- 1Japan Animal Specialty Medical Institute, Tsuzuki, Yokohama, Japan
- 2Laboratory for Mucosal Immunity, Center for Integrative Medical Sciences, RIKEN Yokohama Institute, Yokohama, Japan
- 3Laboratory of Veterinary Radiotherapy, Department of Veterinary Medicine, College of Bioresource Sciences, Nihon University, Fujisawa, Japan
Cardiac fibroblasts participate in the inflammatory process of heart diseases as sentinel cells of the cardiac tissue. In this study, we investigated the effect of the proinflammatory cytokine, interleukin 1β (IL-1β), on the expression of interleukin 8 (IL-8), which contributes to the induction of innate immunity via the activation and recruitment of innate immune cells, such as neutrophils, to the site of inflammation in canine cardiac fibroblasts. IL-1β mediates IL-8 mRNA expression and protein release in a dose- and time-dependent manner. The IL-β-mediated IL-8 protein release and mRNA expression were inhibited by 2-[(aminocarbonyl)amino]-5-(4-fluorophenyl)-3-thiophenecarboxamide, an inhibitor of the transcription factor, nuclear factor (NF)-κB. In cells treated with IL-1β, NF-κB p65 and p105 were transiently phosphorylated, indicating the activation of NF-κB. However, IL-1β failed to induce IL-8 mRNA expression in the cells transfected with p65 small interfering RNA (siRNA), but not in those transfected with p105 siRNA. These observations suggest that IL-1β induces IL-8 expression via the activation of NF-κB p65 in canine cardiac fibroblasts.
Introduction
The myocardium consists of several cell types, namely, cardiomyocytes, cardiac fibroblasts, endothelial cells, and smooth muscles. Of these, cardiac fibroblasts have a large cell population (1), although the relative number of cardiomyocytes and non-cardiomyocytes likely varies among different species (2). Cardiac fibroblasts are involved in many aspects of cardiac function, such as maintaining normal cardiac structure, cell signaling, and electro-mechanical function of the heart (3–5). They also participate in the inflammatory process of heart diseases as sentinel cells of the cardiac tissue (6, 7). They respond to pathogen-associated molecular patterns (PAMPs) or danger-associated molecular patterns (DAMPs) that secrete a wide variety of proinflammatory cytokines and chemokines (6).
Interleukin-8 (IL-8), also known as the C-X-C motif chemokine ligand 8 (CXCL8), is a proinflammatory ELR+ C-X-C chemokine. IL-8 plays an important role in the induction of innate immunity via the activation and recruitment of innate immune cells, such as neutrophils, to the site of inflammation (8, 9). IL-8 is also involved in angiogenesis by promoting the proliferation, growth, and viability of vascular endothelial cells (10, 11). IL-8 is produced in and secreted from various cell types, namely, blood monocytes, alveolar macrophages, and non-immune cells, such as endothelial cells and fibroblasts (12–16). IL-8 expression is stimulated by a variety of stimuli, namely, pro-inflammatory cytokines, although it is virtually undetectable in unstimulated cells (17). The pro-inflammatory cytokine interleukin-1β (IL-1β), which contributes to acute or chronic inflammation by mediating the expression and secretion of cytokines and chemokines in inflamed tissues (18, 19), has been shown to mediate the expression and secretion of IL-8 as demonstrated in various cells, namely, fibroblasts (14–16, 20, 21).
