- 1RemAb Therapeutics, Mòdul de Recerca B, UAB Bellaterra, Barcelona, Spain
- 2Department of Chemical Biology of Glycans and Lipids, Shemyakin-Ovchinnikov Institute of Bioorganic Chemistry Russian Academy of Sciences (RAS), Moscow, Russia
- 3Laboratório de Artrópodes Hematófagos, Departamento de Parasitologia, ICB/UFMG, Belo Horizonte, Brazil
- 4MEGA: Asthma Inception and Progression Mechanisms, Complejo Hospitalario de Navarra (CHN), Pamplona, Spain
- 5Instituto de investigación sanitaria de Navarra (IdiSNA), Pamplona, Spain
- 6ARADyAL Research Network, Instituto de Salud Carlos III (ISCIII), Madrid, Spain
- 7Department of Medicine, Universitat Autònoma de Barcelona (UAB), Barcelona, Spain
- 8Allergy Section, Internal Medicine Department, Hospital Universitari Vall d’Hebron (HUVH), Barcelona, Spain
- 9Immunomediated Diseases and Innovative Therapies, Vall d’Hebron Institut de Recerca (VHIR), Barcelona, Spain
- 10Departamento de Sanidad Animal, Instituto Vasco de Investigación de Desarrollo Agrario (NEIKER), Derio, Spain
- 11Hospital Universitari de Bellvitge, Servicio de Medicina Intensiva, Hospitalet de Llobregat, Barcelona, Spain
- 12Instituto de Investigación Biomédica de Bellvitge (IDIBELL), Grupo Inmunidad Innata y Patología del Paciente Crítico, Hospitalet de Llobregat, Barcelona, Spain
Anti-αGal IgE antibodies mediate a spreading allergic condition known as αGal-syndrome (AGS). People exposed to hard tick bites are sensitized to αGal, producing elevated levels of anti-αGal IgE, which are responsible for AGS. This work presents an immunotherapy based on polymeric αGal-glycoconjugates for potentially treating allergic disorders by selectively inhibiting anti-αGal IgE antibodies. We synthesized a set of αGal-glycoconjugates, based on poly-L-lysine of different degrees of polymerization (DP1000, DP600, and DP100), to specifically inhibit in vitro the anti-αGal IgE antibodies in the serum of αGal-sensitized patients (n=13). Moreover, an animal model for αGal sensitization in GalT-KO mice was developed by intradermal administration of hard tick’ salivary gland extract, mimicking the sensitization mechanism postulated in humans. The in vitro exposure to all polymeric glycoconjugates (5-10-20-50-100 µg/mL) mainly inhibited anti-αGal IgE and IgM isotypes, with a lower inhibition effect on the IgA and IgG, respectively. We demonstrated a differential anti-αGal isotype inhibition as a function of the length of the poly-L-lysine and the number of αGal residues exposed in the glycoconjugates. These results defined a minimum of 27 αGal residues to inhibit most of the induced anti-αGal IgE in vitro. Furthermore, the αGal-glycoconjugate DP1000-RA0118 (10 mg/kg sc.) showed a high capacity to remove the anti-αGal IgE antibodies (≥75% on average) induced in GalT-KO mice, together with similar inhibition for circulating anti-αGal IgG and IgM. Our study suggests the potential clinical use of poly-L-lysine-based αGal-glycoconjugates for treating allergic disorders mediated by anti-αGal IgE antibodies.
Introduction
Type-I allergic conditions are disorders mediated by IgE, eliciting hypersensitivity to various allergens (1). IgE antibodies orchestrate an abnormal adaptive response against non-infectious, harmless, exogenous, and environmental substances, including glycoproteins from grass, pollen, dust mites, insect venom, and food (1). The number of people affected by such disorders is continuously growing globally (2, 3). Plasma exchange, immunosuppressive drugs, and monoclonal antibodies are potential immunotherapies focused on reducing IgE levels (4). Omalizumab (Xolair®), an unspecific treatment directed to total IgE, is the only anti-IgE therapy approved to treat moderate to severe asthma and chronic idiopathic urticaria (5). However, natural IgE has been described to participate in the physiological host resistance against certain parasites such as arthropods and helminths (6, 7). Thus, the total removal of IgE is a concern.
Increasing evidence describes the functional involvement of anti-αGal antibodies in different human disorders (8), including αGal-syndrome (AGS) (9, 10). These antibodies bind to Galα1,3Gal and Galα1,3Galβ1,4GlcNAc oligosaccharides (αGal) (11), although with higher affinity to the free trisaccharide (12). Primates, including apes, and Old-World monkeys, do not express the αGal epitopes due to an evolutive inactivation of the gene coding for the α1,3-galactosyltransferase enzyme (13, 14); consequently, they naturally produce these antibodies. Some evidence links their origin to the gut microbiota (15, 16). αGal residue is expressed in glycolipids and glycoproteins of the cell membrane of different microorganisms, including viruses, bacteria, and protozoans (8). Hence, it has been associated with a possible protective role of anti-αGal antibodies (17). However, the existing epidemiological evidence is controversial. High serological levels of anti-αGal IgM at the start of dialysis therapy have been described as a predictor of later risk for mortality and enteric peritonitis in peritoneal dialysis patients (18). Furthermore, anti-αGal IgM was associated with protection against malaria in infants (19) and children >4 years old (20). On the contrary, anti-αGal IgG has been associated with a higher risk of malaria infection in children (19, 20). Additionally, anti-αGal IgM and IgG, but not IgE antibodies, were significantly higher in uninfected than Plasmodium falciparum- and Mycobacterium tuberculosis-infected individuals (21). Similarly, experimental models have also demonstrated protection against lethal Trypanosoma cruzi challenge (22) and malaria by prophylactic vaccination with an αGal-based compound and oral administration of Escherichia coli O86:B7 (20), respectively. Interestingly, oral administration of the same bacterium protects turkeys from developing acute aspergillosis. Nevertheless, this effect was not associated with augmented anti-αGal IgY levels but with an apparent reduction of anti-αGal IgA in the lungs of infected animals (23).
AGS symptoms occur after red meat intake (24) or exposition to other products containing αGal like Cetuximab (25). Hard ticks are associated with the anti-αGal IgE sensitization and AGS spreading (26, 27) (Figure 1). The prevalence of this pathological condition is higher in countries where individuals are in contact with ticks (27). The natural habitat of this type of tick is being significantly impacted by climate change, occupying ever larger regions worldwide (28). Therefore, a notable increase in patients with AGS is expected in the coming years. Currently, the seventeen countries where there are registries of hard ticks as the causative agent of the αGal syndrome include Australia [Ixodes holocyclus (29)] and the United States [Amblyomma Americanum (30)]. In Europe, the endemic tick is Ixodes ricinus, found in Germany (31), France (32), Spain (33), Belgium (34), Switzerland (35), Sweden (36), United Kingdom (37), Italy (38) and Norway (39). The list of affected countries also includes Korea [Ixodes nipponensis (40)], Japan [Haemaphysalis longicornis (41)], Panama [Ixodes cajennense (42)], Brazil [Amblyomma sculptum (43)], Ivory Coast [Amblyomma variegatum (44)] and South Africa (45). Hard ticks’ saliva, salivary glands, and midgut contain proteins decorated with αGal residues (42, 45). Sensitization is postulated to start with the allergen uptake by APCs in the epidermis (46). Then, the sensitization mechanism promotes the class switch recombination in B cells and the subsequent secretion of IgE antibodies in the skin-draining lymph nodes (46, 47). Anti-αGal IgE-switched B cell precursor seems to be a naive non-switched B cell (48). Re-exposure to the allergen leads to the activation and degranulation of mast cells and basophils. Moreover, the allergic reaction is triggered 3-6 h after mammalian meat ingestion due to the binding of anti-αGal IgE antibodies to αGal epitopes expressed in the meat (24, 49). Regarding Cetuximab allergy, anti-αGal IgE binding to αGal residues in the monoclonal antibody induces an immediate systemic allergic reaction that can be severe enough to trigger a life-threatening anaphylactic shock (50, 51).
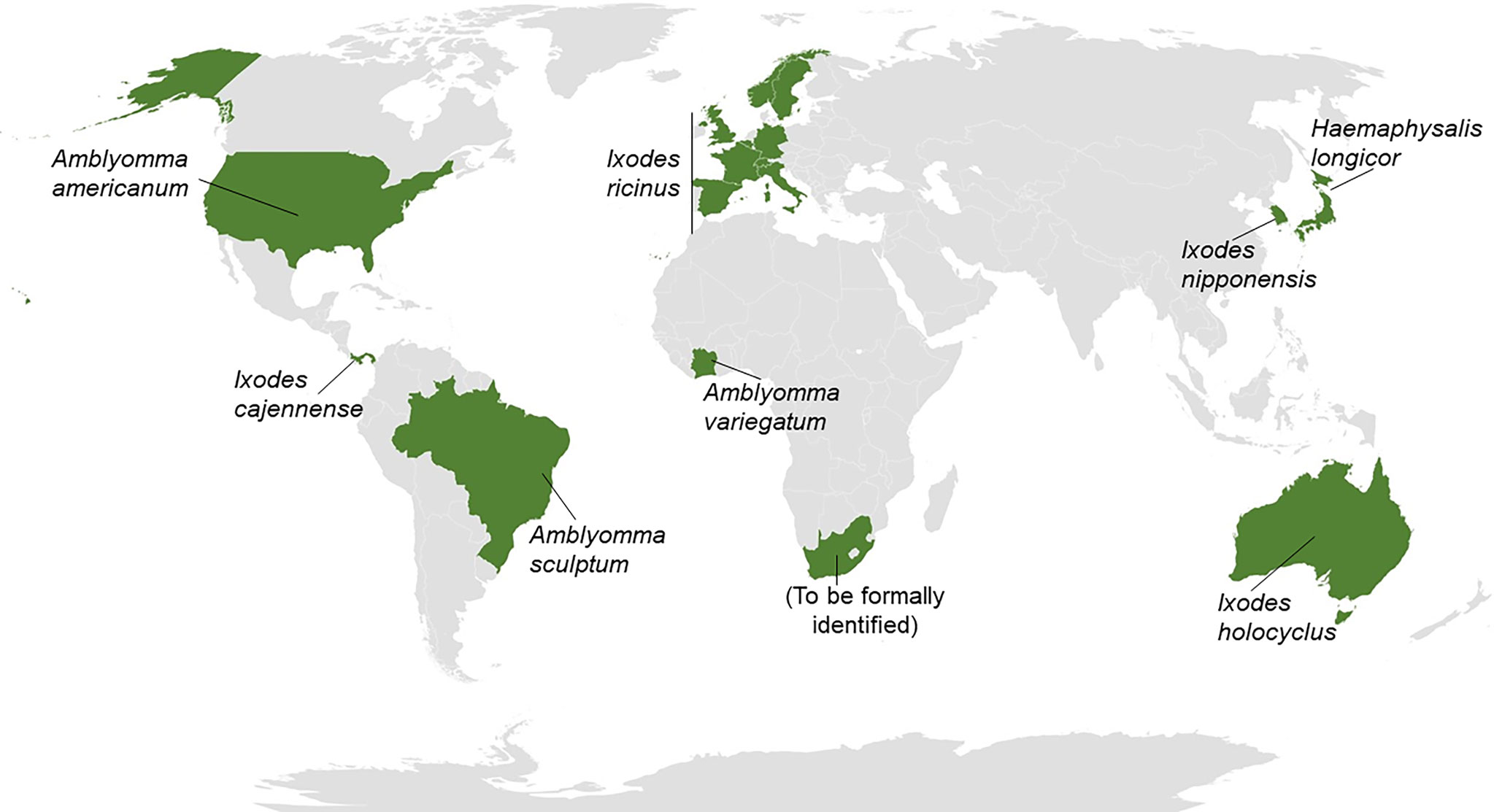
Figure 1 Hard ticks as the cause for αGal syndrome. Worldwide map reflecting (in green) the 17 countries in which hard ticks from different species have been detected to be causative of αGal syndrome (AGS). The list of countries includes Australia, United States, Germany, France, Spain, Belgium, Switzerland, Sweden, United Kingdom, Italy, Norway, Korea, Japan, Panama, Brazil, Ivory Coast, and South Africa.
Patients with AGS exhibit various clinical symptoms, including urticaria, pruritus, angioedema, and systematic anaphylaxis. In addition, some patients have reported specific symptoms, such as nausea, indigestion, diarrhea, and abdominal discomfort (51, 52).
Anti-αGal antibodies (IgM, IgG) have previously been removed in rodents and primates using a poly-L-lysine-based αGal-glycoconjugate (GAS914), without side effects, minimal complement activation, and no sensitization (53). GAS914 was developed to overcome the hyperacute and acute vascular xenograft rejection in pig-to-primate transplantation primarily caused by anti-αGal antibodies (53). However, GAS914 never reached the clinic due to the participation of anti-non-αGal antibodies in the mentioned rejection mechanism (54). Additionally, the development of α1,3-galactosyltransferase gene-knockout transgenic pigs made unnecessary the clinical development of GAS914 for this indication (55, 56).
