- 1Genc Laboratory, Izmir Biomedicine and Genome Center, Izmir, Turkey
- 2Izmir International Biomedicine and Genome Institute, Dokuz Eylul University, Izmir, Turkey
- 3Department of Neuroscience, Health Sciences Institute, Dokuz Eylul University, Izmir, Turkey
The NLRP3 inflammasome is an intracellular multiprotein complex that plays an essential role in the innate immune system by identifying and eliminating a plethora of endogenous and exogenous threats to the host. Upon activation of the NLRP3 complex, pro-inflammatory cytokines are processed and released. Furthermore, activation of the NLRP3 inflammasome complex can induce pyroptotic cell death, thereby propagating the inflammatory response. The aberrant activity and detrimental effects of NLRP3 inflammasome activation have been associated with cardiovascular, neurodegenerative, metabolic, and inflammatory diseases. Therefore, clinical strategies targeting the inhibition of the self-propelled NLRP3 inflammasome activation are required. The transcription factor Nrf2 regulates cellular stress response, controlling the redox equilibrium, metabolic programming, and inflammation. The Nrf2 pathway participates in anti-oxidative, cytoprotective, and anti-inflammatory activities. This prominent regulator, through pharmacologic activation, could provide a therapeutic strategy for the diseases to the etiology and pathogenesis of which NLRP3 inflammasome contributes. In this review, current knowledge on NLRP3 inflammasome activation and Nrf2 pathways is presented; the relationship between NLRP3 inflammasome signaling and Nrf2 pathway, as well as the pre/clinical use of Nrf2 activators against NLRP3 inflammasome activation in disorders of the central nervous system, are thoroughly described. Cumulative evidence points out therapeutic use of Nrf2 activators against NLRP3 inflammasome activation or diseases that NLRP3 inflammasome contributes to would be advantageous to prevent inflammatory conditions; however, the side effects of these molecules should be kept in mind before applying them to clinical practice.
Neuroinflammation and Inflammasomes
Neuroinflammation, a term used to define a wide variety of innate and adaptive immune responses within the brain and the spinal cord, contributes greatly to the pathogenesis of acute and chronic central nervous system (CNS) disorders (1). In the CNS, the primary players of innate immunity are microglia, astrocytes, and trafficking macrophages (2). These cells interact with their surrounding environment and recognize a wide range of endogenous and exogenous stimuli. These stimuli could vary from cytokines, growth factors, chemokines, adenosine triphosphate (ATP) to pathogenic signals. Among these stimuli, inflammatory signals could be grouped as Danger-Associated Molecular Patterns (DAMPs), Pathogen-Associated Molecular Patterns (PAMPs), or Homeostasis-Altering Molecular Processes (HAMPs) (3). Of note, Liston and Master recently suggested the term “HAMPs” to describe the functional consequences of pathogens on cellular processes rather than simple molecular patterns. HAMPs sense loss of cellular homeostasis of cells and initiate immune responses with or without DAMPs or PAMPs. Alteration of homeostasis within the cells, such as low potassium levels, reduced fatty acid oxidation amino acid starvation, and loss of pyrin phosphorylation, could be an inducer of HAMPs. These signals, PAMPs, DAMPs, and HAMPs, are detected by pattern recognition receptors (PRRs) which are primarily localized on innate immune cells of the CNS, especially microglia (4). The nucleotide-binding domain and leucine-rich repeat-containing receptors (NLRs) and absent in melanoma 2 (AIM2)-like receptors (ALRs), a group of cytosolic PRRs, recognize intracellular signals and cause immune response to sustain homeostasis. During this process, these receptors are involved in the formation of multiprotein complexes called inflammasomes. Once formed and activated, inflammasomes trigger proteolytic cleavage and release of pro-inflammatory cytokines, such as interleukin (IL)-1β and IL-18, and further pyroptotic cell death (5). Among all inflammasome complexes, NLR Family Pyrin Domain Containing Protein 3 (NLRP3) inflammasome is the most studied and best-characterized, as it is involved in both pathogenic and sterile inflammation activated by a wide range of signals (6).
Structure of NLRP3 Inflammasomes
NLRP3 inflammasome is an essential component of the innate immune system, which provides defense against infections caused by bacteria, fungi, and viruses (7, 8). The NLRP3 inflammasome also recognizes DAMPs and HAMPs such as ATP, uric acid crystals, silica, asbestos, alum, and protein deposits. The NLRP3 multiprotein complex consists of three components, namely, the sensor protein NLRP3, an adaptor protein apoptosis-associated speck-like protein containing a CARD domain (ASC), and an effector pro-caspase-1 protein. There are three domains in the NLRP3 protein, that is, the NACHT domain, C-terminal leucine-rich repeats (LRRs), and the N-terminal pyrin domain (PYD) (9). It has been shown that the NACHT domain has ATPase activity which is necessary for oligomerization of NLRP3 (10). The LRR domain has both signal recognition and autoinhibition functions. The PYR domain of NLRP3 binds to the adaptor protein ASC via PYR-PYR interaction.
The Mechanisms of NLRP3 Inflammasome Activation
The activation of the NLRP3 inflammasome complex is mediated by the canonical, the non-canonical, and the alternative pathways. The canonical pathway proposes a two-step activation model, the priming and the activation steps (11). The first step primes cells to induce an immune response since the intracellular levels of pro-inflammatory cytokine IL-1β and NLRP3 are insufficient to activate the inflammasome complex. The priming stage can be triggered by ligands of Toll-like receptors (TLRs) such as lipopolysaccharide (LPS), cytokines, as well as NLRs such as NOD1 and NOD2. These stimuli lead to the activation of the TLR4 receptor, which further translocates Nuclear Factor Kappa B (NF-κB) into the nucleus and initiates transcription of NLRP3 and pro-inflammatory cytokines, including pro-IL-1β (12). Recent studies suggested that in the priming step, NLRP3 protein undergoes post-translational modification such as phosphorylation and ubiquitination. JNK1-mediated S194 phosphorylation of NLRP3 is necessary for self-association and inflammasome assembly (13). In the activation step, NLRP3 recognizes various danger signals (PAMPs, DAMPs) or events causing perturbations in homeostasis, such as lysosomal disruption and mitochondrial dysfunction. These result in conformational change and oligomerization of the sensor protein NLRP3, subsequently leading to its interaction with adaptor proteins via its PYD domain. After that, the NLRP3-ASC complex recruits pro-caspase-1 via the CARD domain. The activated caspase-1 cleaves pro-IL-1β and pro-IL-18 (14). Furthermore, caspase-1 cleaves a protein called Gasdermin D (GSDMD), resulting in inflammatory programmed cell death called pyroptosis. Once cleaved, GSDMD yields two different fragments, namely C-terminal and N-terminal GSDMDs. Self-assembled N-terminal GSDMDs create pores on the plasma membrane leading to the release of the pro-inflammatory cytokines IL-1β and IL-18, loss of cellular integrity, and eventually, inflammatory cell death called pyroptosis (15).
Besides the caspase-1-mediated canonical pathway, two different pathways also mediate NLRP3 inflammasome activation. The first one, the non-canonical pathway, is mediated by catalytic activities of caspase 11 and its human homologs caspase-4 and caspase-5 (16, 17). In the non-canonical NLRP3 inflammasome activation model, TLRs are stimulated by LPS and activate NF-κB and type I interferon production, which causes the expression of caspase‐4/5/11 via the JAK/STAT pathway (18). Type I interferons also activate guanylate-binding proteins (GBPs) and eventually contribute to the autocatalytic activation of caspase-4/5/11 (19). Caspase-4/5/11 -mediated cleavage of GSDMD induces K+ efflux-dependent canonical activation of NLRP3 inflammasome complex (20). The second pathway, called alternative activation, only occurs in human and porcine monocytes. This activation pathway, unlike the others, does not depend on potassium efflux, formation of ASC speck, or pyroptotic cell death. The effector caspase-8 is induced in the TLR4–TRIF–RIPK1–FADD axis. Gaidt and colleagues demonstrated that this alternative activation pathway does not end with pyroptotic cell death (21).
Regulation of NLRP3 Inflammasome Activation
Regulation of NLRP3 inflammasome activation can be achieved at many different levels, DNA level, transcriptional, post-transcriptional, translation, and post-translation levels. DNA methylation and histone acetylation are two mechanisms that have been shown to participate in NLRP3 regulation at the DNA level. Promoter hypomethylation of NLRP3 activates the NLRP3 inflammasome, and Histone deacetylase 6 (HDAC6) regulates NLRP3inflammasome via directly binding NLRP3 at the ubiquitin-binding domain (22). Aryl hydrocarbon receptor (AhR) (23), B-cell lymphoma 6 (BCL6) (24), cAMP-PKA signaling (25), AMPK-GSK3β-Nrf2 signaling (26), and Rev-erbα (27) inhibit NLRP3 inflammasome-mediated regulation at the transcriptional level. On the other hand, FADD-caspase-8 signaling pathway (28), TLR signaling pathways including TLR4/6-IRAK4/1 (29) and TLR4/Myd88/NF-κB (30), mTOR signaling pathways such as NF-κB/mTOR (31), MAPK signaling pathways like ROS/TXNIP/MAPK (32), and NF-κB/MAPK (33) activate NLRP3 inflammasome at the transcriptional level. Numerous non-coding RNA products, namely microRNAs (miRNAs) and long non-coding RNAs (lncRNAs) can regulate NLRP3 inflammasome expression at the post-transcriptional level by complementary binding to their target NLRP3 gene. Several miRNAs such as miR-7 (34), miR-22 (35), miR-30e (36), miR-133b (37), miR-223 (38) were found to block NLRP3 inflammasome. LncRNAs represent another type of non-coding RNAs that regulate the expression of their target genes. Some well-studied lncRNAs such as NEAT1 (39), Meg3 (40), HOTAIR (41), MALAT1 (42), NLRP3 (43) were shown to promote NLRP3 inflammasome activation. On the contrary, several lncRNAs like XIST (44), GAS5 (45), and SNHG7 (46) were found to inhibit NLRP3 inflammasome activation at the post-transcriptional level. Post-translational modifications are the enzymatic changes that proteins undergo to convert into a mature form. Such modifications include ubiquitination, phosphorylation, SUMOylation, alkylation, and nitrosylation. Ubiquitination of NLRP3 is accomplished by several E3 ligases and deubiquitinating enzymes including BRCC3 and BRCC36 deubiquitinate (47) and ARIH2 (48), CUL1 (49), FBXL2 (50), and TRIM31 (51) ubiquitinate NLRP3 protein. Phosphorylation by JNK1 (13), dephosphorylation by PTPN22 (52), and PP2A (53) activate the NLRP3 inflammasome. The addition of a Small Ubiquitin-like Modifier (SUMO) is another post-translational modification that helps to regulate transcription, responses to DNA damage, and hypoxic response. UBC9, a Sumo-conjugating enzyme, was shown to activate SUMO1 to SUMOylate NLRP3 and activate it while SUMO-specific protease 3 (SENP3) deSUMOylate NLRP3 for deactivation (54).
NLRP3 Inflammasome Activation in CNS Disorders
There are many diseases of the CNS to which NLRP3 inflammasome activation is linked and involved in their etiology and pathogenesis. The activation of the NLRP3 inflammasome in both acute and chronic CNS disorders including Alzheimer’s disease (AD) (55), Parkinson’s disease (PD) (56), amyotrophic lateral sclerosis (ALS) (57), multiple sclerosis (MS) (58), neuropsychiatric diseases such as depression, schizophrenia (59), stroke, traumatic brain and spinal cord injuries (60) has been extensively studied.
AD is the most common progressive neurodegenerative disorder of the CNS, characterized by abnormal accumulation of β-amyloid plaques in the extracellular space and hyperphosphorylation of tau protein in cytoplasmic neurofibrillary tangles. Previous studies have demonstrated that both hyperphosphorylation of tau (61) and aggregation of β-amyloid peptides (62) caused activation of the NLRP3 inflammasome in microglia. Evidently, a recent postmortem study demonstrated the increased expression of ASC, caspase-1, and IL-1β in the cerebral cortex of AD patients (63). NLRP3 inflammasome is also activated in PD by α-Synuclein deposits acting as DAMPs to activate the microglial NLRP3 inflammasome. Clustered α-Synuclein leads to mitochondrial impairment, Reactive Oxygen Species (ROS) production, enhanced Cathepsin B activity, and the NLRP3 inflammasome complex formation. Furthermore, the use of genetic and MPTP-induced PD models has revealed the involvement of the microglial NLRP3 inflammasome in PD pathogenesis (34, 64–66). NLRP3 inflammasome activation by known inflammasome activators, including nigericin, aluminum potassium sulfate crystals, bacterial LPS, and vitamin K3 (menadione), leads to truncation and aggregation of α-Syn by caspase-1 in a neuronal cell model of PD (67).
Preclinical studies have demonstrated that the inhibition of NLRP3 reduces behavioral outcomes and abnormal protein accumulation associated with neurodegenerative diseases, suggesting that targeting NLRP3 inflammasome could represent a novel therapeutic approach for neurodegenerative disorders. Evaluation of the efficacy, safety, and tolerability of specific NLRP3 inflammasome inhibitors should be completed. These inhibitors could indirectly inhibit NLRP3 inflammasome activation via distinct mechanisms like obstruction of K+ efflux (Glyburide, BHB), hampering oligomerization, or binding of ASC (16673-34-0, BHB), and preventing auto-cleavage of pro-caspase-1 (FC11A-2) (68). They also can act as a direct inhibitor of inflammasome components; Caspase-1 (VX-740, VX-765, Parthenolide) or NLRP3 itself (MCC950, MNS, Oridonin, Parthenolide, OLT1177, CY-09) (68).Clinical studies on the efficacy, safety, and tolerability of these specific NLRP3 inflammatory inhibitors have started (VX-740, VX-765, MCC950, Oridonin), and the results of these studies are awaited for the application of specific NLRP3 inhibitors (69, 70). Although these developments positively impact novel therapeutic approaches, inflammasomes are part of the innate immune system and required for a proper defense system might limit their use in the future. Nevertheless, it may be possible to use naturally derived drug substances such as Nrf2 activators that inhibit NLRP3 inflammasome activation in inflammatory conditions or sterile inflammasome activation.