Nuclear factor kappa B (NF-κB) is a family of transcription factors critical for regulating a variety of cellular functions, namely, the immune response and inflammation (22–24). NF-κB consists of five subunits: p65 (RelA), RelB, c-Rel (Rel), p50/p105 (NF-κB1), and p52/p100 (NF-κB2). These subunits form homo- and heterodimer complexes, resulting in up to 15 dimers with varying cellular functions (22–24). NF-κB activity is mainly regulated by its interaction with inhibitory proteins, such as IκB. In its latent form, dimers of NF-κB occur as a complex with IκB in the cytoplasm, which is an inactive form. NF-κB is activated by the disruption of the interaction between IκB and NF-κB. The dimeric complex of NF-κB released by the degradation of IκB translocates into the nucleus and induces the expression of immune and inflammatory genes by binding to their promoters (22–24). The NF-κB pathway consists of two pathways: canonical and noncanonical. Pro-inflammatory cytokines, such as IL-1β and tumor necrosis factor α (TNF-α), activate the canonical NF-κB signaling pathway, while other stimuli, such as the CD40 ligand, activate the non-canonical pathway (22–24).
In this study, we demonstrated that IL-1β mediated the expression of IL-8 via the activation of NF-κB p65 in canine cardiac fibroblasts.
Materials and Methods
Materials
Dulbecco’s modified Eagle’s medium with 1 g/L glucose (DMEM-LG), 4-(2-hydroxyethyl)-1-piperazineethanesulfonic acid (HEPES), phenylmethanesulfonyl fluoride (PMSF), sodium fluoride, and fetal bovine serum (FBS) was purchased from FUJIFILM Wako Chemical Corp. (Osaka, Japan). Recombinant canine IL-1β and 2-[(aminocarbonyl)amino]-5-(4-fluorophenyl)-3-thiophenecarboxamide (TPCA-1) were purchased from Kingfisher Biotech, Inc. (Saint Paul, MN) and MedChemExpress (Monmouth Junction, NJ, USA), respectively. Lipofectamine 2000 and TRIzol were purchased from Life Technologies (Carlsbad, CA, USA). CELLBANKER 1 plus medium, Thermal Cycler Dice Real Time System II, TP900 Dice Real Time v4.02B, SYBR Premix Ex Taq II, and PrimeScript RT Master Mix were purchased from TaKaRa Bio, Inc. (Shiga, Japan). Rabbit monoclonal anti-phosphorylated p65 (93H1), anti-total p65 (D14E12), anti-phosphorylated p105 (18E6), anti-total p105 (D4P4D), and mouse monoclonal anti-total IκBα (L35A5) antibodies were purchased from Cell Signaling Technology Japan, K.K. (Tokyo, Japan). Anti-β-actin mouse monoclonal antibody (AC74), small interfering RNA (siRNA) for p65 and p105, and scrambled siRNA were obtained from Sigma-Aldrich Inc. (St. Louis, MO, USA). Horseradish peroxidase (HRP)-conjugated anti-rabbit and anti-mouse IgG antibodies, the ECL western blotting Analysis System, and Amersham ImageQuant 800 were purchased from Cytiva Japan (Tokyo, Japan). Mini-PROTEAN TGX gel, polyvinylidene difluoride (PVDF) membranes, and iCycler were obtained from Bio-Rad (Hercules, CA). The complete mini ethylenediaminetetraacetic acid (EDTA)-free protease inhibitor mixture and Block Ace were purchased from Roche (Mannheim, Germany) and KAC Co., Ltd. (Hyogo, Japan), respectively. An enzyme-linked immunosorbent assay (ELISA) kit for canine IL-8 (#CA8000), a freezing vessel (BICELL), and StatMate IV were purchased from R&D Systems, Inc. (Minneapolis, MN), Nihon Freezer Co., Ltd. (Tokyo, Japan), and ATMS (Tokyo, Japan), respectively.
