB infection can be treated using Isoniazid Preventive Therapy (IPT) for 6-12 months. Although IPT has been demonstrated to reduce the risk of reactivation by 70% in high-risk groups, later studies have shown waning protection as soon as 18 months after short-term INH based regimens in high endemic settings (37). This could be due to the low adherence rates, or re-infection. It is thought that Mtb bacilli in individuals with latent infection survive within foamy macrophages in necrotic granulomata that represent an important immunosuppressive barrier (38). Antibiotic treatment is required to kill replicating bacteria as well as eliminate the outermost layer of foamy macrophages and may allow the egress of new phagocytes. Administration of RUTI in this context was proposed to induce a broad of T cells response that would prevent the reactivation of, or kill the remaining NRB (35).
In small animal studies, administration of RUTI to Mtb-infected guinea pigs and mice, following incomplete chemotherapy resulted in increased Mtb-specific CD4+and CD8+ cellular responses and antibody production, and was associated with decreased bacillary organ burden and lung pathology (39). Furthermore, reduction in post-chemotherapy relapse was reported, in which passive immunization with sera, obtained from mice treated with RUTI, exerted significant protection against reactivation of Mtb infection in SCID mice (40). In human studies, a combined phase I/II clinical trial was conducted to test the tolerability and immunogenicity of different RUTI doses in HIV positive and negative patients after completion of one-month INH treatment (13, 41). The results demonstrated that RUTI vaccination was tolerated and that one inoculation of 25μg of RUTI resulted in poly-antigenic responses, especially against the 16 kDa and 38 kDa antigens, which are biomarkers associated with LTBI. There are no reported efficacy data for RUTI, but small phase II trials (NCT04919239, NCT02711735, NCT05136833) are currently underway to evaluate RUTI as adjunctive therapy for drug sensitive and rifampicin-resistant tuberculosis.
VPM1002 is recombinant BCG-ΔureC::hly, i.e. in which the urease C gene, ureC has been deleted and replaced with the listeriolysin O (LLO) gene from Listeria monocytogenes. Urease was known to reduce phagolysosome acidification and promotes mycobacterial survival (17) Listeriolysin O is a thiol-activated, cholesterol-dependent cytolysin that is only active in acidic environments. Hence deletion of ureC would increase LLO activity (17). In Listeria, LLO is absolutely required for virulence (42), and functions to disrupt the phagosome membrane to allow pathogen escape to the cytosol (43). It has now been demonstrated that Mtb can disrupt the phagosome membrane, in an Esx-1-dependent mechanism (44). Esx-1 is a type VII secretion machinery that is essential for Mtb virulence (45, 46) and is missing in BCG. Hence BCG, unlike Mtb, cannot engage in phagolysosomal escape. By expressing LLO, BCG acquires phagolysosomal escape via a different mechanism, and consequently improves antigen accessibility, in particular for loading onto MHC class I. VPM1002 was demonstrated to enhance both innate and adaptive immunity in animal models, in part via inducing apoptosis, autophagy and stimulation of the NRLP3 inflammasome. Compared with wild-type BCG, VPM1002 also resulted in greater stimulation of humoral immunity and germinal center activation (17). Although the initial clinical studies for VPM1002 have been as a candidate preventative vaccine, one animal study suggests potential as a therapeutic vaccine. Mice were infected with Mtb and then treated with antibiotics for 40 days until viable Mtb was no longer recovered from organs. Mice were then treated with BCG, VPM1002 or saline. When organ bacterial burdens were evaluated at 250 days post initial infection, VPM1002 was clearly superior to BCG or saline, although no mice achieved sterile cure (16). A phase II/III clinical trial with VPM1002 is currently ongoing in India to for the prevention of recurrence of active TB in adults following completion of standard therapy (NCT03152903).
Bacillus Calmette-Guerin, a live attenuated strain of Mycobacterium bovis (M. bovis is part of the M. tuberculosis complex and responsible for cattle TB), is the only licensed TB vaccine and was developed a century ago. It is the world’s most extensively used vaccine. BCG has a wide variation in reported efficacy as a preventative vaccine. Despite expert opinion that BCG may be ineffective as a therapeutic in established active TB infection, possibly because active infection masks BCG-induced immunity (47), several animal studies have suggested that BCG may enhance antibiotic activity in experimental M. tuberculosis infection. In an early study, Dhillon and Mitchinson showed that BCG immunization paradoxically decreased the bactericidal activity of isoniazid in Mtb-infected mouse spleens, but not lungs, and had no effect on rifampicin-mediated killing. However, in the guinea-pig model, BCG increased the activity of both drugs in both lungs and spleens (48). In another guinea-pig study, animals immunized +/- BCG were infected with M. tuberculosis and then treated with a combination of rifampicin, isoniazid and pyrazinamide (RHZ), the core of the standard TB regimen. Although BCG immunization had no additional effect on bacterial organ burden on top of antibiotics, it did prolong survival of the infected animals compared with drug-treatment alone and the combination of BCG and drug treatment was also superior to antibiotics alone in reducing pathology (49).
In the context of clinical studies, a secondary analysis of either BCG or rifampicin alone or in combination for the prevention of leprosy transmission in 21,711 close contacts of 1037 index leprosy patients was performed. The original study was designed to determine the efficacy of rifampicin (against placebo), but the observation that ~40% of participants had a history of BCG vaccination given at infancy (well-balanced in both arms of the trial, although in both arms, a larger proportion of young enrollees had a history of BCG vaccination) allowed for the secondary analysis. BCG given at infancy and rifampicin post contact exposure (in the absence of BCG) had an efficacy of 57 and 58% respectively for prevention of leprosy in contacts. However, the combination of BCG and rifampicin had an increased efficacy of 80% (50). Although no efficacy data are available for TB, BCG revaccination is safe and well tolerated in individuals with evidence of LTBI (51). In a phase I study of 82 HIV-ve adults with a strongly positive tuberculin skin test, indicative of LTBI, and who had visible evidence (by scar) of prior BCG vaccination, participants were randomized either to isoniazid preventative therapy (IPT) followed by BCG revaccination, or BCG revaccination followed by IPT (19). IPT had negligible impact on mycobacteria-specific T cell responses. However, BCG revaccination (regardless of IPT order) was associated with the stimulation of a durable, BCG-reactive NK cell response, which was also detected in BCG-immunized infants (19). Given that BCG revaccination was associated with a moderate reduction in primary infection with M. tuberculosis (52), the relevance of these BCG-reactive NK cells on restricting M. tuberculosis, and whether they are relevant in the context of active or recently treated TB warrant further investigation.
