- 1Clinic of Operative Dentistry, Periodontology and Preventive Dentistry, Saarland University, Homburg, Germany
- 2Department of Environment & Biodiversity, University of Salzburg, Salzburg, Austria
- 3Department of Dental Pathology, University of East Sarajevo, East Sarajevo, Bosnia and Herzegovina
- 4Department of Internal Medicine 3 - Rheumatology and Immunology, Friedrich-Alexander-University Erlangen-Nürnberg (FAU) and Universitätsklinikum Erlangen, Erlangen, Germany
- 5Deutsches Zentrum für Immuntherapie (DZI), Friedrich-Alexander-University Erlangen-Nürnberg and Universitätsklinikum Erlangen, Erlangen, Germany
The frequent severe COVID-19 course in patients with periodontitis suggests a link of the aetiopathogenesis of both diseases. The formation of intravascular neutrophil extracellular traps (NETs) is crucial to the pathogenesis of severe COVID-19. Periodontitis is characterised by an increased level of circulating NETs, a propensity for increased NET formation, delayed NET clearance and low-grade endotoxemia (LGE). The latter has an enormous impact on innate immunity and susceptibility to infection with SARS-CoV-2. LPS binds the SARS-CoV-2 spike protein and this complex, which is more active than unbound LPS, precipitates massive NET formation. Thus, circulating NET formation is the common denominator in both COVID-19 and periodontitis and other diseases with low-grade endotoxemia like diabetes, obesity and cardiovascular diseases (CVD) also increase the risk to develop severe COVID-19. Here we discuss the role of propensity for increased NET formation, DNase I deficiency and low-grade endotoxaemia in periodontitis as aggravating factors for the severe course of COVID-19 and possible strategies for the diminution of increased levels of circulating periodontitis-derived NETs in COVID-19 with periodontitis comorbidity.
Introduction
Periodontitis has been considered not a consequence of basic alteration of the oral microbiota, but rather of the inability of the host immunity to resolve chronic inflammation (1–3). Indeed, the capacity of certain bacteria to act as a commensal or pathogen is highly dependent on the immune status of the host (4). Periodontitis patients with late onset have low-grade endotoxaemia (LGE), systemic low-grade inflammation (SLGI) (5) and display neutrophil hyper-responsiveness (6–9). Neutrophils play a central role in the control of bacterial infections and also are the effector immune cells responsible for the antimicrobial defence in the gingiva. They are the first defenders recruited to the sites of bacterial invasion (1, 2, 10). The indispensable role of neutrophils is illustrated by the inevitable development of early-onset periodontitis in patients with neutropenia and with congenital defects of leukocyte adhesion (1). Neutrophils do not recognise individual pathogens or pathogen species, but common danger signals such as: chemokines, cytokines, immune complexes, pathogen-associated molecular patterns (PAMPs), damage-associated molecular patterns (DAMPs), and certain proteins of the complement system (11). PMN hyper-reactivity is characteristic of periodontitis with late onset and can evoke a strong inflammatory response even at low levels of bacterial challenge. Consequently, neutrophils can transform from defenders against pathogens to tissue destroyers regardless of the bacterial challenge (11, 12).
NETs are part of the antimicrobial arsenal of neutrophils (13–17). They are evolutionarily conserved chromatin threads produced by activated neutrophils in response to pathogen challenge. NETs consist of a scaffold of chromatin with histones and neutrophil-derived antimicrobials, such as: proteases, lactoferrin, cathepsins and myeloperoxidase (MPO) (13, 14, 18). NET formation can be triggered via receptors sensing chemokines and cytokines, immune complexes, PAMPs, DAMPs, some complement components (19–21), via pH regulation (22), and via activating the caspase-4/5/GSDMD pathways (23). Human caspases 4 and 5 are receptors for cytosolic LPS (24). Many of the mechanisms for NET formation are linked to the NADPH oxidase (NOX) machinery, but NOX-independent NET formation has also been described (23, 25). Upon activation, the azurophilic granular proteins neutrophil elastase (NE) and MPO translocate to the nucleus to promote chromatin decondensation (26). Histone citrullination by peptidylarginine deiminase 4 (PAD4) further supports this (27, 28). In addition, reactive oxygen species- (ROS), NE- and PAD4-independent pathways for NET formation have been reported. Such as the caspase-4/11-induced NET formation that proceeds independently of MPO, NE, and PAD4 (23).
Circulating NETs can exert strong proinflammatory effects. NETs induce the inflammasome (29), type I interferons and further pro-inflammatory cytokines; they damage the endothelium (30) and can occlude ducts in various organs, promoting organ damage (31–39). In patients with COVID-19 aggregated NETs (aggNETs), can obstruct small and intermediate-sized pulmonary vessels and precipitate COVID-19 pathology (40). Recently, an association between periodontal disease and an increased risk of COVID-19 infection has been reported (41). Periodontitis was associated with a higher risk of intensive care unit (ICU) admission, need for assisted ventilation and death of patients with COVID-19 (41–43). These findings identify periodontitis as comorbidity that drives COVID-19. Both COVID-19 (44–46) and periodontitis (5) share a dysregulated innate immunity.
The aim of this review is to discuss the disbalanced NET formation in periodontitis, especially in the context of COVID-19. Furthermore, we want to highlight possible approaches to counteract the increased level of NETs reported in periodontitis with late onset.
NETs in Periodontitis With and Without COVID-19 Comorbidity
Sources of Circulating NETs in COVID-19 and Periodontitis
NETs in blood can be directly demonstrated by vascular biopsies (40), but this approach is inapplicable for clinical purposes. Blood-born NETs are physiologically digested by DNase 1 into cell-free DNA (cfDNA). The main source of blood-born cfDNA originates from cell necrosis and apoptosis, as cases of neoplasms are, as well as NETs (47). Thus, patients with severe COVID-19 had an increased level of nuclear cfDNA (copies/mL), as compared to hospitalised non-ICU COVID-19 patients and the cfDNA sequencing identified the neutrophils as a predominant cfDNA source (48). Clinically, cfDNA is highlighted as a non-invasive biomarker for COVID-19 severity (49). Additionally, topical formation of NETs, as the case with periodontitis is (50–52) and neutrophil hyper-responsiveness both in periodontitis (6–9) and COVID-19 (53) are strong indicators of blood-born NETs. It has been demonstrated that the circulating carbamylated protein (54, 55) and NET levels are associated with periodontitis severity (56). Blood cfDNA is significantly increased in patients with periodontitis, as compared to orally healthy subjects (57). Thus in periodontitis, topical sources of NETs, neutrophil hyper-responsiveness and circulating NETs alter the host reactivity in the same way as in severe COVID-19 and provide the requirements for aggravation of NET-driven host damages.
Increased NET Formation Due to Trained Immunity
Most proinflammatory gene loci in the quiescent myeloid cells are in a repressed configuration (58), hindering access of the transcriptional machinery to the regulatory regions that drive the expression of inflammatory factors (59). The so-termed “trained immunity” is development of a long-term functional reprogramming of the hematopoietic stem and progenitor cells (HSPCs) evoked by exogenous or endogenous insults, e.g. low level LPS due to LGE, as the case in periodontitis with late onset is. Subsequently, this functional reprogramming of HSPCs leads to an altered responsiveness of differentiated myeloid cells towards a second challenge after returning to a non-activated state (60). The secondary response to the subsequent non-specific stimulus can be altered in such a way that the cells respond more or less strongly when compared to the primary response, conferring context- and time-adjusted responses (60). Thus, low level LPS-exposed HSPCs become epigenetically primed for a myeloid lineage bias with enhancers remaining more accessible than in naive HSPCs. Furthermore, low level LPS-exposed HSPCs keep increased accessibility of numerous genes that predispose to more rapid activation of myeloid lineage commitment in response to secondary stimulation (61). As the innate memory responses depend solely on epigenetic remodelling, the trained immunity lacks specificity. Trained neutrophils are prone to increased NET formation (62, 63). Continuous LGE promotes systemic low-grade inflammation (SLGI) and subsequently dysregulated trained immunity in periodontitis with late onset (3, 5, 64). Thus, the NET hyper-responsiveness might be a main factor for increased NET formation in periodontitis patients and hence responsible for a more severe course of COVID-19 with periodontitis comorbidity (41, 42).