Cell Culture
Canine primary cardiac fibroblasts were purchased from Cell Biologics (Chicago, IL, USA). The thawed-out cell suspension was transferred into a centrifuge tube contained DMEM-LG containing 20% FBS. After centrifugation at 300×g for 1 min and removal of the supernatant, the cell pellet was resuspended in DMEM-LG containing 20% FBS and transferred into a 75-cm2 culture flask. The cells were maintained in static culture in an incubator at 5% carbon dioxide (CO2) and 37°C using DMEM-LG supplemented with 20% fetal calf serum, and the medium was changed once a week. When the fibroblasts reached 90–95% confluence, they were harvested using 0.25% trypsin-EDTA. The collected cardiac fibroblasts were suspended using CELLBANKER 1 plus medium at a density of 2 × 106 cells/500 μl, divided into 500 μl each, and placed into a sterilized serum tube. The tubes were then placed into the freezing vessel BICELL and cryopreserved at −80°C. Before being used in the experiments, serum tubes were removed from the BICELL vessel and immersed in a water bath at 37°C. The thawed-out cell suspension was transferred into a centrifuge tube contained DMEM-LG containing 20% FBS. After centrifugation at 300×g for 1 min and removal of the supernatant, the pellet was suspended in DMEM-LG containing 20% FBS and transferred into a 75-cm2 culture flask. Static cultures were then performed under the same conditions as those before cryopreservation.
Real-Time Quantitative Reverse Transcription-Polymerase Chain Reaction (qRT-PCR)
Real-time RT-PCR was performed as previously reported (25–36). Total RNA was extracted from cultured canine cardiac fibroblasts using TRIzol reagent, following the instructions of the manufacturer. Synthesis of first-strand cDNA was performed with 500 ng of total RNA using PrimeScript RT Master Mix. Real-time RT-PCR was performed with 2 μl of first-strand cDNA in 25 μl (total reaction volume), SYBR Premix Ex Taq II, and primers targeting canine IL-8 or hypoxanthine phosphoribosyltransferase 1 (HPRT1), the housekeeping gene (Table 1). Real-time RT-PCRs of “no-template” controls or “no-reverse transcription” controls were performed with 2 μl of RNase- and DNA-free water or 2 μl of each RNA sample, respectively. PCR was conducted using the Thermal Cycler Dice Real-Time System II. The protocol was as follows: one cycle of denaturation at 95°C for 30 s, 40 cycles of denaturation at 95°C for 5 s, and annealing/extension at 60°C for 30 s. The results were analyzed by the second derivative maximum method and the comparative cycle threshold (ΔΔCt) method using real-time RT-PCR analysis software. The amplification of HPRT1 from the same amount of cDNA was used as an endogenous control, whereas cDNA amplification from canine cardiac fibroblasts at time 0 was used as the calibration standard.
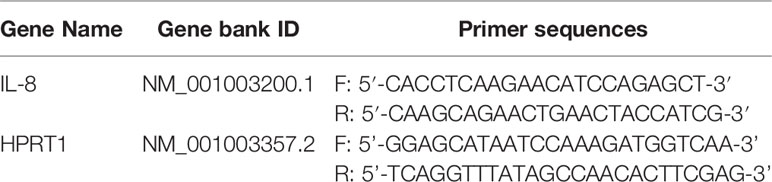
Table 1 Primer sequences for quantitative reverse transcription-polymerase chain reaction (qRT-PCR).
Western Blotting
Western blotting was performed as described previously (25–27, 29–36). Canine cardiac fibroblasts were lysed in 20 mM HEPES buffer (pH 7.4) containing 1 mM PMSF, 10 mM sodium fluoride, and a complete mini EDTA-free protease inhibitor cocktail. Protein concentrations were determined using the Bradford method (37) and adjusted. After boiling at 98°C for 5 min in the sodium dodecyl sulfate buffer, the extracted protein samples were loaded into separate lanes of 12 or 7.5% Mini-PROTEAN TGX gel and electrophoretically separated. Separated proteins were transferred to PVDF membranes, treated with Block Ace for 50 min at room temperature, and incubated with the following primary antibodies [phosphorylated p-65 (p-p65, 1:1,000), total p65 (t-p65, 1:1,000), phosphorylated p-105 (p-p105, 1:1,000), total p105 (t-p105, 1:1,000), total IκBα (t-IκBα, 1:1,000), and β-actin (1:10,000)] for 120 min at room temperature. After washing, membranes were incubated with HRP-conjugated anti-rabbit or anti-mouse IgG (1:10,000) for 90 min at room temperature. Immunoreactivity was detected using the ECL western blotting Analysis System, and chemiluminescent signals of the membranes were measured using an Amersham ImageQuant 8000.
