- Department of Biological Sciences, Old Dominion University, Norfolk, VA, United States
The transforming growth factor-β (TGF-β) family includes cytokines controlling cell behavior, differentiation and homeostasis of various tissues including components of the immune system. Despite well recognized importance of TGF-β in controlling T cell functions, the immunomodulatory roles of many other members of the TGF-β cytokine family, especially bone morphogenetic proteins (BMPs), start to emerge. Bone Morphogenic Protein Receptor 1α (BMPR1α) is upregulated by activated effector and Foxp3+ regulatory CD4+ T cells (Treg cells) and modulates functions of both of these cell types. BMPR1α inhibits generation of proinflammatory Th17 cells and sustains peripheral Treg cells. This finding underscores the importance of the BMPs in controlling Treg cell plasticity and transition between Treg and Th cells. BMPR1α deficiency in in vitro induced and peripheral Treg cells led to upregulation of Kdm6b (Jmjd3) demethylase, an antagonist of polycomb repressive complex 2 (PRC2), and cell cycle inhibitor Cdkn1a (p21Cip1) promoting cell senescence. This indicates that BMPs and BMPR1α may represent regulatory modules shaping epigenetic landscape and controlling proinflammatory reprogramming of Th and Treg cells. Revealing functions of other BMP receptors and their crosstalk with receptors for TGF-β will contribute to our understanding of peripheral immunoregulation.
Introduction
The major polarized Th subsets, Th1, Th2, Th9 and Th17 cells, are generated in response of CD4+ T cells to antigenic stimulation, co-stimulatory signals and cytokines and utilize specialized effector mechanisms to eliminate different types of pathogens (1–4). TGF-β has emerged as the cytokine controlling intrinsic activation of T cells and their antigenic responses (5, 6). In the presence of IL-4 or inflammatory cytokines, especially IL-6, TGF-β supports generation of Th9 or Th17 cells respectively (7, 8). Th cell functions are controlled by regulatory CD4+ T cells (Treg), which express the transcription factor Foxp3 (9, 10). Treg cells maintain immunological self-tolerance and homeostasis but also control clinical conditions including immunometabolic and degenerative diseases, and tissue regeneration (10–13). Population of thymus derived Treg cells is complemented by peripheral Treg cells generated from conventional CD4+ Th cells which upregulate Foxp3 in response to stimulation with antigen and TGF-β (14, 15). Peripheral Treg cells exhibit considerable heterogeneity and utilize specialized mechanisms to constrain inflammatory reactions in response to self and exogenous antigens (16–19). Foxp3 is essential for Treg cell function, especially for its suppressive activity (9). However, Treg cell lineage commitment in the thymus seems to be initiated before Foxp3 expression and Foxp3 expression does not confer all features of Treg phenotype like expression of CTLA-4, lack of IL-2 expression (20–23). Recent reports indicate that Treg-specific epigenetic changes including DNA demethylation and histone modifications establish a pattern of Treg gene expression and stability of Treg cell phenotype (24–27). Treg-specific defects often correlate with the development of several autoimmune disorders such as type 1 diabetes, multiple sclerosis, psoriasis, rheumatoid arthritis and Crohn’s disease (10, 28–31). This includes reduced induction and homing of peripheral Treg cells, alleviated or altered suppressor mechanisms and decreased stability of Treg phenotype. Deficiency of Treg cells caused by mutations of Foxp3 results in early onset autoimmune disease as demonstrated in Foxp3 mutant scurfy mice and humans with IPEX (immune dysregulation, polyendocrinopathy, enteropathy, X-linked) syndrome (32, 33). Deletion of multiple other genes affecting Foxp3 protein stability or altered epigenetic status of Foxp3 gene locus resulted in compromised function of Treg cells and were associated with autoimmune pathology (34, 35). Nevertheless, compromised function of Treg cells is not always associated with their reduced frequency (36, 37). For example, signaling through the IL-27R or TGF-βR, impacted Treg cell function but was not accompanied by major phenotypic or quantitative changes of Treg population resulting in systemic autoimmunity (38, 39).
Uncovering what mechanisms control Treg cell homeostasis become even more important when it was discovered that Treg cells which lost Foxp3 expression (exTreg cells) may produce inflammatory cytokines, IFN-γ and IL-17 (40, 41). While downregulation of Foxp3 may be required to alleviate suppressive effect of Treg cells, allowing for effective immune responses to pathogens, in other cases Treg cell instability exacerbated tissue damage and contributed to immune pathology (42, 43). Treg instability also contributes to the augmentation of anti-tumor immunity (44, 45). exTreg cells promoted destruction of pancreatic islets and accelerated onset of diabetes (41). In rheumatoid arthritis and EAE, pathogenic Th17 cells were shown to arise from Treg cells (46, 47). In contrast, resolution of inflammation may depend on the opposite process of trans differentiation of Th17 cells into Treg cells (47, 48). Thus, regulation of the Th cell lineage plasticity is critical for understanding of immune regulation and pathogenesis of autoimmune diseases (49, 50).
Generation and Maintenance of Treg Population
Multiple reports identified membrane and soluble molecules which proved essential to control abundance and fitness of Treg cell population in peripheral organs and promote their suppressor function. This includes signaling through the TCR, costimulatory molecules (CD28 and CTLA-4) and cytokines receptors (18, 43, 51–56). IL-2 and TGF-β were the most studied cytokines in the context of Treg cell biology. IL-2 is a key cytokine required for induction of Foxp3 in thymic Treg precursors and in peripheral CD4+ T cells (14, 57–59). Mechanistically, Stat5 in response to IL-2 signaling binds enhancer in the Foxp3 gene inducing its expression in the thymus (60). In peripheral Treg cells IL-2 induced transcriptional program controls metabolic fitness of Treg cells, sustains their survival and suppressor function and prevents autoimmunity (61, 62). Foxp3 CNS2 (conserved noncoding sequence) enhancer element acts as an IL-2 sensor by binding Stat5 and conferring stable inheritance of Foxp3 expression (63). IL-2 induced genetic program of Treg cell differentiation and peripheral maintenance depend on activation of Smad3 and the presence of TGF-β (59, 64–66). While both IL-2 and TGF-β promote generation and sustain Treg cells, IL-2 inhibits and TGF-β enhances generation of effector Th17 cells underscoring the importance of context dependent signaling for Th lineage ontogeny (67, 68).
Immunoregulatory role of TGF-β has been known before the discovery of Treg cells (69). TGF-β provides vital signals that limit immune activation so deletion of the TGF-β1 gene in experimental mice, which abrogated TGF-β signaling in multiple T cell subsets, induced severe autoimmune inflammatory disease (5, 70). T cell specific inhibition of TGF-βRII signaling had similar outcome and precipitated systemic autoimmune disease characterized by massive activation and expansion of T cells (71, 72). Co-transfer of naive CD4+ T cells expressing dominant negative TGF-βRII, and Treg cells, into recipient mice demonstrated that effector cells need to respond to TGF-β for the Treg cells to control their activation (73). T cell specific deletion of TGF-βRII revealed that TGF-β signaling is not required for thymic development of Treg cells but supports Foxp3 expression, suppressor function and sustains peripheral population of Treg cells (74, 75). In summary, earlier reports supported conclusions that while Treg thymic development is not affected, both T cell autonomous and Treg dependent tolerance mechanisms are abrogated by elimination of TGF-β signaling in effector Th and in Treg cells (75–77). The caveat of these experiments is that they relied on inhibition of TGF-β signaling in multiple T cell subsets and examined Treg cells in the context of induced severe autoimmune inflammatory disease, complicating interpretation of the role of TGF-β in Treg cells (6). In contrast, analysis of newborn mice with T cell specific TGF-βRI gene deletion and, inhibition of TGF-β signaling in thymic organ cultures identified TGF-β, in connection with IL-2, as cytokines essential for inducing Foxp3 expression and thymocyte commitment to Treg cell differentiation in the thymus (78). However, another report defined TGF-β role in Treg development to be limited to enhancing survival and protection from negative selection of thymocytes committed to become Treg cells (79). This report of limited impact of TGF-β in inducing Treg cell generation was questioned by demonstrating that intrathymic transfer of early thymocytes, where TGF-βRI gene deletion is induced at the double positive stage, failed to produce any Treg cells, corroborating reports that TGF-β signaling is indispensable for Treg lineage commitment (80). In contrast, deletion of the TGF-βRI gene in Treg cells following Foxp3 expression, by Foxp3 controlled cre expression, did not decrease thymic generation of Treg cells, in agreement with reports that TGF-β signaling is dispensable for Treg lineage commitment (80). Moreover, abrogation of TGF-β signaling in already differentiated Treg cells did not decrease proportion of peripheral Treg cells, Foxp3 expression was preserved and no systemic autoimmunity was observed (39). Only aged mice suffered from local skin and gastrointestinal inflammation due to selective defect of TGF-βRI deficient Treg cells to migrate, accumulate and control Th17 cell mediated responses. In contrast to Th17 cells, control of Th1 effector cells by TGF-βRI deficient Treg cells was enhanced. This result suggested that TGF-β does not control overall fitness of Treg cells but rather modulates their suppressor function to selectively impact different Th subsets in specific organs. Another report demonstrated that Treg cell mediated production of TGF-β is necessary to prevent food allergy underscoring the importance of Treg derived TGF-β in allergic responses and maintenance of immune tolerance (81).
Bone Morphogenetic Proteins, Their Receptors and Signaling Pathways
Bone Morphogenetic Proteins (BMPs) are the largest subfamily of the TGF-β cytokine superfamily which also includes TGF-β, a founding member of the family, activins, nodal and growth and differentiation factors. BMPs were identified by their ability to induce bone differentiation (82). It is now well known that in addition of inducing differentiation of osteoblasts, bone-forming cells, BMPs control multiple cellular processes including differentiation of various cell types, adhesion, migration and proliferation and apoptosis (83, 84). They have prominent role in regulating body axes formation during embryonal development, regulate epithelial - mesenchymal transition in cancer and wound healing (83, 85–87). BMPs sustain stem cell renewal and differentiation, including tissue specific and cancer stem cells (88–90). Individual BMPs often have overlapping functions, but they can be highly specific when function as morphogens or cytokines sustaining tissue homeostasis. BMPs are highly pleiotropic cytokines which act in autocrine, paracrine and endocrine fashion determined by tissue environment and intrinsic properties of target cells (91).
