- 1Division of Pulmonary, Allergy, Critical Care and Sleep Medicine, Department of Medicine, Emory University School of Medicine, Atlanta, GA, United States
- 2Section of Infectious Diseases, Department of Medicine, Boston University School of Medicine, Boston, MA, United States
- 3Rollins School of Public Health, Emory University, Atlanta, GA, United States
- 4Atlanta VA Medical Center, Atlanta, GA, United States
Globally, an estimated 107 million people have an alcohol use disorder (AUD) leading to 2.8 million premature deaths each year. Tuberculosis (TB) is one of the leading causes of death globally and over 8% of global TB cases are estimated to be attributable to AUD. Social determinants of health such as poverty and undernutrition are often shared among those with AUD and TB and could explain the epidemiologic association between them. However, recent studies suggest that these shared risk factors do not fully account for the increased risk of TB in people with AUD. In fact, AUD has been shown to be an independent risk factor for TB, with a linear increase in the risk for TB with increasing alcohol consumption. While few studies have focused on potential biological mechanisms underlying the link between AUD and TB, substantial overlap exists between the effects of alcohol on lung immunity and the mechanisms exploited by Mycobacterium tuberculosis (Mtb) to establish infection. Alcohol misuse impairs the immune functions of the alveolar macrophage, the resident innate immune effector in the lung and the first line of defense against Mtb in the lower respiratory tract. Chronic alcohol ingestion also increases oxidative stress in the alveolar space, which could in turn facilitate Mtb growth. In this manuscript, we review the epidemiologic data that links AUD to TB. We discuss the existing literature on the potential mechanisms by which alcohol increases the risk of TB and review the known effects of alcohol ingestion on lung immunity to elucidate other mechanisms that Mtb may exploit. A more in-depth understanding of the link between AUD and TB will facilitate the development of dual-disease interventions and host-directed therapies to improve lung health and long-term outcomes of TB.
1 Introduction
Alcohol misuse is a significant global health issue with wide-ranging and pervasive consequences. In 2016, 5.3% of all global deaths were attributable to alcohol consumption, and alcohol misuse was the 7th leading risk factor for premature death and disability (1, 2). Alcohol use disorder (AUD) has been linked to an increased susceptibility to pulmonary infections and their associated complications for over 200 years (3). More recently, AUD has been found to be an independent risk factor for acute respiratory distress syndrome (ARDS) with a two to four-fold increased risk compared to individuals without AUD (4, 5). Similarly, persons with AUD have an increased risk for bacterial pneumonia and its associated morbidity and mortality (6–11). They also suffer from a higher incidence of serious complications from pneumonia, including bacteremia, parapneumonic effusion, and empyema (9, 12–14). AUD causes a variety of detrimental effects on the lungs including increased alveolar oxidative stress, immune impairments, and alterations in the metabolism of pulmonary cells.
Despite advances in diagnosis and treatment, tuberculosis (TB) is the second leading infectious killer worldwide, only recently surpassed by COVID-19 (15). While the global TB incidence rate has been decreasing annually since 2000, the most recent World Health Organization (WHO) global TB data reported an increase in TB mortality for the first time in 20 years, driven in large part by disruption of TB control programs from the COVID-19 pandemic (15).
The recognition of the association between alcohol and TB occurred even before the causative agent of TB was known. As early as the 19th century, physicians noted the increased incidence of infections, like TB and other causes of pneumonia, among patients that consumed alcohol (3, 16). The co-occurrence of excessive alcohol intake and TB has been continually noted since that time. AUD is one of the most common global risk factors for TB, second only to undernutrition and, notably, ahead of HIV and smoking (15). In this critical review, we will parse the complex relationship between alcohol and TB. We highlight recent epidemiologic work demonstrating a direct relationship between alcohol misuse and TB. We discuss mechanisms by which alcohol causes lung injury and suppresses lung immunity via increases in oxidative stress and impairments to the pulmonary innate immune system. For each of these biological pathways impacted by alcohol, we will highlight the potential mechanisms that might favor Mtb infection and dissemination.
2 Alcohol and TB Epidemiology
Epidemiologic data support a clear relationship between alcohol and TB and reveal the various biologic, immunologic, and clinical impacts that alcohol may have on patients with TB (Figure 1).
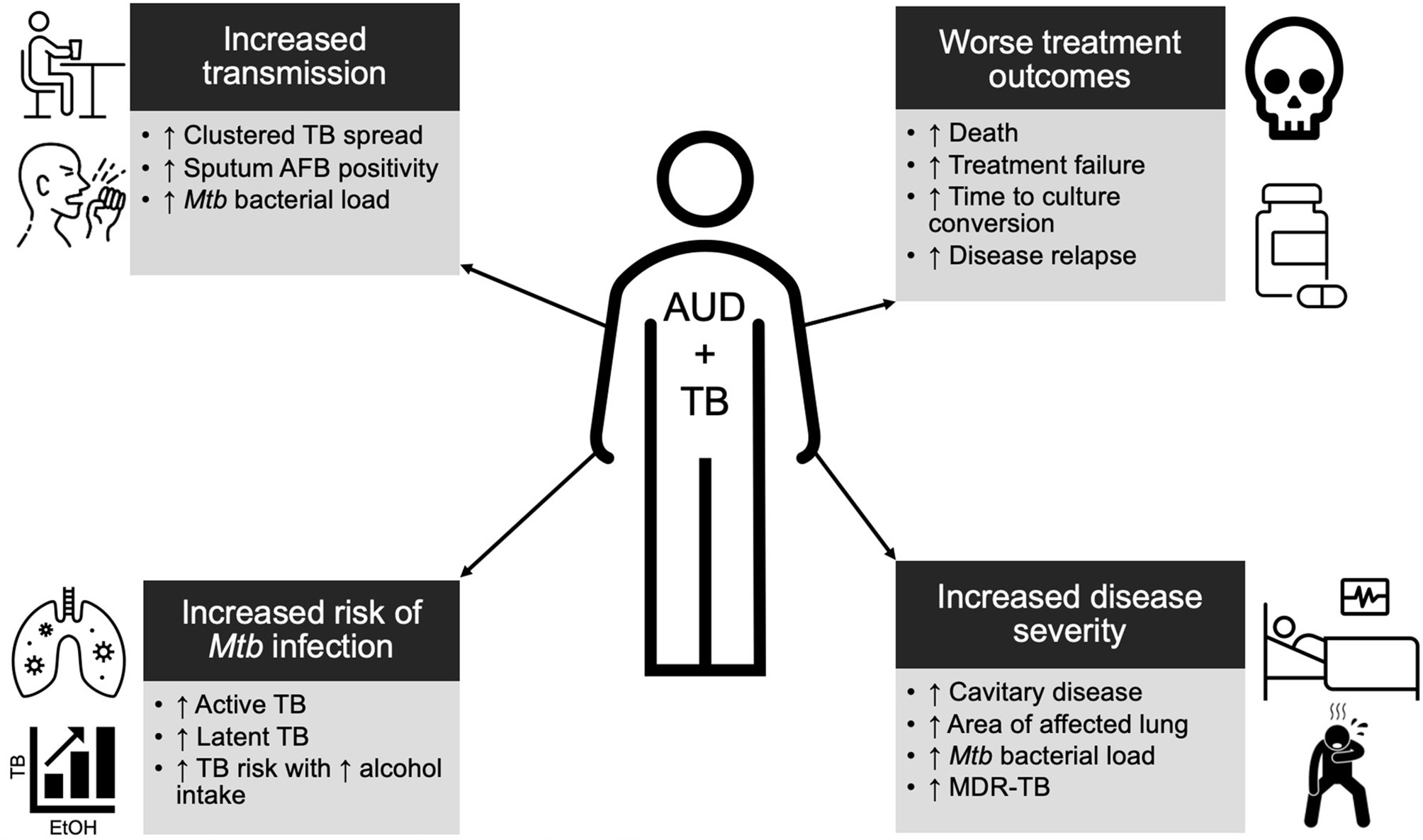
Figure 1 Summary schema of the epidemiologic data of alcohol use disorder (AUD) and tuberculosis (TB). Individuals with AUD are at a higher risk for TB, more infectious, have more severe disease, and are more likely to experience poor outcomes. See main text for further details.
2.1 Risk of Infection
The risk of both latent TB infection (LTBI) and active TB disease is higher among persons with alcohol use disorders (AUD) than those without AUD (17). For example, one study in New York City found a 28-fold higher rate of active TB disease among those with AUD as compared to age-matched individuals without AUD (18). Other studies have documented a dose-response relationship between active TB and alcohol consumption, with the risk of TB rising as a person’s daily alcohol consumption increases (19, 20).
While improvements in diagnostics and broader access to treatment have led to global declines in TB incidence since 2000 (21), cases of TB associated with AUD are on the rise, particularly among men (22, 23). It is estimated that 8-15% of global TB deaths are attributable to alcohol misuse and AUD (15, 20, 24). In high-income countries where non-communicable diseases, including diabetes and AUD, have a greater prevalence, over 35% of TB deaths among those under the age of 65 are linked to alcohol misuse (25).
Although the interactions between poverty, social marginalization, alcohol misuse, and TB remain complicated and difficult to disentangle, their overlap does not fully explain the increased risk of TB with AUD. Studies examining the relationship between AUD and TB have shown that AUD remains a significant independent risk factor for TB, with a relative risk of 2.9, even after controlling for confounders such as comorbidities, lifestyle, or social determinants of health (20, 24, 26–28).
2.2 Risk of Transmission and Severity
Individuals with TB and AUD are more infectious and have more clinically severe TB. Analyses of TB outbreaks have shown clustered TB spread and transmission among persons with AUD, and drinking venues and bars have been identified as sites of TB transmission (29–31). Further, molecular epidemiology studies have shown individuals with AUD are more likely to reflect recent TB transmission and to be part of a transmission cluster (32, 33). While this increased transmission may be due to the social marginalization often seen with AUD, it could also be due to an increased mycobacterial burden in persons with AUD. Cases of TB associated with AUD have higher rates of acid-fast bacilli (AFB) detected in sputum samples and increased Mtb bacterial load compared to TB without an AUD association (25, 26). Both of these characteristics have previously been associated with more severe TB features, including cavitation, as well as increased Mtb transmission and thus may explain some of the increased transmissibility of TB in AUD (25, 26, 34–36). Those with AUD and TB are more likely to present with pulmonary rather than extrapulmonary TB (25, 26). More of their lungs are affected by TB and they are predisposed to advanced, cavitary disease at the time of presentation (25, 26, 35, 37).
2.3 Treatment Outcomes
For those who initiate treatment, patients with TB and AUD have worse clinical outcomes, including increased time to culture conversion, incidence of TB treatment failure, rate of disease relapse, and risk of death (25, 28, 38–43). Again, the intersection of alcohol and certain social determinants complicates the interpretation of these data. For example, it is known that AUD has been associated with delays in accessing TB care and poor adherence to TB treatment, both of which may contribute to the more advanced, severe disease at the time of diagnosis and inadequate therapy (44, 45). More recent data indicate an increased incidence of multi-drug resistant TB in people with AUD, possibly due to poor treatment adherence as well as comorbidities associated with AUD that impact immune function and metabolism (43, 46, 47). However, as described above, poor TB outcomes persist among individuals with AUD even when controlling for behaviors that impact access to and retention in treatment (e.g., individuals with AUD lost to treatment follow-up) (28). A two- to four-fold increased risk of death remains for those with TB associated with AUD compared to those without AUD even when only considering patients being actively treated for TB (25, 48, 49).