Impaired DNA Degradation
The disrupted balance between NET formation and degradation appears to play a crucial role in the pathophysiology of inflammation, coagulopathy, organ damage and immunothrombosis that characterise severe cases of COVID-19 (45). In general, impaired NET degradation results in surplus of NETs causing a multitude of tissue damages. Thus, delayed NET degradation in systemic lupus erythematosus plays a crucial role in the lupus pathology (65, 66). NETs have been shown to initiate several detrimental effects directly on the host (45), especially when the NET degradation is impaired (67), as the case in COVID-19 is (68). One of the mechanisms responsible for delayed NET degradation is surplus of DNase I inhibitors (65), foremost G-actin, which forms a complex with DNase I, thereby inhibiting its nuclease activity (69). LPS signalling induces reorganisation of cell microfilaments and after LPS stimulation a subsequent rapid actin disassembly occurs (70). LPS increases the cellular G-actin pool without a reciprocal decrease in the F-actin pool. The G-actin increment could be explained, in part by F-actin depolymerisation, and in part, by de novo actin synthesis. This new actin synthesis could be a cell response to LPS challenge to maintain actin cytoskeletal integrity and barrier function. That LPS stimulates actin synthesis has been further substantiated by an increased total actin pool (71). In humans, G-actin levels were greatest in systemic inflammatory syndromes, but significantly elevated in septic shock as compared with healthy subjects (72). Similarly, delayed NET degradation in patients with periodontitis has been reported (73, 74). As periodontitis with late onset is paired with low-grade endotoxaemia (5), the connection between endotoxaemia and increased G-actin level respectively delayed NET degradation, suggests itself. Periodontitis treatment decreases endotoxemia and hence attenuates the delayed NET degradation (74).
Endotoxemia in COVID-19 Boosts NET Formation
LGE, i.e. increased plasma levels of LPS and LPS-binding protein (LBP), is associated with obesity (75, 76), diabetes (75–77), cardiovascular diseases (CVD) (78, 79), gut microbiome dysbiosis (80), and periodontitis (81–84). Although the mechanisms responsible for LGE in these diseases differ, they are all characterised by increased LPS blood serum levels. In periodontitis, LPS serum level is in the range of 0.89 ± 2.90 ng/ml (85). SARS-CoV-2 binds to bacteria or directly to free LPS, thereby enhancing their attachment to ACE2 receptors on the host cell surface. SARS-CoV-2 directly interacts with LPS through its S protein (86). Neither SARS-CoV-2 spike protein (S protein) nor LPS alone causes any activation of the pro-inflammatory nuclear factor kappa B (NF-κB), but the combination of S protein and low level of LPS activates NF-κB in a dose-dependent manner (86). The SARS-CoV-2/LPS interactions dramatically increase the viral infectivity and promote the development of hyper-cytokinaemia (75, 87). This mechanism may explain why above mentioned cases of LGE are associated with severe COVID-19 comorbidity (75, 88). Figure 1 demonstrates three main mechanisms responsible for the emergence of blood-born NETs in periodontitis with and without COVID-19 comorbidity.
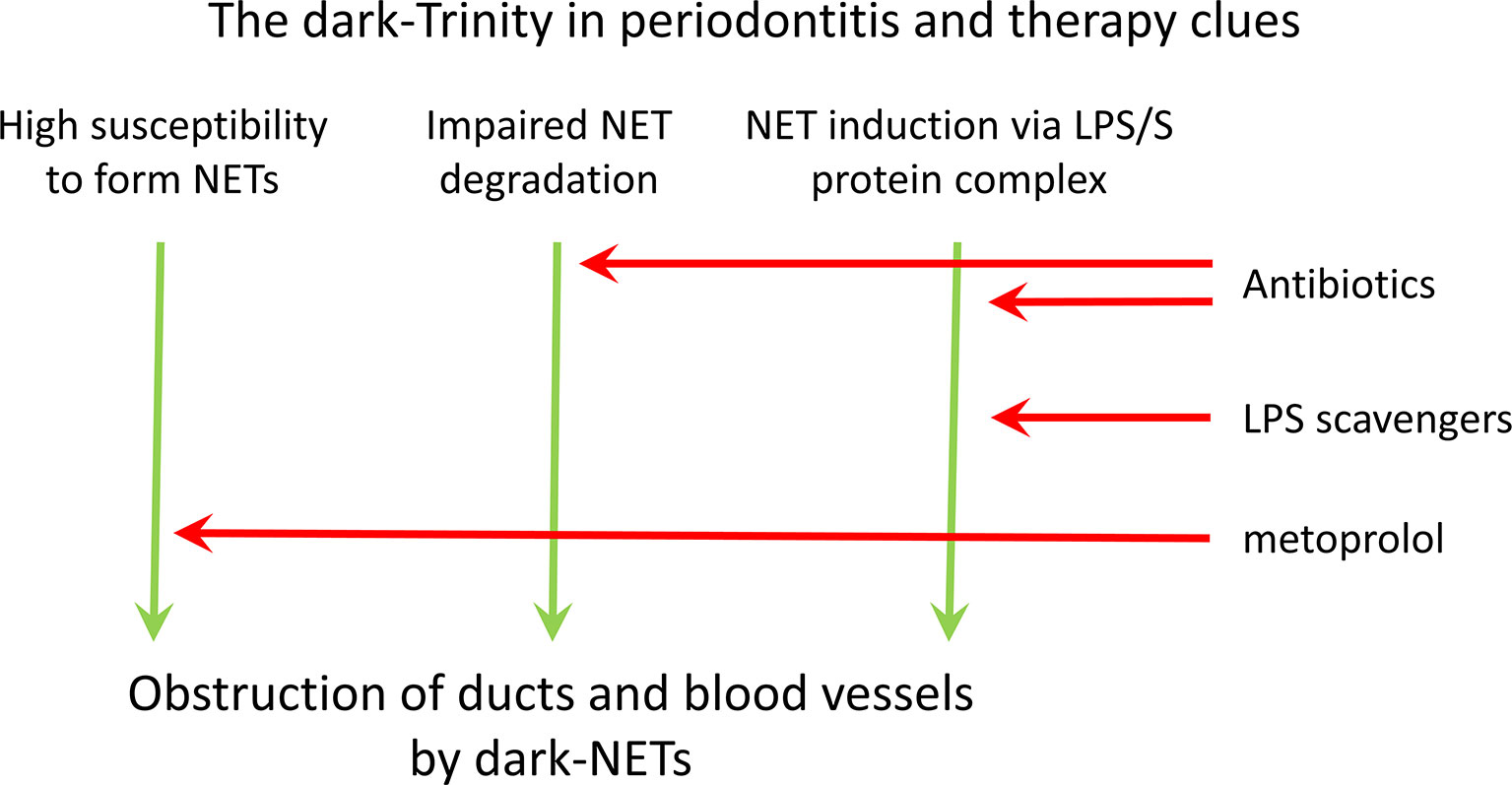
Figure 1 Pathogenic factors and treatment approaches in severe COVID-19 course in patients with periodontitis.