ELISA for IL-8
Canine cardiac fibroblasts were seeded at a density of 3.0 × 105 cells/well in 6-well culture plates. After starvation for 24 h, fibroblasts were treated with IL-1β for 0–24 h and the culture medium was collected. The IL-8 concentration in the culture medium was assayed using an ELISA kit, according to the instructions of the manufacturer.
Transfection of siRNA
siRNA transfection was performed as previously described with slight modifications (25–27, 29–36). Canine cardiac fibroblasts were seeded at a density of 1 × 105 cells/35 mm dish or 5 × 105 cells/90 mm dish, and transfected using Opti-MEM containing 3 μl/ml Lipofectamine 2000 and 2 μM p65, p105, or scramble siRNA (Table 2) for 24 h. After transfection, the medium was changed to DMEM-LG containing 20% FBS and the cultures were maintained in an incubator with 5% CO2 at 37°C for 2 d. The efficiency of siRNA-mediated knockdown was validated by western blotting.
Statistical Analysis
All statistical analyses were performed using EZR (38), a graphical user interface for R (R Foundation for Statistical Computing, Vienna, Austria). More precisely, it is a modified version of the R commander designed to add statistical functions frequently used in biostatistics. Data from all experiments are shown as the mean ± standard error and were analyzed using one-way analysis of variance (ANOVA) with Holm–Bonferroni multiple comparisons test as a post hoc test. Statistical significance was set at p <0.05.
Results
IL-1β-Mediated IL-8 mRNA Expression and Protein Release
