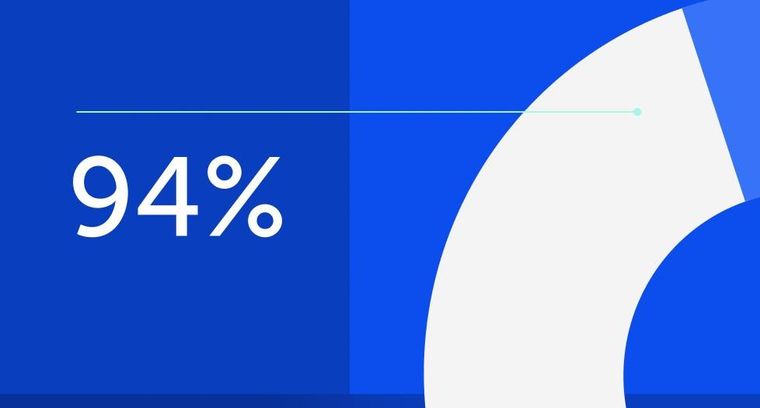
94% of researchers rate our articles as excellent or good
Learn more about the work of our research integrity team to safeguard the quality of each article we publish.
Find out more
REVIEW article
Front. Immunol., 26 April 2022
Sec. Autoimmune and Autoinflammatory Disorders
Volume 13 - 2022 | https://doi.org/10.3389/fimmu.2022.869422
This article is part of the Research TopicRegulation of Osteoclast Differentiation in Autoimmune and Inflammatory DiseasesView all 7 articles
In response to mechanical forces and the aging process, bone in the adult skeleton is continuously remodeled by a process in which old and damaged bone is removed by bone-resorbing osteoclasts and subsequently is replaced by new bone by bone-forming cells, osteoblasts. During this essential process of bone remodeling, osteoclastic resorption is tightly coupled to osteoblastic bone formation. Bone-resorbing cells, multinuclear giant osteoclasts, derive from the monocyte/macrophage hematopoietic lineage and their differentiation is driven by distinct signaling molecules and transcription factors. Critical factors for this process are Macrophage Colony Stimulating Factor (M-CSF) and Receptor Activator Nuclear Factor-κB Ligand (RANKL). Besides their resorption activity, osteoclasts secrete coupling factors which promote recruitment of osteoblast precursors to the bone surface, regulating thus the whole process of bone remodeling. Bone morphogenetic proteins (BMPs), a family of multi-functional growth factors involved in numerous molecular and signaling pathways, have significant role in osteoblast-osteoclast communication and significantly impact bone remodeling. It is well known that BMPs help to maintain healthy bone by stimulating osteoblast mineralization, differentiation and survival. Recently, increasing evidence indicates that BMPs not only help in the anabolic part of bone remodeling process but also significantly influence bone catabolism. The deletion of the BMP receptor type 1A (BMPRIA) in osteoclasts increased osteoblastic bone formation, suggesting that BMPR1A signaling in osteoclasts regulates coupling to osteoblasts by reducing bone-formation activity during bone remodeling. The dual effect of BMPs on bone mineralization and resorption highlights the essential role of BMP signaling in bone homeostasis and they also appear to be involved in pathological processes in inflammatory disorders affecting bones and joints. Certain BMPs (BMP2 and -7) were approved for clinical use; however, increased bone resorption rather than formation were observed in clinical applications, suggesting the role BMPs have in osteoclast activation and subsequent osteolysis. Here, we summarize the current knowledge of BMP signaling in osteoclasts, its role in osteoclast resorption, bone remodeling, and osteoblast–osteoclast coupling. Furthermore, discussion of clinical application of recombinant BMP therapy is based on recent preclinical and clinical studies.
Bone homeostasis can be defined through balance of bone formation and bone resorption. In bone remodeling, a continuous and dynamic process that is going on throughout life, old or damaged mineralized bone is removed by bone-resorbing cells, osteoclasts, and is replaced by new bone matrix (osteoid) made by osteoblasts. Osteoid subsequently becomes fully mineralized bone tissue (1).
Bone morphogenetic proteins (BMPs) were first discovered in 1965 by Marshall Urist as endogenous factors which could induce ectopic bone formation (2). Subsequent research confirmed the role of BMPs in bone formation (3, 4). Today, it is known that all BMPs do not have the same effect and some of them do not induce ectopic bone formation, but for osteogenic BMPs, namely BMP2, -4, -5, -6, -7 and -9, bone-inducing properties in vitro and in vivo have been shown (5, 6). While most of earlier research was focused on effect of BMPs on bone forming cells, like osteo- and chondroprogenitors, increasing evidence indicates that BMPs effect osteoclasts as well, influencing also bone resorption and impacting thus the overall bone homeostasis. In this review, we focused on the role of BMPs on osteoclast differentiation and function and subsequently on bone resorption, as observed on in vivo and in vitro models.
BMPs are secreted signaling molecules which belong to the large protein family consisting of more than 30 ligands, called transforming growth factor-β (TGFβ) superfamily (7, 8) and comprise an evolutionary conserved family of cytokines required for numerous developmental processes. Among the TGFβ superfamily members, the bone-formation activity is unique to BMPs (9); however, it was shown that BMPs have many other biological activities (10). Since their isolation as promotors of bone and cartilage formation, BMPs have been extensively studied and, besides their confirmed role in bone and cartilage, have been found to hold multiple functions in the embryonic development of other tissues and organ systems, including blood vessels, brain, liver, heart, lung, gut, limb, eye, teeth, or kidney. The role of BMP family members in development was confirmed when the deletion of some Bmp genes (including Bmp2 and Bmp4) and their receptors resulted in early embryonic lethality, at the beginning of the development of most gastrointestinal organs (11). Although most BMPs are expressed in a diversity of tissues during embryogenesis (12–14), the expression of some BMP members becomes limited to specific tissues after birth (15).
BMP family members have been identified in vertebrates and invertebrates. Among vertebrates, BMPs have highly conserved structures shared by the members of the TGFβ superfamily. Based on structural homology and known functions, the BMP family members can be further classified into several subgroups, including the BMP2/4 group, BMP5/6/7/8 group, BMP9/10 group, and BMP12/13/14 group. BMP-3, -4, -5, and -6 are highly expressed in lung, whereas BMP7 is mostly expressed in kidney of human embryos (12, 15) and of adult mice (16). Further, BMP4, -7, and -14 are important for proper reproductive tissue development and BMP2, -3, and -7 contribute to cartilage regeneration (17). In vitro experiments using pluripotent mesenchymal progenitor C3H10T1/2 cells and preosteoblastic C2C12 cells showed that BMP-2, -6, and -9 exhibit high ability to induce both early and late osteogenic markers as well as matrix mineralization, while most BMPs can effectively promote the terminal differentiation of committed osteoblastic precursors and osteoblasts (18, 19). In contrast to other BMPs, BMP3 has been proposed to act as an inhibitor of osteogenic BMPs and antagonizes the osteogenic activity of BMP-2, -4, -6, -7, and -9 (20), while deletion of Bmp3 gene results in increased skeletal bone volume (21).
Before being secreted into extracellular space where they become active, BMPs, as well as other TGFβ superfamily members, are first synthesized and folded as precursor proteins in the cytoplasm. BMP precursors form dimers that are subsequently cleaved by proteases during secretion of mature BMP into extracellular space (22). Mature BMPs are secreted as monomers which contain three intramolecular disulfide bonds, whereas fourth disulfide bond dimerizes with another BMP monomer, producing a biologically active dimer which activates corresponding BMP receptors (23, 24).
BMPs have been shown to be activated through reassembling with their prodomain in the process where antagonistic proteins and decoy receptors modulate BMP activity (25). In contrast, TGFβ proteins form a latency complex where TGFβ in inactive form as homodimer, bound by latency-associated peptide (LAP) and latent TGFβ binding protein (LTBP), forms large latent complex (LLC) (26). For its activation, the noncovalent bond between LAP and TGFβ has to be disrupted. Among many activators of TGFβ, a significant role belongs to BMP1. Although able to induce bone and cartilage, BMP1 is not part of the TGFβ superfamily of proteins. Instead, it possesses a metalloproteinase structure and acts as a procollagen C-proteinase which regulates collagen maturation (27, 28). In a process of TGFβ activation, BMP1 cleaves LTBP at two distinct sites enabling thus subsequent cleavage of LAP by other matrix metalloproteinases and liberation of active TGFβ which can then exert its biological functions (29). BMP1 appears to be not only the activator of TGFβ, but is also a significant regulator of its activity (30, 31).
Like other TGFβ superfamily members, BMPs induce their effects through two types of serine-threonine kinase transmembrane receptors, type I and type II receptors. Upon binding to the receptors, a heterotetrameric complex is formed, consisting of two dimers of type I and type II receptors (32). BMPs are able of binding to type I receptors in the absence of type II receptors but their binding affinity increases when both type I and type II receptors are present (33). Activated receptor complexes at the cell surface activate two main types of intracellular pathways, canonical (SMAD-dependent signaling pathway) or non-canonical (p38 mitogen-activated protein kinase, p38 MAPK) (34, 35). Canonical signaling pathway is highly conserved and involves three types of intracellular signal transducer SMAD molecules. Phosphorylated SMAD proteins form complex accumulating in the nucleus, where it binds to the responsive DNA elements and regulates target gene expression (36). On the other hand, non-canonical pathway includes activation of different pathways associated with various protein kinases, like Rho-GTPase, JNK/P38, PI3K/AKT, and MAPK pathway (37).
Osteoclasts, cells primarily responsible for bone resorption, develop from hematopoietic stem cells in bone marrow, passing through several stages of differentiation (38). The two main differentiation factors that drive osteoclast maturation are Macrophage Colony Stimulating Factor (M-CSF) and Receptor-Activated Nuclear κB ligand (RANKL) (39–41), recognized by RANK (a RANKL receptor) expressed on osteoclast surface (42). During maturation, pre-osteoclasts differentiate into mononuclear cells expressing tartrate-resistant acid phosphatase (TRAP), and those TRAP-positive, mononuclear cells then fuse together into giant, multinucleated and polarized mature osteoclasts which can degrade skeletal matrix by secreting lytic enzymes (42, 43). The process of preosteoclast fusion is mediated by transmembrane protein DC-STAMP (44). Bone resorption occurs at the ruffled border, a morphological structure specific for mature osteoclasts consisting of complex folds of plasma membrane surrounded by an actin ring, adherently to the bone surface (45) (Figure 1). Osteoclast formation and subsequent bone resorption are inhibited by osteoprotegerin (OPG), a soluble factor produced by osteoblasts (41, 46).
Figure 1 Schematic representation of osteoclast differentiation. Macrophage Colony Stimulating Factor (M-CSF) induces hematopoietic stem cells to become osteoclast precursors, which, under influence of Receptor-Activated Nuclear κB ligand (RANKL) develop into mononucleated osteoclasts. Further, mononucleated osteoclasts undergo fusion, mediated by DC-STAMP protein, into giant multinucleated osteoclasts, which then, upon interaction with osteoblasts, differentiate into mature bone-resorbing osteoclasts with ruffled border, which secrete acids and matrix metalloproteinases. Image created with BioRender.com.
Bone cell morphology, differentiation patterns and signal transduction are studied using widely used bone cell cultures as models in vitro. Single cell-type culture is commonly used, however, this model cannot reliably reproduce signal transduction between different cell types. On the other hand, simultaneous presence of osteoblasts and osteoclasts can mimic cellular cross-talk and mechanisms of intercellular communication (47). In preclinical studies, usage of co-culture of osteoblasts and osteoclasts is advantageous as it presents more relevant model of bone remodeling process (48).
When describing co-cultures of osteoblasts and osteoclasts, indirect or direct models can be utilized. In indirect models, use of conditioned media (media from one cell type transferred to the other) or transwell inserts, which provide two culture surfaces in the same well by the use of permeable insert, allow the exchange of soluble factors, but without a direct contact between two cell types. Direct co-cultures imply both cell types on the same surface, in two-dimensional (2D) cell culture, or in a three-dimensional scaffold, which allows the immediate physical contact between cell types and enables exploring the effects of membrane-bound signaling factors (49).
Differentiation of osteoclasts is under control of osteoblast paracrine factors, such as RANKL, interleukins (IL) 1 and 6 and Tumor necrosis factor α (TNFα) (50). Another important way of intercellular communication is direct cellular contact between osteoblasts and osteoclasts, driven mainly by Ephrin, Semaphorin 3A and FAS ligand-activated pathways (51). However, osteoclasts also in turn influence osteoblasts by secreting diffusible factors such as sphingosine-1-phosphate, Semaphorin 4D, platelet-derived growth factor and others, as well as by releasing growth factors from extracellular matrix (ECM) during bone resorption, in particular TGFβ1 and insulin-like growth factor (IGF-1) (50, 51). Additionally, osteocytes, cells derived from osteoblasts and embedded in bone matrix, secrete sclerostin (SOST), a protein which inhibits osteoblast differentiation but stimulates osteoclastogenesis (52) (Figure 2). TGFβ, a multifunctional cytokine, has been demonstrated to regulate osteoclastogenesis; however, its role in osteoclast maturation appears to be very complex, since TGFβ has both stimulatory and inhibitory effect on osteoclast precursors and mature osteoclasts (53–55), depending also on intracellular signaling pathways activated upon its binding to the cell surface (56).