ID93 is comprised of four Mtb proteins expressed in tandem. The candidate antigens were identified in a screen of immunodominant human T cell epitopes that were also immunogenic in mice (53). ID93 is formulated with a synthetic toll like receptor 4 (TLR-4) agonist in a stable oil-in water emulsion known as glucopyranosyl lipid A stable emulsion (GLA-SE). In initial studies as a preventative vaccine either alone or as a booster to priming by BCG, ID93-GLA-SE vaccination induced robust polyfunctional Th1 responses, characterized by antigen-specific IFNγ, TNF-α and IL-2 CD4+ T cells, and was superior to BCG alone in both mice and guinea-pigs (54). In a subsequent study, ID-93+GLA-SE was evaluated for therapeutic vaccination in a mouse model as well as cynomologous macaques. The murine studies used SWR/J mice that, unlike the more widely used C57/BL6 mice do not develop chronic infection, but instead progressively sicken and die after ~100 days unless rescued with antibiotic therapy. Adjunctive treatment with vaccination both increased survival of the mice and shortened the length of antibiotics required to rescue the mice (20). Treatment of the non-human primates with 1 month of rifampicin and isoniazid was insufficient to completely resolve infection when given alone, but when combined with three doses of ID-93+GLA-SE following antibiotic treatment, resolved radiographic evidence of disease (20). Building on these studies, ID-93+GLA-SE has been evaluated in an early-stage phase IIa study for prevention of recurrence/relapse in HIV-positive patients who had recently completed a four-month course of standard chemotherapy for pulmonary tuberculosis (21). The vaccine was well tolerated and induced antigen specific CD4-T cell responses in stimulated whole blood and PBMCs. Moreover, IgG-ID93 specific responses were predominantly IgG1 and IgG3. Since only 61 patients were evaluated in all arms, the study was not powered for efficacy (21).
H56:IC31 is a recombinant fusion protein comprised of three Mtb antigens. The vaccine is formulated in a stabilizing agent containing a TLR-9 agonist as adjuvant and designed to drive Th1 immune response against replicating and non-replicating bacilli (6). H56:IC31 has been shown to prevent Mtb reactivation in nonhuman primates (55, 56). A phase 1 clinical trial, carried out with 25 adults, HIV negative, with or without latent TB infection, showed that H56 vaccine induced a polyfunctional CD4+T cells with memory phenotype that persisted for up to 210 days post vaccination (22). Following evidence of both safety and immunogenicity in a phase I study conducted in HIV negative adults who had recently been successfully treated for drug-sensitive pulmonary TB (23), H56:IC31 underwent a phase I/II study to evaluate it as a host-directed therapy alone or in combination with the cyclooxygenase-2 inhibitor (COX2i) etoricoxib. COX inhibition had been shown to both reduce or exacerbate experimental Mtb infection (57). H56:IC31 was safe and immunogenic, as was etoricoxib, but the combination of vaccination with COX2i resulted in blunted immune responses, suggested the two may be antagonistic as host-directed therapies (58). As with ID93, efficacy data is not yet available, although a phase II study of 900 HIV negative TB patients who have recently completed treatment is currently underway (NCT03512249).
M72 is a subunit vaccine comprising a recombinant fusion protein of two Mtb-derived proteins, Mtb32A and Mtb39A; delivered with the adjuvant AS01E, which has been used in the clinically approved malaria vaccine RTS,S as well as the zoster vaccine Shingrix. A phase IIb trial of 3573 adult participants that were HIV-ve and IGRA+, of whom 3289 were included in the per-protocol efficacy calculation, received either the vaccine or placebo. A total of 39 cases of active tuberculosis were detected in the participants, of which 13 were in the vaccine arm, resulting in a vaccine efficacy of ~50% (59). This was the first demonstration of vaccine efficacy for tuberculosis against development of active TB in the post BCG era, and validated that protein subunit vaccines, which have been developed successfully against viral and extra-cellular bacterial (e.g. the acellular pertussis vaccine) infections, could potentially be effective against an intracellular pathogen. Although the mechanisms of protection are not known, both CD4+ T cell and antibody responses were associated with protection (59).
One potential silver lining from the Covid-19 pandemic has been the remarkable progress in novel vaccine platforms. For the first time, both viral- and mRNA-delivered subunit vaccines (against SARS-CoV-2 spike protein) have not only been evaluated in clinical trials but found to be effective and approved (60). Although mRNA vaccine platforms are only now starting to be investigated for TB, virus-delivered subunit vaccines have been investigated in both preclinical and clinical studies.