Nrf2/KEAP1 Signaling Pathway
Maintaining homeostasis is indispensable to an organism’s health and survival. Environmental stressors are present everywhere and are meant to disrupt cell functions. Organisms respond and adapt to these stresses through defined regulatory mechanisms. The transcription factor nuclear factor erythroid 2-related factor 2 (Nrf2 or Nfe2l2) is a basic leucine zipper (bZIP) that belongs to the Cap ‘N’ Collar (CNC) family, and with its cytoplasmic repressor Kelch-like ECH-associated protein 1 (KEAP1), are the master regulators of redox homeostasis in the cells (71). Under non-stress conditions, Ntrf2 is localized in the cytoplasm; after activation, the nuclear translocation of Nrf2 is dependent on the balance of nuclear import and export signals. Nrf2 contains three nuclear localization sequences and two nuclear export sequences. Redox-sensitive signal leads to Nrf2 nuclear accumulation via blocking the interaction between nuclear exportin and nuclear export sequences. Heterodimerization of Nrf2 with Maf protein promotes nuclear retention of Nrf2. The heterodimer of Nrf2-Maf plays a master regulatory role against stress conditions by activating antioxidant-responsive element (ARE) or electrophile-responsive element (EpRE) containing genes. As one of the essential tasks of Nrf2 is to provide the necessary anti-oxidative response, it can be considered as a pioneer cell survival tool. Therefore, Nrf2 can be found dysregulated or disrupted in many diseases such as metabolic diseases, neurodegenerative diseases, aging, inflammatory diseases, cancer, and cardiovascular diseases (72).
Nrf2/KEAP1 Structure
Nrf2 has seven Nrf2-ECH homology regions, Neh1–7, each performing different functions. Neh1 contains the CNC-bZIP region required for DNA binding and association with small musculoaponeurotic fibrosarcoma (sMaf) proteins, which are partners of Nrf2 dimerization (73). Neh2 contains highly conserved DLG and ETGE motifs involved in the interaction with the Nrf2 cytoplasmic repressor KEAP1 and seven lysine residues that serve as ubiquitylation targets (74). The C-terminal Neh3 domain, which has transcriptional activity, co-operates with Neh4 and Neh5 to upregulate Nrf2 target genes (75). Neh4 and Neh5 recruit the transcriptional co-activators CREB-binding protein (CBP) and the repressor-associated coactivator (RAC). The serine-rich Neh6 domain participates in the KEAP1-independent removal of Nrf2 through binding to the β-transducin repeat-containing protein (76). Neh7 domain suppresses Nrf2 activity by interacting with the retinoic X receptor α (77).
KEAP1 is a substrate adaptor molecule for Cul3-containing E3 ubiquitin ligase which interacts with Nrf2 and downregulates Nrf2. KEAP1 has five domains, namely N-terminal region (NTR), Broad complex Tramtrack and Bric-à-Brac (BTB) domain, intervening region (IVR), Kelch domain/double glycine repeat (DGR), and C-terminal region (CTR) (78). The DGR domain binds to DLG (latch) and the ETGE (hinge) domains of Nrf2; the IVR domain, containing specific cysteine residues, facilitates KEAP1-dependent Nrf2 ubiquitination; the BTB domain, known as homodimerization domain, also binds to Cul3-Rbx1 ligase.
Nrf2 Activation and Regulation
Regulation of Nrf2 activation occurs at the transcriptional and post-transcriptional levels and the post-translational level via modification of protein stability and binding partners. KEAP1 protein is responsible for mediating the first and most important mode of regulation of Nrf2 activation. Under homeostatic conditions, two KEAP1 molecules bind to Nrf2 via the ETGE and DLG motifs to the Neh2 domain (79). KEAP1 functions as an adapter protein for the Cul3 E3 ubiquitin ligase, responsible for the sustained ubiquitin addition and degradation of Nrf2 (79). GSK-3 regulates Nrf2 activity by phosphorylating Nrf2 at the Neh6 domain, leading to recruitment of the ubiquitin ligase adapter β-TrCP and initiation of proteasomal degradation of Nrf2 by a Cullin1/Rbx1 complex. KEAP1-interacting region (KIR)-like ETGE motif-containing proteins such as dipeptidyl peptidase 3 (DPP3), partner and localizer of BRCA2 (PALB2), and SQSTM1/p62 contribute to Nrf2 stabilization via the non-canonical mechanism.
Nrf2 is activated by KEAP1-dependent and independent mechanisms. In the former mechanism, when oxidative or electrophilic stress occurs in the cell, KEAP1 is oxidized at the reactive cysteine residues, resulting in its conformational change, which in turn initiates Nrf2 dissociation from KEAP1 (79). The mechanism of Nrf2’s detachment from KEAP1 has not been fully elucidated. Two different models have been proposed to explain this mechanism, i) the hinge and latch model and ii) the quaternary model. Inhibition of GSK3-dependent phosphorylation of the Nrf2 domain, Hrd1-and WDR23-mediated ubiquitination constitute the KEAP1-independent regulation mechanisms of Nrf2 activity. The phosphorylation of Nrf2 by kinases including PKC, MAPK, PI3K, and AMPK regulates nuclear translocation and activity of Nrf2.
Nrf2 gene transcription is controlled by various transcription factors such as aryl hydrocarbon receptor (AhR) (80) and NF-κB (81). The promoter region of Nrf2 also contains an ARE-like sequence which contributes to the autoregulation of Nrf2 activity (82). Recent findings supporting that the expression of Nrf2 is epigenetically suppressed by promoter methylation still need further confirmation (83).
Nrf2 activity can be regulated at the post-transcriptional level by miRNAs and lncRNAs. In an earlier -study, miR-144, the level of which is increased in sickle cell anemia, was shown to modulate oxidative stress tolerance by targeting Nrf2 (84). Later, many miRNAs, including miR-34, miR-27a, miR-142-5p, and miR-153, were shown to participate in the regulation of Nrf2 expression by targeting Nrf2 or KEAP1 (85). As functional gene regulators, lncRNAs may also participate in the epigenetic regulation of Nrf2 activity. MALAT, ROR, ODRUL, and Nrf2-lncRNA are some of the lncRNAs shown to contribute to Nrf2 regulation in different tissues” (86). It has been shown that Nrf2 expression in human cancers can be regulated by alternative splicing. For example, alternative splicing of the Nrf2 gene disrupts the KEAP1/Nrf2 interaction, thereby leading to enhanced Nrf2 activity (87).
The Interplay Between NLRP3 Inflammasome and Nrf2
To develop new strategies based on Nrf2 inducers to target NLRP3 inflammasome activation in different conditions, the cross-talk between NLRP3 inflammasome and Nrf2 signaling pathways must be well acknowledged. The main link between NLRP3 and Nrf2 signaling pathways is their ability to respond to oxidative stress/ROS formation. It is known that ROS accumulation disrupts redox homeostasis and acts as an upstream signal for both NLRP3 inflammasome and Nrf2 activation due to the ROS-generated oxidative stress (88). The NLRP3 inflammasome is activated by a protein called thioredoxin-interacting protein (TXNIP), which negatively regulates the anti-oxidative thioredoxin system. ROS formation leads to binding of TXNIP to the NLRP3 and subsequent conformational change and assembly of the NLRP3 inflammasome complex (89). On the other hand, the presence of ROS or free radicals derived by ROS liberates Nrf2 from its stabilized complex with KEAP1 and causes translocation of Nrf2 to the nucleus, thereby activating transcription of detoxifying genes.
There is also a link between Nrf2 and the transcription factor NF-κB which is an upstream signaling pathway required for the priming step of NLRP3 inflammasome activation (Figure 1). Several studies have reported the anti-inflammatory role of Nrf2 in NF-κB-mediated inflammation (90–92). At this point, the role of HO-1, a Nrf2 target gene, is significant. HO-1 processes heme yielding carbon monoxide, free Fe++, and biliverdin which is catalyzed into bilirubin (93). Free Fe++ and bilirubin inhibited NF-κB activity and the production of pro-inflammatory cytokines TNF-α and IL-1β. Along with HO-1, another Nrf2 target gene, NQO1, reduced NLRP3 inflammasome activation (94). Of note, in a study by Kobayashi et al., it was shown, with the usage of ChIp-Seq technology, that Nrf2 regulates the transcription of IL-6 and IL-1β by binding to the promoter regions of these NF-κB-mediated pro-inflammatory cytokines (95). Importantly, inhibition of transcription of pro-inflammatory cytokines by Nrf2 is achieved by preventing any interaction of RNA Pol II with the NF-κB complex. Along with Nrf2, KEAP1 also plays a regulatory role in NF-κB activity. After Nrf2 disassociation, KEAP1 ubiquitinates the IκB kinase β, leading to its degradation, prevention of phosphorylation of the NF-κB complex, and subsequent inhibition of NF-κB activity (96). Furthermore, the activity of NF-κB induces the release of some secondary inflammatory mediators such as COX2. A COX2 derived product, 15d-PGJ2, inhibits NF-κB via PPARγ (97, 98) and target cysteine residues of KEAP1 via its electrophilic structure (99). Lastly, there is also an inhibitory interaction between the Nrf2 and NF-κB signaling pathways. In particular, the activation of NF-κB leads to translocation of its subunits p65 and p50 to the nucleus, then p65 antagonizes with a transcriptional cofactor of Nrf2, CBP, which further prevents Nrf2 binding to and transcription of its target genes (100).
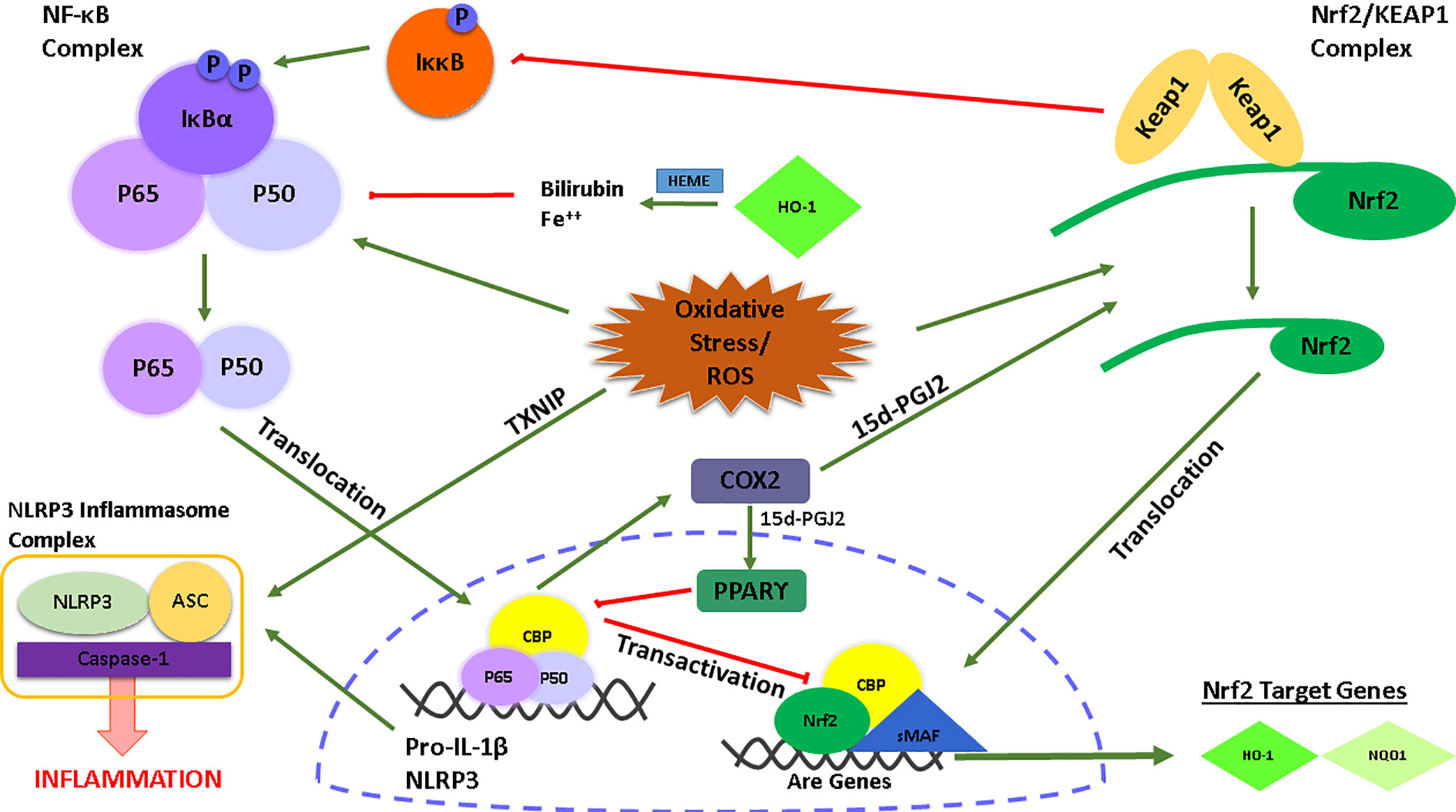
Figure 1 Graphical illustration of the interconnection between the NLRP3/NF-κB signaling and the Nrf2 signaling pathway.
Although the protective and inhibitory effects of Nrf2 on NLRP3 inflammasome activation have been investigated (94), it has also been demonstrated the necessity of Nrf2 for appropriate activation of the NLRP3 inflammasome complex. In a study by Freigang and coworkers, it was shown that Nrf2 positively regulates NLRP3 inflammasome activation and exacerbates atherosclerosis in mice fed with a high-cholesterol diet. Furthermore, Nrf2 deficiency in the diet-induced atherosclerosis model alleviated the production of IL-1β (101). In parallel, Zhao et al. reported the essential role of Nrf2 in both NLRP3 and AIM2 inflammasome activation (102). In this study, Nrf2 was required to sufficiently activate the NLRP3 inflammasome complex and the IL-1β/caspase-1 processing. The deficiency of the Nrf2 gene also negatively affected the ASC speck formation, another indicator of the NLRP3 inflammasome activation.