Figure 2 Interaction between osteoblasts and osteoclasts in bone remodeling process. Differentiated, mature osteoclasts secrete acids and matrix metalloproteinases which degrade mineralized bone. Bone resorption mediated by osteoclasts releases TGFβ and IGF-1 from bone matrix, which induce osteoblast activity and subsequent bone formation. M-CSF, RANKL and OPG secreted by osteoblasts additionally influence osteoclast differentiation and activity. In turn, osteoclasts secrete various factors which positively (S1P, PDGF) or negatively (SEMA4D) influence osteoblast differentiation. At the end of demineralization process, osteoblast precursors (preosteoblasts) are recruited at the resorption site, differentiating into mature osteoblasts which then form new, unmineralized matrix (osteoid). Upon mineralization, mature osteoblast differentiate into osteocytes which secrete sclerostin, additionally stimulating osteoclastogenesis but inhibiting osteoblast differentiation. OPG, osteoprotegerin; TGFβ, transforming growth factor β; IGF-1, insulin-like growth factor 1; S1P, sphingosine-1-phosphate; PDGF, platelet-derived growth factor; SEMA4D, semaphorin 4D; SOST, sclerostin. Image created by BioRender.com.
Bone remodeling process consists of several phases: 1) recruitment and activation of preosteoclasts and their differentiation into osteoclasts, 2) resorption of the mineralized matrix by mature osteoclasts through acidification of extracellular environment, 3) reversal - end of resorption process, apoptosis of osteoclasts and recruitment of preosteoblasts, and 4) deposition of osteoid by mature osteoblasts and subsequent mineralization (48, 57). Upon mineralization, mature osteoblasts undergo apoptosis or differentiate into quiescent osteocytes (58). The majority of new bone formation takes place on resorbed bone surfaces (59) and sites of bone remodeling activity are called basic multicellular units (BMUs), distributed throughout the skeleton in different stages of remodeling cycle, i.e. asynchronously (60).
Bone resorption is followed by bone formation in tightly controlled coupling process in order to preserve bone balance and prevent bone loss (59). As unique cell type which have capability to resorb mineralized bone matrix, osteoclasts have the crucial role in bone remodeling. The dissolution of bone mineral matrix (composed mainly of crystalline hydroxyapatite) is possible due to the osteoclast secretion of hydrochloric acid into resorption lacunae (61). However, besides their catabolic role in bone homeostasis, evidence from human diseases and mouse genetic models indicate that osteoclasts also have anabolic role in this process by coupling activity with osteoblasts (62, 63). Independently of their resorption activity, osteoclasts secrete coupling factors which most likely promote recruitment of osteoblast precursors to the bone surface (60). Osteogenesis-related mRNAs in osteoblasts (Alph, RunX2, Col1) are up-regulated in co-culture of osteoblasts and osteoclasts, indicating their mutual influence (64).
Among wide variety of potential coupling factors [presented in (60)], BMPs have significant role in this process, and central role might have BMP receptor type IA (65), as seen from studies on animal knockout models. Mice with deletion of BMPRIA in osteoclasts showed increased osteoblastic bone formation, which suggests that BMPRIA signaling in osteoclasts negatively regulates osteoblast differentiation and bone mass (66, 67). Another study demonstrated that BMPRIA deletion changed expression of several genes involved in osteoblast-osteoclast communication, notably Cx43/Gja1 which encodes one of gap junction proteins (50). Recently, SMAD1/5 suggested to be regulatory pathway for osteoblast-osteoclast coupling via WNT and sphingosine kinase (SPHK1) (68). Collectively, BMPs act as important mediators in osteoblast-osteoclast communication and thus balance the rate of bone remodeling process (69), which could be of significant importance when considering potential therapies targeting BMP signaling pathways (65).
Better understanding of the important role of BMPs on skeletal development and bone homeostasis came from studies on genetically manipulated mice with global or conditional deletion of some of BMP ligands or their receptors (70, 71). Bmp genes and their downstream signal transducers are expressed early during development, before gastrulation (11). The critical role of BMP signaling during bone formation and developmental processes of whole body has been elucidated through numerous studies on genetically modified mice with conditional or global deletions of various Bmp genes. While some of global BMP deletions (BMP5, BMP6) have minor impact on development, complete loss of function of some BMPs results in prenatal (BMP2, BMP4) or early postnatal death (BMP7) (Table 1). To avoid embryonic lethality, for genetical studies on BMPs in mice a conditional knockout system (Cre-LoxP) has been used in further studies (80).
Among BMP knockout models listed in Table 1, BMP2 is most extensively studied. Conditional ablation of BMP2 in skeletally adult mice revealed that BMP2 affects functions in both osteoblasts and osteoclasts, with its deletion, in combination with deletion of BMP4, leading to the diminished osteoid formation and trabecular bone loss (74). Similar to BMP2 knockout, BMP4 knockout mice die before birth and show abnormal mesodermal differentiation (75). Interestingly, in contrast to BMP2 conditional deletion in chondrocytes, conditional deletion of BMP4 in these cells shows only minor changes in cartilage phenotype (73). During limb development in Bmp2, Bmp4 and Bmp7 conditional knockout mice, initiation of chondrogenesis and chondrogenic differentiation starts in the absence of both BMP2 and BMP4 or BMP2 and BMP7, however, both, BMP2 and BMP4 together are required for completion of osteogenesis (81). An opposite animal model, mice with overexpression of Bmp4 in osteoblasts developed osteopenia due to the increased osteoclastogenesis, implicating mutual influence between main bone cell types (82). It must be emphasized that BMP-induced ectopic bone formation does not mirror the real situation in bone microenvironment, since bone formation at ectopic site initially does not include osteoclasts, which are a significant factor not only in bone resorption, but also in new bone formation and its homeostasis (83).
Besides deletions of BMP ligands, models with mutations in BMP receptors were especially useful in studies of BMP signaling. Complete loss of BMP receptor type 1A due to the null mutation in Bmpr1A gene causes embryonic lethality and no mesoderm formation (84). However, conditional deletion of this gene targeted to osteoclasts caused increased bone volume and increased osteoblastic bone formation, indicating important role BMP signaling might have in osteoblast-osteoclast communication (67). Other transgenic mouse models involving BMP receptor genes are discussed in more detail in the subheading “BMP signaling “later in this review.
It is known that BMPs coordinate many developmental processes, including body axis determination, germ layer specification and tissue morphogenesis (85), and that BMP signaling pathway remained conserved during evolution across distant animal species. In the cell, BMPs are produced as precursor proteins, consisting of a signal peptide, pro-domain and mature peptide. Upon cleavage of the signal peptide, precursor protein undergoes glycosylation and dimerization inside cytoplasm and is secreted in dimeric form as mature protein, whereas the pro-domain is cleaved (86). On the cell surface, BMPs bind to Type I or Type II BMP receptors which are transmembrane proteins with intracellular serine/threonine kinase domain. Activated receptors then mediate signal transduction mainly via canonical SMAD-dependent signaling pathway (SMAD 1/5/8 or SMAD 2/3) (87, 88).
The role of BMPs in bone formation is well described in literature (89). BMP2, -4, -5, -6, -7 and -9 exhibit high osteogenic activity (5, 34). It is known that BMP2 and -7 increase osteoblastic differentiation markers (34, 90), and that BMP signaling promotes chondrocyte differentiation (91). By acting on osteoblasts and chondrocytes, BMPs enable process of endochondral bone formation and ossification (92). In osteoblasts, BMPs act in a complex interaction with several signaling pathways, including Wnt, Notch, Hedgehog and FGF (89, 93). Loss of BMP function caused by genetic deletion of certain BMP genes and BMP receptors induces multiple skeletal defects in various mouse genetic models (80).
BMP activity in bone cells is additionally regulated by several proteins which act as BMP antagonists. The most important BMP inhibitors are noggin and chordin, which bind BMPs (especially BMP2 and -4) with high affinity, preventing thus their interaction with receptors (94). Addition of noggin to bone marrow cultures inhibited both osteoblast and osteoclast formation, whereas addition of noggin-specific antibody increased osteoblast progenitor formation (69). Hence, BMPs, in balance with noggin as their main antagonist, may provide baseline control for the bone remodeling rate (95).
Extracellular matrix is another important regulator of BMP biological activity in bone (96). Binding of TGFβ proteins to type IV collagen, a major component of ECM of basement membrane, has been demonstrated (97), as well as binding of BMP4 (98) and BMP7 (15). Besides collagen, other ECM components, such as small leucine-rich proteoglycans and fibrillins, can also bind BMPs and thus act as regulators of their bioavailability in the extracellular space (26, 34, 99). Extracellular BMP-binding components can act as its inhibitors by sequestering BMPs from their target cellular receptors, but can also promote BMP signaling by different mechanisms (100).
Although the role of BMPs in osteoblast maturation and function is well-known, their role in osteoclasts is not so extensively studied (92). Several studies demonstrated that osteoclasts endogenously express several BMP ligands (BMP2, BMP4, BMP6 and BMP7), BMP receptors (BMPR1A, BMPR1B and BMPR2) and SMAD proteins (57, 101–103). In particular, there are several studies underlining the role of BMP2 and BMP4 in osteoclastogenesis and bone resorption (82, 102–105). Transgenic mice overexpressing Noggin (inhibitor of BMP action) in osteoblasts showed decreased bone formation rate and significant decrease in osteoclast number, implicating the important role of BMP signaling in osteoclasts as well as in osteoblasts (82). Osteoclasts and osteoclast precursors express BMP receptors, which was confirmed in numerous studies (57, 67, 102, 103). These receptors are of key importance for intracellular BMP signal transduction, a process which enables BMPs to exert different effects on osteoclast maturation and function.
Various studies demonstrated different effects of particular BMPs on osteoclasts, depending on the model used and type of experimental cell treatment (Table 2). Most of studies performed so far report stimulatory effect of BMPs on osteoclast formation (117). In the following sub-section, the effect of most frequently studied BMPs on osteoclasts will be presented, which is also summarized in Figure 3.
Figure 3 Effects of individual BMPs on osteoclasts. BMPs can influence osteoclasts either directly, stimulating their differentiation (BMP2, -5, -6, -7) and resorptive activity (BMP4, -7), or indirectly, through stimulation of osteoblasts (BMP2, -4, -7) which then increase expression of RANKL and stimulate osteoclast maturation. In addition, BMP6 expressed by osteoclasts stimulates osteogenic activity of osteoblasts. Image created by BioRender.com.
BMP2, which has been the most studied of BMPs in osteoclasts as well as in osteoblasts, initially demonstrated stimulatory effect on osteoclast differentiation and activity only in the presence of stromal cells, which implicated indirect action of BMP2 on osteoclasts (106). Other studies in vitro also indicated the indirect effect of BMP2 on osteoclasts, acting via regulation of RANK expression in osteoblasts (118), and including 1,25(OH)2D3 as a mediator which decreases production of osteoprotegerin, accelerating thus osteoclastogenesis (119). However, a number of studies demonstrated that BMP2 derived from bone marrow macrophages has also a direct autocrine effect on osteoclast differentiation and maturation, activating canonical intracellular signaling pathway (69, 103, 105). It was also shown that BMP2 in osteoclasts can activate both canonical and non-canonical signaling pathway, depending on the stage of osteoclast differentiation, with p38 phosphorylation in the pre-fusion osteoclasts, and increased SMAD phosphorylation occurring at osteoclast fusion stage (102).
Recently, osteoblast-osteoclast contact ex vivo was facilitated by introduction of BMP2 immune complexes consisting of immobilized antibodies specific for BMP2 which sequestered endogenous BMP2. As a consequence, facilitated osteoblast-osteoclast interaction in vitro stimulated osteoblastogenesis and suppressed osteoclastogenesis, most probably via enhanced EphrinB2/EphB4 signaling pathway (120), suggesting the role of BMP2 not only in particular cell type, but also in their mutual communication.
BMP4 is closely related to BMP2 and both molecules are required, not only for osteoblastogenesis, but also for proper osteoclastogenesis (69). Mouse overexpression models confirmed the stimulatory role of BMP4 in osteoclast differentiation (82, 121), most likely acting indirectly through stimulation of osteoblasts (69, 82). In bone marrow-derived stromal cells BMP4 was shown to induce expression of osteoprotegerin through the activation of p38 kinase, which could be the mechanism for regulation of osteoclast differentiation (122). However, BMP4, as well as BMP2, also acts directly on mature osteoclasts stimulating their bone-resorbing activity (104, 123).
