- 1Irell & Manella Graduate School of Biological Sciences of City of Hope, Duarte, CA, United States
- 2Department of Immuno-Oncology, Beckman Research Institute of City of Hope, Duarte, CA, United States
Background: Epstein-Barr virus (EBV) is the causal agent of infectious mononucleosis and has been associated with various cancers and autoimmune diseases. Despite decades of research efforts to combat this major global health burden, there is no approved prophylactic vaccine against EBV. To facilitate the rational design and assessment of an effective vaccine, we systematically reviewed pre-clinical and clinical prophylactic EBV vaccine studies to determine the antigens, delivery platforms, and animal models used in these studies.
Methods: We searched Cochrane Library, ClinicalTrials.gov, Embase, PubMed, Scopus, Web of Science, WHO’s Global Index Medicus, and Google Scholar from inception to June 20, 2020, for EBV prophylactic vaccine studies focused on humoral immunity.
Results: The search yielded 5,614 unique studies. 36 pre-clinical and 4 clinical studies were included in the analysis after screening against the exclusion criteria. In pre-clinical studies, gp350 was the most commonly used immunogen (33 studies), vaccines were most commonly delivered as monomeric proteins (12 studies), and mice were the most used animal model to test immunogenicity (15 studies). According to an adaptation of the CAMARADES checklist, 4 pre-clinical studies were rated as very high, 5 as high, 13 as moderate quality, 11 as poor, and 3 as very poor. In clinical studies, gp350 was the sole vaccine antigen, delivered in a vaccinia platform (1 study) or as a monomeric protein (3 studies). The present study was registered in PROSPERO (CRD42020198440).
Conclusions: Four major obstacles have prevented the development of an effective prophylactic EBV vaccine: undefined correlates of immune protection, lack of knowledge regarding the ideal EBV antigen(s) for vaccination, lack of an appropriate animal model to test vaccine efficacy, and lack of knowledge regarding the ideal vaccine delivery platform. Our analysis supports a multivalent antigenic approach including two or more of the five main glycoproteins involved in viral entry (gp350, gB, gH/gL, gp42) and a multimeric approach to present these antigens. We anticipate that the application of two underused challenge models, rhesus macaques susceptible to rhesus lymphocryptovirus (an EBV homolog) and common marmosets, will permit the establishment of in vivo correlates of immune protection and attainment of more generalizable data.
Systematic Review Registration: https://www.crd.york.ac.uk/prospero/display_record.php?RecordID=198440, identifier PROSPERO I.D. CRD4202019844.
1 Introduction
In 2011, the U.S. National Institutes of Health held a meeting on Epstein-Barr virus (EBV) that highlighted the urgent need to develop strategies to prevent EBV infection and EBV-associated diseases (1). Indeed, EBV (also known as human herpesvirus 4) has a global infection rate of more than 90%, and each year, it is associated with ~200,000 new cases of lymphoid and epithelial cancers, resulting in ~145,000 deaths world-wide (1, 2). Moreover, it is the causal agent of infectious mononucleosis (IM), leading to more than 125,000 annual cases of IM in the U.S. alone (3), and is associated with the development of various autoimmune disorders (4–6). Nevertheless, more than a decade later, EBV remains without a clinically approved prophylactic vaccine.
EBV was first discovered by Dr. M.A. Epstein, Dr. B.G. Achong, and Dr. Y.M. Barr in Burkitt lymphoma samples from a Ugandan child in 1964 (7). In 1968, it was identified as the causal agent of IM (8). Two years later, it was further identified as the causal agent of nasopharyngeal carcinoma (9, 10). In 1981, EBV was linked to post-transplant lymphoproliferative disorders in renal transplant patients, an association that is now well-established in other solid-organ transplants and hematopoietic stem cell transplants (11, 12). The virus was subsequently linked to two additional lymphomas, Hodgkin lymphoma in 1987 (13) and T-cell lymphoma in 1988 (14), and was later associated with other lymphoid lymphoproliferative disorders, such as natural killer (NK) cell lymphoma, NK/T-cell lymphoma, and NK-cell leukemia (12, 15). The role of EBV infection in the development of some gastric cancers was suggested in the early 1990s (16, 17), and strengthened in more recent studies, including a meta-analysis (18–23). This year, a 20-year a longitudinal study established EBV as the main causal agent of multiple sclerosis (5), with an additional study identifying the EBV protein EBNA1 as a source of cross-reactive antibodies that also target an adhesion molecule expressed in the central nervous system (6), providing a pathological basis for the role of EBV in multiple sclerosis development.
Motivated by the early association of EBV with several human cancers, Dr. Epstein proposed in 1976 the development of a prophylactic vaccine against EBV as a strategy to prevent EBV infection, to prove that EBV is the causal agent of these cancers, and potentially to reduce the burden of EBV-associated cancers (24). Since then, many prophylactic vaccine candidates have been tested in pre-clinical trials and four Phase I/II clinical trials, but to date, none has moved to a Phase III clinical trial.
Neutralizing antibody (nAb) responses correlate with protection in all licensed antiviral prophylactic vaccines (25), including vaccines against other human herpesviruses, such as varicella-zoster virus and herpes-simplex virus 1 (26). In addition, while cellular immunity plays an essential role in controlling EBV replication and re-activation once primary infection has taken place (27–29), it is humoral immunity against viral entry proteins that can prevent primary infection from taking place. Thus, the majority of prophylactic EBV vaccine efforts to date have focused on generating nAbs that can prevent the initiation of viral entry in multiple permissive cell types in vitro (30–32). The attachment protein gp350/220 (gp350, previously known as gp340) and four core fusion glycoproteins—gB, gp42, and the gH/gL complex—are important for EBV entry into both epithelial cells and B cells (Figure 1), the main cellular targets of EBV, making these antigens attractive nAb targets for developing an effective prophylactic vaccine (30). Indeed, nAbs against each of these five EBV glycoproteins have been identified, isolated, and fully characterized for their potency in blocking EBV infection in vitro and, in some cases, in vivo (Table 1). Despite this knowledge and discoveries, it is not known which EBV glycoproteins are required to elicit an effective protective response against primary infection, and the correlates of immune protection against primary EBV infection remain undefined. Furthermore, there is no fully validated EBV challenge animal model in which to test vaccine efficacy and explore correlates of immune protection, and there is a lack of knowledge regarding the ideal vaccine delivery platform to present relevant EBV antigens.
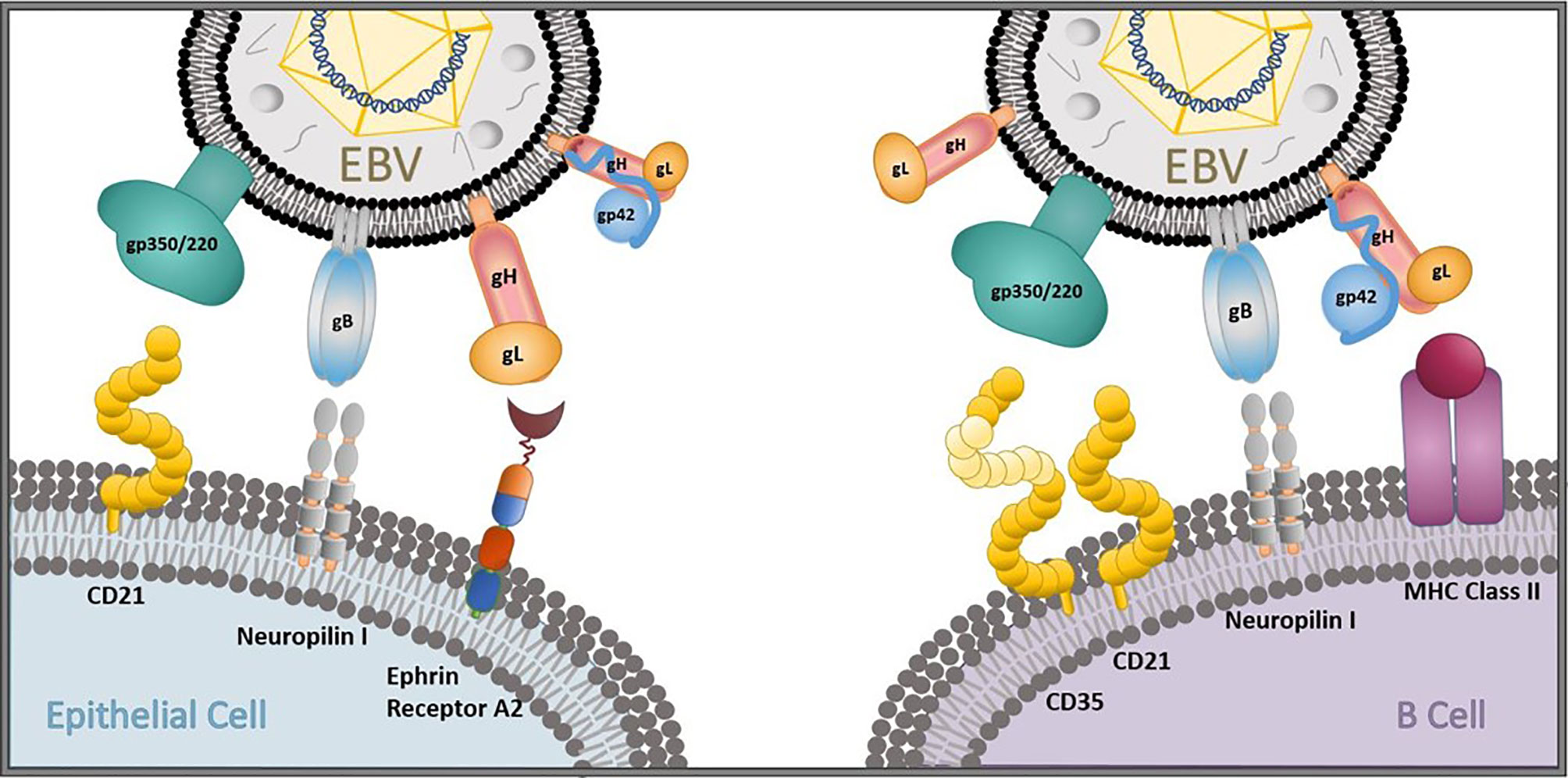
Figure 1 EBV entry and infection of primary target cells. The EBV glycoproteins gp350/220 (gp350), gB, gp42, and the gH/gL complex are important for viral entry into epithelial cells and B cells. gp350 binds to CD21 on epithelial cells and CD35/CD21 on B cells. gB and gH/gL attach to neuropilin 1 and ephrin receptor A2, respectively, on oropharyngeal epithelial cells to gain viral entry, and gB interacts with neuropilin 1 on B cells. gp42 forms a complex with gH/gL that binds to MHC class II to gain viral entry into B cells in collaboration with gB.
To develop a successful prophylactic vaccine against EBV infection, it is necessary to thoroughly investigate all obstacles faced in previous pre-clinical and clinical EBV vaccine candidate studies and guide effective strategies to circumvent them. In this review, we set out to systematically identify all pre-clinical and clinical studies evaluating antibody-based prophylactic EBV vaccine candidates up to June 20, 2020. Specifically, we sought to determine the type and frequency of EBV antigens used in vaccine candidates, the type and frequency of vaccine platforms used (including routes, doses, adjuvants, and immunization schedules), the type of assays used to measure vaccine efficacy, and in the case of pre-clinical studies, the type and frequency of animal/disease models used to test vaccine immune responses. Herein, we report our findings and identify weaknesses in the design of prior pre-clinical vaccine testing studies, including lack of transparency and completeness in reporting methodology. We also provide recommendations to guide the future rational design and evaluation of prophylactic EBV vaccine candidates that can finally be translated to the clinic to reduce the global health burden of EBV-associated diseases.
2 Methods
This systematic review adheres to the Preferred Reporting Items for Systematic Reviews and Meta-Analyses (PRISMA) Statement (Tables S1, S2) (41). Our protocol was registered with the International Prospective Register of Systematic Reviews (PROSPERO; CRD42020198440) in July 2020.
2.1 Search Strategy
We searched the electronic databases of the Cochrane Library, ClinicalTrials.gov, Embase, PubMed, Scopus, Web of Science, the World Health Organization’s Global Index Medicus (WHO-GIM), and Google Scholar for pre-clinical and clinical prophylactic EBV vaccine studies (Table 2). The search was performed with a publication date limit of June 20, 2020, and duplicate articles were automatically removed after electronic comparison across databases (EndNote).
2.2 Selection Criteria and Data Extraction
Articles were excluded if they were not in English or Spanish and if the full text was not available. Selection based on content was performed in two steps: first based on the title and abstract, then by reviewing the full text. In the first step, articles were excluded based on the nature of the article (review/commentary/antibody development study) and the type of targeted immune response (dendritic cell-targeted/T-cell-targeted vaccines). In the second step, pre-clinical studies were excluded based on the nature of the article (conference paper/case study/antibody generation or characterization study/diagnostic or detection study/in silico study/epitope mapping study/immunoglobulin prophylaxis study); the type of targeted immune response (T-cell-targeted vaccines); and lack of data (no in vitro data/no neutralization assessed). Clinical studies were excluded based on the nature of the article (study follow-up) or the type of targeted immune response (therapeutic vaccine). Both selection steps were performed by two independent reviewers (JGO and LZM), and any disagreements were resolved by a third reviewer (GME). A flowchart of this process is presented in Figure 2.