Nrf2 Inducers
Nrf2 activators could be categorized as electrophiles, protein-protein interaction (PPI) inhibitors, and multi-target drugs. Electrophiles are electrophilic molecules that alter cysteine residues of the KEAP1 protein by certain modifications like oxidation. Some electrophiles are triterpenoids like bardoxolone-methyl and RTA-408 (103), fumaric acid esters such as dimethyl fumarate (DMF) (104, 105), and monomethyl fumarate (106), organosulfurs such as oltipraz (107) and biliary acids, ursodiol (108), natural compounds including curcumin (109), resveratrol (110), quercetin (111), sulforaphane (SFN) (112), and melatonin (113). PPIs block the physical interaction of Nrf2 with KEAP1, thus activating Nrf2 and offering more selectivity compared to electrophiles like Tetrahydroisoquinoline (114), thiopyrimidine (115), naphthalene (116). One KEAP1 independent activator is GSK-3; it phosphorylates Nrf2, and thus, E3 ligase β-TrCP ubiquitinates phosphorylated Nrf2, so it is degraded. Therefore, GSK-3 inhibitors have the potential to activate Nrf2; one example is tideglusib (117). Moreover, HRD1 is an E3 ubiquitin ligase that contributes to Nrf2 degradation in a KEAP1-independent manner; LS-102 is another HRD1 inhibitor (118). There are two classes of Nrf2 inhibitors, the agonists of nuclear receptors and the natural compounds. Regarding the agonists of nuclear receptors, glucocorticoid receptor ligands like Dexamethasone (119) and clobetasol propionate (120) suppress Nrf2 transcription. Additionally, agonists of the retinoic acid receptor-α and retinoid X receptor-α, i.e., bexarotene and trans-retinoic acid, display the same effect and block Nrf2 transcription (121, 122). Several natural compounds were shown to repress Nrf2, including flavonoids luteolin (123) and wogonin (124), ascorbic acid (125), mycotoxin ochratoxin A (126), camptothecin (127), halofuginone (128), and coffee alkaloid trigonelline (129).
Preclinical Experience With Nrf2 Inducers Against NLRP3 Inflammasome Activation in CNS Disorders
Due to their anti-oxidative and anti-inflammatory nature, Nrf2 inducers have attracted attention for inhibiting NLRP3 inflammasome activation in numerous NLRP3 inflammasome-related CNS disorders, especially the natural compounds that have been studied comprehensively. SFN, an isothiocyanate, was proved to inhibit NLRP3 inflammasome by upregulating Nrf2 in murine microglial cells (130). Isoliquiritigenin, a phenolic compound obtained from licorice, was shown to heal cognitive impairment (131) and early brain injury (132) by blocking NLRP3 inflammasome through Nr2 upregulation. In another study on the early brain injury model, mangiferin, a glucoside of norathyriol found in mango trees, was also proved to exert neuroprotective effects through Nrf2/HO-1 and NLRP3 pathways regulation (133). Furthermore, Wang et al. showed that Dl‐3‐n‐butylphthalide found in celery oil healed AD‐like pathology and suppressed NLRP3 inflammasome activation through the Nrf2‐TXNIP‐TrX signaling pathway in APP/PS1 mice (134). Celastrol, a pentacyclic triterpene from Tripterygium wilfordi root extract, exhibited neuroprotective properties in a MPTP-induced PD mouse model and AAV-mediated human α-synuclein overexpression in a PD model by inhibiting NLRP3 through the Nrf2 signaling pathway (135). Luteolin, a flavone, exerted cerebroprotection after subarachnoid hemorrhage (136) and neuroprotection after spinal cord ischemia-reperfusion injury (137) by preventing activation of NLRP3 inflammasome via the Nrf2 pathway. Astragaloside IV, a saponin found in Astragalus membranaceus, was found beneficial against cerebral ischemia-reperfusion injury (138), motor deficits, and dopaminergic neuron degeneration in a MPTP PD mouse model (139) via NLRP3/Nrf2 pathway regulation. A meroterpene from the seeds of Psoralea corylifolia, or bakuchiol, displayed similar effects by ameliorating cerebral ischemia-reperfusion injury via NLRP3/Nrf2 pathway (140). Tao et al. indicated that magnalol, biphenolic neolignane found in the bark of Magnolia officinalis, stimulates microglia towards the M2 phenotype to heal depressive-like behaviors through regulating Nrf2/HO-1/NLRP3 axis (141). Bixin, an apocarotenoid in the seeds of the achiote tree, was proved to diminish neuroinflammation and demyelination in an experimental autoimmune encephalomyelitis mouse model via upregulating Nrf2 and suppressing TXNIP/NLRP3 Inflammasome (142). Carvacrol found in the essential oil of oregano upregulates autophagy via the KEAP1/Nrf2/p62 axis and downregulates NLRP3, thereby providing neuroprotection in the unilateral sciatic nerve CCI model (143). Allicin, an organosulfur from garlic, prevented depressive-like behaviors in chronic social defeat stress model mouse by increasing the activities of the superoxide dismutase (SOD) and Nrf2/HO-1 pathways and blocking NLRP3 inflammasome (144). Asiatic acid is a triterpenoid from Centella asiatica and exhibited neuroprotective effects via NLRP3 inflammasome blocking and Nrf2 activation in spinal cord injury model rats (145). Dihydrolipoic acid, a reduced form of lipoic acid, remedied behavioral deficits and neuroinflammation through the Nrf2/HO-1/NLRP3 axis in a LPS rat model (146). Furthermore, saffron extract cured neuroinflammation by stimulating SIRT1, Nrf2, and HMOX1, while decreasing NLRP3 inflammasome activation in a repetitive mild traumatic brain injury model mouse (147). Neopterin, a catabolic product of guanosine triphosphate, blocks NLRP3 inflammasome activation via Nrf2 in human primary astrocytes (148).
Alkaloids are natural compounds produced by many plants, bacteria, fungi. Berberine, an alkaloid found in Berberis species, reduced inflammation through the TXNIP/NLRP3/Nrf2 axis in RAW 264.7 macrophages and rats (149). Another alkaloid, ephedrine, derived from plants of the Ephedra genus, was demonstrated to attenuate cerebral ischemia injury in the middle cerebral artery occlusion rat model and BV2 microglial cells via Akt/GSK3β/Nrf2 pathway regulation and NLRP3 suppression (150). HJ22 is a derivative of piperine, a black pepper alkaloid, heals cognitive impairment and exert cytoprotection through KEAP1/Nrf2/ARE activation and NLRP3 inhibition (151). Another piperine derivative, HJ105, recovered neuroinflammation and oxidative damage in Aβ1-42 AD model rats through KEAP1-Nrf2-TXNIP regulation (152). Other natural products tested against NLRP3 activation are derived from Ginseng species; for example, Ginsenoside Re, which is found in Panax ginseng, was shown to heal cognitive deficits in the chronic restraint stress mouse model by augmenting Nrf2 and suppressing NLRP3 (153). In addition, Pseudoginsenoside-F11 present in American ginseng restored cognitive impairment in APP/PS1 mice by regulating the Nrf2/ARE/NLRP3 pathway (154).
In addition to the abovementioned natural compounds, several hormones were demonstrated to have beneficial effects. Melatonin is a circadian rhythm regulatory enzyme with additional anti-inflammatory, antioxidant, anti-cancer functions (155). Melatonin was shown to activate Nrf2/SIRT1 against NLRP3 in murine microglial cells (113). Another study showed that melatonin enhanced brain function in chronic Gulf War Illness model rats via modulating the NLRP3 inflammasome through the BDNF-ERK-CREB pathway and Nrf2 alteration (156). Another hormone, adiponectin, regulated cerebral ischemia-reperfusion by blocking the NLRP3 inflammasome through regulating AMPK and GSK-3β phosphorylation and Nrf2 translocation (157). Moreover, Cheng et al. indicated that the ghrelin hormone alleviated secondary brain injury by upregulating the Nrf2/ARE pathway and blocking the NLRP3 inflammasome (158). Luo and colleagues found that N-[2-(5-hydroxy-1H-indol-3-yl) ethyl]-2-oxopiperidine-3-carboxamide, a derivative of N-acetylserotonin, prevented NLRP3 inflammasome activation through PI3K/Akt/Nrf2 signaling in a hypoxic-ischemic encephalopathy rat model (159).
Not only natural compounds but also synthetic compounds have been investigated as potential Nrf2 inducers. DMF, a synthetic fumaric acid ester, was found to prevent NLRP3 inflammasome activation through the Nrf2/NF-κB axis in N9 murine microglial cells and a LPS-induced sickness model mouse (105). Moreover, tert-butylhydroquinone (tBHQ), a synthetic phenolic antioxidant, is a well-known Nrf2 inducer; tBHQ-induced Nrf2 blocks NLRP3 inflammasome activation via Trx1/TXNIP in middle cerebral artery occlusion/reperfusion (MCAO/R) model rats (160). In addition, tBHQ abated NLRP3 inflammasome activation by ROS via Nrf2/ARE signaling in oxygen-glucose deprivation/reoxygenation (OGDR) model BV2 microglial cells (161). Moreover, multiple drugs, namely Ezetimibe (162), Ibrutinib (163), Dexmedetomidine (164, 165), Tranilast (166), Probucol (167) and various other synthetic compounds such as sodium butyrate (168), diphenyl diselenide (169), 5-(3,4-Difluorophenyl)-3-(6-methylpyridin-3-yl)-1,2,4-oxadiazole (170) and tert-butylhydroquinone (94, 160, 161), were investigated against NLRP3 inflammasome activation and Nrf2 upregulation in several in vivo and in vitro models.
Clinical Trials With Nrf2 Inducers Against NLRP3 Inflammasome Activation in CNS Disorders
Given that inflammation, particularly NLRP3 inflammasome activation, is considered as one of the factors underlying disorders in CNS, the use of Nrf2 inducers and modulation of NLRP3 inflammasome activation could be an effective therapeutic approach against inflammatory conditions. The studies revealed that the Nrf2 signaling is dysregulated in a variety of CNS-related disorders, especially neurodegenerative diseases, such as AD & PD (171), Friedreich’s Ataxia (172), and Huntington’s disease (173). These neurodegenerative diseases fundamentally share common pathologies; ROS formation, mitochondrial dysfunction, inflammation, and disrupted homeostasis (174). Previous studies have reported different patterns of Nrf2 expression and its target antioxidant proteins in AD. Nrf2-ARE activation may occur in the early stage of disease and nuclear Nrf2 levels decrease in AD patients at a later stage (175, 176). The demonstration that Nrf2 inducer SFN provides an improvement in cognitive function and amyloid pathology in PS1V97L‐Tg mice, has supported the importance of the Nrf2/ARE pathway in Alzheimer’s disease. Postmortem studies in PD exert higher Nrf2 nuclear translocation and upregulated expression of Nrf2-regulated genes NQO1 and HO-1 in the brain of patients. Nrf2 activators DMF and MMF exerted neuroprotective effects against MPTP neurotoxicity in wild-type but not Nrf2 null mice. Demonstration of neuroprotective effects of Nrf2 activators DMF and MMF against MPTP neurotoxicity in wild-type but not Nrf2 null mice revealed the importance of Nrf2 pathway in PD pathogenesis, and stimulation of this pathway may be a therapeutic approach for PD (177).
The mechanism of action by which these inducers modulate Nrf2 may differ and primarily related to the group of Nrf2 inducers, namely electrophiles, PPI inhibitors, and multi-target drugs (178). While electrophiles interact with cysteine residues on KEAP1 by covalent bonding and cause prevention of KEAP1-orchestrated ubiquitination and subsequent degradation of Nrf2, the PPI inhibitors hinder the interaction between Nrf2-KEAP1 or KEAP1-CUL3 non-covalently (179). Lastly, multi-target drugs participate in several pathways which activate or inactivate Nrf2. In particular, GSK3 (76), which phosphorylates Nrf2 leading to its ubiquitination, BACH1 (180), which decreases the transcriptional activity of Nrf2 and subsequent expression of ARE-genes, and SQSTM1/p62 (181), which stabilize Nrf2 and assist its translocation, constitute multiple targets for Nrf2 activity. The modulation of these targets enhances Nrf2 activity and expression of ARE-genes, underscoring their possible clinical application. Numerous Nrf2 inducers with different mechanisms of action have been tested in clinical trials. Although the clinical trials have not focused on the effects of Nrf2 modulators against NLRP3 inflammasome activation parameters, the contribution of NLRP3 inflammasome activation to pathogenesis and clinical outcomes of those CNS-related disorders has been studied thoroughly (182). Among the clinically tested Nrf2 inducers, there are natural compounds such as SFN, curcumin, resveratrol, and synthetic ones such as DMF, Bardoxolone-methyl, Omaveloxolone (178). Furthermore, these compounds have been clinically tested for various disorders ranging from cancers (183) to metabolic disorders (184). The clinical trials where Nrf2 modulators were tested in different CNS-related disorders described in this section are listed in Table 1.
Among the clinically tested Nrf2 inducers, DMF, also known as BG-12 or Tecfidera®, is the only drug approved for Relapsing-Remitting Multiple Sclerosis (RRMS) by both Food and Drug Administration and the European Medicines Agency (185). DMF, a derivative of fumaric acid, exerts immunomodulatory, anti-inflammatory, anti-oxidative, and neuroprotective effects (186). Furthermore, Linker et al. reported that DMF acts on the cysteine 151 on KEAP1 and thus activates Nrf2 (104). a pilot study revealed that DMF could be used against RRMS (187). After that, two separate clinical trials (DEFINE and CONFIRM) demonstrated that DMF is safe for use and can effectively reduce brain lesions and relapse rates in RRMS patients (188). A recent trial which is an extension of DEFINE and CONFIRM, called ENDORSE, supported the results of previous trials in terms of efficacy and positive benefit/risk profile (189). DMF is mainly converted to MMF by intestinal esterases and distributed throughout the body (190). Therefore, an oral formulation of a MMF derivative, ALKS-8700, which exhibits improved bioavailability and efficacy, has also been tested in a phase III trial for MS (191). Due to its cytoprotective and anti-inflammatory nature, DMF is used against acute ischemic stroke and intracerebral hemorrhage, as well as glioblastoma.
Similarly, synthetic Nrf2 modulators, such as electrophilic Omaveloxolone, multi-target drugs, Tideglusib, and Terameprocol, have been developed so as to induce Nrf2 and prevent disease or decrease the clinical symptoms (178). The recently developed Omaveloxolone is another electrophilic Nrf2 inducer that modifies cysteine 151 on KEAP1 and activates Nrf2. It has been shown that Omaveloxolone, clinically tested in Friedreich’s Ataxia, can improve mitochondrial function in vivo (192). Furthermore, multi-target drugs, Tideglusib, Terameprocol, have also undergone clinical trials due to their modulatory function on Nrf2 via GSK-3 inhibition in AD (193), ALS, autism spectrum disorders (194), and CNS cancers (195).