MVA is a viral vector comprising a recombinant replication-deficient modified vaccinia virus, Ankara (MVA) that allows insertion of large immunogenic sequences, up to 10kbp, and is efficient at induction of specific T cell responses. Given their safety and immunogenicity profile, MVA vectors have been extensively tested as prophylactic vaccine platform in endemic settings (61). MVA expressing antigen 85A (MVA85A) was shown to induce a robust Ag85A-specific CD4+ and CD8+ T cell responses and protected animals to the same extent as BCG following low-dose aerosol infection (62). The first phase 1 study using MVA85A was performed in healthy volunteers and was found to promote polyfunctional CD4+ T cells, likewise, the vaccine was deemed to be safe in infants, HIV positive individuals and LTBI subjects in endemic populations from Gambia, South Africa and Nigeria (63–65). MVA85A completed phase II clinical trials to evaluate its safety and protective effect in a prime boost regimen in infants of HIV infected mothers followed by selective BCG boost at 8 weeks for HIV negative infants (NCT01650389). The data showed that MVA85A prime vaccination of HIV uninfected newborns was safe and induced a modest antigen specific immune response. A phase I clinical study in UK to investigate the effect of MVA85A, administrated by the aerosol inhaled or intramuscular route in healthy LTBI+ was terminated due to challenges with recruitment in the UK sites (NCT02532036). However, the vaccine has completed a small non-randomized phase I study to evaluate its safety and immunogenicity in 12 healthy volunteers latently infected with TB (NCT00456183) (25). Although this trial was limited by the heterogeneity of the population used and the small sample size, it showed that MVA85A is immunogenic in LTBI individuals as well as in BCG vaccinated but Mtb-uninfected individuals. Moreover, the vaccine induced a persistent Ag85A-specific polyfunctional T cell response until week 24 post vaccination. MVA85A is yet to be tested as an adjunct to standard drugs in MDR/XDR subjects, however in a chronic post-exposure mouse model, it was shown that combination of an antibiotic regimen with MVA harboring ten Mtb antigens (MVATG18598), each representative of different phases of TB disease, induced a robust Th1 response and improved the efficacy of chemotherapy (66).
Adenoviruses remain the most widely utilized platform for vaccine design, given their safety, high efficiency gene transduction and induction of robust immune responses. Human adenovirus serotype 35 (AdHu35) has been used extensively as a preventative vaccine (AERAS-402). The vaccine is a replication deficient adenovirus serotype 35 containing DNA encoding fusion proteins of three Mtb antigens: Ag85A, Ag85B and TB10.4. Both mucosal (intranasal) and intra-muscular administration of AERAS-402 conferred protective immunity against Mtb infection in mice (67). However, despite eliciting a robust immune response in immunized macaques, aerosol administration of AERAS-402 failed to protect the primates from high-dose (~275 colony forming units) challenge with M. tuberculosis (68). To determine the safety and immunogenicity of AERAS-402 in TB patients and to exclude the possibility of vaccine-induced immunopathology, a dose-escalation study in 72 adults who were either in the middle of treatment of active tuberculosis or had recently completed treatment was performed. Overall, the vaccine was well-tolerated and highly immunogenic. One study participant died, but from unrelated causes (broncheoalveolar carcinoma) (26). Nonetheless, immunogenicity does not imply functional immune responses. In a study of BCG-primed, AERAS-402 boosted participants, robust antigen-specific CD4+ and CD8+ T cells were identified in the vaccinated arm. However, the cytotoxic (CD8+) T cells were unable to recognized Mtb-infected dendritic cells (69). These findings are in keeping with other studies suggesting that M. tuberculosis engages in evasion of immunity, possibly via immunodominant responses (70), which further complicates effective vaccine design and discovery. To our knowledge, AERAS-402 is no longer in active clinical development.
Studies with a different adenoviral vector, this time chimpanzee adenovirus serotype-68 (ChAd-68) expressing Mtb antigen 85A was effective as adjunct to chemotherapy in the Balb/C mouse model of infection – but only when given intra-nasally and not when given intra-muscularly (71). These data suggest that route as well as formulation of viral-vectored vaccines may be an important consideration of potential efficacy.
Vaccines: whether cell- or subunit-based, deliver immunogens that then evoke potentially protective immune responses. Alternatively, passive transfer of immune effectors, such as antibodies, can bypass the need to elicit an appropriate immune response. Recombinantly expressed monoclonal antibodies (mAbs) have been used extensively for non-infectious diseases, but their use in infection prior to Covid-19 had been restricted to a few instances such as for respiratory syncytial virus (72), in part due to the cost of goods and manufacture. The efficacy of virus-specific and neutralizing mAbs for Covid-19, especially if given early in onset of the disease has now been amply demonstrated. However, translating these Covid successes to TB is not entirely straightforward. For decades, there was controversy with regards to the potential role of antibody-mediated immunity to TB. Recent evidence, from studies of human antibodies specific for Mtb, suggest that antibodies may well be protective in TB (reviewed in (73)). However, unlike viral infections, where neutralization of virus is the single most, albeit not complete (74) mechanism mediating antibody efficacy, protective anti-TB antibodies rely on Fc-mediated effector functions (75, 76). Since recombinant mAbs may have different Fc properties than naturally occurring antibodies, for example with regards to glycosylation (77), mAbs may possess different effector functions than the original natural antibodies from which they are derived. We recently demonstrated that recombinant mAbs directed against a subunit of the Mtb phosphate transporter were still protective both in human ex vivo as well as murine assays (76), suggesting that recombinant mAbs may be potentially useful tools. Nonetheless, treatment of an acute respiratory infection (i.e. Covid-19) and a chronic pulmonary disease such as TB are not directly comparable, not least due to the requirement for repeated dosing and the associated increased costs. There is also the potential for detrimental immunopathology that would need to be investigated carefully in both preclinical, and eventually, clinical studies.
The idea of therapeutic immunization is not new. Convalescent plasma/serum therapy was one of the most widely used therapeutics for infectious diseases prior to the discovery of antimicrobial drugs (78, 79). There are a plethora of data to support therapeutic vaccination from preclinical models of tuberculosis. Moreover, trials to assess efficacy of therapeutic vaccines are easier and less costly to design than for preventative vaccines, since they can use much smaller numbers of enrollees due to higher event rates. Despite this, hard evidence supporting a role for therapeutic vaccination for clinically-relevant endpoints such as cure and relapse are still lacking. What is clear is that therapeutic vaccination can influence host immune responses. Given that even in cured TB patients, long-term sequelae of disease due to immunopathology is present in a substantial proportion of patients (80, 81) revisiting therapeutic vaccination through the lens of modifying post-cure pathology may offer a new path for using these reagents for treatment of the world’s deadliest bacterial disease.