Pathogenic Effects of NETsin COVID-19
Most individuals infected with SARS-CoV-2 remain asymptomatic, luckily just a few severe cases transit into acute respiratory distress syndrome (ARDS), multi-organ failure, and even death (89). Individuals that develop severe manifestations of COVID-19 have signs of dysregulated innate and adaptive immune responses (45). Three main COVID-19 traits have to be considered: (I) dysregulated immune responses, (II) surplus of circulating NETs and (III) NET-mediated damage of distant organs via immunothrombosis (45). Nevertheless, many different mechanisms are responsible for excessive NET triggering in COVID-19:
SARS-CoV-2 activates complement regulators and complement (90), which augments the cytokine storm and coagulopathy, two dangerous complications in severe COVID-19 (90). Complement activation also triggers NETs in COVID-19 (19).
Sera from COVID-19 patients triggers NET release by healthy control neutrophils in vitro (91). Indeed, viable SARS-CoV-2 directly stimulate human neutrophils to release NETs in a dose-dependent manner. Neutrophil-expressed ACE2, TMPRSS2, and viral replication are generally required for this SARS-CoV-2-induced NET formation (92).
The conjunction of LPS and SARS-CoV-2 S protein activates toll-like receptor 4 (TLR4) in extremely low concentrations of LPS, e.g. of 1ng/ml, causing NET formation via the NF-κB activation (86), is a phenomenon playing a crucial role for the severe COVID-19 course in patients with LGE such as those with obesity, diabetes, CVD and periodontitis. In addition, LGE in patients with COVID-19 is associated with thrombotic events, possibly due to activation of platelet TLR4 (93). SARS-CoV-2- respectively SARS-CoV-2/LPS-activated platelets form aggregates with leukocytes, in particular in patients with severe disease (40, 94–98). Platelets adhere to injured blood vessels, become activated, and subsequently express adhesion molecules, such as P-selectin and ICAM-1, which induce neutrophil recruitment (45). Platelets are major instigators of direct neutrophil activation (99, 100). Circulating platelets bind neutrophils only in cases of bacterial (101) or viral infection (102) by means of integrins (103). Platelet activation may trigger the formation of intravascular NET aggregates in the pulmonary and renal microcirculation (40, 104) aggravating the course of COVID-19 (105).
Moderation of Circulating NETs in Periodontitis Patients With Covid-19 Comorbidity
Moderating the NET Formation
The main complication in severe COVID-19 appears to be the NET-driven ARDS and thrombosis of pulmonary vessels (40, 45). For that reason, anti-NET therapies should target either acceleration of NET degradation (68) or suppression of NET formation (106).
In general, attenuation of topical periodontal inflammatory events does not alter the systemic neutrophil responsiveness, as an enhanced neutrophil responsiveness persists even years after loss of all teeth. This is probably due to the trained immunity (5). The dysregulated trained immunity is responsible for surplus of circulating NETs in periodontitis (2) and relies on epigenetic alteration of HSPCs (3, 5). HSPCs chronically exposed to low level LPS, e.g. as a consequence of periodontitis, become epigenetically primed for a myeloid lineage bias with enhancers remaining more accessible than in naive HSPCs. Furthermore, LPS-exposed HSPCs keep increased accessibility of numerous genes that predispose to more rapid activation of myeloid lineage commitment in response to secondary stimulation than in naive HSPCs (107). This pre-programed chromatin accessibility for other transcription factors, facilitates the response of DNA regulatory elements to stimulation (108). The molecular basis of the epigenetic modifications includes changes in chromatin organization at the level of the topologically associated domains, transcription of long non-coding RNAs, methylation and acetylation of genes involved in the innate immune responses and reprogramming of cellular metabolism (60). Attenuating the neutrophil hyper-responsiveness due to dysregulated trained immunity (109) is an attractive option to ameliorate the course of NET-induced pathologies. Accordingly, many possibilities come into consideration. Two key epigenetic marks characterise trained immunity: (I) the acetylation of histone 3 lysine 27 (H3K27ac) at distal enhancers (marked by histone 3 lysine 4 methylation (H3K4me1) and (II) the consolidation of histone 3 lysine 4 trimethylation (H3K4me3) at the promoters of stimulated genes (60). Histone deacetylase (HDAC) inhibitors appear at first glance to be suitable candidates for this purpose, as they silence the inflammatory genes (110), which became accessible via acetylation of histone 3 lysine 27 (60). Indeed, HDAC inhibitors efficiently suppress NET formation (62, 63, 111, 112). However, due to severe side effects, they have not yet been used in humans except for cancer therapies.
PAD4 inhibitor GSK484, employed to suppress NET-induced gallstone blocks, completely overturned exaggerated NET formation and prevented gallstone formation in a murine model (31). This indicates the possibility to use PAD4 inhibitors to ameliorate NET-induced pathology. GSK484 is currently used in clinical studies.
The beta-1 blocker metoprolol reportedly stunned neutrophils (113) and suppressed exaggerated NET formation in mice (31). Of all tested beta-blockers, only metoprolol significantly attenuated exacerbated inflammation and reduced neutrophil infiltration and their interactions with other cell types (114). Recently, metoprolol has been employed for the treatment of ARDS in COVID-19. Its administration was safe and lacked serious side effects (106). Administration of beta-blockers is generally safe except for patients with acute pump failure. Metoprolol has also reduced NET levels and other markers of pulmonary inflammation as well as improved oxygenation (106). Metoprolol-treated patients spent fewer days on invasive mechanical ventilation. The use of metoprolol to treat COVID-19–associated ARDS appears to be a safe and inexpensive strategy that can alleviate the burden of the COVID-19 pandemic.
Attenuating the Delayed NET Degradation and Endotoxemia Decreasing
NET degradation in periodontitis is reduced (73, 74), as compared to healthy subjects. DNase I is inhibited by G-actin, which (69), which is LGE-induced (71). Delayed NET degradation in LGE contributes to severe COVID-19 comorbidity (88). In addition, the conjunction of low-level LPS and SARS-CoV-2 S protein results in excessive NET formation (86), a phenomenon playing a crucial role for the severe COVID-19 course in patients with periodontitis. Both LPS effects on the host cause NET increase, hence a reduction of LPS level is needed. The antibiotic treatment is a possible adjunctive option both to attenuate LGE effects and the delayed NET degradation in periodontitis with the severe COVID-19. Endotoxin adsorbent therapy in severe COVID-19 pneumonia has been applied, but despite some amelioration, the clinical benefit remains unclear (115). In contrast, antibiotic therapy is uncomplicated and has few or no side effects. Gut microbiota treatment with broad-spectrum antibiotics reduces metabolic endotoxaemia in patients with type 2 diabetes by reduction of LPS production in Gram-negative bacteria (116). Also antibiotic treatment in periodontitis cases effective reduction of blood serum LPS (117) In contrast, dental surgery and oral hygiene manipulations in patients with the severe COVID-19 have to be avoided, as they induce bacteraemia. Other possibilities to reduce LGE and to restore the impaired NET degradation are beyond the scope of periodontitis-related pathology and treatment. Figure 1 demonstrates the treatment approaches for attenuation of NET surplus in periodontitis with COVID-19 comorbidity.