The lack of sensitization, together with the high capacity of GAS914 to inhibit anti-αGal antibodies (IgM and IgG), prompted us to study the in vitro and in vivo removal of anti-αGal IgE antibodies with a set of poly-L-lysine-based αGal-glycoconjugates as a potential treatment for diseases mediated by such antibodies.
Materials and Methods
Polymeric αGal-Glycoconjugates
GAS914 (Novartis Pharma AG, Basel, Switzerland) is a poly-L-lysine backbone with an average degree of polymerization (DP) of 1,000 L-lysines (DP1000) and with 23-28% of lysines derivatized with the oligosaccharide Galα1,3Galβ1,4GlcNAc- (αGal) (53). GAS914 was used as anti-αGal inhibitor control. Analysis of 1H-NMR allowed determining the load of αGal in GAS914 (Figure S1).
The RA01-compounds were specifically synthesized for this work from a linear poly-L-lysine (57) of a final DP of 100, 600, 1000 (DP100, DP600, and DP1000, respectively) and increasing loads of Galα1,3Galβ1,4GlcNAc- in the final structure. DP1000 glycoconjugates were produced following the synthetic route described by Duthaler et al., 2010 (58). For DP100 and DP600 glycoconjugates, poly-L-lysine hydrobromide was acylated with a calculated amount of Galα1,3Galβ1,4GlcNAc (αGal)-sp-Ad-ONSu active ester in DMSO in the presence of Et3N. The residual amino groups were acylated (in the same reaction mixture) with an excess of glycolic acid acetate succinimide ester AcOCH2(CO)ONSu in the presence of Et3N. To remove acetyl protecting groups by hydrolysis, the reaction mixture was diluted with a twofold volume of water, and Et3N was added (2% of the volume of the solution). Glycopolymers were isolated by gel-permeating chromatography on Sephadex LH-20 in MeCN-water 30:70 by volume. Fractions contained pure conjugate were evaporated to ~2 mL volume and freeze-dried. The purity and composition of the synthesized glycoconjugates (the percentage of modification of poly-L-lysine with Galα1,3Galβ1,4GlcNAc) were determined by the 1H NMR spectroscopy (Figure S2). The complete list of resulting polymeric glycoconjugates is shown in Table S1. Figure 2 provides the general structure for this set of glycopolymers.
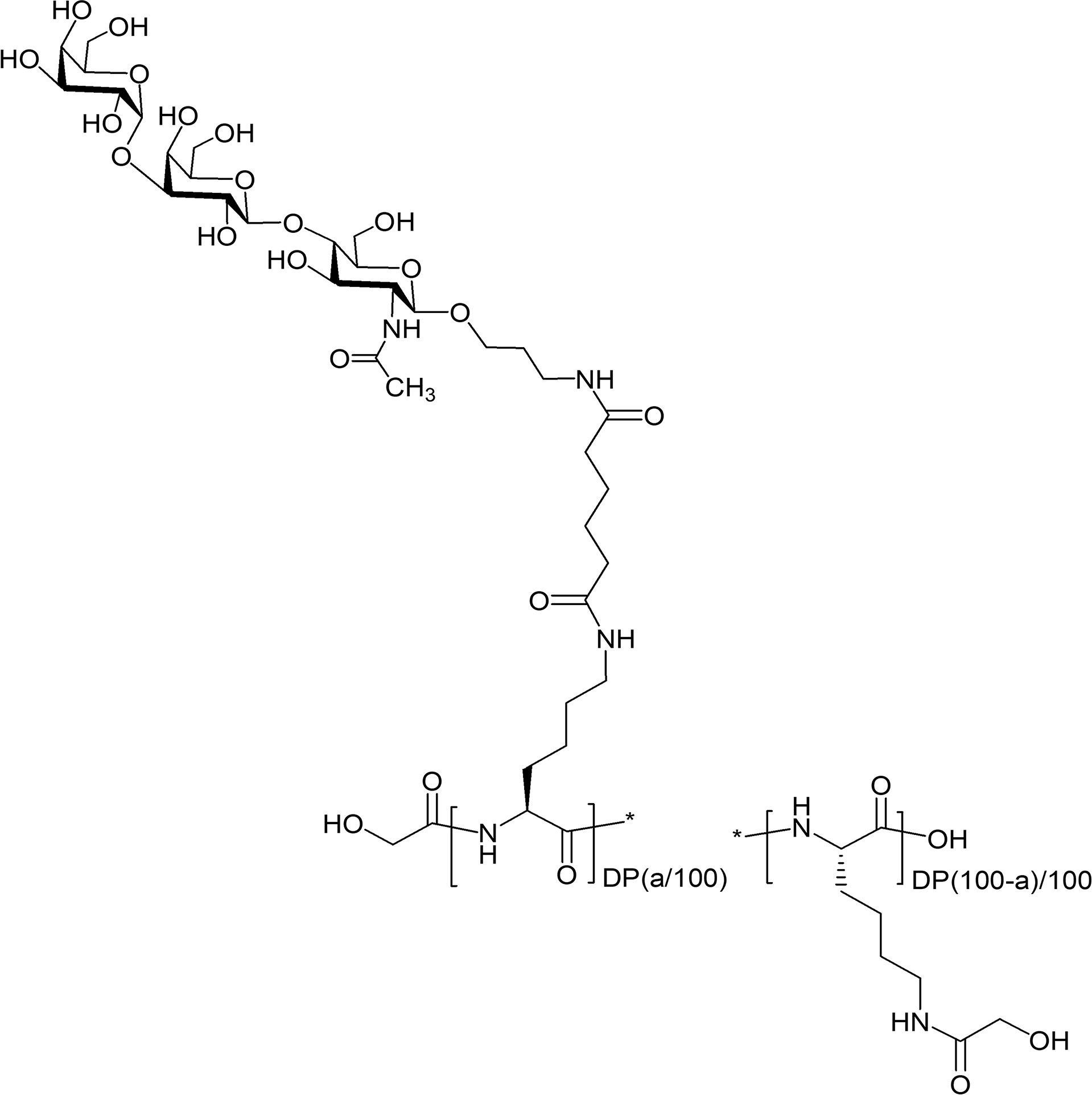
Figure 2 General structure of polymeric αGal-glycoconjugates. Poly-L-lysine backbone (degree of polymerization, DP: 100, 600, and 1000) were derivatized with the trisaccharide Galα1-3Galβ1-4GlcNAcβ-sp, (sp = -O(CH2)3NH2). Percent of modification with αGal = a (9, 12, 18, 27 and 34%). Residual amino groups of poly-L-lysine were acylated with glycolic acid.
Human Serum Samples
Normal Human Sera (NHS) from donors of the Blood Bank of the Hospital Universitari de Bellvitge were used as healthy controls (CN, n=8). Serum samples from αGal-sensitized subjects (PT, n=13) were selected from previous epidemiological studies (59), where anti-αGal IgE prevalence in individuals with acute urticaria or anaphylaxis from different geographical areas of Spain was studied.
In Vitro Inhibition of Anti-αGal With Polymeric αGal-Glycoconjugates
To test the inhibition of anti-αGal antibodies, human serum samples (PT, n=13) were incubated for 15-17 h under mild orbital shaking (225 rpm at 4°C) with each glycopolymer at growing concentrations (5-10-20-50-100 µg/mL). The final volume of the reaction was set at 100 µL. Vehicle (PBS)-treated serum was incubated under the same conditions as a control (baseline). After incubation, the serum samples containing the different glycopolymers or PBS (control for each serum) were conveniently diluted to determine the unbound fraction of the different anti-αGal isotypes, by ELISA, following the general protocol previously described (15). Briefly, Nunc MaxiSorpTM 96-well flat-bottom plates (Thermo Fisher Scientific, Waltham, MA, USA) were coated with 2.5 μg/mL of Galα1,3Galβ1,4GlcNAc glycan conjugated to human serum albumin (HSA). After washing and blocking steps, serum samples diluted in PBS (1:100 for IgM and IgG, 1:25 for IgA, and 1:10 for IgE) were added to the wells and incubated for 1 h at 25°C. After washing, the incubated for 1 h at 25°C with the corresponding horseradish peroxidase (HRP)-labeled anti-human or anti-mouse secondary antibodies diluted in PBS. o-Phenylenediamine dihydrochloride (OPD) was used as HRP substrate, and incubated at 25°C in the dark. The reaction was stopped with 3N hydrochloric acid (HCl). The resulting absorbance was registered at 492 nm using a PowerWave™ XS Microplate Reader (Biotek, Winooski, VT, USA). The resulting data were graphed as optical density units. Moreover, the in vitro data was the result of three independent experiments. The inhibition rate for each glycopolymer (expressed as a percentage of anti-αGal inhibition) was calculated according to the levels of anti-αGal antibodies determined in baseline (PBS) and treated (glycopolymer) conditions for each serum.
α1,3-Galactosyltransferase Knocked Out (GalT-KO) Mice
This study was performed in 48 mice of 24-32 weeks-old (sex parity), in which the gene coding for the α1,3-galactosyltransferase enzyme had been knocked out (GalT-KO mice) and was derived from a highly inbred colony with a hybrid genetic background (B6xCBAx129sv) (60). Animals were handled and housed as previously described (15). Procedures concerning all animals were supervised and approved by the ethics committee for animal experimentation of Bellvitge Biomedical Research Institute (IDIBELL) and the Catalonia Government (Record FUE-2018-00931758). The care, as well as the handling of the animals, were following the Guide for the Care and Use of Laboratory Animals that the US National Institutes of Health published (NIH Publication n° 85–23 revised 1996) as well as the European Agreement of Vertebrate Animal Protection for Experimental Use (86/609). The procedure for euthanasia was established following the European Directive on protecting animals used for scientific purposes (2010/63/EU). Death was never considered a human endpoint.
Amblyomma sculptum Salivary Gland Extract
As previously described (61), salivary gland extract (SGE) was produced from 200 females of unfed A. sculptum. To obtain the SGE, females were washed with sterile water, and their salivary glands were individually dissected in saline (0.9% NaCl). Each pair of glands were transferred to 1.5 mL tubes containing saline solution, placed in an ultrasonic bath for 40 seconds, centrifuged at 14,000g for 5 min. Next, the supernatant was transferred to a new tube, dried under vacuum at 56°C to yield an amount of 3.11 mg, and kept at -20°C until use. The amount of protein in the sample was measured by Bradford et al., 1976 (62) using bovine serum albumin as standard. Sterile PBS was conveniently used as a vehicle to prepare the final aqueous solution injected as an allergen to the GalT-KO mice.
GalT-KO Mice Sensitization
GalT-KO mice were randomly separated into three different groups (sex parity). Group 1 (n=16) was a double negative control (PBS id. or sc. for sensitization and treatment, respectively). Group 2 (n=16) and 3 (n=16) were sensitized with two doses of 20 μg id. of the salivary gland extract (days 0 and 7, Figure 3). Animals were then challenged with three consecutive doses of 5 μg id. of the extract on days 14, 15, and 16 to induce the production of anti-αGal IgE antibodies (63, 64). Group 2 was a negative control for the treatment (vehicle: PBS sc.). In Group 3, animals were treated with three consecutive DP1000-RA0118 doses (10 mg/kg, sc.) on days 16 (4 h after last challenge), 17, and 18. The challenge was repeated in two animals of Group 3 one week after the last treatment with DP1000-RA0118 (day 26). Although each experimental group was composed of sixteen animals, not all the parameters were determined in the totality of mice due to welfare reasons. In the case of multiple blood extractions, volume never exceeded 7.5% of the total blood volume (124-158 µL of fresh blood in ~30 g mice) weekly (65). Animal blood was collected by controlled submandibular bleeding on days -3 (baseline), 16 (3 h after challenge), 18 (3 h after treatment), and 28 (after rechallenging, two animals of Group 3) as previously described (66).
