- 1Department of Anatomy, Yong Loo Lin School of Medicine, National University of Singapore, Singapore, Singapore
- 2Division of Pathology, Singapore General Hospital, Singapore, Singapore
- 3Department of Biomolecular and Chemical Engineering, College of Design and Engineering, National University of Singapore, Singapore, Singapore
- 4Duke-NUS Medical School, Singapore, Singapore
Breast cancer remains the most common malignancy among women worldwide. Although the implementation of mammography has dramatically increased the early detection rate, conventional treatments like chemotherapy, radiation therapy, and surgery, have significantly improved the prognosis for breast cancer patients. However, about a third of treated breast cancer patients are known to suffer from disease recurrences and progression to metastasis. Immunotherapy has recently gained traction due to its ability to establish long-term immune surveillance, and response for the prevention of disease recurrence and extension of patient survival. Current research findings have revealed that gold nanoparticles can enhance the safety and efficacy of cancer immunotherapy, through their unique intrinsic properties of good biocompatibility, durability, convenient surface modification, as well as enhanced permeability and retention effect. Gold nanoparticles are also able to induce innate immune responses through the process of immunogenic cell death, which can lead to the establishment of lasting adaptive immunity. As such gold nanoparticles are considered as good candidates for next generation immunotherapeutic strategies. This mini review gives an overview of gold nanoparticles and their potential applications in breast cancer immunotherapeutic strategies.
Introduction
As reported in GLOBOCAN 2018, breast cancer has the second highest incidence globally, with an incidence rate that has been increasing in both developing and developed countries in comparison with other cancers (1). Breast cancer is much more prevalent in women (2), and contributes most of the cancer-associated morbidity and mortality for females worldwide (3).
Breast cancer is characterized by uncontrollable neoplastic growth in the breast (4), through a multistep tumorigenesis process involving many complex molecular mechanisms (5). This heterogenous cancer may arise from environmental influences, genetic alterations, metabolic changes, or a combination of these factors (6, 7). Although there are many morphological variants of breast cancer, the most common is ductal carcinoma which encompasses about 80% of all diagnosed breast malignancies (8). Ductal carcinoma can be further classified by their molecular subtypes based on the expression levels of estrogen receptor, progesterone receptor, and human epidermal growth factor receptor 2 (HER2) (9–13). Different prognoses have been reported for various breast cancer subtypes, and therapeutic strategies that target these subgroups were therefore developed accordingly (14, 15).
The success rate for breast cancer therapy is largely determined by the point of detection, where the overall mortality rate can be reduced by 20% with early diagnosis (16). The inception of mammography has significantly increased early detection of breast malignancies among females, as clinical and self-examinations for the detection of early abnormalities in the breast have always been challenging (17).
Conventional treatments for breast cancer, like chemotherapy, radiation therapy (RT), and surgery have significantly improved both disease-free survival and overall survival (OS) (18, 19). Unfortunately, post-treatment recurrences of this disease remain a formidable challenge for clinicians (20). Immunotherapy has recently emerged as a promising anti-cancer strategy for prolonged survival in breast cancer patients (4). This novel anti-tumor approach allows the activation and stimulation of the host immune system to recognize and eradicate tumor cells, as well as establish a long-term immunological memory to prevent tumor recurrences. However, this relatively new form of therapy has its own fair share of clinical safety concerns and efficacy issues (20). Lately, the concept of incorporating nanotechnology into cancer immunotherapy, has also been gaining traction (21). Because of its multiple surface functionalities and unique properties, gold nanoparticles (AuNPs) are actively being researched as cancer diagnostics and therapeutics (22). This mini review seeks to highlight the perspectives and potential of AuNP-based platforms as a strategic approach in breast cancer immunotherapy.
Current Treatment for Breast Cancer
Breast cancer diagnostics and therapeutics have made considerable progress in the last decade due to better understanding of disease pathogenesis (23). Current treatments for breast cancer are chemotherapy, RT, and surgery. Although these approaches have been widely employed by clinicians for decades, they have their own limitations and challenges (24). Chemotherapy uses cytotoxic drugs, like 5-fluorouracil, anthracyclines, carboplatin, cyclophosphamide, and taxanes, to eliminate rapidly proliferating cells that include both tumor and normal cells (like immune and epithelial cells) (25). Although the survival rate for breast cancer patients under the age of 50 years, can be improved (by up to 15 years) by 10% and those older by 3% (26), the non-targeted nature of chemotherapy brings unwanted systemic damage to the host body (24). RT employs high doses of radiation, such as γ-rays and X-rays, to destroy tumor cells and shrink tumor size. Like chemotherapy, it also damages healthy cells and tissues near the treatment area (27). Surgery removes tumors that are accessible, large, and resectable (24). Although the prognosis is highly favorable when early diagnosis of breast cancer is met with conventional treatments (14), nearly a third of these patients still suffer from disease recurrences and progression to metastasis (18).
Compared to conventional chemoradiotherapeutic approaches, the emergence of cancer immunotherapy is attributed to its potential to provide better prognosis and prevent disease recurrences (20). Immunotherapy is designed to enhance and/or restore the host immunity to seek and eliminate cancer cells (28). Although more immunotherapeutic drugs and combinatorial treatment strategies involving immunotherapies have been approved lately (29), there are still issues with autoimmunity and systemic inflammation (30), as well as challenges in targeting solid tumors (31). Fortunately, the incorporation of nanotechnology can potentially enhance both the efficacy and delivery of these immunotherapeutic drugs (32).
Nanoparticles in Breast Cancer Nanomedicine
Nanotechnology is defined as the manipulation of materials with dimensions from 1 nm to 100 nm (33). Due to their small physical size, nanomaterials offer unique physical and chemical characteristics that are distinct from their bulky counterparts (34). NPs can be generally classified under organic NPs or inorganic NPs (35).
Organic NPs, like liposomes, polylactic-co-glycolic acid (PLGA) NPs, and extracellular vesicles (EVs), are known to have high drug delivery efficiency and low toxicity (36). Liposomes were the first nanomedicine approved for clinical use (37), and their application in breast cancer has been gaining popularity (38, 39). Liposome has an outer lipid layer that can be modified to mimic the biophysical properties of the host cells, and a core carrying its cargo, usually chemotherapeutic agents (40, 41). Unlike its non-biodegradable predecessors, PLGA NP is a clinically approved copolymer made of lactic and glycolic acids, which can be hydrolyzed into normal metabolites under physiological conditions, and has excellent in vivo biocompatibility (42, 43). Like liposomes, PLGA NPs are used as nanocarriers for breast cancer therapy. EVs are double-layered phospholipid vesicles secreted by cells (44), and can be categorized into apoptotic bodies, exosomes, or microvesicles (45). Doxorubicin (DOX)-loaded into exosomes could be used for breast cancer treatment due to enhanced tumoricidal activity and reduced cardiotoxicity (46). More information on the use of organic NPs in anti-cancer therapies can be found in the review paper by Karpinski and co-workers (44).