Tight regulation of BMP signaling is crucial to maintain homeostasis of tissues and organs, and is achieved by controlling BMP gene expression, secretion and maturation of BMP precursors. Proteases involved in producing active, mature BMPs include furin, which is induced in activated T cells and essential for Treg cell suppressor function (92). Mature BMPs are bound and sequestered by soluble (e.g. chordin, noggin, gremlin) or membrane/matrix proteins (e.g. fibrin, small leucine-rich proteins) or pseudoreceptors like BAMBI (BMP and Activin Membrane-Bound Inhibitor) (93, 94). This complex system regulates BMPs bioavailability by controlling their secretion, proteolytic maturation of BMP precursors, degradation and sequestration.
TGF-β family cytokines, including BMPs, signal through heteromeric complexes of type I and type II receptors, which have activity of serine/threonine kinases (Figure 1). Cytokine ligand binds to a type II receptor and the ligand-receptor complex binds to a type I receptor. Formation of a ternary complex activates receptor kinase activity and induces phosphorylation of transcription factors, Smads, which activates canonical signal transduction pathway (84). TGF-β itself binds TGF-βRII and TGF-βRI (Alk-5) and induces phosphorylation of Smad2/3. BMPs bind one of type II receptors, BMPR2, activin receptor type 2A (ACVR2A) or activin receptor type 2B (ACVR2B). Ligand binding to type II receptor induces recruitment of one of type I receptors, activin receptor-like kinase 1 (Alk-1, ACVRL1), activin A receptor type 1 (Alk-2, ACVR1), activin receptor type 1B (Alk-4, ACVR1B), BMPR1α (Alk-3) or BMPR1β (Alk-6, not expressed by CD4 cells) and leads to conformational change of the heteromeric receptor to induce kinase activity of type I receptor and phosphorylation of Smad1/5/8. Promiscuity of ligand receptor interactions contributes to redundant functions of BMPs but also underlies signaling crosstalk between TGF-β and BMPs. TGF-β bound to TGF-βRII may recruit and transphosphorylate ACVRL1 or BMPR1α with subsequent phosphorylation and activation of Smad1/5/8 (95). Type II receptors ACVR2A or ACVR2B may also bind TGF-βRI with resulting phosphorylation of Smad2/3. Thus, combinatorial activation of both Smad pathways could be essential for signaling crosstalk of TGF-β and BMPs (96). Smad transcription factors phosphorylated by TGF-β or by BMP receptors (R-Smads) form trimeric complexes with Smad4 and translocate into nucleus. They interact with multiple co-activators, including genes controlling Treg phenotype, and bind specific motifs present in regulatory regions of Smad inducible genes, including Foxp3 (84, 97, 98). Besides activating Smads, BMPs signal through multiple Smad-independent (non-canonical) pathways (99). This involves activation of Tak-1 (TGF-β activated kinase 1) and downstream activation of NF-κB (100–104). Smad independent signaling also includes activation of PI3K-Akt pathway (105). Finally, BMPs activate p38/JNK kinases which engages TRAF4 or TRAF6 and activates Tak1 (106–108).
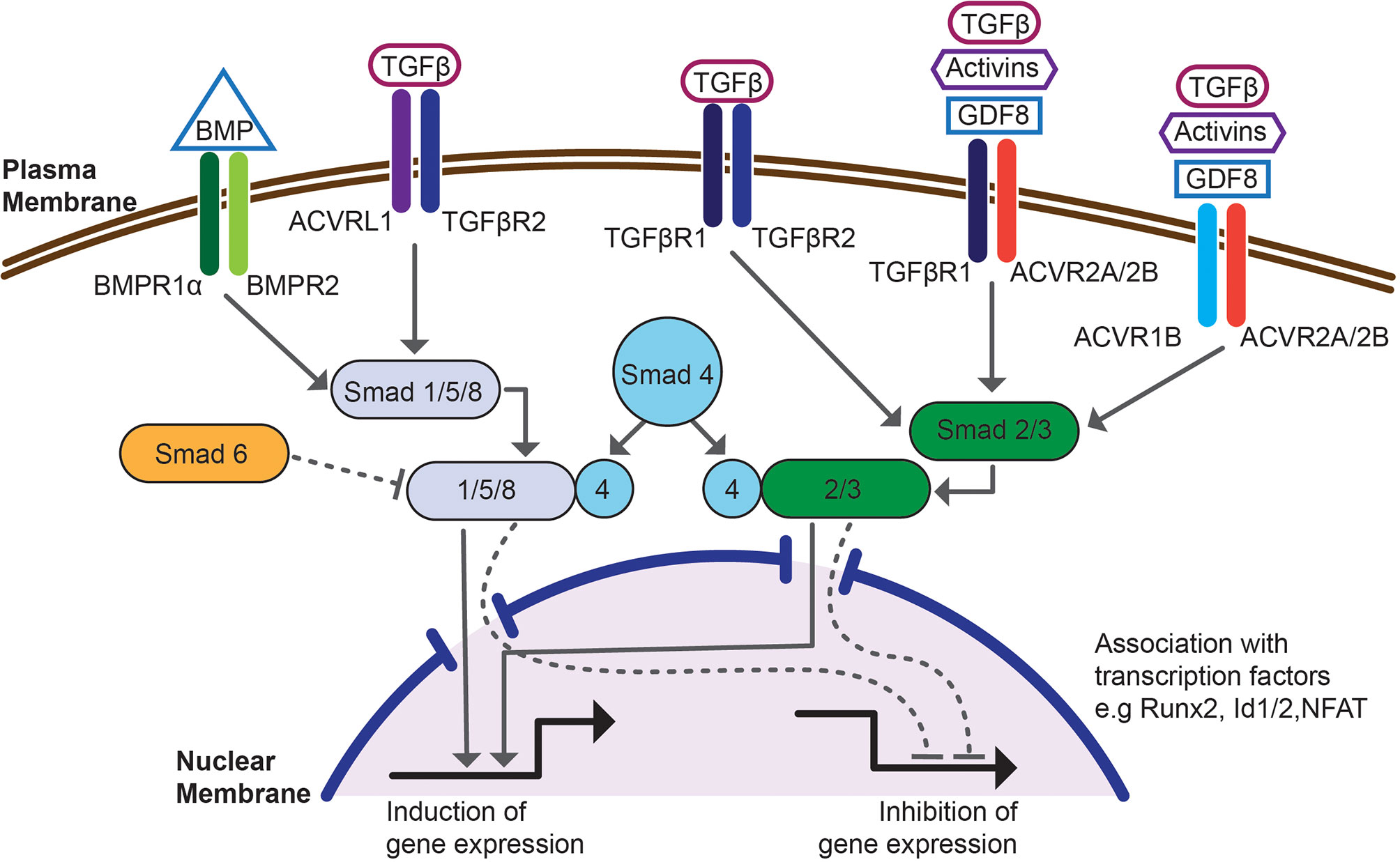
Figure 1 Schematic overview of the canonical, SMAD-dependent BMP and TGF-β signaling pathway. Signaling is initiated by binding to a heteromeric complex of type I receptors, e.g. BMPR1α, TGF-βR1, ACVRL1 or ACVR1, associated with type II receptors, e.g. BMPR2, TGF-βR2, ACVR2A/2B. Intracellular, BMP or TGF-β responsive transcription factors, Smads become phosphorylated and associate with co-Smad4 and translocate into nucleus. This signaling pathway is controlled by inhibitory Smad6. Once in the nucleus Smad complexes associate with transcription factors e.g. Runx2, Id1/2 or NFAT, bind regulatory regions of Smad dependent genes and regulate transcription. The figure shows interdependence of BMP and TGF-β signaling at the level of receptor binding and Smad phosphorylation.
Bone Morphogenetic Proteins Control of Treg Lineage
While TGF-β mediated regulation of Th lineage differentiation and immune system homeostasis have been extensively studied, the role of other members of the TGF-β family, including BMPs is only starting to emerge (109). Recent reports demonstrate that BMPs, similar to TGF-β, are immunomodulatory cytokines which control differentiation and functions of immune cells impacting immune tolerance, inflammation and linage specification of effector Th cells (110). BMPs regulate thymic development of T cells, but published results remain controversial (111–115). Both thymocytes and thymic stromal cells produce BMPs and express BMP receptors. Fetal thymic cultures and signaling inhibitor studies showed that BMPs are required for early thymocyte progenitor homeostasis but block transition from double negative to double positive thymocytes (112, 116). In contrast, analysis of conditional knockout mice where BMPR1α gene was deleted in hematopoietic cells (by crossing to vav-cre mice) did not reveal changes in thymus cellularity and subset proportions (114). Analysis of mice where BMPR1α gene was deleted in double positive thymocytes showed normal development of T cells with the exception of a population of Foxp3+ Treg cells which was severely decreased suggesting a unique role of this receptor in Treg specification (117). However, thymic but not peripheral Treg population was normal when BMPR1α gene was deleted at the later stage, in thymocytes expressing Foxp3 (118).
BMPR1α is expressed in mature CD4+ T cells in lymph nodes, spleen and peripheral organs (118). It is expressed at low level in naive CD4+ T cells and at higher levels in activated Th and Treg cells. It is upregulated following T cell activation within hours. Since expression of BMPR2 is not affected by T cells activation, it is upregulation of BMPR1α which renders activated CD4+ T cell sensitive to BMPs (119). In vitro studies using signaling inhibitors have shown that BMPs regulate proliferation and activation of CD4+ T cells but the role of BMPs in controlling peripheral Treg cells was not addressed (120, 121). Blockade of BMP signaling in rheumatoid arthritis patients augmented inflammation induced by IL-17 and BMPs ameliorated intestinal inflammation suggesting that cellular targets of BMP signaling may include effector Th17 and Treg cells (122–125). BMP2/4 or activin A synergized with the TGF-β to generate inducible Treg (iTreg) cells but were not able to completely replace TGF-β and induce Foxp3 expression (126, 127). Foxp3 enhancer, CNS1, contains canonical Smad1/5/8 binding site that partially overlaps Smad2/3 site. T cells activated in the presence of BMPs differentiated into Th1 or Th2 but Th17 differentiation was inhibited. BMP signaling resulted in inhibition of Rorc and IL-17 upregulation (119). These results were complemented by analysis of CD4+ T cells deficient in BMPR1α (117, 119). Generation of Th17 cells in vitro, induced by IL-6 and TGF-β, is greatly enhanced by abrogation of the BMPR1α signaling but it still requires presence of TGF-β. At the same time, in vitro generation of iTreg cells is impaired, not improved, by BMPR1α deficiency, suggesting complex interaction between BMPR1α and TGF-β signaling pathways (117). Deletion of BMPR1α gene does not affect phosphorylation of Smad2/3 in CD4+ T cells activated for 1 hour in the presence of TGF-β, however genes mediating responses to TGF-β signaling, including Smad3, Tsc22D1, Skil, were differentially expressed when analyzed after 4 days (128, 129). Transcriptome analyses using RNA-seq revealed that of 72 transcription factors identified as differentially expressed between wild type and BMPR1α deficient iTreg cells, 39 included genes identified in previous reports to support Th17 cell differentiation and 17 to support iTreg cell generation (130–135). Transcription factors Rorc, Rxra, Batf, Maf, Ikzf2 and Ikzf4 were overexpressed in BMPR1α deficient Treg cells, while Hopx and Foxp3 had lower expression compared to wild type Treg cells (118). BMPR1α deficient iTreg cells also had lower expression of Crem, Pde3b and Gpr83, genes associated with Treg phenotype (21, 118, 136, 137). Thus, BMPR1α signaling in naive cells affects developmental programme controlling lineage choice of iTreg and Th17 cells and, likely, balance between these two cell subsets.