RB and BJ conceived and wrote the manuscript together. All authors contributed to the article and approved the submitted version.
This work was supported in part by INV-038660 from the Bill & Melinda Gates Foundation. BJ is an Investigator of the Wellcome Trust (207487/C/17/Z).
The authors declare that the research was conducted in the absence of any commercial or financial relationships that could be construed as a potential conflict of interest.
All claims expressed in this article are solely those of the authors and do not necessarily represent those of their affiliated organizations, or those of the publisher, the editors and the reviewers. Any product that may be evaluated in this article, or claim that may be made by its manufacturer, is not guaranteed or endorsed by the publisher.
1. Harding E. ‘WHO Global Progress Report on Tuberculosis Elimination’. Lancet Respir Med (2020) 8:19. doi: 10.1016/S2213-2600(19)30418-7
2. Roberts L. ‘How COVID is Derailing the Fight Against HIV, TB and Malaria’. Nature (2021) 597:314. doi: 10.1038/d41586-021-02469-8
3. Toosky M, Javid B. ‘Novel Diagnostics and Therapeutics for Drug-Resistant Tuberculosis’. Br Med Bull (2014) 110:129–40. doi: 10.1093/bmb/ldu011
4. de Vos M, Ley SD, Wiggins KB, Derendinger B, Dippenaar A, Grobbelaar M, et al. ‘Bedaquiline Microheteroresistance After Cessation of Tuberculosis Treatment’. N Engl J Med (2019) 380:2178–80. doi: 10.1056/NEJMc1815121
5. Migliori GB, Tiberi S, Zumla A, Petersen E, Chakaya JM, Wejse C, et al. ‘Mdr/XDR-TB Management of Patients and Contacts: Challenges Facing the New Decade. The 2020 Clinical Update by the Global Tuberculosis Network’. Int J Infect Dis (2020) 92S:S15–25. doi: 10.1016/j.ijid.2020.01.042
6. Mwaba P, Chakaya JM, Petersen E, Wejse C, Zumla A, Kapata N. ‘Advancing New Diagnostic Tests for Latent Tuberculosis Infection Due to Multidrug-Resistant Strains of Mycobacterium Tuberculosis - End of the Road?’. Int J Infect Dis (2020) 92S:S69–71. doi: 10.1016/j.ijid.2020.02.011
7. Hermans SM, Zinyakatira N, Caldwell J, Cobelens FGJ, Boulle A, Wood R. ‘High Rates of Recurrent Tuberculosis Disease: A Population-Level Cohort Study’. Clin Infect Dis (2021) 72:1919–26. doi: 10.1093/cid/ciaa470
8. Hatherill M, White RG, Hawn TR. ‘Clinical Development of New TB Vaccines: Recent Advances and Next Steps’. Front Microbiol (2019) 10, 3154. doi: 10.3389/fmicb.2019.03154
9. Vekemans J, Brennan MJ, Hatherill M, Schrager L, Fritzell B, Rutkowski K, et al. ‘Preferred Product Characteristics for Therapeutic Vaccines to Improve Tuberculosis Treatment Outcomes: Key Considerations From World Health Organization Consultations’. Vaccine (2020) 38:135–42. doi: 10.1016/j.vaccine.2019.10.072
10. Stanford J, Stanford C, Grange J. ‘Immunotherapy With Mycobacterium Vaccae in the Treatment of Tuberculosis’. Front Biosci (2004) 9:1701–19. doi: 10.2741/1292
11. Dlugovitzky D, Fiorenza G, Farroni M, Bogue C, Stanford C, Stanford J. ‘Immunological Consequences of Three Doses of Heat-Killed Mycobacterium Vaccae in the Immunotherapy of Tuberculosis’. Respir Med (2006) 100:1079–87. doi: 10.1016/j.rmed.2005.09.026
12. Gong WP, Liang Y, Ling YB, Zhang JX, Yang YR, Wang L, et al. ‘Effects of Mycobacterium Vaccae Vaccine in a Mouse Model of Tuberculosis: Protective Action and Differentially Expressed Genes’. Mil Med Res (2020) 7:25. doi: 10.1186/s40779-020-00258-4
13. Nell AS, D'lom E, Bouic P, Sabaté M, Bosser R, Picas J, et al. ‘Safety, Tolerability, and Immunogenicity of the Novel Antituberculous Vaccine RUTI: Randomized, Placebo-Controlled Phase II Clinical Trial in Patients With Latent Tuberculosis Infection’. PloS One (2014) 9:e89612. doi: 10.1371/journal.pone.0089612
14. Gupta A, Ahmad FJ, Ahmad F, Gupta UD, Natarajan M, Katoch V, et al. ‘Efficacy of Mycobacterium Indicus Pranii Immunotherapy as an Adjunct to Chemotherapy for Tuberculosis and Underlying Immune Responses in the Lung’. PloS One (2012) 7:e39215. doi: 10.1371/journal.pone.0039215
15. Sharma SK, Katoch K, Sarin R, Balambal R, Kumar Jain N, Patel N, et al. ‘Efficacy and Safety of Mycobacterium Indicus Pranii as an Adjunct Therapy in Category II Pulmonary Tuberculosis in a Randomized Trial’. Sci Rep (2017) 7, 3354. doi: 10.1038/s41598-017-03514-1