Inorganic NPs, such as carbon nanotubes (CNTs), quantum dots (QDs), and metallic NPs, have higher modifiable surface area to volume ratio that can be easily functionalized (47, 48). Discovered in 1980s, CNTs are rolls of graphene that can be categorized as either single-walled or multi-walled (44). In addition to being good nanocarriers due to their unique biological, chemical, and physical properties, they can also be used for photothermal therapy (PTT) when exposed to near-infrared radiation (NIR) (49). QDs are nanoscale semiconductors that exhibit a quantum confinement (50), and have high photostability with a broad spectrum of absorption and narrow emission bands, allowing them to be mainly used as an imaging modality and/or a therapeutic agent for photodynamic therapy (PDT) and/or PTT (51). However, the main limitation for QDs is the lack of an optimized production process (44). For metallic NPs, AuNPs have been widely studied for use in breast cancer diagnostics and therapeutics (21, 52). Hence, their potential to be incorporated into the next generation of breast cancer immunotherapeutic strategies will be discussed in the subsequent sections.
Anti-Cancer Properties of Gold Nanoparticles
AuNPs can easily be synthesized from the reduction of gold salts (21), into variable sizes and shapes including decahedron, hexagon, icosahedron, octahedron, sub-octahedron, nanocage, nanoprism, nanorod, nanosphere, and nanostar (53, 54). Smaller sized AuNPs allow for greater tissue distribution, deeper tissue penetration, and increased cellular internalization (55). This is largely attributed to the phenomenon, known as the enhanced permeability and retention (EPR) effect, where the nano-sized effect of AuNPs allows for greater accumulation in tumor tissues than healthy tissues, due to their extravasation into the tumor microenvironment (TME) through the wide fenestrations of the angiogenic vascular architecture, as well as the lack of normal lymphatic drainage (56). Hence, this permits AuNPs to achieve passive targeting to tumor tissues in vivo.
The toxicity of AuNPs is also dependent on their shape, size, surface charge, surface chemistry, coating, and contaminants (57). The cytotoxic feature of AuNPs is attributed to their ability to induce oxidative stress (24). There are studies that report AuNPs are non-toxic although there are conflicting reports that state otherwise (58). AuNPs are popular for diagnostic and therapeutic uses due to their unique catalytic, electronic, magnetic, optical, physical, and structural properties (21, 22, 52, 59). Chemicals, drugs, natural products, and/or probes can bind to the negatively charged surfaces of AuNPs to potentiate their therapeutic efficacy (60–64).
Gold Nanoparticle-Based Targeted Therapy
Targeted therapy has been deemed safer than traditional therapies, as the latter usually result in collateral damage to healthy cells and tissues due to their non-specificity. Thus, breast cancer patients are expected to suffer less side effects from targeted therapies (4). Trastuzumab (Tz) or Herceptin is the first humanized monoclonal antibody (mAb) to be approved for routine clinical use as targeted therapy for HER2+ metastatic breast cancer in 1998 (65, 66). HER2 is responsible for cell proliferation in normal cells but an overexpression of these receptors can promote unrestricted cell growth and eventually tumorigenesis (67). HER2 can be neutralized, internalized, and downregulated upon the binding of Tz, with concomitant upregulation of p21Waf1 and p27Kip1 (68). Tz is also known to cause antibody-dependent cellular cytotoxicity on its target cells (69), whereby cells marked with Tz can be targeted for cell lysis by natural killer cells (70).
Interestingly, AuNPs can be conjugated to Tz to allow specific active targeting to breast tumor cells. This concept has been successfully demonstrated in both in vitro experimentation and using a subcutaneous MCF-7 human breast cancer murine model (71). Although there was an increase in targeting efficiency for AuNPs that were conjugated with two mAbs for in vitro experiments, in vivo results showed that one mAb per AuNP (5NP-1Tz) produced the best tumor homing and protracted therapeutic efficacy (71). By conventional wisdom, one may think that a higher number of mAbs on a AuNP can confer superior binding ability. However, a high density of mAbs per AuNP may introduce steric hindrance that reduces the access to its target antigen (71). Hence, it is important to adjust the number of mAbs conjugated per AuNP to achieve the desired active targeting efficacy. Additionally, the same in vivo study has shown that long-term intra-tumoral retention of 5NP-1Tz produced continuous therapeutic effect over time (71).
Immunotherapy with Gold Nanoparticles as Delivery Vehicles
Owing to their EPR effect, AuNPs are able to specifically accumulate in tumor sites and lymph nodes, which allow them to be highly effective delivery vehicles for immunological reagents (30). They are also easily synthesized into different sizes and shapes, to modify properties such as cytotoxicity, distribution, immunogenicity, and metabolism, for various therapeutic uses (20). The high molecular density on their surface and ease of modifications allow conjugation of different molecules like polyethylene glycol (PEG) and arginine-glycine-aspartic acid tripeptide, to improve the overall pharmacodynamics and pharmacokinetics of AuNPs and its cargo (72). This phenomenon has already been elegantly and successfully demonstrated in an in vivo study, where chimeric peptides (glycopeptide sequence from mucin-1 and T cell epitope P30 sequence) were attached to the PEGylated AuNPs (PEG-AuNPs), to be used as an anti-cancer vaccination strategy against MCF-7 human breast cancer cells (73).
AuNPs can also function as protective delivery vehicles for compounds that have low intracellular accumulation, such as siRNA, and/or vulnerable to intrinsic enzymatic degradation (5). Thus, the incorporation of AuNPs as nanocarriers in the drug delivery strategy, not only helps with tumor site targeting, but can also improve the solubility and stability of their sensitive cargo and prolong the half-life (74). Studies have demonstrated the potent immunomodulatory effects of Ganoderma lucidum polysaccharide (GLP) on the maturation of dendritic cells (DCs) (75, 76). However, due to their low prescribed dosages and rapid clearances, the efficacy of GLP for clinical applications remains questionable. One study showed that the half-life of GLP in the blood can be extended and their accumulation in immune organs can be increased, when AuNPs were used as delivery vehicles for GLP (GLP-Au) (77). Compared to free GLP, the in vitro results in the same study have revealed a stronger DC activation and T cell response by GLP-Au. Moreover, combining GLP-Au with DOX produced stronger inhibitory effect against 4T1 murine tumor growth and pulmonary metastasis than free GLP with DOX. Additionally, the activated DCs were also observed to induce both CD4+ and CD8+ T cell expansion in the spleen.
Radiation Therapy with Gold Nanoparticles
RT is one of the least invasive standard of care that has been prescribed for over half of cancer patients (78–80). RT exerts cytotoxic and cytostatic effects via DNA damage by fractionated focal irradiation (81). However, these irradiations are unable to differentiate tumor cells from normal cells which lead to unnecessary destruction of surrounding healthy tissues (82). Additionally, its tumoricidal ability is often limited by the maximum deposited dosage and that has always remained a challenge among radiologists (83). Hence, there is always a clinical interest to specifically maximize the RT dosage on the tumor and minimize the collateral damage to the neighboring healthy tissues (84).
Fortunately, metal radiosensitizers, such as AuNPs, can increase the sensitivity of tumor cells to irradiation due to its high atomic number granting high absorption coefficient (85–87). Generation of reactive oxygen species (ROS) can be induced by the Auger electron production from the surface of AuNPs (20). By coupling with an active targeting strategy or by the passive targeting of the EPR effect alone, using AuNPs as radiosensitizers allows the reduction of total RT dosage whilst increasing the local RT dosage to tumor sites (82). For example, conjugation of Tz to PEG-AuNPs (Tz-PEG-AuNPs) allowed intracellular uptake of AuNPs into SK-BR-3 human breast cancer cells, which then proceeded to cause DNA double-strand breaks by 3.3 to 5.1 times more than RT alone or RT with PEG-AuNPs (88).