Altered Ontogenesis and Phenotypic Stability of BMPR1α Deficient Treg Cells
Abrogation of BMPR1α signaling in mature Treg cells resulted in increased proportion of Treg cells expressing low levels of Foxp3, as mice aged, and significantly altered proportions of Treg cells expressing naive (CD44lowCD62L+) and mature (CD44+CD62Llow) phenotype. Treg cells still expressing high levels of Foxp3, and naive phenotype, were replaced by cells with low expression of Foxp3, and mature phenotype, and these cells dominated peripheral Treg population in aged mice. Acquisition of mature phenotype is associated with Treg activation, or is evidence of cellular senescence indicating disruption of peripheral homeostasis (138, 139). Analysis of cell surface markers showed that BMPR1α-deficient Treg cells expressed lower levels of CD39 and Klrg1, indicating that their suppressor function and terminal maturation are impaired (140, 141). Phenotypic changes of the Treg population were accompanied by gradual upregulation of CD44, and downregulation of CD62L, on conventional CD4+ T cells in aging mice. Progressive loss of Foxp3 expression, associated with senescent phenotype, and increased presence of activated, conventional T cells, are consistent with compromised Treg cell suppressor function and unstable Treg phenotype (118).
When wild type or BMPR1α-deficient Treg cells, expressing high levels of Foxp3, were co-transferred to lymphopenic mice, with naive conventional CD4+ T cells, only wild type Treg cells retained Foxp3 expression, and were able to protect recipient mice from inflammatory bowel disease. BMPR1α-deficient Treg cells had high expression of CCR6 and IL-23R, receptors regulating homing and promoting differentiation of Th17 cells or their precursors. This was associated with increased levels of Rorc, IFN-γ and IL-17 in donor BMPR1α-deficient cells (41).
Immunization of mice with BMPR1α-deficient Treg cells led to robust activation of conventional CD4+ T cells, which expressed higher levels of activation markers, and inflammatory cytokines IFN-γ and IL-17. BMPR1α deficient Treg cells in immunized mice had lower expression of Foxp3, CD39, 4-1BB, and Klrg1. CD39 is an ectonuclease directly involved in Treg suppressor function, and 4-1BB binding of galectin-9 augments Treg function (140, 142–144). Klrg1 is upregulated on antigen activated, highly suppressive Treg cells (141). Similarly, exacerbated inflammatory response was observed in mice infected with Citrobacter rodentium, a mouse model of bacterial colitis (145). These findings indicate unstable phenotype, and decreased ability of BMPR1α-deficient Treg cells, to control inflammation and point to the importance of BMPs signaling to control immune homeostasis in situ and in inflammation.
Signaling Circuits Controlled by BMPR1α Signaling
Transcriptome analyses of Treg and iTreg cells revealed that BMPR1α gene deletion results in elevated levels of genes promoting phenotypic plasticity and functional adaptation of Treg lineage cells including Rorc, IRF4, Hif1α, Batf3 (Figure 2) (118, 146, 147). This finding is consistent with observed downregulation of Foxp3 and enhanced production of Th1/Th17 cells in inflammatory conditions by BMPR1α-deficient Treg cells (46, 148–150). In addition, a set of genes differentially expressed between BMPR1α-sufficient and deficient Treg and iTreg cells included Cdkn1a (p21Cip1) and Kdm6b (Jmjd3). Higher levels of these genes in BMPR1α-deficient cells provided cues how BMP signaling shapes Treg population (Figure 2).
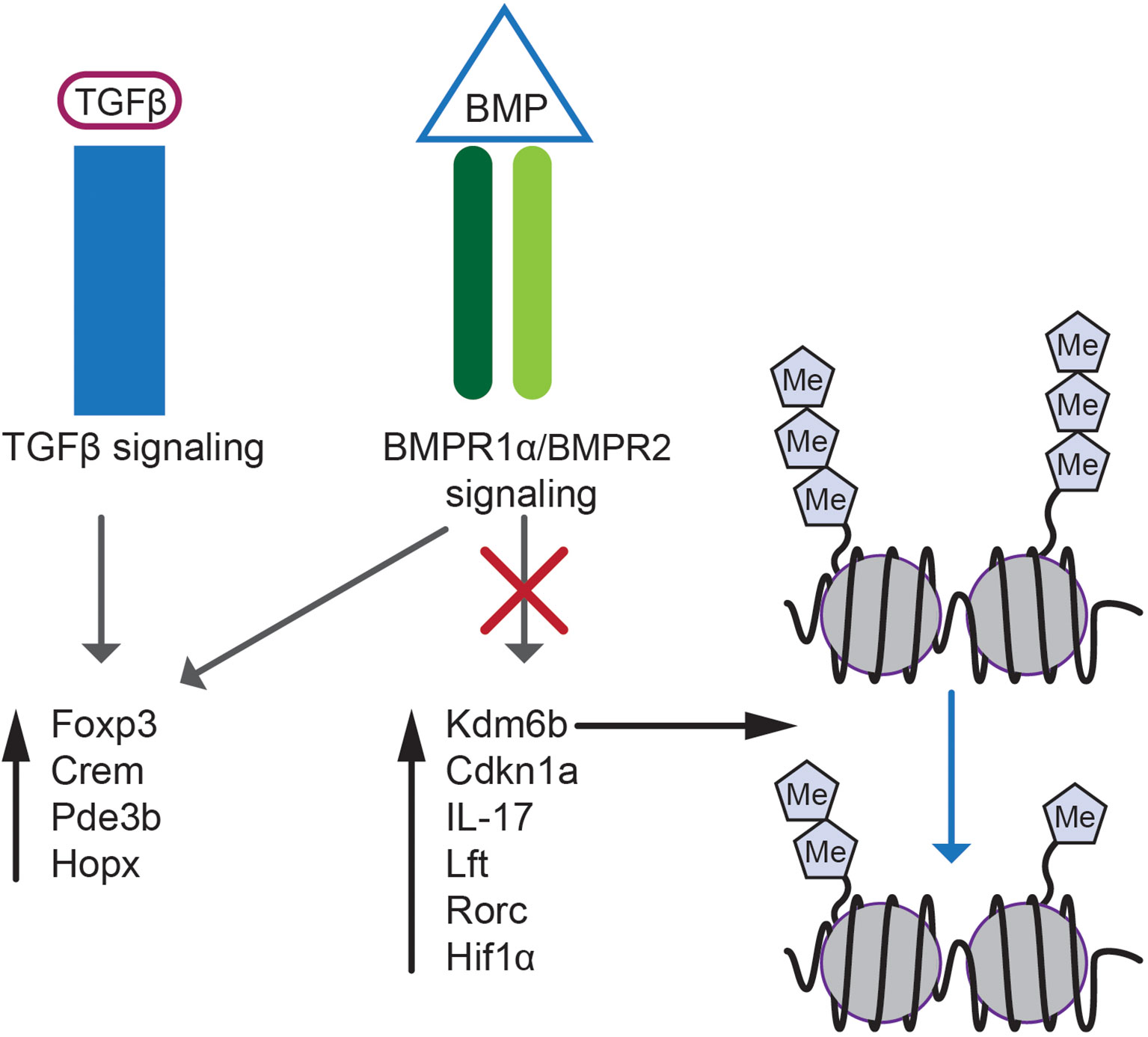
Figure 2 BMP and TGF-β signaling in Treg cell biology. BMP and TGF-β signaling regulates expression of genes essential for Treg lineage specification e.g. Foxp3, Crem, Pde3b and Hopx. Selective abrogation of BMPR1α signaling results in altered gene expression and upregulation of Kdm6b, Cdkn1a, IL-17, Lft, Rorc and Hif1α. Expression of proinflammatory genes is regulated by demethylation of inhibitory H3K27m3 epigenetic marks by Kdm6b demethylase.
Cdkn1a is a cell cycle inhibitor associated with cell maturation and senescence (151). Higher expression of Cdkn1a in peripheral BMPR1α-deficient Treg cells correlates with decreased proliferation and renewal of this subset while promoting maturation and senescence. Cdkn1a also controls CD4+ T cell responses to antigen and generation of memory or anergic cells (152). Kdm6b demethylase is an antagonist of polycomb repressive complex 2 (PRC2) which sustains repressive trimethylation of H3K27. Differentiation of wild type, naive CD4+ T cells into iTreg cells is associated with downregulation of Kdm6b. In contrast, Kdm6b expression remains elevated when BMPR1α-deficient CD4+ T cells when they differentiate into iTreg cells. Kdm6b is also elevated in Treg cells directly isolated from mutant experimental mice (118). In CD4+ T cells Kdm6b promoted proinflammatory immune responses and enhanced cellular senescence (153). Upregulation of Cdkn1a and Cdkn2a (p16Ink4), controlled by Kdm6b, regulated cell cycle and inhibited reprogramming into self-renewing pluripotent stem cells supported by BMP signaling (88, 154, 155). Consistent with these reports, Cdkn1a expression in T cells was found to depend on epigenetic status of DNA and was upregulated by histone deacetylase inhibitors (156).
Mechanistic control of Treg cells by Kdm6b and BMPR1α signaling is consistent with reports demonstrating that inhibition of Ezh2, a H3K27 methyltransferase of the PRC2, compromised Treg cell function in tumors and autoimmune diseases (157, 158). Ezh2 is induced in Treg cells upon activation, and sustains Treg cell stability and function in inflammation (159–161). Deletion of Ezh2 gene in Treg cells increased production of exTreg cells, infiltration of CD8+ and effector CD4+/Treg ratio in tumors, production of TNF-α and IFN-γ (157). Altogether, BMPR1α signaling in Treg cells modulates expression of Kdm6b, an antagonist of Ezh2, and epigenetic landscape controlling Treg cell plasticity.