16. Gengenbacher M, Kaiser P, Schuerer S, Lazar D, Kaufmann SH. ‘Post-Exposure Vaccination With the Vaccine Candidate Bacillus Calmette-Guérin Δurec::Hly Induces Superior Protection in a Mouse Model of Subclinical Tuberculosis’. Microbes Infect (2016) 18:364–8. doi: 10.1016/j.micinf.2016.03.005
17. Nieuwenhuizen NE, Kulkarni PS, Shaligram U, Cotton MF, Rentsch CA, Eisele B, et al. ‘The Recombinant Bacille Calmette-Guerin Vaccine VPM1002: Ready for Clinical Efficacy Testing’. Front Immunol (2017) 8, 1147. doi: 10.3389/fimmu.2017.01147
18. Kaufmann SHE. ‘Vaccine Development Against Tuberculosis Over the Last 140 Years: Failure as Part of Success’. Front Microbiol (2021) 12:750124. doi: 10.3389/fmicb.2021.750124
19. Suliman S, Geldenhuys H, Johnson JL, Hughes JE, Smit E, Murphy M, et al. ‘Bacillus Calmette-Guerin (BCG) Revaccination of Adults With Latent Mycobacterium Tuberculosis Infection Induces Long-Lived BCG-Reactive NK Cell Responses’. J Immunol (2016) 197:1100–10. doi: 10.4049/jimmunol.1501996
20. Coler RN, Bertholet S, Pine SO, Orr MT, Reese V, Windish HP, et al. ‘Therapeutic Immunization Against Mycobacterium Tuberculosis is an Effective Adjunct to Antibiotic Treatment’. J Infect Dis (2013) 207:1242–52. doi: 10.1093/infdis/jis425
21. Day TA, Penn-Nicholson A, Luabeya AKK, Fiore-Gartland A, Du Plessis N, Loxton AG, et al. ‘Safety and Immunogenicity of the Adjunct Therapeutic Vaccine ID93 + GLA-SE in Adults Who Have Completed Treatment for Tuberculosis: A Randomised, Double-Blind, Placebo-Controlled, Phase 2a Trial’. Lancet Respir Med (2021) 9:373–86. doi: 10.1016/S2213-2600(20)30319-2
22. Luabeya AK, Kagina BM, Tameris MD, Geldenhuys H, Hoff ST, Shi Z, et al. ‘First-in-Human Trial of the Post-Exposure Tuberculosis Vaccine H56:IC31 in Mycobacterium Tuberculosis Infected and non-Infected Healthy Adults’. Vaccine (2015) 33:4130–40. doi: 10.1016/j.vaccine.2015.06.051
23. Suliman S, Luabeya AKK, Geldenhuys H, Tameris M, Hoff ST, Shi Z, et al. ‘Dose Optimization of H56:IC31 Vaccine for Tuberculosis-Endemic Populations. A Double-Blind, Placebo-Controlled, Dose-Selection Trial’. Am J Respir Crit Care Med (2019) 199:220–31. doi: 10.1164/rccm.201802-0366OC
24. Andersen P, Scriba TJ. ‘Moving Tuberculosis Vaccines From Theory to Practice’. Nat Rev Immunol (2019) 19:550–62. doi: 10.1038/s41577-019-0174-z
25. Sander CR, Pathan AA, Beveridge NE, Poulton I, Minassian A, Alder N, et al. ‘Safety and Immunogenicity of a New Tuberculosis Vaccine, MVA85A, in Mycobacterium Tuberculosis-Infected Individuals’. Am J Respir Crit Care Med (2009) 179:724–33. doi: 10.1164/rccm.200809-1486OC
26. van Zyl-Smit RN, Esmail A, Bateman ME, Dawson R, Goldin J, van Rikxoort E, et al. ‘Safety and Immunogenicity of Adenovirus 35 Tuberculosis Vaccine Candidate in Adults With Active or Previous Tuberculosis. A Randomized Trial’. Am J Respir Crit Care Med (2017) 195:1171–80. doi: 10.1164/rccm.201603-0654OC
27. Johnson JL, Kamya RM, Okwera A, Loughlin AM, Nyole S, Hom DL, et al. ‘Randomized Controlled Trial of Mycobacterium Vaccae Immunotherapy in non-Human Immunodeficiency Virus-Infected Ugandan Adults With Newly Diagnosed Pulmonary Tuberculosis. The Uganda-Case Western Reserve University Research Collaboration’. J Infect Dis (2000) 181:1304–12. doi: 10.1086/315393
28. Rodríguez-Güell E, Agustí G, Corominas M, Cardona PJ, Luquin M, Julián E. ‘Mice With Pulmonary Tuberculosis Treated With Mycobacterium Vaccae Develop Strikingly Enhanced Recall Gamma Interferon Responses to M. Vaccae Cell Wall Skeleton’. Clin Vaccine Immunol (2008) 15:893–6. doi: 10.1128/CVI.00477-07
29. von Reyn CF, Mtei L, Arbeit RD, Waddell R, Cole B, Mackenzie T, et al. ‘Prevention of Tuberculosis in Bacille Calmette-Guérin-Primed, HIV-Infected Adults Boosted With an Inactivated Whole-Cell Mycobacterial Vaccine’. AIDS (2010) 24:675–85. doi: 10.1097/QAD.0b013e3283350f1b
30. MacAry PA, Javid B, Floto RA, Smith KG, Oehlmann W, Singh M, et al. ‘HSP70 Peptide Binding Mutants Separate Antigen Delivery From Dendritic Cell Stimulation’. Immunity (2004) 20:95–106. doi: 10.1016/S1074-7613(03)00357-1