Phytochemicals, a wide variety of chemical compounds, constitute another group of Nrf2 inducers extracted from medicinal plants and used for therapeutic purposes. Considering the innumerable phytochemicals produced by plants, these natural compounds have great potential to be used as Nrf2 modulators. The most clinically used and tested in clinical trials natural Nrf2 inducers are SFN, Curcumin, Resveratrol, and Nordihydroguaiaretic acid (178). These compounds are considered significant due to their accessibility and fair price as compared to synthetic drugs. SFN, an organosulfur compound abundantly found in cruciferous vegetables like broccoli and cabbage, is a strong electrophile modifying cysteine 151 on KEAP1. Due to its SFN’s cytoprotective features, such as anti-inflammation and anti-oxidation, it is applied in a wide range of disorders in vitro and in vivo (196). SFN is being clinically tested for various CNS-related disorders, including PD, schizophrenia, autism spectrum disorder, and depression. Furthermore, Sulforadex, an SFN-derived active compound, has been administered to patients with subarachnoid hemorrhage (197). Likewise, electrophilic polyphenolic compounds, curcumin extracted from turmeric (Curcuma longa), and resveratrol derived from nuts and berries are the most clinically tested compounds after SFN. Because of their anti-oxidative and anti-inflammatory properties, as well as their ability to react with KEAP1 via its cysteine 151 residue, they could serve as potent Nrf2 modulators (11, 185). They have been clinically tested in commonly occurring neurodegenerative diseases such as AD, Mild Cognitive Impairment, ALS, Huntington’s disease, neuropsychiatric disorders such as schizophrenia, depression, MS, and Friedreich’s Ataxia.
Apart from being tested clinically, as mentioned before, DMF is the only Nrf2 inducer approved exclusively for CNS-related disorders. Although the safety and efficacy of DMF have been shown with large cohorts and even in pregnant women (198), it causes substantial side effects such as a reduced number of lymphocytes (199). DMF or other electrophilic Nrf2 activators induce off-target side effects, which has been explained by S-alkylation of cysteine thiols non-specifically and subsequent deficiency of anti-oxidative glutathione (200). These concerns are valid for both synthetic and natural compounds as the active compounds, electrophilic or other groups of Nrf2 inducers, especially when used at high doses, activate not only the Nrf2 signaling cascade but a considerable number of distinct signaling pathways. However, there are ongoing studies, which require great effort, time, and expenses, focusing on the modulation of Nrf2 as a potential therapeutic approach against diseases with improved efficiency and less off-target effects.
Concluding Remark
Since the discovery and molecular/biochemical elucidation of the NLRP3 inflammasome by Tschopp and his colleagues in 2002, cumulative evidence acquired by preclinical (in vitro and in vivo) and clinical studies indicates that the contribution of NLRP3 inflammasome activation to a wide variety of diseases remains largely unelucidated. Furthermore, the ablation of NLRP3 inflammasome-related genes or small inhibitors against the NLRP3 inflammasome complex alleviates the progress, severity, or clinical outcomes of NLRP3-associated diseases. Therefore, the need for developing therapeutic strategies targeting the structural integrity or activity of the NLRP3 inflammasome complex is indispensable. Although developing novel drugs or repurposing currently used drugs might be effective in terms of specificity, the pharmacological modulation of cytoprotective signaling pathways might be another therapeutic approach to suppress the NLRP3 inflammasome activation and subsequent cell death. Since NLRP3 inflammasome senses ROS, inflammatory cytokines, and endogenous metabolites, which damage homeostasis as a regulator of redox, metabolism, and inflammation, Nrf2 represents a powerful candidate for targeting the NLRP3 inflammasome signaling cascade.
Given that Nrf2 regulates more than 250 homeostatic genes, Nrf2 plays a pivotal role in cytoprotection. This role is also supported by the presence of a plethora of naturally derived and designed Nrf2 inducers, which modulate Nrf2 and enhance its cytoprotective effects. Furthermore, some Nrf2 inducers, such as DMF and SFN, have undergone trials in preclinical and clinical models of diseases with which NLRP3 inflammasome activation is associated. As the recent advances and studies enhance our knowledge on the NLRP3/Nrf2 crosstalk, the need to establish specific therapeutic approaches with precision using Nrf2 inducers increases. To achieve this, more comprehensive and multidisciplinary research efforts are needed. Nevertheless, this research will be translated and broaden our current insight into the regulation of NLRP3 inflammasome and its related diseases.
Author Contributions
BT and BA wrote the first draft of the manuscript. SG revised and approved the submitted version of the manuscript. All authors contributed to the article and approved the submitted version.
Conflict of Interest
The authors declare that the research was conducted in the absence of any commercial or financial relationships that could be construed as a potential conflict of interest.
Publisher’s Note
All claims expressed in this article are solely those of the authors and do not necessarily represent those of their affiliated organizations, or those of the publisher, the editors and the reviewers. Any product that may be evaluated in this article, or claim that may be made by its manufacturer, is not guaranteed or endorsed by the publisher.
Acknowledgments
The authors would like to thank Dr. Athanasia Pavlopoulou for her critical reading and comments.
Abbreviations
AD, Alzheimer’s Disease; ALS, Amyotrophic Lateral Sclerosis; ARE, Antioxidant Response Element; ATP, Adenosine Triphosphate; ASC, Apoptosis-associated Speck-like Protein containing a CARD; CBP, CREB-binding protein; CNS, Central Nervous System; DAMPs, Danger-Associated Molecular Patterns; DMF, Dimethyl Fumarate; GSDMD, Gasdermin D; HAMPs, Homeostasis-Altering Molecular Processes; IL, Interleukin; KEAP1, Kelch-like ECH associated protein; LncRNA, Long Non-coding RNA; LPS, Lipopolysaccharide; miRNA, MicroRNA; MS, Multiple Sclerosis; NF-κB, Nuclear Factor Kappa B; NLRP3, NLR Family Pyrin Domain Containing Protein 3; Nrf2, Nuclear Factor Erythroid 2-related Factor 2; PAMPs, Pathogen-associated molecular patterns; PD, Parkinson’s Disease; PPI, Protein-Protein Inhibitor; PRR, Pattern Recognition Receptors; ROS, Reactive Oxygen Species; RRMS, Relapsing-Remitting Multiple Sclerosis; SFN, Sulforaphane; TLR, Toll-like Receptor; TXNIP, Thioredoxin-interacting protein.
References
1. Yang QQ, Zhou JW. Neuroinflammation in the Central Nervous System: Symphony of Glial Cells. Glia (2019) 67:1017–35. doi: 10.1002/glia.23571
2. Ransohoff RM, Brown MA. Innate Immunity in the Central Nervous System. J Clin Invest (2012) 122:1164–71. doi: 10.1172/JCI58644
3. Liston A, Masters SL. Homeostasis-Altering Molecular Processes as Mechanisms of Inflammasome Activation. Nat Rev Immunol (2017) 17:208–14. doi: 10.1038/nri.2016.151
4. Walsh JG, Muruve DA, Power C. Inflammasomes in the CNS. Nat Rev Neurosci (2014) 15:84–97. doi: 10.1038/nrn3638
5. Hanamsagar R, Torres V, Kielian T. Inflammasome Activation and IL-1beta/IL-18 Processing are Influenced by Distinct Pathways in Microglia. J Neurochem (2011) 119:736–48. doi: 10.1111/j.1471-4159.2011.07481.x
6. He Y, Hara H, Nunez G. Mechanism and Regulation of NLRP3 Inflammasome Activation. Trends Biochem Sci (2016) 41:1012–21. doi: 10.1016/j.tibs.2016.09.002
7. Kanneganti TD, Body-Malapel M, Amer A, Park JH, Whitfield J, Franchi L, et al. Critical Role for Cryopyrin/Nalp3 in Activation of Caspase-1 in Response to Viral Infection and Double-Stranded RNA. J Biol Chem (2006) 281:36560–8. doi: 10.1074/jbc.M607594200
8. Gross O, Poeck H, Bscheider M, Dostert C, Hannesschlager N, Endres S, et al. Syk Kinase Signalling Couples to the Nlrp3 Inflammasome for Anti-Fungal Host Defence. Nature (2009) 459:433–6. doi: 10.1038/nature07965
9. Areschoug T, Gordon S. Pattern Recognition Receptors and Their Role in Innate Immunity: Focus on Microbial Protein Ligands. Contrib Microbiol (2008) 15:45–60. doi: 10.1159/000135685
10. Duncan JA, Bergstralh DT, Wang Y, Willingham SB, Ye Z, Zimmermann AG, et al. Cryopyrin/NALP3 Binds ATP/dATP, is an ATPase, and Requires ATP Binding to Mediate Inflammatory Signaling. Proc Natl Acad Sci U S A (2007) 104:8041–6. doi: 10.1073/pnas.0611496104
11. Olcum M, Tastan B, Ercan I, Eltutan IB, Genc S. Inhibitory Effects of Phytochemicals on NLRP3 Inflammasome Activation: A Review. Phytomedicine (2020) 75:153238. doi: 10.1016/j.phymed.2020.153238
12. Bauernfeind FG, Horvath G, Stutz A, Alnemri ES, Macdonald K, Speert D, et al. Cutting Edge: NF-kappaB Activating Pattern Recognition and Cytokine Receptors License NLRP3 Inflammasome Activation by Regulating NLRP3 Expression. J Immunol (2009) 183:787–91. doi: 10.4049/jimmunol.0901363
13. Song N, Liu ZS, Xue W, Bai ZF, Wang QY, Dai J, et al. NLRP3 Phosphorylation Is an Essential Priming Event for Inflammasome Activation. Mol Cell (2017) 68:185–97.e186. doi: 10.1016/j.molcel.2017.08.017
14. Mangan MSJ, Olhava EJ, Roush WR, Seidel HM, Glick GD, Latz E. Targeting the NLRP3 Inflammasome in Inflammatory Diseases. Nat Rev Drug Discov (2018) 17:588–606. doi: 10.1038/nrd.2018.97
15. Liu X, Zhang Z, Ruan J, Pan Y, Magupalli VG, Wu H, et al. Inflammasome-Activated Gasdermin D Causes Pyroptosis by Forming Membrane Pores. Nature (2016) 535:153–8. doi: 10.1038/nature18629
16. Kayagaki N, Warming S, Lamkanfi M, Vande Walle L, Louie S, Dong J, et al. Non-Canonical Inflammasome Activation Targets Caspase-11. Nature (2011) 479:117–21. doi: 10.1038/nature10558
17. Vigano E, Diamond CE, Spreafico R, Balachander A, Sobota RM, Mortellaro A. Human Caspase-4 and Caspase-5 Regulate the One-Step Non-Canonical Inflammasome Activation in Monocytes. Nat Commun (2015) 6:8761. doi: 10.1038/ncomms9761
18. Schauvliege R, Vanrobaeys J, Schotte P, Beyaert R. Caspase-11 Gene Expression in Response to Lipopolysaccharide and Interferon-Gamma Requires Nuclear Factor-Kappa B and Signal Transducer and Activator of Transcription (STAT) 1. J Biol Chem (2002) 277:41624–30. doi: 10.1074/jbc.M207852200
19. Meunier E, Dick MS, Dreier RF, Schurmann N, Kenzelmann Broz D, Warming S, et al. Caspase-11 Activation Requires Lysis of Pathogen-Containing Vacuoles by IFN-Induced GTPases. Nature (2014) 509:366–70. doi: 10.1038/nature13157
20. Shi J, Zhao Y, Wang K, Shi X, Wang Y, Huang H, et al. Cleavage of GSDMD by Inflammatory Caspases Determines Pyroptotic Cell Death. Nature (2015) 526:660–5. doi: 10.1038/nature15514
21. Gaidt MM, Ebert TS, Chauhan D, Schmidt T, Schmid-Burgk JL, Rapino F, et al. Human Monocytes Engage an Alternative Inflammasome Pathway. Immunity (2016) 44:833–46. doi: 10.1016/j.immuni.2016.01.012