The resultant cell death from RT, known as immunogenic cell death (ICD), can release tumor antigens and improve the host response to cancer immunotherapy through antigen presentation, cytokine secretion, and naïve T cell activation (89–95). Additionally, signals for anti-tumor immunity like adenosine triphosphate (ATP), nuclear protein high-mobility group box-1 (HMGB1) and calreticulin (CALR) are produced upon ICD (92, 96). The release of these danger-associated molecular patterns (DAMPs) help to recruit and activate antigen-presenting cells (APCs) of the innate immune response like DCs and macrophages, and also promote antigen uptake and presentation to CD8+ T cells for the establishment of long-term adaptive immunity (96, 97) (Figure 1). ATP interacts with purinergic receptor P2X 7 on DCs and activates the NOD-, LRR- and pyrin domain-containing protein 3 inflammasome for proteolysis, maturation and secretion of interleukins 18 and 1β (98). HMGB1 engages toll-like receptor 4 on macrophages to enhance the release of proinflammatory cytokines (99, 100), whereas CALR binds to the CD91 receptors on phagocytes to promote phagocytosis of dying and dead cells (101). This process is especially useful against immunologically cold tumors, like triple negative breast cancer, because the increase in TME immunogenicity was found to be correlated with better prognosis due to improved patient responses to immunotherapy and chemotherapy (102). RT with 14 nm AuNPs showed enhanced RT efficacy, and induced ICD that resulted in significant macrophage infiltration, and hence improved OS in an MDA-MB-231 human breast cancer murine model (82).
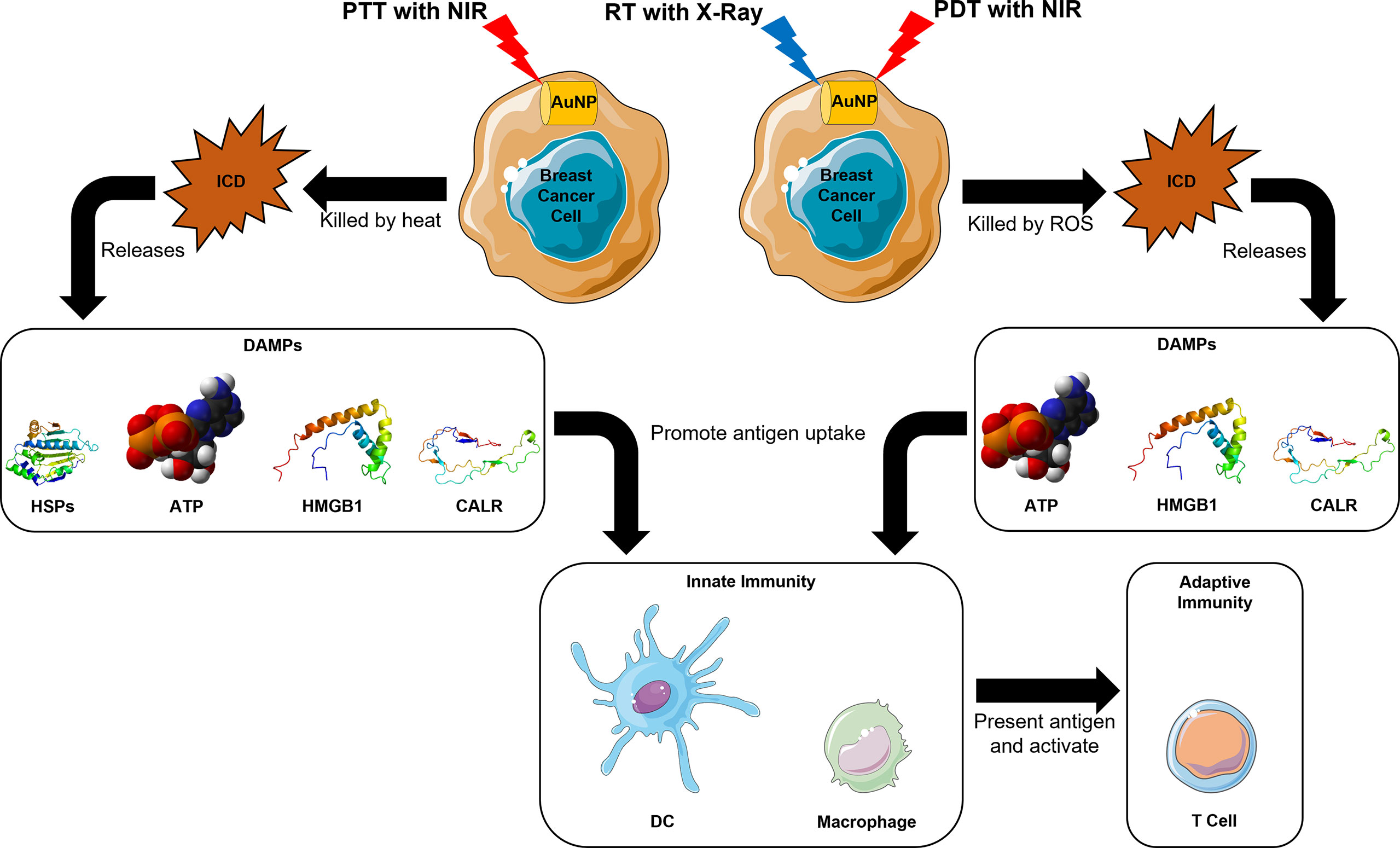
Figure 1 Mechanistic insights for induction of host immune system in AuNP-based RT, PDT, and PTT in breast cancer.
Photodynamic Therapy with Gold Nanoparticles
PDT is a cancer therapy that has spatiotemporal selectivity with minimal invasiveness (103). Its mechanism of action is to generate ROS to destroy tumor cells through a photochemical reaction, using light, photosensitizers like AuNPs, and oxygen (O2) from tissues (5, 20, 104). Briefly, accumulated AuNPs at the tumor site can be excited by NIR to generate ROS in the presence of surrounding O2, which can be devastating to the surrounding tumor cells (20). Just like RT with AuNPs, PDT with AuNPs is also able to trigger anti-cancer immune response through the ICD process (105) (Figure 1).
Unfortunately, PDT is often met with poor efficacy due to the hypoxic TME (106). In addition to promoting tumorigenesis and metastasis (107–109), a hypoxic TME is one of the primary reasons to immunosuppression due to the inhibition of T cell infiltration into the tumor site (110, 111). Hence, tackling tumor hypoxia should be considered before employing a PDT strategy (112). Recently, studies have shown that gold nanocages (AuNCs) have PDT potential due to their hollow porous structures (113, 114). An interesting in vivo study demonstrated the feasibility of using AuNC@manganese dioxide (AuNC@MnO2) for an O2-boosted immunogenic PDT in a 4T1 mouse model, in which MnO2 shells degrade under low pH and produce large amount of O2 to boost the PDT effect in a hypoxic TME (112).
Photothermal Therapy with Gold Nanoparticles
Similar to PDT, PTT is another non-invasive cancer therapy that utilizes AuNPs and NIR, but the only difference here is the generation of heat to destroy cancer cells (115). PTT is commonly employed to treat breast cancer by selectively ablating tumor cells (5). Due to their high photothermal conversion efficiency, AuNPs can reach an excited state through the absorption of light (78), at which vibrational energy is emitted as heat and can consequently destroy nearby cancer cells (116).