2.4 Efficacy of Treatment
Alcohol-associated metabolic dysfunction and adverse drug effects have been a concern regarding individuals with AUD and TB. Studies have shown alcohol’s potential impact on the metabolism, absorption, and resultant concentrations of several TB drugs including isoniazid, rifampicin, and fluoroquinolones (50–58). Proper drug concentrations are essential for successful TB treatment. Sub-therapeutic concentrations are predictive of poor outcomes in TB, including death or disease relapse, while supra-therapeutic concentrations can lead to adverse events and treatment interruptions (59). Historically, such concerns have led to the exclusion of individuals with AUD from studies of TB preventative therapy. However, clinical trials are underway investigating the true benefit vs. harm of TB preventative therapy in persons with AUD (60). Alcohol intervention programs may also play an important role in the future of treatment for TB associated with AUD. Previous studies have shown a desire for such programs among TB patients, and the initiation of intervention programs led to favorable outcomes with improved treatment adherence in individuals with AUD and TB (61, 62).
3 Mechanisms of TB in AUD
3.1 Oxidative Stress
The lung’s constant exposure to the external environment and resultant processing of inhaled smoke, dust particles, microbes, toxins, etc. generates free radicals, including reactive oxygen species (ROS) and reactive nitrogen species (RNS), that are released into the alveolar environment. In the lungs, there are efficient antioxidant defense systems, including antioxidant enzymes and antioxidant stores, that defend against oxidants and other reactive species (63, 64). Maintaining a balanced oxidation-reduction (redox) state is essential for key cellular functions, such as proliferation, differentiation, and apoptosis (63). Redox balance can alter protein structure and reactivity as well as cell signaling pathways (65). These alterations can result in a release of inflammatory mediators and cytokines with subsequent macrophage activation, polymorphonuclear (PMN) cell recruitment, inflammation, and tissue damage (64). The redox state can be assessed by measuring thiol/disulfide couples including glutathione (GSH) and glutathione disulfide (GSSG), the primary thiol redox system within the alveoli (63). Epithelial lining fluid of a healthy lung has abundant extracellular GSH in order to detoxify oxidants and free radicals (63–65). Oxidative stress in the lung results when its antioxidant capacity is depleted. Both chronic alcohol ingestion as well as Mtb infection increase oxidative stress.
3.1.1 Alcohol Increases Pulmonary Oxidative Stress
AUD increases pulmonary oxidative stress and induces an oxidized microenvironment within the lung through a variety of mechanisms. AUD depletes antioxidant stores, including GSH, within the pulmonary environment (66). GSH serves as the primary reducing agent in the alveolar space, acting as a substrate in a reaction with glutathione peroxidase that detoxifies peroxides in the lung including hydrogen peroxide and lipid peroxides (67). AUD alters pulmonary GSH homeostasis, resulting in an oxidation of GSH stores to form GSSG and oxidation of the GSH/GSSG redox potential (66, 68–70). Alcohol-induced depletion of GSH impairs an essential defense mechanism against oxidative stress in the lung.
There are a number of mechanisms through which alcohol depletes pulmonary GSH stores. First, alcohol induces mitochondrial dysfunction which decreases ATP generation and, in turn, may decrease GSH synthesis (68, 71). GSH can also be synthesized by reduction of GSSG, which utilizes NADPH as the electron donor. However, alcohol may reduce NADPH availability, resulting in decreased capacity for reduction and less GSH (71). Alcohol also increases ROS generation which then oxidizes GSH, further depleting GSH stores (71–73). A primary mechanism by which alcohol impairs GSH is its effects on the protein nuclear factor (erythroid-derived 2)-like 2 (Nrf2). Since Nrf2 is a transcription factor that activates hundreds of antioxidant genes and innate immune effectors (74), alcohol-mediated decreases in Nrf2 activation critically impairs the lung redox balance of those with AUD. Downstream effects from Nrf2 impairment include a diminished antioxidant response to oxidative stress and drained GSH stores (74).
AUD further increases alveolar oxidative stress by enhancing the expression and activity of NADPH oxidases (Nox). Nox proteins are membrane-associated enzymes that catalyze the reduction of molecular oxygen to superoxide and hydrogen peroxide, thus serving as major sources of ROS in the lungs. In alcohol-fed mice and rats, increased Nox expression increases alveolar oxidative stress (72, 73, 75). AUD depletes alveolar macrophage levels of peroxisome proliferator-activated receptor gamma (PPARγ) which, in turn, upregulates Nox proteins (76). Nox activity is likely further enhanced by the increase in TGFβ expression seen with AUD, which also upregulates particular Nox proteins (77, 78).
The increased oxidative stress within the alveolar microenvironment of individuals with AUD has important implications for pulmonary innate immunity. GSH deficiency increases alveolar epithelial intercellular permeability and diminishes surfactant synthesis (69, 79, 80). The oxidative stress associated with upregulated Nox protein expression impairs alveolar macrophage phagocytosis in alcohol-fed mice (72, 76). In environments of limited GSH, alveolar macrophage phagocytosis and microbe clearance are also compromised (68, 71). Supplementation of GSH has been demonstrated to restore alveolar macrophage function (71, 81, 82).
3.1.2 Mtb Increases Pulmonary Oxidative Stress
Infection with Mtb has also been shown to increase oxidative stress and deplete antioxidant levels in the lungs. People with active TB disease had lower circulating levels of serum thiol and increased levels of its oxidized product serum disulfide (83). More broadly, mycobacterial infections, including Mtb and Mycobacterium abscessus (a rapid growing mycobacterium), have been associated with increased oxidative stress, as shown by decreases in total serum antioxidant capacity, total GSH, and increased lipid peroxidation (84–86). Treatment with antioxidants, including N-acetylcysteine (a precursor to GSH), have been shown to reduce oxidative stress as well as intracellular and pulmonary Mtb burden, while improving cell viability (84, 85).
Nrf2 is also an important aspect of the response to Mtb. Its expression is upregulated in Mtb infection due to the associated increase in ROS and oxidative stress (87, 88). Studies done in vitro with human macrophages have demonstrated benefit with pharmacologic Nrf2. Specifically, Nrf2 activation decreased oxidative injury, mitochondrial depolarization, and Mtb-induced ROS production in addition to inhibiting programmed necrosis of the macrophage (89).
An increase in oxidative stress, in the context of Mtb infection, allows for enhanced mycobacterial growth and survival. Mtb grows in vitro at a faster rate in more oxidized environments, as in the lung apices where clinical TB disease is most common (90). These observations are supported by a series of experiments with M. abscessus where increased intracellular growth was seen in more oxidized environments (86).
3.1.3 Pulmonary Oxidative Stress: AUD and Mtb Overlap
Taken together, the co-occurrence of AUD and Mtb infection likely results in a significant increase in pulmonary oxidative stress state which benefits Mtb. First, the combination of oxidative stress and impairments in antioxidant defenses, particularly the depletion of GSH stores and Nrf2 inhibition by alcohol, may sufficiently derange immune function and facilitate Mtb infection and growth. Second, Mtb’s growth and survival improves in oxidative environments, making the oxidized alveoli in those with AUD a more ideal environment for Mtb. Collectively, alcohol-induced oxidative stress may generate the ideal combination for Mtb infection: an oxidized alveolus and an impaired host response.
3.2 Pulmonary Innate Immunity
The pulmonary innate immune system is a multilayered system for defense against pathogens, detection of tissue damage, and maintaining pulmonary tissue integrity and homeostasis (91). Chronic alcohol misuse exerts a wide range of effects on this system resulting in impairments of several vital functions of innate immunity (92, 93). Its adverse effects impact the basic defense and barrier functions of the cilia and alveolar epithelium as well as the functions of specialized cells including alveolar macrophages and PMNs. For example, AUD inhibits neutrophil margination, influx from the peripheral circulation into the alveolar space, and subsequent pathogen clearance and killing during infection (92, 94–96). Antigen-presenting cells (APCs) of the innate immune system, necessary for adaptive immune activation, have a decreased peripheral presence and impaired activity due to alcohol (97, 98).
Alongside the growing understanding of the negative impacts of alcohol, the understanding of Mtb infection and host-pathogen interactions is also evolving. With the increased occurrence and severity of TB with AUD, it is likely that Mtb exploits alcohol-mediated impairments in pulmonary innate immunity to establish infection and disseminate. Previous research has elucidated alcohol’s deleterious effects on pulmonary mucociliary function, alveolar epithelium, and the alveolar macrophage. Given this breadth of information, in addition to the alveolar macrophage being first line of defense in the alveolar space and the dominant cell type that Mtb infects, we will spend the remaining portion of this section reviewing the specific effects of chronic alcohol misuse on the pulmonary innate immunity and how these alcohol-mediated alterations may intersect with Mtb pathogenicity.
3.2.1 Pulmonary Mucociliary Function
Ciliated airway cells are the first line of defense against inhaled pathogens and clear foreign particles from the lung. Chronic alcohol use repetitively exposes airways to ethanol eliminated from the bronchial circulation in exhaled breath. This repetitive injury impairs the integrity and function of airway cell cilia, ultimately leading to desensitization and resistance to motility, a phenomenon referred to as alcohol-induced ciliary dysfunction (99–101).
In the context of Mtb, this ciliary dysfunction facilitates transmission of airborne pathogens like Mtb into the lower airways, making it more likely that inhaled microbes will establish infection (102). Given that ciliary dysfunction has been associated with mycobacterial pulmonary infections, alcohol-induced ciliary dysfunction represents a likely mechanism by which AUD could predispose the host to Mtb infection (103).
3.2.2 Alveolar Epithelium
Chronic alcohol use is also known to disrupt the pulmonary epithelial structure and function. AUD prevents the formation of a reliable, physical barrier of the alveolar epithelium by impairing tight junctions within its monolayer. Tight junctions are an important aspect of the epithelium as they closely associate cells and limit the passage of water, proteins, and other solutes across cell layers (104). Chronic alcohol ingestion alters the expression and interaction of essential components of the tight junctions, including claudin-1, claudin-5, claudin-7, occludin, and zonula occludens-1, resulting in a five-fold increase in pulmonary epithelial permeability (79, 105–107). People with AUD have increased alveolar-capillary permeability which predisposes them to the development of non-cardiogenic pulmonary edema compared to individuals without AUD (108, 109). Experiments in animal models support this mechanism of injury, with chronic alcohol consumption in rats increasing susceptibility to edematous lung injury (69). Alcohol also increases TGF-β1 expression while inhibiting granulocyte/macrophage colony-stimulating factor (GM-CSF) in the alveolar space, both of which have been implicated in disrupting and increasing the permeability of the alveolar epithelium (77, 110, 111). Lastly, AUD reduces the alveolar epithelial cell synthesis of surfactant, an important pattern recognition molecule that binds to various microbes and targets them for immune clearance (112, 113). Surfactant proteins have been shown to function as an opsonin that increases the Mtb-macrophage interaction and upregulates phagocytosis (114). Although the mechanism by which Mtb gains access to the lung interstitium from the alveolus is not fully understood, the putative mechanisms proposed in the literature include direct infection of alveolar epithelial cells and migration of Mtb-infected macrophages across the alveolar epithelium (115, 116). Both scenarios would be significantly more likely in the setting of alcohol-induced tight junction impairments and the more permeable alveolar epithelium of the alcohol-affected lung.