Conclusions
Periodontitis with late onset is concomitant with side effects such as neutrophil hyper-responsiveness, propensity to NET formation, circulating NETs and cfDNA, low-grade endotoxaemia and delayed NET degradation. LPS effectuates delayed NET degradation via DNase I inhibition and binds the SARS-CoV-2 S protein causing NET production via NF-κB activation. These immunity alterations appear to contribute to the severe COVID-19 course in patients with periodontitis, as they cause surplus of NETs. In the viscera and in particular within blood vessels, the DNA scaffold of exaggerated NETs causes obstruction of whole organs and blood coagulation, resulting in heavy damages or even death. No canonical therapy for mastering the exaggerated NET formation has been established to date. The only candidate, promising to ameliorate neutrophil and NET hyper-responsiveness with petty side effects if any, is the beta-1 blocker metoprolol, but further investigations are needed. Adjunctive antibiotic treatment may reduce the periodontitis-relied LGE.
Author Contributions
Conceptualization and writing— preparation of the original draft, LV. Writing—review and editing: CS, JS, JKn, JKr, LV, BM, MHe, and MHa. All authors have read and agreed to the published version of the manuscript.
Funding
This research was supported by the German Research Foundation (DFG) Grants No. 2886 PANDORA B3; SCHA 2040/1-1; CRC1181(C03); TRR241(B04), by the EU H2020-FETOPEN-2018-2019-2020-01; 861878 ”NeutroCure”, and by the Volkswagen-Stiftung (Grant 97744).
Conflict of Interest
The authors declare that the research was conducted in the absence of any commercial or financial relationships that could be construed as a potential conflict of interest.
Publisher’s Note
All claims expressed in this article are solely those of the authors and do not necessarily represent those of their affiliated organizations, or those of the publisher, the editors and the reviewers. Any product that may be evaluated in this article, or claim that may be made by its manufacturer, is not guaranteed or endorsed by the publisher.
Acknowledgments
We acknowledge support by the Deutsche Forschungsgemeinschaft (DFG, German Research Foundation) and Saarland University within the funding program Open Access Publishing.
Abbreviations
ACE2, Angiotensin-converting enzyme 2; ARDS, acute respiratory distress syndrome; cfDNA, cell-free DNA; CVD, cardiovascular diseases; GCF, gingival crevicular fluid; HDAC, histone deacetylase; HSPCs, hematopoietic stem and progenitor cells; ICU, intensive care unit; IL, Interleukin; LBP, LPS-binding protein; LGE, low-grade endotoxemia; LPS, lipopolysaccharide; MMP, matrix metalloprotease; MPO, myeloperoxidase; NE, neutrophil elastase; NETs, neutrophil extracellular traps; NF-κB, nuclear factor kappa-light-chain-enhancer of activated B cells; NOX, NADPH oxidase; PAD4, peptidylarginine deiminases 4; PAMPs, pathogen associated molecular patterns; ROS, reactive oxygen species; SLGI, systemic low-grade inflammation; S protein, spike protein of SARS-CoV-2; TLR4, toll-like receptor 4; TMPRSS2, transmembrane serine protease 2; Treg, Regulatory T cells.
References
1. Silva LM, Brenchley L, Moutsopoulos NM. Primary Immunodeficiencies Reveal the Essential Role of Tissue Neutrophils in Periodontitis. Immunol Rev (2019) 287(1):226–35. doi: 10.1111/imr.12724
2. Vitkov L, Muñoz LE, Schoen J, Knopf J, Schauer C, Minnich B, et al. Neutrophils Orchestrate the Periodontal Pocket. Front Immunol (2021) 12:788766(5028). doi: 10.3389/fimmu.2021.788766
3. Hajishengallis G, Chavakis T. Local and Systemic Mechanisms Linking Periodontal Disease and Inflammatory Comorbidities. Nat Rev Immunol (2021) 21(7):426–40. doi: 10.1038/s41577-020-00488-6
4. Zheng D, Liwinski T, Elinav E. Interaction Between Microbiota and Immunity in Health and Disease. Cell Res (2020) 30(6):492–506. doi: 10.1038/s41422-020-0332-7
5. Vitkov L, Muñoz LE, Knopf J, Schauer C, Oberthaler H, Minnich B, et al. Connection Between Periodontitis-Induced Low-Grade Endotoxemia and Systemic Diseases: Neutrophils as Protagonists and Targets. Int J Mol Sci (2021) 22(9):4647. doi: 10.3390/ijms22094647
6. Fredriksson MI, Gustafsson AK, Bergström KG, Åsman BE. Constitutionally Hyperreactive Neutrophils in Periodontitis. J Periodontol (2003) 74(2):219–24. doi: 10.1902/jop.2003.74.2.219
7. Gustafsson A, Ito H, Asman B, Bergstrom K. Hyper-Reactive Mononuclear Cells and Neutrophils in Chronic Periodontitis. J Clin Periodontol (2006) 33(2):126–9. doi: 10.1111/j.1600-051X.2005.00883.x
8. Matthews JB, Wright HJ, Roberts A, Cooper PR, Chapple ILC. Hyperactivity and Reactivity of Peripheral Blood Neutrophils in Chronic Periodontitis: Neutrophil Hyperactivity and Reactivity in Chronic Periodontitis. Clin Exp Immunol (2006) 147(2):255–64. doi: 10.1111/j.1365-2249.2006.03276.x
9. Johnstone AM, Koh A, Goldberg MB, Glogauer M. A Hyperactive Neutrophil Phenotype in Patients With Refractory Periodontitis. J Periodontol (2007) 78(9):1788–94. doi: 10.1902/jop.2007.070107
10. Williams DW, Greenwell-Wild T, Brenchley L, Dutzan N, Overmiller A, Sawaya AP, et al. Human Oral Mucosa Cell Atlas Reveals a Stromal-Neutrophil Axis Regulating Tissue Immunity. Cell (2021) 184(15):4090-104.e15. doi: 10.1016/j.cell.2021.05.013
11. Chen GY, Nuñez G. Sterile Inflammation: Sensing and Reacting to Damage. Nat Rev Immunol (2010) 10(12):826–37. doi: 10.1038/nri2873
12. Kruger P, Saffarzadeh M, Weber ANR, Rieber N, Radsak M, von Bernuth H, et al. Neutrophils: Between Host Defence, Immune Modulation, and Tissue Injury. PloS Pathog (2015) 11(3):e1004651. doi: 10.1371/journal.ppat.1004651
13. Brinkmann V, Reichard U, Goosmann C, Fauler B, Uhlemann Y, Weiss DS, et al. Neutrophil Extracellular Traps Kill Bacteria. Sci (New York NY) (2004) 303(5663):1532–5. doi: 10.1126/science.1092385
14. Fuchs TA, Abed U, Goosmann C, Hurwitz R, Schulze I, Wahn V, et al. Novel Cell Death Program Leads to Neutrophil Extracellular Traps. J Cell Biol (2007) 176(2):231–41. doi: 10.1083/jcb.200606027