When canine cardiac fibroblasts were incubated with 100 pM IL-1β for 0–48 h, the concentration of IL-8 in the incubation medium increased in a time-dependent manner (Figure 1A). In fibroblasts treated with various concentrations of IL-1β (0–200 pM) for 24 h, IL-1β stimulated IL-8 release in a dose-dependent manner (Figure 1B). We examined the effect of IL-1β on IL-8 mRNA expression in canine cardiac fibroblasts. As shown in Figures 1C, D, IL-1β stimulated IL-8 mRNA expression in a time- and dose-dependent manner. These observations suggest that IL-1β induces IL-8 expression and release in canine cardiac fibroblasts.
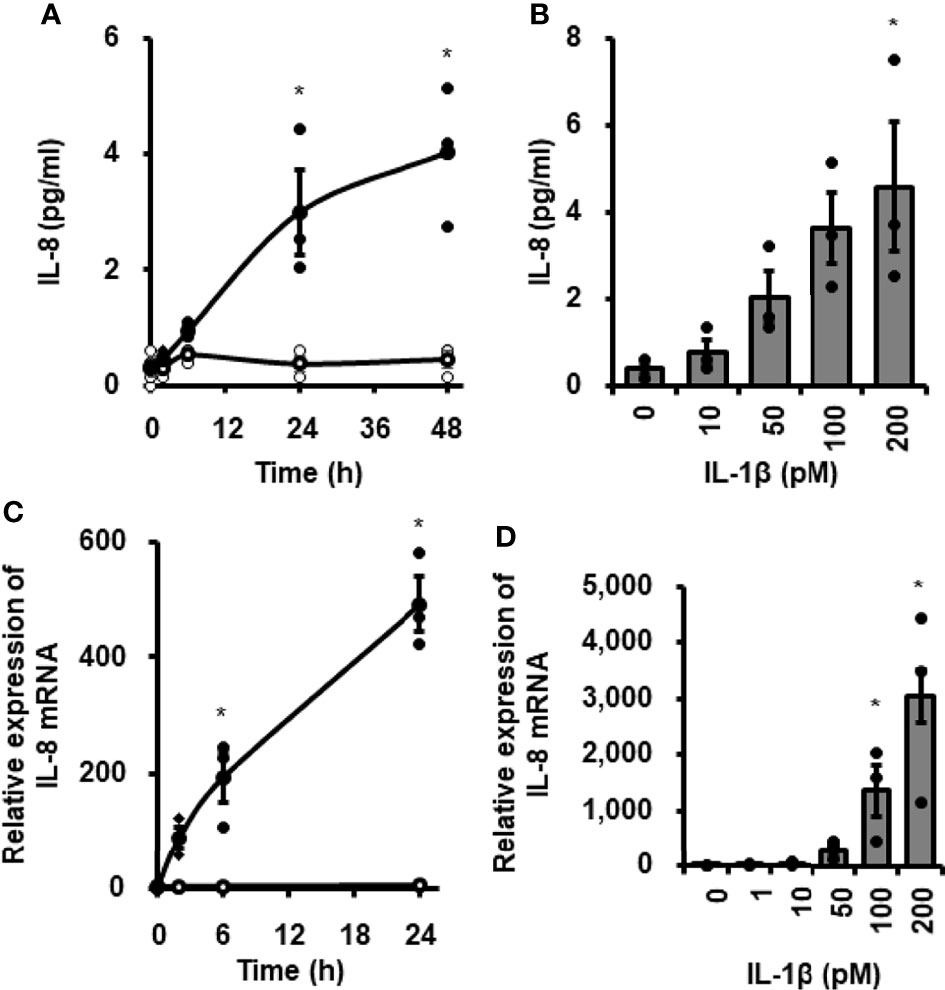
Figure 1 IL-1β-mediated IL-8 release and IL-8 mRNA expression in canine cardiac fibroblasts. Time-dependent increase in IL-8 protein release (A) and IL-8 mRNA expression levels (C) in fibroblasts treated with (closed circle) or without (open circle) canine recombinant IL-1β (100 pM). Dose-dependent IL-8 protein release (B) and IL-8 mRNA expression levels (D) in fibroblasts treated with the indicated concentrations of IL-1β for 24h. HRPT1 was used as an internal standard and the expression levels of IL-8 mRNA in IL-1β-stimulated fibroblasts were compared with the expression at 0h. Results have been represented as mean ± standard error (SE) from biological triplicates. *P <0.05.
Inhibitory Effect of TPCA-1 on IL-1β-Mediated IL-8 mRNA Expression and Protein Release
IL-1β is known to mediate the mRNA expression of various proteins via the activation of the transcription factor NF-κB. We then examined the involvement of NF-κB in IL-1β-mediated IL-8 expression in canine cardiac fibroblasts using the NF-κB inhibitor TPCA-1. In fibroblasts pretreated with TPCA-1 (10 μM) for 1 h, the effects of IL-1β on IL-8 mRNA expression (Figure 2A) and IL-8 release (Figure 2B) were clearly attenuated. These observations suggest that NF-κB activation is involved in the IL-1β-induced IL-8 mRNA expression in canine cardiac fibroblasts.