3.2.3 Alveolar Macrophage
3.2.3.1 Key Functions
The alveolar macrophage is essential for maintaining homeostasis of the lower airways through phagocytosis, removal of debris, and efferocytosis. It is also the first line of pulmonary immune defense in the alveolar space, responsible for recognizing, ingesting, and clearing pathogens, as well as release of cytokines and chemokines to recruit PMNs and monocytes to the site of invasion. Through a multitude of pathways and effects, AUD causes significant alveolar macrophage dysfunction, impairing phagocytosis, pathogen clearance, and cytokine release (71, 72, 76, 81, 82, 117–120).
AUD interferes with the maturation and terminal differentiation of the alveolar macrophage through inhibition of multiple signaling pathways. Alcohol interferes with GM-CSF signaling by downregulating GMCSF-Rβ expression on the alveolar macrophage surface resulting in impaired phagocytic function (117). Impairing GM-CSF signaling results in diminished expression of PU.1, a GM-CSF-dependent regulatory transcription factor required for normal alveolar macrophage cell development and differentiation as well as alveolar macrophage phagocytosis, pathogen killing, and cytokine production (118, 119, 121, 122). In addition to its previously mentioned role in oxidative defensive, Nrf2 additionally participates in alveolar macrophage maturation by increasing PU.1 expression by binding to its promoter region. Alcohol’s inhibition of Nrf2 has been shown to also be responsible for the decreased PU.1 expression of AUD (123).
Mtb’s interaction with the innate immune system is an ongoing area of interest and research. The alveolar macrophage is the primary cell that Mtb infects once it enters the lower respiratory tract (115, 116). Once phagocytosed by the alveolar macrophage, Mtb actively blocks phagosome maturation and fusion with the lysosome to ensure its survival and establish its intracellular niche (115, 124). In some cases, the alveolar macrophage is able to achieve successful intracellular killing of Mtb, likely through IFNγ and nitric oxide synthase signaling pathways (124). However, when Mtb evades killing, it replicates and eventually disrupts the phagosome membrane allowing Mtb into the alveolar macrophage cytosol for further replication. After infecting the alveolar macrophage, Mtb then gains access to the lung interstitium, where granuloma formation occurs (115). Subsequent host-pathogen interactions determine whether infection is cleared or if there is progression to latent TB infection or active TB disease, however a discussion of these later events is beyond the scope of this review (115).
GM-CSF is known to be an important for the innate immune response to mycobacterial infections, particularly with its role in restricting bacillary growth and promoting mycobacterial clearance (125–127). Patients with mycobacterial pulmonary infections have higher rates of GM-CSF signaling dysfunction compared to healthy controls (128). Further, treatment with recombinant GM-CSF (rGM-CSF) prior to mycobacterial infection enhanced intracellular killing and phagolysosomal fusion after Mtb infection as well as intracellular mycobacterial killing and superoxide anion release after Mycobacterium avium complex (MAC) infection (125, 129). Inhibiting GM-CSF by neutralizing GM-CSF antibodies prior to Mtb exposure dampens the proinflammatory cytokine release and neutrophil recruitment and increases Mtb burden in mouse macrophages (127). Thus, alcohol’s downregulation of GMCSF-Rβ on AMs and subsequent decreased GM-CSF expression likely contribute to an impaired response to Mtb infection.
Alcohol-induced impairments in innate immunity provide multiple avenues for facilitating Mtb infection. Data is limited on alcohol’s specific effects on the alveolar macrophage in the case of Mtb infection, however multiple studies have investigated other mycobacterial infections including MAC. Several studies done in vitro using human macrophages demonstrated that exposure to alcohol enhanced the intracellular growth of MAC and diminished the macrophage response to inflammatory cytokines (130, 131). Chronic exposure to alcohol also diminished macrophage production of bactericidal, innate immune effectors in response to MAC infection in a mouse model (132). Additional experiments showed increased dissemination, impaired pulmonary granuloma formation, and increased mycobacterial burden in alcohol-fed mice following mycobacterial infection (130, 133).
3.2.3.2 Alveolar Macrophage Phenotype
Macrophages act as surveillance for the innate immune system, recognizing pathogens and tissue damage via pathogen-associated molecular patterns (PAMPs), damage-associated molecular patterns (DAMPs), and pattern recognition receptors. They have robust phagocytic and killing abilities and act as initiators of the inflammatory response. They function as antigen presenting cells that assist in the activation of the adaptive immune system. Further, macrophages participate in tissue repair, tissue remodeling, and maintain homeostasis (91). This plasticity in macrophage function occurs in response to surrounding physiologic state and cellular signaling and is referred to as macrophage phenotype (134).
Two paradigmatic states of the macrophage were initially described. The first being the “pro-inflammatory” or “classically activated” macrophage that responds to bacteria, viruses, lipopolysaccharide (LPS), and interferon gamma (IFNγ). It produces proinflammatory cytokines and chemokines like interleukin (IL)-12 and tumor necrosis factor alpha (TNFα), induces further inflammation and IFNγ release, and attracts neutrophils, natural killer (NK) cells, and lymphocytes to the site of infection (135). It relies heavily on glycolysis and fatty acid synthesis and a decrease in mitochondrial respiration (136). The second paradigmatic activation state is the “anti-inflammatory” or “alternatively activated” macrophage that is stimulated by IL-4 and participates in wound healing and tissue repair. It produces anti-inflammatory cytokines including IL-10 and IL-13 to reduce inflammation and promote tissue growth (135). Its metabolism is dependent on the tricarboxylic acid cycle (TCA) cycle and enhanced fatty acid oxidation (136). While macrophage differentiation was previously considered to be dichotomous and terminal, recent research suggests a more dynamic spectrum of activation and function. Depending on various extracellular signals, what were previously defined as all “anti-inflammatory” macrophages can exhibit dramatic differences in physiology, including overlap in function with some “pro-inflammatory” macrophages (137). Studies looking at gene expression of macrophages in pathologic conditions have demonstrated heterogenous activation and functionality as well as significant overlap in gene expression whether stimulated with LPS or IL-10 (138–140). Further data showed macrophage functional pattern changes with duration of stimuli and that they are capable of completely changing their phenotype based on the surrounding microenvironment (135, 141, 142). Overall, data collectively support the idea that macrophages are dynamic, plastic, and capable of displaying multiple, distinct, functional patterns.
Chronic alcohol exposure impacts alveolar macrophage functionality and makes phenotyping the alveolar macrophage in the context of alcohol misuse a complex issue (134). At baseline, alveolar macrophages isolated from animal models of chronic alcohol ingestion have increased IL-13 and TGF-β1 production, both associated with suppression of inflammation, as well as decreased phagocytic ability (77, 78, 143). These findings are likely due, at least in part, to alcohol-induced oxidative stress in the alveolar environment (as discussed previously). However, when exposed to alcohol and PAMPs, such as LPS, alveolar macrophages exhibit an exaggerated inflammatory response. In studies utilizing AMs from subjects with AUD, alveolar macrophages produce increased proinflammatory cytokines, including TNFα, IFNγ, IL-1β, and IL-6, in response to LPS stimulation compared to persons without AUD (144–146). Despite this elevation in inflammatory signals, multiple studies note a persistent decrease in alveolar macrophage phagocytosis when exposed to bacterial pathogens or PAMPs (72, 76, 117). This overexuberant response to pathogen stimulation has been postulated to be a contributing factor in the elevated risk for disproportionate inflammatory states, like ARDS, in people with AUD (147). While some of these phenotypic changes are related to the alcohol-induced oxidative stress in the lung, chronic alcohol exposure also alters alveolar macrophage metabolism (76, 82). AUD been shown to impair LPS-induced glycolytic response and induce mitochondrial derangements in the alveolar macrophage which can alter its cytokine response and contribute to phagocytosis impairments (148–150).
Mtb has also been noted to induce phenotypic changes in the alveolar macrophage after infection. In the early stages of infection, Mtb induces a robust production of inflammatory cytokines, increased phagocytosis, and upregulation of glycolysis from the alveolar macrophage (151–155). Glycolysis is important to the immune response because glycolytic inhibition results in an increased mycobacterial burden (156). Once intracellular, Mtb itself attempts to evade the macrophage’s killing processes by secreting virulence factors that inhibit the alveolar macrophage’s expression of the nuclear factor-κB (NF-κB) and IFNγ, ultimately promoting Mtb’s intracellular survival (151, 157). Following the initial response to Mtb, alveolar macrophages increase production of anti-inflammatory cytokines, including IL-10 and TGF-β, with decreased glycolysis and increased oxidative phosphorylation and free fatty acid metabolism (151, 158–162). Successful treatment of TB leads to resolution of these phenotypic changes to the macrophage (163).
Further work is clearly necessary to fully understand the relationship between AUD, Mtb, and alveolar macrophage function. The quiescent, baseline state of alveolar macrophages in subjects with AUD with increased expression of TGF-β1 may provide more favorable conditions for Mtb infection and facilitate intracellular proliferation. Further, glycolysis is integral to the macrophage’s response to Mtb: a decreased glycolytic reserve has been associated with Mtb infection and risk factors for TB (156, 164). Alcohol’s impairments of LPS-induced glycolysis may contribute to alveolar macrophage dysfunction and increase the risk of Mtb infection in those with AUD.
4 Conclusion and Future Directions
Increasing rates of AUD pose a significant barrier to reaching the global goal of TB elimination. AUD is a risk factor for TB infection, severe disease, transmission, and associated death. Despite behavioral determinants of health linking AUD and TB disease, compelling evidence supports a biological impact of alcohol on TB risk and disease (Figure 2). The impact of alcohol on oxidative stress in the alveolar environment as well as impairments to the alveolar epithelium, alveolar macrophage, and remainder of the pulmonary innate immune system may facilitate Mtb infection and evasion of host defenses (Figure 3). To date, research directly investigating the mechanistic causes of TB in persons with AUD has been limited. Future investigations into the roles of innate immunity and oxidative stress specifically in alcohol and TB are needed. A better understanding of these causal pathways will lead to the development of host-directed therapies and better treatment outcomes in individuals with AUD and TB.