22. Ge Q, Chen X, Zhao Y, Mu H, Zhang J. Modulatory Mechanisms of NLRP3: Potential Roles in Inflammasome Activation. Life Sci (2021) 267:118918. doi: 10.1016/j.lfs.2020.118918
23. Huai W, Zhao R, Song H, Zhao J, Zhang L, Zhang L, et al. Aryl Hydrocarbon Receptor Negatively Regulates NLRP3 Inflammasome Activity by Inhibiting NLRP3 Transcription. Nat Commun (2014) 5:4738. doi: 10.1038/ncomms5738
24. Chen D, Xiong XQ, Zang YH, Tong Y, Zhou B, Chen Q, et al. BCL6 Attenuates Renal Inflammation via Negative Regulation of NLRP3 Transcription. Cell Death Dis (2017) 8:e3156. doi: 10.1038/cddis.2017.567
25. Ollivier V, Parry GC, Cobb RR, De Prost D, Mackman N. Elevated Cyclic AMP Inhibits NF-kappaB-Mediated Transcription in Human Monocytic Cells and Endothelial Cells. J Biol Chem (1996) 271:20828–35. doi: 10.1074/jbc.271.34.20828
26. Lv H, Liu Q, Wen Z, Feng H, Deng X, Ci X. Xanthohumol Ameliorates Lipopolysaccharide (LPS)-Induced Acute Lung Injury via Induction of AMPK/GSK3beta-Nrf2 Signal Axis. Redox Biol (2017) 12:311–24. doi: 10.1016/j.redox.2017.03.001
27. Wang S, Lin Y, Yuan X, Li F, Guo L, Wu B. REV-ERBalpha Integrates Colon Clock With Experimental Colitis Through Regulation of NF-Kappab/NLRP3 Axis. Nat Commun (2018) 9:4246. doi: 10.1038/s41467-018-06568-5
28. Gurung P, Anand PK, Malireddi RK, Vande Walle L, Van Opdenbosch N, Dillon CP, et al. FADD and Caspase-8 Mediate Priming and Activation of the Canonical and Noncanonical Nlrp3 Inflammasomes. J Immunol (2014) 192:1835–46. doi: 10.4049/jimmunol.1302839
29. Kang LL, Zhang DM, Ma CH, Zhang JH, Jia KK, Liu JH, et al. Cinnamaldehyde and Allopurinol Reduce Fructose-Induced Cardiac Inflammation and Fibrosis by Attenuating CD36-Mediated TLR4/6-IRAK4/1 Signaling to Suppress NLRP3 Inflammasome Activation. Sci Rep (2016) 6:27460. doi: 10.1038/srep27460
30. Su Q, Li L, Sun Y, Yang H, Ye Z, Zhao J. Effects of the TLR4/Myd88/NF-kappaB Signaling Pathway on NLRP3 Inflammasome in Coronary Microembolization-Induced Myocardial Injury. Cell Physiol Biochem (2018) 47:1497–508. doi: 10.1159/000490866
31. Temiz-Resitoglu M, Kucukkavruk SP, Guden DS, Cecen P, Sari AN, Tunctan B, et al. Activation of mTOR/IkappaB-Alpha/NF-kappaB Pathway Contributes to LPS-Induced Hypotension and Inflammation in Rats. Eur J Pharmacol (2017) 802:7–19. doi: 10.1016/j.ejphar.2017.02.034
32. Cao G, Jiang N, Hu Y, Zhang Y, Wang G, Yin M, et al. Ruscogenin Attenuates Cerebral Ischemia-Induced Blood-Brain Barrier Dysfunction by Suppressing TXNIP/NLRP3 Inflammasome Activation and the MAPK Pathway. Int J Mol Sci (2016) 17(9):1418. doi: 10.3390/ijms17091418
33. Zhao W, Ma L, Cai C, Gong X. Caffeine Inhibits NLRP3 Inflammasome Activation by Suppressing MAPK/NF-kappaB and A2aR Signaling in LPS-Induced THP-1 Macrophages. Int J Biol Sci (2019) 15:1571–81. doi: 10.7150/ijbs.34211
34. Zhou Y, Lu M, Du RH, Qiao C, Jiang CY, Zhang KZ, et al. MicroRNA-7 Targets Nod-Like Receptor Protein 3 Inflammasome to Modulate Neuroinflammation in the Pathogenesis of Parkinson's Disease. Mol Neurodegener (2016) 11:28. doi: 10.1186/s13024-016-0094-3
35. Li S, Liang X, Ma L, Shen L, Li T, Zheng L, et al. MiR-22 Sustains NLRP3 Expression and Attenuates H. Pylori-Induced Gastric Carcinogenesis. Oncogene (2018) 37:884–96. doi: 10.1038/onc.2017.381
36. Li D, Yang H, Ma J, Luo S, Chen S, Gu Q. MicroRNA-30e Regulates Neuroinflammation in MPTP Model of Parkinson's Disease by Targeting Nlrp3. Hum Cell (2018) 31:106–15. doi: 10.1007/s13577-017-0187-5
37. Xiao L, Jiang L, Hu Q, Li Y. MicroRNA-133b Ameliorates Allergic Inflammation and Symptom in Murine Model of Allergic Rhinitis by Targeting Nlrp3. Cell Physiol Biochem (2017) 42:901–12. doi: 10.1159/000478645
38. Long FQ, Kou CX, Li K, Wu J, Wang QQ. MiR-223-3p Inhibits Rtp17-Induced Inflammasome Activation and Pyroptosis by Targeting NLRP3. J Cell Mol Med (2020) 24:14405–14. doi: 10.1111/jcmm.16061
39. Zhang P, Cao L, Zhou R, Yang X, Wu M. The lncRNA Neat1 Promotes Activation of Inflammasomes in Macrophages. Nat Commun (2019) 10:1495. doi: 10.1038/s41467-019-09482-6
40. Meng J, Ding T, Chen Y, Long T, Xu Q, Lian W, et al. LncRNA-Meg3 Promotes Nlrp3-Mediated Microglial Inflammation by Targeting miR-7a-5p. Int Immunopharmacol (2021) 90:107141. doi: 10.1016/j.intimp.2020.107141
41. Zhang Q, Huang XM, Liao JX, Dong YK, Zhu JL, He CC, et al. LncRNA HOTAIR Promotes Neuronal Damage Through Facilitating NLRP3 Mediated-Pyroptosis Activation in Parkinson's Disease via Regulation of miR-326/ELAVL1 Axis. Cell Mol Neurobiol (2021) 41:1773–86. doi: 10.1007/s10571-020-00946-8
42. Du P, Wang J, Han Y, Feng J. Blocking the LncRNA MALAT1/miR-224-5p/NLRP3 Axis Inhibits the Hippocampal Inflammatory Response in T2DM With OSA. Front Cell Neurosci (2020) 14:97. doi: 10.3389/fncel.2020.00097
43. Luo D, Dai W, Feng X, Ding C, Shao Q, Xiao R, et al. Suppression of lncRNA NLRP3 Inhibits NLRP3-Triggered Inflammatory Responses in Early Acute Lung Injury. Cell Death Dis (2021) 12:898. doi: 10.1038/s41419-021-04180-y
44. Ma M, Pei Y, Wang X, Feng J, Zhang Y, Gao MQ. LncRNA XIST Mediates Bovine Mammary Epithelial Cell Inflammatory Response via NF-Kappab/NLRP3 Inflammasome Pathway. Cell Prolif (2019) 52:e12525. doi: 10.1111/cpr.12525
45. Xu Y, Fang H, Xu Q, Xu C, Yang L, Huang C. LncRNA GAS5 Inhibits NLRP3 Inflammasome Activation-Mediated Pyroptosis in Diabetic Cardiomyopathy by Targeting miR-34b-3p/AHR. Cell Cycle (2020) 19:3054–65. doi: 10.1080/15384101.2020.1831245
46. Chen Z, He M, Chen J, Li C, Zhang Q. Long non-Coding RNA SNHG7 Inhibits NLRP3-Dependent Pyroptosis by Targeting the miR-34a/SIRT1 Axis in Liver Cancer. Oncol Lett (2020) 20:893–901. doi: 10.3892/ol.2020.11635
47. Py BF, Kim MS, Vakifahmetoglu-Norberg H, Yuan J. Deubiquitination of NLRP3 by BRCC3 Critically Regulates Inflammasome Activity. Mol Cell (2013) 49:331–8. doi: 10.1016/j.molcel.2012.11.009
48. Kawashima A, Karasawa T, Tago K, Kimura H, Kamata R, Usui-Kawanishi F, et al. ARIH2 Ubiquitinates NLRP3 and Negatively Regulates NLRP3 Inflammasome Activation in Macrophages. J Immunol (2017) 199:3614–22. doi: 10.4049/jimmunol.1700184
49. Wan P, Zhang Q, Liu W, Jia Y, Ai S, Wang T, et al. Cullin1 Binds and Promotes NLRP3 Ubiquitination to Repress Systematic Inflammasome Activation. FASEB J (2019) 33:5793–807. doi: 10.1096/fj.201801681R
50. Han S, Lear TB, Jerome JA, Rajbhandari S, Snavely CA, Gulick DL, et al. Lipopolysaccharide Primes the NALP3 Inflammasome by Inhibiting Its Ubiquitination and Degradation Mediated by the SCFFBXL2 E3 Ligase. J Biol Chem (2015) 290:18124–33. doi: 10.1074/jbc.M115.645549
51. Song H, Liu B, Huai W, Yu Z, Wang W, Zhao J, et al. The E3 Ubiquitin Ligase TRIM31 Attenuates NLRP3 Inflammasome Activation by Promoting Proteasomal Degradation of NLRP3. Nat Commun (2016) 7:13727. doi: 10.1038/ncomms13727
52. Spalinger MR, Kasper S, Gottier C, Lang S, Atrott K, Vavricka SR, et al. NLRP3 Tyrosine Phosphorylation is Controlled by Protein Tyrosine Phosphatase PTPN22. J Clin Invest (2016) 126:1783–800. doi: 10.1172/JCI83669
53. Stutz A, Kolbe CC, Stahl R, Horvath GL, Franklin BS, Van Ray O, et al. NLRP3 Inflammasome Assembly is Regulated by Phosphorylation of the Pyrin Domain. J Exp Med (2017) 214:1725–36. doi: 10.1084/jem.20160933
54. Shao L, Liu Y, Wang W, Li A, Wan P, Liu W, et al. SUMO1 SUMOylates and SENP3 Desumoylates NLRP3 to Orchestrate the Inflammasome Activation. FASEB J (2020) 34:1497–515. doi: 10.1096/fj.201901653R
55. Heneka MT, Kummer MP, Stutz A, Delekate A, Schwartz S, Vieira-Saecker A, et al. NLRP3 is Activated in Alzheimer's Disease and Contributes to Pathology in APP/PS1 Mice. Nature (2013) 493:674–8. doi: 10.1038/nature11729
56. Codolo G, Plotegher N, Pozzobon T, Brucale M, Tessari I, Bubacco L, et al. Triggering of Inflammasome by Aggregated Alpha-Synuclein, an Inflammatory Response in Synucleinopathies. PloS One (2013) 8:e55375. doi: 10.1371/journal.pone.0055375
57. Meissner F, Molawi K, Zychlinsky A. Mutant Superoxide Dismutase 1-Induced IL-1beta Accelerates ALS Pathogenesis. Proc Natl Acad Sci U S A (2010) 107:13046–50. doi: 10.1073/pnas.1002396107
58. Gris D, Ye Z, Iocca HA, Wen H, Craven RR, Gris P, et al. NLRP3 Plays a Critical Role in the Development of Experimental Autoimmune Encephalomyelitis by Mediating Th1 and Th17 Responses. J Immunol (2010) 185:974–81. doi: 10.4049/jimmunol.0904145
59. Bhattacharya A, Jones DNC. Emerging Role of the P2X7-NLRP3-IL1beta Pathway in Mood Disorders. Psychoneuroendocrinology (2018) 98:95–100. doi: 10.1016/j.psyneuen.2018.08.015
60. Mortezaee K, Khanlarkhani N, Beyer C, Zendedel A. Inflammasome: Its Role in Traumatic Brain and Spinal Cord Injury. J Cell Physiol (2018) 233:5160–9. doi: 10.1002/jcp.26287
61. Stancu IC, Cremers N, Vanrusselt H, Couturier J, Vanoosthuyse A, Kessels S, et al. Aggregated Tau Activates NLRP3-ASC Inflammasome Exacerbating Exogenously Seeded and non-Exogenously Seeded Tau Pathology In Vivo. Acta Neuropathol (2019) 137:599–617. doi: 10.1007/s00401-018-01957-y
62. Friker LL, Scheiblich H, Hochheiser IV, Brinkschulte R, Riedel D, Latz E, et al. Beta-Amyloid Clustering Around ASC Fibrils Boosts Its Toxicity in Microglia. Cell Rep (2020) 30:3743–54.e3746. doi: 10.1016/j.celrep.2020.02.025
63. Li L, Ismael S, Nasoohi S, Sakata K, Liao FF, Mcdonald MP, et al. Thioredoxin-Interacting Protein (TXNIP) Associated NLRP3 Inflammasome Activation in Human Alzheimer's Disease Brain. J Alzheimers Dis (2019) 68:255–65. doi: 10.3233/JAD-180814
64. Sarkar S, Malovic E, Harishchandra DS, Ghaisas S, Panicker N, Charli A, et al. Mitochondrial Impairment in Microglia Amplifies NLRP3 Inflammasome Proinflammatory Signaling in Cell Culture and Animal Models of Parkinson's Disease. NPJ Parkinsons Dis (2017) 3:30. doi: 10.1038/s41531-017-0032-2
65. Mouton-Liger F, Rosazza T, Sepulveda-Diaz J, Ieang A, Hassoun SM, Claire E, et al. Parkin Deficiency Modulates NLRP3 Inflammasome Activation by Attenuating an A20-Dependent Negative Feedback Loop. Glia (2018) 66:1736–51. doi: 10.1002/glia.23337
66. Panicker N, Sarkar S, Harischandra DS, Neal M, Kam TI, Jin H, et al. Fyn Kinase Regulates Misfolded Alpha-Synuclein Uptake and NLRP3 Inflammasome Activation in Microglia. J Exp Med (2019) 216:1411–30. doi: 10.1084/jem.20182191
67. Wang W, Nguyen LT, Burlak C, Chegini F, Guo F, Chataway T, et al. Caspase-1 Causes Truncation and Aggregation of the Parkinson's Disease-Associated Protein Alpha-Synuclein. Proc Natl Acad Sci U S A (2016) 113:9587–92. doi: 10.1073/pnas.1610099113
68. Zahid A, Li B, Kombe AJK, Jin T, Tao J. Pharmacological Inhibitors of the NLRP3 Inflammasome. Front Immunol (2019) 10:2538. doi: 10.3389/fimmu.2019.02538
69. Yang Y, Wang H, Kouadir M, Song H, Shi F. Recent Advances in the Mechanisms of NLRP3 Inflammasome Activation and its Inhibitors. Cell Death Dis (2019) 10:128. doi: 10.1038/s41419-019-1413-8
70. El-Sharkawy LY, Brough D, Freeman S. Inhibiting the NLRP3 Inflammasome. Molecules (2020) 25(23):5533. doi: 10.3390/molecules25235533
