- 1Department of Microbiology, College of Medicine, The Catholic University of Korea, Seoul, South Korea
- 2Department of Microbiology, Yonsei University College of Medicine, Seoul, South Korea
- 3Vaccine Innovative Technology ALliance (VITAL)-Korea, Yonsei University, Seoul, South Korea
Live vaccines use attenuated microbes to acquire immunity against pathogens in a safe way. As live attenuated vaccines (LAVs) still maintain infectivity, the vaccination stimulates diverse immune responses by mimicking natural infection. Induction of pathogen-specific antibodies or cell-mediated cytotoxicity provides means of specific protection, but LAV can also elicit unintended off-target effects, termed non-specific effects. Such mechanisms as short-lived genetic interference and non-specific innate immune response or long-lasting trained immunity and heterologous immunity allow LAVs to develop resistance to subsequent microbial infections. Based on their safety and potential for interference, LAVs may be considered as an alternative for immediate mitigation and control of unexpected pandemic outbreaks before pathogen-specific therapeutic and prophylactic measures are deployed.
1 Introduction
As members of the ecosystem, humans encounter an enormous number of microorganisms during their lifetimes. Microorganisms including bacteria, viruses, and parasites are present in the surrounding environment and within the human body. Many microorganisms, especially symbiotic bacteria which contribute to food digestion and immune development, are beneficial but a small fraction, which are termed ‘pathogens,’ can cause infectious disease (1). Infectious disease is the world’s leading cause of death, especially in low-income countries, but vaccines against pathogens effectively contribute to minimizing their impact (2). However, there are no vaccines against many dangerous pathogens. The ongoing coronavirus disease 2019 (COVID-19) outbreak caused by severe acute respiratory syndrome coronavirus 2 (SARS-CoV-2) led to excess mortality in the early stages of the outbreak due to the absence of effective vaccines. The experience with the COVID-19 pandemic points to the need for innovative vaccine platforms that can be adapted quickly upon the emergence of new pathogenic outbreaks.
Vaccine induces antigen-specific immune responses to a certain pathogen by exposing the pathogen’s antigen to an immune system in a safe manner. Although the responses vary by type of vaccine, vaccination can also provide partial protection against phylogenetically close variants due to the similarity of antigenic structures. However, antibody and cell-mediated responses induced by vaccination generally have no reactivity to unrelated pathogens. Moreover, vaccination is recommended ahead of pathogen circulation because adaptive immune response takes time to develop.
Besides the highly selective immunologic nature of vaccines, many studies report beneficial non-specific effects (NSEs) against off-target diseases (3). Unlike traditional vaccine effects, NSEs can be elicited immediately after vaccination and sometimes are perpetuated for longer periods. Live attenuated vaccine (LAV)-induced NSEs have been beneficial to non-infectious diseases such as type 1 diabetes, multiple sclerosis, and cancer (4), but in this review, we focus on the NSEs related to infectious diseases. Also, the review covers the basic concept of superinfection and interference that are important for understanding LAV-induced NSEs.
2 Superinfection
In a microorganism-enriched environment, human infection can involve multiple exogenous pathogens at the same time. Superinfection and coinfection are interchangeable terms that indicate multiple infections in a single host (5). These terms are generally used for pathogenic infections, which are distinct from harmless colonization by indigenous commensal bacteria. The latter do not produce symptoms and are seldom noticeable.
Many superinfections are known to synergistically augment disease severity. Respiratory viral infection by members of Orthomyxoviridae, Paramyxoviridae, and Coronaviridae are frequently associated with secondary bacterial infection (6, 7). Post-influenza secondary bacterial infection, especially with Streptococcus pneumoniae, is clinically important as this superinfection is commonly found in fatal cases (8, 9). Similarly, COVID-19-associated bacterial pneumonia, mostly associated with Klebsiella pneumoniae and Staphylococcus aureus, increase morbidity and mortality (10, 11). Primary infection with respiratory virus stimulates and makes changes in the immune system. Consequently, altered immunity sometimes adversely affects a secondary infection. For example, influenza A virus (IAV) infection induces robust interferon (IFN)-γ secretion and suppresses phagocytosis of alveolar macrophages which are key players in bacterial clearance (12). Similarly, type I IFNs (IFN-I) are important antiviral cytokines but IFN-I produced after IAV infection drives a hyporesponsive immune milieu during secondary bacterial infection (13). Immune responses against mixed infection are difficult to understand because there are so many variables in the course of superinfection. Further studies of the host’s response in a time-dependent manner using animal models are required as the immune response to primary infection constantly changes as the infection progresses. For example, in a mouse model, post-influenza pneumococcal pneumonia has increased susceptibility that doesn’t peak until about 7 days after IAV infection (14).
Secondary bacterial pneumonia, a well-known superinfection, results from serial infections in the lung. It seems natural that pathogenic synergism maximizes when superinfection occurs within the same organ, but in certain superinfections, two infections in different tissues can also be synergistic and aggravate the overall disease. This is common in chronic viral infections. For instance, chronic hepatitis C virus infection is associated with elevated risk for Mycobacterium tuberculosis, S. aureus, and S. pneumoniae infections (15–17). Human immunodeficiency virus (HIV) dramatically affects secondary bacterial infections due to disrupted cellular immunity. The HIV infection studies underscore the alteration of the immune system, an important factor that affects an individual’s susceptibility to superinfection.
3 Interference
3.1 Homologous Interference
Unlike the prevalent synergistic pathology discussed above, pathogens may form an antagonistic relationship, called ‘interference.’ Interference studies have been conducted using defective interfering (DI) virus particles that cannot replicate as they are missing essential genes (18). DI particles were recognized as having greater clinical importance in recent years when they were found to have interfering potential in vivo (19–21). Early studies also focused on the competition between two replication-competent viruses, especially between mutant and their parental wild-type (wt) viruses (22). As mutants largely maintain the original genetic sequences, these viruses can efficiently coinfect the same cell with wt virus and compete for the host’s mechanisms required for their replication. In general, mutant viruses generated by spontaneous or artificial mutation showed superior growth compared to parental wt virus during coinfection (22). The interference was observed in other RNA viruses including poliovirus (22, 23). These studies suggest that interference occurs at earlier steps of viral RNA synthesis. Interference was also reported in DNA viruses, including the temperature-sensitive herpes simplex virus type 1 (HSV-1) mutant that showed interference against a wt strain by delaying viral genome synthesis (24).
The early pioneering studies contributed to establishing the concept of viral interference. Homologous viral interference can be considered ‘genetic interference’ because proliferation of virulent strains is hindered by introduction of suboptimal mutant genomes. Unfortunately, interference at the genetic level based on an in vitro cell culture system has limitations for human disease studies because it is difficult to assess immune-mediated interference.
3.2 Heterologous Interference
Living multicellular organisms provide a niche for growth of both intracellular and extracellular microorganisms. For this reason, heterologous interference is common in humans and animals. Even harmless microorganisms compete with each other for energy sources and sometimes provide ‘colonization resistance’ against pathogenic infection (25, 26). Heterologous interference in living organisms is complicated because they induce counteracting host factors related to innate and adaptive immunity. Despite its complexity, study of heterologous interference is important to understand the pathophysiology of superinfection by unrelated pathogens.
IAV and influenza B virus (IBV) are antigenically distinct but both have significant clinical importance (27). IAV and IBV, like other RNA viruses, frequently yield variant strains that have mutations in antigenic sites. Antibodies against IAV or IBV may cross-react to homologous virus types. For example, IBV hemagglutinin-specific antibodies can provide protection against multiple IBV lineages (28). In contrast, IAV-specific antibodies induced by vaccination are not reactive to heterotypic (i.e., IBV) antigens (29). Thus, antibody-mediated immune response to IAV is not protective against IBV and vice versa. However, attenuated IAV infection provided shot-term protection against IBV in a mouse model (29). Moreover, IAV infection induced protection against IBV in the ferret model and it was achieved within short intervals of less than 7 days (30). Observed immediate protection was associated with decreased lung viral burden of secondarily infected virus. As antigen-specific adaptive immunity takes at least a week to reach the peak level, heterotypic interference should employ other mechanisms, such as innate immunity, to mitigate secondary viral propagation. Another study showed that surviving club cells, a type of lung epithelial cell that secret anti-inflammatory protein (31), are reprogrammed after IAV infection to elicit elevated antiviral responses (32). This antigenically unrelated interference between IAV and IBV was temporary, and animals eventually became susceptible again.
There have been other efforts to prove that IAV infection can provoke non-specific protection against unrelated pathogens. IAV-infected ferrets showed reduced viral burden after secondary respiratory syncytial virus (RSV) infection (33). When 3-, 7-, and 11-day intervals were tested, IAV infection suppressed RSV better during shorter intervals. The heterologous interference was not mediated by adaptive immunity because cross-reactive cellular response was minimal between IAV and RSV. In a mouse model, immediate protection (up to 7~14 days) against RSV was achieved by attenuated infection with IAV. Another study with IAV infection showed interference against the RSV challenge for a longer period (34). In that study, mice were challenged with RSV 5 weeks after IAV infection and showed reduced weight loss and histopathology. As in the ferret model, IAV-infected mice did not develop RSV-specific cellular immunity but transfer of splenocytes from IAV-infected mice resulted in reduced pathology after RSV infection. It was proposed that IAV-specific CD8+ T cells are recruited to RSV-infected lungs to exert bystander activity. This type of protection, called ‘heterologous immunity,’ will be discussed later in this review.
Systemic superinfection of lymphocytic choriomeningitis virus (LCMV) and ectromelia virus (ECTV) in mice also generated heterologous interference (35). When mice were infected with LCMV 1 or 2 days prior to ECTV infection, the coinfection extended survival of mice in an IFN-I–dependent manner; however, infection of LCMV after ECTV infection did not interfere with ECTV infection, indicating that timing of LCMV administration affects the degree of interference. In this case, the CD8+ T cell count in the spleen was comparable between coinfected and ECTV-only infected mice, suggesting immediate heterologous interference was mediated by innate immunity, such as IFN-I, rather than by long-lasting heterologous immunity.
Chronic infection of murine gammaherpesvirus 68 (MHV68) is an animal model for human gammaherpesviruses. MHV68-infected mice showed significantly lower mortality after lethal IAV infection compared to mock-infected control mice (36). The interference lasted for 60 days after the MHV68 infection but had disappeared by day 120. Adoptive transfer of alveolar macrophages isolated from MHV68-infected mice protected recipient mice from IAV challenge. In addition, MHV68 infection induced protection against another virus, bacteria, and malaria infections (37–39). Broad-spectrum heterologous interference achieved by MHV68 further suggests that protection is mediated by non-specific immune responses rather than by antigen-specific immune responses.