In addition to the ability to generate ICD in tumor cells like RT with AuNPs and PDT with AuNPs (117), PTT also cause heat-induced cytotoxicity (118). As a result, heat shock proteins (HSPs) are released, which can then form HSP-peptide complexes with tumor antigen peptides to enhance phagocytosis of dead cells and antigen presentation by APCs (119, 120) (Figure 1).
Conclusion and Perspectives
Current research in nanomedicine has shown that AuNPs have excellent biocompatibility and good resistance against degradation under physiological conditions, thus facilitating long-term therapeutic effects in breast cancer patients. Due to their EPR effect and the option to include active targeting strategies, both in vitro and in vivo studies have demonstrated the promising applications and feasibility of AuNPs in improving the efficacy of various therapies, especially in RT, PDT, and PTT. Additionally, the unique intrinsic properties of AuNPs in stimulating the host immune system through the induction of ICD allows the incorporation of immunotherapeutic strategies. A summary figure depicting a AuNP-based approach as a nanocarrier for immunotherapeutic drugs and stimulation of the host immune system mediated via RT, PDT, and PTT is shown in Figure 2. Although there is a lot of flexibility in the surface modifications of AuNPs, a re-evaluation of the pharmacology and toxicology of every additional modification will be required, to address any concerns regarding efficacy and safety. More work needs to be done before AuNPs can be routinely used for breast cancer immunotherapy. Nevertheless, recent preclinical data have adequately shown that AuNPs can serve as potential next generation immunomodulators for breast cancer therapy.
Author Contributions
X-YC wrote the manuscript. BHB, PHT, and L-YLY reviewed and edited the manuscript. All authors approved the manuscript for publication.
Funding
Kwan Im Thong Hood Cho Temple Professorship to BHB (WBS E-546-00-0042-03).
Conflict of Interest
The authors declare that the research was conducted in the absence of any commercial or financial relationships that could be construed as a potential conflict of interest.
Publisher’s Note
All claims expressed in this article are solely those of the authors and do not necessarily represent those of their affiliated organizations, or those of the publisher, the editors and the reviewers. Any product that may be evaluated in this article, or claim that may be made by its manufacturer, is not guaranteed or endorsed by the publisher.
References
1. Bray F, Ferlay J, Soerjomataram I, Siegel RL, Torre LA, Jemal A. Global Cancer Statistics 2018: Globocan Estimates of Incidence and Mortality Worldwide for 36 Cancers in 185 Countries. CA Cancer J Clin (2018) 68(6):394–424. doi: 10.3322/caac.21492
2. Becker S. A Historic and Scientific Review of Breast Cancer: The Next Global Healthcare Challenge. Int J Gynaecol Obstet (2015) 131(Suppl 1):S36–9. doi: 10.1016/j.ijgo.2015.03.015
3. Lukong KE. Understanding Breast Cancer - the Long and Winding Road. BBA Clin (2017) 7:64–77. doi: 10.1016/j.bbacli.2017.01.001
4. Chen XY, Tan PH. Therapeutic Potential of Lymphoid Infiltrates in Breast Cancer. Curr Med Chem (2021) 28(25):5152–78. doi: 10.2174/0929867328666210430132701
5. Khandker SS, Shakil MS, Hossen MS. Gold Nanoparticles; Potential Nanotheranostic Agent in Breast Cancer: A Comprehensive Review With Systematic Search Strategy. Curr Drug Metab (2020) 21(8):579–98. doi: 10.2174/1389200221666200610173724
6. Al-Hajj M, Wicha MS, Benito-Hernandez A, Morrison SJ, Clarke MF. Prospective Identification of Tumorigenic Breast Cancer Cells. Proc Natl Acad Sci U.S.A. (2003) 100(7):3983–8. doi: 10.1073/pnas.0530291100
7. Mishra P, Ambs S. Metabolic Signatures of Human Breast Cancer. Mol Cell Oncol (2015) 2(3):e992217. doi: 10.4161/23723556.2014.992217
8. Allison KH, Brogi E, Ellis IO, Fox SB, Morris EA, Sahin A, et al. WHO Classification of Tumours Editorial Board. In: Breast Tumours, 5 ed. Lyon: International Agency for Research on Cancer.
9. Bryan BB, Schnitt SJ, Collins LC. Ductal Carcinoma in Situ With Basal-Like Phenotype: A Possible Precursor to Invasive Basal-Like Breast Cancer. Mod Pathol (2006) 19(5):617–21. doi: 10.1038/modpathol.3800570
10. Clark SE, Warwick J, Carpenter R, Bowen RL, Duffy SW, Jones JL. Molecular Subtyping of Dcis: Heterogeneity of Breast Cancer Reflected in Pre-Invasive Disease. Br J Cancer (2011) 104(1):120–7. doi: 10.1038/sj.bjc.6606021
11. Livasy CA, Perou CM, Karaca G, Cowan DW, Maia D, Jackson S, et al. Identification of a Basal-Like Subtype of Breast Ductal Carcinoma in Situ. Hum Pathol (2007) 38(2):197–204. doi: 10.1016/j.humpath.2006.08.017
12. Muggerud AA, Hallett M, Johnsen H, Kleivi K, Zhou W, Tahmasebpoor S, et al. Molecular Diversity in Ductal Carcinoma in Situ (Dcis) and Early Invasive Breast Cancer. Mol Oncol (2010) 4(4):357–68. doi: 10.1016/j.molonc.2010.06.007
13. Chen X-Y, Thike AA, Koh VCY, Nasir NDMD, Bay BH, Tan PH. Breast Ductal Carcinoma in Situ Associated With Microinvasion Induces Immunological Response and Predicts Ipsilateral Invasive Recurrence. Virchows Arch (2021) 478(4):679–86. doi: 10.1007/s00428-020-02959-6
14. García-Aranda M, Redondo M. Protein Kinase Targets in Breast Cancer. Int J Mol Sci (2017) 18(12). doi: 10.3390/ijms18122543