In vivo, both BMP4 and BMP2 are essential for completion of osteogenesis (81). As seen from mouse knock-out models, BMP4 is also essential for mesoderm formation during development (75). Osteoclasts near the fracture site express BMP4, as well as BMP2 and BMP7, implicating their role in bone remodeling and fracture healing (124).
In primary cultures murine bone marrow cells, BMP5 stimulated osteoclastogenesis, but with a biphasic effect, with higher concentrations (>300 ng/mL) being less stimulative on osteoclast formation than lower concentrations (0.1-100 ng/mL), and maximal effect was achieved at 1 ng/mL BMP5 (108). Similar study on primary rat bone marrow cells demonstrated stimulative effect of BMP5 on osteoclast-specific marker expression, it was significantly lower than for BMP2 or BMP4 (109). In cultured bone marrow cells, BMP5 likely acts by decreasing OPG and increasing RANKL mRNA expression, stimulating thus osteoclast differentiation (108). In vivo, numerous non-fatal skeletal defects were described in mice with inactivated Bmp5 gene (76), suggesting the role of BMP5 in bone remodeling. The stimulatory effect of BMP5 seems to be more expressed in osteoblasts than in osteoclasts (108), and stimulation of osteoclastogenesis is enhanced in combination of BMP5 and BMP2 (125).
Among multiple types of BMPs tested, BMP6 was one of most potent osteogenic BMPs (18, 109, 126), also due to its resistance to noggin (127), but it seems to be less potent in stimulation of osteoclasts than in osteoblasts (65, 108, 128). Structurally closely related to BMP5, BMP6 has also been shown to have stimulatory effect on osteoclasts at some concentrations, although both, BMP5 and BMP6, were less effective in stimulating osteoclastogenesis in comparison with BMP2 (108). BMP6 is expressed by mature osteoclasts and it was identified as one of the factors responsible for coupling bone resorption and osteoblast maturation, acting through increased activation of BMP pathways and activating thus osteoprogenitor cells (111). Osteoclasts contain relatively high levels of Bmp6 mRNA, which could be important for regulation of overall bone homeostasis, not only for osteoblastic stimulation, but also for fine regulation of osteoclastogenesis (57). Systemic administration of BMP6 on rat model of osteoporosis in vivo increased osteoprotegerin serum levels uncoupling thus osteoclast from osteoblast activity (110). Interestingly, mice with inactivated BMP6 have only minor skeletal defects, such as prolonged ossification of sternum (77), but after revised phenotype, haemochromatosis with high iron content in organs has been discovered (129). Preferential stimulation of osteoblasts rather than osteoclasts gives advantage to BMP6 when considering its therapeutic use in bone healing (130, 131).
Also known as osteogenic protein-1 (OP-1), BMP7 is, together with BMP2, one of BMPs with recognized therapeutic potential, first in animal studies and then in clinical trials (132). However, its osteogenic activity was accompanied by side effects including enhanced bone resorption and osteolysis at the osteotomy site (133, 134). Several studies in vitro showed that BMP7 promotes osteoclast formation in rodent bone marrow cell cultures, where its effect depends on the applied concentration (109, 112, 135). In combination with 1,25(OH)2D3, BMP7 stimulated not only osteoclast formation, but also resorption activity (112). In contrast, in human CD14+ monocyte culture, BMP7 inhibited osteoclast formation, apparently by down-regulation of transcription factor NFATc1, which is necessary for proper osteoclastogenesis (113). The reason for this difference could be in model of cell culture used (mouse or rat vs. human). It is possible that BMP7, similarly to the BMP2, acts on osteoclasts indirectly, through activation of osteoblasts (6).
With its sequence being closely related to BMP7, BMP8, at first known as osteogenic protein 2 (OP-2) was identified in mouse embryos (16, 136). A recent transcriptomic analysis revealed that BMP8, similarly to BMP2,-4 and -7, can induce SMAD-signaling pathway in mesenchymal stem cells (137). BMP8 seems to have a protective role in osteoblasts exposed to glucocorticoids (138); however, studies about BMP8 action on osteoclasts are still lacking.
First studies in vitro demonstrated positive effect of BMP9 on osteoclastogenesis. In human blood cord monocyte culture, BMP9 did not affect osteoclast formation, but increased their resorption activity, acting probably via SMAD1/5/8 and ERK1/2 pathway (115). Subsequent study on mouse spleen macrophages showed that BMP9 promoted proliferation and differentiation of osteoclast precursor cells in dose-dependent manner (114). However, a recently published study demonstrated an opposite efect of BMP9 on osteoclasts, suppressing RANKL-induced osteoclast differentiation of bone marrow macrophages in vitro, and preventing bone loss in mouse ovariectomy model in vivo, showing thus strong osteogenic effect (116).
BMP stimulates the downstream signaling pathways by activating two types of BMP receptors. Type I and type II BMP receptors are the only known class of transmembrane cell surface receptors in humans with serine/threonine kinase activity. These two types of receptors share similar structural properties comprised of a relatively short extracellular domain and a single pass transmembrane protein with an intracelular serine/threonine kinase domain. Type II receptors are constitutively active, and after ligand binding they phospohorylate a Gly/Ser-rich domain of type I receptors and activate a kinase activity. Type I BMP receptors are Ser/Thr-protein kinase receptor R3 (ALK1), activin receptor type−1 (ACVR1/ALK2), BMP receptor type−1A (ALK3) and BMP receptor type−1B (ALK6), whereas BMP receptor type−2 (BMPR2), activin receptor type−2A (ACVR2A) and ACVR2B can function as type II BMP receptors (87, 139). Several studies revealed that osteoclasts express Bmpr1a, Bmpr1b and Bmpr2 mRNA or protein (50, 67, 102). Regulation of BMP receptor expression in osteoclasts is not yet fully explained.
BMP ligands in osteoclasts act either through the canonical or non-canonical signaling pathways. The canonical pathway, also known as SMAD signaling pathway, involves three types of SMAD proteins: receptor SMADs (R-SMADs) transduce signals, common SMADs (Co-SMADs) support gene transcription activation and inhibitory SMADs negatively regulate BMP signaling. SMADs are homologues of Drosophila melanogaster Mad proteins (mothers against decapentaplegic) and Caenorhabditis elegans SMA proteins (small body size), and encode cytoplasmic proteins required for responsiveness to BMP superfamily ligands (140). Activated (phosphorylated) type I receptors recruit and phosphorylate pathway-specific R−SMADs (SMAD1, SMAD5 and SMAD8), which can form trimers with SMAD4 (Co-SMAD) and translocate to the nucleus where they target the genome via consensus SMAD-binding motifs, integrate with tissue-specific transcription factors and recruit chromatin remodeling machinery (141). A number of studies have shown that osteoclasts express SMADs as well as phosphorylated SMADs (68, 102, 103). An inhibition of SMAD signaling pathway leads to smaller and less active osteoclasts which suggests that BMP-mediated SMAD signaling plays a role in osteoclast fusion and activation (102, 135, 142). Deletion of Smad4 in systems in vitro demonstrated that loss of Smad4 during the early stages of osteoclast differentiation results in the loss of osteoclast differentiation as was measured by decreased expression of Nfatc1 and DC-STAMP, as well as decrease in pSMAD2/3 expression (143). However, conditional deletion of Smad4 in mature osteoclasts resulted in osteopenia due to increased osteoclast formation and bone resorption, and lead to an osteopenic phenotype caused by changes in the sensitivity to TGFβ signaling but not due to changes in BMP signaling (144). Additionaly, research on mice with conditionally deleted Smad1/5 in osteoclast precursors led to mild bone gain due to reduced bone resorption and stimulated bone formation (68). Importance of canonical BMP signaling during the time of osteoclast fusion was shown when using dorsomorphin in fusion staged osteoclasts, where inhibition of type I receptors inhibited intracellular SMAD signaling and osteoclast differentiation (102, 103, 143).
Studies on osteoclasts and osteoclast precursors in transgenic mouse models have indicated different roles of type I and type II BMP receptors in osteoclast formation and bone resorption with complex mechanisms of signal transduction involving both canonical and non-canonical pathways. Conditional knockout of the BMPRIa receptor in osteoclast progenitors resulted in a decrease in osteoclastogenesis and expression of DC-STAMP (145), the master regulator required for preosteoclast fusion (44, 146). Its expression is regulated by an essential transcription factor, Nfatc1, which is activated downstream of RANKL and BMP-signaling pathway (147). Inhibition of BMP signaling leads to a decrease in DC-STAMP and Nfatc1 gene expression, resulting in fewer, smaller, and less active osteoclasts, showing the requirement of BMP signaling in preosteoclast fusion (82, 103). In osteoclasts derived from BMPRIA conditional knockout mice mRNA levels of Pu.1 and Mitf, transcription factors required for osteoclast commitment and early differentiation, were increased, whereas the mRNA levels of late osteoclast differentiation markers and Nfatc1 were downregulated, indicating that BMPRIA deficiency enhanced the initial differentiation but disrupted the maturation of osteoclasts (145). Addition of (soluble) BMPRIA on osteoclast formation in bone marrow macrophage cultures suppressed osteoclast formation induced not only by the combination of RANKL and BMP2, but also by RANKL alone (105). Therefore, BMP signaling may be required for RANKL-mediated osteoclastogenesis.
In contrast to the deletion of Bmpr1a, in mice with global knockout of Bmpr1b enhanced proliferation and survival of osteoclast precursor was observed, along with reduced apoptosis and reduced resorption activity (148). Despite decreased resorption, these mice showed transient osteopenia, probably due to the compromised differentiation of osteoblasts where BMP signaling also plays an important role. However, osteoblast and osteoclast activity in vivo were not observed (148), implicating more subtle role of BMPRIB receptor in the regulation of bone remodeling.
Mutations in BMPRII are more extensively explored in diseases not related to the bone metabolism, such as pulmonary arterial hypertension (PAH) (149). A study with bone marrow-derived Bmpr2-deficient osteoclasts showed decreased osteoclast differentiation and resorptive activity (102). Mice with Bmpr2 conditional knockout had increased bone volume and trabeculae with osteopetrotic phenotype due to the reduced bone resorption. At the cellular level, these mice had changes in the non-canonical signaling (MAPK) and no changes in the canonical signaling (SMAD) pathway, as was measured by levels of phosphorylated and nonphosphorylated forms of SMAD proteins and downstream elements of non-canonical signaling pathway, suggesting rather complex mechanism of intracellular signalization with non-canonical pathway being important for proper osteoclastogenesis (102).
The non-canonical BMP signaling pathway consists of mitogen-activated protein kinase (MAPK) and several downstream signaling molecules, including c-Jun N-terminal kinase (JNK), mitogen-activated protein kinase 38 alpha (p38α) and extracellular regulated kinases (ERK), all of which are activated by BMP 2 in osteoclasts (102, 115). One of upstream signaling molecules of the non-canonical signaling pathway, TGFβ Activated Kinase 1 (TAK1), is required for osteoclast differentiation, as seen from the specific knockout of TAK1 in osteoclasts, which lead to an osteopetrosis-like phenotype with decreased resorptive activity (150). TAB1, an activator of TAK1 protein, participates together with TAK1 in the BMP signaling pathway. It was found that another regulatory molecule, X-linked inhibitor of apoptosis protein (XIAP), serves as an adaptor protein linking the BMP receptors and TAB1-TAK1 complex. XIAP was determined as a TAB1-binding protein and interacts not only with TAB1 but also with BMP type I and type II heteromeric receptor complex, linking BMP signaling pathway with intracellular regulators of osteoclastogenesis (151).
Besides BMP2, which activates MAPKs, ERK1/2, JNK and p38 in osteoclasts (102), it has been shown that BMP9 in osteoclasts also stimulates the activation of two signaling pathways, as seen from activation of both SMAD1/5/8 and ERK1/2 (115), suggesting that BMPs can activate both non-canonical and canonical signaling and that TAK1 has a crucial role in this process (115, 151, 152) (Figure 4).
Figure 4 BMP mechanisms of canonical and non-canonical signaling in osteoclasts. After BMP ligand binding type II receptors phosphorylate (P) the type I receptors. Activated type I receptors recruit and phosphorylate canonical pathway specific R-SMADs (SMAD1/5/8) which, with the help of co-SMAD (SMAD4), transduce the signal into the nucleus. The non-canonical BMP signaling pathway is transduced by the recruitment of TAB1/TAK1 complex through XIAP. Activated TAK1 kinase can stimulate the downstream non-canonical MAP kinase effector proteins or canonical SMAD proteins. Activated MAPKs can translocate to the nucleus to phosphorylate a number of transcription factors (TF), thereby changing target gene transcription. BMPR1 and BMPR2, BMP receptor 1 and 2; XIAP, X-linked inhibitor of apoptosis protein; TAK1, TGFβ Activated Kinase 1; TAB1, TGFβ Activated Kinase 1 binding protein; p38, mitogen-activated protein kinase 38; JNK, c-Jun N-terminal kinase; ERK, extracellular regulated kinases; SRF, serum response factor; TCF, ternary complex factor family member; AP1, activator protein 1 complexes; ATF2, activating transcription factor 2. Image created by BioRender.com.