4 LAV-Induced NSE
LAV is a conventional prophylactic approach that has long been used against bacterial infections by Mycobacterium and Salmonella, and viral infections by polio, measles, mumps, rubella, varicella zoster, rota, yellow fever, smallpox, and influenza viruses. As the LAV is an infectious agent, people who are vaccinated could be at risk of superinfection, and at the same time be protected from secondary infection by interference (Table 1). Live attenuated influenza vaccine (LAIV) has been used against swine influenza, and superinfection of LAIV and a wt strain was detected in a vaccinated swine population (57). It is likely that humans administered the LAV often experience superinfection until LAV strains are cleared by host’s immune system. Also, human studies of Bacillus Calmette–Guérin (BCG) vaccine suggest that LAVs can elicit beneficial protective effects on subsequent infection by unrelated pathogens (41).
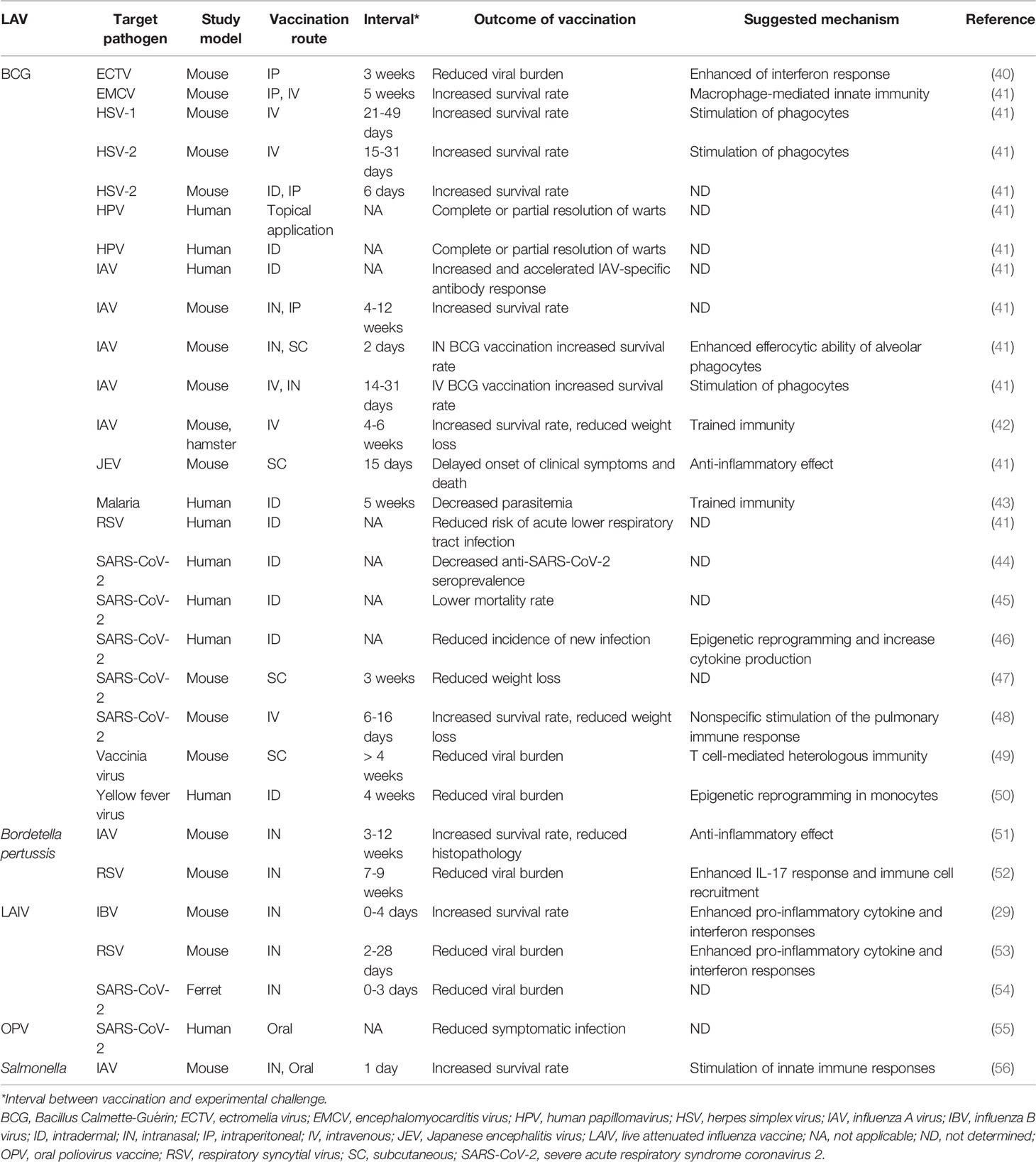
Table 1 Beneficial non-specific effects against unrelated pathogens induced by live attenuated vaccine (LAV) administration in vivo.
4.1 Bacterial LAVs
BCG vaccine is an attenuated Mycobacterium bovis strain developed to protect against tuberculosis. BCG is known for its interference potential against viral infections, including ECTV, encephalomyocarditis virus, HSV-1, HSV-2, human papillomavirus, IAV, Japanese encephalitis virus, RSV, and vaccinia virus (41). Epidemiological studies have shown that all-cause mortality, including non-tuberculous infections, is reduced by BCG vaccination in early life (58–60). BCG also has antifungal effects against Candida albicans and Cryptococcus neoformans (61, 62). Most of the antifungal effects were assessed using immune-disrupted SCID or IFN-γ knock-out mice. Thus, further validation is required.
Similarly, interference against influenza infection by attenuated bacteria is achieved by oral and nasal administration of Salmonella LAV (56). As Salmonella LAV has been widely studied as a mucosal vaccine platform that expresses foreign antigen and is usually introduced orally (14), the potential of interference against infections in different mucosal sites need further study. In other attempts for LAV development, live attenuated Bordetella pertussis vaccine showed protection against influenza and RSV infections (51, 52). Of note, most interference induced by bacterial LAVs was against viral infections.
4.2 Viral LAVs
4.2.1 LAIV
A reverse genetic approach has been frequently used to generate LAIV strains (63, 64), but currently licensed LAIVs were generated by cold-adaptation. Three individual cold-adapted (ca) LAIV backbone strains were prepared by passaging virulent strains at progressively lower temperatures (65–67). These ca backbone strains were used to generate a vaccine strain that contains surface glycoprotein gene segments of circulating virus. For example, hemagglutinin (HA) and neuraminidase (NA) genes of the ca-backbone strain, A/Ann Arbor/6/60 ca (H2N2), were replaced by those of A/Korea/1/82 wt (H3N2) to yield the A/Korea/1/82 ca vaccine strain. As many temperature-sensitive mutant viruses showed homologous interference against parental wt viruses, A/Korea/1/82 ca genes were dominantly synthesized compared with wt genes when these two viruses coinfected MDCK cells (68). The interference of ca LAIV in vivo was confirmed by mixed infection of ferrets (69). Ferrets coinfected with wt and ca vaccine strains produced antibodies to both strains, indicating these two viruses were propagated. While wt virus-infected ferrets showed fever and coryza for 3 days, those symptoms were not observed in wt and ca coinfected ferrets. Interestingly, the interference by ca vaccine (H3N2) was effective for both homosubtypic (H3N2) and heterosubtypic (H1N1) wt challenge. In a double-blind human coinfection study, volunteers who received both wt and ca virus had lower symptom scores than those given only wt virus, although the difference was not statistically significant (70). The series of studies based on A/Ann Arbor/6/60 ca strain extended the use of LAIV from conventional homologous protection to a broader range of influenza virus infections.
4.2.2 Other Viral LAVs
Based on recommended immunization schedules, children receive various vaccines against viral pathogens early in life. Measles vaccine has long been used in low-income countries and children get their first vaccinations at age 9 months. Analysis of 10 cohort studies showed 30% to 85% protective efficacy against all-cause mortality in developing countries (71). NSEs on mortality were particularly effective in children who did not receive neonatal vitamin A (72). Another randomized trial showed that measles vaccine protects against hospital admissions, indicating that NSEs reduce both morbidity and mortality in low-income countries (73). A lower risk of hospital admission was also reported in Danish children vaccinated with measles-mumps-rubella (MMR) vaccine (74). These findings indicate that NSEs conferred by measles vaccine are beneficial regardless of an area’s economic status.
Live enterovirus vaccines (LEVs), including oral polio vaccine (OPV), use nonpathogenic viruses to prevent various enterovirus diseases. While the LEVs successfully control pathogenic enterovirus infection as intended, they also exert prophylactic effects against seasonal influenza (75). OPV is currently recommended for administration at birth and thus can produce NSEs even earlier than measles vaccine. In a randomized trial, OPV showed protection against infant mortality associated with infectious diseases (76). As with MMR vaccinations, OPV was also associated with a lower risk of hospitalization in Denmark (77).
Live attenuated rotavirus vaccine has been proposed as a mechanism to protect against non-rotavirus gastroenteritis (78). However, this concept needs further evaluation because of a conflicting report (79). Overall, accumulated evidence suggests that other viral LAVs may also provide protection against unrelated pathogenic infections.
5 Mechanisms of LAV-Induced NSEs
Beneficial effects of LAV-induced interference suggest its potential use for the control of unrelated infectious diseases that lack efficient prophylactic and therapeutic measures. Although licensed LAVs are confirmed for their safety by clinical trials, new concerns may arise when vaccines are applied for different uses. Superinfection of LAVs and virulent pathogens is unavoidable for interference and may advertently enhance the pathology of secondary infections (80, 81). Therefore, to assure the safety of non-conventional LAV use, it is essential to determine the underlying mechanisms of vaccine-induced NSEs (Figure 1).