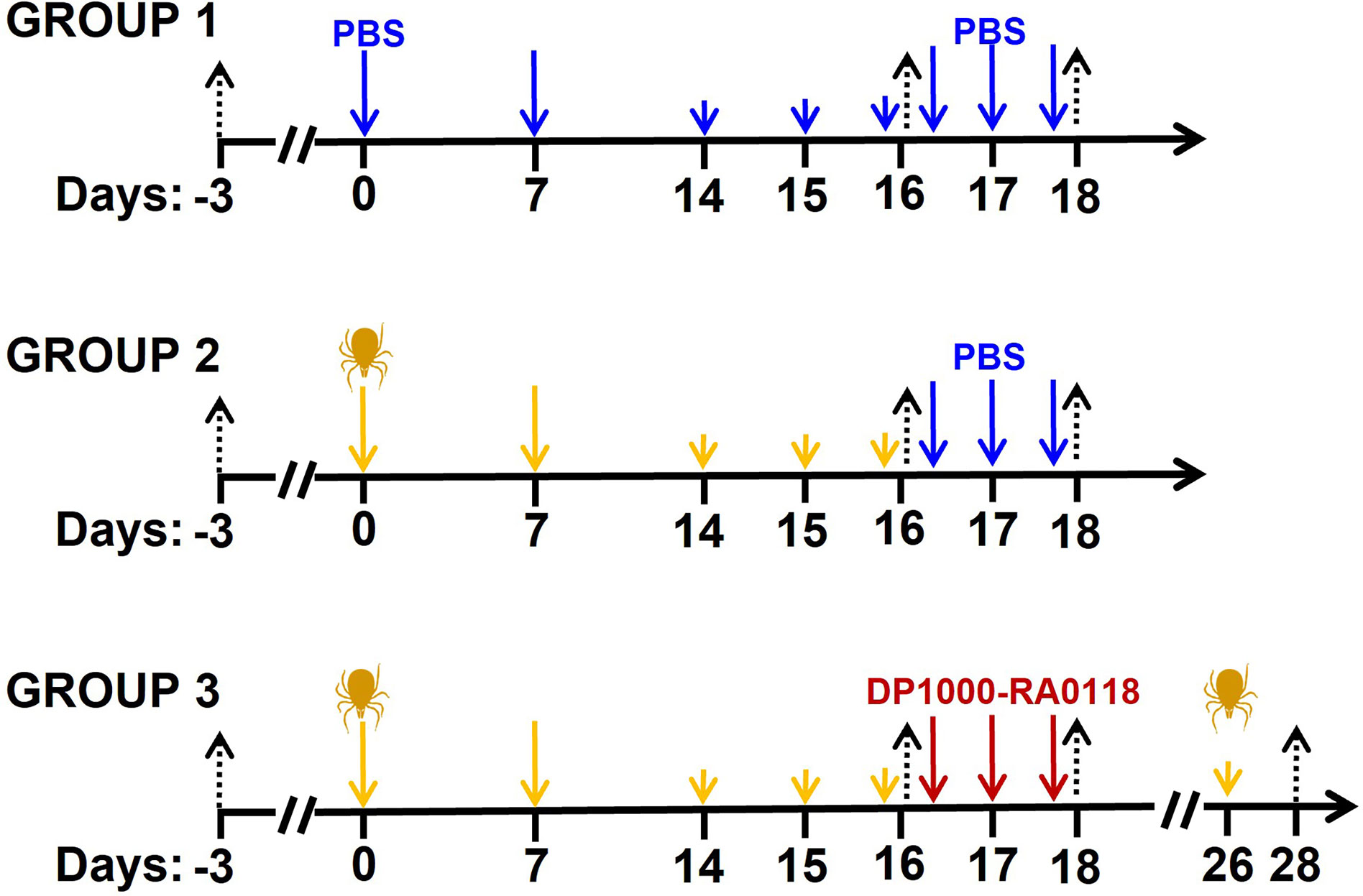
Figure 3 Scheme of GalT-KO mice sensitization for anti-αGal IgE production. Group 1: double negative control (PBS) (n=16). Group 2 (n=16) and Group 3 (n=16) (αGal-sensitized mice) were treated with PBS (control) and DP1000-RA0118, respectively. Black arrows: bleeding (for immunological determinations), blue arrows: PBS id. (control of sensitization) or sc. (control of treatment), yellow arrows: salivary gland extract (20 or 5 µg id.), dark-red arrows: DP1000-RA0118 10 mg/kg sc.
Total Circulating Mouse IgM, IgG1, IgG2a, IgG2b, IgG3, and IgE by ELISA
Total mouse serum immunoglobulins (n=6) were determined on days -3, 16, and 18 using the commercial RayBio® mouse ELISA kit following manufacturer instructions (RayBiotech, GA, USA).
White Blood Cells by Flow Cytometry
Flow cytometry analysis was performed on a Gallios analyzer (Beckman Coulter, IN, USA) equipped with violet (405 nm), blue (488 nm), and red (633 nm) solid-state lasers as an excitation source. Purified rat anti-mouse CD16/CD32 was added to the fresh blood samples to block non-antigen-specific binding (Beckton Dickinson, CA, USA). After 5 min incubation at 25°C, fluorochrome-conjugated antibodies (Table S2, Beckton Dickinson, CA, USA) were added to the samples. BD FACS™ lysing solution was then added to lyse red blood cells. Samples were homogenized with vortex and incubated for 5 min at 25°C in the dark. After centrifugation (5 min, 3,220g, 25°C), the supernatant was discarded, and the pellet was resuspended in 400 µL of PBS for immunophenotyping of different White Blood Cells (WBC) for every experimental group (n=6) on days -3, 16, and 18. Events collected from fresh blood mouse samples were displayed in a CD45 vs. side scatter intensity (SS INT) plot to discard debris and define a total WBC population. Every single FACS determination recorded about 150,000 total events, of which 50,000 were CD45 positive. Fluorescence was collected through the corresponding bandpass filters for each indicated surface cell marker. Data were analyzed using KALUZA software (Beckman Coulter, CA, USA).
Statistics
GraphPad Prism statics software was used for analysis and data graphing. The Gaussian distribution of data was checked by the D’Agostino-Pearson omnibus normality test (alpha = 0.05), and homogeneity of variances was determined by the F test (alpha = 0.05). Most of the statistical analyses were performed using paired or unpaired parametric t-tests. The Wilcoxon matched-pairs signed-rank and Mann-Whitney tests (unpaired data analysis) were used as non-parametric tests when data did not follow a Gaussian distribution. Tukey and Sidak were used as multiple comparison tests. Differences were considered statistically significant when p<0.05 (*: p<0.05; **: p<0.01; ***: p<0.001; ****: p<0.0001), ns: non-significant.
Results
Prevalence of Anti-αGal IgE in αGal-Sensitized Subjects
The prevalence of anti-αGal IgE in αGal-sensitized subjects has been extensively reviewed (67–69). We confirmed by ELISA the significantly elevated circulating levels of anti-αGal IgE antibodies in patients compared to controls. PT also showed higher serological levels for the rest of the anti-αGal isotypes (IgM, IgG, and IgA) than CN (Figure 4).
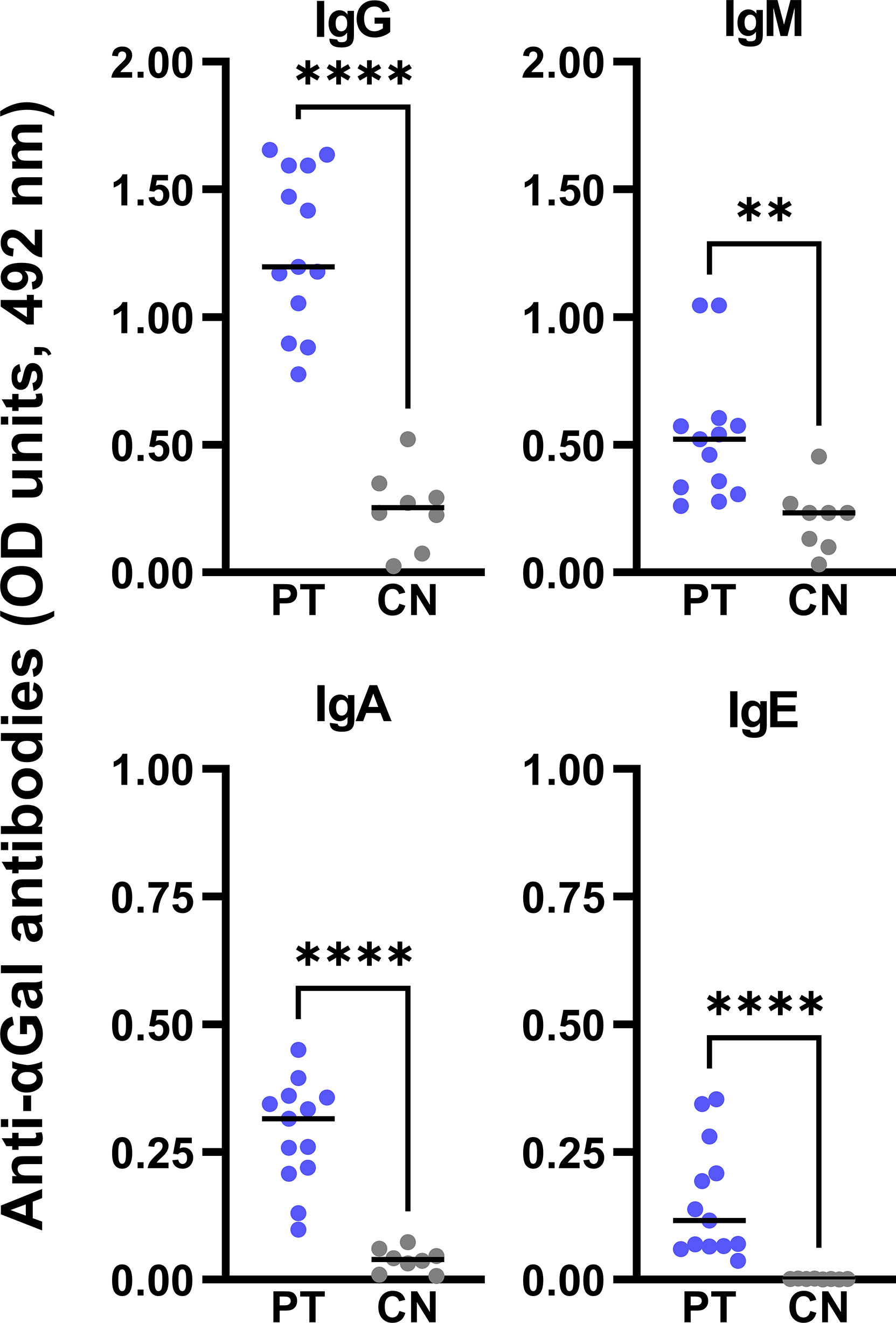
Figure 4 Serological levels of anti-αGal antibodies by ELISA. αGal-sensitized patients (PT, n=13, blue dots) showed significantly higher serological anti-αGal antibodies levels (expressed as optical density units, 492 nm) compared to healthy subjects (CN, n=8, grey dots). To detect each immunoglobulin isotype, serum samples were accordingly diluted: 1:100 for IgM and IgG, 1:25 for IgA, and 1:10 for IgE. Unpaired t-test analysis was performed (**: p < 0.01; ****: p < 0.0001).
Influence of the Multivalent αGal Exposure on the Inhibition of Anti-αGal IgE Antibodies
Anti-αGal antibodies inhibition with monovalent compounds has been demonstrated as inefficient due to their low affinity for single oligosaccharides (53). Previously, GAS914 has shown a maximal increase in avidity (relative to the monomer) by anti-αGal IgM and IgG antibodies (53, 70). Nevertheless, there is no data on whether the anti-αGal IgE inhibition could be affected using multivalent αGal compounds. For that, we synthesized two glycopolymers composed of a backbone of 1,000 L-lysines (DP1000), with 9 and 18% of lysine residues derivatized with Galα1,3Galβ1,4GlcNAc- (DP1000-RA0109 and DP1000-RA0118, respectively). GAS914 (DP1000) with 27% αGal load (Figure 5) was used as a positive control for anti-αGal inhibition.