In bone healing, inflammatory response, a sequentional process involving complex interaction between multiple cell types, modulates microenvironment at the fracture site, and is crucial in initial phase of fracture healing (153). The significance of inflammatory process in fracture healing is additionally underlined by studies on animal models where healing is delayed in the absence of proinflammatory cytokines such as interleukin-6 (IL-6) or tumor necrosis factor-α (TNFα) (154, 155). During fracture healing, mesenchymal stem cells are recruited from the periosteum and bone marrow and differentiate into chondrocytes and osteoblasts which form callus and new bone (156). Among numerous cytokines and growth factors involved in this process, BMPs released by immune and osteoprogenitor cells play a crucial role in inducing osteogenic differentiation following inflammatory phase. Studies on inflammatory and autoimmune disorders pointed to the fine regulation of this process and to the complex interaction between BMPs and inflammatory cytokines. Mostly studied conditions are rheumatoid arthritis (RA) and ankylosing spondylitis (AS), where BMPs seem to have opposite roles. In RA, increased expression of BMP2, -6 and -7 observed in synovial fluid of RA patients indicated the role of BMPs in the development of this disease, probably by inducing proinflammatory phenotype of endothelial cells, stimulating adhesion of monocytes to the endothelium and, additionally, osteoclast differentiation, which subsequently leads to the bone loss observed in RA patients (157). On the other hand, enhanced BMP signaling in AS stimulates osteogenesis and induces heterotopic endochondral bone formation (158, 159). Proinflammatory cytokines upregulate expression of BMP2 and -6, indicating association between BMP activity and inflammatory processes in affected joints (160).
Since BMPs belong to the TGFβ superfamily of proteins, it is noteworthy that patients with chronic inflammation have elevated serum TGFβ levels, due to macrophage activation at the inflammation site. In those patients, decreased bone density is often observed, most likely due to the enhanced osteoclastic bone resorption stimulated by TGFβ (161). In parallel, chronic exposure of osteoblasts to TGFβ leads to the loss of their functionality, probably due to the continuous SMAD2/3 activity with concomitant decreasing of SMAD1/5/8 activity, which is otherwise activated by BMPs (162). Similarly, bone loss observed in affected joints of patients with RA is attributed to the increased osteoclastogenesis enhanced by proinflammatory cytokines (IL-1, IL-6, IL-17, TNFα), which induce RANKL expression in stromal cells and thus stimulate osteoclast precursors (163). IL-1 also directly stimulates osteoclast activity, even in absence of stromal cells (164). Proinflammatory cytokines not only stimulate osteoclastogenesis, but also inhibit osteoblast differentiation contributing to the overall bone loss (158). For example, TNFα acts opposite to the osteogenic transcriptional factors induced by BMPs (165), whereas IL-1 inhibits recruitment and migration of osteoblasts (166). Therefore, in addition to regulation of bone remodeling, BMPs are also involved in inflammatory conditions which indirectly affect bone homeostasis (167).
In order to overcome complications associated with non-healing bone fractures, therapeutic concepts using BMPs were developed (83). Numerous studies confirmed the effectiveness of BMPs in promoting osteogenesis (18, 168). In clinical use, therapeutic BMP devices usually consist of bovine collagen matrix as a carrier with added BMP2 (Infuse Bone Graft) or BMP7 (Osigraft) as an active substance (132, 169). However, complexity of biological effects of BMPs lead to side effects in vivo which were not observed in experimental systems in vitro, among which most prominent effect was stimulation of osteoclastic bone resorption (117). First clinical studies involving BMP-based therapies, namely BMP2, resulted in increased bone resorption rather than formation (170, 171), suggesting the role BMPs have in osteoclast activation (172) and subsequent osteolysis (142). When applied to the bone, BMP2 and BMP7 caused increased bone resorption, as demonstrated in preclinical (173) as well as in clinical studies (174, 175). Although it is well known that BMPs induce new bone formation, conditional deletion of BMP signaling in osteoblast appeared to have an inhibitory effect on osteoclastogenesis, implicating the complex role BMPs have on bone remodeling in vivo (176). Additionally, mice overexpressing BMP4 developed osteopenia due to the increased osteoclast number (82). Based on in vitro and in vivo studies, it became obvious that effect of BMPs on bone in vivo is a result of a stimulation of not only osteoblasts, but also osteoclasts and their progenitors which express BMP receptors (104, 177). It was also shown that BMP2 stimulates osteoclast formation in the presence of proinflammatory cytokine IL-1α and therefore it could enhance bone resorption due to the inflammatory environment at the site of the surgery (107). Furthermore, in ectopic bone formation the development of osteoclasts induced by BMP2 was demonstrated (178).
For BMP2 and BMP7, which were first BMPs tested in clinical trials, it was shown that, although promoting osteoblast differentiation, have significant impact also on osteoclasts, resulting in a net bone loss (83). Indeed, in clinical studies using BMP7 for distal radial osteotomy (133) and spinal fusion surgery (175, 179), pronounced bone resorption was observed. This effect was especially prominent in patients receiving BMPs in spinal fusion (95, 170, 180), whereas use of BMP7 in unstable thoracolumbar fracture resulted in segmental collapse due to the severe bone resorption (134). This pronounced effect on vertebrae was likely caused by the stimulatory effect of large amounts of BMP2 and BMP7 used on osteoclasts at endosteal and trabecular surfaces (83). The stimulation of endosteal osteoclasts could be an important step in bone healing, removing nonfunctional bone pieces following a fracture, accompanied by parallel formation of the new bone tissue (173), however, in clinical use, this BMP-induced osteoclast stimulation could lead to unwanted osteolysis. Further, if applied BMPs promote both bone resorption and formation, the end result may be impaired healing (181). The effect of BMP2 and BMP7 in clinical trials depends also on the amounts used; however, large amounts of BMPs usually used in spinal fusion may lead to the increased resorption in localized areas (95). Also, in currently available commercial devices, large amounts of BMP2 or -7 significantly exceed the biological need. Increased bioavailability due to the large amounts of applied BMP2 or -7 could finally result in unwanted side effects (174, 175), although studies on animal models demonstrated that systemic BMP2 and -7 will not stimulate generalized bone loss (182).
Recently, a new osteogenic device was developed, using BMP6 as an active substance and autologous carrier made from the peripheral blood (83, 183). This device, named Osteogrow, could overcome the limits of previously used BMP2 and BMP7 which were applied in large concentrations (131). BMP6 uses most of BMP receptors type I for signal transduction and stimulates osteoblast activity in cell cultures (102, 148). Studies of osteoclast cultures in vitro demonstrated preferential expression of Bmp6 mRNA compared to expression of other BMPs (57); however, osteoclastogenesis in vitro is more stimulated by addition of BMP2 or BMP5 than by BMP6 (108). Although data on specific BMP6 effects on osteoclasts are scarce, it seems that BMP6 stimulates osteoblasts more than osteoclasts, which puts this protein into advantageous position regarding potential clinical use when compared to other BMPs. Indeed, in recently published clinical studies which applied Osteogrow in patients with distal radius fracture and high tibial osteotomy, no side effects related to the use of BMP6 were recorded, and no osteolysis or other signs of increased osteoclast activity were observed in these patients (184, 185). Therefore, when considering BMPs as therapeutics in delayed bone healing and other complex orthopaedic indications, the potential effect of particular BMPs on osteoclast proliferation and activity would be of great clinical importance.
A large number of in vitro and in vivo studies indicate that osteoclasts are not merely bone-degrading cells, but they also have an important function in osteoblast activation, bone remodeling and maintenance of bone homeostasis. Among numerous signaling molecules regulating their differentiation and activity, BMPs in particular seem to have important role in regulating osteoblast-osteoclast communication. Since BMPs have a therapeutic potential as bone-healing agents, it is of major importance to consider their effects on osteoclasts as well as on osteoblasts, in order to avoid potential unwanted side effects such as increased bone resorption and osteolysis.
TB-N and VK wrote draft of the manuscript and created figures, SV designed review content, edited first draft and approved final version. All authors contributed to the article and approved the submitted version.
This article was supported by the Scientific Center for Excellence for Reproductive and Regenerative Medicine (project “Reproductive and regenerative medicine – exploration of new platforms and potentials” GA KK.01.1.1.01.0008 funded by the EU through the ERDF).
The authors declare that the research was conducted in the absence of any commercial or financial relationships that could be construed as a potential conflict of interest.
All claims expressed in this article are solely those of the authors and do not necessarily represent those of their affiliated organizations, or those of the publisher, the editors and the reviewers. Any product that may be evaluated in this article, or claim that may be made by its manufacturer, is not guaranteed or endorsed by the publisher.
1. Hadjidakis DJ, Androulakis II. Bone Remodeling. Ann NY Acad Sci (2006) 1092:385–96. doi: 10.1196/annals.1365.035
2. Urist MR. Bone: Formation by Autoinduction. Science (1965) 150:893–9. doi: 10.1126/science.150.3698.893
3. Wozney JM, Rosen V, Celeste AJ, Mitsock LM, Whitters MJ, Kriz RW, et al. Novel Regulators of Bone Formation: Molecular Clones and Activities. Science (1988) 242:1528–34. doi: 10.1126/science.3201241
4. Sampath TK, Coughlin JE, Whetstone RM, Banach D, Corbett C, Ridge RJ, et al. Bovine Osteogenic Protein is Composed of Dimers of OP-1 and BMP-2A, Two Members of the Transforming Growth Factor-Beta Superfamily. J Biol Chem (1990) 265:13198–205. doi: 10.1016/S0021-9258(19)38285-7
5. Luu HH, Song WX, Luo X, Manning D, Luo J, Deng ZL, et al. Distinct Roles of Bone Morphogenetic Proteins in Osteogenic Differentiation of Mesenchymal Stem Cells. J Orthop Res (2007) 25:665–77. doi: 10.1002/jor.20359
6. Kamiya N. The Role of BMPs in Bone Anabolism and Their Potential Targets SOST and DKK1. Curr Mol Pharmacol (2012) 5:153–63. doi: 10.2174/1874467211205020153
7. Kingsley DM. What do BMPs do in Mammals? Clues From the Mouse Short-Ear Mutation. Trends Genet (1994) 10:16–21. doi: 10.1016/0168-9525(94)90014-0
8. Nolan K, Thompson TB. BMP and BMP Regulation: Structure and Function. In: Vukicevic S, Sampath TK, editors. Bone Morphogenetic Proteins: Systems Biology Regulators. Cham: Springer (2017). p. 73–111.
9. Sampath TK, Reddi AH. Homology of Bone-Inductive Proteins From Human, Monkey, Bovine, and Rat Extracellular Matrix. Proc Natl Acad Sci USA (1983) 80:6591–5. doi: 10.1073/pnas.80.21.6591
10. Sampath TK. The Systems Biology of Bone Morphogenetic Proteins. In: Vukicevic S, Sampath TK, editors. Bone Morphogenetic Proteins: Systems Biology Regulators. Cham: Springer International Publishing (2017). p. 15–38.