Data extraction was performed by the JGO, GME, LZM, MM and ER. Review of extracted data was performed by JGO and GME, with any disagreements reconciled by LZM. For pre-clinical studies, the following information was extracted: bibliographic information (first author, publication year, title); animal model; characteristics of the population (animal strain, number of animals); characteristics of vaccine (type and source of antigen, dosage, adjuvant, administration route, vaccination schedule); characteristics of control treatments (type of placebo and non-placebo controls, dosage, administration route, vaccination schedule); characteristics of measurement techniques (sample collection schedule, type of antibody detection assay, type of neutralization assay and type of cells used for neutralization assay); and study outcomes. For clinical studies, the following information was extracted: bibliographic information (first author, publication year, title); trial characteristics (country of origin, phase, type, primary endpoint); study population characteristics (age, EBV status, sex, number of participants); characteristics of vaccine and control treatments (type and source of antigen, dosage, adjuvant, administration route, vaccination schedule); characteristics of measurement techniques (sample collection schedule, type of antibody detection assay, type of neutralization assay and type of cells used for neutralization assay, type of EBV diagnostic test); and trial outcomes. Extracted data is presented in Table 3 for pre-clinical studies, and Table 4 for clinical studies. For pre-clinical studies, the author list affiliations were individually checked to determine the country of origin for each study, and a global heat-map was prepared displaying the number of studies performed per country (Figure 3). From the pre-clinical study extracted data, a list was prepared describing the immunogens tested, the type of vaccine delivery platforms used, and the animal models used to test vaccine immunogenicity and efficacy; the number of studies per variable in each category were graphed and are presented in Figures 4A–C.
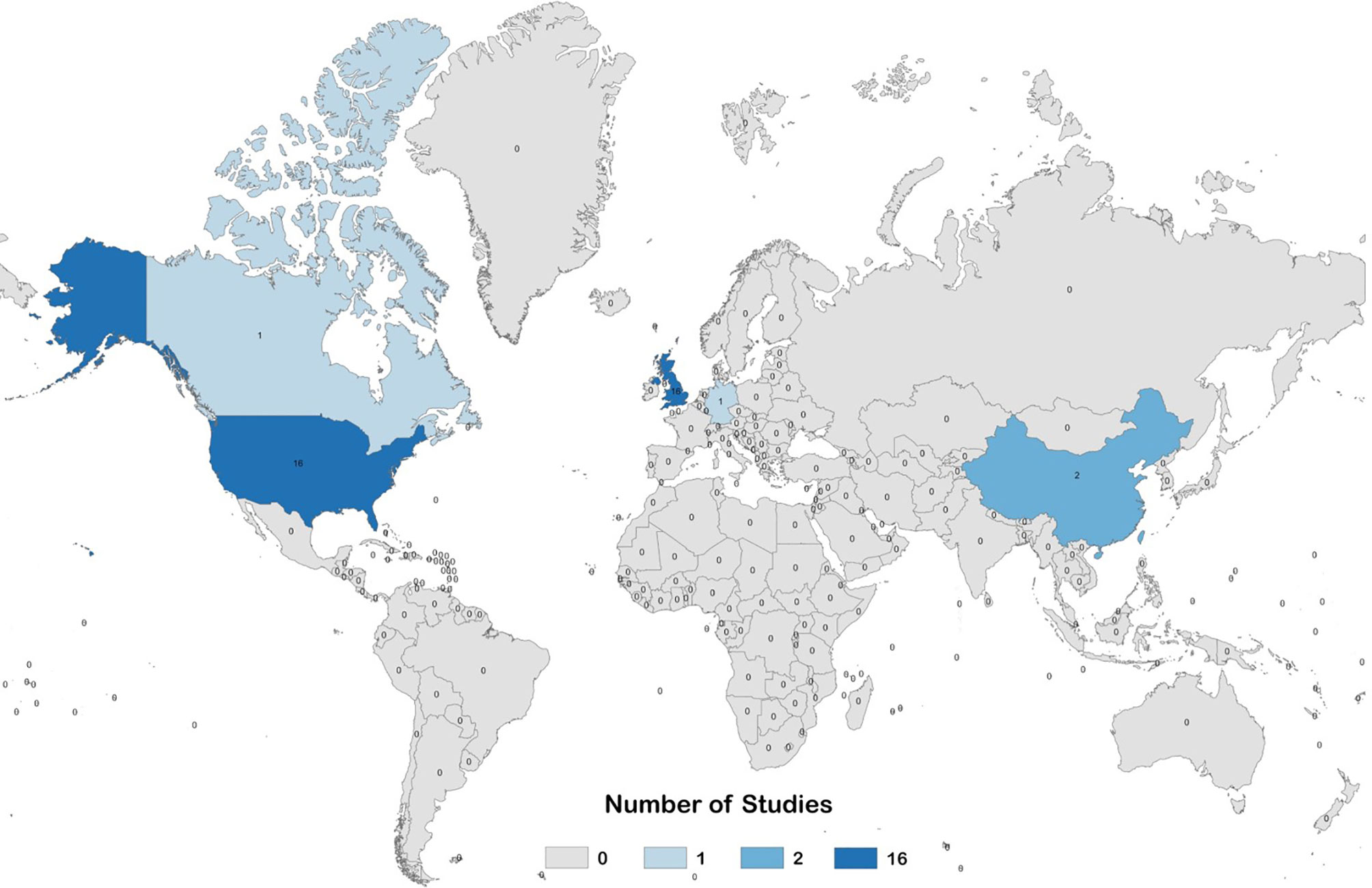
Figure 3 Global heat map describing the number of pre-clinical studies evaluating prophylactic EBV vaccines per country.
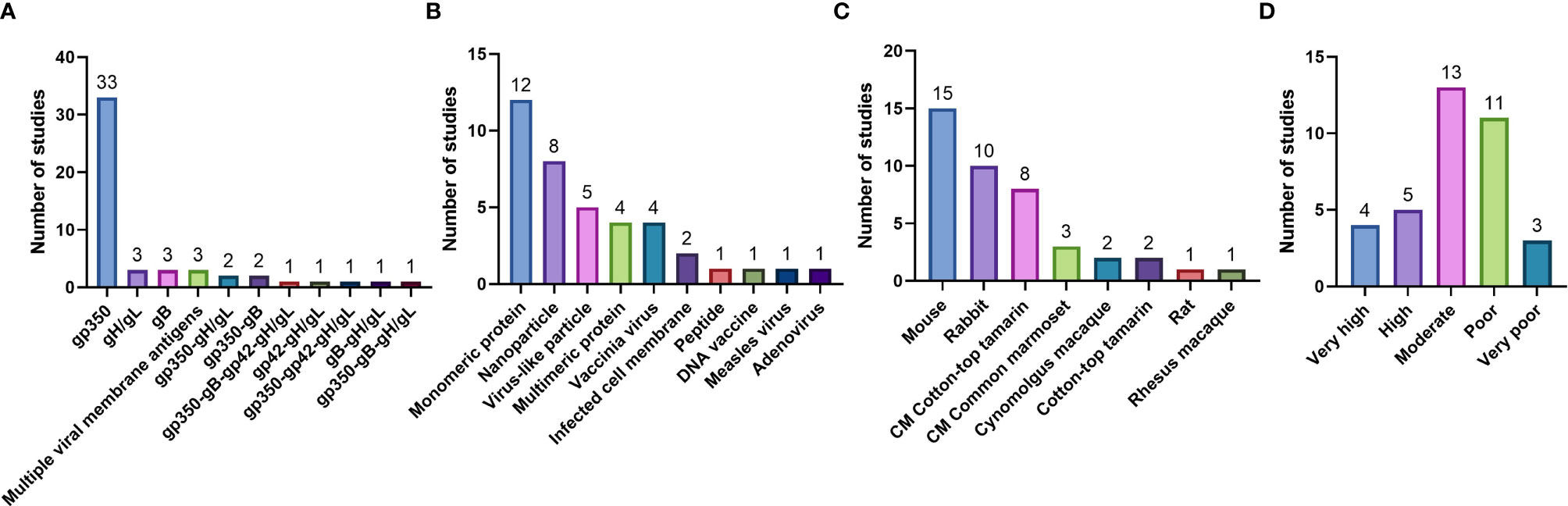
Figure 4 Pre-clinical studies included in the systematic review, enumerated based on (A) Immunogen tested; (B) Type of vaccine delivery platform used; (C) Animal model used to test vaccine immunogenicity and efficacy (CM = challenge model); and (D) Quality assessment.
2.3 Quality Assessment
The quality of all selected pre-clinical studies was assessed using a checklist of 18 items (Table 5) that were modified and expanded from (84), which was developed based on the Collaborative Approach to Meta-Analysis of Animal Data from Experimental Stroke (CAMARADES).
These 18 items were divided into primary (#1-15) and secondary (#16-18) quality criteria. Each primary quality criterion carried a score of one point. In addition, a study was awarded two points for 3/3 of the secondary criteria met, one point for 2/3, and no points for 0-1/3, giving a possible total of 17 points. Because four of the primary criteria (#11-13, #15) did not apply to all studies, studies with total scores of 13 or above were considered very high quality. Studies with scores 10-12 were high quality, 7-9 were moderate quality, 5-8 were poor quality, and 0-4 were very poor quality. Tabulated results for each study analyzed are presented in Table S3, and the overall quality distribution of the studies was graphed and presented in Figure 4D.
2.4 Ethical Statement
No institutional review board approval was required for this study.
3 Results
3.1 Study Selection
Our electronic database search yielded a total of 5,614 unique articles. Of these, 36 pre-clinical studies and 4 clinical studies met the criteria for inclusion in the systematic review (Figure 2). The pre-clinical studies were conducted between 1979 and 2020 (Table 3) and the clinical studies were conducted between 1995 and 2009 (Table 4). Most studies were conducted in the United States and the United Kingdom (Figure 3).
3.2 Description of Included Pre-Clinical Studies
In the 36 pre-clinical studies included in our systematic review, gp350 was the most commonly used individual immunogen, tested in a total of 33 studies (Figure 4A); gH/gL and gB were individually tested in 3 studies each. When multivalent combinations were tested, undefined viral membrane antigens were used in 3 studies, gp350-gH/gL and gp350-gB combinations were used in 2 studies each, and additional antigen combinations were used in 1 study each (Figure 4A). Vaccines were most commonly delivered as monomeric proteins (12 studies); other delivery platforms included nanoparticles (8 studies), virus-like particles (VLPs; 5 studies), multimeric proteins and vaccinia virus (4 studies each), and infected cell membranes (2 studies), as well as peptides, DNA, measles virus, and adenovirus (1 study each) (Figure 4B). Mice were the most commonly used animal for immunogenicity assessments (15 studies), followed by rabbits and cotton-top tamarins (10 studies each), common marmosets (3 studies), and cynomolgus macaques (2 studies), as well as rats and rhesus macaques (1 study each) (Figure 4C). Of these studies, 8 utilized cotton-top tamarins and 3 utilized common marmosets as EBV challenge models (Figure 4C).
Most pre-clinical studies (13) were rated as moderate quality, 11 were rated as poor quality, 5 were rated as high quality, 4 were rated as very high quality, and 3 were rated as very poor quality (Table S3 and Figure 4D).
3.3 Description of Included Clinical Studies
All 4 clinical studies included in our systematic review used gp350 as the clinical immunogen (Table 4). The first study (83), a Phase I study, used vaccinia virus (Tien Tan strain) to deliver the immunogen (Table 4). The second (82), a Phase I/II study, and third (81), a Phase II study, used a monomeric gp350 protein formulation with Adjuvant System 04 (AS04) (Table 4). The most recent study (80), a Phase I study, used a monomeric gp350 protein formulation of the same origin as the others but adjuvanted with alum alone (Table 4). None of these clinical studies led to the prevention of EBV infection/sterile immunity.
4 Discussion
Despite four decades of vaccine research on EBV since its discovery in 1964 (7), there is still no clinically approved prophylactic vaccine against EBV infection. In this systematic review, we sought to examine every pre-clinical and clinical EBV prophylactic vaccine study focused on humoral immunity performed up to June 20, 2020 (36 pre-clinical trials and 4 clinical trials), to provide insight on the four main factors preventing the successful development of an effective EBV prophylactic vaccine (1): undefined correlates of immune protection (2); lack of an appropriate animal model to test vaccine efficacy; (3) lack of knowledge regarding the ideal EBV antigens for vaccination; (4) and lack of knowledge regarding the ideal vaccine delivery platform to present relevant EBV antigens.
4.1 Correlates of Immune Protection Against Primary EBV Infection
The correlates of immune protection against primary EBV infection are not well-defined, partly due to a lack of an appropriate animal model to study EBV infection and test vaccine efficacy, as discussed below in Section 4.2. Thus, pre-clinical vaccine efficacy has been measured primarily using in vitro neutralization assays as a surrogate for in vivo protection. However, in our assessment, we found that there are no clear standards for performing neutralization assays, defining vaccine efficacy, or reporting outcomes.