Discussion
Dysfunction of Treg cells, resulting in altered balance between effector and Treg cells, is considered a main underlying cause of most autoimmune diseases (162). Acquisition of effector Th cell functions, rather than decreased proportions of Treg cells, are the main cause of autoimmune pathologies but little is understood how this process is controlled (163). Heterogeneity of the Treg cell population may account for effector like properties of Treg cells, while Foxp3 expression is retained (43, 164). In addition, genetic cell fate mapping, suggested that phenotypic plasticity of the Treg cell lineage, especially in inflammatory environment, results in the presence of different proportions of effector CD4+ T cells that downregulate Foxp3 expression (41, 165). Finally, the functions of Treg cells are shaped by tissue specific environmental factors, leading to the development of specialized subsets of Treg cells controlling tissue homeostasis and regeneration (11–13, 166).
Foxp3 expression and development of a specific epigenetic signature are required to sustain Treg functions (167, 168). Abrogation of BMPR1α signaling in Treg cells led to a gradual loss of Foxp3 expression, and was associated with upregulation of transcription factors specific for effector Th lineages, Th1 and Th17 cells. Molecular changes were accompanied by decreased suppressor functions in situ and enhanced responses to immunization or bacterial infections. These findings are consistent with reports demonstrating that inhibition of the BMP signaling exacerbated rheumatoid arthritis, and BMPs treatment ameliorated renal inflammation (122, 125). Altered transcriptional landscape in BMPR1α-deficient Treg cells was associated with epigenetic changes, mediated by overexpression of the Kdm6b demethylase (118, 153). Overexpression of Kdm6b impaired generation of iTreg cells, and promoted inflammation by enhanced generation of Th17 cells (169, 170). Overexpression of Cdkn1a in BMPR1α-deficient Treg cells led to acquisition of mature, senescent phenotype and decreased proliferation of Treg cells. This result is consistent with ealier reports of BMPs regulating renewal and differentiation of embryonic and tissue specific stem cells including T cell progenitors (88, 115, 155). Treg cell senescence may be a factor in progression of chronic autoimmune diseases (171). In summary, BMPs and BMPR1α signaling controls critical molecular circuits, impacting both Foxp3 expression and epigenetic landscape of Treg cells. While little is known how BMPs may affect tissue resident Treg cells, one could speculate that tight control of BMP secretion, maturation and stability predisposes them to perform immunoregulatory functions and contribute to the acquisition of organ specific features.
Author Contributions
PK conceived the idea for the review, outlined, and wrote the manuscript.
Funding
This work was supported in part by funding from the R03 AI159280 grant from NIAID to PK.
Conflict of Interest
The author declares that the research was conducted in the absence of any commercial or financial relationships that could be construed as a potential conflict of interest.
Publisher’s Note
All claims expressed in this article are solely those of the authors and do not necessarily represent those of their affiliated organizations, or those of the publisher, the editors and the reviewers. Any product that may be evaluated in this article, or claim that may be made by its manufacturer, is not guaranteed or endorsed by the publisher.
References
1. Murphy KM, Reiner SL. The Lineage Decisions of Helper T Cells. Nat Rev Immunol (2002) 2(12):933–44. doi: 10.1038/nri954
2. Szabo SJ, Sullivan BM, Peng SL, Glimcher LH. Molecular Mechanisms Regulating Th1 Immune Responses. Annu Rev Immunol (2003) 21:713–58. doi: 10.1146/annurev.immunol.21.120601.140942
3. Ansel KM, Djuretic I, Tanasa B, Rao A. Regulation of Th2 Differentiation and Il4 Locus Accessibility. Annu Rev Immunol (2006) 24:607–56. doi: 10.1146/annurev.immunol.23.021704.115821
4. Weaver CT, Hatton RD, Mangan PR, Harrington LE. IL-17 Family Cytokines and the Expanding Diversity of Effector T Cell Lineages. Annu Rev Immunol (2007) 25:821–52. doi: 10.1146/annurev.immunol.25.022106.141557
5. Kulkarni AB, Huh CG, Becker D, Geiser A, Lyght M, Flanders KC, et al. Transforming Growth Factor Beta 1 Null Mutation in Mice Causes Excessive Inflammatory Response and Early Death. Proc Natl Acad Sci USA (1993) 90(2):770–4. doi: 10.1073/pnas.90.2.770
6. Li MO, Flavell RA. TGF-Beta: A Master of All T Cell Trades. Cell (2008) 134(3):392–404. doi: 10.1016/j.cell.2008.07.025
7. Bettelli E, Carrier Y, Gao W, Korn T, Strom TB, Oukka M, et al. Reciprocal Developmental Pathways for the Generation of Pathogenic Effector TH17 and Regulatory T Cells. Nature (2006) 441(7090):235–8. doi: 10.1038/nature04753
8. Kaplan MH. Th9 Cells: Differentiation and Disease. Immunol Rev (2013) 252(1):104–15. doi: 10.1111/imr.12028
9. Josefowicz SZ, Lu LF, Rudensky AY. Regulatory T Cells: Mechanisms of Differentiation and Function. Annu Rev Immunol (2012) 30:531–64. doi: 10.1146/annurev.immunol.25.022106.141623
10. Sakaguchi S, Mikami N, Wing JB, Tanaka A, Ichiyama K, Ohkura N. Regulatory T Cells and Human Disease. Annu Rev Immunol (2020) 38:541–66. doi: 10.1146/annurev-immunol-042718-041717
11. Saigusa R, Winkels H, Ley K. T Cell Subsets and Functions in Atherosclerosis. Nat Rev Cardiol (2020) 17(7):387–401. doi: 10.1038/s41569-020-0352-5
12. Burzyn D, Benoist C, Mathis D. Regulatory T Cells in Nonlymphoid Tissues. Nat Immunol (2013) 14(10):1007–13. doi: 10.1038/ni.2683
13. Munoz-Rojas AR, Mathis D. Tissue Regulatory T Cells: Regulatory Chameleons. Nat Rev Immunol (2021) 21(9):597–611. doi: 10.1038/s41577-021-00519-w
14. Chen W, Jin W, Hardegen N, Lei KJ, Li L, Marinos N, et al. Conversion of Peripheral CD4+CD25- Naive T Cells to CD4+CD25- Regulatory T Cells TGF-Beta Induction of Trasnscription Factor Foxp3. J Exp Med (2003) 198(12):1875–86. doi: 10.1084/jem.20030152
15. Thornton AM, Donovan EE, Piccirillo CA, Shevach EM. Cutting Edge: IL-2 Is Critically Required for the In Vitro Activation of CD4+CD25+ T Cell Suppressor Function. J Immunol (2004) 172(11):6519–23. doi: 10.4049/jimmunol.172.11.6519
16. Kuczma M, Pawlikowska I, Kopij M, Podolsky R, Rempala GA, Kraj P. TCR Repertoire and Foxp3 Expression Define Functionally Distinct Subsets of CD4+ Regulatory T Cells. J Immunol (2009) 183(5):3118–29. doi: 10.4049/jimmunol.0900514
17. Komatsu N, Mariotti-Ferrandiz ME, Wang Y, Malissen B, Waldmann H, Hori S. Heterogeneity of Natural Foxp3+ T Cells: A Committed Regulatory T-Cell Lineage and an Uncommitted Minor Population Retaining Plasticity. Proc Natl Acad Sci USA (2009) 106(6):1903–8. doi: 10.1073/pnas.0811556106
18. Tang Q, Bluestone JA. The Foxp3+ Regulatory T Cell: A Jack of All Trades, Master of Regulation. Nat Immunol (2008) 9(3):239–44. doi: 10.1038/ni1572
19. Wing JB, Sakaguchi S. Multiple Treg Suppressive Modules and Their Adaptability. Front Immunol (2012) 3:178. doi: 10.3389/fimmu.2012.00178
20. Kuczma M, Podolsky R, Garge N, Daniely D, Pacholczyk R, Ignatowicz L, et al. Foxp3-Deficient Regulatory T Cells do Not Revert Into Conventional Effector CD4+ T Cells But Constitute a Unique Cell Subset. J Immunol (2009) 183:3731–41. doi: 10.4049/jimmunol.0800601
21. Gavin MA, Rasmussen JP, Fontenot JD, Vasta V, Manganiello VC, Beavo JA, et al. Foxp3-Dependent Programme of Regulatory T-Cell Differentiation. Nature (2007) 445(7129):771–5. doi: 10.1038/nature05543
22. Williams LM, Rudensky AY. Maintenance of the Foxp3-Dependent Developmental Program in Mature Regulatory T Cells Requires Continued Expression of Foxp3. Nat Immunol (2007) 8(3):277–84. doi: 10.1038/ni1437
23. Lin W, Haribhai D, Relland LM, Truong N, Carlson MR, Williams CB, et al. Regulatory T Cell Development in the Absence of Functional Foxp3. Nat Immunol (2007) 8(4):359–68. doi: 10.1038/ni1445
24. Ohkura N, Hamaguchi M, Morikawa H, Sugimura K, Tanaka A, Ito Y, et al. T Cell Receptor Stimulation-Induced Epigenetic Changes and Foxp3 Expression Are Independent and Complementary Events Required for Treg Cell Development. Immunity (2012) 37(5):785–99. doi: 10.1016/j.immuni.2012.09.010
25. Floess S, Freyer J, Siewert C, Baron U, Olek S, Polansky J, et al. Epigenetic Control of the Foxp3 Locus in Regulatory T Cells. PLoS Biol (2007) 5(2):e38. doi: 10.1371/journal.pbio.0050038
26. Zheng Y, Josefowicz S, Chaudhry A, Peng XP, Forbush K, Rudensky AY. Role of Conserved Non-Coding DNA Elements in the Foxp3 Gene in Regulatory T-Cell Fate. Nature (2010) 463(7282):808–12. doi: 10.1038/nature08750
27. Polansky JK, Schreiber L, Thelemann C, Ludwig L, Kruger M, Baumgrass R, et al. Methylation Matters: Binding of Ets-1 to the Demethylated Foxp3 Gene Contributes to the Stabilization of Foxp3 Expression in Regulatory T Cells. J Mol Med (2010) 88:1029–40. doi: 10.1007/s00109-010-0642-1