15. Boeltz S, Amini P, Anders H-J, Andrade F, Bilyy R, Chatfield S, et al. To NET or Not to NET:current Opinions and State of the Science Regarding the Formation of Neutrophil Extracellular Traps. Cell Death Differ (2019) 26(3):395–408. doi: 10.1038/s41418-018-0261-x
16. Leppkes M, Schick M, Hohberger B, Mahajan A, Knopf J, Schett G, et al. Updates on NET Formation in Health and Disease. Semin Arthritis Rheum (2019) 49(3s):S43–s8. doi: 10.1016/j.semarthrit.2019.09.011
17. Schultz BM, Acevedo OA, Kalergis AM, Bueno SM. Role of Extracellular Trap Release During Bacterial and Viral Infection. Front Microbiol (2022) 13:798853. doi: 10.3389/fmicb.2022.798853
18. Urban CF, Ermert D, Schmid M, Abu-Abed U, Goosmann C, Nacken W, et al. Neutrophil Extracellular Traps Contain Calprotectin, a Cytosolic Protein Complex Involved in Host Defense Against Candida Albicans. PloS Pathog (2009) 5(10):e1000639. doi: 10.1371/journal.ppat.1000639
19. Skendros P, Mitsios A, Chrysanthopoulou A, Mastellos DC, Metallidis S, Rafailidis P, et al. Complement and Tissue Factor-Enriched Neutrophil Extracellular Traps are Key Drivers in COVID-19 Immunothrombosis. J Clin Invest (2020) 130(11):6151–7. doi: 10.1172/jci141374
20. Chen T, Li Y, Sun R, Hu H, Liu Y, Herrmann M, et al. Receptor-Mediated NETosis on Neutrophils. Front Immunol (2021) 12:775267. doi: 10.3389/fimmu.2021.775267
21. Desai J, Kumar SV, Mulay SR, Konrad L, Romoli S, Schauer C, et al. PMA and Crystal-Induced Neutrophil Extracellular Trap Formation Involves RIPK1-RIPK3-MLKL Signaling. Eur J Immunol (2016) 46(1):223–9. doi: 10.1002/eji.201545605
22. Maueröder C, Mahajan A, Paulus S, Gößwein S, Hahn J, Kienhöfer D, et al. Ménage-À-Trois: The Ratio of Bicarbonate to CO2 and the pH Regulate the Capacity of Neutrophils to Form NETs. Front Immunol (2016) 7:583. doi: 10.3389/fimmu.2016.00583
23. Chen KW, Monteleone M, Boucher D, Sollberger G, Ramnath D, Condon ND, et al. Noncanonical Inflammasome Signaling Elicits Gasdermin D–dependent Neutrophil Extracellular Traps. Sci Immunol (2018) 3(26):eaar6676. doi: 10.1126/sciimmunol.aar6676
24. Shi J, Zhao Y, Wang Y, Gao W, Ding J, Li P, et al. Inflammatory Caspases are Innate Immune Receptors for Intracellular LPS. Nature (2014) 514(7521):187–92. doi: 10.1038/nature13683
25. Douda DN, Khan MA, Grasemann H, Palaniyar N. SK3 Channel and Mitochondrial ROS Mediate NADPH Oxidase-Independent NETosis Induced by Calcium Influx. Proc Natl Acad Sci (2015) 112(9):2817–22. doi: 10.1073/pnas.1414055112
26. Papayannopoulos V, Metzler KD, Hakkim A, Zychlinsky A. Neutrophil Elastase and Myeloperoxidase Regulate the Formation of Neutrophil Extracellular Traps. J Cell Biol (2010) 191(3):677–91. doi: 10.1083/jcb.201006052
27. Li P, Li M, Lindberg MR, Kennett MJ, Xiong N, Wang Y. PAD4 is Essential for Antibacterial Innate Immunity Mediated by Neutrophil Extracellular Traps. J Exp Med (2010) 207(9):1853–62. doi: 10.1084/jem.20100239
28. Gößwein S, Lindemann A, Mahajan A, Maueröder C, Martini E, Patankar J, et al. Citrullination Licenses Calpain to Decondense Nuclei in Neutrophil Extracellular Trap Formation. Front Immunol (2019) 10:2481. doi: 10.3389/fimmu.2019.02481
29. Kahlenberg JM, Carmona-Rivera C, Smith CK, Kaplan MJ. Neutrophil Extracellular Trap-Associated Protein Activation of the NLRP3 Inflammasome Is Enhanced in Lupus Macrophages. J Immunol (2013) 190(3):1217–26. doi: 10.4049/jimmunol.1202388
30. Lood C, Blanco LP, Purmalek MM, Carmona-Rivera C, De Ravin SS, Smith CK, et al. Neutrophil Extracellular Traps Enriched in Oxidized Mitochondrial DNA are Interferogenic and Contribute to Lupus-Like Disease. Nat Med (2016) 22(2):146–53. doi: 10.1038/nm.4027
31. Muñoz LE, Boeltz S, Bilyy R, Schauer C, Mahajan A, Widulin N, et al. Neutrophil Extracellular Traps Initiate Gallstone Formation. Immunity (2019) 51(3):443–50.e4. doi: 10.1016/j.immuni.2019.07.002
32. Leppkes M, Maueröder C, Hirth S, Nowecki S, Günther C, Billmeier U, et al. Externalized Decondensed Neutrophil Chromatin Occludes Pancreatic Ducts and Drives Pancreatitis. Nat Commun (2016) 7:10973. doi: 10.1038/ncomms10973
33. Schapher M, Koch M, Weidner D, Scholz M, Wirtz S, Mahajan A, et al. Neutrophil Extracellular Traps Promote the Development and Growth of Human Salivary Stones. Cells (2020) 9(9):2139. doi: 10.3390/cells9092139
34. Vitkov L, Minnich B, Knopf J, Schauer C, Hannig M, Herrmann M. NETs Are Double-Edged Swords With the Potential to Aggravate or Resolve Periodontal Inflammation. Cells (2020) 9(12):2614. doi: 10.3390/cells9122614
35. Jiménez-Alcázar M, Rangaswamy C, Panda R, Bitterling J, Simsek YJ, Long AT, et al. Host DNases Prevent Vascular Occlusion by Neutrophil Extracellular Traps. Science (2017) 358(6367):1202–6. doi: 10.1126/science.aam8897
36. Boeltz S, Hagen M, Knopf J, Mahajan A, Schick M, Zhao Y, et al. Towards a Pro-Resolving Concept in Systemic Lupus Erythematosus. Semin Immunopathol (2019) 41(6):681–97. doi: 10.1007/s00281-019-00760-5
37. Appelgren D, Dahle C, Knopf J, Bilyy R, Vovk V, Sundgren PC, et al. Active NET Formation in Libman-Sacks Endocarditis Without Antiphospholipid Antibodies: A Dramatic Onset of Systemic Lupus Erythematosus. Autoimmunity (2018) 51(6):310–8. doi: 10.1080/08916934.2018.1514496
38. Magán-Fernández A, Rasheed Al-Bakri SM, O’Valle F, Benavides-Reyes C, Abadía-Molina F, Mesa F. Neutrophil Extracellular Traps in Periodontitis. Cells (2020) 9(6):1494. doi: 10.3390/cells9061494
39. Castanheira FVS, Kubes P. Neutrophils and NETs in Modulating Acute and Chronic Inflammation. Blood (2019) 133(20):2178–85. doi: 10.1182/blood-2018-11-844530
40. Leppkes M, Knopf J, Naschberger E, Lindemann A, Singh J, Herrmann I, et al. Vascular Occlusion by Neutrophil Extracellular Traps in COVID-19. EBioMedicine (2020) 58:102925. doi: 10.1016/j.ebiom.2020.102925
41. Larvin H, Wilmott S, Wu J, Kang J. The Impact of Periodontal Disease on Hospital Admission and Mortality During COVID-19 Pandemic. Front Med (Lausanne) (2020) 7:604980. doi: 10.3389/fmed.2020.604980