Before the turn of the century, in vitro neutralization was assessed via transformation assays, in which primary human lymphoid B cells were exposed to EBV previously incubated with immune serum or plasma and then allowed to transform and immortalize over several weeks (Table 3) (85). A reduction in or lack of transformation indicated the presence of nAbs in the serum/plasma sample. The advent of fluorescent recombinant EBV (86–88) allowed the emergence of a simpler and faster method that enables the neutralization assay to be performed on various virus-susceptible cells, including established epithelial and B cell lines—eliminating the required use of primary B cells—with a maximum turnaround of three days after infection. Thus, most pre-clinical studies, beginning with Ruiss et al. in 2011 (56) (Table 3), have utilized a flow cytometry-based assay that was optimized by Sashihara et al. in 2009 to measure infection by quantifying the number of fluorescent cells in a given sample (89). Although these assays can provide invaluable insights, they are no substitute for in vivo studies and cannot provide true correlates of immune protection. Indeed, in the clinical trials analyzed in our study (Table 4), the presence of nAbs could still be identified via in vitro assays in vaccinees who later became EBV-positive. Moreover, we found that there is extensive variability in the type of cell lines used in neutralization assays (e.g., Raji, HEK-293, SVKCR2, Akata, AGS) and in how outcomes are reported (e.g., % neutralization, % infected cells, IC50 titer) (Table 3). We also found that most studies did not provide any information regarding the infectivity of the EBV batch used for the assays (e.g., infectivity curve or virus titer) or the target level of infection used as the negative control for neutralization; based on our assessment, only 14/36 studies appropriately reported virus titer (Table S3). Similarly, we found that only 21/36 studies appropriately reported the immune serum/plasma/antibody concentration or dilution used for neutralization assays (Table S3).
Competitive ELISA against a known nAb has also been used as a surrogate in vitro neutralization assay (Table 3). Although this assay provides important information regarding the types of known neutralizing epitopes a vaccine can target, it serves more as a complementary assay as it does not consider all potential targeted epitopes or any neutralizing mechanisms that can otherwise be probed using the traditional virus-based neutralization assay. Therefore, our review demonstrates a need to establish clear in vitro EBV neutralization protocols, reporting standards, and guidelines so that universal surrogate correlates of immune protection can be compared across in vitro efficacy studies. Moving forward, we propose that all studies perform virus-based neutralization assays using a full panel of established EBV-susceptible cell lines, when possible, especially if the vaccine tested targets multiple antigens that might be important for the infection of different cell types. In addition, viral titer information for the EBV batch used and the serum/plasma/antibody concentration in which the virus is incubated should be clearly reported; otherwise, an accurate assessment of vaccine efficacy and immune protection correlates is not possible.
Most studies in our analysis focused on assessing serum IgG responses, but it is important to note that mucosal IgA responses might also be a relevant source of protective nAbs, given that EBV is transmitted via the oral mucosa. In our assessment, we found that only 1/36 of the pre-clinical studies, and none of the clinical studies, assessed vaccine-induced IgA responses. In the one study that did assess IgA responses, these responses were measured in the serum [(45), Table 3]. A recent study assessing the presence of EBV glycoprotein-specific antibodies in patients with EBV-associated nasopharyngeal carcinoma, found that nAb activity in both epithelial and B cells was most strongly correlated with glycoprotein-specific IgGs rather than IgAs in the serum. However, IgA-related nAb activity might be most relevant in the saliva, as saliva is the first line of defense against EBV once the virus enters the oral mucosa. Since IgGs can also be found in saliva, both salivary IgAs and IgGs might be important to provide protection at the site of EBV infection (90). A recent study using the EBV-homologue rhesus lymphocryptovirus (rhLCV) model (described in Section 4.2 below) showed that oral transfer of glycoprotein-specific monoclonal IgGs can provide partial protection against rhLCV infection in rhesus macaques, demonstrating the importance of antibodies in the oral mucosa in infection prevention (91). While vaccine-induced salivary Ig responses might be difficult to study in small animal models, future pre-clinical non-human primate (NHP) studies and clinical studies should also assess both salivary and serum IgA/IgG responses. This will lead to better understanding of correlates of immune protection.
4.2 Animal Model(s) to Test Vaccine Efficacy and Explore Correlates of Immune Protection
EBV is a human-tropic virus, making it difficult to study in vivo. Rodents, rabbits, and various NHPs have been historically used to test EBV vaccine immunogenicity (Table 4 and Figure 4C). However, only the cotton-top tamarin and common marmoset have been used to test in vivo vaccine efficacy. The cotton-top tamarin was the first animal to be established as an experimental model for EBV infection and the development of EBV-driven lymphoma (92). In 1985, the model was used for the first time to test the in vivo efficacy of a gp350 vaccine candidate (76). After that, 7 more studies used the model to test the efficacy of other gp350 vaccine candidates (Figure 4C), with the last study in 1994, as the model was abandoned in the early 2000s due to the endangered status of the species. Perhaps the greatest utility of this model was that it offered a direct readout of virus-associated disease (i.e., lymphoma) that was easy to measure in a relatively short timeline. However, the translatability of this model is limited because it did not truly recapitulate EBV infection in humans in terms of infection route, disease pathogenesis, or immune response to infection. The common marmoset, established as an experimental model of EBV infection in parallel with the cotton-top tamarin, was first used to test in vivo vaccine efficacy in 1989 (69, 93). Compared to the cotton-top tamarin model, this model is more translatable to human EBV infection (94, 95); nevertheless, only 2 more studies used it to test vaccine efficacy in the late 1990s, and it has since been abandoned for unclear reasons.
As a consequence of this dearth of in vivo models, in vitro neutralization assays using sera antibodies from immunized animals have been used as the primary vaccine “efficacy” readout in most pre-clinical EBV vaccine studies (Table 3), as described above. Humanized mice with a reconstituted human immune system are also susceptible to EBV infection of B cells, and like cotton-top tamarins develop lymphoma upon infection, providing a relatively quick readout of disease. However, this model suffers from the same drawbacks as cotton-top tamarins in terms of dissimilarities to human EBV infection; their epithelial cells are not susceptible to infection and their use is more suited for testing therapeutics rather than prophylactics. Rabbits have also been reported as susceptible to EBV infection (96–99), but no EBV-associated pathologies have been shown, and as humanized mice, their epithelial cells are not susceptible to infection (100). Similarly, Chinese tree shrews were recently reported to be susceptible to EBV B cell infection (101), but a previous study by the same group suggests that their epithelial cells are not infected by EBV (102). Both rabbits and tree shrews have yet to be used as challenge models to test EBV vaccine efficacy.
A promising alternative to test the efficacy of EBV vaccine candidates is to use rhesus macaques infected with rhesus lymphocryptovirus (rhLCV) as a surrogate for EBV infection. rhLCV shares high amino-acid homology with EBV (103), and rhLCV genes can complement the viral activities of EBV orthologs (104). Importantly, rhLCV infection in rhesus macaques recapitulates most aspects of EBV infection in humans, including the routes of infection, use of homologous glycoproteins to infect the host, disease establishment, and host immune responses (105). Furthermore, rhLCV-infected rhesus macaques that become immunosuppressed develop virus-associated malignancies of both epithelial and lymphoid origin, similar to immunosuppressed EBV-infected humans (106). The similarities between EBV and rhLCV were underscored in a recent study from the McGuire group, in which AMMO1, an EBV-specific nAb, was able to reduce rhLCV infection in rhesus macaques that had been passively infused with the nAb and subsequently challenged with rhLCV (107). Thus, the rhLCV infection model in rhesus macaques offers an opportunity to test the efficacy of EBV vaccines in the form of surrogate rhLCV vaccines in both immunocompetent and immunosuppressed settings, and future EBV vaccines studies should consider using this model to validate their in vitro findings.
The field could also re-evaluate the use of the common marmoset as an EBV challenge model. Early EBV studies using this model demonstrated the establishment of EBV infection in challenged animals and uncovered pathogenic similarities between common marmoset and human EBV infection (93). For example, infected common marmosets sporadically shed the virus in the oral mucosa and can transmit it to EBV-naïve animals through close contact, similar to how EBV transmission is thought to occur in humans (60). Infected common marmosets also share similar EBV antibody kinetics to infected humans (94). With the support of more modern EBV diagnostic methods and the rapid growth of common marmosets as a research NHP, this model has the potential to provide a powerful tool for testing EBV vaccine efficacy.
Use of NHPs such as rhesus macaques and common marmosets in EBV research will also facilitate the study of salivary antibodies to arrive at a better understanding of correlates of immune protection. However, one commonly overlooked caveat to the use of NHPs in this field, whether to test general vaccine immunogenicity or vaccine efficacy in a challenge model, is that all NHPs should be screened for infection with EBV-homologue LCVs. Cynomolgus macaques, rhesus macaques, and common marmosets are all naturally infected by species-specific LCVs (108), and as is the case in humans, infection is usually pervasive due to the high transmissibility of these viruses. This presents a problem due to the potential antibody cross-reactivity between the viruses, which could skew the assessment of any anti-EBV response induced in immunized animals. In our evaluation, only 1/16 studies that used NHPs acknowledged this issue [(50), Table 3], and no NHP study reported the use of LCV-negative animals. Thus, it is critical that moving forward all NHPs should be screened for native LCVs prior to any EBV vaccine immunogenicity studies. Development of specific-pathogen-free NHP colonies worldwide that are LCV-negative would be ideal.
4.3 Vaccine Antigen(s) Required to Elicit a Sufficiently Protective Response Against Primary EBV Infection
To date, there is still no consensus as to which glycoprotein(s) would serve as ideal vaccine antigens. Before the EBV glycoproteins were identified, early studies used whole virus or undefined membrane antigens from EBV-infected cells to begin exploring the general immunogenicity of EBV (109–111). By 1979, the analysis of purified cellular membranes from EBV-infected Raji cells allowed separation of various membrane-associated antigens with different molecular weights, which later became known as gp350/220, gB and gH (112). Despite this discovery and the identification of various nAbs specific to different glycoproteins in the 1980s and 2000s, most pre-clinical vaccine studies and all four clinical studies that followed focused on gp350 as the main vaccine target up until the 2010s, when other glycoproteins began to be seriously explored as additional potential vaccine antigens. The likely culprit for this focus seems to be the 1980 study by North, Morgan and Epstein, where neutralizing sera from rabbits immunized with whole virus was used to immunoprecipitate antigens from EBV producer cell lines, and gp350 was the only antigen precipitated at a detectable level (113). Furthermore, two gp350-specific nAbs were isolated and reported that same year (Table 1), strengthening the support for a gp350-based vaccine. Most gp350 vaccine studies that followed did result in the generation of gp350-specific antibodies with neutralizing capabilities in vitro, but no pre-clinical in vivo vaccine efficacy study has definitively shown protection against primary EBV infection. Ragot et al. (64) and Morgan et al. (70) demonstrated that gp350-expressing adenovirus and ISCOM-based gp350 nanoparticles fully protected cotton-top tamarins from developing EBV-induced lymphoma, respectively, but only n=4 animals were tested in each study (Table 3). Epstein et al. (76) similarly showed that cotton-top tamarins were fully protected from lymphoma when administered purified B95-8 membrane, but only n=2 animals were tested in this study. Thus, in these few cases showing positive results, too few experimental animals were tested to provide definitive conclusions, and Epstein et al. (76) also mentioned the presence of trace amounts of EBV in the administered vaccine product, which could have resulted in the emergence of nAbs against additional glycoproteins, skewing the results. Furthermore, there is no guarantee that protection from disease means primary infection did not take place; Morgan et al. (68) measured EBV infection in the blood and showed that although all cotton-top tamarins (n=4) were protected against lymphoma, only half were protected from infection (Table 3). Epstein et al. (76) demonstrated that cotton-top tamarins administered gp350 liposomes were ultimately protected against disease (n=2), but they developed transient lesions during the observation period (Table 3), suggesting that primary infection did take place.
This pattern continued in the clinical setting, and the first EBV vaccine clinical trial in 1995, which used a gp350 vaccinia-based vaccine, did not result in sterilizing immunity in vaccinated infants (83). Similar to the inconclusive results of the pre-clinical studies, this trial suggested that gp350 as a single vaccine antigen might not be sufficient to protect against EBV infection. Then, in 2000, the Delecluse group showed that recombinant EBV lacking gp350 could still infect both epithelial and B cells in vitro (114), suggesting that EBV could remain infectious even in the presence of gp350-specific nAbs. Nevertheless, three more clinical trials using gp350 vaccines were put forward in the 2000s (80–82). Although these vaccines reduced the incidence of IM in some cases, they did not provide sterilizing immunity against infection.