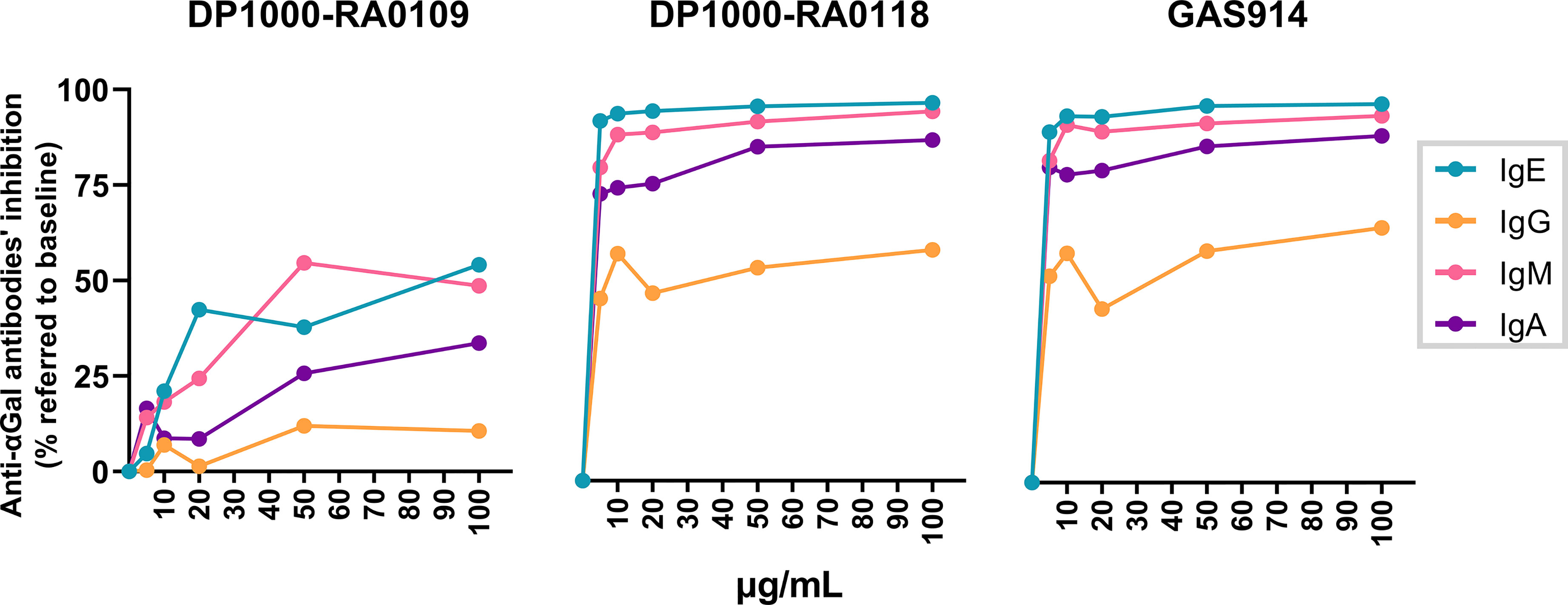
Figure 5 Influence of αGal load on anti-αGal antibodies inhibition. Serum samples from αGal-sensitized patients (n=13) were incubated with DP1000-RA0109, DP1000-RA0118 or GAS914 (positive control of inhibition) at growing concentrations (5-10-20-50-100 µg/mL). PBS-treated serum was similarly incubated as a baseline condition. After incubation, samples were conveniently diluted to determine by ELISA the unbound fraction of the different anti-αGal isotypes (1:100 for IgM and IgG, 1:25 for IgA, and 1:10 for IgE). The inhibition rate for each glycopolymer (expressed as a percentage of anti-αGal inhibition) was calculated according to the quantity of anti-αGal antibodies determined in baseline (PBS) and treated (glycopolymer) conditions for each serum. IgE green line, IgG orange line, IgM pink line, and IgA purple line.
It was previously demonstrated that the chromatographic affinity purification process could favor the preferential binding and elution of IgM over the rest of anti-αGal isotypes masking the real inhibitory capacity of polyacrylamide-based αGal-conjugates (71). Therefore, we used in the in vitro inhibitory studies human serum from αGal-sensitized patients instead of affinity-purified fractions of anti-αGal antibodies.
Overall, the exposure to all polymeric glycoconjugates mainly inhibited anti-αGal IgE and IgM isotypes, with a lower inhibition effect on the IgA and IgG isotypes, respectively (Figure 5). We found very similar inhibition rates for all the anti-αGal isotypes when comparing DP1000-RA0118 vs. GAS914 (positive control). Indeed, for IgE, DP1000-RA0118 showed slightly higher inhibition rates than GAS914. Additionally, reducing the αGal load in the same poly-L-lysine backbone to 9% (DP1000-RA0109) significantly reduced the inhibitory capacity of the glycopolymer (Figure 5). Hence, DP1000-RA0118 was selected for the in vivo proof of concept.
In Vivo Inhibition of Anti-αGal IgE in Sensitized Mice
The preliminary data obtained in vitro prompted us to investigate, as proof of concept, the anti-αGal IgE inhibitory capacity of DP1000-RA0118 in a small animal model of αGal sensitization. Therefore, the main objective of this study was to induce anti-αGal IgE in a relevant animal model and to study its intracorporeal removal with DP1000-RA0118.
GalT-KO mice are considered an adequate model for αGal sensitization and production of anti-αGal IgE antibodies (15, 61, 72, 73). The sensitization (Figure 3) was conducted in immunologically mature GalT-KO mice (15). Significant induction of anti-αGal IgE antibodies was achieved in all the animals of Groups 2 and 3 on day 16 (Figure 6). This induction was also accompanied by significantly augmented anti-αGal IgG and IgM. Elevated levels of anti-αGal IgE antibodies were again induced on day 28 in two mice of Group 3 rechallenged with the SGE on day 26 (Figure 6). The treatment with DP1000-RA018 (day 18) produced a significant decrease in the levels of anti-αGal IgE in Group 3 (≥75% on average, Figure 6) as well as in anti-αGal IgG and IgM compared to the induced condition (Group 3, day 16). This decrease in the levels of anti-αGal can only be attributed to the in vivo inhibitory capacity of DP1000-RA0118 because in Group 2 (treated with PBS) the levels of these antibodies remained constant from day 16 (end of challenge) to day 18 (end of PBS treatment).
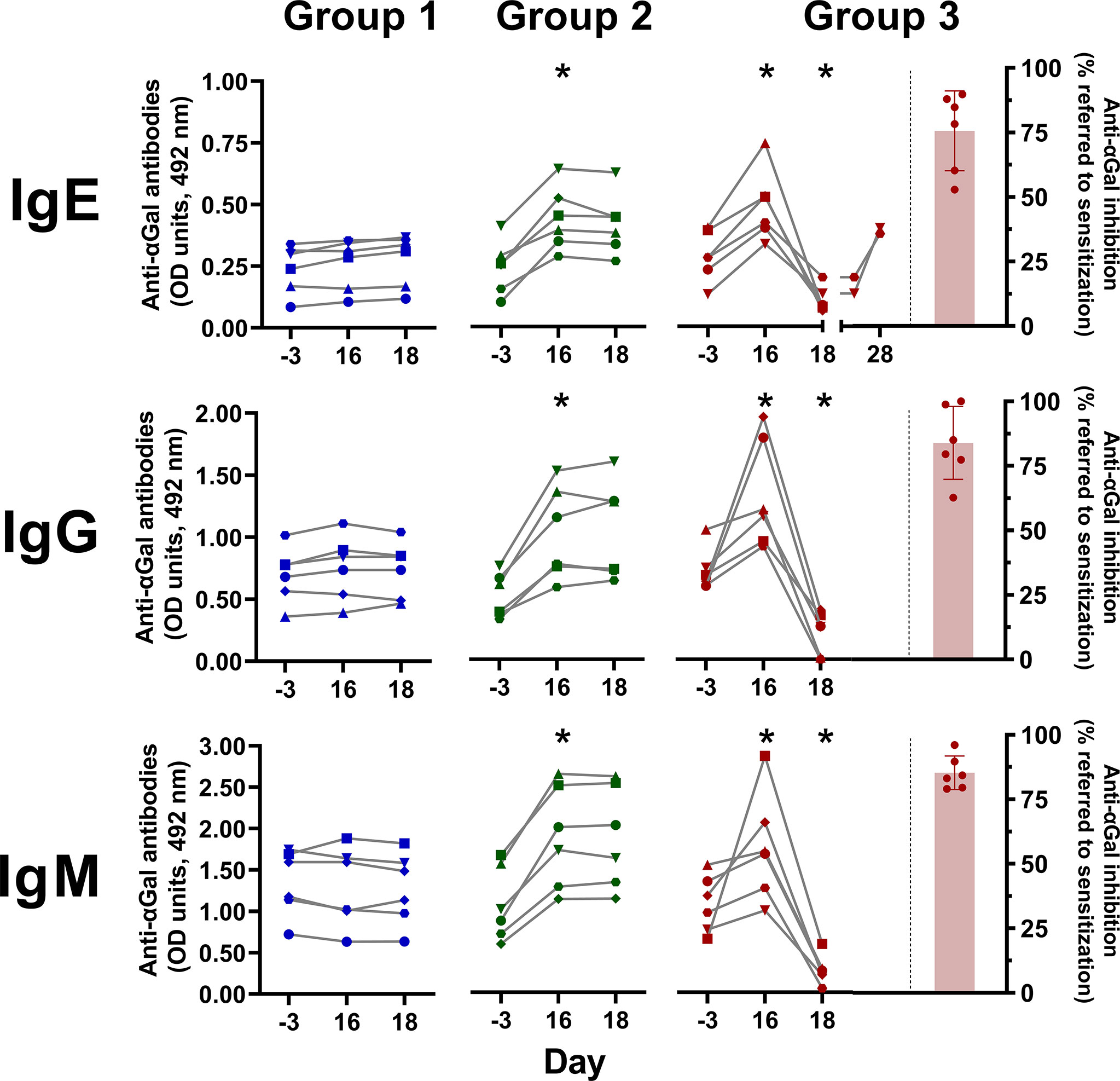
Figure 6 A. sculptum salivary gland extract significantly induced anti-αGal antibodies in GalT-KO mice. Animals from Group 1 (in blue, n=6) are a double negative control (PBS for sensitization and treatment, respectively). Animals of Group 2 (in green, n=6) and 3 (in dark-red n=6) were sensitized to αGal according to Figure 2. In Group 3, after sensitization, animals were treated with three consecutive DP1000-RA0118 doses (10 mg/kg, sc.) on days 16, 17, and 18. The challenge was repeated in two animals of Group 3 on day 26, one week after the last treatment with DP1000-RA0118. Red columns represent the anti-αGal inhibition on day 18 (% referred to sensitization on day 16) in Group 3 (treated with DP1000-RA0118). Wilcoxon matched-pairs signed-rank was used as a non-parametric test (*: p < 0.05).
Impact of Sensitization and Treatment With DP1000-RA0118 on Humoral and Cellular Immune Mediators
Anti-αGal antibodies (IgM, IgG) have previously been removed in rodents and primates using GAS914 (53). Since these studies were conducted in healthy animals (no sensitized), we investigated whether some of the humoral and cellular mediators of the immune system were affected by the sensitization protocol and the treatment with DP1000-RA0118.
Regarding immunoglobulins, despite the increase obtained for anti-αGal antibodies after sensitization for Groups 2 and 3, the total IgM, IgG1, IgG2a, IgG3 remained constant during the evaluated days for all the experimental groups (Figure 7). However, total IgM slightly decreased from day 16 to 18 only in Group 3. The treatment with DP1000-RA0118 (day 18) removed preexisting and induced anti-αGal IgM, which impacted the total IgM levels measured. Interestingly, a slight increase in total IgE and IgG2b subtype was observed for Groups 2 and 3 after mice sensitization (day 16, Figure 7). These trends in both experimental groups seem to be partially associated with the induction on day 16 of augmented levels of anti-αGal antibodies for the IgE and IgG isotype, respectively.