31. Matthys P, Billiau A. ‘Cytokines and Cachexia’. Nutrition (1997) 13:763–70. doi: 10.1016/S0899-9007(97)00185-8
32. Ramappa V, Aithal GP. ‘Hepatotoxicity Related to Anti-Tuberculosis Drugs: Mechanisms and Management’. J Clin Exp Hepatol (2013) 3:37–49. doi: 10.1016/j.jceh.2012.12.001
33. Saini V, Raghuvanshi S, Talwar GP, Ahmed N, Khurana JP, Hasnain SE, et al. ‘Polyphasic Taxonomic Analysis Establishes Mycobacterium Indicus Pranii as a Distinct Species’. PloS One (2009) 4:e6263. doi: 10.1371/journal.pone.0006263
34. Sharma P, Misra RS, Kar HK, Mukherjee A, Poricha D, Kaur H, et al. ‘Mycobacterium W Vaccine, a Useful Adjuvant to Multidrug Therapy in Multibacillary Leprosy: A Report on Hospital Based Immunotherapeutic Clinical Trials With a Follow-Up of 1-7 Years After Treatment’. Lepr Rev (2000) 71:179–92. doi: 10.5935/0305-7518.20000020
35. Cardona PJ, Amat I. ‘[Origin and Development of RUTI, a New Therapeutic Vaccine Against Mycobacterium Tuberculosis Infection]’. Arch Bronconeumol (2006) 42:25–32. doi: 10.1157/13083277
36. Cardona PJ. ‘The Progress of Therapeutic Vaccination With Regard to Tuberculosis’. Front Microbiol (2016) 7, 1536. doi: 10.3389/fmicb.2016.01536
37. Biraro IA, Egesa M, Kimuda S, Smith SG, Toulza F, Levin J, et al. ‘Effect of Isoniazid Preventive Therapy on Immune Responses to Mycobacterium Tuberculosis: An Open Label Randomised, Controlled, Exploratory Study’. BMC Infect Dis (2015) 15:438. doi: 10.1186/s12879-015-1201-8
38. Russell DG, Cardona PJ, Kim MJ, Allain S, Altare F. ‘Foamy Macrophages and the Progression of the Human Tuberculosis Granuloma’. Nat Immunol (2009) 10:943–8. doi: 10.1038/ni.1781
39. Guirado E, Gil O, Cáceres N, Singh M, Vilaplana C, Cardona PJ. ‘Induction of a Specific Strong Polyantigenic Cellular Immune Response After Short-Term Chemotherapy Controls Bacillary Reactivation in Murine and Guinea Pig Experimental Models of Tuberculosis’. Clin Vaccine Immunol (2008) 15:1229–37. doi: 10.1128/CVI.00094-08
40. Guirado E, Amat I, Gil O, Diaz J, Arcos V, Caceres N, et al. ‘Passive Serum Therapy With Polyclonal Antibodies Against Mycobacterium Tuberculosis Protects Against Post-Chemotherapy Relapse of Tuberculosis Infection in SCID Mice’. Microbes Infect (2006) 8:1252–9. doi: 10.1016/j.micinf.2005.12.004
41. Vilaplana C, Montané E, Pinto S, Barriocanal AM, Domenech G, Torres F, et al. ‘Double-Blind, Randomized, Placebo-Controlled Phase I Clinical Trial of the Therapeutical Antituberculous Vaccine RUTI’. Vaccine (2010) 28:1106–16. doi: 10.1016/j.vaccine.2009.09.134
42. Cossart P. ‘The Listeriolysin O Gene: A Chromosomal Locus Crucial for the Virulence of Listeria Monocytogenes’. Infection (1988) 16 Suppl 2:S157–9. doi: 10.1007/BF01639740
43. Dramsi S, Cossart P. ‘Listeriolysin O: A Genuine Cytolysin Optimized for an Intracellular Parasite’. J Cell Biol (2002) 156:943–6. doi: 10.1083/jcb.200202121
44. Houben D, Demangel C, van Ingen J, Perez J, Baldeón L, Abdallah AM, et al. ‘ESX-1-Mediated Translocation to the Cytosol Controls Virulence of Mycobacteria’. Cell Microbiol (2012) 14:1287–98. doi: 10.1111/j.1462-5822.2012.01799.x
45. Stanley SA, Johndrow JE, Manzanillo P, Cox JS. ‘The Type I IFN Response to Infection With Mycobacterium Tuberculosis Requires ESX-1-Mediated Secretion and Contributes to Pathogenesis’. J Immunol (2007) 178:3143–52. doi: 10.4049/jimmunol.178.5.3143
46. Pym AS, Brodin P, Brosch R, Huerre M, Cole ST. ‘Loss of RD1 Contributed to the Attenuation of the Live Tuberculosis Vaccines Mycobacterium Bovis BCG and Mycobacterium Microti’. Mol Microbiol (2002) 46:709–17. doi: 10.1046/j.1365-2958.2002.03237.x
47. Mangtani P, Abubakar I, Ariti C, Beynon R, Pimpin L, Fine PE, et al. ‘Protection by BCG Vaccine Against Tuberculosis: A Systematic Review of Randomized Controlled Trials’. Clin Infect Dis (2014) 58:470–80. doi: 10.1093/cid/cit790
48. Dhillon J, Mitchison DA. ‘Influence of BCG-Induced Immunity on the Bactericidal Activity of Isoniazid and Rifampicin in Experimental Tuberculosis of the Mouse and Guinea-Pig’. Br J Exp Pathol (1989) 70:103–10.