In the 2000s, several groups began to fully uncover the mechanisms of EBV entry in both epithelial and B cells, and the importance of the core fusion glycoproteins, gp42, gH/gL, and gB became apparent, as summarized in Figure 1. Our group and others have shown that gp350 binds complement receptor type 1 (CD35) (115) or type 2 (CD21) (116) on B cells and triggers endocytosis of the virions (30). Although this interaction is not essential as discussed above, it does enhance infection (114). Once the virus is bound to its target cell, the fusion machinery is required to achieve virus–cell fusion. gB is highly conserved among herpesviruses and is considered the core fusogen, achieving fusion through attachment to the host receptor neuropilin 1 (117). gB exists in two conformations, the pre- and the post-fusion states (118), and it is unclear which conformation might be best suited as a vaccine antigen (i.e., is more immunogenic) in the case of vaccines that rely on conformational epitopes; this remains an area of active investigation. In epithelial cells, the fusogenic activity of gB is triggered by the interaction of the gH/gL complex with the host ephrin A2 receptor (117, 119, 120). In B cells, gp42, in complex with gH/gL, activates gB after interacting with major histocompatibility complex (MHC) class II; in this way, the level of gp42 expression on the virion confers host cell specificity, promoting the infection of B cells and inhibiting the infection of epithelial cells (121, 122). Importantly, although it can mature and egress upon lytic induction, recombinant EBV lacking gH cannot infect epithelial cells, and recombinant EBV lacking gp42 or gH cannot infect B cells, suggesting that these glycoproteins are essential for EBV infection (37, 123). nAbs against these glycoproteins were also discovered between 1982 and 2000 (Table 1). Despite these discoveries, there have only been a few pre-clinical studies exploring non-gp350 glycoproteins as vaccine antigens (Figure 4A).
Given what we now know about the EBV infection process and how several glycoproteins collaborate to infect different types of target cells, we expect that the most effective vaccine strategy would be a multivalent approach targeting multiple important glycoproteins. Indeed, the fusion machinery constitutes the most obvious candidate to test in a multivalent EBV vaccine, but gp350 remains an important source of nAbs, and as such, it might still be an important vaccine target when used in combination with other antigens. Moreover, a recent study identified gp350 as the entry glycoprotein for T cells, which has implications for EBV-associated T and NK/T-cell lymphomas (124). Following this reasoning, the first glycoprotein combination vaccine was tested in 2008 by Lockey et al., which combined gp350 and gB (57). Although results were not conclusive on whether the combination was better than targeting gp350 alone, further combination studies that followed did provide support for a multivalent approach. For example, a recent pre-clinical study by the Cohen group reported better in vitro neutralization outcomes in mice after immunization with vaccines that combined the gH/gL and gp42/gH/gL complexes with gp350, compared to a vaccine that targeted gp350 alone; in the same study, a vaccine targeting the gp42/gH/gL complex resulted in better fusion inhibition outcomes than a vaccine targeting gH/gL complex alone (44). Similarly, in a recent study, our group reported that a multivalent vaccine incorporating gp350, gB, gp42, and gH/gL resulted in better neutralizing outcomes in immunized rabbits than a recombinant gp350 vaccine, with comparable results to immunization with UV-inactivated EBV (43). However, it is still not clear what combination of glycoproteins would best to prevent infection in vivo or whether incorporating all five glycoproteins is necessary. Thus, future studies should focus on identifying the required components of a multivalent EBV vaccine that can generate effective neutralizing activity both in vitro and in vivo. Indeed, ModernaTX Inc. has recently started recruiting participants for a Phase I clinical trial that will explore the safety and immunogenicity of their mRNA-based EBV vaccine, mRNA-1189, which targets multiple EBV glycoproteins (125–127).
While gp350, gB, gp42 and gH/gL have been the most studied entry glycoproteins, there are additional EBV envelope glycoproteins that could also prove to be important vaccine targets. BMRF2 has been reported to participate in viral entry by facilitating viral attachment to epithelial cells through its interactions with various host integrins (128, 129), and it has been implicated in cell-to-cell spread as well (130). There is data suggesting its potential to elicit nAbs (131), and it might be a relevant target if the virus escapes initial neutralization and breaches the oral mucosa, where it could spread from B cells to monocytes and to epithelial cells (132) in vivo. BDLF2 is co-expressed with BMRF2 (133) and thus might also be involved in cell-to-cell spread (134), but not much has been further uncovered about this glycoprotein. BDLF3/gp150 has been shown to bind to heparan sulfate proteoglyclans on the surface of epithelial cells, but this binding does not enhance infection (135), and its role in immune evasion may preclude any role in viral entry (136). BILF2 might be involved in glycoprotein transport (137), but otherwise is considered an orphan glycoprotein as no definite function has been uncovered for it (122). Future studies might reveal the potential utility of these additional glycoproteins as vaccine targets. Importantly, while the purpose of this review was to study previous prophylactic vaccine efforts focused on humoral immunity, we cannot ignore the possibility that a vaccine designed to target both humoral and cellular immunity could prove protective. Indeed, although we did not discuss the details, a few studies in our analysis [(46, 56, 57); Table 3] tested vaccines incorporating both glycoproteins and different targets of cellular immunity. The importance of these combinations remains to be tested in vivo. Furthermore, ideal vaccine regimen, dose, and route might differ between humoral and cellular immune targets, thus these parameters may be first tested separately for their immunogenicity before combining immune targets.
4.4 Ideal Vaccine Platform to Present Relevant EBV Antigen(s) in a Sufficiently Immunogenic Format
In our analysis, most pre-clinical trials (Figure 4B, Table 3) and clinical trials (Table 4) delivered EBV antigens as monomeric proteins, the most widely used platform in the earlier half of EBV vaccine research. Monomeric proteins are perhaps the least immunogenic of the vaccine platforms available, requiring the use of adjuvants to induce a robust immune response. The pre-clinical studies in our analysis used a wide variety of adjuvants, including aluminum hydroxide/alum, glucopyranosyl lipid A/GLA, stable emulsion/SE, SAF-1, and Freund’s adjuvant (Table 3) (138). In addition to low immunogenicity, another limitation to the use of monomeric proteins is that the higher-level protein structure of the antigens might be impaired in the monomeric state, inhibiting the induction of conformation-dependent nAbs (139). It is perhaps for these reasons that, after a lag in pre-clinical prophylactic EBV vaccine development between 1999 and 2008, several additional antigen delivery approaches were adopted to incorporate protein structural support and to provide the ability to present multiple antigens per unit. For example, two pre-clinical studies by the Snapper group explored the use of multimeric proteins [(48, 54), Table 3]. In both studies, the multimeric protein vaccines were more immunogenic than their corresponding monomeric protein vaccines. The use of self-assembling nanoparticles incorporating EBV glycoproteins has also been explored. The Cohen group (44, 50) showed that ferritin-based nanoparticles incorporating gp350, gH/gL, or gH/gL/gp42 were more immunogenic than the monomeric soluble forms of these glycoproteins, and the National Institute of Allergy and Infectious Diseases is currently recruiting participants to test the safety and immunogenicity of the gp350-ferritin-based nanoparticle in a Phase I clinical trial (140). Nanoparticles in the form of liposomes were also tested in the early stages of EBV vaccine research [(74, 76–78), Table 3], which focused on gp350. The platform was not pursued further, perhaps due to its ultimate inability to prevent lymphoma in cotton-top tamarins after viral challenge; however, as discussed in Section 4.3, this result may have occurred due to the use of gp350 as a single immunogen rather than insufficiency of the platform. Other recent studies have explored the use of VLPs, a special type of nanoparticle that resembles viruses in structure and, like other nanoparticles, can present multiple antigens or epitopes in a repetitive array, increasing antigen immunogenicity (141). Importantly, VLPs lack viral DNA, which is critical in the development of vaccines for oncogenic viruses such as EBV. The success of VLPs as a vaccine platform is underscored by the many licensed VLP-based vaccines on the market, including vaccines against human papillomavirus and hepatitis B and E. Five pre-clinical studies have investigated the use of VLPs for EBV, including VLPs produced from EBV-packaging cell lines (56), VLPs based on Newcastle disease virus (49), and VLPs produced from the hepatitis B virus core antigen (42). Although vaccines that present proteins in a multimeric form, whether as multimeric proteins or nanoparticles, are a better alternative to monomeric proteins, they might be expensive to produce in large quantities and still require adjuvants to achieve an adequate level of immunogenicity.
Viral vectors have the advantage of possessing intrinsic immune-stimulating capabilities and thus do not require administration with adjuvants; in addition, they can be combined with VLP platforms to produce VLPs in vivo (142–144). The 6 pre-clinical EBV vaccine studies using viral vectors tested measles virus (55), adenovirus (64), and vaccinia virus vectors (57, 61, 70, 75) (Table 3); the latter was also tested in one of the 4 EBV vaccine clinical trials [(83), Table 4]. In these studies, the vectors were engineered to express single antigens, but viral vectors with the necessary genetic insert capacity can be engineered to express multiple antigens. Measles virus and adenovirus vectors have good clinical track records, but they do not possess the genetic capacity to express multiple EBV antigens (145, 146). Vaccinia virus vectors, on the other hand, have the largest genetic insert capacity of all available viral vaccine vectors (>30 kb), and although the original vaccinia strains can pose serious health complications, the highly attenuated modified vaccinia Ankara (MVA) strain is safe even in immunocompromised populations and is highly immunogenic (147–149). Hence, future multivalent vaccine studies looking to use a viral vector-based platform should consider an MVA vaccine platform.
Although we did not encounter any studies within our search limits that utilized mRNA-based vaccines, the new Phase I clinical trial by ModernaTX Inc. that is currently recruiting participants to test a multivalent mRNA-based EBV vaccine will open the way for this technology to the field of EBV vaccine research (127). Having only recently been clinically tested at a large scale against SARS-CoV-2, questions about the response durability of mRNA-based vaccines remain (150), and whether this will be a successful approach for targeting multiple antigens in the context of EBV remains to be studied.
While not a concern for viral or nucleic-acid-based vaccines, an important consideration when producing protein antigens for vaccination is the choice of expression system. Post-translational modifications, such as glycosylation, are an important element that can affect antigen immunogenicity. Indeed, the Hayes group in 2015 studied the conformational requirements of gp350 to generate a nAb response [(51), Table 3], and found that glycosylation is essential to gp350 nAb generation, which can only properly be achieved in mammalian expression systems. This was previously demonstrated by two studies in 1991 and 1988 [(67, 72), Table 3] which explored expression of gp350 in bacteria and yeast, respectively, and found the resulting products incapable of generating nAbs, presumably due to the inability of these systems to perform complex glycosylation (151). Protein production in simpler systems such as bacteria and yeast do offer various advantages in terms of large-scale production feasibility, but unless glycosylation engineering approaches are applied in this context (152), mammalian cells remain the ideal production system for EBV glycoproteins.
A final consideration when it comes to vaccine platforms pertains to the feasibility of large-scale manufacturing, including processing, purification, and quality testing. An early EBV vaccine study by Epstein and his group [(74), Table 3] demonstrates the potential relevance of establishing appropriate processing methods. In this study, the authors used a protocol established by Randle et al., 1985 (153) to perform large-scale purification of gp350 from EBV producer cells using immunoaffinity chromatography; however, they found that the resulting product had a specific activity of 20% of that of a product prepared by molecular weight-based separation methods in small-scale, and failed to protect animals against EBV disease when delivered in liposomes. The authors attribute this to either the type of purification used resulting in antigen denaturation, or the isolated protein fraction not being immunogenic enough to confer protection as the previous formulation did. Today, biological manufacturing techniques have greatly improved, but the fact that different vaccine platforms possess different biochemical and structural qualities that require unique processing needs and quality testing should still be considered when designing a new vaccine that can be produced in enough quantity and with high enough quality for large-scale immunization (154). Thus, an ideal vaccine platform should not only be sufficiently immunogenic and protective, but also allow for the necessary infrastructure to facilitate future clinical translation.
4.5 Pre-Clinical Study Quality
Most of the pre-clinical studies included in our systematic review were of moderate, high, or very high quality (Figure 4D and Table S3); all pre-clinical studies of poor or very poor quality were performed before the year 2000. Although there was a clear shift toward higher-quality studies after 1999, our analysis demonstrated that there is still room for improvement. For example, none of the studies analyzed performed a blinded analysis, which would reduce potential bias and enhance scientific rigor. In addition, only 3 studies explicitly evaluated the toxicity of the vaccine tested (Table S3), which is essential for selecting vaccine candidates that are suitable for clinical use. As discussed in Section 4.1, it is particularly important to report neutralization assay details to accurately assess vaccine efficacy, including titer/infectivity information for the viruses used, but this was not the case for many of the analyzed studies. The use of both positive and negative control treatment groups and appropriately powered group sizes are also critical but were frequently not reported. Future studies should focus on these and other qualities (Table S3) to generate high-caliber studies with accurate, generalizable conclusions.
4.6 Limitations of the Study
While we aimed for our report to be as robust as possible, we do acknowledge a few limitations in our study. The small number of clinical trials included in our analysis, together with the variability in the quality (Table S3) and content of the pre-clinical trials, did not allow us to perform a meta-analysis or any additional meaningful statistical analysis or comparison between the studies, so our results lack statistical relevance. In addition, although our search was thorough to the best of our efforts, it is possible that we might have missed articles that fit our selection criteria.