28. Luu M, Steinhoff U, Visekruna A. Functional Heterogeneity of Gut-Resident Regulatory T Cells. Clin Transl Immunol (2017) 6(9):e156. doi: 10.1038/cti.2017.39
29. Haque M, Fino K, Lei F, Xiong X, Song J. Utilizing Regulatory T Cells Against Rheumatoid Arthritis. Front Oncol (2014) 4:209. doi: 10.3389/fonc.2014.00209
30. Paust S, Cantor H. Regulatory T Cells and Autoimmune Disease. Immunol Rev (2005) 204:195–207. doi: 10.1111/j.0105-2896.2005.00247.x
31. Selck C, Dominguez-Villar M. Antigen-Specific Regulatory T Cell Therapy in Autoimmune Diseases and Transplantation. Front Immunol (2021) 12:661875. doi: 10.3389/fimmu.2021.661875
32. Bennett CL, Christie J, Ramsdell F, Brunkow ME, Ferguson PJ, Whitesell L, et al. The Immune Dysregulation, Polyendocrinopathy, Enteropathy, X-Linked Syndrome (IPEX) Is Caused by Mutations of FOXP3. Nat Genet (2001) 27(1):20–1. doi: 10.1038/83713
33. Brunkow ME, Jeffery EW, Hjerrild KA, Paeper B, Clark LB, Yasayko SA, et al. Disruption of a New Forkhead/Winged-Helix Protein, Scurfin, Results in the Fatal Lymphoproliferative Disorder of the Scurfy Mouse. Nat Genet (2001) 27(1):68–73. doi: 10.1038/83784
34. Wan YY, Flavell RA. Regulatory T-Cell Functions Are Subverted and Converted Owing to Attenuated Foxp3 Expression. Nature (2007) 445(7129):766–70. doi: 10.1038/nature05479
35. Min B. Heterogeneity and Stability in Foxp3+ Regulatory T Cells. J Interferon Cytokine Res (2017) 37(9):386–97. doi: 10.1089/jir.2017.0027
36. Long SA, Buckner JH. CD4+FOXP3+ T Regulatory Cells in Human Autoimmunity: More Than a Numbers Game. J Immunol (2011) 187(5):2061–6. doi: 10.4049/jimmunol.1003224
37. Kuchroo VK, Ohashi PS, Sartor RB, Vinuesa CG. Dysregulation of Immune Homeostasis in Autoimmune Diseases. Nat Med (2012) 18(1):42–7. doi: 10.1038/nm.2621
38. Do J, Kim D, Kim S, Valentin-Torres A, Dvorina N, Jang E, et al. Treg-Specific IL-27Ralpha Deletion Uncovers a Key Role for IL-27 in Treg Function to Control Autoimmunity. Proc Natl Acad Sci USA (2017) 114(38):10190–5. doi: 10.1073/pnas.1703100114
39. Konkel JE, Zhang D, Zanvit P, Chia C, Zangarle-Murray T, Jin W, et al. Transforming Growth Factor-Beta Signaling in Regulatory T Cells Controls T Helper-17 Cells and Tissue-Specific Immune Responses. Immunity (2017) 46(4):660–74. doi: 10.1016/j.immuni.2017.03.015
40. Guo J, Zhou X. Regulatory T Cells Turn Pathogenic. Cell Mol Immunol (2015) 12(5):525–32. doi: 10.1038/cmi.2015.12
41. Zhou X, Bailey-Bucktrout SL, Jeker LT, Penaranda C, Martinez-Llordella M, Ashby M, et al. Instability of the Transcription Factor Foxp3 Leads to the Generation of Pathogenic Memory T Cells In Vivo. Nat Immunol (2009) 15(12):1000–7. doi: 10.1038/ni.1774
42. Belkaid Y, Piccirillo CA, Mendez S, Shevach EM, Sacks DL. CD4+CD25+ Regulatory T Cells Control Leishmania Major Persistence and Immunity. Nature (2002) 420(6915):502–7. doi: 10.1038/nature01152
43. Sawant DV, Vignali DA. Once a Treg, Always a Treg? Immunol Rev (2014) 259(1):173–91. doi: 10.1111/imr.12173
44. Overacre-Delgoffe AE, Chikina M, Dadey RE, Yano H, Brunazzi EA, Shayan G, et al. Interferon-Gamma Drives Treg Fragility to Promote Anti-Tumor Immunity. Cell (2017) 169(6):1130–41.e11. doi: 10.1016/j.cell.2017.05.005
45. Munn DH, Sharma MD, Johnson TS. Treg Destabilization and Reprogramming: Implications for Cancer Immunotherapy. Cancer Res (2018) 78(18):5191–9. doi: 10.1158/0008-5472.CAN-18-1351
46. Komatsu N, Okamoto K, Sawa S, Nakashima T, Oh-hora M, Kodama T, et al. Pathogenic Conversion of Foxp3+ T Cells Into TH17 Cells in Autoimmune Arthritis. Nat Med (2014) 20(1):62–8. doi: 10.1038/nm.3432
47. Bailey-Bucktrout SL, Martinez-Llordella M, Zhou X, Anthony B, Rosenthal W, Luche H, et al. Self-Antigen-Driven Activation Induces Instability of Regulatory T Cells During an Inflammatory Autoimmune Response. Immunity (2013) 39(5):949–62. doi: 10.1016/j.immuni.2013.10.016
48. Gagliani N, Amezcua Vesely MC, Iseppon A, Brockmann L, Xu H, Palm NW, et al. Th17 Cells Transdifferentiate Into Regulatory T Cells During Resolution of Inflammation. Nature (2015) 523(7559):221–5. doi: 10.1038/nature14452
49. Sakaguchi S, Vignali DA, Rudensky AY, Niec RE, Waldmann H. The Plasticity and Stability of Regulatory T Cells. Nat Rev Immunol (2013) 13(6):461–7. doi: 10.1038/nri3464
50. Miyao T, Floess S, Setoguchi R, Luche H, Fehling HJ, Waldmann H, et al. Plasticity of Foxp3(+) T Cells Reflects Promiscuous Foxp3 Expression in Conventional T Cells But Not Reprogramming of Regulatory T Cells. Immunity (2012) 36(2):262–75. doi: 10.1016/j.immuni.2011.12.012
51. Levine AG, Arvey A, Jin W, Rudensky AY. Continuous Requirement for the TCR in Regulatory T Cell Function. Nat Immunol (2014) 15(11):1070–8. doi: 10.1038/ni.3004
52. Vahl JC, Drees C, Heger K, Heink S, Fischer JC, Nedjic J, et al. Continuous T Cell Receptor Signals Maintain a Functional Regulatory T Cell Pool. Immunity (2014) 41(5):722–36. doi: 10.1016/j.immuni.2014.10.012
53. Salomon B, Lenschow DJ, Rhee L, Ashourian N, Singh B, Sharpe A, et al. B7/CD28 Costimulation Is Essential for the Homeostasis of the CD4+CD25+ Immunoregulatory T Cells That Control Autoimmune Diabetes. Immunity (2000) 12(4):431–40. doi: 10.1016/S1074-7613(00)80195-8
54. Vogel I, Kasran A, Cremer J, Kim YJ, Boon L, Van Gool SW, et al. CD28/CTLA-4/B7 Costimulatory Pathway Blockade Affects Regulatory T-Cell Function in Autoimmunity. Eur J Immunol (2015) 45(6):1832–41. doi: 10.1002/eji.201445190
55. Gogishvili T, Luhder F, Goebbels S, Beer-Hammer S, Pfeffer K, Hunig T. Cell-Intrinsic and -Extrinsic Control of Treg-Cell Homeostasis and Function Revealed by Induced CD28 Deletion. Eur J Immunol (2013) 43(1):188–93. doi: 10.1002/eji.201242824
56. Wing K, Onishi Y, Prieto-Martin P, Yamaguchi T, Miyara M, Fehervari Z, et al. CTLA-4 Control Over Foxp3+ Regulatory T Cell Function. Science (2008) 322(5899):271–5. doi: 10.1126/science.1160062
57. Lio CW, Hsieh CS. A Two-Step Process for Thymic Regulatory T Cell Development. Immunity (2008) 28(1):100–11. doi: 10.1016/j.immuni.2007.11.021
58. Malek TR, Yu A, Vincek V, Scibelli P, Kong L. CD4 Regulatory T Cells Prevent Lethal Autoimmunity in IL-2Rbeta-Deficient Mice. Implications for the Nonredundant Function of IL-2. Immunity (2002) 17(2):167–78. doi: 10.1016/s1074-7613(02)00367-9
59. Davidson TS, DiPaolo RJ, Andersson J, Shevach EM. Cutting Edge: IL-2 Is Essential for TGF-Beta-Mediated Induction of Foxp3+ T Regulatory Cells. J Immunol (2007) 178(7):4022–6. doi: 10.4049/jimmunol.178.7.4022
60. Dikiy S, Li J, Bai L, Jiang M, Janke L, Zong X, et al. A Distal Foxp3 Enhancer Enables Interleukin-2 Dependent Thymic Treg Cell Lineage Commitment for Robust Immune Tolerance. Immunity (2021) 54(5):931–46.e11. doi: 10.1016/j.immuni.2021.03.020
61. Fontenot JD, Rasmussen JP, Gavin MA, Rudensky AY. A Function for Interleukin 2 in Foxp3-Expressing Regulatory T Cells. Nat Immunol (2005) 6(11):1142–51. doi: 10.1038/ni1263
62. Setoguchi R, Hori S, Takahashi T, Sakaguchi S. Homeostatic Maintenance of Natural Foxp3(+) CD25(+) CD4(+) Regulatory T Cells by Interleukin (IL)-2 and Induction of Autoimmune Disease by IL-2 Neutralization. J Exp Med (2005) 201(5):723–35. doi: 10.1084/jem.20041982
63. Feng Y, Arvey A, Chinen T, van der Veeken J, Gasteiger G, Rudensky AY. Control of the Inheritance of Regulatory T Cell Identity by a Cis Element in the Foxp3 Locus. Cell (2014) 158(4):749–63. doi: 10.1016/j.cell.2014.07.031
64. Burchill MA, Yang J, Vang KB, Moon JJ, Chu HH, Lio CW, et al. Linked T Cell Receptor and Cytokine Signaling Govern the Development of the Regulatory T Cell Repertoire. Immunity (2008) 28(1):112–21. doi: 10.1016/j.immuni.2007.11.022
65. Ogawa C, Tone Y, Tsuda M, Peter C, Waldmann H, Tone M. TGF-Beta-Mediated Foxp3 Gene Expression Is Cooperatively Regulated by Stat5, Creb, and AP-1 Through CNS2. J Immunol (2014) 192(1):475–83. doi: 10.4049/jimmunol.1301892
66. Chinen T, Kannan AK, Levine AG, Fan X, Klein U, Zheng Y, et al. An Essential Role for the IL-2 Receptor in Treg Cell Function. Nat Immunol (2016) 17(11):1322–33. doi: 10.1038/ni.3540
67. Laurence A, Tato CM, Davidson TS, Kanno Y, Chen Z, Yao Z, et al. Interleukin-2 Signaling via STAT5 Constrains T Helper 17 Cell Generation. Immunity (2007) 26(3):371–81. doi: 10.1016/j.immuni.2007.02.009
68. Kryczek I, Wei S, Zou L, Altuwaijri S, Szeliga W, Kolls J, et al. Cutting Edge: Th17 and Regulatory T Cell Dynamics and the Regulation by IL-2 in the Tumor Microenvironment. J Immunol (2007) 178(11):6730–3. doi: 10.4049/jimmunol.178.11.6730
69. Sakaguchi S, Sakaguchi N, Asano M, Itoh M, Toda M. Immunologic Self-Tolerance Maintained by Activated T Cells Expressing IL-2 Receptor Alpha-Chains (CD25). Breakdown of a Single Mechanism of Self-Tolerance Causes Various Autoimmune Diseases. J Immunol (1995) 155(3):1151–64.