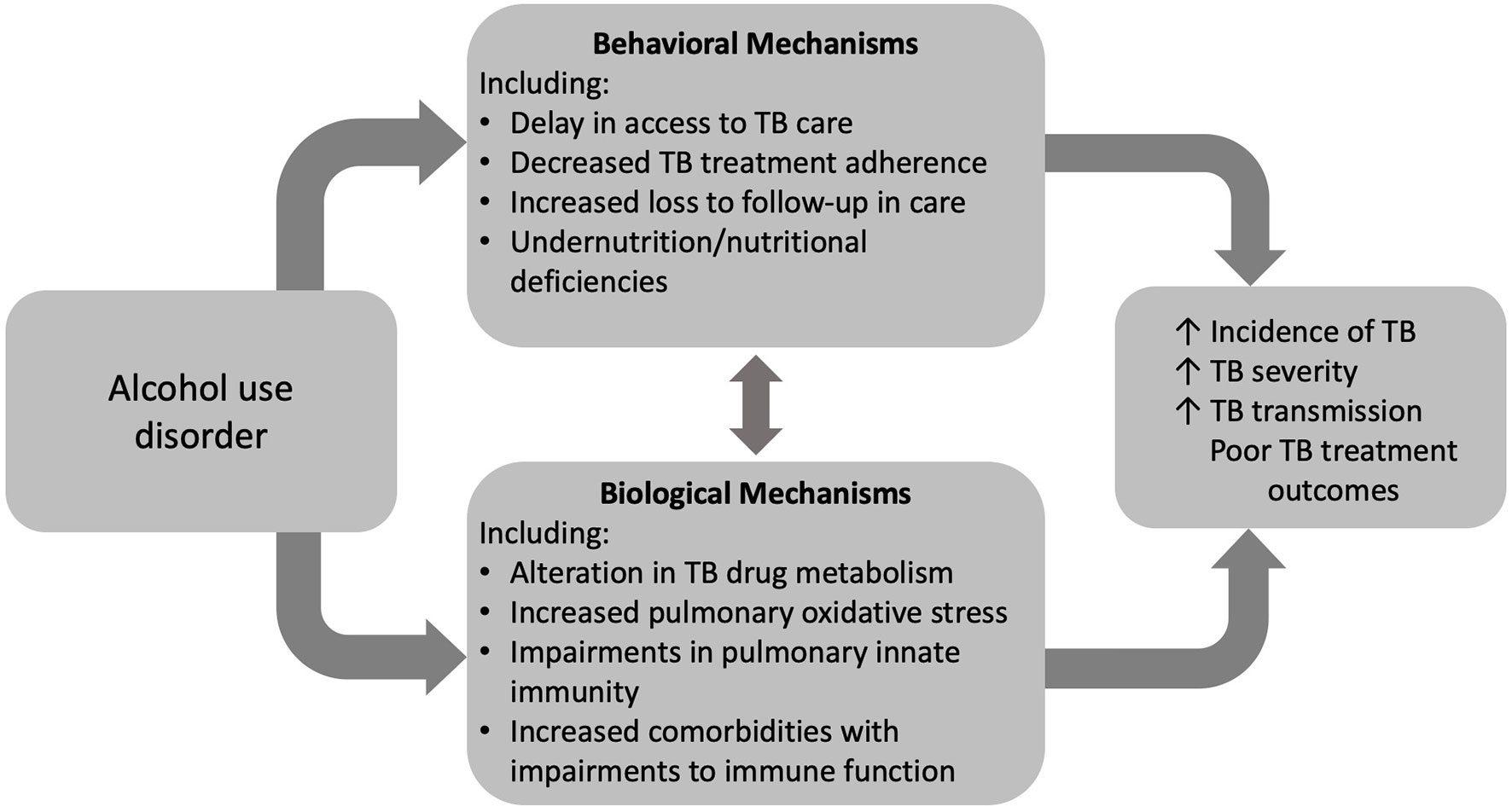
Figure 2 Alcohol use disorder (AUD) influences tuberculosis (TB) care and outcomes through both behavioral and biologic mechanisms.
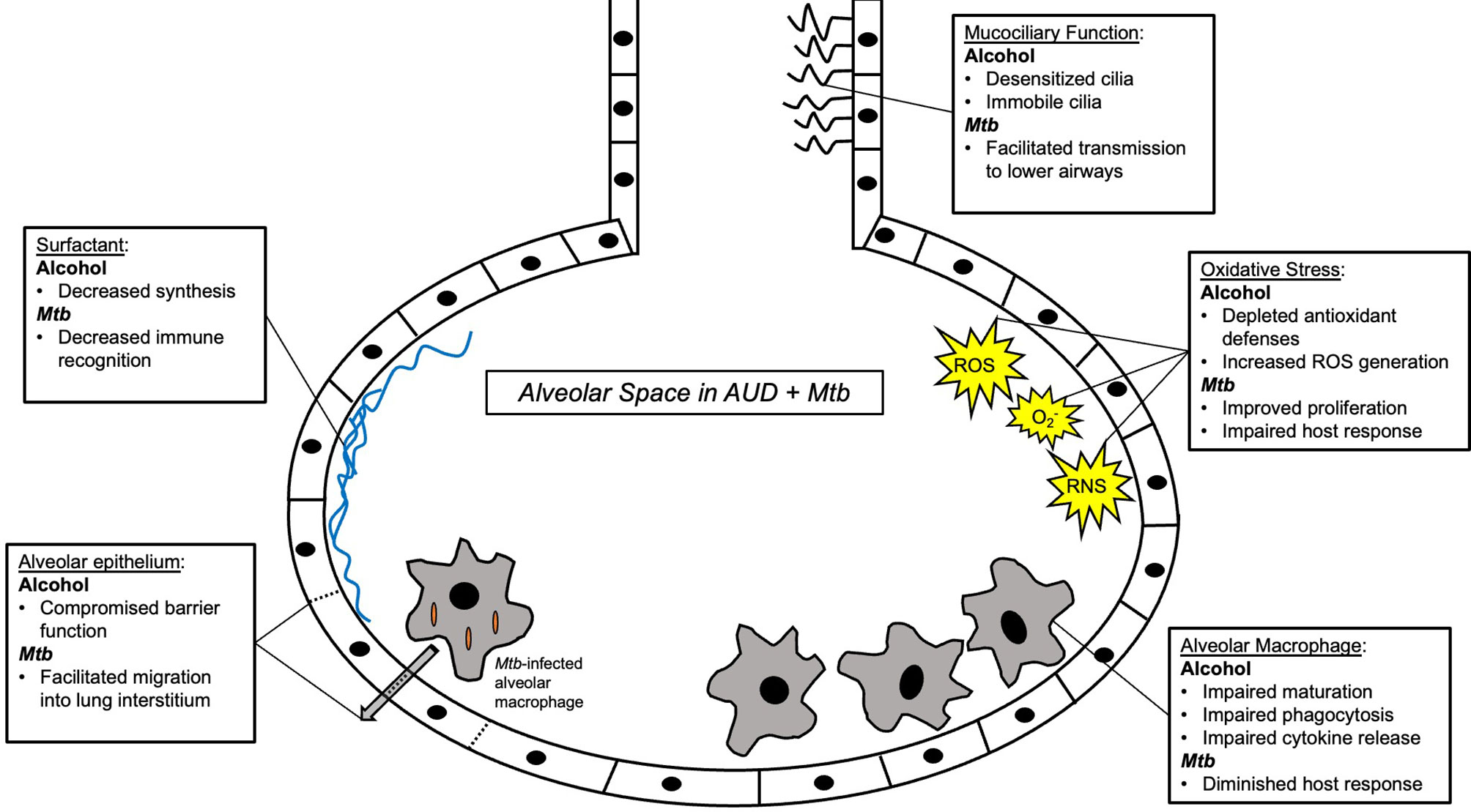
Figure 3 Summary schema of the alveolar space in alcohol use disorder (AUD) and Mycobacterium tuberculosis (Mtb) infection. Multiple components of innate immunity are affected by AUD, including macrophages, surfactant, the alveolar epithelium, and the alveolar oxidative state. See main text for further details.
Author Contributions
Design and conception - GW. Drafting and revising manuscript – GW, TB, KJ, SA, SY, and BS. All authors contributed to the article and approved the submitted version.
Funding
This study was funded by the National Heart, Lung, and Blood Institute [T32 HL116271], the National Institute of Allergy and Infectious Diseases (NIAID) [K23 AI152930; R01 AI119037; K23 AI134182], and the National Institute on Alcohol Abuse and Alcoholism (NIAAA) [R01 AA026086; K08 AA024512].
Conflict of Interest
The authors declare that the research was conducted in the absence of any commercial or financial relationships that could be construed as a potential conflict of interest.
Publisher’s Note
All claims expressed in this article are solely those of the authors and do not necessarily represent those of their affiliated organizations, or those of the publisher, the editors and the reviewers. Any product that may be evaluated in this article, or claim that may be made by its manufacturer, is not guaranteed or endorsed by the publisher.
References
1. WHO. Alcohol: Fact Sheet 2018. Available at: https://www.who.int/en/news-room/fact-sheets/detail/alcohol.
2. Alcohol GBD, Drug Use C. The Global Burden of Disease Attributable to Alcohol and Drug Use in 195 Countries and Territories, 1990-2016: A Systematic Analysis for the Global Burden of Disease Study 2016. Lancet Psychiatry (2018) 5(12):987–1012. doi: 10.1016/S2215-0366(18)30337-7
3. Rush B. An Inquiry Into the Effects of Ardent Spirits Upon the Human Body and Mind : With an Account of the Means of Preventing, and of the Remedies for Curing Them. 4th ed. Philadelphia: Printed for Thomas Dobson. Archibald Bartram (1805).
4. Moss M, Bucher B, Moore FA, Moore EE, Parsons PE. The Role of Chronic Alcohol Abuse in the Development of Acute Respiratory Distress Syndrome in Adults. JAMA (1996) 275(1):50–4. doi: 10.1001/jama.275.1.50
5. Moss M, Parsons PE, Steinberg KP, Hudson LD, Guidot DM, Burnham EL, et al. Chronic Alcohol Abuse is Associated With an Increased Incidence of Acute Respiratory Distress Syndrome and Severity of Multiple Organ Dysfunction in Patients With Septic Shock. Crit Care Med (2003) 31(3):869–77. doi: 10.1097/01.CCM.0000055389.64497.11
6. Happel KI, Nelson S. Alcohol, Immunosuppression, and the Lung. Proc Am Thorac Soc (2005) 2(5):428–32. doi: 10.1513/pats.200507-065JS
7. Mehta AJ, Guidot DM. Alcohol Abuse, the Alveolar Macrophage and Pneumonia. Am J Med Sci (2012) 343(3):244–7. doi: 10.1097/MAJ.0b013e31823ede77
8. Simou E, Britton J, Leonardi-Bee J. Alcohol and the Risk of Pneumonia: A Systematic Review and Meta-Analysis. BMJ Open (2018) 8(8):e022344. doi: 10.1136/bmjopen-2018-022344
9. Fernandez-Sola J, Junque A, Estruch R, Monforte R, Torres A, Urbano-Marquez A. High Alcohol Intake as a Risk and Prognostic Factor for Community-Acquired Pneumonia. Arch Intern Med (1995) 155(15):1649–54. doi: 10.1001/archinte.155.15.1649
10. Clark BJ, Williams A, Feemster LM, Bradley KA, Macht M, Moss M, et al. Alcohol Screening Scores and 90-Day Outcomes in Patients With Acute Lung Injury. Crit Care Med (2013) 41(6):1518–25. doi: 10.1097/CCM.0b013e318287f1bb
11. O'Brien JM Jr, Lu B, Ali NA, Martin GS, Aberegg SK, Marsh CB, et al. Alcohol Dependence is Independently Associated With Sepsis, Septic Shock, and Hospital Mortality Among Adult Intensive Care Unit Patients. Crit Care Med (2007) 35(2):345–50. doi: 10.1097/01.CCM.0000254340.91644.B2
12. Chalmers JD, Singanayagam A, Murray MP, Scally C, Fawzi A, Hill AT. Risk Factors for Complicated Parapneumonic Effusion and Empyema on Presentation to Hospital With Community-Acquired Pneumonia. Thorax (2009) 64(7):592–7. doi: 10.1136/thx.2008.105080
13. Gentile JH, Sparo MD, Mercapide ME, Luna CM. Adult Bacteremic Pneumococcal Pneumonia Acquired in the Community. A Prospective Study on 101 Patients. Medicina (B Aires) (2003) 63(1):9–14.