Finally, while we structured our discussion towards developing a humoral-based vaccine to prevent primary EBV infection, this goal might be unrealistic. No sterilizing immunity has yet been achieved in pre-clinical or clinical studies against any herpesvirus. In the case of EBV, recent passive immunization studies in humanized mice (155) and in rhesus macaques using the rhLCV model (91, 107), did not achieve sterilizing immunity, although these studies were not focused on multivalent approaches. Cellular immunity might also be an important component to improve protective vaccine responses, which we did not consider in our review. Nevertheless, even if sterilizing immunity cannot be achieved against EBV via vaccination, an EBV vaccine might still be effective in reducing the rates of EBV diseases and their associated morbidities and mortality, such as is the case for the approved varicella zoster virus vaccines (156), and the EBV gp350-based vaccine tested in a Phase II clinical trial that was 78% successful in preventing infectious mononucleosis [(81), Table 4].
5 Conclusions
Our analysis of 36 pre-clinical studies and 4 clinical studies conducted over the last four decades strongly supports the use of a multivalent approach to develop an effective prophylactic EBV vaccine. In addition, testing in vivo vaccine efficacy in more robust animal models, such as the common marmoset and rhLCV-susceptible rhesus macaques, is expected to facilitate the establishment of standardized in vivo correlates of immune protection and the attainment of more generalizable and translatable data. Finally, our analysis suggests that new vaccine-developing studies should explore vaccine platforms that can enhance immunogenicity via multimeric approaches. We anticipate that evidence-based rational vaccine design, guided by the studies presented here, will yield an effective EBV vaccine that can finally be translated into the clinic to prevent more than 200,000 cases of cancer and numerous cases of IM and autoimmune disease each year.
Data Availability Statement
The original contributions presented in the study are included in the article/Supplementary Material. Further inquiries can be directed to the corresponding author.
Author Contributions
Conceived and designed the study: JO, GE, and LM. Performed the systematic review: JO, GE, LM, MM, and ER. Analyzed the data: JO, GE, LM, MM, and ER. Wrote the paper: JO, GE, LM, MM, and ER. All authors contributed to the article and approved the submitted version.
Funding
This study was funded by the National Institute for Allergy and Infectious Diseases R56AI148295 grant to JO, and Department of Defense W81XWH-20-1-0401 Horizon Award to GE. The funding agencies were not involved in the design of the study, data analysis or interpretation, nor manuscript preparation or study publication.
Conflict of Interest
The authors declare that the research was conducted in the absence of any commercial or financial relationships that could be construed as a potential conflict of interest.
Publisher’s Note
All claims expressed in this article are solely those of the authors and do not necessarily represent those of their affiliated organizations, or those of the publisher, the editors and the reviewers. Any product that may be evaluated in this article, or claim that may be made by its manufacturer, is not guaranteed or endorsed by the publisher.
Acknowledgments
We are grateful to Dr. Paul Bain of Countway, Library of Medicine, Harvard Medical School, for help in designing the search strategies for all databases used, as well as Ms. Kyra Love and Ms. Jeannette Duffels, clinical librarians of Lee Graff Medical and Scientific Library at City of Hope, for performing the databases searches. We thank Dr. Marta Jankowska, Population Sciences, City of Hope, and Victoria Erickson of Chapman University, for assisting us with figure design. We are also grateful to Dr. Kerin Higa for her critical review of the manuscript and language editing.
Supplementary Material
The Supplementary Material for this article can be found online at: https://www.frontiersin.org/articles/10.3389/fimmu.2022.867918/full#supplementary-material
References
1. Cohen JI, Fauci AS, Varmus H, Nabel GJ. Epstein-Barr Virus: An Important Vaccine Target for Cancer Prevention. Sci Transl Med (2011) 3(107):107fs7. doi: 10.1126/scitranslmed.3002878
2. Khan G, Hashim MJ. Global Burden of Deaths From Epstein-Barr Virus Attributable Malignancies 1990-2010. Infect Agents Cancer (2014) 9(1):38. doi: 10.1186/1750-9378-9-38
3. Cohen JI, Mocarski ES, Raab-Traub N, Corey L, Nabel GJ. The Need and Challenges for Development of an Epstein-Barr Virus Vaccine. Vaccine (2013) 31:B194–6. doi: 10.1016/j.vaccine.2012.09.041
4. Houen G, Trier NH. Epstein-Barr Virus and Systemic Autoimmune Diseases. Front Immunol (2020) 11:587380. doi: 10.3389/fimmu.2020.587380
5. Bjornevik K, Cortese M, Healy BC, Kuhle J, Mina MJ, Leng Y, et al. Longitudinal Analysis Reveals High Prevalence of Epstein-Barr Virus Associated With Multiple Sclerosis. Science (2022) 375(6578):296–301. doi: 10.1126/science.abj8222
6. Lanz TV, Brewer RC, Ho PP, Moon JS, Jude KM, Fernandez D, et al. Clonally Expanded B Cells in Multiple Sclerosis Bind Ebv Ebna1 and Glialcam. Nature (2022) 603(7900):321–27. doi: 10.1038/s41586-022-04432-7
7. Epstein MA, Achong BG, Barr YM. Virus Particles in Cultured Lymphoblasts From Burkitt’s Lymphoma. Lancet (1964) 1(7335):702–3. doi: 10.1016/s0140-6736(64)91524-7
8. Henle G, Henle W, Diehl V. Relation of Burkitt’s Tumor-Associated Herpes-Ytpe Virus to Infectious Mononucleosis. Proc Natl Acad Sci USA (1968) 59(1):94–101. doi: 10.1073/pnas.59.1.94
9. Henle W, Henle G, Ho HC, Burtin P, Cachin Y, Clifford P, et al. Antibodies to Epstein-Barr Virus in Nasopharyngeal Carcinoma, Other Head and Neck Neoplasms, and Control Groups. J Natl Cancer Inst (1970) 44(1):225–31.10.1093/jnci/44.1.225
10. zur Hausen H, Schulte-Holthausen H, Klein G, Henle W, Henle G, Clifford P, et al. Ebv DNA in Biopsies of Burkitt Tumours and Anaplastic Carcinomas of the Nasopharynx. Nature (1970) 228(5276):1056–8. doi: 10.1038/2281056a0
11. Hanto DW, Frizzera G, Purtilo DT, Sakamoto K, Sullivan JL, Saemundsen AK, et al. Clinical Spectrum of Lymphoproliferative Disorders in Renal Transplant Recipients and Evidence for the Role of Epstein-Barr Virus. Cancer Res (1981) 41(11 Pt 1):4253–61.
13. Weiss LM, Movahed LA, Warnke RA, Sklar J. Detection of Epstein-Barr Viral Genomes in Reed-Sternberg Cells of Hodgkin’s Disease. N Engl J Med (1989) 320(8):502–6. doi: 10.1056/NEJM198902233200806
14. Jones JF, Shurin S, Abramowsky C, Tubbs RR, Sciotto CG, Wahl R, et al. T-Cell Lymphomas Containing Epstein-Barr Viral DNA in Patients With Chronic Epstein-Barr Virus Infections. N Engl J Med (1988) 318(12):733–41. doi: 10.1056/NEJM198803243181203
15. Kimura H, Fujiwara S. Overview of Ebv-Associated T/Nk-Cell Lymphoproliferative Diseases. Front Pediatr (2018) 6:417. doi: 10.3389/fped.2018.00417
16. Burke AP, Yen TS, Shekitka KM, Sobin LH. Lymphoepithelial Carcinoma of the Stomach With Epstein-Barr Virus Demonstrated by Polymerase Chain Reaction. Mod Pathol (1990) 3(3):377–80.
17. Shibata D, Weiss LM. Epstein-Barr Virus-Associated Gastric Adenocarcinoma. Am J Pathol (1992) 140(4):769–74.
18. Harn HJ, Chang JY, Wang MW, Ho LI, Lee HS, Chiang JH, et al. Epstein-Barr Virus-Associated Gastric Adenocarcinoma in Taiwan. Hum Pathol (1995) 26(3):267–71. doi: 10.1016/0046-8177(95)90056-x
19. Hsieh LL, Lin PJ, Chen TC, Ou JT. Frequency of Epstein-Barr Virus-Associated Gastric Adenocarcinoma in Taiwan. Cancer Lett (1998) 129(2):125–9. doi: 10.1016/s0304-3835(98)00111-6
20. Levine PH, Stemmermann G, Lennette ET, Hildesheim A, Shibata D, Nomura A. Elevated Antibody Titers to Epstein-Barr Virus Prior to the Diagnosis of Epstein-Barr-Virus-Associated Gastric Adenocarcinoma. Int J Cancer (1995) 60(5):642–4. doi: 10.1002/ijc.2910600513
21. Shibata D, Hawes D, Stemmermann GN, Weiss LM. Epstein-Barr Virus-Associated Gastric Adenocarcinoma Among Japanese Americans in Hawaii. Cancer Epidemiol Biomarkers Prev (1993) 2(3):213–7.
22. Tavakoli A, Monavari SH, Solaymani Mohammadi F, Kiani SJ, Armat S, Farahmand M. Association Between Epstein-Barr Virus Infection and Gastric Cancer: A Systematic Review and Meta-Analysis. BMC Cancer (2020) 20(1):493. doi: 10.1186/s12885-020-07013-x
23. Wang H, Chen XL, Liu K, Bai D, Zhang WH, Chen XZ, et al. Associations Between Gastric Cancer Risk and Virus Infection Other Than Epstein-Barr Virus: A Systematic Review and Meta-Analysis Based on Epidemiological Studies. Clin Transl Gastroenterol (2020) 11(7):e00201. doi: 10.14309/ctg.0000000000000201
24. Epstein MA. Epstein-Barr Virus–Is It Time to Develop a Vaccine Program? J Natl Cancer Inst (1976) 56(4):697–700. doi: 10.1093/jnci/56.4.697
25. Iwasaki A. Exploiting Mucosal Immunity for Antiviral Vaccines. Annu Rev Immunol (2016) 34:575–608. doi: 10.1146/annurev-immunol-032414-112315
26. Krause PR, Klinman DM. Efficacy, Immunogenicity, Safety, and Use of Live Attenuated Chickenpox Vaccine. J Pediatr (1995) 127(4):518–25. doi: 10.1016/s0022-3476(95)70106-0
27. Rickinson AB, Kieff E. Epstein-Barr Virus. In: Knipe D, Howley P, editors. Fields Virology Epstein-Barr Virus, Fifth ed, vol. 2 . Philadelphia: Lippincott Wilkins and Williams (2007). p. 2680–700.