71. Jaramillo MC, Zhang DD. The Emerging Role of the Nrf2-Keap1 Signaling Pathway in Cancer. Genes Dev (2013) 27:2179–91. doi: 10.1101/gad.225680.113
72. Motohashi H, Yamamoto M. Nrf2-Keap1 Defines a Physiologically Important Stress Response Mechanism. Trends Mol Med (2004) 10:549–57. doi: 10.1016/j.molmed.2004.09.003
73. Moi P, Chan K, Asunis I, Cao A, Kan YW. Isolation of NF-E2-Related Factor 2 (Nrf2), a NF-E2-Like Basic Leucine Zipper Transcriptional Activator That Binds to the Tandem NF-E2/AP1 Repeat of the Beta-Globin Locus Control Region. Proc Natl Acad Sci U S A (1994) 91:9926–30. doi: 10.1073/pnas.91.21.9926
74. Mcmahon M, Thomas N, Itoh K, Yamamoto M, Hayes JD. Redox-Regulated Turnover of Nrf2 is Determined by at Least Two Separate Protein Domains, the Redox-Sensitive Neh2 Degron and the Redox-Insensitive Neh6 Degron. J Biol Chem (2004) 279:31556–67. doi: 10.1074/jbc.M403061200
75. Nioi P, Nguyen T, Sherratt PJ, Pickett CB. The Carboxy-Terminal Neh3 Domain of Nrf2 is Required for Transcriptional Activation. Mol Cell Biol (2005) 25:10895–906. doi: 10.1128/MCB.25.24.10895-10906.2005
76. Chowdhry S, Zhang Y, Mcmahon M, Sutherland C, Cuadrado A, Hayes JD. Nrf2 is Controlled by Two Distinct Beta-TrCP Recognition Motifs in its Neh6 Domain, One of Which can be Modulated by GSK-3 Activity. Oncogene (2013) 32:3765–81. doi: 10.1038/onc.2012.388
77. Wang H, Liu K, Geng M, Gao P, Wu X, Hai Y, et al. RXRalpha Inhibits the NRF2-ARE Signaling Pathway Through a Direct Interaction With the Neh7 Domain of NRF2. Cancer Res (2013) 73:3097–108. doi: 10.1158/0008-5472.CAN-12-3386
78. Song MY, Lee DY, Chun KS, Kim EH. The Role of NRF2/KEAP1 Signaling Pathway in Cancer Metabolism. Int J Mol Sci (2021) 22(9):4376. doi: 10.3390/ijms22094376
79. Ahmed SM, Luo L, Namani A, Wang XJ, Tang X. Nrf2 Signaling Pathway: Pivotal Roles in Inflammation. Biochim Biophys Acta Mol Basis Dis (2017) 1863:585–97. doi: 10.1016/j.bbadis.2016.11.005
80. Miao W, Hu L, Scrivens PJ, Batist G. Transcriptional Regulation of NF-E2 P45-Related Factor (NRF2) Expression by the Aryl Hydrocarbon Receptor-Xenobiotic Response Element Signaling Pathway: Direct Cross-Talk Between Phase I and II Drug-Metabolizing Enzymes. J Biol Chem (2005) 280:20340–8. doi: 10.1074/jbc.M412081200
81. Rushworth SA, Zaitseva L, Murray MY, Shah NM, Bowles KM, Macewan DJ. The High Nrf2 Expression in Human Acute Myeloid Leukemia is Driven by NF-kappaB and Underlies its Chemo-Resistance. Blood (2012) 120:5188–98. doi: 10.1182/blood-2012-04-422121
82. Valadi H, Ekstrom K, Bossios A, Sjostrand M, Lee JJ, Lotvall JO. Exosome-Mediated Transfer of mRNAs and microRNAs is a Novel Mechanism of Genetic Exchange Between Cells. Nat Cell Biol (2007) 9:654–9. doi: 10.1038/ncb1596
83. Fabrizio FP, Sparaneo A, Trombetta D, Muscarella LA. Epigenetic Versus Genetic Deregulation of the KEAP1/NRF2 Axis in Solid Tumors: Focus on Methylation and Noncoding RNAs. Oxid Med Cell Longev (2018) 2018:2492063. doi: 10.1155/2018/2492063
84. Sangokoya C, Telen MJ, Chi JT. microRNA miR-144 Modulates Oxidative Stress Tolerance and Associates With Anemia Severity in Sickle Cell Disease. Blood (2010) 116:4338–48. doi: 10.1182/blood-2009-04-214817
85. Tonelli C, Chio IIC, Tuveson DA. Transcriptional Regulation by Nrf2. Antioxid Redox Signal (2018) 29:1727–45. doi: 10.1089/ars.2017.7342
86. Jayasuriya R, Ramkumar KM. Role of Long non-Coding RNAs on the Regulation of Nrf2 in Chronic Diseases. Life Sci (2021) 270:119025. doi: 10.1016/j.lfs.2021.119025
87. Goldstein LD, Lee J, Gnad F, Klijn C, Schaub A, Reeder J, et al. Recurrent Loss of NFE2L2 Exon 2 Is a Mechanism for Nrf2 Pathway Activation in Human Cancers. Cell Rep (2016) 16:2605–17. doi: 10.1016/j.celrep.2016.08.010
88. Cano Sanchez M, Lancel S, Boulanger E, Neviere R. Targeting Oxidative Stress and Mitochondrial Dysfunction in the Treatment of Impaired Wound Healing: A Systematic Review. Antioxidants (Basel) (2018) 7(8):98. doi: 10.3390/antiox7080098
89. Zhou R, Tardivel A, Thorens B, Choi I, Tschopp J. Thioredoxin-Interacting Protein Links Oxidative Stress to Inflammasome Activation. Nat Immunol (2010) 11:136–40. doi: 10.1038/ni.1831
90. Thimmulappa RK, Lee H, Rangasamy T, Reddy SP, Yamamoto M, Kensler TW, et al. Nrf2 is a Critical Regulator of the Innate Immune Response and Survival During Experimental Sepsis. J Clin Invest (2006) 116:984–95. doi: 10.1172/JCI25790
91. Pan H, Wang H, Wang X, Zhu L, Mao L. The Absence of Nrf2 Enhances NF-kappaB-Dependent Inflammation Following Scratch Injury in Mouse Primary Cultured Astrocytes. Mediators Inflamm (2012) 2012:217580. doi: 10.1155/2012/217580
92. Frakes AE, Ferraiuolo L, Haidet-Phillips AM, Schmelzer L, Braun L, Miranda CJ, et al. Microglia Induce Motor Neuron Death via the Classical NF-kappaB Pathway in Amyotrophic Lateral Sclerosis. Neuron (2014) 81:1009–23. doi: 10.1016/j.neuron.2014.01.013
93. Soares MP, Seldon MP, Gregoire IP, Vassilevskaia T, Berberat PO, Yu J, et al. Heme Oxygenase-1 Modulates the Expression of Adhesion Molecules Associated With Endothelial Cell Activation. J Immunol (2004) 172:3553–63. doi: 10.4049/jimmunol.172.6.3553
94. Liu X, Zhang X, Ding Y, Zhou W, Tao L, Lu P, et al. Nuclear Factor E2-Related Factor-2 Negatively Regulates NLRP3 Inflammasome Activity by Inhibiting Reactive Oxygen Species-Induced NLRP3 Priming. Antioxid Redox Signal (2017) 26:28–43. doi: 10.1089/ars.2015.6615
95. Kobayashi EH, Suzuki T, Funayama R, Nagashima T, Hayashi M, Sekine H, et al. Nrf2 Suppresses Macrophage Inflammatory Response by Blocking Proinflammatory Cytokine Transcription. Nat Commun (2016) 7:11624. doi: 10.1038/ncomms11624
96. Lee D-F, Kuo H-P, Liu M, Chou C-K, Xia W, Du Y, et al. KEAP1 E3 Ligase-Mediated Downregulation of NF-κB Signaling by Targeting IKKβ. Mol Cell (2009) 36:131–40. doi: 10.1016/j.molcel.2009.07.025
97. Ricote M, Li AC, Willson TM, Kelly CJ, Glass CK. The Peroxisome Proliferator-Activated Receptor-γ is a Negative Regulator of Macrophage Activation. Nature (1998) 391:79–82. doi: 10.1038/34178
98. Sánchez-Gómez FJ, Cernuda-Morollón E, Stamatakis K, Pérez-Sala D. Protein Thiol Modification by 15-Deoxy-Δ12,14-Prostaglandin J2Addition in Mesangial Cells: Role in the Inhibition of Pro-Inflammatory Genes. Mol Pharmacol (2004) 66:1349–58. doi: 10.1124/mol.104.002824
99. Hosoya T, Maruyama A, Kang M-I, Kawatani Y, Shibata T, Uchida K, et al. Differential Responses of the Nrf2-Keap1 System to Laminar and Oscillatory Shear Stresses in Endothelial Cells. J Biol Chem (2005) 280:27244–50. doi: 10.1074/jbc.M502551200
100. Hwang Y-J, Lee E-W, Song J, Kim H-R, Jun Y-C, Hwang K-A. MafK Positively Regulates NF-κB Activity by Enhancing CBP-Mediated P65 Acetylation. Sci Rep (2013) 3:3242. doi: 10.1038/srep03242
101. Freigang S, Ampenberger F, Spohn G, Heer S, Shamshiev AT, Kisielow J, et al. Nrf2 is Essential for Cholesterol Crystal-Induced Inflammasome Activation and Exacerbation of Atherosclerosis. Eur J Immunol (2011) 41:2040–51. doi: 10.1002/eji.201041316
102. Zhao C, Gillette DD, Li X, Zhang Z, Wen H. Nuclear Factor E2-Related Factor-2 (Nrf2) is Required for NLRP3 and AIM2 Inflammasome Activation. J Biol Chem (2014) 289:17020–9. doi: 10.1074/jbc.M114.563114
103. Cleasby A, Yon J, Day PJ, Richardson C, Tickle IJ, Williams PA, et al. Structure of the BTB Domain of Keap1 and its Interaction With the Triterpenoid Antagonist CDDO. PloS One (2014) 9:e98896. doi: 10.1371/journal.pone.0098896
104. Linker RA, Lee DH, Ryan S, Van Dam AM, Conrad R, Bista P, et al. Fumaric Acid Esters Exert Neuroprotective Effects in Neuroinflammation via Activation of the Nrf2 Antioxidant Pathway. Brain (2011) 134:678–92. doi: 10.1093/brain/awq386
105. Tastan B, Arioz BI, Tufekci KU, Tarakcioglu E, Gonul CP, Genc K, et al. Dimethyl Fumarate Alleviates NLRP3 Inflammasome Activation in Microglia and Sickness Behavior in LPS-Challenged Mice. Front Immunol (2021) 12:737065. doi: 10.3389/fimmu.2021.737065
106. Cho H, Hartsock MJ, Xu Z, He M, Duh EJ. Monomethyl Fumarate Promotes Nrf2-Dependent Neuroprotection in Retinal Ischemia-Reperfusion. J Neuroinflamm (2015) 12:239. doi: 10.1186/s12974-015-0452-z
107. Merrell MD, Jackson JP, Augustine LM, Fisher CD, Slitt AL, Maher JM, et al. The Nrf2 Activator Oltipraz Also Activates the Constitutive Androstane Receptor. Drug Metab Dispos (2008) 36:1716–21. doi: 10.1124/dmd.108.020867
108. Okada K, Shoda J, Taguchi K, Maher JM, Ishizaki K, Inoue Y, et al. Ursodeoxycholic Acid Stimulates Nrf2-Mediated Hepatocellular Transport, Detoxification, and Antioxidative Stress Systems in Mice. Am J Physiol Gastrointest Liver Physiol (2008) 295:G735–747. doi: 10.1152/ajpgi.90321.2008
109. Lin X, Bai D, Wei Z, Zhang Y, Huang Y, Deng H, et al. Curcumin Attenuates Oxidative Stress in RAW264.7 Cells by Increasing the Activity of Antioxidant Enzymes and Activating the Nrf2-Keap1 Pathway. PloS One (2019) 14:e0216711. doi: 10.1371/journal.pone.0216711
110. Kim EN, Lim JH, Kim MY, Ban TH, Jang IA, Yoon HE, et al. Resveratrol, an Nrf2 Activator, Ameliorates Aging-Related Progressive Renal Injury. Aging (Albany NY) (2018) 10:83–99. doi: 10.18632/aging.101361
111. Jin Y, Huang ZL, Li L, Yang Y, Wang CH, Wang ZT, et al. Quercetin Attenuates Toosendanin-Induced Hepatotoxicity Through Inducing the Nrf2/GCL/GSH Antioxidant Signaling Pathway. Acta Pharmacol Sin (2019) 40:75–85. doi: 10.1038/s41401-018-0024-8
112. Bai Y, Wang X, Zhao S, Ma C, Cui J, Zheng Y. Sulforaphane Protects Against Cardiovascular Disease via Nrf2 Activation. Oxid Med Cell Longev (2015) 2015:407580. doi: 10.1155/2015/407580
113. Arioz BI, Tastan B, Tarakcioglu E, Tufekci KU, Olcum M, Ersoy N, et al. Melatonin Attenuates LPS-Induced Acute Depressive-Like Behaviors and Microglial NLRP3 Inflammasome Activation Through the SIRT1/Nrf2 Pathway. Front Immunol (2019) 10:1511. doi: 10.3389/fimmu.2019.01511
114. Jnoff E, Albrecht C, Barker JJ, Barker O, Beaumont E, Bromidge S, et al. Binding Mode and Structure-Activity Relationships Around Direct Inhibitors of the Nrf2-Keap1 Complex. ChemMedChem (2014) 9:699–705. doi: 10.1002/cmdc.201300525
115. Marcotte D, Zeng W, Hus JC, Mckenzie A, Hession C, Jin P, et al. Small Molecules Inhibit the Interaction of Nrf2 and the Keap1 Kelch Domain Through a non-Covalent Mechanism. Bioorg Med Chem (2013) 21:4011–9. doi: 10.1016/j.bmc.2013.04.019
116. Jiang ZY, Lu MC, Xu LL, Yang TT, Xi MY, Xu XL, et al. Discovery of Potent Keap1-Nrf2 Protein-Protein Interaction Inhibitor Based on Molecular Binding Determinants Analysis. J Med Chem (2014) 57:2736–45. doi: 10.1021/jm5000529
117. Armagan G, Sevgili E, Gurkan FT, Kose FA, Bilgic T, Dagci T, et al. Regulation of the Nrf2 Pathway by Glycogen Synthase Kinase-3beta in MPP(+)-Induced Cell Damage. Molecules (2019) 24(7):1377. doi: 10.3390/molecules24071377
118. Yagishita N, Aratani S, Leach C, Amano T, Yamano Y, Nakatani K, et al. RING-Finger Type E3 Ubiquitin Ligase Inhibitors as Novel Candidates for the Treatment of Rheumatoid Arthritis. Int J Mol Med (2012) 30:1281–6. doi: 10.3892/ijmm.2012.1129