11. Zhao GQ. Consequences of Knocking Out BMP Signaling in the Mouse. Genesis (2003) 35:43–56. doi: 10.1002/gene.10167
12. Helder MN, Ozkaynak E, Sampath KT, Luyten FP, Latin V, Oppermann H, et al. Expression Pattern of Osteogenic Protein-1 (Bone Morphogenetic Protein-7) in Human and Mouse Development. J Histochem Cytochem (1995) 43:1035–44. doi: 10.1177/43.10.7560881
13. Vukicevic S, Kopp JB, Luyten FP, Sampath TK. Induction of Nephrogenic Mesenchyme by Osteogenic Protein 1 (Bone Morphogenetic Protein 7). Proc Natl Acad Sci USA (1996) 93:9021–6. doi: 10.1073/pnas.93.17.9021
14. Vukicevic S, Helder MN, Luyten FP. Developing Human Lung and Kidney are Major Sites for Synthesis of Bone Morphogenetic Protein-3 (Osteogenin). J Histochem Cytochem (1994) 42:869–75. doi: 10.1177/42.7.8014470
15. Vukicevic S, Latin V, Chen P, Batorsky R, Reddi AH, Sampath TK. Localization of Osteogenic Protein-1 (Bone Morphogenetic Protein-7) During Human Embryonic Development: High Affinity Binding to Basement Membranes. Biochem Biophys Res Commun (1994) 198:693–700. doi: 10.1006/bbrc.1994.1100
16. Ozkaynak E, Schnegelsberg PN, Jin DF, Clifford GM, Warren FD, Drier EA, et al. Osteogenic Protein-2. A New Member of the Transforming Growth Factor-Beta Superfamily Expressed Early in Embryogenesis. J Biol Chem (1992) 267:25220–7. doi: 10.1016/S0021-9258(19)74028-9
17. Kang Q, Sun MH, Cheng H, Peng Y, Montag AG, Deyrup AT, et al. Characterization of the Distinct Orthotopic Bone-Forming Activity of 14 BMPs Using Recombinant Adenovirus-Mediated Gene Delivery. Gene Ther (2004) 11:1312–20. doi: 10.1038/sj.gt.3302298
18. Cheng H, Jiang W, Phillips FM, Haydon RC, Peng Y, Zhou L, et al. Osteogenic Activity of the Fourteen Types of Human Bone Morphogenetic Proteins (BMPs). J Bone Joint Surg Am (2003) 85:1544–52. doi: 10.2106/00004623-200308000-00017
19. Luther G, Wagner ER, Zhu G, Kang Q, Luo Q, Lamplot J, et al. BMP-9 Induced Osteogenic Differentiation of Mesenchymal Stem Cells: Molecular Mechanism and Therapeutic Potential. Curr Gene Ther (2011) 11:229–40. doi: 10.2174/156652311795684777
20. Bahamonde ME, Lyons KM. BMP3: To be or Not to be a BMP. J Bone Joint Surg Am (2001) 83-A Suppl 1:S56–62. doi: 10.2106/00004623-200100001-00008
21. Banovac I, Grgurevic L, Rumenovic V, Vukicevic S, Erjavec I. BMP3 Affects Cortical and Trabecular Long Bone Development in Mice. Int J Mol Sci (2022) 23:785–800. doi: 10.3390/ijms23020785
22. Jones WK, Richmond EA, White K, Sasak H, Kusmik W, Smart J, et al. Osteogenic Protein-1 (OP-1) Expression and Processing in Chinese Hamster Ovary Cells: Isolation of a Soluble Complex Containing the Mature and Pro-Domains of OP-1. Growth Factors (1994) 11:215–25. doi: 10.3109/08977199409046919
23. Scheufler C, Sebald W, Hulsmeyer M. Crystal Structure of Human Bone Morphogenetic Protein-2 at 2. 7 A Resolut J Mol Biol (1999) 287:103–15. doi: 10.1006/jmbi.1999.2590
24. Bragdon B, Moseychuk O, Saldanha S, King D, Julian J, Nohe A. Bone Morphogenetic Proteins: A Critical Review. Cell Signal (2011) 23:609–20. doi: 10.1016/j.cellsig.2010.10.003
25. Sengle G, Ono RN, Sasaki T, Sakai LY. Prodomains of Transforming Growth Factor Beta (TGFbeta) Superfamily Members Specify Different Functions: Extracellular Matrix Interactions and Growth Factor Bioavailability. J Biol Chem (2011) 286:5087–99. doi: 10.1074/jbc.M110.188615
26. Robertson IB, Rifkin DB. Regulation of the Bioavailability of TGF-Beta and TGF-Beta-Related Proteins. Cold Spring Harb Perspect Biol (2016) 8. doi: 10.1101/cshperspect.a021907
27. Kessler E, Takahara K, Biniaminov L, Brusel M, Greenspan DS. Bone Morphogenetic Protein-1: The Type I Procollagen C-Proteinase. Science (1996) 271:360–2. doi: 10.1126/science.271.5247.360
28. Vukicevic S, Colliva A, Kufner V, Martinelli V, Moimas S, Vodret S, et al. Bone Morphogenetic Protein 1.3 Inhibition Decreases Scar Formation and Supports Cardiomyocyte Survival After Myocardial Infarction. Nat Commun (2022) 13:81. doi: 10.1038/s41467-021-27622-9
29. Ge G, Greenspan DS. BMP1 Controls TGFbeta1 Activation via Cleavage of Latent TGFbeta-Binding Protein. J Cell Biol (2006) 175:111–20. doi: 10.1083/jcb.200606058
30. Anastasi C, Rousselle P, Talantikite M, Tessier A, Cluzel C, Bachmann A, et al. BMP-1 Disrupts Cell Adhesion and Enhances TGF-Beta Activation Through Cleavage of the Matricellular Protein Thrombospondin-1. Sci Signal (2020) 13. doi: 10.1126/scisignal.aba3880
31. Predes D, Cruz JVR, Abreu JG, Mendes FA. CUB Domain-Containing Protein 1 (CDCP1) Binds Transforming Growth Factor Beta Family Members and Increase TGF-Beta1 Signaling Pathway. Exp Cell Res (2019) 383:111499. doi: 10.1016/j.yexcr.2019.111499
32. Rahman MS, Akhtar N, Jamil HM, Banik RS, Asaduzzaman SM. TGF-Beta/BMP Signaling and Other Molecular Events: Regulation of Osteoblastogenesis and Bone Formation. Bone Res (2015) 3:15005. doi: 10.1038/boneres.2015.5
33. Rosenzweig BL, Imamura T, Okadome T, Cox GN, Yamashita H, ten Dijke P, et al. Cloning and Characterization of a Human Type II Receptor for Bone Morphogenetic Proteins. Proc Natl Acad Sci USA (1995) 92:7632–6. doi: 10.1073/pnas.92.17.7632
34. Wu M, Chen G, Li YP. TGF-Beta and BMP Signaling in Osteoblast, Skeletal Development, and Bone Formation, Homeostasis and Disease. Bone Res (2016) 4:16009. doi: 10.1038/boneres.2016.9
35. Jann J, Gascon S, Roux S, Faucheux N. Influence of the TGF-Beta Superfamily on Osteoclasts/Osteoblasts Balance in Physiological and Pathological Bone Conditions. Int J Mol Sci (2020) 21:7597–650. doi: 10.3390/ijms21207597
36. Shi Y, Massague J. Mechanisms of TGF-Beta Signaling From Cell Membrane to the Nucleus. Cell (2003) 113:685–700. doi: 10.1016/s0092-8674(03)00432-x
37. Derynck R, Zhang YE. Smad-Dependent and Smad-Independent Pathways in TGF-Beta Family Signalling. Nature (2003) 425:577–84. doi: 10.1038/nature02006
38. Vaananen HK, Zhao H. Osteoclast Function - Biology and Mechanisms. In: Bilezikian JP, Raisz LG, Rodan GA, editors. Principles of Bone Biology, 2nd ed, vol. 1. San Diego: Academic Press (2002). p. 127–39.
39. Quinn JM, Elliott J, Gillespie MT, Martin TJ. A Combination of Osteoclast Differentiation Factor and Macrophage-Colony Stimulating Factor is Sufficient for Both Human and Mouse Osteoclast Formation In Vitro. Endocrinology (1998) 139:4424–7. doi: 10.1210/endo.139.10.6331
40. Nakagawa N, Kinosaki M, Yamaguchi K, Shima N, Yasuda H, Yano K, et al. RANK is the Essential Signaling Receptor for Osteoclast Differentiation Factor in Osteoclastogenesis. Biochem Biophys Res Commun (1998) 253:395–400. doi: 10.1006/bbrc.1998.9788
41. Yasuda H, Shima N, Nakagawa N, Mochizuki SI, Yano K, Fujise N, et al. Identity of Osteoclastogenesis Inhibitory Factor (OCIF) and Osteoprotegerin (OPG): A Mechanism by Which OPG/OCIF Inhibits Osteoclastogenesis In Vitro. Endocrinology (1998) 139:1329–37. doi: 10.1210/endo.139.3.5837
42. Boyle WJ, Simonet WS, Lacey DL. Osteoclast Differentiation and Activation. Nature (2003) 423:337–42. doi: 10.1038/nature01658
43. Kodama J, Kaito T. Osteoclast Multinucleation: Review of Current Literature. Int J Mol Sci (2020) 21:5685–715. doi: 10.3390/ijms21165685
44. Verma SK, Leikina E, Melikov K, Gebert C, Kram V, Young MF, et al. Cell-Surface Phosphatidylserine Regulates Osteoclast Precursor Fusion. J Biol Chem (2018) 293:254–70. doi: 10.1074/jbc.M117.809681
45. Teitelbaum SL. The Osteoclast and its Unique Cytoskeleton. Ann N Y Acad Sci (2011) 1240:14–7. doi: 10.1111/j.1749-6632.2011.06283.x
46. Suda T, Takahashi N, Udagawa N, Jimi E, Gillespie MT, Martin TJ. Modulation of Osteoclast Differentiation and Function by the New Members of the Tumor Necrosis Factor Receptor and Ligand Families. Endocr Rev (1999) 20:345–57. doi: 10.1210/edrv.20.3.0367
47. Borciani G, Montalbano G, Baldini N, Cerqueni G, Vitale-Brovarone C, Ciapetti G. Co-Culture Systems of Osteoblasts and Osteoclasts: Simulating In Vitro Bone Remodeling in Regenerative Approaches. Acta Biomater (2020) 108:22–45. doi: 10.1016/j.actbio.2020.03.043
48. Sieberath A, Della Bella E, Ferreira AM, Gentile P, Eglin D, Dalgarno K. A Comparison of Osteoblast and Osteoclast In Vitro Co-Culture Models and Their Translation for Preclinical Drug Testing Applications. Int J Mol Sci (2020) 21:912–34. doi: 10.3390/ijms21030912
49. Owen R, Reilly GC. In Vitro Models of Bone Remodelling and Associated Disorders. Front Bioeng Biotechnol (2018) 6:134. doi: 10.3389/fbioe.2018.00134
50. Shi C, Zhang H, Louie K, Mishina Y, Sun H. BMP Signaling Mediated by BMPR1A in Osteoclasts Negatively Regulates Osteoblast Mineralization Through Suppression of Cx43. J Cell Biochem (2017) 118:605–14. doi: 10.1002/jcb.25746
51. Kim JM, Lin C, Stavre Z, Greenblatt MB, Shim JH. Osteoblast-Osteoclast Communication and Bone Homeostasis. Cells (2020) 9:2073–86. doi: 10.3390/cells9092073
52. Winkler DG, Sutherland MK, Geoghegan JC, Yu C, Hayes T, Skonier JE, et al. Osteocyte Control of Bone Formation via Sclerostin, a Novel BMP Antagonist. EMBO J (2003) 22:6267–76. doi: 10.1093/emboj/cdg599
53. Yan T, Riggs BL, Boyle WJ, Khosla S. Regulation of Osteoclastogenesis and RANK Expression by TGF-Beta1. J Cell Biochem (2001) 83:320–5. doi: 10.1002/jcb.1200
54. Quinn JM, Itoh K, Udagawa N, Hausler K, Yasuda H, Shima N, et al. Transforming Growth Factor Beta Affects Osteoclast Differentiation via Direct and Indirect Actions. J Bone Miner Res (2001) 16:1787–94. doi: 10.1359/jbmr.2001.16.10.1787
55. Kasagi S, Chen W. TGF-Beta1 on Osteoimmunology and the Bone Component Cells. Cell Biosci (2013) 3:4. doi: 10.1186/2045-3701-3-4
56. Lee B, Oh Y, Jo S, Kim TH, Ji JD. A Dual Role of TGF-Beta in Human Osteoclast Differentiation Mediated by Smad1 Versus Smad3 Signaling. Immunol Lett (2019) 206:33–40. doi: 10.1016/j.imlet.2018.12.003
57. Garimella R, Tague SE, Zhang J, Belibi F, Nahar N, Sun BH, et al. Expression and Synthesis of Bone Morphogenetic Proteins by Osteoclasts: A Possible Path to Anabolic Bone Remodeling. J Histochem Cytochem (2008) 56:569–77. doi: 10.1369/jhc.2008.950394
58. Sanchez-Duffhues G, Hiepen C, Knaus P, Ten Dijke P. Bone Morphogenetic Protein Signaling in Bone Homeostasis. Bone (2015) 80:43–59. doi: 10.1016/j.bone.2015.05.025
59. Henriksen K, Karsdal MA, Martin TJ. Osteoclast-Derived Coupling Factors in Bone Remodeling. Calcif Tissue Int (2014) 94:88–97. doi: 10.1007/s00223-013-9741-7
60. Sims NA, Martin TJ. Osteoclasts Provide Coupling Signals to Osteoblast Lineage Cells Through Multiple Mechanisms. Annu Rev Physiol (2020) 82:507–29. doi: 10.1146/annurev-physiol-021119-034425
61. Vaananen HK, Laitala-Leinonen T. Osteoclast Lineage and Function. Arch Biochem Biophys (2008) 473:132–8. doi: 10.1016/j.abb.2008.03.037
62. Martin TJ, Sims NA. Osteoclast-Derived Activity in the Coupling of Bone Formation to Resorption. Trends Mol Med (2005) 11:76–81. doi: 10.1016/j.molmed.2004.12.004
63. Karsdal MA, Martin TJ, Bollerslev J, Christiansen C, Henriksen K. Are Nonresorbing Osteoclasts Sources of Bone Anabolic Activity? J Bone Miner Res (2007) 22:487–94. doi: 10.1359/jbmr.070109
64. Luo G, Sun S, Weng T, Li X, Wang Z, Zhang B. Effect of Osteoclasts on Murine Osteoblastic Differentiation in Early Stage of Co-Culture. Int J Clin Exp Med (2016) 9:1062–72.