28. Rickinson AB, Long HM, Palendira U, Munz C, Hislop AD. Cellular Immune Controls Over Epstein-Barr Virus Infection: New Lessons From the Clinic and the Laboratory. Trends Immunol (2014) 35(4):159–69. doi: 10.1016/j.it.2014.01.003
29. Rickinson AB, Moss DJ. Human Cytotoxic T Lymphocyte Responses to Epstein-Barr Virus Infection. Annu Rev Immunol (1997) 15:405–31. doi: 10.1146/annurev.immunol.15.1.405
30. Cohen JI. Epstein-Barr Virus Vaccines. Clin Trans Immunol (2015) 4(1):e32. doi: 10.1038/cti.2014.27
31. Cohen JI. Vaccine Development for Epstein-Barr Virus. Adv Exp Med Biol (2018) 1045:477–93. doi: 10.1007/978-981-10-7230-7_22
32. Balfour HH Jr, Schmeling DO, Grimm-Geris JM. The Promise of a Prophylactic Epstein-Barr Virus Vaccine. Pediatr Res (2020) 87(2):345–52. doi: 10.1038/s41390-019-0591-5
33. Hoffman GJ, Lazarowitz SG, Hayward SD. Monoclonal Antibody Against a 250,000-Dalton Glycoprotein of Epstein-Barr Virus Identifies a Membrane Antigen and a Neutralizing Antigen. Proc Natl Acad Sci USA (1980) 77(5):2979–83. doi: 10.1073/pnas.77.5.2979
34. Thorley-Lawson DA, Geilinger K. Monoclonal Antibodies Against the Major Glycoprotein (Gp350/220) of Epstein-Barr Virus Neutralize Infectivity. Proc Natl Acad Sci USA (1980) 77(9):5307–11. doi: 10.1073/pnas.77.9.5307
35. Strnad BC, Schuster T, Klein R, Hopkins RF 3rd, Witmer T, Neubauer RH, et al. Production and Characterization of Monoclonal Antibodies Against the Epstein-Barr Virus Membrane Antigen. J Virol (1982) 41(1):258–64. doi: 10.1128/JVI.41.1.258-264.1982
36. Oba DE, Hutt-Fletcher LM. Induction of Antibodies to the Epstein-Barr Virus Glycoprotein Gp85 With a Synthetic Peptide Corresponding to a Sequence in the Bxlf2 Open Reading Frame. J Virol (1988) 62(4):1108–14. doi: 10.1128/JVI.62.4.1108-1114.1988
37. Molesworth SJ, Lake CM, Borza CM, Turk SM, Hutt-Fletcher LM. Epstein-Barr Virus Gh Is Essential for Penetration of B Cells But Also Plays a Role in Attachment of Virus to Epithelial Cells. J Virol (2000) 74(14):6324–32. doi: 10.1128/jvi.74.14.6324-6332.2000
38. Snijder J, Ortego MS, Weidle C, Stuart AB, Gray MD, McElrath MJ, et al. An Antibody Targeting the Fusion Machinery Neutralizes Dual-Tropic Infection and Defines a Site of Vulnerability on Epstein-Barr Virus. Immunity (2018) 48(4):799–811 e9. doi: 10.1016/j.immuni.2018.03.026
39. Mutsvunguma LZ, Rodriguez E, Escalante GM, Muniraju M, Williams JC, Warden C, et al. Identification of Multiple Potent Neutralizing and Non-Neutralizing Antibodies Against Epstein-Barr Virus Gp350 Protein With Potential for Clinical Application and as Reagents for Mapping Immunodominant Epitopes. Virology (2019) 536:1–15. doi: 10.1016/j.virol.2019.07.026
40. Zhu Q-Y, Shan S, Yu J, Peng S-Y, Sun C, Zuo Y, et al. A Potent and Protective Human Neutralizing Antibody Targeting a Key Vulnerable Site of Epstein-Barr Virus. Nat Portfolio (2021) 12(1):6624. doi: 10.21203/rs.3.rs-151895/v1
41. Moher D, Liberati A, Tetzlaff J, Altman DG, Group P. Preferred Reporting Items for Systematic Reviews and Meta-Analyses: The Prisma Statement. PloS Med (2009) 6(7):e1000097. doi: 10.1371/journal.pmed.1000097
42. Zhang X, Zhao B, Ding M, Song S, Kang Y, Yu Y, et al. A Novel Vaccine Candidate Based on Chimeric Virus-Like Particle Displaying Multiple Conserved Epitope Peptides Induced Neutralizing Antibodies Against Ebv Infection. Theranostics (2020) 10(13):5704–18. doi: 10.7150/thno.42494
43. Escalante GM, Foley J, Mutsvunguma LZ, Rodriguez E, Mulama DH, Muniraju M, et al. A Pentavalent Epstein-Barr Virus-Like Particle Vaccine Elicits High Titers of Neutralizing Antibodies Against Epstein-Barr Virus Infection in Immunized Rabbits. Vaccines (Basel) (2020) 8(2):169. doi: 10.3390/vaccines8020169
44. Bu W, Joyce MG, Nguyen H, Banh DV, Aguilar F, Tariq Z, et al. Immunization With Components of the Viral Fusion Apparatus Elicits Antibodies That Neutralize Epstein-Barr Virus in B Cells and Epithelial Cells. Immunity (2019) 50(5):1305–16.e6. doi: 10.1016/j.immuni.2019.03.010
45. Zhao B, Zhang X, Krummenacher C, Song S, Gao L, Zhang H, et al. Immunization With Fc-Based Recombinant Epstein-Barr Virus Gp350 Elicits Potent Neutralizing Humoral Immune Response in a Balb/C Mice Model. Front Immunol (2018) 9:932. doi: 10.3389/fimmu.2018.00932
46. Perez EM, Foley J, Tison T, Silva R, Ogembo JG. Novel Epstein-Barr Virus-Like Particles Incorporating Gh/Gl-Ebna1 or Gb-Lmp2 Induce High Neutralizing Antibody Titers and Ebv-Specific T-Cell Responses in Immunized Mice. Oncotarget (2017) 8(12):19255–73. doi: 10.18632/oncotarget.13770
47. Heeke DS, Lin R, Rao E, Woo JC, McCarthy MP, Marshall JD. Identification of Gla/Se as an Effective Adjuvant for the Induction of Robust Humoral and Cell-Mediated Immune Responses to Ebv-Gp350 in Mice and Rabbits. Vaccine (2016) 34(23):2562–9. doi: 10.1016/j.vaccine.2016.04.012
48. Cui X, Cao Z, Chen Q, Arjunaraja S, Snow AL, Snapper CM. Rabbits Immunized With Epstein-Barr Virus Gh/Gl or Gb Recombinant Proteins Elicit Higher Serum Virus Neutralizing Activity Than Gp350. Vaccine (2016) 34(34):4050–5. doi: 10.1016/j.vaccine.2016.06.021
49. Ogembo JG, Muraswki MR, McGinnes LW, Parcharidou A, Sutiwisesak R, Tison T, et al. A Chimeric Ebv Gp350/220-Based Vlp Replicates the Virion B-Cell Attachment Mechanism and Elicits Long-Lasting Neutralizing Antibodies in Mice. J Transl Med (2015) 13:50. doi: 10.1186/s12967-015-0415-2
50. Kanekiyo M, Bu W, Joyce MG, Meng G, Whittle JR, Baxa U, et al. Rational Design of an Epstein-Barr Virus Vaccine Targeting the Receptor-Binding Site. Cell (2015) 162(5):1090–100. doi: 10.1016/j.cell.2015.07.043
51. Servat E, Ro BW, Cayatte C, Gemmell L, Barton C, Rao E, et al. Identification of the Critical Attribute(S) of Ebv Gp350 Antigen Required for Elicitation of a Neutralizing Antibody Response In Vivo. Vaccine (2015) 33(48):6771–7. doi: 10.1016/j.vaccine.2015.10.024
52. Tanner JE, Coincon M, Leblond V, Hu J, Fang JM, Sygusch J, et al. Peptides Designed to Spatially Depict the Epstein-Barr Virus Major Virion Glycoprotein Gp350 Neutralization Epitope Elicit Antibodies That Block Virus-Neutralizing Antibody 72a1 Interaction With the Native Gp350 Molecule. J Virol (2015) 89(9):4932–41. doi: 10.1128/JVI.03269-14
53. Herrman M, Muhe J, Quink C, Wang F. Epstein-Barr Virus Gp350 Can Functionally Replace the Rhesus Lymphocryptovirus Major Membrane Glycoprotein and Does Not Restrict Infection of Rhesus Macaques. J Virol (2016) 90(3):1222–30. doi: 10.1128/JVI.02531-15
54. Cui X, Cao Z, Sen G, Chattopadhyay G, Fuller DH, Fuller JT, et al. A Novel Tetrameric Gp350 1-470 as a Potential Epstein-Barr Virus Vaccine. Vaccine (2013) 31(30):3039–45. doi: 10.1016/j.vaccine.2013.04.071
55. Mok H, Cheng X, Xu Q, Zengel JR, Parhy B, Zhao J, et al. Evaluation of Measles Vaccine Virus as a Vector to Deliver Respiratory Syncytial Virus Fusion Protein or Epstein-Barr Virus Glycoprotein Gp350. Open Virol J (2012) 6:12–22. doi: 10.2174/1874357901206010012
56. Ruiss R, Jochum S, Wanner G, Reisbach G, Hammerschmidt W, Zeidler R. A Virus-Like Particle-Based Epstein-Barr Virus Vaccine. J Virol (2011) 85(24):13105–13. doi: 10.1128/JVI.05598-11
57. Lockey TD, Zhan X, Surman S, Sample CE, Hurwitz JL. Epstein-Barr Virus Vaccine Development: A Lytic and Latent Protein Cocktail. Front Biosci (2008) 13:5916–27. doi: 10.2741/3126
58. Wilson AD, Lovgren-Bengtsson K, Villacres-Ericsson M, Morein B, Morgan AJ. The Major Epstein-Barr Virus (Ebv) Envelope Glycoprotein Gp340 When Incorporated Into Iscoms Primes Cytotoxic T-Cell Responses Directed Against Ebv Lymphoblastoid Cell Lines. Vaccine (1999) 17(9-10):1282–90. doi: 10.1016/s0264-410x(98)00351-x
59. Jackman WT, Mann KA, Hoffmann HJ, Spaete RR. Expression of Epstein-Barr Virus Gp350 as a Single Chain Glycoprotein for an Ebv Subunit Vaccine. Vaccine (1999) 17(7-8):660–8. doi: 10.1016/s0264-410x(98)00248-5
60. Cox C, Naylor BA, Mackett M, Arrand JR, Griffin BE, Wedderburn N. Immunization of Common Marmosets With Epstein-Barr Virus (Ebv) Envelope Glycoprotein Gp340: Effect on Viral Shedding Following Ebv Challenge. J Med Virol (1998) 55(4):255–61. doi: 10.1002/(sici)1096-9071(199808)55:4<255::aid-jmv1>3.0.co;2-#
61. Mackett M, Cox C, Pepper SD, Lees JF, Naylor BA, Wedderburn N, et al. Immunisation of Common Marmosets With Vaccinia Virus Expressing Epstein-Barr Virus (Ebv) Gp340 and Challenge With Ebv. J Med Virol (1996) 50(3):263–71. doi: 10.1002/(SICI)1096-9071(199611)50:3<263::AID-JMV9>3.0.CO;2-7
62. de Freitas LFD, Oliveira RP, Miranda MCG, Rocha RP, Barbosa-Stancioli EF, Faria AMC, et al. The Virulence of Different Vaccinia Virus Strains Is Directly Proportional to Their Ability to Downmodulate Specific Cell-Mediated Immune Compartments in Vivo. J Virol (2019) 93(6):e02191–18. doi: 10.1128/JVI.02191-18
63. Finerty S, Mackett M, Arrand JR, Watkins PE, Tarlton J, Morgan AJ. Immunization of Cottontop Tamarins and Rabbits With a Candidate Vaccine Against the Epstein-Barr Virus Based on the Major Viral Envelope Glycoprotein Gp340 and Alum. Vaccine (1994) 12(13):1180–4. doi: 10.1016/0264-410x(94)90240-2
64. Ragot T, Finerty S, Watkins PE, Perricaudet M, Morgan AJ. Replication-Defective Recombinant Adenovirus Expressing the Epstein-Barr Virus (Ebv) Envelope Glycoprotein Gp340/220 Induces Protective Immunity Against Ebv-Induced Lymphomas in the Cottontop Tamarin. J Gen Virol (1993) 74(Pt 3):501–7. doi: 10.1099/0022-1317-74-3-501
65. Madej M, Conway MJ, Morgan AJ, Sweet J, Wallace L, Qualtiere LF, et al. Purification and Characterization of Epstein-Barr Virus Gp340/220 Produced by a Bovine Papillomavirus Virus Expression Vector System. Vaccine (1992) 10(11):777–82. doi: 10.1016/0264-410x(92)90513-j
66. Finerty S, Tarlton J, Mackett M, Conway M, Arrand JR, Watkins PE, et al. Protective Immunization Against Epstein-Barr Virus-Induced Disease in Cottontop Tamarins Using the Virus Envelope Glycoprotein Gp340 Produced From a Bovine Papillomavirus Expression Vector. J Gen Virol (1992) 73(Pt 2):449–53. doi: 10.1099/0022-1317-73-2-449
67. Zhang PF, Klutch M, Armstrong G, Qualtiere L, Pearson G, Marcus-Sekura CJ. Mapping of the Epitopes of Epstein-Barr Virus Gp350 Using Monoclonal Antibodies and Recombinant Proteins Expressed in Escherichia Coli Defines Three Antigenic Determinants. J Gen Virol (1991) 72(Pt 11):2747–55. doi: 10.1099/0022-1317-72-11-2747
68. Morgan AJ, Allison AC, Finerty S, Scullion FT, Byars NE, Epstein MA. Validation of a First-Generation Epstein-Barr Virus Vaccine Preparation Suitable for Human Use. J Med Virol (1989) 29(1):74–8. doi: 10.1002/jmv.1890290114
69. Emini EA, Schleif WA, Silberklang M, Lehman D, Ellis RW. Vero Cell-Expressed Epstein-Barr Virus (Ebv) Gp350/220 Protects Marmosets From Ebv Challenge. J Med Virol (1989) 27(2):120–3. doi: 10.1002/jmv.1890270210
70. Morgan AJ, Mackett M, Finerty S, Arrand JR, Scullion FT, Epstein MA. Recombinant Vaccinia Virus Expressing Epstein-Barr Virus Glycoprotein Gp340 Protects Cottontop Tamarins Against Eb Virus-Induced Malignant Lymphomas. J Med Virol (1988) 25(2):189–95. doi: 10.1002/jmv.1890250209
71. Morgan AJ, Finerty S, Lovgren K, Scullion FT, Morein B. Prevention of Epstein-Barr (Eb) Virus-Induced Lymphoma in Cottontop Tamarins by Vaccination With the Eb Virus Envelope Glycoprotein Gp340 Incorporated Into Immune-Stimulating Complexes. J Gen Virol (1988) 69(Pt 8):2093–6. doi: 10.1099/0022-1317-69-8-2093
72. Emini EA, Schleif WA, Armstrong ME, Silberklang M, Schultz LD, Lehman D, et al. Antigenic Analysis of the Epstein-Barr Virus Major Membrane Antigen (Gp350/220) Expressed in Yeast and Mammalian Cells: Implications for the Development of a Subunit Vaccine. Virology (1988) 166(2):387–93. doi: 10.1016/0042-6822(88)90509-0
73. David EM, Morgan AJ. Efficient Purification of Epstein-Barr Virus Membrane Antigen Gp340 by Fast Protein Liquid Chromatography. J Immunol Methods (1988) 108(1-2):231–6. doi: 10.1016/0022-1759(88)90424-3
74. Epstein MA, Randle BJ, Finerty S, Kirkwood JK. Not All Potently Neutralizing, Vaccine-Induced Antibodies to Epstein-Barr Virus Ensure Protection of Susceptible Experimental Animals. Clin Exp Immunol (1986) 63(3):485–90.