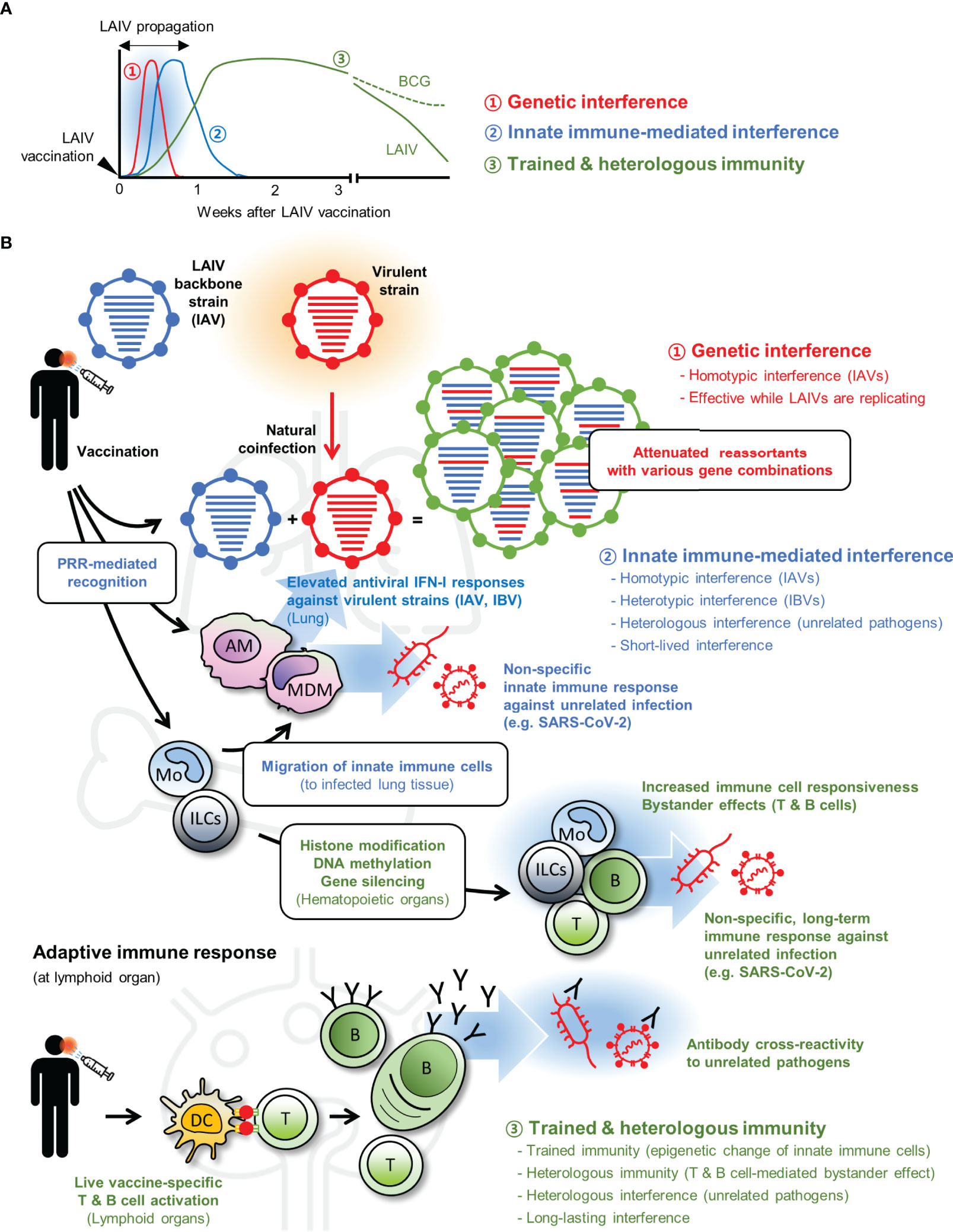
Figure 1 Live attenuated vaccine (LAV)-induced non-specific effects (NSEs). (A) LAIV can induce three different types of interference (1, genetic interference; 2, innate immune-mediated interference; and 3, trained and heterologous immunity) with each protective effect elicited at different time points after vaccination. (B) When virulent strain and LAIV are coinfected, both viruses can infect the same cell and produce progenies with diverse genomic combinations. Generation of suboptimal progenies induces ‘genetic interference’ during early infection (shown in red). At the same time, LAIV vaccination triggers innate immune-mediated interference (shown in blue) via stimulation of pattern recognition receptors (PRRs). Many immune cells, including alveolar macrophage (AM), monocyte (Mo), monocyte-derived macrophage (MDM), elicit antiviral type I interferon (IFN-I) response and provide broad-spectrum protection to homotypic influenza A virus (IAV), heterotypic influenza B virus (IBV), and other heterologous unrelated pathogens. Whereas the innate immune-mediated interference subsides after LAIV clearance, LAIV can induce long-lasting interference (shown in green). LAIV administration triggers epigenetic alteration in innate immune cells including innate lymphoid cells (ILCs) and Mo (trained immunity) and prolonged bystander effects by LAIV-specific T and B cells (heterologous immunity); both contribute to boost host resistance against unrelated pathogens in an antigen-independent manner. In some cases, LAIV-specific antibodies may cross-react with unrelated pathogens. Genetic interference is an LAIV-specific event, but innate immune-mediated interference and long-term interference can be induced by other LAVs. Certain LAVs, such as Bacillus Calmette–Guérin (BCG), can elicit longer trained and heterologous immunity. DC, dendritic cells.
5.1 Genetic Interference
Genetic interference to based on the replication dominance of attenuated virus. For this reason, genetic interference by LAV is observed in interrelated pathogens. In addition to genetic dominance studies using A/Ann Arbor/6/60 ca LAIV, mechanistic analysis of genetic interference was performed with another LAIV backbone strain, X-31 ca (H3N2). When X-31 ca was administered to mice 1 to 4 days before virulent challenge with heterosubtypic A/New Caledonia/20/99 (H1N1), these mice showed reduced weight loss and mortality compared to non-vaccinated control mice (29). Even simultaneous introduction of X-31 ca with virulent virus showed protective effects. As this early protection was achieved without specific antibody responses, which started to increase 5 days after vaccination, interference was analyzed at the genetic level. IAV has eight segmented genomes and coinfection of two viruses enables them to interchange their genes to generate various combinations of reassortant progenies. Attenuated, temperature-sensitive, and ca phenotypes are the consequence of accumulated mutations dispersed in the segmented RNA genome (65, 82). Thus, an increased ratio of reassortant progenies that contain ca-originated gene(s) contributes toward overall attenuation of virulence. The ratio of reassortant virus was 10% to 46% in mice that received both wt and ca viruses at different intervals, indicating active genetic exchanges occur in vivo. Clearly, LAIV can maximize the genetic interference based on the advantage of segmented gene structure.
As shown in interference between virulent IAV and IBV (30), X-31 ca induced protection against heterotypic IBV challenge in mice (29). Basically, genetic interference can be best achieved when genes of two viruses are compatible. Although IBV contains segmented genomes with similarity in terms of protein functions they encode, natural reassortment between influenza A and B viruses has not yet been reported. However, the polymerase complex of IAV and IBV recognize the non-coding terminal sequence of heterotypic genes and the polymerase complex that contains heterotypic subunits are still functional albeit at a low level (83–85). Genetic interference seems clearly to contribute interference between homotypic IAV infections. Although the possibility of heterotypic genetic interference between IAV and IBV were suggested by other studies, it seems other mechanisms, including immediate induction of innate immune response, play a major role.
5.2 Innate Immunity
Innate immunity provides general barrier function because it is designed to respond to a wide range of microbial infections. Therefore, innate immunity induced by certain microorganisms can elicit broad protection against other pathogens. Vaccination with LAIV induces innate immunity similar to natural infection as the vaccine contains every element required for self-replication and actually ‘infects’ the host (86). Although LAIV administration is generally asymptomatic, it elicits sufficient innate immune response (87).
As a prototype LAIV, X-31 ca induced the production of multiple inflammatory cytokines and IFN-I (29, 53). Such inflammatory and IFN responses are initiated via pattern recognition receptors (PRRs). Toll-like receptor (TLR) 3 and 7 are major intracellular sensors for viral RNA genomes and recognize influenza virus infections (88, 89). Mice with defects in TLR signaling were highly susceptible to infection, confirming the crucial role of TLR-mediated innate immunity in protection against IAV (90). When TLR3 and 7 agonists were administered, they elicited immediate protection against IAV challenge as seen with LAIV vaccination (29). Other studies also proved that agonists for TLRs, including TLR2, 3, 4, and 7, induced therapeutic effects on influenza infection (91). When tested for prophylactic effects, TLR agonists protected mice against different strains including H5 avian influenza and 2009 pandemic strains within several days (92–96). Agonists targeting other PRRs, including RIG-I and NOD2, also induced antiviral innate immune responses and protected mice from IAV infection (97, 98). Based on extensive studies that proved the protective innate immune response via PRR stimulation, LAIVs are expected to be sufficiently immunogenic to mitigate subsequent viral infection, even antigenically unrelated pathogens. Indeed, when X-31 ca was administered 2 days before RSV challenge, it significantly suppressed RSV propagation in a TLR3- and TLR7-dependent manner (53).
IFN-I triggers the expression of multiple IFN-stimulated genes (ISGs) that cooperate to inhibit the virus replication cycle in infected cells (99). For example, IFN-induced transmembrane (IFITM) family proteins restrict entry (100), Mx GTPases block early replication of viral genomes (101), and 2’-5’-oligoadenylate synthetase (OAS) family proteins degrade viral genomes by activating the ribonuclease (RNase) L (102). Virus infection is the main stimulator that potentiates cells to produce IFN-I via PRR signaling (88, 103, 104). Likewise, LAIV induces IFN-I in the lung shortly after vaccination (29, 53). Even non-viral stimulation can increase the host’s antiviral status by inducing IFN-I production (105). IFN-I induced by host DNA in a mouse lung fibrosis model attenuated IAV infection (106). It is important to note that ISGs, which can be produced by LAIV and other LAVs, also induce antiviral effects to unrelated viral infections. Furthermore, IFN-I not only provides direct protection to infected cells but also increases the antiviral status of non-infected cells, which together mitigate overall virus propagation in infected hosts.
IFN-γ, another subtype of IFN, is involved in both innate and adaptive immune responses. Vaccination with BCG allows the host to induce prolonged IFN-γ responses that contribute non-specific protection against ECTV and vaccinia virus (40, 49).
5.3 Long-Lasting Immunity
5.3.1 Trained Immunity
Vaccination with BCG maintains a prolonged NSE, and even acute IAV infection maintained NSEs over 1 month (34, 107). The immune mechanism that drives prolonged immune response by LAV seems to differ from those of immediate or short-lived interference. The concept of ‘trained immunity,’ also known as ‘innate immune memory,’ was established based on the observation that innate immune cells of recently challenged hosts retain the elevated responsiveness to subsequent microbial stimulation (108). When a host experiences an initial challenge, innate immune cells, including monocytes, macrophages, and natural killer cells, undergo epigenetic changes and this genetic reprogramming affects how immune cells respond to secondary infection. Bone marrow and spleen are hematopoietic organs where immune cells are differentiated and educated. BCG can directly access the bone marrow upon vaccination and induces epigenetic modification of hematopoietic stem cells (HSCs) in mice (42). These reprogrammed monocytes are protective against M. tuberculosis infection; however, heterologous protection against unrelated pathogens was not tested.
A human study showed that BCG administration trained monocytes to produce more pro-inflammatory cytokines upon unrelated microbial stimulation in a NOD2-dependent manner (61). Macrophages are an important source of immune modulators for pro-inflammatory and anti-inflammatory responses. As monocytes are recruited to the site of infection and can differentiate into monocyte-derived macrophages, alteration of the transcriptomic profile by epigenetic change will significantly affect the pathologic outcome. In consecutive human studies, nonspecific protection by BCG vaccination was proved by experimental infection with attenuated yellow fever virus or malaria (43, 50). Genome-wide epigenetic changes were observed in monocytes and early activation of innate immune cells, including neutrophils, NK cells, and monocytes was observed in BCG-vaccinated adult volunteers. These subjects developed earlier symptoms of malaria infection, but it eventually was associated with lower parasitemia (43). BCG vaccination did not alter the composition of immune cells and their progenitors but reprogrammed gene transcription to draw functional alteration in CD14+ peripheral monocytes (109). Trained immunity lasted more than 90 days. Thus, BCG vaccination may induce prolonged NSEs against pathogenic infections. Because these experimental human studies were in adults, trained immunity induced by early BCG vaccination needs further study.