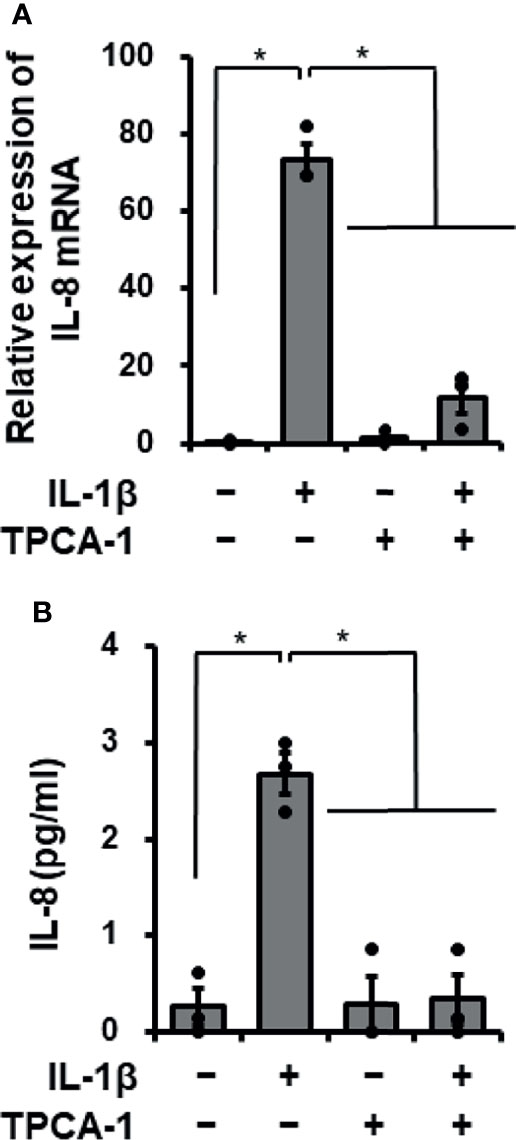
Figure 2 Effect of the NF-κB inhibitor TPCA-1 on IL-1β-mediated IL-8 mRNA expression. Canine cardiac fibroblasts were pretreated with or without TPCA-1 (10 µM) for 1 h and subsequently stimulated with or without IL-1β (100 pM) for 24 h. After stimulation, IL-8 mRNA expression levels (A) and the release of IL-8 (B) were determined. HRPT1 was used as an internal standard and the expression levels of IL-8 mRNA in IL-1β-stimulated fibroblasts were compared with the expression at 0 h. Results have been represented as mean ± standard error (SE) from biological triplicates. *P <0.05.
IL-1β-Mediated Activation of NF-κB
Next, we examined the effects of IL-1β on the activation of NF-κB in canine cardiac fibroblasts. When fibroblasts were stimulated with 100 pM IL-1β for 0–300 min, IκBα degradation was observed at 15–30 min (Figures 3A, B). Phosphorylation of p65 and p105 was also observed 15–30 min after stimulation, indicating