75. Mackett M, Arrand JR. Recombinant Vaccinia Virus Induces Neutralising Antibodies in Rabbits Against Epstein-Barr Virus Membrane Antigen Gp340. EMBO J (1985) 4(12):3229–34. doi: 10.1002/j.1460-2075.1985.tb04070.x
76. Epstein MA, Morgan AJ, Finerty S, Randle BJ, Kirkwood JK. Protection of Cottontop Tamarins Against Epstein-Barr Virus-Induced Malignant Lymphoma by a Prototype Subunit Vaccine. Nature (1985) 318(6043):287–9. doi: 10.1038/318287a0
77. Morgan AJ, Epstein MA, North JR. Comparative Immunogenicity Studies on Epstein-Barr Virus Membrane Antigen (Ma) Gp340 With Novel Adjuvants in Mice, Rabbits, and Cotton-Top Tamarins. J Med Virol (1984) 13(3):281–92. doi: 10.1002/jmv.1890130310
78. North JR, Morgan AJ, Thompson JL, Epstein MA. Purified Epstein-Barr Virus Mr 340,000 Glycoprotein Induces Potent Virus-Neutralizing Antibodies When Incorporated in Liposomes. Proc Natl Acad Sci USA (1982) 79(23):7504–8. doi: 10.1073/pnas.79.23.7504
79. Thorley-Lawson DA. A Virus-Free Immunogen Effective Against Epstein-Barr Virus. Nature (1979) 281(5731):486–8. doi: 10.1038/281486a0
80. Rees L, Tizard EJ, Morgan AJ, Cubitt WD, Finerty S, Oyewole-Eletu TA, et al. A Phase I Trial of Epstein-Barr Virus Gp350 Vaccine for Children With Chronic Kidney Disease Awaiting Transplantation. Transplantation (2009) 88(8):1025–9. doi: 10.1097/TP.0b013e3181b9d918
81. Sokal EM, Hoppenbrouwers K, Vandermeulen C, Moutschen M, Leonard P, Moreels A, et al. Recombinant Gp350 Vaccine for Infectious Mononucleosis: A Phase 2, Randomized, Double-Blind, Placebo-Controlled Trial to Evaluate the Safety, Immunogenicity, and Efficacy of an Epstein-Barr Virus Vaccine in Healthy Young Adults. J Infect Dis (2007) 196(12):1749–53. doi: 10.1086/523813
82. Moutschen M, Leonard P, Sokal EM, Smets F, Haumont M, Mazzu P, et al. Phase I/Ii Studies to Evaluate Safety and Immunogenicity of a Recombinant Gp350 Epstein-Barr Virus Vaccine in Healthy Adults. Vaccine (2007) 25(24):4697–705. doi: 10.1016/j.vaccine.2007.04.008
83. Gu SY, Huang TM, Ruan L, Miao YH, Lu H, Chu CM, et al. First Ebv Vaccine Trial in Humans Using Recombinant Vaccinia Virus Expressing the Major Membrane Antigen. Devel Biol Stand (1995) 84:171–7.
84. Dhingra K, Vandana KL. Prophylactic Vaccination Against Periodontal Disease: A Systematic Review of Preclinical Studies. J Periodontol (2010) 81(11):1529–46. doi: 10.1902/jop.2010.100138
85. Rocchi G, Hewetson JF. A Practical and Quantitative Microtest for Determination of Neutralizing Antibodies Against Epstein-Barr Virus. J Gen Virol (1973) 18(3):385–91. doi: 10.1099/0022-1317-18-3-385
86. Speck P, Longnecker R. Epstein-Barr Virus (Ebv) Infection Visualized by Egfp Expression Demonstrates Dependence on Known Mediators of Ebv Entry. Arch Virol (1999) 144(6):1123–37. doi: 10.1007/s007050050574
87. Delecluse HJ, Hilsendegen T, Pich D, Zeidler R, Hammerschmidt W. Propagation and Recovery of Intact, Infectious Epstein-Barr Virus From Prokaryotic to Human Cells. Proc Natl Acad Sci USA (1998) 95(14):8245–50. doi: 10.1073/pnas.95.14.8245
88. Kanda T, Yajima M, Ahsan N, Tanaka M, Takada K. Production of High-Titer Epstein-Barr Virus Recombinants Derived From Akata Cells by Using a Bacterial Artificial Chromosome System. J Virol (2004) 78(13):7004–15. doi: 10.1128/JVI.78.13.7004-7015.2004
89. Sashihara J, Burbelo PD, Savoldo B, Pierson TC, Cohen JI. Human Antibody Titers to Epstein-Barr Virus (Ebv) Gp350 Correlate With Neutralization of Infectivity Better Than Antibody Titers to Ebv Gp42 Using a Rapid Flow Cytometry-Based Ebv Neutralization Assay. Virology (2009) 391(2):249–56. doi: 10.1016/j.virol.2009.06.013
90. Brandtzaeg P. Do Salivary Antibodies Reliably Reflect Both Mucosal and Systemic Immunity? Ann N Y Acad Sci (2007) 1098(1):288–311. doi: 10.1196/annals.1384.012
91. Muhe J, Aye PP, Quink C, Eng JY, Engelman K, Reimann KA, et al. Neutralizing Antibodies Against Epstein-Barr Virus Infection of B Cells Can Protect From Oral Viral Challenge in the Rhesus Macaque Animal Model. Cell Rep Med (2021) 2(7):100352. doi: 10.1016/j.xcrm.2021.100352
92. Miller G. Experimental Carcinogenicity by the Virus In Vivo. In: Epstein MA, Achong BG, editors. The Epstein-Barr Virus. Berlin, Heidelberg: Springer (1979) p. 351–72. doi: 10.1007/978-3-642-67236-1_16
93. Falk L, Deinhardt F, Wolfe L, Johnson D, Hilgers J, de-The G. Epstein-Barr Virus: Experimental Infection of Callithrix Jacchus Marmosets. Int J Cancer (1976) 17(6):785–8. doi: 10.1002/ijc.2910170615
94. Wedderburn N, Edwards JM, Desgranges C, Fontaine C, Cohen B, de The G. Infectious Mononucleosis-Like Response in Common Marmosets Infected With Epstein-Barr Virus. J Infect Dis (1984) 150(6):878–82. doi: 10.1093/infdis/150.6.878
95. de-The G, Dubouch P, Fontaine C, Wedderburn N, Carter RL, Edwards MB, et al. Natural Antibodies to Ebv-Vca Antigens in Common Marmosets (Callithrix Jacchus) and Response After Ebv Inoculation. Intervirology (1980) 14(5-6):284–91. doi: 10.1159/000149198
96. Khan G, Ahmed W, Philip PS, Ali MH, Adem A. Healthy Rabbits Are Susceptible to Epstein-Barr Virus Infection and Infected Cells Proliferate in Immunosuppressed Animals. Virol J (2015) 12:28. doi: 10.1186/s12985-015-0260-1
97. Osborne AJ, Atkins HM, Balogh KK, Brendle SA, Shearer DA, Hu J, et al. Antibody-Mediated Immune Subset Depletion Modulates the Immune Response in a Rabbit (Oryctolagus Cuniculus) Model of Epstein-Barr Virus Infection. Comp Med (2020) 70(5):312–22. doi: 10.30802/AALAS-CM-20-000019
98. Okuno K, Takashima K, Kanai K, Ohashi M, Hyuga R, Sugihara H, et al. Epstein-Barr Virus Can Infect Rabbits by the Intranasal or Peroral Route: An Animal Model for Natural Primary Ebv Infection in Humans. J Med Virol (2010) 82(6):977–86. doi: 10.1002/jmv.21597
99. Kanai K, Takashima K, Okuno K, Kato K, Sano H, Kuwamoto S, et al. Lifelong Persistent Ebv Infection of Rabbits With Eber1-Positive Lymphocyte Infiltration and Mild Sublethal Hemophagocytosis. Virus Res (2010) 153(1):172–8. doi: 10.1016/j.virusres.2010.07.026
100. Reguraman N, Hassani A, Philip P, Khan G. Uncovering Early Events in Primary Epstein-Barr Virus Infection Using a Rabbit Model. Sci Rep (2021) 11(1):21220. doi: 10.1038/s41598-021-00668-x
101. Xia W, Chen H, Feng Y, Shi N, Huang Z, Feng Q, et al. Tree Shrew Is a Suitable Animal Model for the Study of Epstein Barr Virus. Front Immunol (2021) 12:789604. doi: 10.3389/fimmu.2021.789604
102. Wang Z, Yi X, Du L, Wang H, Tang J, Wang M, et al. A Study of Epstein-Barr Virus Infection in the Chinese Tree Shrew(Tupaia Belangeri Chinensis). Virol J (2017) 14(1):193. doi: 10.1186/s12985-017-0859-5
103. Rivailler P, Jiang H, Cho YG, Quink C, Wang F. Complete Nucleotide Sequence of the Rhesus Lymphocryptovirus: Genetic Validation for an Epstein-Barr Virus Animal Model. J Virol (2002) 76(1):421–6. doi: 10.1128/jvi.76.1.421-426.2002
104. Wu L, Hutt-Fletcher LM. Compatibility of the Gh Homologues of Epstein-Barr Virus and Related Lymphocryptoviruses. J Gen Virol (2007) 88(Pt 8):2129–36. doi: 10.1099/vir.0.82949-0
105. Moghaddam A, Rosenzweig M, Lee-Parritz D, Annis B, Johnson RP, Wang F. An Animal Model for Acute and Persistent Epstein-Barr Virus Infection. Science (1997) 276(5321):2030–3. doi: 10.1126/science.276.5321.2030
106. Kutok JL, Klumpp S, Simon M, MacKey JJ, Nguyen V, Middeldorp JM, et al. Molecular Evidence for Rhesus Lymphocryptovirus Infection of Epithelial Cells in Immunosuppressed Rhesus Macaques. J Virol (2004) 78(7):3455–61. doi: 10.1128/jvi.78.7.3455-3461.2004
107. Singh S, Homad LJ, Akins NR, Stoffers CM, Lackhar S, Malhi H, et al. Neutralizing Antibodies Protect Against Oral Transmission of Lymphocryptovirus. Cell Rep Med (2020) 1(3):100033. doi: 10.1016/j.xcrm.2020.100033
108. Wang F. Nonhuman Primate Models for Epstein-Barr Virus Infection. Curr Opin Virol (2013) 3(3):233–7. doi: 10.1016/j.coviro.2013.03.003
109. Coope D, Heston L, Brandsma J, Miller G. Cross-Neutralization of Infectious Mononucleosis and Burkitt Lymphoma Strains of Epstein-Barr Virus With Hyperimmune Rabbit Antisera. J Immunol (1979) 123(1):232–8.