119. Ki SH, Cho IJ, Choi DW, Kim SG. Glucocorticoid Receptor (GR)-Associated SMRT Binding to C/EBPbeta TAD and Nrf2 Neh4/5: Role of SMRT Recruited to GR in GSTA2 Gene Repression. Mol Cell Biol (2005) 25:4150–65. doi: 10.1128/MCB.25.10.4150-4165.2005
120. Choi EJ, Jung BJ, Lee SH, Yoo HS, Shin EA, Ko HJ, et al. A Clinical Drug Library Screen Identifies Clobetasol Propionate as an NRF2 Inhibitor With Potential Therapeutic Efficacy in KEAP1 Mutant Lung Cancer. Oncogene (2017) 36:5285–95. doi: 10.1038/onc.2017.153
121. Wang XJ, Hayes JD, Henderson CJ, Wolf CR. Identification of Retinoic Acid as an Inhibitor of Transcription Factor Nrf2 Through Activation of Retinoic Acid Receptor Alpha. Proc Natl Acad Sci U S A (2007) 104:19589–94. doi: 10.1073/pnas.0709483104
122. Kankia IH, Khalil HS, Langdon SP, Moult PR, Bown JL, Deeni YY. NRF2 Regulates HER1 Signaling Pathway to Modulate the Sensitivity of Ovarian Cancer Cells to Lapatinib and Erlotinib. Oxid Med Cell Longev (2017) 2017:1864578. doi: 10.1155/2017/1864578
123. Tang X, Wang H, Fan L, Wu X, Xin A, Ren H, et al. Luteolin Inhibits Nrf2 Leading to Negative Regulation of the Nrf2/ARE Pathway and Sensitization of Human Lung Carcinoma A549 Cells to Therapeutic Drugs. Free Radic Biol Med (2011) 50:1599–609. doi: 10.1016/j.freeradbiomed.2011.03.008
124. Zhong Y, Zhang F, Sun Z, Zhou W, Li ZY, You QD, et al. Drug Resistance Associates With Activation of Nrf2 in MCF-7/DOX Cells, and Wogonin Reverses it by Down-Regulating Nrf2-Mediated Cellular Defense Response. Mol Carcinog (2013) 52:824–34. doi: 10.1002/mc.21921
125. Tarumoto T, Nagai T, Ohmine K, Miyoshi T, Nakamura M, Kondo T, et al. Ascorbic Acid Restores Sensitivity to Imatinib via Suppression of Nrf2-Dependent Gene Expression in the Imatinib-Resistant Cell Line. Exp Hematol (2004) 32:375–81. doi: 10.1016/j.exphem.2004.01.007
126. Limonciel A, Jennings P. A Review of the Evidence That Ochratoxin A is an Nrf2 Inhibitor: Implications for Nephrotoxicity and Renal Carcinogenicity. Toxins (Basel) (2014) 6:371–9. doi: 10.3390/toxins6010371
127. Chen F, Wang H, Zhu J, Zhao R, Xue P, Zhang Q, et al. Camptothecin Suppresses NRF2-ARE Activity and Sensitises Hepatocellular Carcinoma Cells to Anticancer Drugs. Br J Cancer (2017) 117:1495–506. doi: 10.1038/bjc.2017.317
128. Tsuchida K, Tsujita T, Hayashi M, Ojima A, Keleku-Lukwete N, Katsuoka F, et al. Halofuginone Enhances the Chemo-Sensitivity of Cancer Cells by Suppressing NRF2 Accumulation. Free Radic Biol Med (2017) 103:236–47. doi: 10.1016/j.freeradbiomed.2016.12.041
129. Arlt A, Sebens S, Krebs S, Geismann C, Grossmann M, Kruse ML, et al. Inhibition of the Nrf2 Transcription Factor by the Alkaloid Trigonelline Renders Pancreatic Cancer Cells More Susceptible to Apoptosis Through Decreased Proteasomal Gene Expression and Proteasome Activity. Oncogene (2013) 32:4825–35. doi: 10.1038/onc.2012.493
130. Tufekci KU, Ercan I, Isci KB, Olcum M, Tastan B, Gonul CP, et al. Sulforaphane Inhibits NLRP3 Inflammasome Activation in Microglia Through Nrf2-Mediated miRNA Alteration. Immunol Lett (2021) 233:20–30. doi: 10.1016/j.imlet.2021.03.004
131. Zhu X, Liu J, Huang S, Zhu W, Wang Y, Chen O, et al. Neuroprotective Effects of Isoliquiritigenin Against Cognitive Impairment via Suppression of Synaptic Dysfunction, Neuronal Injury, and Neuroinflammation in Rats With Kainic Acid-Induced Seizures. Int Immunopharmacol (2019) 72:358–66. doi: 10.1016/j.intimp.2019.04.028
132. Zeng J, Chen Y, Ding R, Feng L, Fu Z, Yang S, et al. Isoliquiritigenin Alleviates Early Brain Injury After Experimental Intracerebral Hemorrhage via Suppressing ROS- and/or NF-kappaB-Mediated NLRP3 Inflammasome Activation by Promoting Nrf2 Antioxidant Pathway. J Neuroinflamm (2017) 14:119. doi: 10.1186/s12974-017-0895-5
133. Zhu W, Cao FS, Feng J, Chen HW, Wan JR, Lu Q, et al. NLRP3 Inflammasome Activation Contributes to Long-Term Behavioral Alterations in Mice Injected With Lipopolysaccharide. Neuroscience (2017) 343:77–84. doi: 10.1016/j.neuroscience.2016.11.037
134. Wang CY, Xu Y, Wang X, Guo C, Wang T, Wang ZY. Dl-3-N-Butylphthalide Inhibits NLRP3 Inflammasome and Mitigates Alzheimer's-Like Pathology via Nrf2-TXNIP-TrX Axis. Antioxid Redox Signal (2019) 30:1411–31. doi: 10.1089/ars.2017.7440
135. Zhang C, Zhao M, Wang B, Su Z, Guo B, Qin L, et al. The Nrf2-NLRP3-Caspase-1 Axis Mediates the Neuroprotective Effects of Celastrol in Parkinson's Disease. Redox Biol (2021) 47:102134. doi: 10.1016/j.redox.2021.102134
136. Zhang ZH, Liu JQ, Hu CD, Zhao XT, Qin FY, Zhuang Z, et al. Luteolin Confers Cerebroprotection After Subarachnoid Hemorrhage by Suppression of NLPR3 Inflammasome Activation Through Nrf2-Dependent Pathway. Oxid Med Cell Longev (2021) 2021:5838101. doi: 10.1155/2021/5838101
137. Fu J, Sun H, Zhang Y, Xu W, Wang C, Fang Y, et al. Neuroprotective Effects of Luteolin Against Spinal Cord Ischemia-Reperfusion Injury by Attenuation of Oxidative Stress, Inflammation, and Apoptosis. J Med Food (2018) 21:13–20. doi: 10.1089/jmf.2017.4021
138. Xiao L, Dai Z, Tang W, Liu C, Tang B. Astragaloside IV Alleviates Cerebral Ischemia-Reperfusion Injury Through NLRP3 Inflammasome-Mediated Pyroptosis Inhibition via Activating Nrf2. Oxid Med Cell Longev (2021) 2021:9925561. doi: 10.1155/2021/9925561
139. Yang C, Mo Y, Xu E, Wen H, Wei R, Li S, et al. Astragaloside IV Ameliorates Motor Deficits and Dopaminergic Neuron Degeneration via Inhibiting Neuroinflammation and Oxidative Stress in a Parkinson's Disease Mouse Model. Int Immunopharmacol (2019) 75:105651. doi: 10.1016/j.intimp.2019.05.036
140. Xu Y, Gao X, Wang L, Yang M, Xie R. Bakuchiol Ameliorates Cerebral Ischemia-Reperfusion Injury by Modulating NLRP3 Inflammasome and Nrf2 Signaling. Respir Physiol Neurobiol (2021) 292:103707. doi: 10.1016/j.resp.2021.103707
141. Tao W, Hu Y, Chen Z, Dai Y, Hu Y, Qi M. Magnolol Attenuates Depressive-Like Behaviors by Polarizing Microglia Towards the M2 Phenotype Through the Regulation of Nrf2/HO-1/NLRP3 Signaling Pathway. Phytomedicine (2021) 91:153692. doi: 10.1016/j.phymed.2021.153692
142. Yu Y, Wu DM, Li J, Deng SH, Liu T, Zhang T, et al. Bixin Attenuates Experimental Autoimmune Encephalomyelitis by Suppressing TXNIP/NLRP3 Inflammasome Activity and Activating NRF2 Signaling. Front Immunol (2020) 11:593368. doi: 10.3389/fimmu.2020.593368
143. Arruri VK, Gundu C, Kalvala AK, Sherkhane B, Khatri DK, Singh SB. Carvacrol Abates NLRP3 Inflammasome Activation by Augmenting Keap1/Nrf-2/P62 Directed Autophagy and Mitochondrial Quality Control in Neuropathic Pain. Nutr Neurosci (2021), 1–16. doi: 10.1080/1028415X.2021.1892985
144. Gao W, Wang W, Liu G, Zhang J, Yang J, Deng Z. Allicin Attenuated Chronic Social Defeat Stress Induced Depressive-Like Behaviors Through Suppression of NLRP3 Inflammasome. Metab Brain Dis (2019) 34:319–29. doi: 10.1007/s11011-018-0342-z
145. Jiang W, Li M, He F, Bian Z, He Q, Wang X, et al. Neuroprotective Effect of Asiatic Acid Against Spinal Cord Injury in Rats. Life Sci (2016) 157:45–51. doi: 10.1016/j.lfs.2016.05.004
146. Bian H, Wang G, Huang J, Liang L, Zheng Y, Wei Y, et al. Dihydrolipoic Acid Protects Against Lipopolysaccharide-Induced Behavioral Deficits and Neuroinflammation via Regulation of Nrf2/HO-1/NLRP3 Signaling in Rat. J Neuroinflamm (2020) 17:166. doi: 10.1186/s12974-020-01836-y
147. Shaheen MJ, Bekdash AM, Itani HA, Borjac JM. Saffron Extract Attenuates Neuroinflammation in rmTBI Mouse Model by Suppressing NLRP3 Inflammasome Activation via SIRT1. PloS One (2021) 16:e0257211. doi: 10.1371/journal.pone.0257211
148. De Paula Martins R, Ghisoni K, Lim CK, Aguiar AS Jr., Guillemin GJ, Latini A. Neopterin Preconditioning Prevents Inflammasome Activation in Mammalian Astrocytes. Free Radic Biol Med (2018) 115:371–82. doi: 10.1016/j.freeradbiomed.2017.11.022
149. Dinesh P, Rasool M. Berberine, an Isoquinoline Alkaloid Suppresses TXNIP Mediated NLRP3 Inflammasome Activation in MSU Crystal Stimulated RAW 264.7 Macrophages Through the Upregulation of Nrf2 Transcription Factor and Alleviates MSU Crystal Induced Inflammation in Rats. Int Immunopharmacol (2017) 44:26–37. doi: 10.1016/j.intimp.2016.12.031
150. Li Q, Wu J, Huang L, Zhao B, Li Q. Ephedrine Ameliorates Cerebral Ischemia Injury via Inhibiting NOD-Like Receptor Pyrin Domain 3 Inflammasome Activation Through the Akt/GSK3beta/NRF2 Pathway. Hum Exp Toxicol (2021) 40:S540–52. doi: 10.1177/09603271211052981
151. Yang X, Ji J, Liu C, Zhou M, Li H, Ye S, et al. HJ22, a Novel Derivative of Piperine, Attenuates Ibotenic Acid-Induced Cognitive Impairment, Oxidativestress, Apoptosis and Inflammation via Inhibiting the Protein-Protein Interaction of Keap1-Nrf2. Int Immunopharmacol (2020) 83:106383. doi: 10.1016/j.intimp.2020.106383
152. Yang X, Zhi J, Leng H, Chen Y, Gao H, Ma J, et al. The Piperine Derivative HJ105 Inhibits Abeta1-42-Induced Neuroinflammation and Oxidative Damage via the Keap1-Nrf2-TXNIP Axis. Phytomedicine (2021) 87:153571. doi: 10.1016/j.phymed.2021.153571
153. Wang H, Lv J, Jiang N, Huang H, Wang Q, Liu X. Ginsenoside Re Protects Against Chronic Restraint Stress-Induced Cognitive Deficits Through Regulation of NLRP3 and Nrf2 Pathways in Mice. Phytother Res (2021) 35:2523–5. doi: 10.1002/ptr.6947
154. Zhang Z, Yang H, Yang J, Xie J, Xu J, Liu C, et al. Pseudoginsenoside-F11 Attenuates Cognitive Impairment by Ameliorating Oxidative Stress and Neuroinflammation in Dgalactose-Treated Mice. Int Immunopharmacol (2019) 67:78–86. doi: 10.1016/j.intimp.2018.11.026
155. Arioz BI, Tarakcioglu E, Olcum M, Genc S. The Role of Melatonin on NLRP3 Inflammasome Activation in Diseases. Antioxidants (Basel) (2021) 10(7):1020. doi: 10.3390/antiox10071020
156. Madhu LN, Kodali M, Attaluri S, Shuai B, Melissari L, Rao X, et al. Melatonin Improves Brain Function in a Model of Chronic Gulf War Illness With Modulation of Oxidative Stress, NLRP3 Inflammasomes, and BDNF-ERK-CREB Pathway in the Hippocampus. Redox Biol (2021) 43:101973. doi: 10.1016/j.redox.2021.101973
157. Liu H, Wu X, Luo J, Zhao L, Li X, Guo H, et al. Adiponectin Peptide Alleviates Oxidative Stress and NLRP3 Inflammasome Activation After Cerebral Ischemia-Reperfusion Injury by Regulating AMPK/GSK-3beta. Exp Neurol (2020) 329:113302. doi: 10.1016/j.expneurol.2020.113302
158. Cheng Y, Chen B, Xie W, Chen Z, Yang G, Cai Y, et al. Ghrelin Attenuates Secondary Brain Injury Following Intracerebral Hemorrhage by Inhibiting NLRP3 Inflammasome Activation and Promoting Nrf2/ARE Signaling Pathway in Mice. Int Immunopharmacol (2020) 79:106180. doi: 10.1016/j.intimp.2019.106180
159. Luo X, Zeng H, Fang C, Zhang BH. N-Acetylserotonin Derivative Exerts a Neuroprotective Effect by Inhibiting the NLRP3 Inflammasome and Activating the PI3K/Akt/Nrf2 Pathway in the Model of Hypoxic-Ischemic Brain Damage. Neurochem Res (2021) 46:337–48. doi: 10.1007/s11064-020-03169-x