15. Fragomeni SM, Sciallis A, Jeruss JS. Molecular Subtypes and Local-Regional Control of Breast Cancer. Surg Oncol Clin N Am (2018) 27(1):95–120. doi: 10.1016/j.soc.2017.08.005
16. Shah TA, Guraya SS. Breast Cancer Screening Programs: Review of Merits, Demerits, and Recent Recommendations Practiced Across the World. J Microsc Ultrastruct (2017) 5(2):59–69. doi: 10.1016/j.jmau.2016.10.002
17. Lee RJ, Vallow LA, McLaughlin SA, Tzou KS, Hines SL, Peterson JL. Ductal Carcinoma in Situ of the Breast. Int J Surg Oncol (2012) 2012:12. doi: 10.1155/2012/123549
18. Redig AJ, McAllister SS. Breast Cancer as a Systemic Disease: A View of Metastasis. J Intern Med (2013) 274(2):113–26. doi: 10.1111/joim.12084
19. Rossi L, Stevens D, Pierga JY, Lerebours F, Reyal F, Robain M, et al. Impact of Adjuvant Chemotherapy on Breast Cancer Survival: A Real-World Population. PloS One (2015) 10(7):e0132853. doi: 10.1371/journal.pone.0132853
20. He J-s, Liu S-j, Zhang Y-r, Chu X-d, Lin Z-b, Zhao Z, et al. The Application of and Strategy for Gold Nanoparticles in Cancer Immunotherapy. Front Pharmacol (2021) 12:687399(1430). doi: 10.3389/fphar.2021.687399
21. Guo J, Rahme K, He Y, Li LL, Holmes JD, O'Driscoll CM. Gold Nanoparticles Enlighten the Future of Cancer Theranostics. Int J Nanomedicine (2017) 12:6131–52. doi: 10.2147/ijn.S140772
22. Yeh Y-C, Creran B, Rotello VM. Gold Nanoparticles: Preparation, Properties, and Applications in Bionanotechnology. Nanoscale (2012) 4(6):1871–80. doi: 10.1039/C1NR11188D
23. Chen XY, Yeong J, Thike AA, Bay BH, Tan PH. Prognostic Role of Immune Infiltrates in Breast Ductal Carcinoma in Situ. Breast Cancer Res Treat (2019) 177:17–27. doi: 10.1007/s10549-019-05272-2
24. Lim Z-ZJ, Li J-EJ, Ng C-T, Yung L-YL, Bay B-H. Gold Nanoparticles in Cancer Therapy. Acta Pharmacol Sin (2011) 32(8):983–90. doi: 10.1038/aps.2011.82
25. Wagstaff KM, Jans DA. Nuclear Drug Delivery to Target Tumour Cells. Eur J Pharmacol (2009) 625(1-3):174–80. doi: 10.1016/j.ejphar.2009.06.069
26. Weigelt B, Peterse JL, van 't Veer LJ. Breast Cancer Metastasis: Markers and Models. Nat Rev Cancer (2005) 5(8):591–602. doi: 10.1038/nrc1670
27. Boisselier E, Astruc D. Gold Nanoparticles in Nanomedicine: Preparations, Imaging, Diagnostics, Therapies and Toxicity. Chem Soc Rev (2009) 38(6):1759–82. doi: 10.1039/b806051g
28. Whiteside T. Immune Responses to Cancer: Are They Potential Biomarkers of Prognosis? Front Oncol (2013) 3:107(107). doi: 10.3389/fonc.2013.00107
29. Yu WD, Sun G, Li J, Xu J, Wang X. Mechanisms and Therapeutic Potentials of Cancer Immunotherapy in Combination With Radiotherapy and/or Chemotherapy. Cancer Lett (2019) 452:66–70. doi: 10.1016/j.canlet.2019.02.048
30. Riley RS, June CH, Langer R, Mitchell MJ. Delivery Technologies for Cancer Immunotherapy. Nat Rev Drug Discov (2019) 18(3):175–96. doi: 10.1038/s41573-018-0006-z
31. Yang M, Li J, Gu P, Fan X. The Application of Nanoparticles in Cancer Immunotherapy: Targeting Tumor Microenvironment. Bioact Mater (2021) 6(7):1973–87. doi: 10.1016/j.bioactmat.2020.12.010
32. Li S, Yang Y, Lin X, Li Z, Ma G, Su Z, et al. Biocompatible Cationic Solid Lipid Nanoparticles as Adjuvants Effectively Improve Humoral and T Cell Immune Response of Foot and Mouth Disease Vaccines. Vaccine (2020) 38(11):2478–86. doi: 10.1016/j.vaccine.2020.02.004
33. Liu Y, Miyoshi H, Nakamura M. Nanomedicine for Drug Delivery and Imaging: A Promising Avenue for Cancer Therapy and Diagnosis Using Targeted Functional Nanoparticles. Int J Cancer (2007) 120(12):2527–37. doi: 10.1002/ijc.22709
34. Lanone S, Boczkowski J. Biomedical Applications and Potential Health Risks of Nanomaterials: Molecular Mechanisms. Curr Mol Med (2006) 6(6):651–63. doi: 10.2174/156652406778195026
35. Yao Y, Zhou Y, Liu L, Xu Y, Chen Q, Wang Y, et al. Nanoparticle-Based Drug Delivery in Cancer Therapy and its Role in Overcoming Drug Resistance. Front Mol Biosci (2020) 7:193. doi: 10.3389/fmolb.2020.00193
36. Tang L, Mei Y, Shen Y, He S, Xiao Q, Yin Y, et al. Nanoparticle-Mediated Targeted Drug Delivery to Remodel Tumor Microenvironment for Cancer Therapy. Int J Nanomedicine (2021) 16:5811–29. doi: 10.2147/ijn.S321416
37. Zylberberg C, Matosevic S. Pharmaceutical Liposomal Drug Delivery: A Review of New Delivery Systems and a Look at the Regulatory Landscape. Drug Delivery (2016) 23(9):3319–29. doi: 10.1080/10717544.2016.1177136
38. Satsangi A, Roy SS, Satsangi RK, Tolcher AW, Vadlamudi RK, Goins B, et al. Synthesis of a Novel, Sequentially Active-Targeted Drug Delivery Nanoplatform for Breast Cancer Therapy. Biomaterials (2015) 59:88–101. doi: 10.1016/j.biomaterials.2015.03.039
39. Tang B, Peng Y, Yue Q, Pu Y, Li R, Zhao Y, et al. Design, Preparation and Evaluation of Different Branched Biotin Modified Liposomes for Targeting Breast Cancer. Eur J Med Chem (2020) 193:112204. doi: 10.1016/j.ejmech.2020.112204
40. Hua S, Wu S. The Use of Lipid-Based Nanocarriers for Targeted Pain Therapies. Front Pharmacol (2013) 4:143. doi: 10.3389/fphar.2013.00143
41. Lemière J, Carvalho K, Sykes C. Chapter 14 - Cell-Sized Liposomes That Mimic Cell Motility and the Cell Cortex. In: Ross J, Marshall WF, editors. Methods in Cell Biology, vol. 128. Cambridge, Massachusetts, United States: Academic Press (2015). p. 271–85.