49. Shang S, Shanley CA, Caraway ML, Orme EA, Henao-Tamayo M, Hascall-Dove L, et al. ‘Drug Treatment Combined With BCG Vaccination Reduces Disease Reactivation in Guinea Pigs Infected With Mycobacterium Tuberculosis’. Vaccine (2012) 30:1572–82. doi: 10.1016/j.vaccine.2011.12.114
50. Schuring RP, Richardus JH, Pahan D, Oskam L. ‘Protective Effect of the Combination BCG Vaccination and Rifampicin Prophylaxis in Leprosy Prevention’. Vaccine (2009) 27:7125–8. doi: 10.1016/j.vaccine.2009.09.054
51. Hatherill M, Geldenhuys H, Pienaar B, Suliman S, Chheng P, Debanne SM, et al. ‘Safety and Reactogenicity of BCG Revaccination With Isoniazid Pretreatment in TST Positive Adults’. Vaccine (2014) 32:3982–8. doi: 10.1016/j.vaccine.2014.04.084
52. Nemes E, Geldenhuys H, Rozot V, Rutkowski KT, Ratangee F, Bilek N, et al. ‘Prevention of M. Tuberculosis Infection With H4:IC31 Vaccine or BCG Revaccination’. New Engl J Med (2018) 379:138–49. doi: 10.1056/NEJMoa1714021
53. Bertholet S, Ireton GC, Kahn M, Guderian J, Mohamath R, Stride N, et al. ‘Identification of Human T Cell Antigens for the Development of Vaccines Against Mycobacterium Tuberculosis’. J Immunol (2008) 181:7948–57. doi: 10.4049/jimmunol.181.11.7948
54. Bertholet S, Ireton GC, Ordway DJ, Windish HP, Pine SO, Kahn M, et al. ‘A Defined Tuberculosis Vaccine Candidate Boosts BCG and Protects Against Multidrug-Resistant Mycobacterium Tuberculosis’. Sci Transl Med (2010) 2:53ra74. doi: 10.1126/scitranslmed.3001094
55. Aagaard C, Hoang T, Dietrich J, Cardona PJ, Izzo A, Dolganov G, et al. ‘A Multistage Tuberculosis Vaccine That Confers Efficient Protection Before and After Exposure’. Nat Med (2011) 17:189–94. doi: 10.1038/nm.2285
56. Lin PL, Dietrich J, Tan E, Abalos RM, Burgos J, Bigbee C, et al. ‘The Multistage Vaccine H56 Boosts the Effects of BCG to Protect Cynomolgus Macaques Against Active Tuberculosis and Reactivation of Latent Mycobacterium Tuberculosis Infection’. J Clin Invest (2012) 122:303–14. doi: 10.1172/JCI46252
57. Mortensen R, Clemmensen HS, Woodworth JS, Therkelsen ML, Mustafa T, Tonby K, et al. ‘Cyclooxygenase Inhibitors Impair CD4 T Cell Immunity and Exacerbate’. Commun Biol (2019) 2:288. doi: 10.1038/s42003-019-0530-3
58. Jenum S, Tonby K, Rueegg CS, Rühwald M, Kristiansen MP, Bang P, et al. A Phase I/II Randomized Trial of H56:IC31 Vaccination and Adjunctive Cyclooxygenase-2-Inhibitor Treatment in Tuberculosis Patients’. Nat Commun (2021) 12, 6774. doi: 10.1038/s41467-021-27029-6
59. Tait DR, Hatherill M, Meeren Ovd, Ginsberg AM, Brakel EV, Salaun B, et al. ‘Final Analysis of a Trial of M72/AS01E Vaccine to Prevent Tuberculosis’. New Engl J Med (2019) 381:2429–39. doi: 10.1056/NEJMoa1909953
60. Kyriakidis NC, López-Cortés A, González EV, Grimaldos AB, Prado EO. ‘Sars-CoV-2 Vaccines Strategies: A Comprehensive Review of Phase 3 Candidates’. NPJ Vaccines (2021) 6:28. doi: 10.1038/s41541-021-00292-w
61. Afkhami S, Villela AD, D'Agostino MR, Jeyanathan M, Gillgrass A, Xing Z. ‘Advancing Immunotherapeutic Vaccine Strategies Against Pulmonary Tuberculosis’. Front Immunol (2020) 11:557809. doi: 10.3389/fimmu.2020.557809
62. Williams A, Hatch GJ, Clark SO, Gooch KE, Hatch KA, Hall GA, et al. ‘Evaluation of Vaccines in the EU TB Vaccine Cluster Using a Guinea Pig Aerosol Infection Model of Tuberculosis’. Tuberculosis (Edinb) (2005) 85:29–38. doi: 10.1016/j.tube.2004.09.009
63. McShane H, Pathan AA, Sander CR, Keating SM, Gilbert SC, Huygen K, et al. Recombinant Modified Vaccinia Virus Ankara Expressing Antigen 85A Boosts BCG-Primed and Naturally Acquired Antimycobacterial Immunity in Humans. Nat Med (2004) 10:1240–4. doi: 10.1038/nm1128.