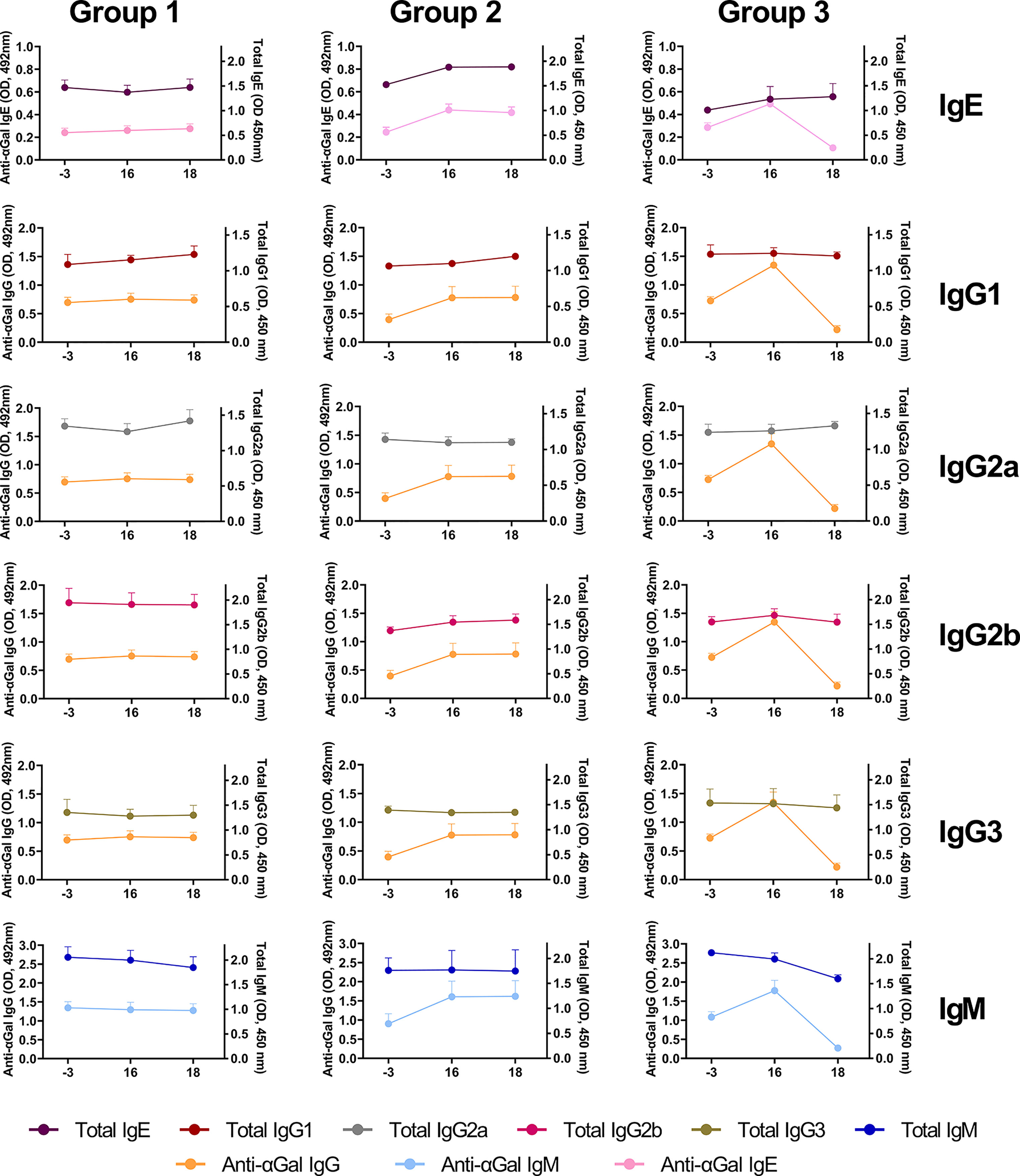
Figure 7 The pattern of total immunoglobulin levels (IgE, IgG1, IgG2a, IgG2b, IgG3, and IgM) and their association with the different isotypes assessed for anti-αGal antibodies (IgE, IgG, and IgM). Relative serological levels of total immunoglobulins (expressed as OD units, 450 nm) and anti-αGal antibodies (expressed as OD units, 492 nm) were determined by ELISA on days -3 (baseline), 16 (after sensitization), and 18 (after treatment). GalT-KO mice from Group 1 are a double negative control (PBS for sensitization and treatment, respectively) (n=6). Animals of Groups 2 and 3 were sensitized to αGal, according to Figure 2. In Group 3, after sensitization, animals were treated with three consecutive DP1000-RA0118 doses (10 mg/kg, sc.) on days 16, 17, and 18.
During αGal sensitization, the major change in the WBC population was registered for basophils (Figure S3). The rest of the evaluated WBC remained unchanged between days and experimental groups. Basophils were gated using rat anti-mouse Ly6G and IgE (Figure S3A). The later monoclonal antibody allowed quantifying the IgE bound to Fcϵ receptors on basophils. On day 16, Groups 2 and 3 showed a significant increase in fluorescence compared to day -3 (baseline) (Figures S3B, C). Since the gated basophil population between experimental groups was similar, the augmented fluorescence obtained for Groups 2 and 3 seems to be associated with the increased serological levels of anti-αGal IgE antibodies after the sensitization procedure. On day 18, the basophil fluorescence returned to baseline levels (Figure S3C).
Influence of Degree of Polymerization on the Differential Inhibition of Anti-αGal Isotypes
Along with specific inhibition of anti-αGal IgE, DP1000-RA0118 showed a high inhibitory capacity to the rest of circulating anti-αGal isotypes. Although removing most of the circulating anti-αGal isotypes might not represent an obstacle to the potential clinical development of DP1000-RA0118 (74), we investigated whether the DP can impact the differential inhibition of anti-αGal isotypes. For that, we synthesized a complementary set of αGal-glycoconjugates using polymeric backbones containing 600 (DP600) and 100 (DP100) L-lysine residues. In addition, DP1000 αGal-glycoconjugates with 12% and 27% Galα1,3Galβ1,4GlcNAc load were also synthesized. All glycopolymers were assessed in the same experimental setting to avoid biases. Like the pilot study, the exposure to all polymeric glycoconjugates mainly inhibited anti-αGal IgE and IgM isotypes, with a lower inhibition effect on the IgA and IgG isotypes, respectively (Figure 8).
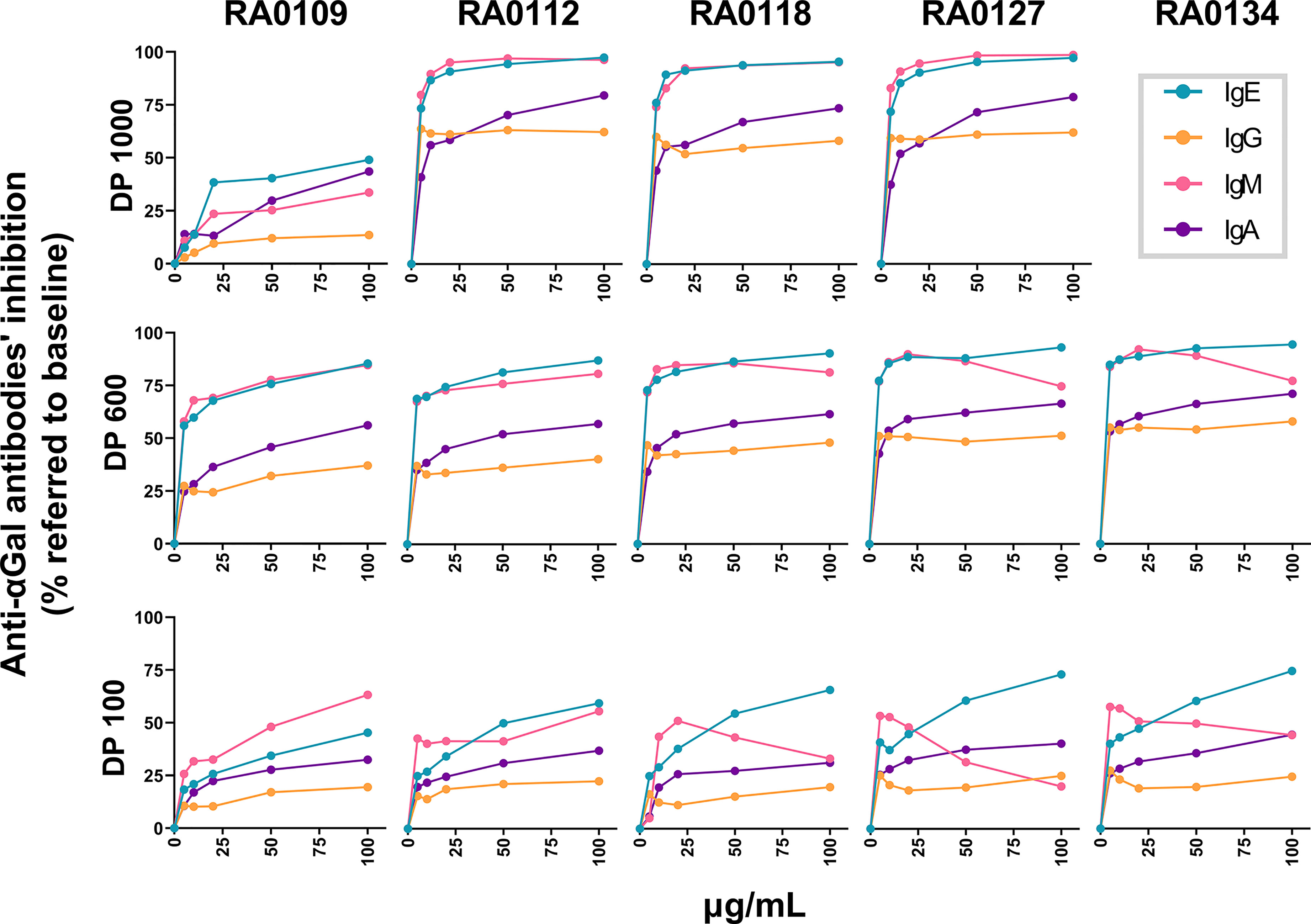
Figure 8 Differential antibody inhibitory capacity of αGal glycoconjugates with different DP and αGal loads in serum from αGal-sensitized patients. Serum samples (n=13) were incubated with the glycopolymers at growing concentrations (5-10-20-50-100 µg/mL). PBS-treated serum was similarly incubated as a baseline condition. After incubation, samples were conveniently diluted to determine by ELISA the unbound fraction of the different anti-αGal isotypes (1:100 for IgM and IgG, 1:25 for IgA, and 1:10 for IgE). The inhibition rate for each glycopolymer (expressed as a percentage of anti-αGal inhibition) was calculated according to the quantity of anti-αGal antibodies determined in baseline (PBS) and treated (glycopolymer) conditions for each serum. IgE green line, IgG orange line, IgM pink line, and IgA purple line.
DP1000-Glycopolymers
From DP1000-RA0112 to DP1000-RA0127, glycoconjugates showed a similar inhibitory capacity for all isotypes at the assessed concentrations. Starting at 10 µg/mL, the inhibition was >85% for IgE and IgM, ~60% for IgG, and 50-80% for IgA. In contrast, the inhibition for DP1000-RA0109 was significantly lower than the other glycopolymers for all Ig-isotypes (Figure 8).
DP600-Glycopolymers
From DP600-RA0112 to DP600-RA0134, glycoconjugates showed a similar inhibitory capacity for all isotypes. From 10 µg/mL, the inhibition was >70% for IgE and IgM, 33-60% for IgG, and 40-72% for IgA. Surprisingly, IgM inhibition rates slightly decreased from 50 µg/mL in the case of RA0118, RA0127, and RA0134. DP600-RA0109 showed a similar trend but with lower inhibition rates. Regardless of the glycan load, all the glycopolymers inhibited >85% of circulating anti-αGal IgE at 100 µg/mL (Figure 8).
DP100-Glycopolymers
From DP100-RA0112, all DP100 glycoconjugates showed similar inhibitory capacity regardless of the Galα1,3Galβ1,4GlcNAc percentage. Starting at 5 µg/mL, the inhibition was 25-75% for IgE, 40-56% for IgM, 12-25% for IgG, and 20-45% for IgA. Similar to DP600, IgM inhibition rates decrease from 20 µg/mL in the case of RA0118, RA0127, and RA0134. To note, DP100-RA0127 and DP100-RA0134 (100 µg/mL) showed a high IgE-inhibition rate (>75%), with minimal IgG inhibition (<25%, Figure 8).
Interestingly, RA0112, RA0118, and RA0127 (DP600) showed similar IgE and IgA inhibitory capacities compared to DP1000 glycopolymers with the same αGal load, except for RA0112 at 10 µg/mL for IgE. Additionally, RA0118 and RA0127 (DP600) showed similar IgM and IgG inhibitory capacity compared to DP1000-homologues.
Discussion
This work shows that immunotherapy based on the intracorporeal inhibition of anti-αGal IgE antibodies with poly-L-lysine-based αGal-glycoconjugates may be a potential solution to treat AGS. The rationale for using poly-L-lysine-based αGal-glycoconjugates on removing anti-αGal antibodies lies in: i) anti-αGal antibodies have naturally high avidity for multivalent antigens, ii) absence of immune response against either the carbohydrate or the poly-L-lysine in different murine and primate species that spontaneously produce the anti-αGal antibodies (53), and iii) anti-AB0 group antibodies (similar in origin and structure to anti-αGal antibodies) have been safely maintained at low concentrations (plasmapheresis plus immunosuppression) for a prolonged time in blood type-incompatible kidney transplantation without side effects (74).
Firstly, elevated levels of anti-αGal IgE antibodies were detected in patients compared to healthy volunteers (67–69). The same trend was observed for the rest of the anti-αGal isotypes. In the initial pilot study, we demonstrated the feasibility of improving the inhibitory capacity of αGal-glycopolymers by reducing the αGal load. This finding was unexpected according to the differences in αGal density in DP1000-RA0118 (180 residues) and GAS914 (270 residues), respectively. An unfavorable spatial conformation due to a higher αGal density in GAS914 compared to DP1000-RA0118 could explain these results (sterical hindrances) (71, 75). Conversely, DP1000-RA0109 (90 residues) showed a drastically reduced capacity to inhibit anti-αGal antibodies. The limited exposure of the antigenic determinant (antigen/antibody ratio) could explain this finding.