14. Plevneshi A, Svoboda T, Armstrong I, Tyrrell GJ, Miranda A, Green K, et al. Population-Based Surveillance for Invasive Pneumococcal Disease in Homeless Adults in Toronto. PloS One (2009) 4(9):e7255. doi: 10.1371/journal.pone.0007255
16. Osler W. The Principles and Practice of Medicine, Designed for the Use of Practitioners and Students of Medicine. 3d ed. New York: D. Appleton and company (1898). p. xvii, 1 , 1181.
17. Puryear SB, Fatch R, Beesiga B, Kekibiina A, Lodi S, Marson K, et al. Higher Levels of Alcohol Use Are Associated With Latent Tuberculosis Infection in Adults Living With Human Immunodeficiency Virus. Clin Infect Dis (2021) 72(5):865–8. doi: 10.1093/cid/ciaa527
18. Friedman LN, Sullivan GM, Bevilaqua RP, Loscos R. Tuberculosis Screening in Alcoholics and Drug Addicts. Am Rev Respir Dis (1987) 136(5):1188–92. doi: 10.1164/ajrccm/136.5.1188
19. Francisco J, Oliveira O, Felgueiras O, Gaio AR, Duarte R. How Much is Too Much Alcohol in Tuberculosis? Eur Respir J (2017) 49(1):1–4. doi: 10.1183/13993003.01468-2016
20. Imtiaz S, Shield KD, Roerecke M, Samokhvalov AV, Lonnroth K, Rehm J. Alcohol Consumption as a Risk Factor for Tuberculosis: Meta-Analyses and Burden of Disease. Eur Respir J (2017) 50(1):1–13. doi: 10.1183/13993003.00216-2017
21. Fan CY, Katsuyama M, Yabe-Nishimura C. PKCdelta Mediates Up-Regulation of NOX1, a Catalytic Subunit of NADPH Oxidase, via Transactivation of the EGF Receptor: Possible Involvement of PKCdelta in Vascular Hypertrophy. Biochem J (2005) 390(Pt 3):761–7. doi: 10.1042/BJ20050287
22. Collaborators GBDA. Alcohol Use and Burden for 195 Countries and Territories, 1990-2016: A Systematic Analysis for the Global Burden of Disease Study 2016. Lancet (2018) 392(10152):1015–35. doi: 10.1016/S0140-6736(18)31310-2
23. Collaborators GBDT. Global, Regional, and National Sex Differences in the Global Burden of Tuberculosis by HIV Status, 1990-2019: Results From the Global Burden of Disease Study 2019. Lancet Infect Dis (2021) 22(2):222–41. doi: 10.1016/S1473-3099(21)00449-7
24. Rehm J, Samokhvalov AV, Neuman MG, Room R, Parry C, Lonnroth K, et al. The Association Between Alcohol Use, Alcohol Use Disorders and Tuberculosis (TB). A Systematic Review. BMC Public Health (2009) 9:450. doi: 10.1186/1471-2458-9-450
25. Volkmann T, Moonan PK, Miramontes R, Oeltmann JE. Tuberculosis and Excess Alcohol Use in the United States, 1997-2012. Int J Tuberc Lung Dis (2015) 19(1):111–9. doi: 10.5588/ijtld.14.0516
26. Fiske CT, Hamilton CD, Stout JE. Alcohol Use and Clinical Manifestations of Tuberculosis. J Infect (2009) 58(5):395–401. doi: 10.1016/j.jinf.2009.02.015
27. Lonnroth K, Williams BG, Stadlin S, Jaramillo E, Dye C. Alcohol Use as a Risk Factor for Tuberculosis - a Systematic Review. BMC Public Health (2008) 8:289. doi: 10.1186/1471-2458-8-289
28. Ragan EJ, Kleinman MB, Sweigart B, Gnatienko N, Parry CD, Horsburgh CR, et al. The Impact of Alcohol Use on Tuberculosis Treatment Outcomes: A Systematic Review and Meta-Analysis. Int J Tuberc Lung Dis (2020) 24(1):73–82. doi: 10.5588/ijtld.19.0080
29. Kline SE, Hedemark LL, Davies SF. Outbreak of Tuberculosis Among Regular Patrons of a Neighborhood Bar. N Engl J Med (1995) 333(4):222–7. doi: 10.1056/NEJM199507273330404
30. Diel R, Meywald-Walter K, Gottschalk R, Rusch-Gerdes S, Niemann S. Ongoing Outbreak of Tuberculosis in a Low-Incidence Community: A Molecular-Epidemiological Evaluation. Int J Tuberc Lung Dis (2004) 8(7):855–61.
31. Classen CN, Warren R, Richardson M, Hauman JH, Gie RP, Ellis JH, et al. Impact of Social Interactions in the Community on the Transmission of Tuberculosis in a High Incidence Area. Thorax (1999) 54(2):136–40. doi: 10.1136/thx.54.2.136
32. Nava-Aguilera E, Andersson N, Harris E, Mitchell S, Hamel C, Shea B, et al. Risk Factors Associated With Recent Transmission of Tuberculosis: Systematic Review and Meta-Analysis. Int J Tuberc Lung Dis (2009) 13(1):17–26.
33. Fok A, Numata Y, Schulzer M, FitzGerald MJ. Risk Factors for Clustering of Tuberculosis Cases: A Systematic Review of Population-Based Molecular Epidemiology Studies. Int J Tuberc Lung Dis (2008) 12(5):480–92.
34. Lohmann EM, Koster BF, le Cessie S, Kamst-van Agterveld MP, van Soolingen D, Arend SM. Grading of a Positive Sputum Smear and the Risk of Mycobacterium Tuberculosis Transmission. Int J Tuberc Lung Dis (2012) 16(11):1477–84. doi: 10.5588/ijtld.12.0129
35. Wang H, Hosford J, Lauzardo M. The Effect of Alcohol Use on the Clinical Presentation of Tuberculosis. J Mycobac Dis (2012) 2(5):2–5. doi: 10.4172/2161-1068.1000123
36. Palaci M, Dietze R, Hadad DJ, Ribeiro FK, Peres RL, Vinhas SA, et al. Cavitary Disease and Quantitative Sputum Bacillary Load in Cases of Pulmonary Tuberculosis. J Clin Microbiol (2007) 45(12):4064–6. doi: 10.1128/JCM.01780-07
37. Kan CK, Ragan EJ, Sarkar S, Knudsen S, Forsyth M, Muthuraj M, et al. Alcohol Use and Tuberculosis Clinical Presentation at the Time of Diagnosis in Puducherry and Tamil Nadu, India. PloS One (2020) 15(12):e0240595. doi: 10.1371/journal.pone.0240595
38. Przybylski G, Dabrowska A, Trzcinska H. Alcoholism and Other Socio-Demographic Risk Factors for Adverse TB-Drug Reactions and Unsuccessful Tuberculosis Treatment - Data From Ten Years' Observation at the Regional Centre of Pulmonology, Bydgoszcz, Poland. Med Sci Monit (2014) 20:444–53. doi: 10.12659/MSM.890012
39. Shin SS, Pasechnikov AD, Gelmanova IY, Peremitin GG, Strelis AK, Mishustin S, et al. Treatment Outcomes in an Integrated Civilian and Prison MDR-TB Treatment Program in Russia. Int J Tuberc Lung Dis (2006) 10(4):402–8.
40. de Albuquerque Mde F, Ximenes RA, Lucena-Silva N, de Souza WV, Dantas AT, Dantas OM, et al. Factors Associated With Treatment Failure, Dropout, and Death in a Cohort of Tuberculosis Patients in Recife, Pernambuco State, Brazil. Cad Saude Publica (2007) 23(7):1573–82. doi: 10.1590/S0102-311X2007000700008
41. Thomas BE, Thiruvengadam K SR, Kadam D, Ovung S, Sivakumar S, Bala Yogendra Shivakumar SV, et al. Smoking, Alcohol Use Disorder and Tuberculosis Treatment Outcomes: A Dual Co-Morbidity Burden That Cannot be Ignored. PloS One (2019) 14(7):e0220507. doi: 10.1371/journal.pone.0220507
42. Duraisamy K, Mrithyunjayan S, Ghosh S, Nair SA, Balakrishnan S, Subramoniapillai J, et al. Does Alcohol Consumption During Multidrug-Resistant Tuberculosis Treatment Affect Outcome? A Population-Based Study in Kerala, India. Ann Am Thorac Soc (2014) 11(5):712–8. doi: 10.1513/AnnalsATS.201312-447OC
43. Fleming MF, Krupitsky E, Tsoy M, Zvartau E, Brazhenko N, Jakubowiak W, et al. Alcohol and Drug Use Disorders, HIV Status and Drug Resistance in a Sample of Russian TB Patients. Int J Tuberc Lung Dis (2006) 10(5):565–70.
44. Storla DG, Yimer S, Bjune GA. A Systematic Review of Delay in the Diagnosis and Treatment of Tuberculosis. BMC Public Health (2008) 8:15. doi: 10.1186/1471-2458-8-15
45. Moro RN, Borisov AS, Saukkonen J, Khan A, Sterling TR, Villarino ME, et al. Factors Associated With Noncompletion of Latent Tuberculosis Infection Treatment: Experience From the PREVENT TB Trial in the United States and Canada. Clin Infect Dis (2016) 62(11):1390–400. doi: 10.1093/cid/ciw126
46. Lasebikan VO, Ige OM. Alcohol Use Disorders in Multidrug Resistant Tuberculosis (MDR-TB) Patients and Their non-Tuberculosis Family Contacts in Nigeria. Pan Afr Med J (2020) 36:321. doi: 10.11604/pamj.2020.36.321.17118
47. Ignatyeva O, Balabanova Y, Nikolayevskyy V, Koshkarova E, Radiulyte B, Davidaviciene E, et al. Resistance Profile and Risk Factors of Drug Resistant Tuberculosis in the Baltic Countries. Tuberculosis (Edinb) (2015) 95(5):581–8. doi: 10.1016/j.tube.2015.05.018
48. Mathew TA, Ovsyanikova TN, Shin SS, Gelmanova I, Balbuena DA, Atwood S, et al. Causes of Death During Tuberculosis Treatment in Tomsk Oblast, Russia. Int J Tuberc Lung Dis (2006) 10(8):857–63.
49. Kattan JA, Sosa LE, Lobato MN. Tuberculosis Mortality: Death From a Curable Disease, Connecticut, 2007-2009. Int J Tuberc Lung Dis (2012) 16(12):1657–62. doi: 10.5588/ijtld.12.0169
50. Olsen H, Morland J. Ethanol-Induced Increase in Drug Acetylation in Man and Isolated Rat Liver Cells. Br Med J (1978) 2(6147):1260–2. doi: 10.1136/bmj.2.6147.1260
51. Koriakin VA, Sokolova GB, Grinchar NA, Iurchenko LN. Pharmacokinetics of Isoniazid in Patients With Pulmonary Tuberculosis and Alcoholism. Probl Tuberk (1986) 12):43–6.
52. Ortenberg ZA. Pharmacokinetics of Isoniazid, PAS, and Cycloserin in Acute and Chronic Alcoholic Intoxication (Experimental Study). Probl Tuberk (1978) 9):60–4.
53. Lester D. The Acetylation of Isoniazid in Alcoholics. Q J Stud Alcohol (1964) 25:541–3. doi: 10.15288/qjsa.1964.25.541
54. Thomas BH, Solomonraj G. Drug Interactions With Isoniazid Metabolism in Rats. J Pharm Sci (1977) 66(9):1322–6. doi: 10.1002/jps.2600660930
55. Wilcke JT, Dossing M, Angelo HR, Askgaard D, Ronn A, Christensen HR. Unchanged Acetylation of Isoniazid by Alcohol Intake. Int J Tuberc Lung Dis (2004) 8(11):1373–6.