70. Shull MM, Ormsby I, Kier AB, Pawlowski S, Diebold RJ, Yin M, et al. Targeted Disruption of the Mouse Transforming Growth Factor-Beta 1 Gene Results in Multifocal Inflammatory Disease. Nature (1992) 359(6397):693–9. doi: 10.1038/359693a0
71. Gorelik L, Flavell RA. Abrogation of TGFbeta Signaling in T Cells Leads to Spontaneous T Cell Differentiation and Autoimmune Disease. Immunity (2000) 12(2):171–81. doi: 10.1016/S1074-7613(00)80170-3
72. Lucas PJ, Kim SJ, Melby SJ, Gress RE. Disruption of T Cell Homeostasis in Mice Expressing a T Cell-Specific Dominant Negative Transforming Growth Factor Beta II Receptor. J Exp Med (2000) 191(7):1187–96. doi: 10.1084/jem.191.7.1187
73. Fahlen L, Read S, Gorelik L, Hurst SD, Coffman RL, Flavell RA, et al. T Cells That Cannot Respond to TGF-Beta Escape Control by CD4(+)CD25(+) Regulatory T Cells. J Exp Med (2005) 201(5):737–46. doi: 10.1084/jem.20040685
74. Marie JC, Letterio JJ, Gavin M, Rudensky AY. TGF-{Beta}1 Maintains Suppressor Function and Foxp3 Expression in CD4+CD25+ Regulatory T Cells. J Exp Med (2005) 201(7):1061–7. doi: 10.1084/jem.20042276
75. Li MO, Sanjabi S, Flavell RA. Transforming Growth Factor-Beta Controls Development, Homeostasis, and Tolerance of T Cells by Regulatory T Cell-Dependent and -Independent Mechanisms. Immunity (2006) 25(3):455–71. doi: 10.1016/j.immuni.2006.07.011
76. Chen ML, Pittet MJ, Gorelik L, Flavell RA, Weissleder R, von BH, et al. Regulatory T Cells Suppress Tumor-Specific CD8 T Cell Cytotoxicity Through TGF-Beta Signals In Vivo. Proc Natl Acad Sci USA (2005) 102(2):419–24. doi: 10.1073/pnas.0408197102
77. Du W, Wong FS, Li MO, Peng J, Qi H, Flavell RA, et al. TGF-Beta Signaling Is Required for the Function of Insulin-Reactive T Regulatory Cells. J Clin Invest (2006) 116(5):1360–70. doi: 10.1172/JCI27030
78. Liu Y, Zhang P, Li J, Kulkarni AB, Perruche S, Chen W. A Critical Function for TGF-Beta Signaling in the Development of Natural CD4+CD25+Foxp3+ Regulatory T Cells. Nat Immunol (2008) 9(6):632–40. doi: 10.1038/ni.1607
79. Ouyang W, Beckett O, Ma Q, Li MO. Transforming Growth Factor-Beta Signaling Curbs Thymic Negative Selection Promoting Regulatory T Cell Development. Immunity (2010) 32(5):642–53. doi: 10.1016/j.immuni.2010.04.012
80. Konkel JE, Jin W, Abbatiello B, Grainger JR, Chen W. Thymocyte Apoptosis Drives the Intrathymic Generation of Regulatory T Cells. Proc Natl Acad Sci USA (2014) 111(4):E465–73. doi: 10.1073/pnas.1320319111
81. Turner JA, Stephen-Victor E, Wang S, Rivas MN, Abdel-Gadir A, Harb H, et al. Regulatory T Cell-Derived TGF-Beta1 Controls Multiple Checkpoints Governing Allergy and Autoimmunity. Immunity (2020) 53(6):1331–2. doi: 10.1016/j.immuni.2020.11.011
82. Urist MR. Bone: Formation by Autoinduction. Science (1965) 150(3698):893–9. doi: 10.1126/science.150.3698.893
83. Kishigami S, Mishina Y. BMP Signaling and Early Embryonic Patterning. Cytokine Growth Factor Rev (2005) 16(3):265–78. doi: 10.1016/j.cytogfr.2005.04.002
84. Miyazono K, Kamiya Y, Morikawa M. Bone Morphogenetic Protein Receptors and Signal Transduction. J Biochem (2010) 147(1):35–51. doi: 10.1093/jb/mvp148
85. Ferguson EL, Anderson KV. Localized Enhancement and Repression of the Activity of the TGF-Beta Family Member, Decapentaplegic, Is Necessary for Dorsal-Ventral Pattern Formation in the Drosophila Embryo. Development (1992) 114(3):583–97. doi: 10.1242/dev.114.3.583
86. Zou H, Niswander L. Requirement for BMP Signaling in Interdigital Apoptosis and Scale Formation. Science (1996) 272(5262):738–41. doi: 10.1126/science.272.5262.738
87. Wu MY, Hill CS. Tgf-Beta Superfamily Signaling in Embryonic Development and Homeostasis. Dev Cell (2009) 16(3):329–43. doi: 10.1016/j.devcel.2009.02.012
88. Ying QL, Nichols J, Chambers I, Smith A. BMP Induction of Id Proteins Suppresses Differentiation and Sustains Embryonic Stem Cell Self-Renewal in Collaboration With STAT3. Cell (2003) 115(3):281–92. doi:S009286740300847X [pii]. doi: 10.1016/S0092-8674(03)00847-X
89. Chen X, Xu H, Yuan P, Fang F, Huss M, Vega VB, et al. Integration of External Signaling Pathways With the Core Transcriptional Network in Embryonic Stem Cells. Cell (2008) 133(6):1106–17. doi: 10.1016/j.cell.2008.04.043
90. Reya T, Morrison SJ, Clarke MF, Weissman IL. Stem Cells, Cancer, and Cancer Stem Cells. Nature (2001) 414(6859):105–11. doi: 10.1038/35102167
91. Morikawa M, Derynck R, Miyazono K. TGF-Beta and the TGF-Beta Family: Context-Dependent Roles in Cell and Tissue Physiology. Cold Spring Harb Perspect Biol (2016) 8(5):e021783. doi: 10.1101/cshperspect.a021873
92. Pesu M, Watford WT, Wei L, Xu L, Fuss I, Strober W, et al. T-Cell-Expressed Proprotein Convertase Furin Is Essential for Maintenance of Peripheral Immune Tolerance. Nature (2008) 455(7210):246–50. doi: 10.1038/nature07210
93. Umulis D, O’Connor MB, Blair SS. The Extracellular Regulation of Bone Morphogenetic Protein Signaling. Development (2009) 136(22):3715–28. doi: 10.1242/dev.031534
94. Nelsen SM, Christian JL. Site-Specific Cleavage of BMP4 by Furin, PC6, and PC7. J Biol Chem (2009) 284(40):27157–66. doi: 10.1074/jbc.M109.028506
95. Mueller TD, Nickel J. Promiscuity and Specificity in BMP Receptor Activation. FEBS Lett (2012) 586(14):1846–59. doi: 10.1016/j.febslet.2012.02.043
96. Ramachandran A, Vizan P, Das D, Chakravarty P, Vogt J, Rogers KW, et al. TGF-Beta Uses a Novel Mode of Receptor Activation to Phosphorylate SMAD1/5 and Induce Epithelial-to-Mesenchymal Transition. Elife (2018) 7:e31756. doi: 10.7554/eLife.31756
97. Chen D, Zhao M, Mundy GR. Bone Morphogenetic Proteins. Growth Factors (2004) 22(4):233–41. doi: 10.1080/08977190412331279890
98. Tone Y, Furuuchi K, Kojima Y, Tykocinski ML, Greene MI, Tone M. Smad3 and NFAT Cooperate to Induce Foxp3 Expression Through Its Enhancer. Nat Immunol (2008) 9(2):194–202. doi: 10.1038/ni1549
99. Guo X, Wang XF. Signaling Cross-Talk Between TGF-Beta/BMP and Other Pathways. Cell Res (2009) 19(1):71–88. doi: 10.1038/cr.2008.302
100. Shim JH, Greenblatt MB, Xie M, Schneider MD, Zou W, Zhai B, et al. TAK1 Is an Essential Regulator of BMP Signalling in Cartilage. EMBO J (2009) 28(14):2028–41. doi: 10.1038/emboj.2009.162
101. Yamaguchi K, Nagai S, Ninomiya-Tsuji J, Nishita M, Tamai K, Irie K, et al. XIAP, a Cellular Member of the Inhibitor of Apoptosis Protein Family, Links the Receptors to TAB1-TAK1 in the BMP Signaling Pathway. EMBO J (1999) 18(1):179–87. doi: 10.1093/emboj/18.1.179
102. Kawabata M, Imamura T, Miyazono K. Signal Transduction by Bone Morphogenetic Proteins. Cytokine Growth Factor Rev (1998) 9(1):49–61. doi: 10.1016/S1359-6101(97)00036-1
103. Weiskirchen R, Meurer SK. BMP-7 Counteracting TGF-Beta1 Activities in Organ Fibrosis. Front Biosci (2013) 18:1407–34. doi: 10.2741/4189
104. Yao J, Kim TW, Qin J, Jiang Z, Qian Y, Xiao H, et al. Interleukin-1 (IL-1)-Induced TAK1-Dependent Versus MEKK3-Dependent NFkappaB Activation Pathways Bifurcate at IL-1 Receptor-Associated Kinase Modification. J Biol Chem (2007) 282(9):6075–89. doi: 10.1074/jbc.M609039200
105. Hamidi A, Song J, Thakur N, Itoh S, Marcusson A, Bergh A, et al. TGF-Beta Promotes PI3K-AKT Signaling and Prostate Cancer Cell Migration Through the TRAF6-Mediated Ubiquitylation of P85alpha. Sci Signal (2017) 10(486):eaal4186. doi: 10.1126/scisignal.aal4186
106. Zhang L, Zhou F, Garcia de Vinuesa A, de Kruijf EM, Mesker WE, Hui L, et al. TRAF4 Promotes TGF-Beta Receptor Signaling and Drives Breast Cancer Metastasis. Mol Cell (2013) 51(5):559–72. doi: 10.1016/j.molcel.2013.07.014
107. Sorrentino A, Thakur N, Grimsby S, Marcusson A, von Bulow V, Schuster N, et al. The Type I TGF-Beta Receptor Engages TRAF6 to Activate TAK1 in a Receptor Kinase-Independent Manner. Nat Cell Biol (2008) 10(10):1199–207. doi: 10.1038/ncb1780
108. Choi KC, Lee YS, Lim S, Choi HK, Lee CH, Lee EK, et al. Smad6 Negatively Regulates Interleukin 1-Receptor-Toll-Like Receptor Signaling Through Direct Interaction With the Adaptor Pellino-1. Nat Immunol (2006) 7(10):1057–65. doi: 10.1038/ni1383