Additionally, anti-αGal IgE was the most inhibited isotype in vitro with similar behavior for IgM and a lower inhibition for IgA and IgG, respectively. The later isotypes typically have higher affinities for protein antigens (single binding site) than IgM. However, IgM is a pentamer with ten Fab capable of a multivalent binding (76). In addition, 20% of serological IgA exists as oligomers with multi-binding sites. On the other hand, IgG only exists as a monomer with two binding sites (71). These properties of antibodies were confirmed in our in vitro study, where the IgM avidity was increased, exceeding the affinity of IgA and IgG. Similar results have been reported for polyacrylamide-based αGal-glycoconjugates (71). However, the behavior of anti-αGal IgE, similar to IgM, was unexpected since it is a monomer like IgG (77). Despite some structural differences (78), IgE and IgG share the highest homology compared to other isotypes (78, 79). However, the inhibition of anti-αGal IgG in patients was lower compared to IgE. This finding could be explained by the fact that anti-αGal IgE are strictly induced antibodies, whereas the circulating anti-αGal IgG are mainly composed of natural antibodies. Thus, despite the relatively lower levels of anti-αGal IgE, their affinity seems to be higher than anti-αGal IgG. Consequently, we hypothesize that anti-αGal IgE might come from IgG-switched B cells, unlike the classic atopic sensitization to pollen and mite allergens that come from naive B cells (48). In contrast, previous studies described that anti-αGal IgE might be predominantly formed by class switch from non-switched (IgM) B cells (80).
We also developed a model of αGal-sensitization that reproduced the findings obtained for patients. We achieved a clear induction of anti-αGal IgE, together with IgM and IgG after intradermal administration of hard ticks’ SGE. Unlike natural anti-αGal antibodies (15), anti-αGal IgE are induced after processing the αGal residues in tick saliva by APCs (Langerhans and dermal Dendritic Cells). APCs migrate then to skin draining lymph-node where αGal-specific B cells undergo clonal selection (46). The increased levels of anti-αGal antibodies in sensitized animals confirmed the implication of components from A. sculptum SGE in the induction of anti-αGal antibodies (43, 81).
Furthermore, DP1000-RA0118 showed high efficacy for the intracorporeal removal of anti-αGal in GalT-KO mice. At the cellular level, the WBC population remained unaltered except for basophils that showed a higher fluorescence after the sensitization process in Groups 2 and 3 because of an augmented IgE binding to Fcϵ receptors. In humans, basophil activation can determine the severity of the clinical picture in patients with delayed anaphylaxis due to the consumption of red meat (82, 83).
The immune stimulation with the SGE was not restricted to IgE. Elevated titers of anti-αGal IgG antibodies have been previously described in IgE-positive subjects (67, 80). Specifically, the authors found more anti-αGal IgG1 antibodies in IgE-positive subjects, contrasting the expected more IgG2 production specific for natural anti-αGal antibodies (67). These results suggest that IgE directed to a carbohydrate antigen results from the stimulation of a glycoprotein or glycolipid, even a bacterial immune stimulation with essentially the same antigen already exists (80). In our study, the increase of anti-αGal IgG and IgE directly impacted the rise of total IgG2b and IgE immunoglobulins, respectively.
Additionally, high levels of anti-αGal IgE antibodies in αGal-sensitized patients have been directly correlated to a total IgE antibody increase (9). However, the significant contribution of anti-αGal IgG to the total IgG2b was surprising, indicating a preferential induction of this IgG subclass. GalT-KO mice naturally produce anti-αGal antibodies (15, 70), with IgG3 as the predominant IgG subclass (manuscript submitted to publication). In mice, the IgG3 subclass is functionally equivalent to human IgG2, which predominantly recognizes carbohydrate epitopes (84, 85). Accordingly, two immune responses could be distinguished against the αGal epitope in the animal model: (I) the typical T-independent response promoted by the continuous antigenic stimulation of the intestinal microbiota (15), with IgG3 as the predominant subclass and (II) an “atypical” Th2-response (hypersensitivity type-I) which leads to the production of IgG2b and IgE in GalT-KO mice. This immunological response is similar to that described in humans sensitized after tick bites (9, 80) and in GalT-KO models (46, 86) confirming the robustness of our animal model for αGal sensitization.
DP1000-RA0118 inhibited anti-αGal IgE and IgG, with a discrete impact on the total level of mouse IgE and IgG2b, respectively. However, since the sensitization process was conducted with SGE, we hypothesize that, together with the anti-αGal, other IgE and IgG2b specificities may be induced too. That is why the induction process impacted the total levels of IgE and IgG2b antibodies. Conversely, the treatment with DP1000-RA0118 was specific and only removed the anti-αGal antibodies. Interestingly, total circulating IgM was reduced after DP1000-RA0118 treatment, likely due to the significant contribution of anti-αGal to the circulating IgM repertoire of GalT-KO mice (15).
Finally, glycopolymers with DP<1000 have shown a high capacity to inhibit anti-αGal IgM and IgG in vitro but have been entirely ineffective in vivo (53). However, there is no data regarding the impact of DP on anti-αGal IgE inhibition. In our research, we found that DP100-RA0127 and DP100-RA0134 (100 µg/mL) showed high IgE-inhibition activity (>75%), with reduced IgG inhibition (<25%). This IgE isotype-dependent inhibition might be helpful for IgE-mediated diseases. Despite the in vivo administration of DP1000 αGal-conjugates has shown to be safe in primates and rodents (53), the impact of a lower DP on safety has not been evaluated so far. The polysaccharide chain length affects the immunogenicity of glycan-conjugated vaccines (87). In addition, the polysaccharide hapten size is critical in the immune response to carbohydrate vaccines (88). Indeed, reducing polysaccharide chain lengths improved vaccine immunogenicity (89, 90). Our experience developing monoclonal antibodies using Poly-L-lysine-OVA conjugate as the immunogen shows the poor immunogenicity of DP1000-Poly-L-lysine. Currently, we are improving the immunogenicity of Poly-L-lysine–OVA conjugates by reducing the length of the poly-L-lysine backbone (data not shown). With all these findings, we might expect higher toxicities associated with DP100-glycopolymers than DP1000. Therefore, before studying the efficacy of DP100-αGal-glycopolymers in the selective in vivo removal of IgE over the other anti-αGal isotypes, we consider conducting immunogenicity studies with DP100 crucial to rule out possible safety concerns.
Although the results presented here are promising, they have some limitations. First, the primary objective of this work was to develop a model of αGal-sensitization in mice; however, a more robust model of clinical AGS needs to be addressed to study the clinical impact of DP1000-RA0118 administration. Second, the most promising compounds (including DP100-RA0127 and DP100-RA0134) will need to be tested for their ability to elicit B cell hyporesponsiveness in GalT-KO mice sensitized to αGal. Finally, according to some epidemiological studies, anti-αGal, mainly IgM, may play a protective role against protozoan infections (19–21). Therefore, the potential benefit of having these induced antibodies in regions endemic for protozoan infections must be considered in case our therapy is implemented to treat any disease mediated by anti-αGal IgE. Nevertheless, it is essential to clarify that our therapy produced an immediate but transient inhibition of anti-αGal antibodies. More importantly, our data suggest that it is possible to get a differential anti-αGal isotype inhibition as a function of the length of the poly-L-lysine backbone and total residues of αGal exposure in the polymers.
The present work confirmed that hard ticks’ SGE elements are responsible for the induction of anti-αGal IgE antibodies. We postulate that the αGal sensitization mechanism may go through an “atypical” Th2-response (hypersensitivity type-I), which primarily led to IgG2b and IgE production in GalT-KO mice. Due to the high affinity showed by anti-αGal IgE antibodies for the assessed polymeric αGal-glycoconjugates, we hypothesize that the anti-αGal IgE in sensitized patients might come from IgG-switched B cells, unlike the classic atopic sensitization to pollen and mite allergens where IgE come from naive B cells. We demonstrated the potentiality of poly-L-lysine-based αGal-glycoconjugates for treating allergic disorders mediated by anti-αGal IgE antibodies. As AGS is spreading due to the expansion and changes of hard ticks’ habitats, the immunotherapy concept presented here, based on the selective removal of induced anti-αGal IgE antibodies with poly-L-lysine αGal-glycoconjugates, may provide a clinical solution to this disorder.
Data Availability Statement
The raw data supporting the conclusions of this article will be made available by the authors, without undue reservation.
Ethics Statement
The studies involving human participants were reviewed and approved by Clinical Research Committee of the Hospital Universitari de Bellvitge (PR212/17). The patients/participants provided their written informed consent to participate in this study. The animal study was reviewed and approved by ethics committee for animal experimentation of Bellvitge Biomedical Research Institute (IDIBELL). Serum samples from αGal-sensitized subjects (PT, n=13) were selected from previous epidemiological studies (59), where anti-αGal IgE prevalence of anti-αGal IgE in individuals with acute urticaria or anaphylaxis from different geographical areas of Spain was studied.
Author Contributions
SO-A contributed to the experimental design, performed the experimental work related to animal samples collection, ELISA, and white blood cell profiling, and participated in the manuscript drafting. DB-G designed all the experimental work, coordinated the study, made a substantial contribution to data management and analysis, and wrote the body of the manuscript. RA performed the tick saliva extraction and contributed to the manuscript drafting. YF-A performed the experimental work related to ELISA and white blood cell profiling. BG and ML-H provided the clinical samples and significantly contributed to manuscript drafting. AG-P provided technical insights regarding the preliminary preparation of salivary gland extract and contributed during the manuscript drafting. NB and AT participated in synthetic conjugation strategy and contributed during manuscript drafting. RM significantly contributed to the manuscript conception and drafting. All authors contributed to the article and approved the submitted version.
Conflict of Interest
SO-A, DB-G, and YF-A are employees of RemAb Therapeutics SL. DB-G and RM are shareholders of RemAb Therapeutics SL. SO-A and DB-G hold a patent on new glycoconjugates and medical uses thereof.
The remaining authors declare that the research was conducted in the absence of any commercial or financial relationships that could be construed as a potential conflict of interest.
The authors declare that this study received funding from RemAb Therapeutics SL. The funder had the following involvement in the study: design and complete execution, data generation, interpretation and graphing, article writing, edition, and submission.
Publisher’s Note
All claims expressed in this article are solely those of the authors and do not necessarily represent those of their affiliated organizations, or those of the publisher, the editors and the reviewers. Any product that may be evaluated in this article, or claim that may be made by its manufacturer, is not guaranteed or endorsed by the publisher.
Acknowledgments
The authors want to acknowledge and express their gratitude to Magdiel Pérez-Cruz for initial technical insights related to the animal sensitization model.