42. Jain RA. The Manufacturing Techniques of Various Drug Loaded Biodegradable Poly(Lactide-Co-Glycolide) (Plga) Devices. Biomaterials (2000) 21(23):2475–90. doi: 10.1016/s0142-9612(00)00115-0
43. Bala I, Hariharan S, Kumar MN. Plga Nanoparticles in Drug Delivery: The State of the Art. Crit Rev Ther Drug Carrier Syst (2004) 21(5):387–422. doi: 10.1615/critrevtherdrugcarriersyst.v21.i5.20
44. Gavas S, Quazi S, Karpiński TM. Nanoparticles for Cancer Therapy: Current Progress and Challenges. Nanoscale Res Lett (2021) 16(1):173. doi: 10.1186/s11671-021-03628-6
45. György B, Szabó TG, Pásztói M, Pál Z, Misják P, Aradi B, et al. Membrane Vesicles, Current State-of-the-Art: Emerging Role of Extracellular Vesicles. Cell Mol Life Sci (2011) 68(16):2667–88. doi: 10.1007/s00018-011-0689-3
46. Raposo G, Stoorvogel W. Extracellular Vesicles: Exosomes, Microvesicles, and Friends. J Cell Biol (2013) 200(4):373–83. doi: 10.1083/jcb.201211138
47. Sailor MJ, Park JH. Hybrid Nanoparticles for Detection and Treatment of Cancer. Adv Mater (2012) 24(28):3779–802. doi: 10.1002/adma.201200653
48. Ashfaq UA, Riaz M, Yasmeen E, Yousaf MZ. Recent Advances in Nanoparticle-Based Targeted Drug-Delivery Systems Against Cancer and Role of Tumor Microenvironment. Crit Rev Ther Drug Carrier Syst (2017) 34(4):317–53. doi: 10.1615/CritRevTherDrugCarrierSyst.2017017845
49. Luo E, Song G, Li Y, Shi P, Hu J, Lin Y. The Toxicity and Pharmacokinetics of Carbon Nanotubes as an Effective Drug Carrier. Curr Drug Metab (2013) 14(8):879–90. doi: 10.2174/138920021131400110
50. Yang M, Yang T, Mao C. Enhancement of Photodynamic Cancer Therapy by Physical and Chemical Factors. Angew Chem Int Ed (2019) 58(40):14066–80. doi: 10.1002/anie.201814098
51. Heister E, Neves V, Tîlmaciu C, Lipert K, Beltrán VS, Coley HM, et al. Triple Functionalisation of Single-Walled Carbon Nanotubes With Doxorubicin, a Monoclonal Antibody, and a Fluorescent Marker for Targeted Cancer Therapy. Carbon (2009) 47(9):2152–60. doi: 10.1016/j.carbon.2009.03.057
52. Ambrosi A, Airò F, Merkoçi A. Enhanced Gold Nanoparticle Based Elisa for a Breast Cancer Biomarker. Anal Chem (2010) 82(3):1151–6. doi: 10.1021/ac902492c
53. Dreaden EC, Alkilany AM, Huang X, Murphy CJ, El-Sayed MA. The Golden Age: Gold Nanoparticles for Biomedicine. Chem Soc Rev (2012) 41(7):2740–79. doi: 10.1039/C1CS15237H
54. Kim C-k, Ghosh P, Rotello VM. Multimodal Drug Delivery Using Gold Nanoparticles. Nanoscale (2009) 1(1):61–7. doi: 10.1039/B9NR00112C
55. Johnston HJ, Hutchison G, Christensen FM, Peters S, Hankin S, Stone V. A Review of the in Vivo and in Vitro Toxicity of Silver and Gold Particulates: Particle Attributes and Biological Mechanisms Responsible for the Observed Toxicity. Crit Rev Toxicol (2010) 40(4):328–46. doi: 10.3109/10408440903453074
56. Nakamura M. Organosilica Nanoparticles and Medical Imaging. Enzymes (2018) 44:137–73. doi: 10.1016/bs.enz.2018.08.002
57. Bailly A-L, Correard F, Popov A, Tselikov G, Chaspoul F, Appay R, et al. In Vivo Evaluation of Safety, Biodistribution and Pharmacokinetics of Laser-Synthesized Gold Nanoparticles. Sci Rep (2019) 9(1):12890. doi: 10.1038/s41598-019-48748-3
58. Sani A, Cao C, Cui D. Toxicity of Gold Nanoparticles (Aunps): A Review. Biochem Biophys Rep (2021) 26:100991. doi: 10.1016/j.bbrep.2021.100991
59. Cobley CM, Chen J, Cho EC, Wang LV, Xia Y. Gold Nanostructures: A Class of Multifunctional Materials for Biomedical Applications. Chem Soc Rev (2011) 40(1):44–56. doi: 10.1039/B821763G
60. Xiao W, Ruan S, Yu W, Wang R, Hu C, Liu R, et al. Normalizing Tumor Vessels to Increase the Enzyme-Induced Retention and Targeting of Gold Nanoparticle for Breast Cancer Imaging and Treatment. Mol Pharm (2017) 14(10):3489–98. doi: 10.1021/acs.molpharmaceut.7b00475
61. Li J, Huang J, Yang X, Yang Y, Quan K, Xie N, et al. Gold Nanoparticle-Based 2'-O-Methyl Modified DNA Probes for Breast Cancerous Theranostics. Talanta (2018) 183:11–7. doi: 10.1016/j.talanta.2018.02.036
62. Lee D, Ko W-K, Hwang D-S, Heo DN, Lee SJ, Heo M, et al. Use of Baicalin-Conjugated Gold Nanoparticles for Apoptotic Induction of Breast Cancer Cells. Nanoscale Res Lett (2016) 11(1):381–. doi: 10.1186/s11671-016-1586-3
63. Barai AC, Paul K, Dey A, Manna S, Roy S, Bag BG, et al. Green Synthesis of Nerium Oleander-Conjugated Gold Nanoparticles and Study of its in Vitro Anticancer Activity on Mcf-7 Cell Lines and Catalytic Activity. Nano Converg (2018) 5(1):10–. doi: 10.1186/s40580-018-0142-5
64. Singh P, Pandit S, Mokkapati VRSS, Garg A, Ravikumar V, Mijakovic I. Gold Nanoparticles in Diagnostics and Therapeutics for Human Cancer. Int J Mol Sci (2018) 19(7):1979. doi: 10.3390/ijms19071979
65. Willems A, Gauger K, Henrichs C, Harbeck N. Antibody Therapy for Breast Cancer. Anticancer Res (2005) 25(3a):1483–9.
66. García-Aranda M, Redondo M. Immunotherapy: A Challenge of Breast Cancer Treatment. Cancers (Basel) (2019) 11(12). doi: 10.3390/cancers11121822
67. Mitri Z, Constantine T, O'Regan R. The Her2 Receptor in Breast Cancer: Pathophysiology, Clinical Use, and New Advances in Therapy. Chemother Res Pract (2012) 2012:743193. doi: 10.1155/2012/743193
68. Bange J, Zwick E, Ullrich A. Molecular Targets for Breast Cancer Therapy and Prevention. Nat Med (2001) 7(5):548–52. doi: 10.1038/87872
69. Clynes RA, Towers TL, Presta LG, Ravetch JV. Inhibitory Fc Receptors Modulate in Vivo Cytoxicity Against Tumor Targets. Nat Med (2000) 6(4):443–6. doi: 10.1038/74704
70. Hashimoto G, Wright PF, Karzon DT. Antibody-Dependent Cell-Mediated Cytotoxicity Against Influenza Virus-Infected Cells. J Infect Dis (1983) 148(5):785–94. doi: 10.1093/infdis/148.5.785
71. Colombo M, Fiandra L, Alessio G, Mazzucchelli S, Nebuloni M, De Palma C, et al. Tumour Homing and Therapeutic Effect of Colloidal Nanoparticles Depend on the Number of Attached Antibodies. Nat Commun (2016) 7(1):13818. doi: 10.1038/ncomms13818