56. Kimerling ME, Phillips P, Patterson P, Hall M, Robinson CA, Dunlap NE. Low Serum Antimycobacterial Drug Levels in non-HIV-Infected Tuberculosis Patients. Chest (1998) 113(5):1178–83. doi: 10.1378/chest.113.5.1178
57. Ferrando R, Garrigues TM, Bermejo MV, Martin-Algarra R, Merino V, Polache A. Effects of Ethanol on Intestinal Absorption of Drugs: In Situ Studies With Ciprofloxacin Analogs in Acute and Chronic Alcohol-Fed Rats. Alcohol Clin Exp Res (1999) 23(8):1403–8. doi: 10.1111/j.1530-0277.1999.tb04363.x
58. Merino V, Martin-Algarra RV, Rocher A, Garrigues TM, Freixas J, Polache A. Effects of Ethanol on Intestinal Absorption of Drugs. I. In Situ Studies With Ciprofloxacin Analogs in Normal and Chronic Alcohol-Fed Rats. Alcohol Clin Exp Res (1997) 21(2):326–33. doi: 10.1111/j.1530-0277.1997.tb03768.x
59. Pasipanodya JG, McIlleron H, Burger A, Wash PA, Smith P, Gumbo T. Serum Drug Concentrations Predictive of Pulmonary Tuberculosis Outcomes. J Infect Dis (2013) 208(9):1464–73. doi: 10.1093/infdis/jit352
60. Hahn J. Alcohol Drinkers' Exposure to Preventive Therapy for TB (ADEPTT). Identifier NCT0330229. (2017). Available at: https://clinicaltrials.gov/ct2/show/NCT03302299.
61. Thomas B, Suhadev M, Mani J, Ganapathy BG, Armugam A, Faizunnisha F, et al. Feasibility of an Alcohol Intervention Programme for TB Patients With Alcohol Use Disorder (AUD)–a Qualitative Study From Chennai, South India. PloS One (2011) 6(11):e27752. doi: 10.1371/journal.pone.0027752
62. Thomas B, Watson B, Senthil EK, Deepalakshmi A, Balaji G, Chandra S, et al. Alcohol Intervention Strategy Among Tuberculosis Patients: A Pilot Study From South India. Int J Tuberc Lung Dis (2017) 21(8):947–52. doi: 10.5588/ijtld.16.0693
63. Moriarty-Craige SE, Jones DP. Extracellular Thiols and Thiol/Disulfide Redox in Metabolism. Annu Rev Nutr (2004) 24:481–509. doi: 10.1146/annurev.nutr.24.012003.132208
64. Rahman I, Yang SR, Biswas SK. Current Concepts of Redox Signaling in the Lungs. Antioxid Redox Signal (2006) 8(3-4):681–9. doi: 10.1089/ars.2006.8.681
65. Go YM, Jones DP. Thiol/disulfide Redox States in Signaling and Sensing. Crit Rev Biochem Mol Biol (2013) 48(2):173–81. doi: 10.3109/10409238.2013.764840
66. Moss M, Guidot DM, Wong-Lambertina M, Ten Hoor T, Perez RL, Brown LA. The Effects of Chronic Alcohol Abuse on Pulmonary Glutathione Homeostasis. Am J Respir Crit Care Med (2000) 161(2 Pt 1):414–9. doi: 10.1164/ajrccm.161.2.9905002
67. Rahman I, MacNee W. Oxidative Stress and Regulation of Glutathione in Lung Inflammation. Eur Respir J (2000) 16(3):534–54. doi: 10.1034/j.1399-3003.2000.016003534.x
68. Liang Y, Yeligar SM, Brown LA. Chronic-Alcohol-Abuse-Induced Oxidative Stress in the Development of Acute Respiratory Distress Syndrome. Scientific World Journal (2012) 2012:740308. doi: 10.1100/2012/740308
69. Holguin F, Moss I, Brown LA, Guidot DM. Chronic Ethanol Ingestion Impairs Alveolar Type II Cell Glutathione Homeostasis and Function and Predisposes to Endotoxin-Mediated Acute Edematous Lung Injury in Rats. J Clin Invest (1998) 101(4):761–8. doi: 10.1172/JCI1396
70. Velasquez A, Bechara RI, Lewis JF, Malloy J, McCaig L, Brown LA, et al. Glutathione Replacement Preserves the Functional Surfactant Phospholipid Pool Size and Decreases Sepsis-Mediated Lung Dysfunction in Ethanol-Fed Rats. Alcohol Clin Exp Res (2002) 26(8):1245–51. doi: 10.1111/j.1530-0277.2002.tb02663.x
71. Liang Y, Harris FL, Brown LA. Alcohol Induced Mitochondrial Oxidative Stress and Alveolar Macrophage Dysfunction. BioMed Res Int (2014) 2014:371593. doi: 10.1155/2014/371593
72. Yeligar SM, Harris FL, Hart CM, Brown LA. Ethanol Induces Oxidative Stress in Alveolar Macrophages via Upregulation of NADPH Oxidases. J Immunol (2012) 188(8):3648–57. doi: 10.4049/jimmunol.1101278
73. Wagner MC, Yeligar SM, Brown LA, Michael Hart C. PPARgamma Ligands Regulate NADPH Oxidase, eNOS, and Barrier Function in the Lung Following Chronic Alcohol Ingestion. Alcohol Clin Exp Res (2012) 36(2):197–206. doi: 10.1111/j.1530-0277.2011.01599.x
74. Jensen JS, Fan X, Guidot DM. Alcohol Causes Alveolar Epithelial Oxidative Stress by Inhibiting the Nuclear Factor (Erythroid-Derived 2)-Like 2-Antioxidant Response Element Signaling Pathway. Am J Respir Cell Mol Biol (2013) 48(4):511–7. doi: 10.1165/rcmb.2012-0334OC
75. Polikandriotis JA, Rupnow HL, Elms SC, Clempus RE, Campbell DJ, Sutliff RL, et al. Chronic Ethanol Ingestion Increases Superoxide Production and NADPH Oxidase Expression in the Lung. Am J Respir Cell Mol Biol (2006) 34(3):314–9. doi: 10.1165/rcmb.2005-0320OC
76. Yeligar SM, Mehta AJ, Harris FL, Brown LA, Hart CM. Peroxisome Proliferator-Activated Receptor Gamma Regulates Chronic Alcohol-Induced Alveolar Macrophage Dysfunction. Am J Respir Cell Mol Biol (2016) 55(1):35–46. doi: 10.1165/rcmb.2015-0077OC
77. Bechara RI, Brown LA, Roman J, Joshi PC, Guidot DM. Transforming Growth Factor Beta1 Expression and Activation is Increased in the Alcoholic Rat Lung. Am J Respir Crit Care Med (2004) 170(2):188–94. doi: 10.1164/rccm.200304-478OC
78. Brown SD, Brown LA. Ethanol (EtOH)-Induced TGF-Beta1 and Reactive Oxygen Species Production are Necessary for EtOH-Induced Alveolar Macrophage Dysfunction and Induction of Alternative Activation. Alcohol Clin Exp Res (2012) 36(11):1952–62. doi: 10.1111/j.1530-0277.2012.01825.x
79. Guidot DM, Modelska K, Lois M, Jain L, Moss IM, Pittet JF, et al. Ethanol Ingestion via Glutathione Depletion Impairs Alveolar Epithelial Barrier Function in Rats. Am J Physiol Lung Cell Mol Physiol (2000) 279(1):L127–35. doi: 10.1152/ajplung.2000.279.1.L127
80. Brown LA, Harris FL, Bechara R, Guidot DM. Effect of Chronic Ethanol Ingestion on Alveolar Type II Cell: Glutathione and Inflammatory Mediator-Induced Apoptosis. Alcohol Clin Exp Res (2001) 25(7):1078–85. doi: 10.1111/j.1530-0277.2001.tb02320.x
81. Brown LA, Ping XD, Harris FL, Gauthier TW. Glutathione Availability Modulates Alveolar Macrophage Function in the Chronic Ethanol-Fed Rat. Am J Physiol Lung Cell Mol Physiol (2007) 292(4):L824–32. doi: 10.1152/ajplung.00346.2006
82. Yeligar SM, Harris FL, Hart CM, Brown LA. Glutathione Attenuates Ethanol-Induced Alveolar Macrophage Oxidative Stress and Dysfunction by Downregulating NADPH Oxidases. Am J Physiol Lung Cell Mol Physiol (2014) 306(5):L429–41. doi: 10.1152/ajplung.00159.2013
83. Demir E, Giden R, Sak ZHA, Demir Giden Z. Thiol-Disulphide Homoeostasis as a Novel Oxidative Stress Biomarker in Lung Tuberculosis Patient. Int J Clin Pract (2021) 75(5):e13998. doi: 10.1111/ijcp.13998
84. Palanisamy GS, Kirk NM, Ackart DF, Shanley CA, Orme IM, Basaraba RJ. Evidence for Oxidative Stress and Defective Antioxidant Response in Guinea Pigs With Tuberculosis. PloS One (2011) 6(10):e26254. doi: 10.1371/journal.pone.0026254
85. Amaral EP, Conceicao EL, Costa DL, Rocha MS, Marinho JM, Cordeiro-Santos M, et al. N-Acetyl-Cysteine Exhibits Potent Anti-Mycobacterial Activity in Addition to its Known Anti-Oxidative Functions. BMC Microbiol (2016) 16(1):251. doi: 10.1186/s12866-016-0872-7
86. Oberley-Deegan RE, Rebits BW, Weaver MR, Tollefson AK, Bai X, McGibney M, et al. An Oxidative Environment Promotes Growth of Mycobacterium Abscessus. Free Radic Biol Med (2010) 49(11):1666–73. doi: 10.1016/j.freeradbiomed.2010.08.026
87. Rothchild AC, Olson GS, Nemeth J, Amon LM, Mai D, Gold ES, et al. Alveolar Macrophages Generate a Noncanonical NRF2-Driven Transcriptional Response to Mycobacterium Tuberculosis In Vivo. Sci Immunol (2019) 4(37):1–14. doi: 10.1126/sciimmunol.aaw6693
88. Rockwood N, Costa DL, Amaral EP, Du Bruyn E, Kubler A, Gil-Santana L, et al. Mycobacterium Tuberculosis Induction of Heme Oxygenase-1 Expression Is Dependent on Oxidative Stress and Reflects Treatment Outcomes. Front Immunol (2017) 8:542. doi: 10.3389/fimmu.2017.00542
89. Sun Q, Shen X, Ma J, Lou H, Zhang Q. Activation of Nrf2 Signaling by Oltipraz Inhibits Death of Human Macrophages With Mycobacterium Tuberculosis Infection. Biochem Biophys Res Commun (2020) 531(3):312–9. doi: 10.1016/j.bbrc.2020.07.026
90. Meylan PR, Richman DD, Kornbluth RS. Reduced Intracellular Growth of Mycobacteria in Human Macrophages Cultivated at Physiologic Oxygen Pressure. Am Rev Respir Dis (1992) 145(4 Pt 1):947–53. doi: 10.1164/ajrccm/145.4_Pt_1.947
91. Chaudhuri N, Sabroe I. Basic Science of the Innate Immune System and the Lung. Paediatr Respir Rev (2008) 9(4):236–42. doi: 10.1016/j.prrv.2008.03.002
92. Zhang P, Bagby GJ, Happel KI, Summer WR, Nelson S. Pulmonary Host Defenses and Alcohol. Front Biosci (2002) 7:d1314–30. doi: 10.2741/A842