160. Hou Y, Wang Y, He Q, Li L, Xie H, Zhao Y, et al. Nrf2 Inhibits NLRP3 Inflammasome Activation Through Regulating Trx1/TXNIP Complex in Cerebral Ischemia Reperfusion Injury. Behav Brain Res (2018) 336:32–9. doi: 10.1016/j.bbr.2017.06.027
161. Xu X, Zhang L, Ye X, Hao Q, Zhang T, Cui G, et al. Nrf2/ARE Pathway Inhibits ROS-Induced NLRP3 Inflammasome Activation in BV2 Cells After Cerebral Ischemia Reperfusion. Inflammation Res (2018) 67:57–65. doi: 10.1007/s00011-017-1095-6
162. Yu J, Wang WN, Matei N, Li X, Pang JW, Mo J, et al. Ezetimibe Attenuates Oxidative Stress and Neuroinflammation via the AMPK/Nrf2/TXNIP Pathway After MCAO in Rats. Oxid Med Cell Longev (2020) 2020:4717258. doi: 10.1155/2020/4717258
163. Li W, Ali T, He K, Liu Z, Shah FA, Ren Q, et al. Ibrutinib Alleviates LPS-Induced Neuroinflammation and Synaptic Defects in a Mouse Model of Depression. Brain Behav Immun (2021) 92:10–24. doi: 10.1016/j.bbi.2020.11.008
164. Shan W, Liao X, Tang Y, Liu J. Dexmedetomidine Alleviates Inflammation in Neuropathic Pain by Suppressing NLRP3 via Nrf2 Activation. Exp Ther Med (2021) 22:1046. doi: 10.3892/etm.2021.10479
165. Wang N, Nie H, Zhang Y, Han H, Wang S, Liu W, et al. Dexmedetomidine Exerts Cerebral Protective Effects Against Cerebral Ischemic Injury by Promoting the Polarization of M2 Microglia via the Nrf2/HO-1/NLRP3 Pathway. Inflamm Res (2022) 71:93–106. doi: 10.1007/s00011-021-01515-5
166. Fahmy EK, El-Sherbiny M, Said E, Elkattawy HA, Qushawy M, Elsherbiny N. Tranilast Ameliorated Subchronic Silver Nanoparticles-Induced Cerebral Toxicity in Rats: Effect on TLR4/NLRP3 and Nrf-2. Neurotoxicology (2021) 82:167–76. doi: 10.1016/j.neuro.2020.12.008
167. Derangula K, Javalgekar M, Kumar Arruri V, Gundu C, Kumar Kalvala A, Kumar A. Probucol Attenuates NF-Kappab/NLRP3 Signalling and Augments Nrf-2 Mediated Antioxidant Defence in Nerve Injury Induced Neuropathic Pain. Int Immunopharmacol (2022) 102:108397. doi: 10.1016/j.intimp.2021.108397
168. Bayazid AB, Jang YA, Kim YM, Kim JG, Lim BO. Neuroprotective Effects of Sodium Butyrate Through Suppressing Neuroinflammation and Modulating Antioxidant Enzymes. Neurochem Res (2021) 46:2348–58. doi: 10.1007/s11064-021-03369-z
169. Zhang C, Wang H, Liang W, Yang Y, Cong C, Wang Y, et al. Diphenyl Diselenide Protects Motor Neurons Through Inhibition of Microglia-Mediated Inflammatory Injury in Amyotrophic Lateral Sclerosis. Pharmacol Res (2021) 165:105457. doi: 10.1016/j.phrs.2021.105457
170. Xu LL, Wu YF, Yan F, Li CC, Dai Z, You QD, et al. 5-(3,4-Difluorophenyl)-3-(6-Methylpyridin-3-Yl)-1,2,4-Oxadiazole (DDO-7263), a Novel Nrf2 Activator Targeting Brain Tissue, Protects Against MPTP-Induced Subacute Parkinson's Disease in Mice by Inhibiting the NLRP3 Inflammasome and Protects PC12 Cells Against Oxidative Stress. Free Radic Biol Med (2019) 134:288–303. doi: 10.1016/j.freeradbiomed.2019.01.003
171. Wang Q, Li WX, Dai SX, Guo YC, Han FF, Zheng JJ, et al. Meta-Analysis of Parkinson's Disease and Alzheimer's Disease Revealed Commonly Impaired Pathways and Dysregulation of NRF2-Dependent Genes. J Alzheimers Dis (2017) 56:1525–39. doi: 10.3233/JAD-161032
172. Paupe V, Dassa EP, Goncalves S, Auchere F, Lonn M, Holmgren A, et al. Impaired Nuclear Nrf2 Translocation Undermines the Oxidative Stress Response in Friedreich Ataxia. PloS One (2009) 4:e4253. doi: 10.1371/journal.pone.0004253
173. Prasad KN, Bondy SC. Inhibition of Early Biochemical Defects in Prodromal Huntington's Disease by Simultaneous Activation of Nrf2 and Elevation of Multiple Micronutrients. Curr Aging Sci (2016) 9:61–70. doi: 10.2174/1874609809666151124231127
174. Dinkova-Kostova AT, Kostov RV, Kazantsev AG. The Role of Nrf2 Signaling in Counteracting Neurodegenerative Diseases. FEBS J (2018) 285:3576–90. doi: 10.1111/febs.14379
175. Fao L, Mota SI, Rego AC. Shaping the Nrf2-ARE-Related Pathways in Alzheimer's and Parkinson's Diseases. Ageing Res Rev (2019) 54:100942. doi: 10.1016/j.arr.2019.100942
176. Saha S, Buttari B, Profumo E, Tucci P, Saso L. A Perspective on Nrf2 Signaling Pathway for Neuroinflammation: A Potential Therapeutic Target in Alzheimer's and Parkinson's Diseases. Front Cell Neurosci (2021) 15:787258. doi: 10.3389/fncel.2021.787258
177. Ahuja M, Ammal Kaidery N, Yang L, Calingasan N, Smirnova N, Gaisin A, et al. Distinct Nrf2 Signaling Mechanisms of Fumaric Acid Esters and Their Role in Neuroprotection Against 1-Methyl-4-Phenyl-1,2,3,6-Tetrahydropyridine-Induced Experimental Parkinson's-Like Disease. J Neurosci (2016) 36:6332–51. doi: 10.1523/JNEUROSCI.0426-16.2016
178. Robledinos-Anton N, Fernandez-Gines R, Manda G, Cuadrado A. Activators and Inhibitors of NRF2: A Review of Their Potential for Clinical Development. Oxid Med Cell Longev (2019) 2019:9372182. doi: 10.1155/2019/9372182
179. Pallesen JS, Tran KT, Bach A. Non-Covalent Small-Molecule Kelch-Like ECH-Associated Protein 1-Nuclear Factor Erythroid 2-Related Factor 2 (Keap1-Nrf2) Inhibitors and Their Potential for Targeting Central Nervous System Diseases. J Med Chem (2018) 61:8088–103. doi: 10.1021/acs.jmedchem.8b00358
180. Dhakshinamoorthy S, Jain AK, Bloom DA, Jaiswal AK. Bach1 Competes With Nrf2 Leading to Negative Regulation of the Antioxidant Response Element (ARE)-Mediated NAD(P)H:quinone Oxidoreductase 1 Gene Expression and Induction in Response to Antioxidants. J Biol Chem (2005) 280:16891–900. doi: 10.1074/jbc.M500166200
181. Jain A, Lamark T, Sjottem E, Larsen KB, Awuh JA, Overvatn A, et al. P62/SQSTM1 is a Target Gene for Transcription Factor NRF2 and Creates a Positive Feedback Loop by Inducing Antioxidant Response Element-Driven Gene Transcription. J Biol Chem (2010) 285:22576–91. doi: 10.1074/jbc.M110.118976
182. Shao BZ, Cao Q, Liu C. Targeting NLRP3 Inflammasome in the Treatment of CNS Diseases. Front Mol Neurosci (2018) 11:320. doi: 10.3389/fnmol.2018.00320
183. Lozanovski VJ, Polychronidis G, Gross W, Gharabaghi N, Mehrabi A, Hackert T, et al. Broccoli Sprout Supplementation in Patients With Advanced Pancreatic Cancer is Difficult Despite Positive Effects-Results From the POUDER Pilot Study. Invest New Drugs (2020) 38:776–84. doi: 10.1007/s10637-019-00826-z
184. Rabbani N, Xue M, Weickert MO, Thornalley PJ. Reversal of Insulin Resistance in Overweight and Obese Subjects by Trans-Resveratrol and Hesperetin Combination-Link to Dysglycemia, Blood Pressure, Dyslipidemia, and Low-Grade Inflammation. Nutrients (2021) 13(7):2374. doi: 10.3390/nu13072374
185. Lastra D, Fernandez-Gines R, Manda G, Cuadrado A. Perspectives on the Clinical Development of NRF2-Targeting Drugs. Handb Exp Pharmacol (2021) 264:93–141. doi: 10.1007/164_2020_381
186. Rosito M, Testi C, Parisi G, Cortese B, Baiocco P, Di Angelantonio S. Exploring the Use of Dimethyl Fumarate as Microglia Modulator for Neurodegenerative Diseases Treatment. Antioxidants (Basel) (2020) 9(8):700. doi: 10.3390/antiox9080700
187. Schimrigk S, Brune N, Hellwig K, Lukas C, Bellenberg B, Rieks M, et al. Oral Fumaric Acid Esters for the Treatment of Active Multiple Sclerosis: An Open-Label, Baseline-Controlled Pilot Study. Eur J Neurol (2006) 13:604–10. doi: 10.1111/j.1468-1331.2006.01292.x
188. Gold R, Kappos L, Arnold DL, Bar-Or A, Giovannoni G, Selmaj K, et al. Placebo-Controlled Phase 3 Study of Oral BG-12 for Relapsing Multiple Sclerosis. N Engl J Med (2012) 367:1098–107. doi: 10.1056/NEJMoa1114287
189. Gold R, Arnold DL, Bar-Or A, Fox RJ, Kappos L, Mokliatchouk O, et al. Long-Term Safety and Efficacy of Dimethyl Fumarate for Up to 13 Years in Patients With Relapsing-Remitting Multiple Sclerosis: Final ENDORSE Study Results. Mult Scler (2021), 13524585211037909. doi: 10.1177/13524585211037909
190. Ruggieri S, Tortorella C, Gasperini C. Pharmacology and Clinical Efficacy of Dimethyl Fumarate (BG-12) for Treatment of Relapsing-Remitting Multiple Sclerosis. Ther Clin Risk Manag (2014) 10:229–39. doi: 10.2147/TCRM.S53285
191. Paik J. Diroximel Fumarate in Relapsing Forms of Multiple Sclerosis: A Profile of Its Use. CNS Drugs (2021) 35:691–700. doi: 10.1007/s40263-021-00830-z
192. Lynch DR, Chin MP, Delatycki MB, Subramony SH, Corti M, Hoyle JC, et al. Safety and Efficacy of Omaveloxolone in Friedreich Ataxia (MOXIe Study). Ann Neurol (2021) 89:212–25. doi: 10.1002/ana.25934
193. Lovestone S, Boada M, Dubois B, Hull M, Rinne JO, Huppertz HJ, et al. A Phase II Trial of Tideglusib in Alzheimer's Disease. J Alzheimers Dis (2015) 45:75–88. doi: 10.3233/JAD-141959
194. Martinez-Gonzalez L, Gonzalo-Consuegra C, Gomez-Almeria M, Porras G, De Lago E, Martin-Requero A, et al. Tideglusib, a Non-ATP Competitive Inhibitor of GSK-3beta as a Drug Candidate for the Treatment of Amyotrophic Lateral Sclerosis. Int J Mol Sci (2021) 22(16):8975. doi: 10.3390/ijms22168975
195. Grossman SA, Ye X, Peereboom D, Rosenfeld MR, Mikkelsen T, Supko JG, et al. Phase I Study of Terameprocol in Patients With Recurrent High-Grade Glioma. Neuro Oncol (2012) 14:511–7. doi: 10.1093/neuonc/nor230
196. Kiser C, Gonul CP, Olcum M, Genc S. Inhibitory Effects of Sulforaphane on NLRP3 Inflammasome Activation. Mol Immunol (2021) 140:175–85. doi: 10.1016/j.molimm.2021.10.014
197. Zolnourian AH, Franklin S, Galea I, Bulters DO. Study Protocol for SFX-01 After Subarachnoid Haemorrhage (SAS): A Multicentre Randomised Double-Blinded, Placebo Controlled Trial. BMJ Open (2020) 10:e028514. doi: 10.1136/bmjopen-2018-028514
198. Hellwig K, Rog D, Mcguigan C, Houtchens MK, Bruen DR, Mokliatchouk O, et al. Interim Analysis of Pregnancy Outcomes After Exposure to Dimethyl Fumarate in a Prospective International Registry. Neurol Neuroimmunol Neuroinflamm (2022) 9. doi: 10.1212/NXI.0000000000001114
199. Chaves C, Ganguly R, Ceresia C, Camac A. Lymphocyte Subtypes in Relapsing-Remitting Multiple Sclerosis Patients Treated With Dimethyl Fumarate. Mult Scler J Exp Transl Clin (2017) 3:2055217317702933. doi: 10.1177/2055217317702933
Keywords: NLRP3 inflammasome, Nrf2, inflammation, central nervous system, dimethyl fumarate, sulforaphane
Citation: Tastan B, Arioz BI and Genc S (2022) Targeting NLRP3 Inflammasome With Nrf2 Inducers in Central Nervous System Disorders. Front. Immunol. 13:865772. doi: 10.3389/fimmu.2022.865772
Received: 30 January 2022; Accepted: 04 March 2022;
Published: 28 March 2022.
Edited by:
Pietro Ghezzi, Brighton and Sussex Medical School, United KingdomReviewed by:
Elena Milanesi, Victor Babes National Institute of Pathology (INCDVB), RomaniaMichael Sporn, Triterpenoid Therapeutics, United States
Paolo Tucci, University of Foggia, Italy
Copyright © 2022 Tastan, Arioz and Genc. This is an open-access article distributed under the terms of the Creative Commons Attribution License (CC BY). The use, distribution or reproduction in other forums is permitted, provided the original author(s) and the copyright owner(s) are credited and that the original publication in this journal is cited, in accordance with accepted academic practice. No use, distribution or reproduction is permitted which does not comply with these terms.
*Correspondence: Sermin Genc, c2VybWluLmdlbmNAZGV1LmVkdS50cg==