72. Lopez-Campos F, Candini D, Carrasco E, Berenguer Francés MA. Nanoparticles Applied to Cancer Immunoregulation. Rep Pract Oncol Radiother (2019) 24(1):47–55. doi: 10.1016/j.rpor.2018.10.001
73. Cai H, Degliangeli F, Palitzsch B, Gerlitzki B, Kunz H, Schmitt E, et al. Glycopeptide-Functionalized Gold Nanoparticles for Antibody Induction Against the Tumor Associated Mucin-1 Glycoprotein. Biorg Med Chem (2016) 24(5):1132–5. doi: 10.1016/j.bmc.2016.01.044
74. Qiu H, Min Y, Rodgers Z, Zhang L, Wang AZ. Nanomedicine Approaches to Improve Cancer Immunotherapy. Wiley Interdiscip Rev Nanomed Nanobiotechnol (2017) 9(5):e1456. doi: 10.1002/wnan.1456
75. Lv X, Chen D, Yang L, Zhu N, Li J, Zhao J, et al. Comparative Studies on the Immunoregulatory Effects of Three Polysaccharides Using High Content Imaging System. Int J Biol Macromol (2016) 86:28–42. doi: 10.1016/j.ijbiomac.2016.01.048
76. Zhu N, Lv X, Wang Y, Li J, Liu Y, Lu W, et al. Comparison of Immunoregulatory Effects of Polysaccharides From Three Natural Herbs and Cellular Uptake in Dendritic Cells. Int J Biol Macromol (2016) 93(Pt A):940–51. doi: 10.1016/j.ijbiomac.2016.09.064
77. Zhang S, Pang G, Chen C, Qin J, Yu H, Liu Y, et al. Effective Cancer Immunotherapy by Ganoderma Lucidum Polysaccharide-Gold Nanocomposites Through Dendritic Cell Activation and Memory T Cell Response. Carbohydr Polym (2019) 205:192–202. doi: 10.1016/j.carbpol.2018.10.028
78. Sztandera K, Gorzkiewicz M, Klajnert-Maculewicz B. Gold Nanoparticles in Cancer Treatment. Mol Pharm (2019) 16(1):1–23. doi: 10.1021/acs.molpharmaceut.8b00810
79. Atun R, Jaffray DA, Barton MB, Bray F, Baumann M, Vikram B, et al. Expanding Global Access to Radiotherapy. Lancet Oncol (2015) 16(10):1153–86. doi: 10.1016/S1470-2045(15)00222-3
80. Delaney G, Jacob S, Featherstone C, Barton M. The Role of Radiotherapy in Cancer Treatment. Cancer (2005) 104(6):1129–37. doi: 10.1002/cncr.21324
81. Stupp R, Mason WP, van den Bent MJ, Weller M, Fisher B, Taphoorn MJB, et al. Radiotherapy Plus Concomitant and Adjuvant Temozolomide for Glioblastoma. N Engl J Med (2005) 352(10):987–96. doi: 10.1056/NEJMoa043330
82. Janic B, Brown SL, Neff R, Liu F, Mao G, Chen Y, et al. Therapeutic Enhancement of Radiation and Immunomodulation by Gold Nanoparticles in Triple Negative Breast Cancer. Cancer Biol Ther (2021) 22(2):124–35. doi: 10.1080/15384047.2020.1861923
83. Bentzen SM. Preventing or Reducing Late Side Effects of Radiation Therapy: Radiobiology Meets Molecular Pathology. Nat Rev Cancer (2006) 6(9):702–13. doi: 10.1038/nrc1950
84. Wolff D, Stieler F, Welzel G, Lorenz F, Abo-Madyan Y, Mai S, et al. Volumetric Modulated Arc Therapy (Vmat) vs. Serial Tomotherapy, Step-and-Shoot Imrt and 3d-Conformal Rt for Treatment of Prostate Cancer. Radiother Oncol (2009) 93(2):226–33. doi: 10.1016/j.radonc.2009.08.011
85. Linam J, Yang LX. Recent Developments in Radiosensitization. Anticancer Res (2015) 35(5):2479–85.
86. Jain S, Coulter JA, Hounsell AR, Butterworth KT, McMahon SJ, Hyland WB, et al. Cell-Specific Radiosensitization by Gold Nanoparticles at Megavoltage Radiation Energies. Int J Radiat Oncol Biol Phys (2011) 79(2):531–9. doi: 10.1016/j.ijrobp.2010.08.044
87. McMahon SJ, Hyland WB, Muir MF, Coulter JA, Jain S, Butterworth KT, et al. Nanodosimetric Effects of Gold Nanoparticles in Megavoltage Radiation Therapy. Radiother Oncol (2011) 100(3):412–6. doi: 10.1016/j.radonc.2011.08.026
88. Chattopadhyay N, Cai Z, Pignol JP, Keller B, Lechtman E, Bendayan R, et al. Design and Characterization of Her-2-Targeted Gold Nanoparticles for Enhanced X-Radiation Treatment of Locally Advanced Breast Cancer. Mol Pharm (2010) 7(6):2194–206. doi: 10.1021/mp100207t
89. Jeynes JCG, Merchant MJ, Spindler A, Wera AC, Kirkby KJ. Investigation of Gold Nanoparticle Radiosensitization Mechanisms Using a Free Radical Scavenger and Protons of Different Energies. Phys Med Biol (2014) 59(21):6431–43. doi: 10.1088/0031-9155/59/21/6431
90. Retif P, Pinel S, Toussaint M, Frochot C, Chouikrat R, Bastogne T, et al. Nanoparticles for Radiation Therapy Enhancement: The Key Parameters. Theranostics (2015) 5(9):1030–44. doi: 10.7150/thno.11642
91. Galluzzi L, Buqué A, Kepp O, Zitvogel L, Kroemer G. Immunogenic Cell Death in Cancer and Infectious Disease. Nat Rev Immunol (2017) 17(2):97–111. doi: 10.1038/nri.2016.107
92. Demaria S, Golden EB, Formenti SC. Role of Local Radiation Therapy in Cancer Immunotherapy. JAMA Oncol (2015) 1(9):1325–32. doi: 10.1001/jamaoncol.2015.2756
93. Reits EA, Hodge JW, Herberts CA, Groothuis TA, Chakraborty M, K.Wansley E, et al. Radiation Modulates the Peptide Repertoire, Enhances Mhc Class I Expression, and Induces Successful Antitumor Immunotherapy. J Exp Med (2006) 203(5):1259–71. doi: 10.1084/jem.20052494
94. Burnette BC, Liang H, Lee Y, Chlewicki L, Khodarev NN, Weichselbaum RR, et al. The Efficacy of Radiotherapy Relies Upon Induction of Type I Interferon–Dependent Innate and Adaptive Immunity. Cancer Res (2011) 71(7):2488–96. doi: 10.1158/0008-5472.Can-10-2820
95. Thike AA, Chen XY, Koh VCY, Md Nasir ND, Poh Sheng Yeong J, Bay BH, et al. Higher Densities of Tumour-Infiltrating Lymphocytes and Cd4(+) T Cells Predict Recurrence and Progression of Ductal Carcinoma in Situ of the Breast. Histopathology (2019) 76:852–64. doi: 10.1111/his.14055
96. Kepp O, Senovilla L, Vitale I, Vacchelli E, Adjemian S, Agostinis P, et al. Consensus Guidelines for the Detection of Immunogenic Cell Death. Oncoimmunology (2014) 3(9):e955691. doi: 10.4161/21624011.2014.955691
97. Galluzzi L, Vitale I, Warren S, Adjemian S, Agostinis P, Martinez AB, et al. Consensus Guidelines for the Definition, Detection and Interpretation of Immunogenic Cell Death. J Immunother Cancer (2020) 8(1):e000337. doi: 10.1136/jitc-2019-000337