Supplementary Material
The Supplementary Material for this article can be found online at: https://www.frontiersin.org/articles/10.3389/fimmu.2022.873019/full#supplementary-material
References
1. Hu J, Chen J, Ye L, Cai Z, Sun J, Ji K. Anti-IgE Therapy for IgE-Mediated Allergic Diseases: From Neutralizing IgE Antibodies to Eliminating IgE+B Cells. Clin Transl Allergy (2018) 8(1):1–8. doi: 10.1186/s13601-018-0213-z
2. D’Amato G, Chong-Neto HJ, Monge Ortega OP, Vitale C, Ansotegui I, Rosario N, et al. The Effects of Climate Change on Respiratory Allergy and Asthma Induced by Pollen and Mold Allergens. Allergy Eur J Allergy Clin Immunol (2020) 75(9):2219–28. doi: 10.1111/all.14476
3. Sicherer SH, Sampson HA. Food Allergy: A Review and Update on Epidemiology, Pathogenesis, Diagnosis, Prevention, and Management. J Allergy Clin Immunol (2018) 141(1):41–58. doi: 10.1016/j.jaci.2017.11.003
4. Ring J, Akdis C, Lauener R, Schäppi G, Traidl-Hoffmann C, Akdis M, et al. Global Allergy Forum and Second Davos Declaration 2013 Allergy: Barriers to Cure - Challenges and Actions to be Taken. Allergy Eur J Allergy Clin Immunol (2014) 69(8):978–82. doi: 10.1111/all.12406
5. Kopp MV. Omalizumab: Anti-IgE Therapy in Allergy. Curr Allergy Asthma Rep (2011) 11(2):101–6. doi: 10.1007/s11882-010-0173-4
6. Fitzsimmons CM, Falcone FH, Dunne DW. Helminth Allergens, Parasite-Specific IgE, and Its Protective Role in Human Immunity. Front Immunol (2014) 5(FEB):1–13. doi: 10.3389/fimmu.2014.00061
7. Levin ME, Le Souëf PN, Motala C. Total IgE in Urban Black South African Teenagers: The Influence of Atopy and Helminth Infection. Pediatr Allergy Immunol (2008) 19(5):449–54. doi: 10.1111/j.1399-3038.2007.00663.x
8. Galili U. Anti-Gal: An Abundant Human Natural Antibody of Multiple Pathogeneses and Clinical Benefits. Immunology (2013) 140(1):1–11. doi: 10.1111/imm.12110
9. Commins SP, James HR, Kelly LA, Pochan SL, Workman LJ, Perzanowski MS, et al. The Relevance of Tick Bites to the Production of IgE Antibodies to the Mammalian Oligosaccharide Galactose-α-1,3-Galactose. J Allergy Clin Immunol (2011) 127(5):1286–1293.e6. doi: 10.1016/j.jaci.2011.02.019
10. Jappe U, Minge S, Kreft B, Ludwig A, Przybilla B, Walker A, et al. Meat Allergy Associated With Galactosyl-a-(1,3)-Galactose (a-Gal)—Closing Diagnostic Gaps by Anti-a-Gal IgE Immune Profiling. Allergy (2018) 73:93–105. doi: 10.1111/all.13238
11. Parker W, Lin SS, Yu PB, Sood A, Nakamura YC, Song A, et al. Naturally Occurring Anti-α-Galactosyl Antibodies: Relationship to Xenoreactive Anti-α-Galactosyl Antibodies. Glycobiology (1999) 9(9):865–73. doi: 10.1093/glycob/9.9.865
12. Galili U, Matta KL. Inhibition of Anti-Gal IgG Binding to Porcine Endothelial Cellss by Synthetic Oligosaccharides. Transplantation (1996) 62(2):256–62. doi: 10.1097/00007890-199607270-00018
13. Galili U. Significance of the Evolutionary α1,3-Galactosyltransferase (GGTA1) Gene Inactivation in Preventing Extinction of Apes and Old World Monkeys. J Mol Evol (2015) 80(1):1–9. doi: 10.1007/s00239-014-9652-x
14. Galili U, Macher BA, Buehler J, Shohet SB. Human Natural Anti-α-Galactosyl IgG: II. The Specific Recognition of a(1 → 3)-Linked Galactose Residues. J Exp Med (1985) 162(2):573–82. doi: 10.1084/jem.162.2.573
15. Bello-Gil D, Audebert C, Olivera-Ardid S, Pérez-Cruz M, Even G, Khasbiullina N, et al. The Formation of Glycan-Specific Natural Antibodies Repertoire in GalT-KO Mice Is Determined by Gut Microbiota. Front Immunol (2019) 10:342/full(March). doi: 10.3389/fimmu.2019.00342/full
16. Boussamet L, Montassier E, Soulillou JP, Berthelot L. Anti α1-3Gal Antibodies and Gal Content in Gut Microbiota in Immune Disorders and Multiple Sclerosis. Clin Immunol (2021) :108693. doi: 10.1016/j.clim.2021.108693
17. Cabezas-Cruz A, de la Fuente J. Immunity to α-Gal: The Opportunity for Malaria and Tuberculosis Control. Front Immunol (2017) 8(DEC):1–6. doi: 10.3389/fimmu.2017.01733
18. Fontán MP, Máñez R, Rodríguez-Carmona A, Peteiro J, Martínez V, García-Falcón T, et al. Serum Levels of Anti-αgalactosyl Antibodies Predict Survival and Peritoneal Dialysis-Related Enteric Peritonitis Rates in Patients Undergoing Renal Replacement Therapy. Am J Kidney Dis (2006) 48(6):972–82. doi: 10.1053/j.ajkd.2006.08.027
19. Aguilar R, Ubillos I, Vidal M, Balanza N, Crespo N, Jiménez A, et al. Antibody Responses to α -Gal in African Children Vary With Age and Site and are Associated With Malaria Protection. Sci Rep (2018) 8(June):1–15. doi: 10.1038/s41598-018-28325-w
20. Yilmaz B, Portugal S, Tran TM, Gozzelino R, Ramos S, Gomes J, et al. Gut Microbiota Elicits a Protective Immune Response Against Malaria Transmission. Cell (2014) 159(6):1277–89. doi: 10.1016/j.cell.2014.10.053
21. Cabezas-Cruz A, Mateos-Hernández L, Alberdi P, Villar M, Riveau G, Hermann E, et al. Effect of Blood Type on Anti-a-Gal Immunity and the Incidence of Infectious Diseases. Exp Mol Med (2017) 49(3):e301. doi: 10.1038/emm.2016.164
22. Portillo S, Zepeda BG, Iniguez E, Olivas JJ, Karimi NH, Moreira OC, et al. A Prophylactic α-Gal-Based Glycovaccine Effectively Protects Against Murine Acute Chagas Disease. NPJ Vaccines (2019) 4(1):13. doi: 10.1038/s41541-019-0107-7
23. Mateos-Hernández L, Risco-Castillo V, Torres-Maravilla E, Bermúdez-Humarán LG, Alberdi P, Hodžić A, et al. Gut Microbiota Abrogates Anti-α-Gal Iga Response in Lungs and Protects Against Experimental Aspergillus Infection in Poultry. Vaccines (2020) 8(2):1–21. doi: 10.3390/vaccines8020285
24. Commins SP, Platts-Mills TAE. Delayed Anaphylaxis to Red Meat in Patients With Ige Specific for Galactose Alpha-1,3-Galactose (Alpha-Gal). Curr Allergy Asthma Rep (2013) 13(1):72–7. doi: 10.1007/s11882-012-0315-y
25. Castells M. Diagnosis and Management of Anaphylaxis in Precision Medicine. J Allergy Clin Immunol (2017) 140(2):321–33. doi: 10.1016/j.jaci.2017.06.012
26. Stewart PH, McMullan KL, Leblanc SB. Delayed Red Meat Allergy: Clinical Ramifications of Galactose-α-1,3-Galactose Sensitization. Ann Allergy Asthma Immunol (2015) 115(4):260–4. doi: 10.1016/j.anai.2015.08.003
27. Fischer J, Lupberger E, Hebsaker J, Blumenstock G, Aichinger E, Yazdi AS, et al. P. Prevalence of Type I Sensitization to Alpha-Gal in Forest Service Employees and Hunters. Allergy (2017) 72:1540–7. doi: 10.1111/all.13156
28. Gilbert L. The Impacts of Climate Change on Ticks and Tick-Borne Disease Risk. Annu Rev Entomol (2021) 66(1):373–88. doi: 10.1146/annurev-ento-052720-094533
29. Van Nunen SA, O’Connor KS, Clarke LR, Boyle RX, Fernando SL. An Association Between Tick Bite Reactions and Red Meat Allergy in Humans | Medical Journal of Australia. Med J Aust (2009) 190(9):510–1. doi: 10.5694/j.1326-5377.2009.tb02533.x
30. Carter MC, Ruiz-Esteves KN, Workman L, Lieberman P, Platts-Mills TAE MD. Identification of Alpha-Gal Sensitivity in Patients With a Diagnosis of Idiopathic Anaphylaxis. Allergy (2017) 73:1131–4. doi: 10.1111/all.13366
31. Jappe U. Allergieauf Säugetierfleisch: α-Gal: Neues Epitop, Neue Entität? Hautarzt (2012) 63(4):299–306. doi: 10.1007/s00105-011-2266-y
32. Morisset M, Richard C, Astier C, Jacquenet S, Croizier A, Beaudouin E, et al. Anaphylaxis to Pork Kidney is Related to IgE Antibodies Specific for Galactose-Alpha-1,3-Galactose. Allergy Eur J Allergy Clin Immunol (2012) 67(5):699–704. doi: 10.1111/j.1398-9995.2012.02799.x
33. Nuñez R, Carballada F, Gonzalez-Quintela A, Gomez-Rial J, Boquete M, Vidal C. Delayed Mammalian Meat-Induced Anaphylaxis Due to Galactose-α-1,3- Galactose in 5 European Patients. J Allergy Clin Immunol (2011) 128(5):1122–4.e1. doi: 10.1016/j.jaci.2011.07.020
34. Uyttebroek A, Sabato V, Bridts CH, De Clerck LS, Ebo DG. Anaphylaxis to Succinylated Gelatin in a Patient With a Meat Allergy: Galactose-α(1, 3)-Galactose (α-Gal) as Antigenic Determinant. J Clin Anesth (2014) 26(7):574–6. doi: 10.1016/j.jclinane.2014.04.014
35. Michel S, Scherer K, Heijnen IAFM, Bircher AJ. Skin Prick Test and Basophil Reactivity to Cetuximab in Patients With IgE to Alpha-Gal and Allergy to Red Meat. Allergy Eur J Allergy Clin Immunol (2014) 69(3):403–5. doi: 10.1111/all.12344
36. Hamsten C, Starkhammar M, Tran TAT, Johansson M, Bengtsson U, Ahlén G, et al. Identification of Galactose-α-1,3-Galactose in the Gastrointestinal Tract of the Tick Ixodes Ricinus; Possible Relationship With Red Meat Allergy. Allergy Eur J Allergy Clin Immunol (2013) 68(4):549–52. doi: 10.1111/all.12128
37. Harper V, Wagner A, Sowa P, Rutkowski K. Allergy in Frequent Travelers: The Alpha-Gal Story and the First UK Case Series. Ann Allergy Asthma Immunol (2019) 123(6):616–8. doi: 10.1016/j.anai.2019.09.018
38. Calamari AM, Poppa M, Villalta D, Pravettoni V. Alpha-Gal Anaphylaxis: The First Case Report in Italy. Eur Ann Allergy Clin Immunol (2015) 47(5):161–2.
39. Arslan Lied G. Red Meat Allergy Induced by Tick Bites: A Norwegian Case Report. Eur Ann Allergy Clin Immunol (2017) 49(4):186–8. doi: 10.23822/EurAnnACI.1764-1489.04
40. Lee JH, Kim JH, Kim TH, Kim SC. Delayed Mammalian Meat-Induced Anaphylaxis Confirmed by Skin Test to Cetuximab. J Dermatol (2013) 40(7):577–8. doi: 10.1111/1346-8138.12140
41. Chinuki Y, Ishiwata K, Yamaji K, Takahashi H, Morita E. Haemaphysalis Longicornis Tick Bites are a Possible Cause of Red Meat Allergy in Japan. Allergy Eur J Allergy Clin Immunol (2016) 71(3):421–5. doi: 10.1111/all.12804
42. Wickner PG, Commins SP. The First 4 Central American Cases Of Delayed Meat Allergy With Galactose-Alpha-1,3-Galactose Positivity Clustered Among Field Biologists In Panama. J Allergy Clin Immunol Elsevier Ltd (2014) 133:AB212. doi: 10.1016/j.jaci.2013.12.760
43. Araujo RN, Franco PF, Rodrigues H, Santos LCB, McKay CS, Sanhueza CA, et al. Amblyomma Sculptum Tick Saliva: α-Gal Identification, Antibody Response and Possible Association With Red Meat Allergy in Brazil. Int J Parasitol (2016) 46(3):213–20. doi: 10.1016/j.ijpara.2015.12.005
44. Kaloga M, Kourouma S, Kouassi YI, Ecra EJ, Gbery IP, Allou AS, et al. Allergy to Red Meat: A Diagnosis Made by the Patient and Confirmed by an Assay for IgE Antibodies Specific for Alpha-1,3-Galactose. Case Rep Dermatol (2016) 8(1):10–3. doi: 10.1159/000443631
45. Kwak M, Somerville C, van Nunen S. A Novel Australian Tick Ixodes (Endopalpiger) Australiensis Inducing Mammalian Meat Allergy After Tick Bite. Asia Pac Allergy (2018) 8(3):8–11. doi: 10.5415/apallergy.2018.8.e31
46. Chandrasekhar JL, Cox KM, Erickson LD. B Cell Responses in the Development of Mammalian Meat Allergy. Front Immunol (2020) 11(July):1–16. doi: 10.3389/fimmu.2020.01532
47. Berg EA, Platts-Mills TAE, Commins SP. Drug Allergens and Food - The Cetuximab and Galactose-α-1,3-Galactose Story. Ann Allergy Asthma Immunol (2014) 112(2):97–101. doi: 10.1016/j.anai.2013.11.014
48. Davies JM, Platts-Mills TA, Aalberse RC. The Enigma of IgE+ B-Cell Memory in Human Subjects. J Allergy Clin Immunol (2013) 131(4):972–6. doi: 10.1016/j.jaci.2012.12.1569