109. Chen W, Ten Dijke P. Immunoregulation by Members of the TGFbeta Superfamily. Nat Rev Immunol (2016) 16(12):723–40. doi: 10.1038/nri.2016.112
110. Grgurevic L, Christensen GL, Schulz TJ, Vukicevic S. Bone Morphogenetic Proteins in Inflammation, Glucose Homeostasis and Adipose Tissue Energy Metabolism. Cytokine Growth Factor Rev (2016) 27:105–18. doi: 10.1016/j.cytogfr.2015.12.009
111. Graf D, Nethisinghe S, Palmer DB, Fisher AG, Merkenschlager M. The Developmentally Regulated Expression of Twisted Gastrulation Reveals a Role for Bone Morphogenetic Proteins in the Control of T Cell Development. J Exp Med (2002) 196(2):163–71. doi: 10.1084/jem.20020276
112. Tsai PT, Lee RA, Wu H. BMP4 Acts Upstream of FGF in Modulating Thymic Stroma and Regulating Thymopoiesis. Blood (2003) 102(12):3947–53. doi: 10.1182/blood-2003-05-1657
113. Bleul CC, Boehm T. BMP Signaling Is Required for Normal Thymus Development. JImmunol (2005) 175(8):5213–21. doi: 10.4049/jimmunol.175.8.5213
114. Hager-Theodorides AL, Ross SE, Sahni H, Mishina Y, Furmanski AL, Crompton T. Direct BMP2/4 Signaling Through BMP Receptor IA Regulates Fetal Thymocyte Progenitor Homeostasis and Differentiation to CD4+CD8+ Double-Positive Cell. Cell Cycle (2014) 13(2):324–33. doi: 10.4161/cc.27118
115. Varas A, Hager-Theodorides AL, Sacedon R, Vicente A, Zapata AG, Crompton T. The Role of Morphogens in T-Cell Development. Trends Immunol (2003) 24(4):197–206. doi: 10.1016/S1471-4906(03)00033-4
116. Hager-Theodorides AL, Outram SV, Shah DK, Sacedon R, Shrimpton RE, Vicente A, et al. Bone Morphogenetic Protein 2/4 Signaling Regulates Early Thymocyte Differentiation. J Immunol (2002) 169(10):5496–504. doi: 10.4049/jimmunol.169.10.5496
117. Kuczma M, Kurczewska A, Kraj P. Modulation of Bone Morphogenic Protein Signaling in T-Cells for Cancer Immunotherapy. J Immunotoxicol (2014) 11(4):319–27. doi: 10.3109/1547691X.2013.864736
118. Browning LM, Miller C, Kuczma M, Pietrzak M, Jing Y, Rempala G, et al. Bone Morphogenic Proteins Are Immunoregulatory Cytokines Controlling FOXP3(+) Treg Cells. Cell Rep (2020) 33(1):108219. doi: 10.1016/j.celrep.2020.108219
119. Browning LM, Pietrzak M, Kuczma M, Simms CP, Kurczewska A, Refugia JM, et al. TGF-Beta-Mediated Enhancement of TH17 Cell Generation Is Inhibited by Bone Morphogenetic Protein Receptor 1alpha Signaling. Sci Signal (2018) 11(545):eaar2125. doi: 10.1126/scisignal.aar2125
120. Martinez VG, Sacedon R, Hidalgo L, Valencia J, Fernandez-Sevilla LM, Hernandez-Lopez C, et al. The BMP Pathway Participates in Human Naive CD4+ T Cell Activation and Homeostasis. PLoS One (2015) 10(6):e0131453. doi: 10.1371/journal.pone.0131453
121. Yoshioka Y, Ono M, Osaki M, Konishi I, Sakaguchi S. Differential Effects of Inhibition of Bone Morphogenic Protein (BMP) Signalling on T-Cell Activation and Differentiation. Eur J Immunol (2012) 42(3):749–59. doi: 10.1002/eji.201141702
122. Varas A, Valencia J, Lavocat F, Martinez VG, Thiam NN, Hidalgo L, et al. Blockade of Bone Morphogenetic Protein Signaling Potentiates the Pro-Inflammatory Phenotype Induced by Interleukin-17 and Tumor Necrosis Factor-Alpha Combination in Rheumatoid Synoviocytes. Arthritis Res Ther (2015) 17:192. doi: 10.1186/s13075-015-0710-6
123. Maric I, Wensveen TT, Smoljan I, Orlic ZC, Bobinac D. Bone Morphogenetic Proteins and Signaling Pathway in Inflammatory Bowel Disease. In: Karoui S, editor. Advances in Pathogenesis and Management. London: InTech (2012).
124. Takabayashi H, Shinohara M, Mao M, Phaosawasdi P, El-Zaatari M, Zhang M, et al. Anti-Inflammatory Activity of Bone Morphogenetic Protein Signaling Pathways in Stomachs of Mice. Gastroenterology (2014) 147(2):396–406.e7. doi: 10.1053/j.gastro.2014.04.015
125. Zeisberg M, Hanai J, Sugimoto H, Mammoto T, Charytan D, Strutz F, et al. BMP-7 Counteracts TGF-Beta1-Induced Epithelial-to-Mesenchymal Transition and Reverses Chronic Renal Injury. Nat Med (2003) 9(7):964–8. doi: 10.1038/nm888
126. Huber S, Stahl FR, Schrader J, Luth S, Presser K, Carambia A, et al. Activin A Promotes the TGF-Beta-Induced Conversion of CD4+CD25- T Cells Into Foxp3+ Induced Regulatory T Cells. J Immunol (2009) 182(8):4633–40. doi: 10.4049/jimmunol.0803143
127. Lu L, Ma J, Wang X, Wang J, Zhang F, Yu J, et al. Synergistic Effect of TGF-Beta Superfamily Members on the Induction of Foxp3+ Treg. Eur J Immunol (2010) 40(1):142–52. doi: 10.1002/eji.200939618i
128. Ahn H, Han T. Regulation of TGF-Beta Signaling by PKC Depends on Tsc-22 Inducibility. Mol Cell Biochem (2012) 360(1-2):47–50. doi: 10.1007/s11010-011-1042-8
129. Tecalco-Cruz AC, Sosa-Garrocho M, Vazquez-Victorio G, Ortiz-Garcia L, Dominguez-Huttinger E, Macias-Silva M. Transforming Growth Factor-Beta/SMAD Target Gene SKIL Is Negatively Regulated by the Transcriptional Cofactor Complex SNON-Smad4. J Biol Chem (2012) 287(32):26764–76. doi: 10.1074/jbc.M112.386599
130. Fu W, Ergun A, Lu T, Hill JA, Haxhinasto S, Fassett MS, et al. A Multiply Redundant Genetic Switch ‘Locks In’ the Transcriptional Signature of Regulatory T Cells. Nat Immunol (2012) 13(10):972–80. doi: 10.1038/ni.2420i
131. Rudra D, deRoos P, Chaudhry A, Niec RE, Arvey A, Samstein RM, et al. Transcription Factor Foxp3 and Its Protein Partners Form a Complex Regulatory Network. Nat Immunol (2012) 13(10):1010–9. doi: 10.1038/ni.2402i
132. Ciofani M, Madar A, Galan C, Sellars M, Mace K, Pauli F, et al. A Validated Regulatory Network for Th17 Cell Specification. Cell (2012) 151(2):289–303. doi: 10.1016/j.cell.2012.09.016
133. Yosef N, Shalek AK, Gaublomme JT, Jin H, Lee Y, Awasthi A, et al. Dynamic Regulatory Network Controlling TH17 Cell Differentiation. Nature (2013) 496(7446):461–8. doi: 10.1038/nature11981
134. Lee YK, Mukasa R, Hatton RD, Weaver CT. Developmental Plasticity of Th17 and Treg Cells. Curr Opin Immunol (2009) 21(3):274–80. doi: 10.1016/j.coi.2009.05.021
135. Hill JA, Feuerer M, Tash K, Haxhinasto S, Perez J, Melamed R, et al. Foxp3 Transcription-Factor-Dependent and -Independent Regulation of the Regulatory T Cell Transcriptional Signature. Immunity (2007) 27(5):786–800. doi: 10.1016/j.immuni.2007.09.010
136. Lu LF, Gavin MA, Rasmussen JP, Rudensky AY. G Protein-Coupled Receptor 83 Is Dispensable for the Development and Function of Regulatory T Cells. Mol Cell Biol (2007) 27(23):8065–72. doi: 10.1128/MCB.01075-07
137. Vaeth M, Gogishvili T, Bopp T, Klein M, Berberich-Siebelt F, Gattenloehner S, et al. Regulatory T Cells Facilitate the Nuclear Accumulation of Inducible cAMP Early Repressor (ICER) and Suppress Nuclear Factor of Activated T Cell C1 (Nfatc1). Proc Natl Acad Sci USA (2011) 108(6):2480–5. doi: 10.1073/pnas.1009463108i
138. Gratz IK, Rosenblum MD, Maurano MM, Paw JS, Truong HA, Marshak-Rothstein A, et al. Cutting Edge: Self-Antigen Controls the Balance Between Effector and Regulatory T Cells in Peripheral Tissues. J Immunol (2014) 192(4):1351–5. doi: 10.4049/jimmunol.1301777
139. Toomer KH, Yuan X, Yang J, Dee MJ, Yu A, Malek TR. Developmental Progression and Interrelationship of Central and Effector Regulatory T Cell Subsets. J Immunol (2016) 196(9):3665–76. doi: 10.4049/jimmunol.1500595
140. Deaglio S, Dwyer KM, Gao W, Friedman D, Usheva A, Erat A, et al. Adenosine Generation Catalyzed by CD39 and CD73 Expressed on Regulatory T Cells Mediates Immune Suppression. J Exp Med (2007) 204(6):1257–65. doi: 10.1084/jem.20062512i
141. Cheng G, Yuan X, Tsai MS, Podack ER, Yu A, Malek TR. IL-2 Receptor Signaling Is Essential for the Development of Klrg1+ Terminally Differentiated T Regulatory Cells. J Immunol (2012) 189(4):1780–91. doi: 10.4049/jimmunol.1103768
142. Fletcher JM, Lonergan R, Costelloe L, Kinsella K, Moran B, O’Farrelly C, et al. CD39+Foxp3+ Regulatory T Cells Suppress Pathogenic Th17 Cells and Are Impaired in Multiple Sclerosis. J Immunol (2009) 183(11):7602–10. doi: 10.4049/jimmunol.0901881.