Of note, BCG vaccination induces immediate interference. Neonatal BCG vaccination induced granulocyte colony-stimulating factor and activated emergency granulopoiesis (110). Elevated neutrophil numbers were associated with reduced mortality by polymicrobial sepsis in vaccinated neonates and the protection was achieved within 3 days. The study indicates that long-lasting NSEs can be achieved quickly after vaccination.
5.3.2 Heterologous Immunity
Heterologous immunity is another term that is used to explain prolonged NSEs, but this phenomenon mainly focuses on lymphocyte responses rather than on innate immune cells. Heterologous immunity may have been induced based on cross-reactive T cell antigens between unrelated pathogens (111–113). While heterologous immunity can provide protection against experimental infections (111, 114), in some cases, heterologous immunity can adversely increase disease severity through cross-reactive CD8+ T cells. Two studies reported that the degree of acute infectious mononucleosis caused by Epstein-Barr virus infection correlated with development of IAV-specific cross-reactive memory T cells (115, 116). Therefore, heterologous immunity induced by LAVs can be beneficial but also can be detrimental during subsequent pathogenic infections through the activation of cross-reactive lymphocytes.
Alternatively, heterologous immunity can interfere with heterologous infection through the antigen-independent bystander effects of lymphocytes. When peripheral blood mononuclear cells were isolated from BCG-vaccinated individuals and stimulated ex vivo with unrelated microbial stimulants, increased production of Th1- and Th17-related cytokines were detected. When mice were infected LCMV, it generated LCMV-specific CD8+ T cells that produced IFN-γ in lungs and draining lymph nodes. Subsequent systemic vaccinia virus infection re-activated these cells to produce increased serum IFN-γ compared with mock-infected control mice (117). Influenza infection also induces similar long-lasting IFN-γ producing CD8+ T cells and suppresses Th2 responses induced by RSV infection (34). These results suggest that LAV administration, in general, boosts Th1 and Th17 responses in the early phase of secondary infection and contributes to attenuation of overall pathology by secondary infection.
Long-lasting NSEs are mediated by mixed contributions of innate and adaptive cell populations. Both mechanisms may induce cellular reprogramming in hematopoietic organs, such as bone marrow and spleen, after primary infection (118). Further studies are required to define the detailed mechanism of how LAV administration communicates with hematopoietic organs to educate immune cells.
6 Use of LAVs for Control of Newly Emerging Viruses
6.1 NSEs Induced by LAVs Against SARS-CoV-2
New viral infectious diseases, including highly pathogenic avian influenza, 2009 pandemic influenza, SARS, and Middle East respiratory syndrome, have evolved during the last two decades. More recently, COVID-19 spread rapidly and yielded more than 481 million confirmed cases as of 29 March 2022. SARS-CoV-2 vaccines were developed in an exceptionally short time period and applied to human use under provisional approval beginning December 2020. COVID-19 caused significant social and health disruptions worldwide in the early stages of the pandemic and options to minimize the disease burden were discussed.
Because of the well-known heterologous protection of BCG vaccination, its potential use for control of COVID-19 was suggested (119, 120). Multiple clinical studies have assessed the impact of BCG vaccination against COVID-19. These fall into two categories (1): the effect of childhood BCG vaccination on COVID-19 and (2) the effect of re-vaccination on COVID-19 in adults. BCG vaccination in childhood was associated with decreased SARS-CoV-2 prevalence or mortality among health care workers (44, 45); however, in clinical studies, the protective effect of re-vaccination with BCG in adults was controversial. One randomized clinical trial showed that BCG vaccination in the elderly was correlated with lower incidence of SARS-CoV-2 infection (46). Yet other studies found no significant protective effect with BCG vaccination (121–123). Similarly, studies of COVID-19 in animal models also produced contradictory results. In studies using mice expressing the human SARS-CoV-2 receptor, administration of BCG vaccine reduced morbidity and mortality upon SARS-CoV-2 infection (47, 48). But in studies with mice, hamsters, and rhesus macaques, BCG vaccination did not protect animals from SARS-CoV-2 infection (124, 125). The results need to be compared in terms of animals used, study methods and administration routes, and BCG strains. Despite the disparate findings, the positive results support the possible use of BCG vaccine for NSE-mediated control of the current pandemic. In addition, one study reported that peptides derived from BCG vaccine induced SARS-CoV-2-specific T cells based on antigen homology (126). That study showed that heterologous immunity can be induced by BCG vaccination.
OPV may also induce heterologous protection (119). Mothers who were passively exposed to OPV by their children had a decreased incidence of COVID-19 infection (55). This suggests that OPV exposure may be one means of lowering the viral load of unrelated viruses in a population. Another attempt to induce heterologous protection using DI poliovirus particles showed promising prophylactic and therapeutic effects against various RNA virus infections including SARS-CoV-2 (127). When mice received the defective poliovirus genome intranasally, DI particles were generated to induce systemic IFN-I–dependent antiviral responses. This successful example of IFN-I–mediated protection suggests LAV-mediated IFN-I production, which is also induced by LAIV, will similarly protect hosts from SARS-CoV-2 infection.
During the influenza season, there were concerns that people infected with COVID-19 might experience comorbidity. Although coinfection with SARS-CoV-2 and influenza did not affect the overall mortality rate, some studies suggested significant increases in morbidity and mortality (128, 129). In a mouse model, coinfection with SARS-CoV-2 and IAV resulted in increased mortality and severe histopathology (130). However, coinfection with SARS-CoV-2 and LAIV did not enhance the histological pathology in the ferret model (54). In the latter study, prior administration of LAIV 3 days before challenge reduced the replication of SARS-CoV-2 from throat swabs, indicating the potential of LAIV as an immediate prophylactic measure for highly pathogenic coronavirus infection.
6.2 Control of Unrelated Pathogens in Future Pandemics
BCG vaccine has been used for more than 100 years and administered to billions of children (131). As planned, BCG vaccination significantly lowered the incidence of tuberculosis while simultaneously providing unintended beneficial effects. The COVID-19 outbreak provided an opportunity to study the NSEs of BCG and other vaccines. Although the effectiveness of LAV-induced NSEs against SARS-CoV-2 needs further study, the evidence of BCG and OPV vaccination-induced interference suggests promising potential therapeutic use of LAV in future pandemics, especially during early stages when vaccines and drug therapies have not been developed.
Results of animal and human studies found that NSE duration differs by type of LAV (Figure 2). For example, BCG vaccines led to long-lasting NSEs while LAIVs typically were associated with short-lived effects. Therefore, two distinct types of NSE-based vaccine programs need to be considered. Long-lasting NSEs induced by BGC and OPV vaccination can be considered as passive outcomes. Epidemiological studies showed that effectiveness of vaccination correlates with population-wide coverage (132). Thus, NSE-based protection will be more effective in communities with higher LAV coverage (133). The beneficial effects associated with long-lasting NSEs will be most striking when sufficient vaccines are provided to countries with poor medical infrastructures (Figure 2A). Pending the distribution of vaccines, we suggest that LAIVs might be a solution for immediate protection against newly emerging infectious pathogens. However, this approach has been proven mostly at the research level and studies are urgently needed to address safety issues related to NSEs resulting from superinfection of the LAIV and the pathogen. There needs to be an assessment of the risk from superinfection and the expected benefits from NSEs. For example, before the availability of vaccines, most COVID-19 fatalities occurred in the elderly. Assuming this scenario is likely in future pandemics, it seems reasonable to selectively administer LAIV to the elderly in communities in which the infection is actively spreading. Another hurdle for immediate use of an NSE strategy is vaccine supply. To solve this problem, planning might focus on alternative uses of stockpiled pre-pandemic and seasonal LAIV (Figure 2B).
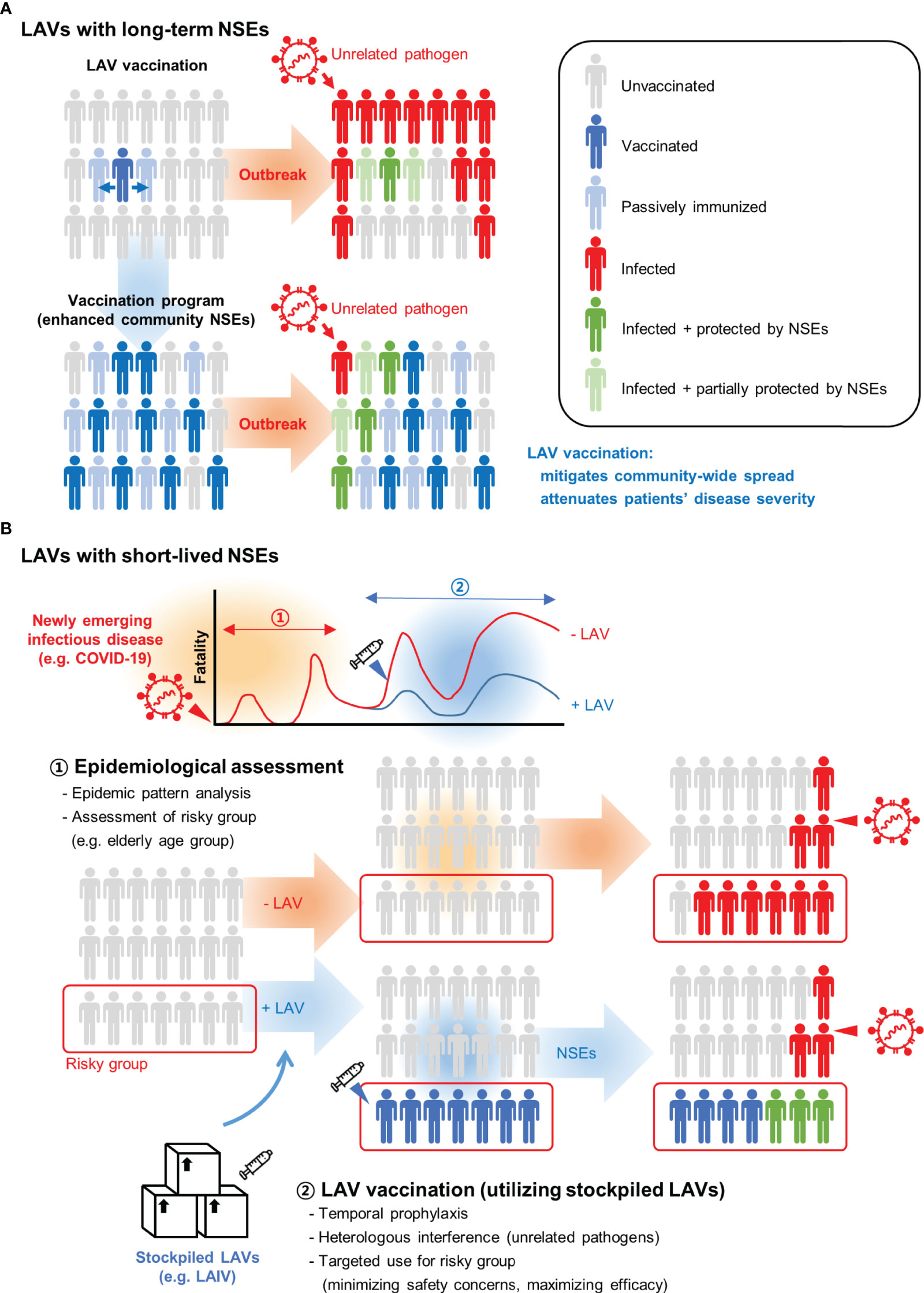
Figure 2 Strategic use of live attenuated vaccines (LAVs) for unrelated pathogen outbreaks. (A) LAVs that elicit beneficial non-specific effects (NSEs) can be transferred from a vaccinated person to passively immunized contacts. Persons exposed to LAV may develop NSEs that attenuate the infection of unrelated pathogens. With higher vaccination rates, community-wide NSEs mitigate the spread of pathogens and limit the impact of an outbreak. (B) LAVs with short-lived NSEs need to be administered during the outbreak. This approach can be effective in the early stage of newly emerging infectious diseases when no effective vaccines and drugs are available. The optimal timing of vaccination must be determined through epidemic pattern analysis. Also, for targeted vaccination, it is important to identify groups at highest risk for fatality. For example, most fatalities were elderly people during the COVID-19 outbreak. A minimal dose of live attenuated influenza vaccine (LAIV) can be selectively administered to an at-risk population to reduce the fatality rate. Stockpiled LAIVs such as pre-pandemic or seasonal vaccines can be considered for alternative use during unexpected new respiratory virus outbreaks.