98. Andersson U, Tracey KJ. Hmgb1 is a Therapeutic Target for Sterile Inflammation and Infection. Annu Rev Immunol (2011) 29(1):139–62. doi: 10.1146/annurev-immunol-030409-101323
99. Ma Y, Kepp O, Ghiringhelli F, Apetoh L, Aymeric L, Locher C, et al. Chemotherapy and Radiotherapy: Cryptic Anticancer Vaccines. Semin Immunol (2010) 22(3):113–24. doi: 10.1016/j.smim.2010.03.001
100. Chen XY, Thike AA, Md Nasir ND, Koh VCY, Bay BH, Tan PH. Higher Density of Stromal M2 Macrophages in Breast Ductal Carcinoma in Situ Predicts Recurrence. Virchows Arch (2020) 476(6):825–33. doi: 10.1007/s00428-019-02735-1
101. Sia J, Szmyd R, Hau E, Gee HE. Molecular Mechanisms of Radiation-Induced Cancer Cell Death: A Primer. Front Cell Dev Biol (2020) 8:41(41). doi: 10.3389/fcell.2020.00041
102. Voorwerk L, Slagter M, Horlings HM, Sikorska K, van de Vijver KK, de Maaker M, et al. Immune Induction Strategies in Metastatic Triple-Negative Breast Cancer to Enhance the Sensitivity to Pd-1 Blockade: The Tonic Trial. Nat Med (2019) 25(6):920–8. doi: 10.1038/s41591-019-0432-4
103. Dolmans DEJGJ, Fukumura D, Jain RK. Photodynamic Therapy for Cancer. Nat Rev Cancer (2003) 3(5):380–7. doi: 10.1038/nrc1071
104. Näkki S, Martinez JO, Evangelopoulos M, Xu W, Lehto V-P, Tasciotti E. Chlorin E6 Functionalized Theranostic Multistage Nanovectors Transported by Stem Cells for Effective Photodynamic Therapy. ACS Appl Mater Interfaces (2017) 9(28):23441–9. doi: 10.1021/acsami.7b05766
105. Castano AP, Mroz P, Hamblin MR. Photodynamic Therapy and Anti-Tumour Immunity. Nat Rev Cancer (2006) 6(7):535–45. doi: 10.1038/nrc1894
106. Castano AP, Mroz P, Wu MX, Hamblin MR. Photodynamic Therapy Plus Low-Dose Cyclophosphamide Generates Antitumor Immunity in a Mouse Model. Proc Natl Acad Sci USA (2008) 105(14):5495–500. doi: 10.1073/pnas.0709256105
107. Wilson WR, Hay MP. Targeting Hypoxia in Cancer Therapy. Nat Rev Cancer (2011) 11(6):393–410. doi: 10.1038/nrc3064
108. Rankin EB, Giaccia AJ. Hypoxic Control of Metastasis. Science (2016) 352(6282):175–80. doi: 10.1126/science.aaf4405
109. Tian H, Luo Z, Liu L, Zheng M, Chen Z, Ma A, et al. Cancer Cell Membrane-Biomimetic Oxygen Nanocarrier for Breaking Hypoxia-Induced Chemoresistance. Adv Funct Mater (2017) 27(38):1703197. doi: 10.1002/adfm.201703197
110. Hatfield SM, Kjaergaard J, Lukashev D, Schreiber TH, Belikoff B, Abbott R, et al. Immunological Mechanisms of the Antitumor Effects of Supplemental Oxygenation. Sci Transl Med (2015) 7(277):277ra30–ra30. doi: 10.1126/scitranslmed.aaa1260
111. Chouaib S, Noman MZ, Kosmatopoulos K, Curran MA. Hypoxic Stress: Obstacles and Opportunities for Innovative Immunotherapy of Cancer. Oncogene (2017) 36(4):439–45. doi: 10.1038/onc.2016.225
112. Liang R, Liu L, He H, Chen Z, Han Z, Luo Z, et al. Oxygen-Boosted Immunogenic Photodynamic Therapy With Gold Nanocages@Manganese Dioxide to Inhibit Tumor Growth and Metastases. Biomaterials (2018) 177:149–60. doi: 10.1016/j.biomaterials.2018.05.051
113. Pang B, Yang X, Xia Y. Putting Gold Nanocages to Work for Optical Imaging, Controlled Release and Cancer Theranostics. Nanomedicine (Lond) (2016) 11(13):1715–28. doi: 10.2217/nnm-2016-0109
114. Gao L, Liu R, Gao F, Wang Y, Jiang X, Gao X. Plasmon-Mediated Generation of Reactive Oxygen Species From Near-Infrared Light Excited Gold Nanocages for Photodynamic Therapy in Vitro. ACS Nano (2014) 8(7):7260–71. doi: 10.1021/nn502325j
115. Hussein EA, Zagho MM, Rizeq BR, Younes NN, Pintus G, Mahmoud KA, et al. Plasmonic Mxene-Based Nanocomposites Exhibiting Photothermal Therapeutic Effects With Lower Acute Toxicity Than Pure Mxene. Int J Nanomedicine (2019) 14:4529–39. doi: 10.2147/ijn.S202208
116. Gupta N, Malviya R. Understanding and Advancement in Gold Nanoparticle Targeted Photothermal Therapy of Cancer. Biochim Biophys Acta (2021) 1875(2):188532. doi: 10.1016/j.bbcan.2021.188532
117. Zhou L, Liu H, Liu K, Wei S. Gold Compounds and the Anticancer Immune Response. Front Pharmacol (2021) 12:739481(2489). doi: 10.3389/fphar.2021.739481
118. Bear AS, Kennedy LC, Young JK, Perna SK, Mattos Almeida JP, Lin AY, et al. Elimination of Metastatic Melanoma Using Gold Nanoshell-Enabled Photothermal Therapy and Adoptive T Cell Transfer. PloS One (2013) 8(7):e69073. doi: 10.1371/journal.pone.0069073
119. Miragem AA, Homem de Bittencourt PI Jr.Nitric Oxide-Heat Shock Protein Axis in Menopausal Hot Flushes: Neglected Metabolic Issues of Chronic Inflammatory Diseases Associated With Deranged Heat Shock Response. Hum Reprod Update (2017) 23(5):600–28. doi: 10.1093/humupd/dmx020
Keywords: adaptive immunity, breast cancer, gold nanoparticle, immune response, immunogenic cell death, immunotherapy, innate immunity, tumor microenvironment
Citation: Chen X-Y, Yung L-YL, Tan PH and Bay BH (2022) Harnessing the Immunogenic Potential of Gold Nanoparticle-Based Platforms as a Therapeutic Strategy in Breast Cancer Immunotherapy: A Mini Review. Front. Immunol. 13:865554. doi: 10.3389/fimmu.2022.865554
Received: 30 January 2022; Accepted: 14 March 2022;
Published: 31 March 2022.
Edited by:
Kee Woei Ng, Nanyang Technological University, SingaporeReviewed by:
Yaqing Qie, Mayo Clinic Florida, United StatesCopyright © 2022 Chen, Yung, Tan and Bay. This is an open-access article distributed under the terms of the Creative Commons Attribution License (CC BY). The use, distribution or reproduction in other forums is permitted, provided the original author(s) and the copyright owner(s) are credited and that the original publication in this journal is cited, in accordance with accepted academic practice. No use, distribution or reproduction is permitted which does not comply with these terms.
*Correspondence: Puay Hoon Tan, dGFuLnB1YXkuaG9vbkBzaW5naGVhbHRoLmNvbS5zZw==; Boon Huat Bay, YW50YmF5YmhAbnVzLmVkdS5zZw==