65. Lademann F, Hofbauer LC, Rauner M. The Bone Morphogenetic Protein Pathway: The Osteoclastic Perspective. Front Cell Dev Biol (2020) 8:586031. doi: 10.3389/fcell.2020.586031
66. Kamiya N, Shuxian L, Yamaguchi R, Phipps M, Aruwajoye O, Adapala NS, et al. Targeted Disruption of BMP Signaling Through Type IA Receptor (BMPR1A) in Osteocyte Suppresses SOST and RANKL, Leading to Dramatic Increase in Bone Mass, Bone Mineral Density and Mechanical Strength. Bone (2016) 91:53–63. doi: 10.1016/j.bone.2016.07.002
67. Okamoto M, Murai J, Imai Y, Ikegami D, Kamiya N, Kato S, et al. Conditional Deletion of Bmpr1a in Differentiated Osteoclasts Increases Osteoblastic Bone Formation, Increasing Volume of Remodeling Bone in Mice. J Bone Miner Res (2011) 26:2511–22. doi: 10.1002/jbmr.477
68. Tasca A, Astleford K, Blixt NC, Jensen ED, Gopalakrishnan R, Mansky KC. SMAD1/5 Signaling in Osteoclasts Regulates Bone Formation via Coupling Factors. PloS One (2018) 13:e0203404. doi: 10.1371/journal.pone.0203404
69. Abe E, Yamamoto M, Taguchi Y, Lecka-Czernik B, O’Brien CA, Economides AN, et al. Essential Requirement of BMPs-2/4 for Both Osteoblast and Osteoclast Formation in Murine Bone Marrow Cultures From Adult Mice: Antagonism by Noggin. J Bone Miner Res (2000) 15:663–73. doi: 10.1359/jbmr.2000.15.4.663
70. Cao X, Chen D. The BMP Signaling and In Vivo Bone Formation. Gene (2005) 357:1–8. doi: 10.1016/j.gene.2005.06.017
71. Beederman M, Lamplot JD, Nan G, Wang J, Liu X, Yin L, et al. BMP Signaling in Mesenchymal Stem Cell Differentiation and Bone Formation. J BioMed Sci Eng (2013) 6:32–52. doi: 10.4236/jbise.2013.68A1004
72. Zhang H, Bradley A. Mice Deficient for BMP2 are Nonviable and Have Defects in Amnion/Chorion and Cardiac Development. Development (1996) 122:2977–86. doi: 10.1242/dev.122.10.2977
73. Shu B, Zhang M, Xie R, Wang M, Jin H, Hou W, et al. BMP2, But Not BMP4, is Crucial for Chondrocyte Proliferation and Maturation During Endochondral Bone Development. J Cell Sci (2011) 124:3428–40. doi: 10.1242/jcs.083659
74. Khan MP, Khan K, Yadav PS, Singh AK, Nag A, Prasahar P, et al. BMP Signaling is Required for Adult Skeletal Homeostasis and Mediates Bone Anabolic Action of Parathyroid Hormone. Bone (2016) 92:132–44. doi: 10.1016/j.bone.2016.08.018
75. Winnier G, Blessing M, Labosky PA, Hogan BL. Bone Morphogenetic Protein-4 is Required for Mesoderm Formation and Patterning in the Mouse. Genes Dev (1995) 9:2105–16. doi: 10.1101/gad.9.17.2105
76. King JA, Marker PC, Seung KJ, Kingsley DM. BMP5 and the Molecular, Skeletal, and Soft-Tissue Alterations in Short Ear Mice. Dev Biol (1994) 166:112–22. doi: 10.1006/dbio.1994.1300
77. Solloway MJ, Dudley AT, Bikoff EK, Lyons KM, Hogan BL, Robertson EJ. Mice Lacking Bmp6 Function. Dev Genet (1998) 22:321–39. doi: 10.1002/(SICI)1520-6408(1998)22:4<321::AID-DVG3>3.0.CO;2-8
78. Luo G, Hofmann C, Bronckers AL, Sohocki M, Bradley A, Karsenty G. BMP-7 is an Inducer of Nephrogenesis, and is Also Required for Eye Development and Skeletal Patterning. Genes Dev (1995) 9:2808–20. doi: 10.1101/gad.9.22.2808
79. Dudley AT, Lyons KM, Robertson EJ. A Requirement for Bone Morphogenetic Protein-7 During Development of the Mammalian Kidney and Eye. Genes Dev (1995) 9:2795–807. doi: 10.1101/gad.9.22.2795
80. Mishina Y, Kamiya N. Embryonic Skeletogenesis and Craniofacial Development. In: Vukicevic S, Sampath TK, editors. Bone Morphogenetic Proteins: Systems Biology Regulators. Cham: Springer (2017). p. 39–72.
81. Bandyopadhyay A, Tsuji K, Cox K, Harfe BD, Rosen V, Tabin CJ. Genetic Analysis of the Roles of BMP2, BMP4, and BMP7 in Limb Patterning and Skeletogenesis. PloS Genet (2006) 2:e216. doi: 10.1371/journal.pgen.0020216
82. Okamoto M, Murai J, Yoshikawa H, Tsumaki N. Bone Morphogenetic Proteins in Bone Stimulate Osteoclasts and Osteoblasts During Bone Development. J Bone Miner Res (2006) 21:1022–33. doi: 10.1359/jbmr.060411
83. Vukicevic S, Oppermann H, Verbanac D, Jankolija M, Popek I, Curak J, et al. The Clinical Use of Bone Morphogenetic Proteins Revisited: A Novel Biocompatible Carrier Device OSTEOGROW for Bone Healing. Int Orthop (2014) 38:635–47. doi: 10.1007/s00264-013-2201-1
84. Mishina Y, Suzuki A, Ueno N, Behringer RR. Bmpr Encodes a Type I Bone Morphogenetic Protein Receptor That is Essential for Gastrulation During Mouse Embryogenesis. Genes Dev (1995) 9:3027–37. doi: 10.1101/gad.9.24.3027
85. Salazar VS, Gamer LW, Rosen V. BMP Signalling in Skeletal Development, Disease and Repair. Nat Rev Endocrinol (2016) 12:203–21. doi: 10.1038/nrendo.2016.12
86. ten Dijke P. Bone Morphogenetic Protein Signal Transduction in Bone. Curr Med Res Opin (2006) 22 Suppl:1:S7–11. doi: 10.1185/030079906X80576
87. Miyazono K, Kamiya Y, Morikawa M. Bone Morphogenetic Protein Receptors and Signal Transduction. J Biochem (2010) 147:35–51. doi: 10.1093/jb/mvp148
88. Lowery JW, Rosen V. The BMP Pathway and Its Inhibitors in the Skeleton. Physiol Rev (2018) 98:2431–52. doi: 10.1152/physrev.00028.2017
89. Chen G, Deng C, Li YP. TGF-Beta and BMP Signaling in Osteoblast Differentiation and Bone Formation. Int J Biol Sci (2012) 8:272–88. doi: 10.7150/ijbs.2929
90. Pountos I, Georgouli T, Henshaw K, Bird H, Jones E, Giannoudis PV. The Effect of Bone Morphogenetic Protein-2, Bone Morphogenetic Protein-7, Parathyroid Hormone, and Platelet-Derived Growth Factor on the Proliferation and Osteogenic Differentiation of Mesenchymal Stem Cells Derived From Osteoporotic Bone. J Orthop Trauma (2010) 24:552–6. doi: 10.1097/BOT.0b013e3181efa8fe
91. Kobayashi T, Lyons KM, McMahon AP, Kronenberg HM. BMP Signaling Stimulates Cellular Differentiation at Multiple Steps During Cartilage Development. Proc Natl Acad Sci USA (2005) 102:18023–7. doi: 10.1073/pnas.0503617102
92. Heubel B, Nohe A. The Role of BMP Signaling in Osteoclast Regulation. J Dev Biol (2021) 9:24–41. doi: 10.3390/jdb9030024
93. Lin GL, Hankenson KD. Integration of BMP, Wnt, and Notch Signaling Pathways in Osteoblast Differentiation. J Cell Biochem (2011) 112:3491–501. doi: 10.1002/jcb.23287
94. Zimmerman LB, De Jesus-Escobar JM, Harland RM. The Spemann Organizer Signal Noggin Binds and Inactivates Bone Morphogenetic Protein 4. Cell (1996) 86:599–606. doi: 10.1016/s0092-8674(00)80133-6
95. Poynton AR, Lane JM. Safety Profile for the Clinical Use of Bone Morphogenetic Proteins in the Spine. Spine (Phila Pa 1976) (2002) 27:S40–8. doi: 10.1097/00007632-200208151-00010
96. Migliorini E, Guevara-Garcia A, Albiges-Rizo C, Picart C. Learning From BMPs and Their Biophysical Extracellular Matrix Microenvironment for Biomaterial Design. Bone (2020) 141:115540. doi: 10.1016/j.bone.2020.115540
97. Paralkar VM, Vukicevic S, Reddi AH. Transforming Growth Factor Beta Type 1 Binds to Collagen IV of Basement Membrane Matrix: Implications for Development. Dev Biol (1991) 143:303–8. doi: 10.1016/0012-1606(91)90081-d
98. Paralkar VM, Weeks BS, Yu YM, Kleinman HK, Reddi AH. Recombinant Human Bone Morphogenetic Protein 2B Stimulates PC12 Cell Differentiation: Potentiation and Binding to Type IV Collagen. J Cell Biol (1992) 119:1721–8. doi: 10.1083/jcb.119.6.1721
99. Sedlmeier G, Sleeman JP. Extracellular Regulation of BMP Signaling: Welcome to the Matrix. Biochem Soc Trans (2017) 45:173–81. doi: 10.1042/BST20160263
100. Umulis D, O’Connor MB, Blair SS. The Extracellular Regulation of Bone Morphogenetic Protein Signaling. Development (2009) 136:3715–28. doi: 10.1242/dev.031534
101. McCullough KA, Waits CA, Garimella R, Tague SE, Sipe JB, Anderson HC. Immunohistochemical Localization of Bone Morphogenetic Proteins (BMPs) 2, 4, 6, and 7 During Induced Heterotopic Bone Formation. J Orthop Res (2007) 25:465–72. doi: 10.1002/jor.20340
102. Broege A, Pham L, Jensen ED, Emery A, Huang TH, Stemig M, et al. Bone Morphogenetic Proteins Signal via SMAD and Mitogen-Activated Protein (MAP) Kinase Pathways at Distinct Times During Osteoclastogenesis. J Biol Chem (2013) 288:37230–40. doi: 10.1074/jbc.M113.496950
103. Jensen ED, Pham L, Billington CJ Jr., Espe K, Carlson AE, Westendorf JJ, et al. Bone Morphogenic Protein 2 Directly Enhances Differentiation of Murine Osteoclast Precursors. J Cell Biochem (2010) 109:672–82. doi: 10.1002/jcb.22462
104. Kaneko H, Arakawa T, Mano H, Kaneda T, Ogasawara A, Nakagawa M, et al. Direct Stimulation of Osteoclastic Bone Resorption by Bone Morphogenetic Protein (BMP)-2 and Expression of BMP Receptors in Mature Osteoclasts. Bone (2000) 27:479–86. doi: 10.1016/s8756-3282(00)00358-6
105. Itoh K, Udagawa N, Katagiri T, Iemura S, Ueno N, Yasuda H, et al. Bone Morphogenetic Protein 2 Stimulates Osteoclast Differentiation and Survival Supported by Receptor Activator of Nuclear factor-kappaB Ligand. Endocrinology (2001) 142:3656–62. doi: 10.1210/endo.142.8.8300
106. Kanatani M, Sugimoto T, Kaji H, Kobayashi T, Nishiyama K, Fukase M, et al. Stimulatory Effect of Bone Morphogenetic Protein-2 on Osteoclast-Like Cell Formation and Bone-Resorbing Activity. J Bone Miner Res (1995) 10:1681–90. doi: 10.1002/jbmr.5650101110
107. Koide M, Murase Y, Yamato K, Noguchi T, Okahashi N, Nishihara T. Bone Morphogenetic Protein-2 Enhances Osteoclast Formation Mediated by Interleukin-1alpha Through Upregulation of Osteoclast Differentiation Factor and Cyclooxygenase-2. Biochem Biophys Res Commun (1999) 259:97–102. doi: 10.1006/bbrc.1999.0715
108. Wutzl A, Brozek W, Lernbass I, Rauner M, Hofbauer G, Schopper C, et al. Bone Morphogenetic Proteins 5 and 6 Stimulate Osteoclast Generation. J BioMed Mater Res A (2006) 77:75–83. doi: 10.1002/jbm.a.30615
109. Paul S, Lee JC, Yeh LC. A Comparative Study on BMP-Induced Osteoclastogenesis and Osteoblastogenesis in Primary Cultures of Adult Rat Bone Marrow Cells. Growth Factors (2009) 27:121–31. doi: 10.1080/08977190802707324