110. Thorley-Lawson DA. Characterization of Cross-Reacting Antigens on the Epstein-Barr Virus Envelope and Plasma Membranes of Producer Cells. Cell (1979) 16(1):33–42. doi: 10.1016/0092-8674(79)90185-5
111. Pearson GR, Qualtiere LF. Papain Solubilization of the Epstein-Barr Virus-Induced Membrane Antigen. J Virol (1978) 28(1):344–51. doi: 10.1128/JVI.28.1.344-351.1978
112. Qualtiere LF, Pearson GR. Epstein-Barr Virus-Induced Membrane Antigens: Immunochemical Characterization of Triton X-100 Solubilized Viral Membrane Antigens From Ebv-Superinfected Raji Cells. Int J Cancer (1979) 23(6):808–17. doi: 10.1002/ijc.2910230612
113. North JR, Morgan AJ, Epstein MA. Observations on the Eb Virus Envelope and Virus-Determined Membrane Antigen (Ma) Polypeptides. Int J Cancer (1980) 26(2):231–40. doi: 10.1002/ijc.2910260216
114. Janz A, Oezel M, Kurzeder C, Mautner J, Pich D, Kost M, et al. Infectious Epstein-Barr Virus Lacking Major Glycoprotein Bllf1 (Gp350/220) Demonstrates the Existence of Additional Viral Ligands. J Virol (2000) 74(21):10142–52. doi: 10.1128/jvi.74.21.10142-10152.2000
115. Ogembo JG, Kannan L, Ghiran I, Nicholson-Weller A, Finberg RW, Tsokos GC, et al. Human Complement Receptor Type 1/Cd35 Is an Epstein-Barr Virus Receptor. Cell Rep (2013) 3(2):371–85. doi: 10.1016/j.celrep.2013.01.023
116. Fingeroth JD, Weis JJ, Tedder TF, Strominger JL, Biro PA, Fearon DT. Epstein-Barr Virus Receptor of Human B Lymphocytes Is the C3d Receptor Cr2. Proc Natl Acad Sci USA (1984) 81(14):4510–4. doi: 10.1073/pnas.81.14.4510
117. Wang HB, Zhang H, Zhang JP, Li Y, Zhao B, Feng GK, et al. Neuropilin 1 Is an Entry Factor That Promotes Ebv Infection of Nasopharyngeal Epithelial Cells. Nat Commun (2015) 6:6240. doi: 10.1038/ncomms7240
118. Backovic M, Longnecker R, Jardetzky TS. Structure of a Trimeric Variant of the Epstein-Barr Virus Glycoprotein B. Proc Natl Acad Sci USA (2009) 106(8):2880–5. doi: 10.1073/pnas.0810530106
119. Chen J, Sathiyamoorthy K, Zhang X, Schaller S, Perez White BE, Jardetzky TS, et al. Ephrin Receptor A2 Is a Functional Entry Receptor for Epstein-Barr Virus. Nat Microbiol (2018) 3(2):172–80. doi: 10.1038/s41564-017-0081-7
120. Zhang H, Li Y, Wang HB, Zhang A, Chen ML, Fang ZX, et al. Ephrin Receptor A2 Is an Epithelial Cell Receptor for Epstein-Barr Virus Entry. Nat Microbiol (2018) 3(2):1–8. doi: 10.1038/s41564-017-0080-8
121. Connolly SA, Jackson JO, Jardetzky TS, Longnecker R. Fusing Structure and Function: A Structural View of the Herpesvirus Entry Machinery. Nat Rev Microbiol (2011) 9(5):369–81. doi: 10.1038/nrmicro2548
122. Hutt-Fletcher LM. Ebv Glycoproteins: Where Are We Now? Future Virol (2015) 10(10):1155–62. doi: 10.2217/fvl.15.80
123. Wang X, Hutt-Fletcher LM. Epstein-Barr Virus Lacking Glycoprotein Gp42 Can Bind to B Cells But Is Not Able to Infect. J Virol (1998) 72(1):158–63. doi: 10.1128/JVI.72.1.158-163.1998
124. Smith NA, Coleman CB, Gewurz BE, Rochford R. Cd21 (Complement Receptor 2) Is the Receptor for Epstein-Barr Virus Entry Into T Cells. J Virol (2020) 94(11):e00428–20. doi: 10.1128/JVI.00428-20
125. Sun C, Chen XC, Kang YF, Zeng MS. The Status and Prospects of Epstein-Barr Virus Prophylactic Vaccine Development. Front Immunol (2021) 12:677027. doi: 10.3389/fimmu.2021.677027
126. Cui X, Snapper CM. Epstein Barr Virus: Development of Vaccines and Immune Cell Therapy for Ebv-Associated Diseases. Front Immunol (2021) 12:734471. doi: 10.3389/fimmu.2021.734471
127. ClinicalTrials.gov[Internet]. A Study of an Epstein-Barr Virus (Ebv) Candidate Vaccine, Mrna-1189, in 18- to 30-Year-Old Healthy Adults. Identifier Nct05164094 (2021). Bethesda (MD: National Library of Medicine (US. Available at: https://ClinicalTrials.gov/show/NCT05164094 (Accessed March 04, 2022).
128. Xiao J, Palefsky JM, Herrera R, Berline J, Tugizov SM. The Epstein-Barr Virus Bmrf-2 Protein Facilitates Virus Attachment to Oral Epithelial Cells. Virology (2008) 370(2):430–42. doi: 10.1016/j.virol.2007.09.012
129. Xiao J, Palefsky JM, Herrera R, Tugizov SM. Characterization of the Epstein-Barr Virus Glycoprotein Bmrf-2. Virology (2007) 359(2):382–96. doi: 10.1016/j.virol.2006.09.047
130. Xiao J, Palefsky JM, Herrera R, Berline J, Tugizov SM. Ebv Bmrf-2 Facilitates Cell-To-Cell Spread of Virus Within Polarized Oral Epithelial Cells. Virology (2009) 388(2):335–43. doi: 10.1016/j.virol.2009.03.030
131. Xiao J, Palefsky JM, Herrera R, Sunshine C, Tugizov SM. Ebv-Positive Human Sera Contain Antibodies Against the Ebv Bmrf-2 Protein. Virology (2009) 393(1):151–9. doi: 10.1016/j.virol.2009.07.025
132. Tugizov S, Herrera R, Veluppillai P, Greenspan J, Greenspan D, Palefsky JM. Epstein-Barr Virus (Ebv)-Infected Monocytes Facilitate Dissemination of Ebv Within the Oral Mucosal Epithelium. J Virol (2007) 81(11):5484–96. doi: 10.1128/JVI.00171-07
133. Gore M, Hutt-Fletcher LM. The Bdlf2 Protein of Epstein-Barr Virus Is a Type Ii Glycosylated Envelope Protein Whose Processing Is Dependent on Coexpression With the Bmrf2 Protein. Virology (2009) 383(1):162–7. doi: 10.1016/j.virol.2008.10.010
134. Loesing JB, Di Fiore S, Ritter K, Fischer R, Kleines M. Epstein-Barr Virus Bdlf2-Bmrf2 Complex Affects Cellular Morphology. J Gen Virol (2009) 90(Pt 6):1440–9. doi: 10.1099/vir.0.009571-0
135. Chesnokova LS, Valencia SM, Hutt-Fletcher LM. The Bdlf3 Gene Product of Epstein-Barr Virus, Gp150, Mediates Non-Productive Binding to Heparan Sulfate on Epithelial Cells and Only the Binding Domain of Cd21 Is Required for Infection. Virology (2016) 494:23–8. doi: 10.1016/j.virol.2016.04.002
136. Quinn LL, Williams LR, White C, Forrest C, Zuo J, Rowe M. The Missing Link in Epstein-Barr Virus Immune Evasion: The Bdlf3 Gene Induces Ubiquitination and Downregulation of Major Histocompatibility Complex Class I (Mhc-I) and Mhc-Ii. J Virol (2016) 90(1):356–67. doi: 10.1128/JVI.02183-15
137. Smith PR, Gao Y, Karran L, Jones MD, Snudden D, Griffin BE. Complex Nature of the Major Viral Polyadenylated Transcripts in Epstein-Barr Virus-Associated Tumors. J Virol (1993) 67(6):3217–25. doi: 10.1128/JVI.67.6.3217-3225.1993
138. Brisse M, Vrba SM, Kirk N, Liang Y, Ly H. Emerging Concepts and Technologies in Vaccine Development. Front Immunol (2020) 11(2578):583077. doi: 10.3389/fimmu.2020.583077
139. Kanekiyo M, Ellis D, King NP. New Vaccine Design and Delivery Technologies. J Infect Dis (2019) 219(Suppl_1):S88–96. doi: 10.1093/infdis/jiy745
140. ClinicalTrials.gov[Internet]. Safety and Immunogenicity of an Epstein-Barr Virus (Ebv) Gp350-Ferritin Nanoparticle Vaccine in Healthy Adults With or Without EBV Infection. Identifier Nct04645147 (2020). Bethesda (MD: National Library of Medicine (US. Available at: https://ClinicalTrials.gov/show/NCT04645147 (Accessed March 04, 2022).
141. Chackerian B. Virus-Like Particles: Flexible Platforms for Vaccine Development. Expert Rev Vaccines (2007) 6(3):381–90. doi: 10.1586/14760584.6.3.381
142. Schweneker M, Laimbacher AS, Zimmer G, Wagner S, Schraner EM, Wolferstatter M, et al. Recombinant Modified Vaccinia Virus Ankara Generating Ebola Virus-Like Particles. J Virol (2017) 91(11):e00343-17. doi: 10.1128/JVI.00343-17
143. Bridge SH, Sharpe SA, Dennis MJ, Dowall SD, Getty B, Anson DS, et al. Heterologous Prime-Boost-Boost Immunisation of Chinese Cynomolgus Macaques Using DNA and Recombinant Poxvirus Vectors Expressing Hiv-1 Virus-Like Particles. Virol J (2011) 8:429. doi: 10.1186/1743-422X-8-429
144. Schmeisser F, Adamo JE, Blumberg B, Friedman R, Muller J, Soto J, et al. Production and Characterization of Mammalian Virus-Like Particles From Modified Vaccinia Virus Ankara Vectors Expressing Influenza H5n1 Hemagglutinin and Neuraminidase. Vaccine (2012) 30(23):3413–22. doi: 10.1016/j.vaccine.2012.03.033
145. Lee CS, Bishop ES, Zhang R, Yu X, Farina EM, Yan S, et al. Adenovirus-Mediated Gene Delivery: Potential Applications for Gene and Cell-Based Therapies in the New Era of Personalized Medicine. Genes Dis (2017) 4(2):43–63. doi: 10.1016/j.gendis.2017.04.001
146. Muhlebach MD. Vaccine Platform Recombinant Measles Virus. Virus Genes (2017) 53(5):733–40. doi: 10.1007/s11262-017-1486-3
147. Garcia F, Bernaldo de Quiros JC, Gomez CE, Perdiguero B, Najera JL, Jimenez V, et al. Safety and Immunogenicity of a Modified Pox Vector-Based Hiv/Aids Vaccine Candidate Expressing Env, Gag, Pol and Nef Proteins of Hiv-1 Subtype B (Mva-B) in Healthy Hiv-1-Uninfected Volunteers: A Phase I Clinical Trial (Risvac02). Vaccine (2011) 29(46):8309–16. doi: 10.1016/j.vaccine.2011.08.098
148. Taylor GS, Jia H, Harrington K, Lee LW, Turner J, Ladell K, et al. A Recombinant Modified Vaccinia Ankara Vaccine Encoding Epstein-Barr Virus (Ebv) Target Antigens: A Phase I Trial in Uk Patients With Ebv-Positive Cancer. Clin Cancer Res (2014) 20(19):5009–22. doi: 10.1158/1078-0432.CCR-14-1122-T
149. Hui EP, Taylor GS, Jia H, Ma BB, Chan SL, Ho R, et al. Phase I Trial of Recombinant Modified Vaccinia Ankara Encoding Epstein-Barr Viral Tumor Antigens in Nasopharyngeal Carcinoma Patients. Cancer Res (2013) 73(6):1676–88. doi: 10.1158/0008-5472.CAN-12-2448
150. Chaudhary N, Weissman D, Whitehead KA. Mrna Vaccines for Infectious Diseases: Principles, Delivery and Clinical Translation. Nat Rev Drug Discovery (2021) 20(11):817–38. doi: 10.1038/s41573-021-00283-5
151. Brooks SA. Appropriate Glycosylation of Recombinant Proteins for Human Use - Implications of Choice of Expression System. Mol Biotechnol (2004) 28(3):241–55. doi: 10.1385/Mb:28:3:241
152. Clausen H, Wandall HH, Steentoft C, Stanley P, Schnaar RL. Glycosylation Engineering. In: Varki A, Cummings RD, Esko JD, Stanley P, Hart GW, et al, editors. Essentials of Glycobiology [Internet]. Cold Spring Harbor (NY): Cold Spring Harbor Laboratory Press (2017) p. 713–28. doi: 10.1101/glycobiology.3e.056
153. Randle BJ, Morgan AJ, Stripp SA, Epstein MA. Large-Scale Purification of Epstein-Barr Virus Membrane Antigen Gp340 With a Monoclonal Antibody Immunoabsorbent. J Immunol Methods (1985) 77(1):25–36. doi: 10.1016/0022-1759(85)90180-2
154. Metz B, van den Dobbelsteen G, van Els C, van der Gun J, Levels L, van der Pol L, et al. Quality-Control Issues and Approaches in Vaccine Development. Expert Rev Vaccines (2009) 8(2):227–38. doi: 10.1586/14760584.8.2.227
155. Kim J, Bu W, Mine S, Tariq Z, Nguyen H, Wang Y, et al. Epstein-Barr Virus (Ebv) Hyperimmune Globulin Isolated From Donors With High Gp350 Antibody Titers Protect Humanized Mice From Challenge With Ebv. Virology (2021) 561:80–6. doi: 10.1016/j.virol.2021.06.006
Keywords: Epstein-Barr virus, infectious mononucleosis, cancer, prophylactic vaccine, glycoprotein, neutralizing antibody, herpesvirus, pre-clinical
Citation: Escalante GM, Mutsvunguma LZ, Muniraju M, Rodriguez E and Ogembo JG (2022) Four Decades of Prophylactic EBV Vaccine Research: A Systematic Review and Historical Perspective. Front. Immunol. 13:867918. doi: 10.3389/fimmu.2022.867918
Received: 01 February 2022; Accepted: 11 March 2022;
Published: 14 April 2022.
Edited by:
Georges Michel Verjans, Erasmus Medical Center, NetherlandsReviewed by:
Jaap M. Middeldorp, VU Medical Center, NetherlandsJosef Mautner, Technical University of Munich, Germany
Copyright © 2022 Escalante, Mutsvunguma, Muniraju, Rodriguez and Ogembo. This is an open-access article distributed under the terms of the Creative Commons Attribution License (CC BY). The use, distribution or reproduction in other forums is permitted, provided the original author(s) and the copyright owner(s) are credited and that the original publication in this journal is cited, in accordance with accepted academic practice. No use, distribution or reproduction is permitted which does not comply with these terms.
*Correspondence: Javier Gordon Ogembo, am9nZW1ib0Bjb2gub3Jn