7 Conclusion
Although a strategic use of LAV-induced NSEs appears promising, the immediate concern is safety and effectiveness in humans. However, new infectious diseases will continue to emerge in the post-COVID-19 era for which no effective therapeutic or prophylactic means are available. Based on extensive research and clinical analysis, we suggest that the use of LAVs not only strengthens the preparedness for future pandemics but also provides a beneficial option for controlling infectious diseases in the absence of licensed vaccines. A balanced view of the pros and cons of use of LAVs must be developed as a basis for a judicious choice of LAV candidates for immediate mitigation and control of unexpected pandemic outbreaks.
Author Contributions
S-US and B-LS contributed to the preparation of the manuscript. All authors contributed to the article and approved the submitted version.
Funding
This work was supported by the Korea Health Technology R&D Project through the Korea Health Industry Development Institute (KHIDI), the Ministry of Health & Welfare (HV20C0070), and the Bio & Medical Technology Development Program of the National Research Foundation (NRF) funded by the Ministry of Science & ICT (2021M3E5E3080927).
Conflict of Interest
The authors declare that the research was conducted in the absence of any commercial or financial relationships that could be construed as a potential conflict of interest.
Publisher’s Note
All claims expressed in this article are solely those of the authors and do not necessarily represent those of their affiliated organizations, or those of the publisher, the editors and the reviewers. Any product that may be evaluated in this article, or claim that may be made by its manufacturer, is not guaranteed or endorsed by the publisher.
References
1. Kamada N, Seo SU, Chen GY, Nunez G. Role of the Gut Microbiota in Immunity and Inflammatory Disease. Nat Rev Immunol (2013) 13(5):321–35. doi: 10.1038/nri3430
2. Plotkin SA, Plotkin SL. The Development of Vaccines: How the Past Led to the Future. Nat Rev Microbiol (2011) 9(12):889–93. doi: 10.1038/nrmicro2668
3. Goodridge HS, Ahmed SS, Curtis N, Kollmann TR, Levy O, Netea MG, et al. Harnessing the Beneficial Heterologous Effects of Vaccination. Nat Rev Immunol (2016) 16(6):392–400. doi: 10.1038/nri.2016.43
4. Covian C, Fernandez-Fierro A, Retamal-Diaz A, Diaz FE, Vasquez AE, Lay MK, et al. BCG-Induced Cross-Protection and Development of Trained Immunity: Implication for Vaccine Design. Front Immunol (2019) 10:2806. doi: 10.3389/fimmu.2019.02806
5. Youngner JS, Quagliana DO. Temperature-Sensitive Mutants of Vesicular Stomatitis Virus Are Conditionally Defective Particles That Interfere With and Are Rescued by Wild-Type Virus. J Virol (1976) 19(1):102–7. doi: 10.1128/JVI.19.1.102-107.1976
6. Crotty MP, Meyers S, Hampton N, Bledsoe S, Ritchie DJ, Buller RS, et al. Epidemiology, Co-Infections, and Outcomes of Viral Pneumonia in Adults: An Observational Cohort Study. Med (Baltimore) (2015) 94(50):e2332. doi: 10.1097/MD.0000000000002332
7. Mirzaei R, Goodarzi P, Asadi M, Soltani A, Aljanabi HAA, Jeda AS, et al. Bacterial Co-Infections With SARS-CoV-2. IUBMB Life (2020) 72(10):2097–111. doi: 10.1002/iub.2356
8. MacIntyre CR, Chughtai AA, Barnes M, Ridda I, Seale H, Toms R, et al. The Role of Pneumonia and Secondary Bacterial Infection in Fatal and Serious Outcomes of Pandemic Influenza A (H1n1)Pdm09. BMC Infect Dis (2018) 18(1):637. doi: 10.1186/s12879-018-3548-0
9. McCullers JA. Insights Into the Interaction Between Influenza Virus and Pneumococcus. Clin Microbiol Rev (2006) 19(3):571–82. doi: 10.1128/CMR.00058-05
10. Catano-Correa JC, Cardona-Arias JA, Porras Mancilla JP, Garcia MT. Bacterial Superinfection in Adults With COVID-19 Hospitalized in Two Clinics in Medellin-Colombia, 2020. PLoS One (2021) 16(7):e0254671. doi: 10.1371/journal.pone.0254671
11. Duployez C, Le Guern R, Tinez C, Lejeune AL, Robriquet L, Six S, et al. Panton-Valentine Leukocidin-Secreting Staphylococcus Aureus Pneumonia Complicating COVID-19. Emerg Infect Dis (2020) 26(8):1939–41. doi: 10.3201/eid2608.201413
12. Sun K, Metzger DW. Inhibition of Pulmonary Antibacterial Defense by Interferon-Gamma During Recovery From Influenza Infection. Nat Med (2008) 14(5):558–64. doi: 10.1038/nm1765
13. Paget C, Trottein F. Mechanisms of Bacterial Superinfection Post-Influenza: A Role for Unconventional T Cells. Front Immunol (2019) 10:336. doi: 10.3389/fimmu.2019.00336
14. Seo SU, Kim JJ, Yang H, Kwon HJ, Yang JY, Curtiss Iii R, et al. Effective Protection Against Secondary Pneumococcal Pneumonia by Oral Vaccination With Attenuated Salmonella Delivering PspA Antigen in Mice. Vaccine (2012) 30(48):6816–23. doi: 10.1016/j.vaccine.2012.09.015
15. Wu PH, Lin YT, Hsieh KP, Chuang HY, Sheu CC. Hepatitis C Virus Infection Is Associated With an Increased Risk of Active Tuberculosis Disease: A Nationwide Population-Based Study. Med (Baltimore) (2015) 94(33):e1328. doi: 10.1097/MD.0000000000001328
16. Marrie TJ, Tyrrell GJ, Majumdar SR, Eurich DT. Concurrent Infection With Hepatitis C Virus and Streptococcus Pneumoniae. Emerg Infect Dis (2017) 23(7):1118–23. doi: 10.3201/eid2307.161858
17. Kaka AS, Filice GA, Kuskowski M, Musher DM. Does Active Hepatitis C Virus Infection Increase the Risk for Infection Due to Staphylococcus Aureus? Eur J Clin Microbiol Infect Dis (2017) 36(7):1217–23. doi: 10.1007/s10096-017-2912-0
18. Huang AS. Defective Interfering Viruses. Annu Rev Microbiol (1973) 27:101–17. doi: 10.1146/annurev.mi.27.100173.000533
19. Dimmock NJ, Marriott AC. In Vivo Antiviral Activity: Defective Interfering Virus Protects Better Against Virulent Influenza A Virus Than Avirulent Virus. J Gen Virol (2006) 87(Pt 5):1259–65. doi: 10.1099/vir.0.81678-0
20. Levi LI, Rezelj VV, Henrion-Lacritick A, Erazo D, Boussier J, Vallet T, et al. Defective Viral Genomes From Chikungunya Virus Are Broad-Spectrum Antivirals and Prevent Virus Dissemination in Mosquitoes. PLoS Pathog (2021) 17(2):e1009110. doi: 10.1371/journal.ppat.1009110
21. Rezelj VV, Carrau L, Merwaiss F, Levi LI, Erazo D, Tran QD, et al. Defective Viral Genomes as Therapeutic Interfering Particles Against Flavivirus Infection in Mammalian and Mosquito Hosts. Nat Commun (2021) 12(1):2290. doi: 10.1038/s41467-021-22341-7
22. Whitaker-Dowling P, Youngner JS. Viral Interference-Dominance of Mutant Viruses Over Wild-Type Virus in Mixed Infections. Microbiol Rev (1987) 51(2):179–91. doi: 10.1128/mr.51.2.179-191.1987
23. Pohjanpelto P, Cooper PD. Interference Between Polioviruses Induced by Strains That Cannot Multiply. Virology (1965) 25:350–7. doi: 10.1016/0042-6822(65)90054-1
24. Jofre JT, Courtney RJ, Schaffer PA. A Dominant Lethal Temperature-Sensitive Mutant of Herpes Simplex Virus Type 1. Virology (1981) 111(1):173–90. doi: 10.1016/0042-6822(81)90663-2
25. Kim YG, Sakamoto K, Seo SU, Pickard JM, Gillilland MG 3rd, Pudlo NA, et al. Neonatal Acquisition of Clostridia Species Protects Against Colonization by Bacterial Pathogens. Science (2017) 356(6335):315–9. doi: 10.1126/science.aag2029
26. Kamada N, Kim YG, Sham HP, Vallance BA, Puente JL, Martens EC, et al. Regulated Virulence Controls the Ability of a Pathogen to Compete With the Gut Microbiota. Science (2012) 336(6086):1325–9. doi: 10.1126/science.1222195
27. Yazici Ozkaya P, Turanli EE, Metin H, Aydin Uysal A, Cicek C, Karapinar B. Severe Influenza Virus Infection in Children Admitted to the Picu: Comparison of Influenza A and Influenza B Virus Infection. J Med Virol (2022) 94(2):575–81. doi: 10.1002/jmv.27400
28. Liu Y, Tan HX, Koutsakos M, Jegaskanda S, Esterbauer R, Tilmanis D, et al. Cross-Lineage Protection by Human Antibodies Binding the Influenza B Hemagglutinin. Nat Commun (2019) 10(1):324. doi: 10.1038/s41467-018-08165-y
29. Seo SU, Lee KH, Byun YH, Kweon MN, Seong BL. Immediate and Broad-Spectrum Protection Against Heterologous and Heterotypic Lethal Challenge in Mice by Live Influenza Vaccine. Vaccine (2007) 25(47):8067–76. doi: 10.1016/j.vaccine.2007.09.012
30. Laurie KL, Guarnaccia TA, Carolan LA, Yan AW, Aban M, Petrie S, et al. Interval Between Infections and Viral Hierarchy Are Determinants of Viral Interference Following Influenza Virus Infection in a Ferret Model. J Infect Dis (2015) 212(11):1701–10. doi: 10.1093/infdis/jiv260