64. Sander CR, Pathan AA, Beveridge NE, Poulton I, Minassian A, Alder N, et al. Safety and Immunogenicity of a New Tuberculosis Vaccine, MVA85A, in Mycobacterium Tuberculosis-Infected Individuals. Am J Respir Crit Care Med. (2009) 179:724–33. doi: 10.1164/rccm.200809-1486OC
65. Hawkridge T, Scriba TJ, Gelderbloem S, Smit E, Tameris M, Moyo S, et al. Safety and Immunogenicity of a New Tuberculosis Vaccine, MVA85A, in Healthy Adults in South Africa. J Infect Dis (2008) 198:544–52. doi: 10.1086/590185
66. Leung-Theung-Long S, Coupet CA, Gouanvic M, Schmitt D, Ray A, Hoffmann C, et al. A Multi-Antigenic MVA Vaccine Increases Efficacy of Combination Chemotherapy Against Mycobacterium Tuberculosis. PLoS One. (2018) 13(5):e0196815. doi: 10.1371/journal.pone.0196815
67. Radosevic K, Wieland CW, Rodriguez A, Weverling GJ, Mintardjo R, Gillissen G, et al. ‘Protective Immune Responses to a Recombinant Adenovirus Type 35 Tuberculosis Vaccine in Two Mouse Strains: CD4 and CD8 T-Cell Epitope Mapping and Role of Gamma Interferon’. Infect Immun (2007) 75:4105–15. doi: 10.1128/IAI.00004-07
68. Darrah PA, Bolton DL, Lackner AA, Kaushal D, Aye PP, Mehra S, et al. ‘Aerosol Vaccination With AERAS-402 Elicits Robust Cellular Immune Responses in the Lungs of Rhesus Macaques But Fails to Protect Against High-Dose Mycobacterium Tuberculosis Challenge’. J Immunol (2014) 193:1799–811. doi: 10.4049/jimmunol.1400676
69. Nyendak M, Swarbrick GM, Duncan A, Cansler M, Huff EW, Hokey D, et al. ‘Adenovirally-Induced Polyfunctional T Cells Do Not Necessarily Recognize the Infected Target: Lessons From a Phase I Trial of the AERAS-402 Vaccine’. Sci Rep (2016) 6:36355. doi: 10.1038/srep36355
70. Sutiwisesak R, Hicks ND, Boyce S, Murphy KC, Papavinasasundaram K, Carpenter SM, et al. ‘A Natural Polymorphism of Mycobacterium Tuberculosis in the esxH Gene Disrupts Immunodomination by the TB10.4-Specific CD8 T Cell Response’. PloS Pathog (2020) 16:e1009000. doi: 10.1371/journal.ppat.1009000
71. Afkhami S, Lai R, D'Agostino M R, Vaseghi-Shanjani M, Zganiacz A, Yao Y, et al. ‘Single-Dose Mucosal Immunotherapy With Chimpanzee Adenovirus-Based Vaccine Accelerates Tuberculosis Disease Control and Limits Its Rebound After Antibiotic Cessation’. J Infect Dis (2019) 220:1355–66. doi: 10.1093/infdis/jiz306
72. Vogel AM, Lennon DR, Broadbent R, Byrnes CA, Grimwood K, Mildenhall L, et al. ‘Palivizumab Prophylaxis of Respiratory Syncytial Virus Infection in High-Risk Infants’. J Paediatr Child Health (2002) 38:550–4. doi: 10.1046/j.1440-1754.2002.00057.x
73. Li H, Javid B. ‘Antibodies and Tuberculosis: Finally Coming of Age?’. Nat Rev Immunol (2018) 18:591–96. doi: 10.1038/s41577-018-0028-0
74. DiLillo DJ, Tan GS, Palese P, Ravetch JV. ‘Broadly Neutralizing Hemagglutinin Stalk-Specific Antibodies Require Fcγr Interactions for Protection Against Influenza Virus In Vivo’. Nat Med (2014) 20:143–51. doi: 10.1038/nm.3443
75. Li H, Wang XX, Wang B, Fu L, Liu G, Lu Y, et al. ‘Latently and Uninfected Healthcare Workers Exposed to TB Make Protective Antibodies Against’. Proc Natl Acad Sci U.S.A. (2017) 114:5023–28. doi: 10.1073/pnas.1611776114
76. Watson A, Li H, Ma B, Weiss R, Bendayan D, Abramovitz L, et al. ‘Human Antibodies Targeting a Mycobacterium Transporter Protein Mediate Protection Against Tuberculosis’. Nat Commun (2021) 12:602. doi: 10.1038/s41467-021-20930-0
77. Lu LL, Chung AW, Rosebrock TR, Ghebremichael M, Yu WH, Grace PS, et al. ‘A Functional Role for Antibodies in Tuberculosis’. Cell (2016) 167:433–43.e14. doi: 10.1016/j.cell.2016.08.072
78. Casadevall A, Scharff MD. ‘Return to the Past: The Case for Antibody-Based Therapies in Infectious Diseases’. Clin Infect Dis (1995) 21:150–61. doi: 10.1093/clinids/21.1.150
79. Glatman-Freedman A, Casadevall A. ‘Serum Therapy for Tuberculosis Revisited: Reappraisal of the Role of Antibody-Mediated Immunity Against Mycobacterium Tuberculosis’. Clin Microbiol Rev (1998) 11:514–32. doi: 10.1128/CMR.11.3.514
80. Nightingale R, Chinoko B, Lesosky M, Rylance SJ, Mnesa B, Banda NPK, et al. ‘Respiratory Symptoms and Lung Function in Patients Treated for Pulmonary Tuberculosis in Malawi: A Prospective Cohort Study’. Thorax (2021). doi: 10.1136/thoraxjnl-2021-217190
Keywords: tuberculosis, mycobacterium, therapeutic vaccines, prevention of recurrence, monoclonal antibody, mRNA vaccine
Citation: Bouzeyen R and Javid B (2022) Therapeutic Vaccines for Tuberculosis: An Overview. Front. Immunol. 13:878471. doi: 10.3389/fimmu.2022.878471
Received: 18 February 2022; Accepted: 26 May 2022;
Published: 24 June 2022.
Edited by:
Rhea Coler, University of Washington, United StatesReviewed by:
Dhiraj Kumar Singh, Texas Biomedical Research Institute, United StatesCopyright © 2022 Bouzeyen and Javid. This is an open-access article distributed under the terms of the Creative Commons Attribution License (CC BY). The use, distribution or reproduction in other forums is permitted, provided the original author(s) and the copyright owner(s) are credited and that the original publication in this journal is cited, in accordance with accepted academic practice. No use, distribution or reproduction is permitted which does not comply with these terms.
*Correspondence: Babak Javid, YmFiYWsuamF2aWRAdWNzZi5lZHU=
Disclaimer: All claims expressed in this article are solely those of the authors and do not necessarily represent those of their affiliated organizations, or those of the publisher, the editors and the reviewers. Any product that may be evaluated in this article or claim that may be made by its manufacturer is not guaranteed or endorsed by the publisher.
Research integrity at Frontiers
Learn more about the work of our research integrity team to safeguard the quality of each article we publish.