93. Kany S, Janicova A, Relja B. Innate Immunity and Alcohol. J Clin Med (2019) 8(11):1–31. doi: 10.3390/jcm8111981
94. Hallengren B, Forsgren A. Effect of Alcohol on Chemotaxis, Adherence and Phagocytosis of Human Polymorphonuclear Leucocytes. Acta Med Scand (1978) 204(1-2):43–8. doi: 10.1111/j.0954-6820.1978.tb08396.x
95. Boe DM, Nelson S, Zhang P, Bagby GJ. Acute Ethanol Intoxication Suppresses Lung Chemokine Production Following Infection With Streptococcus Pneumoniae. J Infect Dis (2001) 184(9):1134–42. doi: 10.1086/323661
96. Malacco N, Souza JAM, Martins FRB, Rachid MA, Simplicio JA, Tirapelli CR, et al. Chronic Ethanol Consumption Compromises Neutrophil Function in Acute Pulmonary Aspergillus Fumigatus Infection. Elife (2020) 9:1–23. doi: 10.7554/eLife.58855
97. Laso FJ, Vaquero JM, Almeida J, Marcos M, Orfao A. Chronic Alcohol Consumption is Associated With Changes in the Distribution, Immunophenotype, and the Inflammatory Cytokine Secretion Profile of Circulating Dendritic Cells. Alcohol Clin Exp Res (2007) 31(5):846–54. doi: 10.1111/j.1530-0277.2007.00377.x
98. Szabo G, Catalano D, White B, Mandrekar P. Acute Alcohol Consumption Inhibits Accessory Cell Function of Monocytes and Dendritic Cells. Alcohol Clin Exp Res (2004) 28(5):824–8. doi: 10.1097/01.ALC.0000127104.80398.9B
99. Wyatt TA, Gentry-Nielsen MJ, Pavlik JA, Sisson JH. Desensitization of PKA-Stimulated Ciliary Beat Frequency in an Ethanol-Fed Rat Model of Cigarette Smoke Exposure. Alcohol Clin Exp Res (2004) 28(7):998–1004. doi: 10.1097/01.ALC.0000130805.75641.F4
100. Wyatt TA, Sisson JH. Chronic Ethanol Downregulates PKA Activation and Ciliary Beating in Bovine Bronchial Epithelial Cells. Am J Physiol Lung Cell Mol Physiol (2001) 281(3):L575–81. doi: 10.1152/ajplung.2001.281.3.L575
101. Price ME, Case AJ, Pavlik JA, DeVasure JM, Wyatt TA, Zimmerman MC, et al. S-Nitrosation of Protein Phosphatase 1 Mediates Alcohol-Induced Ciliary Dysfunction. Sci Rep (2018) 8(1):9701. doi: 10.1038/s41598-018-27924-x
102. Torrelles JB, Schlesinger LS. Integrating Lung Physiology, Immunology, and Tuberculosis. Trends Microbiol (2017) 25(8):688–97. doi: 10.1016/j.tim.2017.03.007
103. Noone PG, Leigh MW, Sannuti A, Minnix SL, Carson JL, Hazucha M, et al. Primary Ciliary Dyskinesia: Diagnostic and Phenotypic Features. Am J Respir Crit Care Med (2004) 169(4):459–67. doi: 10.1164/rccm.200303-365OC
104. Schneeberger EE, Lynch RD. Structure, Function, and Regulation of Cellular Tight Junctions. Am J Physiol (1992) 262(6 Pt 1):L647–61. doi: 10.1152/ajplung.1992.262.6.L647
105. Fernandez AL, Koval M, Fan X, Guidot DM. Chronic Alcohol Ingestion Alters Claudin Expression in the Alveolar Epithelium of Rats. Alcohol (2007) 41(5):371–9. doi: 10.1016/j.alcohol.2007.04.010
106. Koval M. Claudin Heterogeneity and Control of Lung Tight Junctions. Annu Rev Physiol (2013) 75:551–67. doi: 10.1146/annurev-physiol-030212-183809
107. Schlingmann B, Overgaard CE, Molina SA, Lynn KS, Mitchell LA, Dorsainvil White S, et al. Regulation of Claudin/Zonula Occludens-1 Complexes by Hetero-Claudin Interactions. Nat Commun (2016) 7:12276. doi: 10.1038/ncomms12276
108. Burnham EL, Halkar R, Burks M, Moss M. The Effects of Alcohol Abuse on Pulmonary Alveolar-Capillary Barrier Function in Humans. Alcohol Alcohol (2009) 44(1):8–12. doi: 10.1093/alcalc/agn051
109. Berkowitz DM, Danai PA, Eaton S, Moss M, Martin GS. Alcohol Abuse Enhances Pulmonary Edema in Acute Respiratory Distress Syndrome. Alcohol Clin Exp Res (2009) 33(10):1690–6. doi: 10.1111/j.1530-0277.2009.01005.x
110. Pelaez A, Bechara RI, Joshi PC, Brown LA, Guidot DM. Granulocyte/macrophage Colony-Stimulating Factor Treatment Improves Alveolar Epithelial Barrier Function in Alcoholic Rat Lung. Am J Physiol Lung Cell Mol Physiol (2004) 286(1):L106–11. doi: 10.1152/ajplung.00148.2003
111. Overgaard CE, Schlingmann B, Dorsainvil White S, Ward C, Fan X, Swarnakar S, et al. The Relative Balance of GM-CSF and TGF-Beta1 Regulates Lung Epithelial Barrier Function. Am J Physiol Lung Cell Mol Physiol (2015) 308(12):L1212–23. doi: 10.1152/ajplung.00042.2014
112. Guidot DM, Brown LA. Mitochondrial Glutathione Replacement Restores Surfactant Synthesis and Secretion in Alveolar Epithelial Cells of Ethanol-Fed Rats. Alcohol Clin Exp Res (2000) 24(7):1070–6. doi: 10.1111/j.1530-0277.2000.tb04652.x
113. Wright JR, Borron P, Brinker KG, Folz RJ. Surfactant Protein A: Regulation of Innate and Adaptive Immune Responses in Lung Inflammation. Am J Respir Cell Mol Biol (2001) 24(5):513–7. doi: 10.1165/ajrcmb.24.5.f208
114. Ferguson JS, Schlesinger LS. Pulmonary Surfactant in Innate Immunity and the Pathogenesis of Tuberculosis. Tuber Lung Dis (2000) 80(4-5):173–84. doi: 10.1054/tuld.2000.0242
115. Pai M, Behr MA, Dowdy D, Dheda K, Divangahi M, Boehme CC, et al. Tuberculosis. Nat Rev Dis Primers (2016) 2:16076. doi: 10.1038/nrdp.2016.76
116. Cohen SB, Gern BH, Delahaye JL, Adams KN, Plumlee CR, Winkler JK, et al. Alveolar Macrophages Provide an Early Mycobacterium Tuberculosis Niche and Initiate Dissemination. Cell Host Microbe (2018) 24(3):439–46 e4. doi: 10.1016/j.chom.2018.08.001
117. Brown SD, Gauthier TW, Brown LA. Impaired Terminal Differentiation of Pulmonary Macrophages in a Guinea Pig Model of Chronic Ethanol Ingestion. Alcohol Clin Exp Res (2009) 33(10):1782–93. doi: 10.1111/j.1530-0277.2009.01017.x
118. Joshi PC, Applewhite L, Ritzenthaler JD, Roman J, Fernandez AL, Eaton DC, et al. Chronic Ethanol Ingestion in Rats Decreases Granulocyte-Macrophage Colony-Stimulating Factor Receptor Expression and Downstream Signaling in the Alveolar Macrophage. J Immunol (2005) 175(10):6837–45. doi: 10.4049/jimmunol.175.10.6837
119. Joshi PC, Applewhite L, Mitchell PO, Fernainy K, Roman J, Eaton DC, et al. GM-CSF Receptor Expression and Signaling is Decreased in Lungs of Ethanol-Fed Rats. Am J Physiol Lung Cell Mol Physiol (2006) 291(6):L1150–8. doi: 10.1152/ajplung.00150.2006
120. Joshi PC, Mehta A, Jabber WS, Fan X, Guidot DM. Zinc Deficiency Mediates Alcohol-Induced Alveolar Epithelial and Macrophage Dysfunction in Rats. Am J Respir Cell Mol Biol (2009) 41(2):207–16. doi: 10.1165/rcmb.2008-0209OC
121. Shibata Y, Berclaz PY, Chroneos ZC, Yoshida M, Whitsett JA, Trapnell BC. GM-CSF Regulates Alveolar Macrophage Differentiation and Innate Immunity in the Lung Through PU.1. Immunity (2001) 15(4):557–67. doi: 10.1016/S1074-7613(01)00218-7
122. Lloberas J, Soler C, Celada A. The Key Role of PU.1/SPI-1 in B Cells, Myeloid Cells and Macrophages. Immunol Today (1999) 20(4):184–9. doi: 10.1016/S0167-5699(99)01442-5
123. Staitieh BS, Fan X, Neveu W, Guidot DM. Nrf2 Regulates PU.1 Expression and Activity in the Alveolar Macrophage. Am J Physiol Lung Cell Mol Physiol (2015) 308(10):L1086–93. doi: 10.1152/ajplung.00355.2014
124. Russell DG. Mycobacterium Tuberculosis and the Intimate Discourse of a Chronic Infection. Immunol Rev (2011) 240(1):252–68. doi: 10.1111/j.1600-065X.2010.00984.x
125. Bryson BD, Rosebrock TR, Tafesse FG, Itoh CY, Nibasumba A, Babunovic GH, et al. Heterogeneous GM-CSF Signaling in Macrophages is Associated With Control of Mycobacterium Tuberculosis. Nat Commun (2019) 10(1):2329. doi: 10.1038/s41467-019-10065-8
126. Mishra A, Singh VK, Actor JK, Hunter RL, Jagannath C, Subbian S, et al. GM-CSF Dependent Differential Control of Mycobacterium Tuberculosis Infection in Human and Mouse Macrophages: Is Macrophage Source of GM-CSF Critical to Tuberculosis Immunity? Front Immunol (2020) 11:1599. doi: 10.3389/fimmu.2020.01599
127. Benmerzoug S, Marinho FV, Rose S, Mackowiak C, Gosset D, Sedda D, et al. GM-CSF Targeted Immunomodulation Affects Host Response to M. Tuberculosis Infection. Sci Rep (2018) 8(1):8652. doi: 10.1038/s41598-018-26984-3
128. Kim K, Waterer G, Thomson R, Yang IA, Nashi N, Tan DB, et al. Levels of Anti-Cytokine Antibodies may be Elevated in Patients With Pulmonary Disease Associated With non-Tuberculous Mycobacteria. Cytokine (2014) 66(2):160–3. doi: 10.1016/j.cyto.2014.01.005
129. Suzuki K, Lee WJ, Hashimoto T, Tanaka E, Murayama T, Amitani R, et al. Recombinant Granulocyte-Macrophage Colony-Stimulating Factor (GM-CSF) or Tumour Necrosis Factor-Alpha (TNF-Alpha) Activate Human Alveolar Macrophages to Inhibit Growth of Mycobacterium Avium Complex. Clin Exp Immunol (1994) 98(1):169–73. doi: 10.1111/j.1365-2249.1994.tb06625.x
130. Bermudez LE, Young LS. Ethanol Augments Intracellular Survival of Mycobacterium Avium Complex and Impairs Macrophage Responses to Cytokines. J Infect Dis (1991) 163(6):1286–92. doi: 10.1093/infdis/163.6.1286