31. Almuntashiri S, Zhu Y, Han Y, Wang X, Somanath PR, Zhang D. Club Cell Secreted Protein CC16: Potential Applications in Prognosis and Therapy for Pulmonary Diseases. J Clin Med (2020) 9(12):4039. doi: 10.3390/jcm9124039
32. Hamilton JR, Sachs D, Lim JK, Langlois RA, Palese P, Heaton NS. Club Cells Surviving Influenza A Virus Infection Induce Temporary Nonspecific Antiviral Immunity. Proc Natl Acad Sci USA (2016) 113(14):3861–6. doi: 10.1073/pnas.1522376113
33. Chan KF, Carolan LA, Korenkov D, Druce J, McCaw J, Reading PC, et al. Investigating Viral Interference Between Influenza A Virus and Human Respiratory Syncytial Virus in a Ferret Model of Infection. J Infect Dis (2018) 218(3):406–17. doi: 10.1093/infdis/jiy184
34. Walzl G, Tafuro S, Moss P, Openshaw PJ, Hussell T. Influenza Virus Lung Infection Protects From Respiratory Syncytial Virus-Induced Immunopathology. J Exp Med (2000) 192(9):1317–26. doi: 10.1084/jem.192.9.1317
35. McAfee MS, Huynh TP, Johnson JL, Jacobs BL, Blattman JN. Interaction Between Unrelated Viruses During In Vivo Co-Infection to Limit Pathology and Immunity. Virology (2015) 484:153–62. doi: 10.1016/j.virol.2015.05.021
36. Saito F, Ito T, Connett JM, Schaller MA, Carson WFT, Hogaboam CM, et al. MHV68 Latency Modulates the Host Immune Response to Influenza A Virus. Inflammation (2013) 36(6):1295–303. doi: 10.1007/s10753-013-9668-1
37. Barton ES, White DW, Cathelyn JS, Brett-McClellan KA, Engle M, Diamond MS, et al. Herpesvirus Latency Confers Symbiotic Protection From Bacterial Infection. Nature (2007) 447(7142):326–9. doi: 10.1038/nature05762
38. Haque A, Rachinel N, Quddus MR, Haque S, Kasper LH, Usherwood E. Co-Infection of Malaria and Gamma-Herpesvirus: Exacerbated Lung Inflammation or Cross-Protection Depends on the Stage of Viral Infection. Clin Exp Immunol (2004) 138(3):396–404. doi: 10.1111/j.1365-2249.2004.02652.x
39. Nguyen Y, McGuffie BA, Anderson VE, Weinberg JB. Gammaherpesvirus Modulation of Mouse Adenovirus Type 1 Pathogenesis. Virology (2008) 380(2):182–90. doi: 10.1016/j.virol.2008.07.031
40. Suenaga T, Okuyama T, Yoshida I, Azuma M. Effect of Mycobacterium Tuberculosis BCG Infection on the Resistance of Mice to Ectromelia Virus Infection: Participation of Interferon in Enhanced Resistance. Infect Immun (1978) 20(1):312–4. doi: 10.1128/iai.20.1.312-314.1978
41. Moorlag S, Arts RJW, van Crevel R, Netea MG. Non-Specific Effects of BCG Vaccine on Viral Infections. Clin Microbiol Infect (2019) 25(12):1473–8. doi: 10.1016/j.cmi.2019.04.020
42. Kaufmann E, Sanz J, Dunn JL, Khan N, Mendonca LE, Pacis A, et al. BCG Educates Hematopoietic Stem Cells to Generate Protective Innate Immunity Against Tuberculosis. Cell (2018) 172(1-2):176–90.e19. doi: 10.1016/j.cell.2017.12.031
43. Walk J, de Bree LCJ, Graumans W, Stoter R, van Gemert GJ, van de Vegte-Bolmer M, et al. Outcomes of Controlled Human Malaria Infection After BCG Vaccination. Nat Commun (2019) 10(1):874. doi: 10.1038/s41467-019-08659-3
44. Rivas MN, Ebinger JE, Wu M, Sun N, Braun J, Sobhani K, et al. BCG Vaccination History Associates With Decreased SARS-CoV-2 Seroprevalence Across a Diverse Cohort of Health Care Workers. J Clin Invest (2021) 131(2):e145157. doi: 10.1172/JCI145157
45. Torun S, Ozkaya S, Sen N, Kanat F, Karaman I, Yosunkaya S, et al. The Relationship Between COVID-19 Severity and Bacillus Calmette-Guerin (BCG)/ Mycobacterium Tuberculosis Exposure History in Healthcare Workers: A Multi-Center Study. Pathog Glob Health (2021) 115(6):405–11. doi: 10.1080/20477724.2021.1927605
46. Giamarellos-Bourboulis EJ, Tsilika M, Moorlag S, Antonakos N, Kotsaki A, Dominguez-Andres J, et al. Activate: Randomized Clinical Trial of BCG Vaccination Against Infection in the Elderly. Cell (2020) 183(2):315–23.e9. doi: 10.1016/j.cell.2020.08.051
47. Counoupas C, Johansen MD, Stella AO, Nguyen DH, Ferguson AL, Aggarwal A, et al. A Single Dose, BCG-Adjuvanted COVID-19 Vaccine Provides Sterilising Immunity Against SARS-CoV-2 Infection. NPJ Vaccines (2021) 6(1):143. doi: 10.1038/s41541-021-00406-4
48. Hilligan KL, Namasivayam S, Clancy CS, O'Mard D, Oland SD, Robertson SJ, et al. Intravenous Administration of BCG Protects Mice Against Lethal SARS-CoV-2 Challenge. J Exp Med (2022) 219(2):e20211862. doi: 10.1084/jem.20211862
49. Mathurin KS, Martens GW, Kornfeld H, Welsh RM. Cd4 T-Cell-Mediated Heterologous Immunity Between Mycobacteria and Poxviruses. J Virol (2009) 83(8):3528–39. doi: 10.1128/JVI.02393-08
50. Arts RJW, Moorlag S, Novakovic B, Li Y, Wang SY, Oosting M, et al. BCG Vaccination Protects Against Experimental Viral Infection in Humans Through the Induction of Cytokines Associated With Trained Immunity. Cell Host Microbe (2018) 23(1):89–100.e5. doi: 10.1016/j.chom.2017.12.010
51. Li R, Lim A, Phoon MC, Narasaraju T, Ng JK, Poh WP, et al. Attenuated Bordetella Pertussis Protects Against Highly Pathogenic Influenza A Viruses by Dampening the Cytokine Storm. J Virol (2010) 84(14):7105–13. doi: 10.1128/JVI.02542-09
52. Schnoeller C, Roux X, Sawant D, Raze D, Olszewska W, Locht C, et al. Attenuated Bordetella Pertussis Vaccine Protects Against Respiratory Syncytial Virus Disease via an IL-17-Dependent Mechanism. Am J Respir Crit Care Med (2014) 189(2):194–202. doi: 10.1164/rccm.201307-1227OC
53. Lee YJ, Lee JY, Jang YH, Seo SU, Chang J, Seong BL. Non-Specific Effect of Vaccines: Immediate Protection Against Respiratory Syncytial Virus Infection by a Live Attenuated Influenza Vaccine. Front Microbiol (2018) 9:83. doi: 10.3389/fmicb.2018.00083
54. Ryan KA, Schewe KE, Crowe J, Fotheringham SA, Hall Y, Humphreys R, et al. Sequential Delivery of LAIV and SARS-CoV-2 in the Ferret Model Can Reduce SARS-CoV-2 Shedding and Does Not Result in Enhanced Lung Pathology. J Infect Dis (2022) 225(3):404–12. doi: 10.1093/infdis/jiab594
55. Habibzadeh F, Sajadi MM, Chumakov K, Yadollahie M, Kottilil S, Simi A, et al. COVID-19 Infection Among Women in Iran Exposed Vs Unexposed to Children Who Received Attenuated Poliovirus Used in Oral Polio Vaccine. JAMA Netw Open (2021) 4(11):e2135044. doi: 10.1001/jamanetworkopen.2021.35044
56. Kamble NM, Hajam IA, Lee JH. Orally Administered Live Attenuated Salmonella Typhimurium Protects Mice Against Lethal Infection With H1N1 Influenza Virus. Vet Microbiol (2017) 201:1–6. doi: 10.1016/j.vetmic.2017.01.006
57. Sharma A, Zeller MA, Li G, Harmon KM, Zhang J, Hoang H, et al. Detection of Live Attenuated Influenza Vaccine Virus and Evidence of Reassortment in the U.S. Swine Population. J Vet Diagn Invest (2020) 32(2):301–11. doi: 10.1177/1040638720907918
58. Biering-Sorensen S, Aaby P, Lund N, Monteiro I, Jensen KJ, Eriksen HB, et al. Early BCG-Denmark and Neonatal Mortality Among Infants Weighing <2500 G: A Randomized Controlled Trial. Clin Infect Dis (2017) 65(7):1183–90. doi: 10.1093/cid/cix525
59. Garly M-L, Martins CL, Balé C, Baldé MA, Hedegaard KL, Gustafson P, et al. BCG Scar and Positive Tuberculin Reaction Associated With Reduced Child Mortality in West Africa. Vaccine (2003) 21(21-22):2782–90. doi: 10.1016/s0264-410x(03)00181-6
60. Prentice S, Nassanga B, Webb EL, Akello F, Kiwudhu F, Akurut H, et al. BCG-Induced Non-Specific Effects on Heterologous Infectious Disease in Ugandan Neonates: An Investigator-Blind Randomised Controlled Trial. Lancet Infect Dis (2021) 21(7):993–1003. doi: 10.1016/s1473-3099(20)30653-8
61. Kleinnijenhuis J, Quintin J, Preijers F, Joosten LA, Ifrim DC, Saeed S, et al. Bacille Calmette-Guerin Induces NOD2-Dependent Nonspecific Protection From Reinfection via Epigenetic Reprogramming of Monocytes. Proc Natl Acad Sci USA (2012) 109(43):17537–42. doi: 10.1073/pnas.1202870109
62. Walzl G, Humphreys IR, Marshall BG, Edwards L, Openshaw PJ, Shaw RJ, et al. Prior Exposure to Live Mycobacterium Bovis BCG Decreases Cryptococcus Neoformans-Induced Lung Eosinophilia in a Gamma Interferon-Dependent Manner. Infect Immun (2003) 71(6):3384–91. doi: 10.1128/IAI.71.6.3384-3391.2003
63. Donelan NR, Basler CF, Garcia-Sastre A. A Recombinant Influenza A Virus Expressing an RNA-Binding-Defective NS1 Protein Induces High Levels of Beta Interferon and Is Attenuated in Mice. J Virol (2003) 77(24):13257–66. doi: 10.1128/jvi.77.24.13257-13266.2003
64. Xie H, Liu TM, Lu X, Wu Z, Belser JA, Katz JM, et al. A Live Attenuated H1N1 M1 Mutant Provides Broad Cross-Protection Against Influenza A Viruses, Including Highly Pathogenic a/Vietnam/1203/2004, in Mice. J Infect Dis (2009) 200(12):1874–83. doi: 10.1086/648405