the activation of NF-κB by IL-1β (Figures 3A, C, D). In fibroblasts pretreated with the NF-κB inhibitor, TPCA-1, for 1 h, IL-1β-mediated phosphorylation of p65 and p105 was clearly attenuated (Figure 4). These observations suggest that IL-1β induces IL-8 expression via the activation of NF-κB.
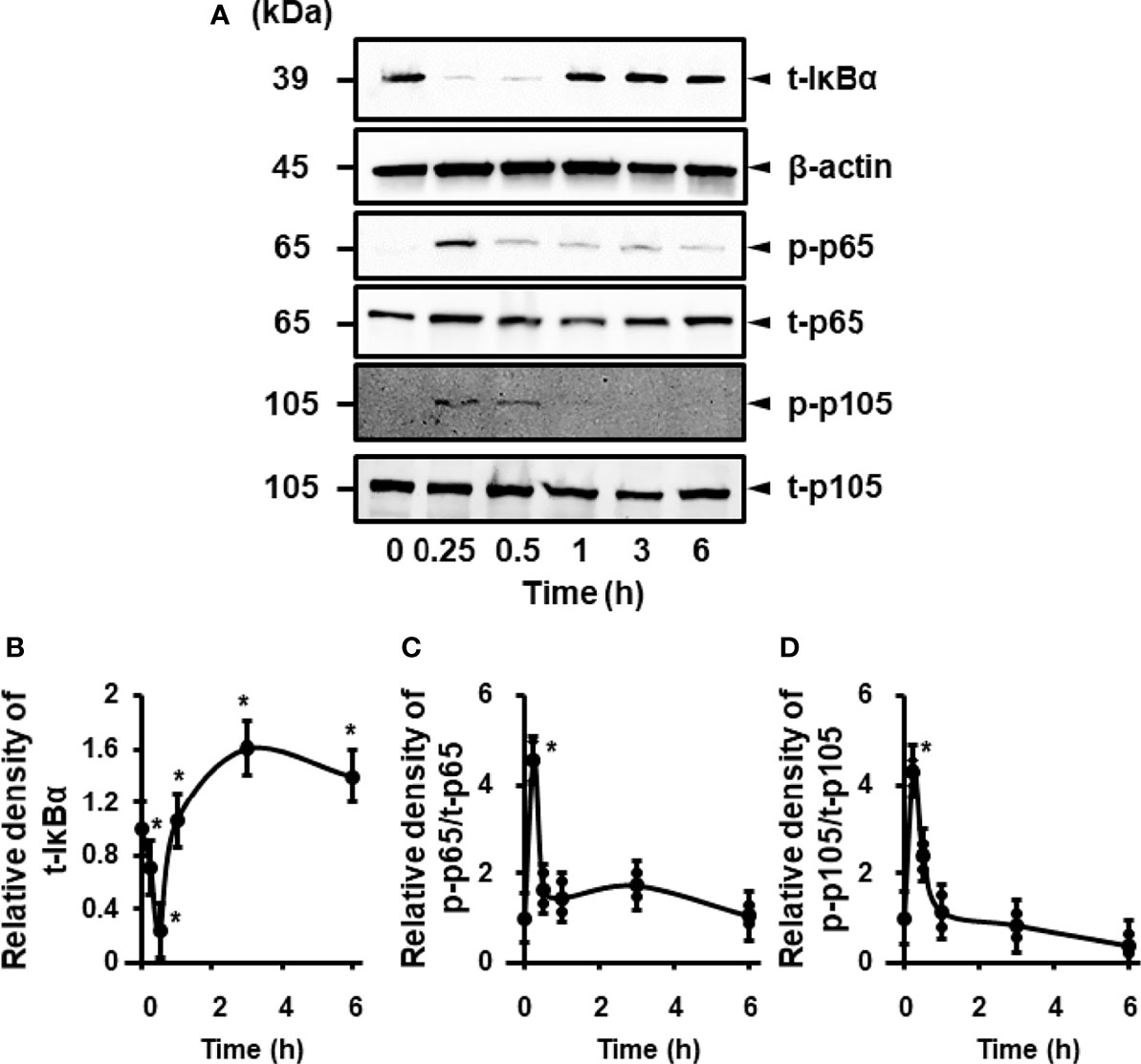
Figure 3 IL-1β-mediated activation of NF-κB. (A) Representative western blotting results of levels of total IκBα (t-IκBα), phosphorylated p65 (p-p65), total p65 (t-p65), phosphorylated p105 (p-p105), and total p105 (t-p105) in canine cardiac fibroblasts treated with IL-1β (100 pM). β-actin was used as an internal standard. Time-dependent changes of levels of t-IκBα, p-p65 and p-p105 were observed. Relative levels of t-IκBα (B), [p-p65]/[t-p65] (C), and [p-p105]/[t-p105] (D) in IL-1β-stimulated fibroblasts compared to the levels at 0h. Results have been represented as mean ± SE from biological triplicates. *P <0.05.