143. So T, Lee SW, Croft M. Immune Regulation and Control of Regulatory T Cells by OX40 and 4-1BB. Cytokine Growth Factor Rev (2008) 19(3-4):253–62. doi: 10.1016/j.cytogfr.2008.04.003
144. Madireddi S, Eun SY, Lee SW, Nemcovicova I, Mehta AK, Zajonc DM, et al. Galectin-9 Controls the Therapeutic Activity of 4-1BB-Targeting Antibodies. J Exp Med (2014) 211(7):1433–48. doi: 10.1084/jem.20132687
145. Crepin VF, Collins JW, Habibzay M, Frankel G. Citrobacter Rodentium Mouse Model of Bacterial Infection. Nat Protoc (2016) 11(10):1851–76. doi: 10.1038/nprot.2016.100
146. Ivanov II, McKenzie BS, Zhou L, Tadokoro CE, Lepelley A, Lafaille JJ, et al. The Orphan Nuclear Receptor RORgammat Directs the Differentiation Program of Proinflammatory IL-17+ T Helper Cells. Cell (2006) 126(6):1121–33. doi: 10.1016/j.cell.2006.07.035
147. Zhou X, Bailey-Bucktrout S, Jeker LT, Bluestone JA. Plasticity of CD4(+) FoxP3(+) T Cells. Curr Opin Immunol (2009) 21(3):281–5. doi: 10.1016/j.coi.2009.05.007
148. Yang XO, Nurieva R, Martinez GJ, Kang HS, Chung Y, Pappu BP, et al. Molecular Antagonism and Plasticity of Regulatory and Inflammatory T Cell Programs. Immunity (2008) 29(1):44–56. doi: 10.1016/j.immuni.2008.05.007
149. Gao Y, Tang J, Chen W, Li Q, Nie J, Lin F, et al. Inflammation Negatively Regulates FOXP3 and Regulatory T-Cell Function via DBC1. Proc Natl Acad Sci USA (2015) 112(25):E3246–54. doi: 10.1073/pnas.1421463112
150. Blatner NR, Mulcahy MF, Dennis KL, Scholtens D, Bentrem DJ, Phillips JD, et al. Expression of RORgammat Marks a Pathogenic Regulatory T Cell Subset in Human Colon Cancer. Sci Transl Med (2012) 4(164):164ra159. doi: 10.1126/scitranslmed.3004566
151. Munoz-Espin D, Canamero M, Maraver A, Gomez-Lopez G, Contreras J, Murillo-Cuesta S, et al. Programmed Cell Senescence During Mammalian Embryonic Development. Cell (2013) 155(5):1104–18. doi: 10.1016/j.cell.2013.10.019
152. Arias CF, Ballesteros-Tato A, Garcia MI, Martin-Caballero J, Flores JM, Martinez AC, et al. P21cip1/WAF1 Controls Proliferation of Activated/Memory T Cells and Affects Homeostasis and Memory T Cell Responses. J Immunol (2007) 178(4):2296–306. doi: 10.4049/jimmunol.178.4.2296
153. Salminen A, Kaarniranta K, Hiltunen M, Kauppinen A. Histone Demethylase Jumonji D3 (JMJD3/KDM6B) at the Nexus of Epigenetic Regulation of Inflammation and the Aging Process. J Mol Med (Berl) (2014) 92(10):1035–43. doi: 10.1007/s00109-014-1182-x
154. Zhao W, Li Q, Ayers S, Gu Y, Shi Z, Zhu Q, et al. Jmjd3 Inhibits Reprogramming by Upregulating Expression of INK4a/Arf and Targeting PHF20 for Ubiquitination. Cell (2013) 152(5):1037–50. doi: 10.1016/j.cell.2013.02.006
155. Li Z, Fei T, Zhang J, Zhu G, Wang L, Lu D, et al. BMP4 Signaling Acts via Dual-Specificity Phosphatase 9 to Control ERK Activity in Mouse Embryonic Stem Cells. Cell Stem Cell (2012) 10(2):171–82. doi: 10.1016/j.stem.2011.12.016
156. Selma Dagtas A, Gilbert KM. P21(Cip1) Up-Regulated During Histone Deacetylase Inhibitor-Induced CD4(+) T-Cell Anergy Selectively Associates With Mitogen-Activated Protein Kinases. Immunology (2010) 129(4):589–99. doi: 10.1111/j.1365-2567.2009.03161.x
157. Wang D, Quiros J, Mahuron K, Pai CC, Ranzani V, Young A, et al. Targeting EZH2 Reprograms Intratumoral Regulatory T Cells to Enhance Cancer Immunity. Cell Rep (2018) 23(11):3262–74. doi: 10.1016/j.celrep.2018.05.050
158. Xiao XY, Li YT, Jiang X, Ji X, Lu X, Yang B, et al. EZH2 Deficiency Attenuates Treg Differentiation in Rheumatoid Arthritis. J Autoimmun (2020) 108:102404. doi: 10.1016/j.jaut.2020.102404
159. Arvey A, van der Veeken J, Samstein RM, Feng Y, Stamatoyannopoulos JA, Rudensky AY. Inflammation-Induced Repression of Chromatin Bound by the Transcription Factor Foxp3 in Regulatory T Cells. Nat Immunol (2014) 15(6):580–7. doi: 10.1038/ni.2868
160. DuPage M, Chopra G, Quiros J, Rosenthal WL, Morar MM, Holohan D, et al. The Chromatin-Modifying Enzyme Ezh2 Is Critical for the Maintenance of Regulatory T Cell Identity After Activation. Immunity (2015) 42(2):227–38. doi: 10.1016/j.immuni.2015.01.007
161. Sarmento OF, Svingen PA, Xiong Y, Sun Z, Bamidele AO, Mathison AJ, et al. The Role of the Histone Methyltransferase Enhancer of Zeste Homolog 2 (EZH2) in the Pathobiological Mechanisms Underlying Inflammatory Bowel Disease (IBD). J Biol Chem (2017) 292(2):706–22. doi: 10.1074/jbc.M116.749663
162. Dominguez-Villar M, Hafler DA. Regulatory T Cells in Autoimmune Disease. Nat Immunol (2018) 19(7):665–73. doi: 10.1038/s41590-018-0120-4
163. Grant CR, Liberal R, Mieli-Vergani G, Vergani D, Longhi MS. Regulatory T-Cells in Autoimmune Diseases: Challenges, Controversies and–Yet–Unanswered Questions. Autoimmun Rev (2015) 14(2):105–16. doi: 10.1016/j.autrev.2014.10.012
164. Weinmann AS. Roles for Helper T Cell Lineage-Specifying Transcription Factors in Cellular Specialization. Adv Immunol (2014) 124:171–206. doi: 10.1016/B978-0-12-800147-9.00006-6
165. Rubtsov YP, Niec RE, Josefowicz S, Li L, Darce J, Mathis D, et al. Stability of the Regulatory T Cell Lineage In Vivo. Science (2010) 329(5999):1667–71. doi: 10.1126/science.1191996i
166. Burzyn D, Kuswanto W, Kolodin D, Shadrach JL, Cerletti M, Jang Y, et al. A Special Population of Regulatory T Cells Potentiates Muscle Repair. Cell (2013) 155(6):1282–95. doi: 10.1016/j.cell.2013.10.054
167. Luo CT, Li MO. Transcriptional Control of Regulatory T Cell Development and Function. Trends Immunol (2013) 34(11):531–9. doi: 10.1016/j.it.2013.08.003
168. Ohkura N, Sakaguchi S. Transcriptional and Epigenetic Basis of Treg Cell Development and Function: Its Genetic Anomalies or Variations in Autoimmune Diseases. Cell Res (2020) 30(6):465–74. doi: 10.1038/s41422-020-0324-7
169. Liu Z, Cao W, Xu L, Chen X, Zhan Y, Yang Q, et al. The Histone H3 Lysine-27 Demethylase Jmjd3 Plays a Critical Role in Specific Regulation of Th17 Cell Differentiation. J Mol Cell Biol (2015) 7(6):505–16. doi: 10.1093/jmcb/mjv022
170. Cribbs AP, Terlecki-Zaniewicz S, Philpott M, Baardman J, Ahern D, Lindow M, et al. Histone H3K27me3 Demethylases Regulate Human Th17 Cell Development and Effector Functions by Impacting on Metabolism. Proc Natl Acad Sci USA (2020) 117(11):6056–66. doi: 10.1073/pnas.1919893117
Keywords: Treg, Th17, BMP, BMPR1α, immunity, epigenetic, Kdm6b, Cdkn1a
Citation: Kraj P (2022) Bone Morphogenetic Proteins Shape Treg Cells. Front. Immunol. 13:865546. doi: 10.3389/fimmu.2022.865546
Received: 30 January 2022; Accepted: 07 March 2022;
Published: 28 March 2022.
Edited by:
Akihiko Yoshimura, Keio University, JapanReviewed by:
Wanjun Chen, National Institutes of Health (NIH), United StatesKenji Ichiyama, Osaka University, Japan
Copyright © 2022 Kraj. This is an open-access article distributed under the terms of the Creative Commons Attribution License (CC BY). The use, distribution or reproduction in other forums is permitted, provided the original author(s) and the copyright owner(s) are credited and that the original publication in this journal is cited, in accordance with accepted academic practice. No use, distribution or reproduction is permitted which does not comply with these terms.
*Correspondence: Piotr Kraj, cGtyYWpAb2R1LmVkdQ==