65. Lee KH, Seo SU, Song JM, Lee CM, Kim HA, Seong BL. Characterization of Live Influenza Vaccine Donor Strain Derived From Cold-Adaptation of X-31 Virus. Vaccine (2006) 24(11):1966–74. doi: 10.1016/j.vaccine.2005.10.051
66. Maassab HF. Adaptation and Growth Characteristics of Influenza Virus at 25 Degrees C. Nature (1967) 213(5076):612–4. doi: 10.1038/213612a0
67. van Voorthuizen F, Jens D, Saes F. Characterization and Clinical Evaluation of Live Influenza A Vaccine Prepared From a Recombinant of the A/USSR/92/77 (H1N1) and the Cold-Adapted A/Ann Arbor/6/60 (H2N2) Strains. Antiviral Res (1981) 1(2):107–22. doi: 10.1016/0166-3542(81)90037-1
68. Maloy ML, Whitaker-Dowling P, Youngner JS. Dominance of Cold-Adapted Influenza A Virus Over Wild-Type Viruses Is at the Level of RNA Synthesis. Virology (1994) 205(1):44–50. doi: 10.1006/viro.1994.1618
69. Whitaker-Dowling P, Maassab HF, Youngner JS. Dominant-Negative Mutants as Antiviral Agents: Simultaneous Infection With the Cold-Adapted Live-Virus Vaccine for Influenza A Protects Ferrets From Disease Produced by Wild-Type Influenza a. J Infect Dis (1991) 164(6):1200–2. doi: 10.1093/infdis/164.6.1200
70. Youngner JS, Treanor JJ, Betts RF, Whitaker-Dowling P. Effect of Simultaneous Administration of Cold-Adapted and Wild-Type Influenza A Viruses on Experimental Wild-Type Influenza Infection in Humans. J Clin Microbiol (1994) 32(3):750–4. doi: 10.1128/jcm.32.3.750-754.1994
71. Aaby P, Samb B, Simondon F, Seck AM, Knudsen K, Whittle H. Non-Specific Beneficial Effect of Measles Immunisation: Analysis of Mortality Studies From Developing Countries. BMJ (1995) 311(7003):481–5. doi: 10.1136/bmj.311.7003.481
72. Aaby P, Martins CL, Garly ML, Bale C, Andersen A, Rodrigues A, et al. Non-Specific Effects of Standard Measles Vaccine at 4.5 and 9 Months of Age on Childhood Mortality: Randomised Controlled Trial. BMJ (2010) 341:c6495. doi: 10.1136/bmj.c6495
73. Martins CL, Benn CS, Andersen A, Bale C, Schaltz-Buchholzer F, Do VA, et al. A Randomized Trial of a Standard Dose of Edmonston-Zagreb Measles Vaccine Given at 4.5 Months of Age: Effect on Total Hospital Admissions. J Infect Dis (2014) 209(11):1731–8. doi: 10.1093/infdis/jit804
74. Sorup S, Benn CS, Poulsen A, Krause TG, Aaby P, Ravn H. Live Vaccine Against Measles, Mumps, and Rubella and the Risk of Hospital Admissions for Nontargeted Infections. JAMA (2014) 311(8):826–35. doi: 10.1001/jama.2014.470
75. Voroshilova MK. Potential Use of Nonpathogenic Enteroviruses for Control of Human Disease. Prog Med Virol (1989) 36:191–202.
76. Lund N, Andersen A, Hansen AS, Jepsen FS, Barbosa A, Biering-Sorensen S, et al. The Effect of Oral Polio Vaccine at Birth on Infant Mortality: A Randomized Trial. Clin Infect Dis (2015) 61(10):1504–11. doi: 10.1093/cid/civ617
77. Sorup S, Stensballe LG, Krause TG, Aaby P, Benn CS, Ravn H. Oral Polio Vaccination and Hospital Admissions With Non-Polio Infections in Denmark: Nationwide Retrospective Cohort Study. Open Forum Infect Dis (2016) 3(1):ofv204. doi: 10.1093/ofid/ofv204
78. Benedicto-Matambo P, Bines JE, Malamba-Banda C, Shawa IT, Barnes K, Kamng'ona AW, et al. Leveraging Beneficial Off-Target Effects of Live-Attenuated Rotavirus Vaccines. Vaccines (Basel) (2022) 10(3):418. doi: 10.3390/vaccines10030418
79. Grant L, Watt J, Moulton L, Weatherholtz R, Reid R, Santosham M, et al. Lack of Nonspecific Protection Against All-Cause Nonrotavirus Gastroenteritis by Vaccination With Orally Administered Rotavirus Vaccine. J Pediatr Gastroenterol Nutr (2013) 56(6):635–40. doi: 10.1097/MPG.0b013e318287c5cc
80. Mina MJ, McCullers JA, Klugman KP. Live Attenuated Influenza Vaccine Enhances Colonization of Streptococcus Pneumoniae and Staphylococcus Aureus in Mice. mBio (2014) 5(1):e01040-13. doi: 10.1128/mBio.01040-13
81. Sharma S, Thomas PG. The Two Faces of Heterologous Immunity: Protection or Immunopathology. J Leukoc Biol (2014) 95(3):405–16. doi: 10.1189/jlb.0713386
82. Lee KH, Youn JW, Kim HJ, Seong BL. Identification and Characterization of Mutations in the High Growth Vaccine Strain of Influenza Virus. Arch Virol (2001) 146(2):369–77. doi: 10.1007/s007050170181
83. Baker SF, Nogales A, Finch C, Tuffy KM, Domm W, Perez DR, et al. Influenza A and B Virus Intertypic Reassortment Through Compatible Viral Packaging Signals. J Virol (2014) 88(18):10778–91. doi: 10.1128/JVI.01440-14
84. Iwatsuki-Horimoto K, Hatta Y, Hatta M, Muramoto Y, Chen H, Kawaoka Y, et al. Limited Compatibility Between the RNA Polymerase Components of Influenza Virus Type A and B. Virus Res (2008) 135(1):161–5. doi: 10.1016/j.virusres.2008.03.010
85. Wunderlich K, Juozapaitis M, Manz B, Mayer D, Gotz V, Zohner A, et al. Limited Compatibility of Polymerase Subunit Interactions in Influenza A and B Viruses. J Biol Chem (2010) 285(22):16704–12. doi: 10.1074/jbc.M110.102533
86. Rajao DS, Perez DR. Universal Vaccines and Vaccine Platforms to Protect Against Influenza Viruses in Humans and Agriculture. Front Microbiol (2018) 9:123. doi: 10.3389/fmicb.2018.00123
87. Fischer WA 2nd, Chason KD, Brighton M, Jaspers I. Live Attenuated Influenza Vaccine Strains Elicit a Greater Innate Immune Response Than Antigenically-Matched Seasonal Influenza Viruses During Infection of Human Nasal Epithelial Cell Cultures. Vaccine (2014) 32(15):1761–7. doi: 10.1016/j.vaccine.2013.12.069
88. Diebold SS, Kaisho T, Hemmi H, Akira S, Reis e Sousa C. Innate Antiviral Responses by Means of TLR7-Mediated Recognition of Single-Stranded RNA. Science (2004) 303(5663):1529–31. doi: 10.1126/science.1093616
89. Guillot L, Le Goffic R, Bloch S, Escriou N, Akira S, Chignard M, et al. Involvement of Toll-Like Receptor 3 in the Immune Response of Lung Epithelial Cells to Double-Stranded RNA and Influenza A Virus. J Biol Chem (2005) 280(7):5571–80. doi: 10.1074/jbc.M410592200
90. Seo SU, Kwon HJ, Song JH, Byun YH, Seong BL, Kawai T, et al. MyD88 Signaling Is Indispensable for Primary Influenza A Virus Infection But Dispensable for Secondary Infection. J Virol (2010) 84(24):12713–22. doi: 10.1128/JVI.01675-10
91. Shinya K, Okamura T, Sueta S, Kasai N, Tanaka M, Ginting TE, et al. Toll-Like Receptor Pre-Stimulation Protects Mice Against Lethal Infection With Highly Pathogenic Influenza Viruses. Virol J (2011) 8:97. doi: 10.1186/1743-422X-8-97
92. Bae EH, Seo SH, Kim CU, Jang MS, Song MS, Lee TY, et al. Bacterial Outer Membrane Vesicles Provide Broad-Spectrum Protection Against Influenza Virus Infection via Recruitment and Activation of Macrophages. J Innate Immun (2019) 11(4):316–29. doi: 10.1159/000494098
93. Leiva-Juarez MM, Kirkpatrick CT, Gilbert BE, Scott B, Tuvim MJ, Dickey BF, et al. Combined Aerosolized Toll-Like Receptor Ligands Are an Effective Therapeutic Agent Against Influenza Pneumonia When Co-Administered With Oseltamivir. Eur J Pharmacol (2018) 818:191–7. doi: 10.1016/j.ejphar.2017.10.035
94. Mifsud EJ, Tan AC, Reading PC, Jackson DC. Mapping the Pulmonary Environment of Animals Protected From Virulent H1N1 Influenza Infection Using the TLR-2 Agonist Pam(2)Cys. Immunol Cell Biol (2016) 94(2):169–76. doi: 10.1038/icb.2015.81
95. Tuvim MJ, Gilbert BE, Dickey BF, Evans SE. Synergistic TLR2/6 and TLR9 Activation Protects Mice Against Lethal Influenza Pneumonia. PLoS One (2012) 7(1):e30596. doi: 10.1371/journal.pone.0030596
96. Wu CC, Crain B, Yao S, Sabet M, Lao FS, Tawatao RI, et al. Innate Immune Protection Against Infectious Diseases by Pulmonary Administration of a Phospholipid-Conjugated TLR7 Ligand. J Innate Immun (2014) 6(3):315–24. doi: 10.1159/000355217
97. Le Bel M, Gosselin J. Leukotriene B4 Enhances NOD2-Dependent Innate Response Against Influenza Virus Infection. PLoS One (2015) 10(10):e0139856. doi: 10.1371/journal.pone.0139856
98. Lin L, Liu Q, Berube N, Detmer S, Zhou Y. 5'-Triphosphate-Short Interfering RNA: Potent Inhibition of Influenza A Virus Infection by Gene Silencing and RIG-I Activation. J Virol (2012) 86(19):10359–69. doi: 10.1128/JVI.00665-12
99. Schoggins JW. Interferon-Stimulated Genes: What Do They All Do? Annu Rev Virol (2019) 6(1):567–84. doi: 10.1146/annurev-virology-092818-015756
100. Brass AL, Huang IC, Benita Y, John SP, Krishnan MN, Feeley EM, et al. The IFITM Proteins Mediate Cellular Resistance to Influenza A H1N1 Virus, West Nile Virus, and Dengue Virus. Cell (2009) 139(7):1243–54. doi: 10.1016/j.cell.2009.12.017