110. Simic P, Culej JB, Orlic I, Grgurevic L, Draca N, Spaventi R, et al. Systemically Administered Bone Morphogenetic Protein-6 Restores Bone in Aged Ovariectomized Rats by Increasing Bone Formation and Suppressing Bone Resorption. J Biol Chem (2006) 281:25509–21. doi: 10.1074/jbc.M513276200
111. Pederson L, Ruan M, Westendorf JJ, Khosla S, Oursler MJ. Regulation of Bone Formation by Osteoclasts Involves Wnt/BMP Signaling and the Chemokine Sphingosine-1-Phosphate. Proc Natl Acad Sci USA (2008) 105:20764–9. doi: 10.1073/pnas.0805133106
112. Hentunen TA, Lakkakorpi PT, Tuukkanen J, Lehenkari PP, Sampath TK, Vaananen HK. Effects of Recombinant Human Osteogenic Protein-1 on the Differentiation of Osteoclast-Like Cells and Bone Resorption. Biochem Biophys Res Commun (1995) 209:433–43. doi: 10.1006/bbrc.1995.1521
113. Maurer T, Zimmermann G, Maurer S, Stegmaier S, Wagner C, Hansch GM. Inhibition of Osteoclast Generation: A Novel Function of the Bone Morphogenetic Protein 7/Osteogenic Protein 1. Mediators Inflamm (2012) 2012:171209. doi: 10.1155/2012/171209
114. Li H, Zhao D, Wang S, Ding J, Zhao L. Bone Morphogenetic Protein9 Promotes the Differentiation of Mouse Spleen Macrophages Into Osteoclasts via the ALK1 Receptor and ERK 1/2 Pathways In Vitro. Mol Med Rep (2016) 14:4545–50. doi: 10.3892/mmr.2016.5803
115. Fong D, Bisson M, Laberge G, McManus S, Grenier G, Faucheux N, et al. Bone Morphogenetic Protein-9 Activates Smad and ERK Pathways and Supports Human Osteoclast Function and Survival In Vitro. Cell Signal (2013) 25:717–28. doi: 10.1016/j.cellsig.2012.12.003
116. Zhou YM, Yang YY, Jing YX, Yuan TJ, Sun LH, Tao B, et al. BMP9 Reduces Bone Loss in Ovariectomized Mice by Dual Regulation of Bone Remodeling. J Bone Miner Res (2020) 35:978–93. doi: 10.1002/jbmr.3957
117. Giannoudis PV, Kanakaris NK, Einhorn TA. Interaction of Bone Morphogenetic Proteins With Cells of the Osteoclast Lineage: Review of the Existing Evidence. Osteoporos Int (2007) 18:1565–81. doi: 10.1007/s00198-007-0441-x
118. Granholm S, Henning P, Lindholm C, Lerner UH. Osteoclast Progenitor Cells Present in Significant Amounts in Mouse Calvarial Osteoblast Isolations and Osteoclastogenesis Increased by BMP-2. Bone (2013) 52:83–92. doi: 10.1016/j.bone.2012.09.019
119. Tachi K, Takami M, Zhao B, Mochizuki A, Yamada A, Miyamoto Y, et al. Bone Morphogenetic Protein 2 Enhances Mouse Osteoclast Differentiation via Increased Levels of Receptor Activator of NF-kappaB Ligand Expression in Osteoblasts. Cell Tissue Res (2010) 342:213–20. doi: 10.1007/s00441-010-1052-y
120. Xu Y, Yang Y, Hua Z, Li S, Yang Z, Liu Q, et al. BMP2 Immune Complexes Promote New Bone Formation by Facilitating the Direct Contact Between Osteoclasts and Osteoblasts. Biomaterials (2021) 275:120890. doi: 10.1016/j.biomaterials.2021.120890
121. Westhrin M, Holien T, Zahoor M, Moen SH, Buene G, Stordal B, et al. Bone Morphogenetic Protein 4 Gene Therapy in Mice Inhibits Myeloma Tumor Growth, But Has a Negative Impact on Bone. JBMR Plus (2020) 4:e10247. doi: 10.1002/jbm4.10247
122. Tazoe M, Mogi M, Goto S, Togari A. Involvement of P38map Kinase in Bone Morphogenetic Protein-4-Induced Osteoprotegerin in Mouse Bone-Marrow-Derived Stromal Cells. Arch Oral Biol (2003) 48:615–9. doi: 10.1016/s0003-9969(03)00100-6
123. Mishina Y, Starbuck MW, Gentile MA, Fukuda T, Kasparcova V, Seedor JG, et al. Bone Morphogenetic Protein Type IA Receptor Signaling Regulates Postnatal Osteoblast Function and Bone Remodeling. J Biol Chem (2004) 279:27560–6. doi: 10.1074/jbc.M404222200
124. Spector JA, Luchs JS, Mehrara BJ, Greenwald JA, Smith LP, Longaker MT. Expression of Bone Morphogenetic Proteins During Membranous Bone Healing. Plast Reconstr Surg (2001) 107:124–34. doi: 10.1097/00006534-200101000-00018
125. Wutzl A, Rauner M, Seemann R, Millesi W, Krepler P, Pietschmann P, et al. Bone Morphogenetic Proteins 2, 5, and 6 in Combination Stimulate Osteoblasts But Not Osteoclasts In Vitro. J Orthop Res (2010) 28:1431–9. doi: 10.1002/jor.21144
126. Friedman MS, Long MW, Hankenson KD. Osteogenic Differentiation of Human Mesenchymal Stem Cells is Regulated by Bone Morphogenetic Protein-6. J Cell Biochem (2006) 98:538–54. doi: 10.1002/jcb.20719
127. Song K, Krause C, Shi S, Patterson M, Suto R, Grgurevic L, et al. Identification of a Key Residue Mediating Bone Morphogenetic Protein (BMP)-6 Resistance to Noggin Inhibition Allows for Engineered BMPs With Superior Agonist Activity. J Biol Chem (2010) 285:12169–80. doi: 10.1074/jbc.M109.087197
128. Vukicevic S, Grgurevic L. BMP-6 and Mesenchymal Stem Cell Differentiation. Cytokine Growth Factor Rev (2009) 20:441–8. doi: 10.1016/j.cytogfr.2009.10.020
129. Andriopoulos B Jr., Corradini E, Xia Y, Faasse SA, Chen S, Grgurevic L, et al. BMP6 is a Key Endogenous Regulator of Hepcidin Expression and Iron Metabolism. Nat Genet (2009) 41:482–7. doi: 10.1038/ng.335
130. Vukicevic S, Grgurevic L, Erjavec I, Pecin M, Bordukalo-Niksic T, Stokovic N, et al. Autologous Blood Coagulum is a Physiological Carrier for BMP6 to Induce New Bone Formation and Promote Posterolateral Lumbar Spine Fusion in Rabbits. J Tissue Eng Regener Med (2020) 14:147–59. doi: 10.1002/term.2981
131. Sampath TK, Vukicevic S. Biology of Bone Morphogenetic Protein in Bone Repair and Regeneration: A Role for Autologous Blood Coagulum as Carrier. Bone (2020) 141:115602. doi: 10.1016/j.bone.2020.115602
132. White AP, Vaccaro AR, Hall JA, Whang PG, Friel BC, McKee MD. Clinical Applications of BMP-7/OP-1 in Fractures, Nonunions and Spinal Fusion. Int Orthop (2007) 31:735–41. doi: 10.1007/s00264-007-0422-x
133. Ekrol I, Hajducka C, Court-Brown C. McQueen MM. A Comparison of RhBMP-7 (OP-1) and Autogenous Graft for Metaphyseal Defects After Osteotomy of the Distal Radius. Injury (2008) 39 Suppl 2:S73–82. doi: 10.1016/S0020-1383(08)70018-4
134. Laursen M, Hoy K, Hansen ES, Gelineck J, Christensen FB, Bunger CE. Recombinant Bone Morphogenetic Protein-7 as an Intracorporal Bone Growth Stimulator in Unstable Thoracolumbar Burst Fractures in Humans: Preliminary Results. Eur Spine J (1999) 8:485–90. doi: 10.1007/s005860050210
135. Omi M, Kaartinen V, Mishina Y. Activin A Receptor Type 1-Mediated BMP Signaling Regulates RANKL-Induced Osteoclastogenesis via Canonical SMAD-Signaling Pathway. J Biol Chem (2019) 294:17818–36. doi: 10.1074/jbc.RA119.009521
136. DiLeone RJ, King JA, Storm EE, Copeland NG, Jenkins NA, Kingsley DM. The Bmp8 Gene is Expressed in Developing Skeletal Tissue and Maps Near the Achondroplasia Locus on Mouse Chromosome 4. Genomics (1997) 40:196–8. doi: 10.1006/geno.1996.4533
137. Zhang L, Luo Q, Shu Y, Zeng Z, Huang B, Feng Y, et al. Transcriptomic Landscape Regulated by the 14 Types of Bone Morphogenetic Proteins (BMPs) in Lineage Commitment and Differentiation of Mesenchymal Stem Cells (MSCs). Genes Dis (2019) 6:258–75. doi: 10.1016/j.gendis.2019.03.008
138. Kosa JP, Kis A, Bacsi K, Balla B, Nagy Z, Takacs I, et al. The Protective Role of Bone Morphogenetic Protein-8 in the Glucocorticoid-Induced Apoptosis on Bone Cells. Bone (2011) 48:1052–7. doi: 10.1016/j.bone.2011.01.017
139. ten Dijke P, Yamashita H, Sampath TK, Reddi AH, Estevez M, Riddle DL, et al. Identification of Type I Receptors for Osteogenic Protein-1 and Bone Morphogenetic Protein-4. J Biol Chem (1994) 269:16985–8. doi: 10.1016/S0021-9258(17)32506-1
140. Newfeld SJ, Chartoff EH, Graff JM, Melton DA, Gelbart WM. Mothers Against Dpp Encodes a Conserved Cytoplasmic Protein Required in DPP/TGF-Beta Responsive Cells. Development (1996) 122:2099–108. doi: 10.1242/dev.122.7.2099
141. Trompouki E, Bowman TV, Lawton LN, Fan ZP, Wu DC, DiBiase A, et al. Lineage Regulators Direct BMP and Wnt Pathways to Cell-Specific Programs During Differentiation and Regeneration. Cell (2011) 147:577–89. doi: 10.1016/j.cell.2011.09.044
142. Miao X, Yuan J, Wu J, Zheng J, Zheng W, Wang F, et al. Bone Morphogenetic Protein-2 Promotes Osteoclasts-Mediated Osteolysis via Smad1 and P65 Signaling Pathways. Spine (Phila Pa 1976) (2021) 46:E234–E42. doi: 10.1097/BRS.0000000000003770
143. Tasca A, Stemig M, Broege A, Huang B, Davydova J, Zwijsen A, et al. Smad1/5 and Smad4 Expression are Important for Osteoclast Differentiation. J Cell Biochem (2015) 116:1350–60. doi: 10.1002/jcb.25092
144. Morita M, Yoshida S, Iwasaki R, Yasui T, Sato Y, Kobayashi T, et al. Smad4 is Required to Inhibit Osteoclastogenesis and Maintain Bone Mass. Sci Rep (2016) 6:35221. doi: 10.1038/srep35221
145. Li A, Cong Q, Xia X, Leong WF, Yeh J, Miao D, et al. Pharmacologic Calcitriol Inhibits Osteoclast Lineage Commitment via the BMP-Smad1 and IkappaB-NF-kappaB Pathways. J Bone Miner Res (2017) 32:1406–20. doi: 10.1002/jbmr.3146
146. Courtial N, Smink JJ, Kuvardina ON, Leutz A, Gothert JR, Lausen J. Tal1 Regulates Osteoclast Differentiation Through Suppression of the Master Regulator of Cell Fusion DC-STAMP. FASEB J (2012) 26:523–32. doi: 10.1096/fj.11-190850
147. Mandal CC, Das F, Ganapathy S, Harris SE, Choudhury GG, Ghosh-Choudhury N. Bone Morphogenetic Protein-2 (BMP-2) Activates NFATc1 Transcription Factor via an Autoregulatory Loop Involving Smad/Akt/Ca2+ Signaling. J Biol Chem (2016) 291:1148–61. doi: 10.1074/jbc.M115.668939
148. Shi C, Iura A, Terajima M, Liu F, Lyons K, Pan H, et al. Deletion of BMP Receptor Type IB Decreased Bone Mass in Association With Compromised Osteoblastic Differentiation of Bone Marrow Mesenchymal Progenitors. Sci Rep (2016) 6:24256. doi: 10.1038/srep24256
149. Sanchez-Duffhues G, Williams E, Goumans MJ, Heldin CH, Ten Dijke P. Bone Morphogenetic Protein Receptors: Structure, Function and Targeting by Selective Small Molecule Kinase Inhibitors. Bone (2020) 138:115472. doi: 10.1016/j.bone.2020.115472
150. Qi B, Cong Q, Li P, Ma G, Guo X, Yeh J, et al. Ablation of Tak1 in Osteoclast Progenitor Leads to Defects in Skeletal Growth and Bone Remodeling in Mice. Sci Rep (2014) 4:7158. doi: 10.1038/srep07158