42. Marouf N, Cai W, Said KN, Daas H, Diab H, Chinta VR, et al. Association Between Periodontitis and Severity of COVID-19 Infection: A Case–Control Study. J Clin Periodontol (2021): 48(4):483–91. doi: 10.1111/jcpe.13435
43. Aquino-Martinez R, Hernández-Vigueras S. Severe COVID-19 Lung Infection in Older People and Periodontitis. J Clin Med (2021) 10(2):279. doi: 10.3390/jcm10020279
44. Rodrigues P, Alrubayyi A, Pring E, Bart V, Jones R, Coveney C, et al. Innate Immunology in COVID-19—a Living Review. Part II: Dysregulated Inflammation Drives Immunopathology. Ox Open Immunol (2020) 1(1):iqaa005. doi: 10.1093/oxfimm/iqaa005
45. Ackermann M, Anders HJ, Bilyy R, Bowlin GL, Daniel C, De Lorenzo R, et al. Patients With COVID-19: In the Dark-NETs of Neutrophils. Cell Death Differ (2021) (11):3125–39. doi: 10.1038/s41418-021-00805-z
46. Gillot C, Favresse J, Mullier F, Lecompte T, Dogné JM, Douxfils J. NETosis and the Immune System in COVID-19: Mechanisms and Potential Treatments. Front Pharmacol (2021) 12:708302. doi: 10.3389/fphar.2021.708302
47. Grabuschnig S, Bronkhorst AJ, Holdenrieder S, Rosales Rodriguez I, Schliep KP, Schwendenwein D, et al. Putative Origins of Cell-Free DNA in Humans: A Review of Active and Passive Nucleic Acid Release Mechanisms. Int J Mol Sci (2020) 21(21):8062. doi: 10.3390/ijms21218062
48. Andargie TE, Tsuji N, Seifuddin F, Jang MK, Yuen PS, Kong H, et al. Cell-Free DNA Maps COVID-19 Tissue Injury and Risk of Death and can Cause Tissue Injury. JCI Insight (2021) 6(7):e147610. doi: 10.1172/jci.insight.147610
49. Chen X, Wu T, Li L, Lin Y, Ma Z, Xu J, et al. Transcriptional Start Site Coverage Analysis in Plasma Cell-Free DNA Reveals Disease Severity and Tissue Specificity of COVID-19 Patients. Front Genet (2021) 12:663098. doi: 10.3389/fgene.2021.663098
50. Vitkov L, Klappacher M, Hannig M, Krautgartner WD. Extracellular Neutrophil Traps in Periodontitis. J Periodontal Res (2009) 44(5):664–72. doi: 10.1111/j.1600-0765.2008.01175.x
51. Vitkov L, Klappacher M, Hannig M, Krautgartner WD. Neutrophil Fate in Gingival Crevicular Fluid. Ultrastruct Pathol (2010) 34(1):25–30. doi: 10.3109/01913120903419989
52. Magán-Fernández A, O’Valle F, Abadía-Molina F, Muñoz R, Puga-Guil P, Mesa F. Characterization and Comparison of Neutrophil Extracellular Traps in Gingival Samples of Periodontitis and Gingivitis: A Pilot Study. J Periodontal Res (2019) 54(3):218–24. doi: 10.1111/jre.12621
53. Masso-Silva JA, Moshensky A, Lam MTY, Odish MF, Patel A, Xu L, et al. Increased Peripheral Blood Neutrophil Activation Phenotypes and Neutrophil Extracellular Trap Formation in Critically Ill Coronavirus Disease 2019 (COVID-19) Patients: A Case Series and Review of the Literature. Clin Infect Dis (2021) 74(3):479–89. doi: 10.1093/cid/ciab437
54. O’Neil LJ, Barrera-Vargas A, Sandoval-Heglund D, Merayo-Chalico J, Aguirre-Aguilar E, Aponte AM, et al. Neutrophil-Mediated Carbamylation Promotes Articular Damage in Rheumatoid Arthritis. Sci Adv (2020) 6(44):eabd2688. doi: 10.1126/sciadv.abd2688
55. Clarke J. NETs Revealed as Source of Carbamylated Proteins in RA. Nat Rev Rheumatol (2021) 17(1):4. doi: 10.1038/s41584-020-00548-0
56. Kaneko C, Kobayashi T, Ito S, Sugita N, Murasawa A, Nakazono K, et al. Circulating Levels of Carbamylated Protein and Neutrophil Extracellular Traps are Associated With Periodontitis Severity in Patients With Rheumatoid Arthritis: A Pilot Case-Control Study. PloS One (2018) 13(2):e0192365. doi: 10.1371/journal.pone.0192365
57. Oliveira SR, de Arruda JAA, Schneider AH, Carvalho VF, Machado C, Moura MF, et al. Are Neutrophil Extracellular Traps the Link for the Cross-Talk Between Periodontitis and Rheumatoid Arthritis Physiopathology? Rheumatol (Oxford) (2021) 61(1):174–84. doi: 10.1093/rheumatology/keab289
58. Smale ST, Tarakhovsky A, Natoli G. Chromatin Contributions to the Regulation of Innate Immunity. Annu Rev Immunol (2014) 32(1):489–511. doi: 10.1146/annurev-immunol-031210-101303
59. Ghisletti S, Barozzi I, Mietton F, Polletti S, De Santa F, Venturini E, et al. Identification and Characterization of Enhancers Controlling the Inflammatory Gene Expression Program in Macrophages. Immunity (2010) 32(3):317–28. doi: 10.1016/j.immuni.2010.02.008
60. Netea MG, Domínguez-Andrés J, Barreiro LB, Chavakis T, Divangahi M, Fuchs E, et al. Defining Trained Immunity and its Role in Health and Disease. Nat Rev Immunol (2020) 20(6):375–88. doi: 10.1038/s41577-020-0285-6
61. de Laval B, Maurizio J, Kandalla PK, Brisou G, Simonnet L, Huber C, et al. C/EBPbeta-Dependent Epigenetic Memory Induces Trained Immunity in Hematopoietic Stem Cells. Cell Stem Cell (2020) 26(5):657–74 e8. doi: 10.1016/j.stem.2020.01.017
62. Hamam H, Palaniyar N. Post-Translational Modifications in NETosis and NETs-Mediated Diseases. Biomolecules (2019) 9(8):369. doi: 10.3390/biom9080369
63. Hamam H, Khan M, Palaniyar N. Histone Acetylation Promotes Neutrophil Extracellular Trap Formation. Biomolecules (2019) 9(1):32. doi: 10.3390/biom9010032
64. Noz M, Plachokova A, Smeets E, Aarntzen E, Bekkering S, Vart P, et al. An Explorative Study on Monocyte Reprogramming in the Context of Periodontitis In Vitro and In Vivo. Front Immunol (2021) 12:695227. doi: 10.3389/fimmu.2021.695227
65. Hakkim A, Fürnrohr BG, Amann K, Laube B, Abed UA, Brinkmann V, et al. Impairment of Neutrophil Extracellular Trap Degradation is Associated With Lupus Nephritis. Proc Natl Acad Sci U.S.A. (2010) 107(21):9813–8. doi: 10.1073/pnas.0909927107
66. Leffler J, Gullstrand B, Jönsen A, Nilsson J, Martin M, Blom AM, et al. Degradation of Neutrophil Extracellular Traps Co-Varies With Disease Activity in Patients With Systemic Lupus Erythematosus. Arthritis Res Ther (2013) 15(4):R84. doi: 10.1186/ar4264
67. Mayadas TN, Cullere X, Lowell CA. The Multifaceted Functions of Neutrophils. Annu Rev Pathol: Mech Dis (2014) 9(1):181–218. doi: 10.1146/annurev-pathol-020712-164023
68. Englert H, Rangaswamy C, Deppermann C, Sperhake J-P, Krisp C, Schreier D, et al. Defective NET Clearance Contributes to Sustained FXII Activation in COVID-19-Associated Pulmonary Thrombo-Inflammation. EBioMedicine (2021) 67:103382. doi: 10.1016/j.ebiom.2021.103382