131. Bermudez LE. Effect of Ethanol on the Interaction Between the Macrophage and Mycobacterium Avium. Alcohol (1994) 11(2):69–73. doi: 10.1016/0741-8329(94)90046-9
132. Bermudez LE, Young LS, Martinelli J, Petrofsky M. Exposure to Ethanol Up-Regulates the Expression of Mycobacterium Avium Complex Proteins Associated With Bacterial Virulence. J Infect Dis (1993) 168(4):961–8. doi: 10.1093/infdis/168.4.961
133. Mason CM, Dobard E, Zhang P, Nelson S. Alcohol Exacerbates Murine Pulmonary Tuberculosis. Infect Immun (2004) 72(5):2556–63. doi: 10.1128/IAI.72.5.2556-2563.2004
134. Murray PJ, Allen JE, Biswas SK, Fisher EA, Gilroy DW, Goerdt S, et al. Macrophage Activation and Polarization: Nomenclature and Experimental Guidelines. Immunity (2014) 41(1):14–20. doi: 10.1016/j.immuni.2014.06.008
135. Malyshev I, Malyshev Y. Current Concept and Update of the Macrophage Plasticity Concept: Intracellular Mechanisms of Reprogramming and M3 Macrophage "Switch" Phenotype. BioMed Res Int (2015) 2015:341308. doi: 10.1155/2015/341308
136. O'Neill LA, Kishton RJ, Rathmell J. A Guide to Immunometabolism for Immunologists. Nat Rev Immunol (2016) 16(9):553–65. doi: 10.1038/nri.2016.70
137. Edwards JP, Zhang X, Frauwirth KA, Mosser DM. Biochemical and Functional Characterization of Three Activated Macrophage Populations. J Leukoc Biol (2006) 80(6):1298–307. doi: 10.1189/jlb.0406249
138. Pettersen JS, Fuentes-Duculan J, Suarez-Farinas M, Pierson KC, Pitts-Kiefer A, Fan L, et al. Tumor-Associated Macrophages in the Cutaneous SCC Microenvironment are Heterogeneously Activated. J Invest Dermatol (2011) 131(6):1322–30. doi: 10.1038/jid.2011.9
139. Lang R, Patel D, Morris JJ, Rutschman RL, Murray PJ. Shaping Gene Expression in Activated and Resting Primary Macrophages by IL-10. J Immunol (2002) 169(5):2253–63. doi: 10.4049/jimmunol.169.5.2253
140. Laskin DL, Weinberger B, Laskin JD. Functional Heterogeneity in Liver and Lung Macrophages. J Leukoc Biol (2001) 70(2):163–70. doi: 10.1189/jlb.70.2.163
141. Wells CA, Ravasi T, Faulkner GJ, Carninci P, Okazaki Y, Hayashizaki Y, et al. Genetic Control of the Innate Immune Response. BMC Immunol (2003) 4:5. doi: 10.1186/1471-2172-4-5
142. Stout RD, Suttles J. Functional Plasticity of Macrophages: Reversible Adaptation to Changing Microenvironments. J Leukoc Biol (2004) 76(3):509–13. doi: 10.1189/jlb.0504272
143. Curry-McCoy TV, Venado A, Guidot DM, Joshi PC. Alcohol Ingestion Disrupts Alveolar Epithelial Barrier Function by Activation of Macrophage-Derived Transforming Growth Factor Beta1. Respir Res (2013) 14:39. doi: 10.1186/1465-9921-14-39
144. Gaydos J, McNally A, Guo R, Vandivier RW, Simonian PL, Burnham EL. Alcohol Abuse and Smoking Alter Inflammatory Mediator Production by Pulmonary and Systemic Immune Cells. Am J Physiol Lung Cell Mol Physiol (2016) 310(6):L507–18. doi: 10.1152/ajplung.00242.2015
145. O'Halloran EB, Curtis BJ, Afshar M, Chen MM, Kovacs EJ, Burnham EL. Alveolar Macrophage Inflammatory Mediator Expression is Elevated in the Setting of Alcohol Use Disorders. Alcohol (2016) 50:43–50. doi: 10.1016/j.alcohol.2015.11.003
146. Crews FT, Bechara R, Brown LA, Guidot DM, Mandrekar P, Oak S, et al. Cytokines and Alcohol. Alcohol Clin Exp Res (2006) 30(4):720–30. doi: 10.1111/j.1530-0277.2006.00084.x
147. Yeligar SM, Chen MM, Kovacs EJ, Sisson JH, Burnham EL, Brown LA. Alcohol and Lung Injury and Immunity. Alcohol (2016) 55:51–9. doi: 10.1016/j.alcohol.2016.08.005
148. Romero F, Shah D, Duong M, Stafstrom W, Hoek JB, Kallen CB, et al. Chronic Alcohol Ingestion in Rats Alters Lung Metabolism, Promotes Lipid Accumulation, and Impairs Alveolar Macrophage Functions. Am J Respir Cell Mol Biol (2014) 51(6):840–9. doi: 10.1165/rcmb.2014-0127OC
149. Slovinsky WS, Shaghaghi H, Para R, Romero F, Summer R. Alcohol-Induced Lipid Dysregulation Impairs Glycolytic Responses to LPS in Alveolar Macrophages. Alcohol (2020) 83:57–65. doi: 10.1016/j.alcohol.2019.08.009
150. Morris NL, Harris FL, Brown LAS, Yeligar SM. Alcohol Induces Mitochondrial Derangements in Alveolar Macrophages by Upregulating NADPH Oxidase 4. Alcohol (2021) 90:27–38. doi: 10.1016/j.alcohol.2020.11.004
151. Lugo-Villarino G, Verollet C, Maridonneau-Parini I, Neyrolles O. Macrophage Polarization: Convergence Point Targeted by Mycobacterium Tuberculosis and HIV. Front Immunol (2011) 2:43. doi: 10.3389/fimmu.2011.00043
152. Braverman J, Sogi KM, Benjamin D, Nomura DK, Stanley SA. HIF-1alpha Is an Essential Mediator of IFN-Gamma-Dependent Immunity to Mycobacterium Tuberculosis. J Immunol (2016) 197(4):1287–97. doi: 10.4049/jimmunol.1600266
153. Shi L, Salamon H, Eugenin EA, Pine R, Cooper A, Gennaro ML. Infection With Mycobacterium Tuberculosis Induces the Warburg Effect in Mouse Lungs. Sci Rep (2015) 5:18176. doi: 10.1038/srep18176
154. Gleeson LE, Sheedy FJ, Palsson-McDermott EM, Triglia D, O'Leary SM, O'Sullivan MP, et al. Cutting Edge: Mycobacterium Tuberculosis Induces Aerobic Glycolysis in Human Alveolar Macrophages That Is Required for Control of Intracellular Bacillary Replication. J Immunol (2016) 196(6):2444–9. doi: 10.4049/jimmunol.1501612
155. Lachmandas E, Beigier-Bompadre M, Cheng SC, Kumar V, van Laarhoven A, Wang X, et al. Rewiring Cellular Metabolism via the AKT/mTOR Pathway Contributes to Host Defence Against Mycobacterium Tuberculosis in Human and Murine Cells. Eur J Immunol (2016) 46(11):2574–86. doi: 10.1002/eji.201546259
156. Huang L, Nazarova EV, Tan S, Liu Y, Russell DG. Growth of Mycobacterium Tuberculosis In Vivo Segregates With Host Macrophage Metabolism and Ontogeny. J Exp Med (2018) 215(4):1135–52. doi: 10.1084/jem.20172020
157. Benoit M, Desnues B, Mege JL. Macrophage Polarization in Bacterial Infections. J Immunol (2008) 181(6):3733–9. doi: 10.4049/jimmunol.181.6.3733
158. Almeida AS, Lago PM, Boechat N, Huard RC, Lazzarini LC, Santos AR, et al. Tuberculosis is Associated With a Down-Modulatory Lung Immune Response That Impairs Th1-Type Immunity. J Immunol (2009) 183(1):718–31. doi: 10.4049/jimmunol.0801212
159. Bonecini-Almeida MG, Ho JL, Boechat N, Huard RC, Chitale S, Doo H, et al. Down-Modulation of Lung Immune Responses by Interleukin-10 and Transforming Growth Factor Beta (TGF-Beta) and Analysis of TGF-Beta Receptors I and II in Active Tuberculosis. Infect Immun (2004) 72(5):2628–34. doi: 10.1128/IAI.72.5.2628-2634.2004
160. Hackett EE, Charles-Messance H, O'Leary SM, Gleeson LE, Munoz-Wolf N, Case S, et al. Mycobacterium Tuberculosis Limits Host Glycolysis and IL-1beta by Restriction of PFK-M via MicroRNA-21. Cell Rep (2020) 30(1):124–36 e4. doi: 10.1016/j.celrep.2019.12.015
161. Shi L, Jiang Q, Bushkin Y, Subbian S, Tyagi S. Biphasic Dynamics of Macrophage Immunometabolism During Mycobacterium Tuberculosis Infection. mBio (2019) 10(2):e02550-18. doi: 10.1128/mBio.02550-18
162. Mohareer K, Medikonda J, Vadankula GR, Banerjee S. Mycobacterial Control of Host Mitochondria: Bioenergetic and Metabolic Changes Shaping Cell Fate and Infection Outcome. Front Cell Infect Microbiol (2020) 10:457. doi: 10.3389/fcimb.2020.00457
163. Raju B, Hoshino Y, Belitskaya-Levy I, Dawson R, Ress S, Gold JA, et al. Gene Expression Profiles of Bronchoalveolar Cells in Pulmonary TB. Tuberculosis (Edinb) (2008) 88(1):39–51. doi: 10.1016/j.tube.2007.07.003
Keywords: alcohol, alcohol use disorder (AUD), tuberculosis, alveolar macrophage (AM), innate immunity, oxidative stress
Citation: Wigger GW, Bouton TC, Jacobson KR, Auld SC, Yeligar SM and Staitieh BS (2022) The Impact of Alcohol Use Disorder on Tuberculosis: A Review of the Epidemiology and Potential Immunologic Mechanisms. Front. Immunol. 13:864817. doi: 10.3389/fimmu.2022.864817
Received: 28 January 2022; Accepted: 09 March 2022;
Published: 31 March 2022.
Edited by:
Bruno Rivas-Santiago, Unidad de Investigación Biomédica de Zacatecas (IMSS), MexicoReviewed by:
Katrin Doris Mayer-Barber, National Institutes of Health (NIH), United StatesRoberta Provvedi, University of Padua, Italy
Alejandra Montoya-Rosales, Biomark Life Sciences, Mexico
Copyright © 2022 Wigger, Bouton, Jacobson, Auld, Yeligar and Staitieh. This is an open-access article distributed under the terms of the Creative Commons Attribution License (CC BY). The use, distribution or reproduction in other forums is permitted, provided the original author(s) and the copyright owner(s) are credited and that the original publication in this journal is cited, in accordance with accepted academic practice. No use, distribution or reproduction is permitted which does not comply with these terms.
*Correspondence: Gregory W. Wigger, Z3dpZ2dlckBlbW9yeS5lZHU=