151. Yamaguchi K, Nagai S, Ninomiya-Tsuji J, Nishita M, Tamai K, Irie K, et al. XIAP, a Cellular Member of the Inhibitor of Apoptosis Protein Family, Links the Receptors to TAB1-TAK1 in the BMP Signaling Pathway. EMBO J (1999) 18:179–87. doi: 10.1093/emboj/18.1.179
152. Shibuya H, Iwata H, Masuyama N, Gotoh Y, Yamaguchi K, Irie K, et al. Role of TAK1 and TAB1 in BMP Signaling in Early Xenopus Development. EMBO J (1998) 17:1019–28. doi: 10.1093/emboj/17.4.1019
153. Lukac N, Katavic V, Novak S, Sucur A, Filipovic M, Kalajzic I, et al. What do We Know About Bone Morphogenetic Proteins and Osteochondroprogenitors in Inflammatory Conditions? Bone (2020) 137:115403. doi: 10.1016/j.bone.2020.115403
154. Gerstenfeld LC, Cho TJ, Kon T, Aizawa T, Tsay A, Fitch J, et al. Impaired Fracture Healing in the Absence of TNF-Alpha Signaling: The Role of TNF-Alpha in Endochondral Cartilage Resorption. J Bone Miner Res (2003) 18:1584–92. doi: 10.1359/jbmr.2003.18.9.1584
155. Yang X, Ricciardi BF, Hernandez-Soria A, Shi Y, Pleshko Camacho N, Bostrom MP. Callus Mineralization and Maturation are Delayed During Fracture Healing in Interleukin-6 Knockout Mice. Bone (2007) 41:928–36. doi: 10.1016/j.bone.2007.07.022
156. Colnot C. Skeletal Cell Fate Decisions Within Periosteum and Bone Marrow During Bone Regeneration. J Bone Miner Res (2009) 24:274–82. doi: 10.1359/jbmr.081003
157. Varas A, Valencia J, Lavocat F, Martinez VG, Thiam NN, Hidalgo L, et al. Blockade of Bone Morphogenetic Protein Signaling Potentiates the Pro-Inflammatory Phenotype Induced by Interleukin-17 and Tumor Necrosis Factor-Alpha Combination in Rheumatoid Synoviocytes. Arthritis Res Ther (2015) 17:192. doi: 10.1186/s13075-015-0710-6
158. Baum R, Gravallese EM. Bone as a Target Organ in Rheumatic Disease: Impact on Osteoclasts and Osteoblasts. Clin Rev Allergy Immunol (2016) 51:1–15. doi: 10.1007/s12016-015-8515-6
159. Wu DH, Hatzopoulos AK. Bone Morphogenetic Protein Signaling in Inflammation. Exp Biol Med (Maywood) (2019) 244:147–56. doi: 10.1177/1535370219828694
160. Lories RJ, Derese I, Luyten FP. Modulation of Bone Morphogenetic Protein Signaling Inhibits the Onset and Progression of Ankylosing Enthesitis. J Clin Invest (2005) 115:1571–9. doi: 10.1172/JCI23738
161. Hattersley G, Chambers TJ. Effects of Transforming Growth Factor Beta 1 on the Regulation of Osteoclastic Development and Function. J Bone Miner Res (1991) 6:165–72. doi: 10.1002/jbmr.5650060210
162. Ehnert S, Baur J, Schmitt A, Neumaier M, Lucke M, Dooley S, et al. TGF-Beta1 as Possible Link Between Loss of Bone Mineral Density and Chronic Inflammation. PloS One (2010) 5:e14073. doi: 10.1371/journal.pone.0014073
163. Wei S, Kitaura H, Zhou P, Ross FP, Teitelbaum SL. IL-1 Mediates TNF-Induced Osteoclastogenesis. J Clin Invest (2005) 115:282–90. doi: 10.1172/JCI23394
164. Jimi E, Nakamura I, Duong LT, Ikebe T, Takahashi N, Rodan GA, et al. Interleukin 1 Induces Multinucleation and Bone-Resorbing Activity of Osteoclasts in the Absence of Osteoblasts/Stromal Cells. Exp Cell Res (1999) 247:84–93. doi: 10.1006/excr.1998.4320
165. Gilbert L, He X, Farmer P, Boden S, Kozlowski M, Rubin J, et al. Inhibition of Osteoblast Differentiation by Tumor Necrosis Factor-Alpha. Endocrinology (2000) 141:3956–64. doi: 10.1210/endo.141.11.7739
166. Hengartner NE, Fiedler J, Ignatius A, Brenner RE. IL-1beta Inhibits Human Osteoblast Migration. Mol Med (2013) 19:36–42. doi: 10.2119/molmed.2012.00058
167. Grgurevic L, Christensen GL, Schulz TJ, Vukicevic S. Bone Morphogenetic Proteins in Inflammation, Glucose Homeostasis and Adipose Tissue Energy Metabolism. Cytokine Growth Factor Rev (2016) 27:105–18. doi: 10.1016/j.cytogfr.2015.12.009
168. Tsumaki N, Yoshikawa H. The Role of Bone Morphogenetic Proteins in Endochondral Bone Formation. Cytokine Growth Factor Rev (2005) 16:279–85. doi: 10.1016/j.cytogfr.2005.04.001
169. Bishop GB, Einhorn TA. Current and Future Clinical Applications of Bone Morphogenetic Proteins in Orthopaedic Trauma Surgery. Int Orthop (2007) 31:721–7. doi: 10.1007/s00264-007-0424-8
170. Pradhan BB, Bae HW, Dawson EG, Patel VV, Delamarter RB. Graft Resorption With the Use of Bone Morphogenetic Protein: Lessons From Anterior Lumbar Interbody Fusion Using Femoral Ring Allografts and Recombinant Human Bone Morphogenetic Protein-2. Spine (Phila Pa 1976) (2006) 31:E277-84. doi: 10.1097/01.brs.0000216442.12092.01
171. Vaidya R, Weir R, Sethi A, Meisterling S, Hakeos W, Wybo CD. Interbody Fusion With Allograft and rhBMP-2 Leads to Consistent Fusion But Early Subsidence. J Bone Joint Surg Br (2007) 89:342–5. doi: 10.1302/0301-620X.89B3.18270
172. Seeherman HJ, Li XJ, Bouxsein ML, Wozney JM. rhBMP-2 Induces Transient Bone Resorption Followed by Bone Formation in a Nonhuman Primate Core-Defect Model. J Bone Joint Surg Am (2010) 92:411–26. doi: 10.2106/JBJS.H.01732
173. McGee MA, Findlay DM, Howie DW, Carbone A, Ward P, Stamenkov R, et al. The Use of OP-1 in Femoral Impaction Grafting in a Sheep Model. J Orthop Res (2004) 22:1008–15. doi: 10.1016/j.orthres.2004.01.005
174. Fu R, Selph S, McDonagh M, Peterson K, Tiwari A, Chou R, et al. Effectiveness and Harms of Recombinant Human Bone Morphogenetic Protein-2 in Spine Fusion: A Systematic Review and Meta-Analysis. Ann Intern Med (2013) 158:890–902. doi: 10.7326/0003-4819-158-12-201306180-00006
175. Simmonds MC, Brown JV, Heirs MK, Higgins JP, Mannion RJ, Rodgers MA, et al. Safety and Effectiveness of Recombinant Human Bone Morphogenetic Protein-2 for Spinal Fusion: A Meta-Analysis of Individual-Participant Data. Ann Intern Med (2013) 158:877–89. doi: 10.7326/0003-4819-158-12-201306180-00005
176. Kamiya N, Ye L, Kobayashi T, Lucas DJ, Mochida Y, Yamauchi M, et al. Disruption of BMP Signaling in Osteoblasts Through Type IA Receptor (BMPRIA) Increases Bone Mass. J Bone Miner Res (2008) 23:2007–17. doi: 10.1359/jbmr.080809
177. Otsuka E, Notoya M, Hagiwara H. Treatment of Myoblastic C2C12 Cells With BMP-2 Stimulates Vitamin D-Induced Formation of Osteoclasts. Calcif Tissue Int (2003) 73:72–7. doi: 10.1007/s00223-002-1071-0
178. Irie K, Alpaslan C, Takahashi K, Kondo Y, Izumi N, Sakakura Y, et al. Osteoclast Differentiation in Ectopic Bone Formation Induced by Recombinant Human Bone Morphogenetic Protein 2 (rhBMP-2). J Bone Miner Metab (2003) 21:363–9. doi: 10.1007/s00774-003-0430-x
179. Mroz TE, Wang JC, Hashimoto R, Norvell DC. Complications Related to Osteobiologics Use in Spine Surgery: A Systematic Review. Spine (Phila Pa 1976) (2010) 35:S86–104. doi: 10.1097/BRS.0b013e3181d81ef2
180. Hansen SM, Sasso RC. Resorptive Response of Rhbmp2 Simulating Infection in an Anterior Lumbar Interbody Fusion With a Femoral Ring. J Spinal Disord Tech (2006) 19:130–4. doi: 10.1097/01.bsd.0000168512.61351.3a
181. Little DG, McDonald M, Bransford R, Godfrey CB, Amanat N. Manipulation of the Anabolic and Catabolic Responses With OP-1 and Zoledronic Acid in a Rat Critical Defect Model. J Bone Miner Res (2005) 20:2044–52. doi: 10.1359/JBMR.050712
182. Dumic-Cule I, Brkljacic J, Rogic D, Bordukalo Niksic T, Tikvica Luetic A, Draca N, et al. Systemically Available Bone Morphogenetic Protein Two and Seven Affect Bone Metabolism. Int Orthop (2014) 38:1979–85. doi: 10.1007/s00264-014-2425-8
183. Dumic-Cule I, Pecina M, Jelic M, Jankolija M, Popek I, Grgurevic L, et al. Biological Aspects of Segmental Bone Defects Management. Int Orthop (2015) 39:1005–11. doi: 10.1007/s00264-015-2728-4
184. Durdevic D, Vlahovic T, Pehar S, Miklic D, Oppermann H, Bordukalo-Niksic T, et al. A Novel Autologous Bone Graft Substitute Comprised of Rhbmp6 Blood Coagulum as Carrier Tested in a Randomized and Controlled Phase I Trial in Patients With Distal Radial Fractures. Bone (2020) 140:115551. doi: 10.1016/j.bone.2020.115551
185. Chiari C, Grgurevic L, Bordukalo-Niksic T, Oppermann H, Valentinitsch A, Nemecek E, et al. Recombinant Human BMP6 Applied Within Autologous Blood Coagulum Accelerates Bone Healing: Randomized Controlled Trial in High Tibial Osteotomy Patients. J Bone Miner Res (2020) 35:1893–903. doi: 10.1002/jbmr.4107
Keywords: BMPs, osteoclast differentiation, bone resorption, osteoblast-osteoclast coupling, bone fracture healing, recombinant BMP therapy
Citation: Bordukalo-Nikšić T, Kufner V and Vukičević S (2022) The Role Of BMPs in the Regulation of Osteoclasts Resorption and Bone Remodeling: From Experimental Models to Clinical Applications. Front. Immunol. 13:869422. doi: 10.3389/fimmu.2022.869422
Received: 04 February 2022; Accepted: 28 March 2022;
Published: 26 April 2022.
Edited by:
Cristina Sobacchi, National Research Council (CNR), ItalyReviewed by:
Elena Ambrogini, United States Department of Veterans Affairs, United StatesCopyright © 2022 Bordukalo-Nikšić, Kufner and Vukičević. This is an open-access article distributed under the terms of the Creative Commons Attribution License (CC BY). The use, distribution or reproduction in other forums is permitted, provided the original author(s) and the copyright owner(s) are credited and that the original publication in this journal is cited, in accordance with accepted academic practice. No use, distribution or reproduction is permitted which does not comply with these terms.
*Correspondence: Slobodan Vukičević, c2xvYm9kYW4udnVraWNldmljQG1lZi5ocg==
Disclaimer: All claims expressed in this article are solely those of the authors and do not necessarily represent those of their affiliated organizations, or those of the publisher, the editors and the reviewers. Any product that may be evaluated in this article or claim that may be made by its manufacturer is not guaranteed or endorsed by the publisher.
Research integrity at Frontiers
Learn more about the work of our research integrity team to safeguard the quality of each article we publish.