69. Kabsch W, Mannherz HG, Suck D, Pai EF, Holmes KC. Atomic Structure of the Actin:DNase I Complex. Nature (1990) 347(6288):37–44. doi: 10.1038/347037a0
70. Shinji H, Kaiho S, Nakano T, Yoshida T. Reorganization of Microfilaments in Macrophages After LPS Stimulation. Exp Cell Res (1991) 193(1):127–33. doi: 10.1016/0014-4827(91)90546-7
71. Goldblum SE, Ding X, Brann TW, Campbell-Washington J. Bacterial Lipopolysaccharide Induces Actin Reorganization, Intercellular Gap Formation, and Endothelial Barrier Dysfunction in Pulmonary Vascular Endothelial Cells: Concurrent F-Actin Depolymerization and New Actin Synthesis. J Cell Physiol (1993) 157(1):13–23. doi: 10.1002/jcp.1041570103
72. Belsky JB, Morris DC, Bouchebl R, Filbin MR, Bobbitt KR, Jaehne AK, et al. Plasma Levels of F-Actin and F:G-Actin Ratio as Potential New Biomarkers in Patients With Septic Shock. Biomarkers (2016) 21(2):180–5. doi: 10.3109/1354750x.2015.1126646
73. White P, Sakellari D, Roberts H, Risafi I, Ling M, Cooper P, et al. Peripheral Blood Neutrophil Extracellular Trap Production and Degradation in Chronic Periodontitis. J Clin Periodontol (2016) 43(12):1041–9. doi: 10.1111/jcpe.12628
74. Moonen CG, Buurma KG, Faruque MR, Balta MG, Liefferink E, Bizzarro S, et al. Periodontal Therapy Increases Neutrophil Extracellular Trap Degradation. Innate Immun (2020) 26(5):331–40. doi: 10.1177/1753425919889392
75. Kruglikov IL, Shah M, Scherer PE. Obesity and Diabetes as Comorbidities for COVID-19: Underlying Mechanisms and the Role of Viral-Bacterial Interactions. Elife (2020) 9:e61330. doi: 10.7554/eLife.61330
76. Hulme KD, Noye EC, Short KR, Labzin LI. Dysregulated Inflammation During Obesity: Driving Disease Severity in Influenza Virus and SARS-CoV-2 Infections. Front Immunol (2021) 12:770066. doi: 10.3389/fimmu.2021.770066
77. Fang L, Karakiulakis G, Roth M. Are Patients With Hypertension and Diabetes Mellitus at Increased Risk for COVID-19 Infection? Lancet Respir Med (2020) 8(4):e21. doi: 10.1016/S2213-2600(20)30116-8
78. Ciornei RT. Prevention of Severe Coronavirus Disease 2019 Outcomes by Reducing Low-Grade Inflammation in High-Risk Categories. Front Immunol (2020) 11:1762. doi: 10.3389/fimmu.2020.01762
79. Korakas E, Ikonomidis I, Kousathana F, Balampanis K, Kountouri A, Raptis A, et al. Obesity and COVID-19: Immune and Metabolic Derangement as a Possible Link to Adverse Clinical Outcomes. Am J Physiol Endocrinol Metab (2020) 319(1):E105–e9. doi: 10.1152/ajpendo.00198.2020
80. Belančić A. Gut Microbiome Dysbiosis and Endotoxemia - Additional Pathophysiological Explanation for Increased COVID-19 Severity in Obesity. Obes Med (2020) 20:100302. doi: 10.1016/j.obmed.2020.100302
81. Geerts SO, Nys M, Mol PD, Charpentier J, Albert A, Legrand V, et al. Systemic Release of Endotoxins Induced by Gentle Mastication: Association With Periodontitis Severity. J Periodontol (2002) 73(1):73–8. doi: 10.1902/jop.2002.73.1.73
82. Lockhart PB, Brennan MT, Sasser HC, Fox PC, Paster BJ, Bahrani-Mougeot FK. Bacteremia Associated With Toothbrushing and Dental Extraction. Circulation (2008) 117(24):3118–25. doi: 10.1161/CIRCULATIONAHA.107.758524
83. Crasta K, Daly CG, Mitchell D, Curtis B, Stewart D, Heitz-Mayfield LJA. Bacteraemia Due to Dental Flossing. J Clin Periodontol (2009) 36(4):323–32. doi: 10.1111/j.1600-051X.2008.01372.x
84. Tomás I, Diz P, Tobías A, Scully C, Donos N. Periodontal Health Status and Bacteraemia From Daily Oral Activities: Systematic Review/Meta-Analysis. J Clin Periodontol (2012) 39(3):213–28. doi: 10.1111/j.1600-051X.2011.01784.x
85. Paju S, Pussinen PJ, Sinisalo J, Mattila K, Doğan B, Ahlberg J, et al. Clarithromycin Reduces Recurrent Cardiovascular Events in Subjects Without Periodontitis. Atherosclerosis (2006) 188(2):412–9. doi: 10.1016/j.atherosclerosis.2005.11.019
86. Petruk G, Puthia M, Petrlova J, Samsudin F, Strömdahl AC, Cerps S, et al. SARS-CoV-2 Spike Protein Binds to Bacterial Lipopolysaccharide and Boosts Proinflammatory Activity. J Mol Cell Biol (2020) 12(12):916–32. doi: 10.1093/jmcb/mjaa067
87. Neu U, Mainou BA. Virus Interactions With Bacteria: Partners in the Infectious Dance. PloS Pathog (2020) 16(2):e1008234. doi: 10.1371/journal.ppat.1008234
88. Kruglikov IL, Scherer PE. Preexisting and Inducible Endotoxemia as Crucial Contributors to the Severity of COVID-19 Outcomes. PloS Pathog (2021) 17(2):e1009306. doi: 10.1371/journal.ppat.1009306
89. Zhou F, Yu T, Du R, Fan G, Liu Y, Liu Z, et al. Clinical Course and Risk Factors for Mortality of Adult Inpatients With COVID-19 in Wuhan, China: A Retrospective Cohort Study. Lancet (2020) 395(10229):1054–62. doi: 10.1016/s0140-6736(20)30566-3
90. Lo MW, Kemper C, Woodruff TM. COVID-19: Complement, Coagulation, and Collateral Damage. J Immunol (2020) 205(6):1488–95. doi: 10.4049/jimmunol.2000644
91. Zuo Y, Yalavarthi S, Shi H, Gockman K, Zuo M, Madison JA, et al. Neutrophil Extracellular Traps in COVID-19. JCI Insight (2020). doi: 10.1172/jci.insight.138999
92. Veras FP, Pontelli MC, Silva CM, Toller-Kawahisa JE, de Lima M, Nascimento DC, et al. SARS-CoV-2–Triggered Neutrophil Extracellular Traps Mediate COVID-19 Pathology. J Exp Med (2020) 217(12):e20201129. doi: 10.1084/jem.20201129
93. Oliva A, Cammisotto V, Cangemi R, Ferro D, Miele MC, De Angelis M, et al. Low-Grade Endotoxemia and Thrombosis in COVID-19. Clin Transl Gastroenterol (2021) 12(6):e00348-e. doi: 10.14309/ctg.0000000000000348
94. Manne BK, Denorme F, Middleton EA, Portier I, Rowley JW, Stubben C, et al. Platelet Gene Expression and Function in Patients With COVID-19. Blood (2020) 136(11):1317–29. doi: 10.1182/blood.2020007214
95. Hottz ED, Azevedo-Quintanilha IG, Palhinha L, Teixeira L, Barreto EA, Pão CRR, et al. Platelet Activation and Platelet-Monocyte Aggregate Formation Trigger Tissue Factor Expression in Patients With Severe COVID-19. Blood (2020) 136(11):1330–41. doi: 10.1182/blood.2020007252