49. Commins SP, Satinover SM, Hosen J, Mozena J, Borish L, Lewis BD, et al. Delayed Anaphylaxis, Angioedema, or Urticaria After Consumption of Red Meat in Patients With IgE Antibodies Specific for Galactose-α-1,3-Galactose. J Allergy Clin Immunol (2009) 123(2):426–33.e2. doi: 10.1016/j.jaci.2008.10.052
50. Platts-Mills TAE, Schuyler AJ, Hoyt AEW, Commins SP. Delayed Anaphylaxis Involving IgE to Galactose-Alpha-1,3-Galactose. Curr Allergy Asthma Rep (2015) 15(4):1–6. doi: 10.1007/s11882-015-0512-6
51. Commins SP, Jerath MR, Cox K, Erickson LD, Platts-Mills T. Delayed Anaphylaxis to Alpha-Gal, an Oligosaccharide in Mammalian Meat. Allergol Int (2016) 65(1):16–20. doi: 10.1016/j.alit.2015.10.001
52. Kiewiet MBG, Apostolovic D, Starkhammar M, Grundström J, Hamsten C, van Hage M. Clinical and Serological Characterization of the α-Gal Syndrome—Importance of Atopy for Symptom Severity in a European Cohort. J Allergy Clin Immunol Pract (2020) 8(6):2027–2034.e2. doi: 10.1016/j.jaip.2020.02.016
53. Katopodis AG, Warner RG, Duthaler RO, Streiff MB, Bruelisauer A, Kretz O, et al. Removal of Anti-Galα1,3Gal Xenoantibodies With an Injectable Polymer. J Clin Invest (2002) 110(12):1869–77. doi: 10.1172/JCI16526
54. Tseng YL, Moran K, Dor FJMF, Sanderson TM, Li W, Lancos CJ, et al. Elicited Antibodies in Baboons Exposed to Tissues From α1,3-Galactosyltransferase Gene-Knockout Pigs. Transplantation (2006) 81(7):1058–62. doi: 10.1097/01.tp.0000197555.16093.98
55. Lai L, Kolber-simonds D, Park K, Cheong H, Greenstein JL, Im G, et al. Production of Knockout Pigs by Nuclear Transfer Cloning. Science (80-) (2002) 295(February):1089–92. doi: 10.1126/science.1068228
56. Reardon S. First Pig-To-Human Heart Transplant: What can Scientists Learn? Pract Manag (2021) 31(4):8–16. doi: 10.1038/d41586-022-00111-9
57. Hadjichristidis N, Iatrou H, Pitsikalis M, Sakellariou G. Synthesis of Well-Defined Polypeptide-Based Materials via the Ring-Opening Polymerization of α-Amino Acid N-Carboxyanhydrides. Chem Rev (2009) 109(11):5528–78. doi: 10.1021/cr900049t
58. Duthaler RO, Ernst B, Fischer R, Katopodis AG, Kinzy W, Marterer W, et al. In Vivo Neutralization of Naturally Existing Antibodies Against Linear α (1,3)-Galactosidic Carbohydrate Epitopes by Multivalent Antigen Presentation: A Solution for the First Hurdle of Pig-to-Human Xenotransplantation. Chimia (Aarau) (2010) 64(1–2):23–8. doi: 10.2533/chimia.2010.23
59. Mateo-Borrega MB, Garcia B, Larramendi CH, Azofra J, González-Mancebo E, Alvarado MI, et al. Ige-Mediated Sensitization to Galactose-α-1,3-Galactose (α-Gal) in Urticaria and Anaphylaxis in Spain: Geographical Variations and Risk Factors. J Investig Allergol Clin Immunol (2019) 29(6):436–43. doi: 10.18176/jiaci.0373
60. Costa C, Brokaw JL, Wang Y, Fodor WL. Delayed Rejection of Porcine Cartilage is Averted by Transgenic Expression of Alpha1,2-Fucosyltransferase. FASEB J (2003) 17(1):109–11. doi: 10.1096/fj.02-0630fje
61. Franco PF, Silva NC, Fazito do Vale V, Abreu JF, Santos VC, Gontijo NF, et al. Inhibition of the Classical Pathway of the Complement System by Saliva of Amblyomma Cajennense (Acari: Ixodidae). Physiol Behav (2019) 176(3):139–48. doi: 10.1016/j.exppara.2016.03.002
62. A rapid MMB. And Sensitive Method for the Quantitation of Microgram Quantities of Protein Utilizing the Principle of Protein-Dye Binding. Anal Biochem (1976) 72:248–54. doi: 10.1016/0003-2697(76)90527-3
63. Togbe D, Fauconnier L, Madouri F, Marchiol T, Chenuet P, Rouxel N, et al. Thymic Stromal Lymphopoietin Enhances Th2/Th22 and Reduces IL-17A in Protease-Allergen-Induced Airways Inflammation. Fukushima A, Comeau MR, Gibbs BF, Editors. ISRN Allergy (2013) 2013:971036. doi: 10.1155/2013/971036
64. Choudhary SK, Karim S, Iweala OI, Choudhary S, Crispell G, Sharma SR, et al. Tick Salivary Gland Extract Induces Alpha-Gal Syndrome in Alpha-Gal Deficient Mice. Immunity Inflamm Dis (2021) 9(3):984–90. doi: 10.1002/iid3.457
65. Guidelines for Blood Collection in Mice and Rats (2021). Available at: https://oacu.oir.nih.gov/system/files/media/file/2021-02/b2_blood_collection_in_mice_and_rats.pdf.
66. Golde WT, Gollobin P, Rodriguez LL. A Rapid, Simple, and Humane Method for Bleeding of Mice. Lab Anim (NY) (2005) 34(9):39–43. doi: 10.1038/laban1005-39
67. Kollmann D, Nagl B, Ebner C, Emminger W, Wöhrl S, Kitzm Müller C, et al. The Quantity and Quality of α-Gal-Specific Antibodies Differs in Individuals With and Without Delayed Red Meat Allergy. Allergy (2017) 72:266–73. doi: 10.1111/all.12948
68. Bianchi J, Walters A, Fitch ZW, Turek JW. Alpha-Gal Syndrome: Implications for Cardiovascular Disease. Glob Cardiol Sci Pract (2020) 2019(3):0–16. doi: 10.21542/gcsp.2019.20
69. Apostolovic D, Tran TAT, Sanchez-Vidaurre S, Cirkovic Velickovic T, Starkhammar M, Hamsten C van HMR. Red Meat Allergic Patients Have a Selective IgE Response to the α-Gal Glycan. Allergy (2015) 70:1497–500. doi: 10.1111/all.12695
70. Cruz MP, Gil DB, Costa C, Mañez R. Cytokine Profile Associated With Selective Removal of Natural Anti-αgal Antibodies in a Sepsis Model in Gal-KO Mice. Biochem (2017) 82(2):205–12. doi: 10.1134/S0006297917020122
71. Wang JQ, Chen X, Zhang W, Zacharek S, Chen Y, Wang PG. Enhanced Inhibition of Human Anti-Gal Antibody Binding to Mammalian Cells by Synthetic α-Gal Epitope Polymers. J Am Chem Soc (1999) 121(36):8174–81. doi: 10.1021/ja990219h
72. Choudhary SK, Karim S, Iweala OI, Choudhary S, Crispell G, Sharma SR, et al. T. Tick Salivary Gland Extract Induces Alpha-Gal Syndrome in Alpha-Gal Deficient Mice. Immun Inflamm Dis (2021) 9:984–90. doi: 10.1002/iid3.457
73. Ohdan H, Swenson KG, Kruger Gray HS, Yang Y-G, Xu Y, Thall AD, et al. Mac-1-Negative B-1b Phenotype of Natural Antibody-Producing Cells, Including Those Responding to Galα1,3gal Epitopes in α1,3-Galactosyltransferase-Deficient Mice. J Immunol (2000) 165(10):5518–29. doi: 10.4049/jimmunol.165.10.5518
74. Lipshutz GS, McGuire S, Zhu Q, Ziman A, Davis R, Goldfinger D, et al. ABO Blood Type-Incompatible Kidney Transplantation and Access to Organs. Arch Surg (2011) 146(4):453–8. doi: 10.1001/archsurg.2011.40
75. Uvyn A, De Geest BG. Multivalent Antibody-Recruiting Macromolecules: Linking Increased Binding Affinity With Enhanced Innate Immune Killing. ChemBioChem (2020) 21(21):3036–43. doi: 10.1002/cbic.202000261
76. Muthana SM, Xia L, Campbell CT, Zhang Y, Gildersleeve JC. Competition Between Serum IgG, IgM, and IgA Anti-Glycan Antibodies. PloS One (2015) 10(3):1–16. doi: 10.1371/journal.pone.0119298
77. Eisen HN. Affinity Enhancement of Antibodies: How Low-Affinity Antibodies Produced Early in Immune Responses Are Followed by High-Affinity Antibodies Later and in Memory B-Cell Responses. Cancer Immunol Res (2014) 2(5):381–92. doi: 10.1158/2326-6066.CIR-14-0029
78. Sutton B, Davies A, Bax H, Karagiannis S. IgE Antibodies: From Structure to Function and Clinical Translation. Antibodies (2019) 8(1):19. doi: 10.3390/antib8010019
79. Wurzburg BA, Garman SC, Jardetzky TS. Structure of the Human IgE-Fc Cϵ3-Cϵ4 Reveals Conformational Flexibility in the Antibody Effector Domains. Immunity (2000) 13(3):375–85. doi: 10.1016/S1074-7613(00)00037-6
80. Rispens T, Derksen NIL, Commins SP, Platts-Mills TA, Aalberse RC. IgE Production to α-Gal Is Accompanied by Elevated Levels of Specific IgG1 Antibodies and Low Amounts of IgE to Blood Group B. PloS One (2013) 8(2):1–7. doi: 10.1371/journal.pone.0055566
81. Commins SP, Karim S. Development of a Novel Murine Model of Alpha-Gal Meat Allergy. J Allergy Clin Immunol (2017) 139(2):AB193. doi: 10.1016/j.jaci.2016.12.628
82. Choudhary SK, Commins SP. Association of Alpha-Gal Red Meat Allergy Severity With Concentration of Antigen in Basophil Activation. J Allergy Clin Immunol (2017) 139(2):AB125. doi: 10.1016/j.jaci.2016.12.406
83. Commins SP, James HR, Stevens W, Pochan SL, Land MH, King C, et al. Delayed Clinical and Ex Vivo Response to Mammalian Meat in Patients With IgE to Galactose-Alpha-1,3-Galactose. J Allergy Clin Immunol (2014) 134(1):108–15. doi: 10.1016/j.jaci.2014.01.024
84. Hussain R, Dawood G, Abrar N, Toossi Z, Minai A, Dojki M, et al. Selective Increases in Antibody Isotypes and Immunoglobulin G Subclass Responses to Secreted Antigens in Tuberculosis Patients and Healthy Household Contacts of the Patients. Clin Diagn Lab Immunol (1995) 2(6):726–32. doi: 10.1128/cdli.2.6.726-732.1995
85. Apostolovic D, Rodrigues R, Thomas P, Starkhammar M, Hamsten C van HM. Immunoprofile of a-Gal- and B-Antigen-Specific Responses Differentiates Red Meat- Allergic Patients From Healthy Individuals. Allergy Allergy (2018) 73:1525–31. doi: 10.1111/all.13400
86. Chandrasekhar JL, Cox KM, Loo WM, Qiao H, Tung KS, Erickson LD. Cutaneous Exposure to Clinically Relevant Lone Star Ticks Promotes IgE Production and Hypersensitivity Through CD4 + T Cell– and MyD88-Dependent Pathways in Mice. J Immunol (2019) 203(4):813–24. doi: 10.4049/jimmunol.1801156
87. Anish C, Beurret M, Poolman J. Combined Effects of Glycan Chain Length and Linkage Type on the Immunogenicity of Glycoconjugate Vaccines. NPJ Vaccines (2021) 6(1):150. doi: 10.1038/s41541-021-00409-1
88. Bröker M, Berti F, Costantino P. Factors Contributing to the Immunogenicity of Meningococcal Conjugate Vaccines. Hum Vaccines Immunother (2016) 12(7):1808–24. doi: 10.1080/21645515.2016.1153206
89. Rana R, Dalal J, Singh D, Kumar N, Hanif S, Joshi N, et al. Development and Characterization of Haemophilus Influenzae Type B Conjugate Vaccine Prepared Using Different Polysaccharide Chain Lengths. Vaccine (2015) 33(23):2646–54. doi: 10.1016/j.vaccine.2015.04.031
Keywords: αGal-syndrome, poly-L-lysine-based αGal-glycoconjugates, anti-αGal IgE inhibition, GalT-KO mice, immunotherapy
Citation: Olivera-Ardid S, Bello-Gil D, Tuzikov A, Araujo RN, Ferrero-Alves Y, García Figueroa BE, Labrador-Horrillo M, García-Pérez AL, Bovin N and Mañez R (2022) Poly-L-Lysine-Based αGal-Glycoconjugates for Treating Anti-αGal IgE-Mediated Diseases. Front. Immunol. 13:873019. doi: 10.3389/fimmu.2022.873019
Received: 10 February 2022; Accepted: 02 March 2022;
Published: 31 March 2022.
Edited by:
Pedro A Reche, Complutense University of Madrid, SpainReviewed by:
Lourdes Mateos Hernández, Agence Nationale de Sécurité Sanitaire de l’Alimentation, de l’Environnement et du Travail (ANSES), FranceSaid Rabbani, University of Basel, Switzerland
Copyright © 2022 Olivera-Ardid, Bello-Gil, Tuzikov, Araujo, Ferrero-Alves, García Figueroa, Labrador-Horrillo, García-Pérez, Bovin and Mañez. This is an open-access article distributed under the terms of the Creative Commons Attribution License (CC BY). The use, distribution or reproduction in other forums is permitted, provided the original author(s) and the copyright owner(s) are credited and that the original publication in this journal is cited, in accordance with accepted academic practice. No use, distribution or reproduction is permitted which does not comply with these terms.
*Correspondence: Daniel Bello-Gil, ZGFuaWVsLmJlbGxvQHJlbWFidHguY29t