101. Haller O, Kochs G, Weber F. Interferon, Mx, and Viral Countermeasures. Cytokine Growth Factor Rev (2007) 18(5-6):425–33. doi: 10.1016/j.cytogfr.2007.06.001
102. Silverman RH. Viral Encounters With 2',5'-Oligoadenylate Synthetase and RNase L During the Interferon Antiviral Response. J Virol (2007) 81(23):12720–9. doi: 10.1128/JVI.01471-07
103. Ioannidis I, Ye F, McNally B, Willette M, Flano E. Toll-Like Receptor Expression and Induction of Type I and Type III Interferons in Primary Airway Epithelial Cells. J Virol (2013) 87(6):3261–70. doi: 10.1128/JVI.01956-12
104. Matsumoto M, Oshiumi H, Seya T. Antiviral Responses Induced by the TLR3 Pathway. Rev Med Virol (2011) 21(2):67–77. doi: 10.1002/rmv.680
105. Ivashkiv LB, Donlin LT. Regulation of Type I Interferon Responses. Nat Rev Immunol (2014) 14(1):36–49. doi: 10.1038/nri3581
106. Seo SU, Jeong JH, Baek BS, Choi JM, Choi YS, Ko HJ, et al. Bleomycin-Induced Lung Injury Increases Resistance to Influenza Virus Infection in a Type I Interferon-Dependent Manner. Front Immunol (2021) 12:697162. doi: 10.3389/fimmu.2021.697162
107. de Castro MJ, Pardo-Seco J, Martinon-Torres F. Nonspecific (Heterologous) Protection of Neonatal BCG Vaccination Against Hospitalization Due to Respiratory Infection and Sepsis. Clin Infect Dis (2015) 60(11):1611–9. doi: 10.1093/cid/civ144
108. Netea MG, Joosten LA, Latz E, Mills KH, Natoli G, Stunnenberg HG, et al. Trained Immunity: A Program of Innate Immune Memory in Health and Disease. Science (2016) 352(6284):aaf1098. doi: 10.1126/science.aaf1098
109. Cirovic B, de Bree LCJ, Groh L, Blok BA, Chan J, van der Velden W, et al. BCG Vaccination in Humans Elicits Trained Immunity via the Hematopoietic Progenitor Compartment. Cell Host Microbe (2020) 28(2):322–34.e5. doi: 10.1016/j.chom.2020.05.014
110. Brook B, Harbeson DJ, Shannon CP, Cai B, He D, Ben-Othman R, et al. BCG Vaccination-Induced Emergency Granulopoiesis Provides Rapid Protection From Neonatal Sepsis. Sci Transl Med (2020) 12(542):eaax4517. doi: 10.1126/scitranslmed.aax4517
111. Selin LK, Brehm MA, Naumov YN, Cornberg M, Kim SK, Clute SC, et al. Memory of Mice and Men: CD8+ T-Cell Cross-Reactivity and Heterologous Immunity. Immunol Rev (2006) 211:164–81. doi: 10.1111/j.0105-2896.2006.00394.x
112. Wedemeyer H, Mizukoshi E, Davis AR, Bennink JR, Rehermann B. Cross-Reactivity Between Hepatitis C Virus and Influenza A Virus Determinant-Specific Cytotoxic T Cells. J Virol (2001) 75(23):11392–400. doi: 10.1128/JVI.75.23.11392-11400.2001
113. Welsh RM, Che JW, Brehm MA, Selin LK. Heterologous Immunity Between Viruses. Immunol Rev (2010) 235(1):244–66. doi: 10.1111/j.0105-2896.2010.00897.x
114. Che JW, Selin LK, Welsh RM. Evaluation of Non-Reciprocal Heterologous Immunity Between Unrelated Viruses. Virology (2015) 482:89–97. doi: 10.1016/j.virol.2015.03.002
115. Aslan N, Watkin LB, Gil A, Mishra R, Clark FG, Welsh RM, et al. Severity of Acute Infectious Mononucleosis Correlates With Cross-Reactive Influenza CD8 T-Cell Receptor Repertoires. mBio (2017) 8(6):e01841-17. doi: 10.1128/mBio.01841-17
116. Cornberg M, Clute SC, Watkin LB, Saccoccio FM, Kim SK, Naumov YN, et al. CD8 T Cell Cross-Reactivity Networks Mediate Heterologous Immunity in Human EBV and Murine Vaccinia Virus Infections. J Immunol (2010) 184(6):2825–38. doi: 10.4049/jimmunol.0902168
117. Chen HD, Fraire AE, Joris I, Brehm MA, Welsh RM, Selin LK. Memory CD8+ T Cells in Heterologous Antiviral Immunity and Immunopathology in the Lung. Nat Immunol (2001) 2(11):1067–76. doi: 10.1038/ni727
118. Netea MG, Dominguez-Andres J, Barreiro LB, Chavakis T, Divangahi M, Fuchs E, et al. Defining Trained Immunity and Its Role in Health and Disease. Nat Rev Immunol (2020) 20(6):375–88. doi: 10.1038/s41577-020-0285-6
119. Chumakov K, Benn CS, Aaby P, Kottilil S, Gallo R. Can Existing Live Vaccines Prevent COVID-19? Science (2020) 368(6496):1187–8. doi: 10.1126/science.abc4262
120. O'Neill LAJ, Netea MG. BCG-Induced Trained Immunity: Can It Offer Protection Against COVID-19? Nat Rev Immunol (2020) 20(6):335–7. doi: 10.1038/s41577-020-0337-y
121. Czajka H, Zapolnik P, Krzych L, Kmiecik W, Stopyra L, Nowakowska A, et al. A Multi-Center, Randomised, Double-Blind, Placebo-Controlled Phase III Clinical Trial Evaluating the Impact of BCG Re-Vaccination on the Incidence and Severity of SARS-CoV-2 Infections Among Symptomatic Healthcare Professionals During the COVID-19 Pandemic in Poland-First Results. Vaccines (Basel) (2022) 10(2):314. doi: 10.3390/vaccines10020314
122. Hensel J, McAndrews KM, McGrail DJ, Dowlatshahi DP, LeBleu VS, Kalluri R. Protection Against SARS-CoV-2 by BCG Vaccination Is Not Supported by Epidemiological Analyses. Sci Rep (2020) 10(1):18377. doi: 10.1038/s41598-020-75491-x
123. Moorlag S, Taks E, Ten Doesschate T, van der Vaart TW, Janssen AB, Muller L, et al. Efficacy of Bacillus Calmette-Guerin Vaccination Against Respiratory Tract Infections in the Elderly During the COVID-19 Pandemic. Clin Infect Dis (2022). doi: 10.1093/cid/ciac182
124. Kaufmann E, Khan N, Tran KA, Ulndreaj A, Pernet E, Fontes G, et al. BCG Vaccination Provides Protection Against IAV But Not SARS-CoV-2. Cell Rep (2022) 38(10):110502. doi: 10.1016/j.celrep.2022.110502
125. White AD, Sibley L, Sarfas C, Morrison AL, Bewley K, Churchward C, et al. Influence of Aerosol Delivered BCG Vaccination on Immunological and Disease Parameters Following SARS-CoV-2 Challenge in Rhesus Macaques. Front Immunol (2021) 12:801799. doi: 10.3389/fimmu.2021.801799
126. Eggenhuizen PJ, Ng BH, Chang J, Fell AL, Cheong RMY, Wong WY, et al. BCG Vaccine Derived Peptides Induce SARS-CoV-2 T Cell Cross-Reactivity. Front Immunol (2021) 12:692729. doi: 10.3389/fimmu.2021.692729
127. Xiao Y, Lidsky PV, Shirogane Y, Aviner R, Wu CT, Li W, et al. A Defective Viral Genome Strategy Elicits Broad Protective Immunity Against Respiratory Viruses. Cell (2021) 184(25):6037–51.e14. doi: 10.1016/j.cell.2021.11.023
128. Guan Z, Chen C, Li Y, Yan D, Zhang X, Jiang D, et al. Impact of Coinfection With SARS-CoV-2 and Influenza on Disease Severity: A Systematic Review and Meta-Analysis. Front Public Health (2021) 9:773130. doi: 10.3389/fpubh.2021.773130
129. Stowe J, Tessier E, Zhao H, Guy R, Muller-Pebody B, Zambon M, et al. Interactions Between SARS-CoV-2 and Influenza, and the Impact of Coinfection on Disease Severity: A Test-Negative Design. Int J Epidemiol (2021) 50(4):1124–33. doi: 10.1093/ije/dyab081
130. Achdout H, Vitner EB, Politi B, Melamed S, Yahalom-Ronen Y, Tamir H, et al. Increased Lethality in Influenza and SARS-CoV-2 Coinfection Is Prevented by Influenza Immunity But Not SARS-CoV-2 Immunity. Nat Commun (2021) 12(1):5819. doi: 10.1038/s41467-021-26113-1
131. Lobo N, Brooks NA, Zlotta AR, Cirillo JD, Boorjian S, Black PC, et al. 100 Years of Bacillus Calmette-Guerin Immunotherapy: From Cattle to COVID-19. Nat Rev Urol (2021) 18(10):611–22. doi: 10.1038/s41585-021-00481-1
132. Stoner MCD, Angulo FJ, Rhea S, Brown LM, Atwell JE, Nguyen JL, et al. Estimates of Presumed Population Immunity to SARS-CoV-2 by State in the United States, August 2021. Open Forum Infect Dis (2022) 9(2):ofab647. doi: 10.1093/ofid/ofab647
Keywords: live attenuated vaccine, non-specific effects of vaccination, pandemic, genetic interference, innate immunity, trained immunity, heterologous immunity
Citation: Seo S-U and Seong B-L (2022) Prospects on Repurposing a Live Attenuated Vaccine for the Control of Unrelated Infections. Front. Immunol. 13:877845. doi: 10.3389/fimmu.2022.877845
Received: 17 February 2022; Accepted: 31 March 2022;
Published: 16 May 2022.
Edited by:
Konstantin Chumakov, Food and Drug Administration, United StatesReviewed by:
Christine Benn, Statens Serum Institut (SSI), DenmarkMihai Netea, Radboud University Nijmegen, Netherlands
Copyright © 2022 Seo and Seong. This is an open-access article distributed under the terms of the Creative Commons Attribution License (CC BY). The use, distribution or reproduction in other forums is permitted, provided the original author(s) and the copyright owner(s) are credited and that the original publication in this journal is cited, in accordance with accepted academic practice. No use, distribution or reproduction is permitted which does not comply with these terms.
*Correspondence: Baik-Lin Seong, YmxzZW9uZ0B5b25zZWkuYWMua3I=