96. Nikolai LA, Meyer CG, Kremsner PG, Velavan TP. Asymptomatic SARS Coronavirus 2 Infection: Invisible Yet Invincible. Int J Infect Dis (2020) 100:112–6. doi: 10.1016/j.ijid.2020.08.076
97. Taus F, Salvagno G, Canè S, Fava C, Mazzaferri F, Carrara E, et al. Platelets Promote Thromboinflammation in SARS-CoV-2 Pneumonia. Arterioscler Thromb Vasc Biol (2020) 40(12):2975–89. doi: 10.1161/atvbaha.120.315175
98. Zaid Y, Puhm F, Allaeys I, Naya A, Oudghiri M, Khalki L, et al. Platelets Can Associate With SARS-Cov-2 RNA and Are Hyperactivated in COVID-19. Circ Res (2020) 127(11):1404–18. doi: 10.1161/circresaha.120.317703
99. Sreeramkumar V, Adrover JM, Ballesteros I, Cuartero MI, Rossaint J, Bilbao I, et al. Neutrophils Scan for Activated Platelets to Initiate Inflammation. Science (2014) 346(6214):1234–8. doi: 10.1126/science.1256478
100. Martinod K, Deppermann C. Immunothrombosis and Thromboinflammation in Host Defense and Disease. Platelets (2021) 32(3):314–24. doi: 10.1080/09537104.2020.1817360
101. Clark SR, Ma AC, Tavener SA, McDonald B, Goodarzi Z, Kelly MM, et al. Platelet TLR4 Activates Neutrophil Extracellular Traps to Ensnare Bacteria in Septic Blood. Nat Med (2007) 13(4):463–9. doi: 10.1038/nm1565
102. Jenne Craig N, Wong Connie HY, Zemp Franz J, McDonald B, Rahman Masmudur M, Forsyth Peter A, et al. Neutrophils Recruited to Sites of Infection Protect From Virus Challenge by Releasing Neutrophil Extracellular Traps. Cell Host Microbe (2013) 13(2):169–80. doi: 10.1016/j.chom.2013.01.005
103. McDonald B, Urrutia R, Yipp BG, Jenne CN, Kubes P. Intravascular Neutrophil Extracellular Traps Capture Bacteria From the Bloodstream During Sepsis. Cell Host Microbe (2012) 12(3):324–33. doi: 10.1016/j.chom.2012.06.011
104. Nicolai L, Leunig A, Brambs S, Kaiser R, Weinberger T, Weigand M, et al. Immunothrombotic Dysregulation in COVID-19 Pneumonia Is Associated With Respiratory Failure and Coagulopathy. Circulation (2020) 142(12):1176–89. doi: 10.1161/circulationaha.120.048488
105. Lou M, Yuan D, Liao S, Tong L, Li J. Potential Mechanisms of Cerebrovascular Diseases in COVID-19 Patients. J Neurovirol (2021) 27(1):35–51. doi: 10.1007/s13365-021-00948-2
106. Clemente-Moragón A, Martínez-Milla J, Oliver E, Santos A, Flandes J, Fernández I, et al. Metoprolol in Critically Ill Patients With COVID-19. J Am Coll Cardiol (2021) 78(10):1001–11. doi: 10.1016/j.jacc.2021.07.003
107. de Laval B, Maurizio J, Kandalla PK, Brisou G, Simonnet L, Huber C, et al. C/Ebpβ-Dependent Epigenetic Memory Induces Trained Immunity in Hematopoietic Stem Cells. Cell Stem Cell (2020) 26(5):657–74.e8. doi: 10.1016/j.stem.2020.01.017
108. Grøntved L, John S, Baek S, Liu Y, Buckley JR, Vinson C, et al. C/EBP Maintains Chromatin Accessibility in Liver and Facilitates Glucocorticoid Receptor Recruitment to Steroid Response Elements. EMBO J (2013) 32(11):1568–83. doi: 10.1038/emboj.2013.106
109. Netea MG, Giamarellos-Bourboulis EJ, Domínguez-Andrés J, Curtis N, van Crevel R, van de Veerdonk FL, et al. Trained Immunity: A Tool for Reducing Susceptibility to and the Severity of SARS-CoV-2 Infection. Cell (2020) 181(5):969–77. doi: 10.1016/j.cell.2020.04.042
110. Blanchard F, Chipoy C. Histone Deacetylase Inhibitors: New Drugs for the Treatment of Inflammatory Diseases? Drug Discovery Today (2005) 10(3):197–204. doi: 10.1016/s1359-6446(04)03309-4
111. Chen Z, Liu C, Jiang Y, Liu H, Shao L, Zhang K, et al. HDAC Inhibitor Attenuated NETs Formation Induced by Activated Platelets In Vitro, Partially Through Downregulating Platelet Secretion. Shock (2020) 54(3):321–9. doi: 10.1097/shk.0000000000001518
112. Poli V, Ma V, Di Gioia M, Broggi A, Benamar M, Chen Q, et al. Zinc-Dependent Histone Deacetylases Drive Neutrophil Extracellular Trap Formation and Potentiate Local and Systemic Inflammation. iScience (2021) 24:103256. doi: 10.1016/j.isci.2021.103256
113. García-Prieto J, Villena-Gutiérrez R, Gómez M, Bernardo E, Pun-García A, García-Lunar I, et al. Neutrophil Stunning by Metoprolol Reduces Infarct Size. Nat Commun (2017) 8:14780. doi: 10.1038/ncomms14780
114. Clemente-Moragón A, Gómez M, Villena-Gutiérrez R, Lalama DV, García-Prieto J, Martínez F, et al. Metoprolol Exerts a non-Class Effect Against Ischaemia-Reperfusion Injury by Abrogating Exacerbated Inflammation. Eur Heart J (2020) 41(46):4425–40. doi: 10.1093/eurheartj/ehaa733
115. Peerapornratana S, Sirivongrangson P, Tungsanga S, Tiankanon K, Kulvichit W, Putcharoen O, et al. Endotoxin Adsorbent Therapy in Severe COVID-19 Pneumonia. Blood Purif (2021), 51(1):47–54. doi: 10.1159/000515628
116. Chou CJ, Membrez M, Blancher F. Gut Decontamination With Norfloxacin and Ampicillin Enhances Insulin Sensitivity in Mice. Nestle Nutr Workshop Ser Pediatr Program (2008) 62:127–37. doi: 10.1159/000146256
Keywords: neutrophil hyper-responsiveness, dysregulated immunity, trained immunity, NET hyper-responsiveness, NET-induced damage, inhibition of NET formation
Citation: Vitkov L, Knopf J, Krunić J, Schauer C, Schoen J, Minnich B, Hannig M and Herrmann M (2022) Periodontitis-Derived Dark-NETs in Severe Covid-19. Front. Immunol. 13:872695. doi: 10.3389/fimmu.2022.872695
Received: 09 February 2022; Accepted: 23 March 2022;
Published: 12 April 2022.
Edited by:
Kim Maree O’Sullivan, Monash University, AustraliaReviewed by:
Francisco Mesa, University of Granada, SpainCopyright © 2022 Vitkov, Knopf, Krunić, Schauer, Schoen, Minnich, Hannig and Herrmann. This is an open-access article distributed under the terms of the Creative Commons Attribution License (CC BY). The use, distribution or reproduction in other forums is permitted, provided the original author(s) and the copyright owner(s) are credited and that the original publication in this journal is cited, in accordance with accepted academic practice. No use, distribution or reproduction is permitted which does not comply with these terms.
*Correspondence: Matthias Hannig, bWF0dGhpYXMuaGFubmlnQHVrcy5ldQ==
†These authors have contributed equally to this work and share senior authorship