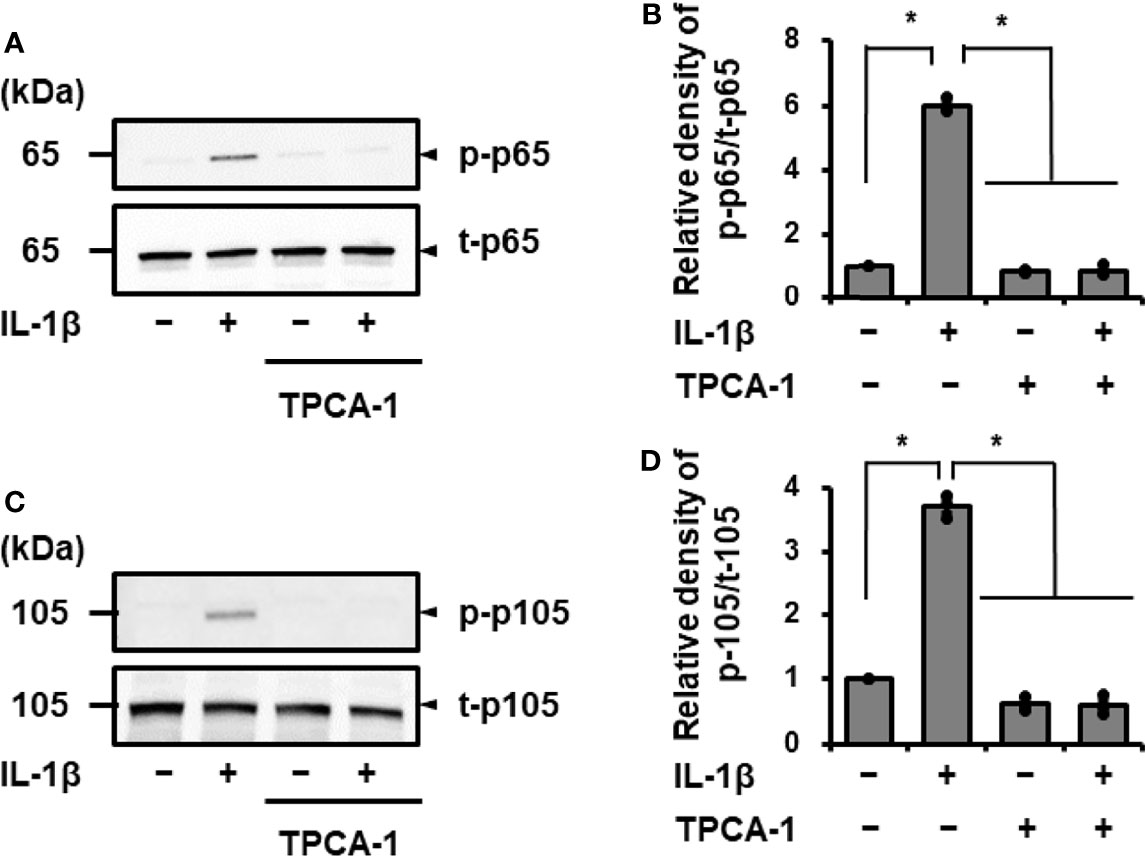
Figure 4 Effect of the NF-κB inhibitor TPCA-1 on IL-1β mediated phosphorylation of p65 and p105. Canine cardiac fibroblasts were pretreated with or without TPCA-1 (10 µM) for 1 h and stimulated with IL-1β (100 pM) for 15 min. Representative western blotting results of inhibitory effect of TPCA-1 on IL-1β-mediated phosphorylation of p65 (A) and p105 (C), and relative levels of [p-65]/[t-65] (B) and [p-105]/[t-105] (D) as compared to those without the inhibitor and IL-1β. Results have been represented as mean ± SE from biological triplicates. *P <0.05.
IL-1β-Mediated IL-8 mRNA Expression in Cells Transfected With siRNA of p65 or p105
To confirm the contribution of NF-κB to IL-1β-induced IL-8 expression, we examined the effect of IL-1β on IL-8 mRNA expression in p65 or p105 knockdown fibroblasts using siRNA transfection. In fibroblasts transfected with p65 or p105 siRNA, p65 or p105 protein expression was clearly reduced compared with that in control fibroblasts transfected with scramble RNA (Figures 5A–C). IL-1β-mediated IL-8 mRNA expression was clearly decreased in fibroblasts transfected with p65 siRNA compared to that in the control (Figure 5D). However, p105 siRNA transfection failed to attenuate the IL-1β-mediated IL-8 mRNA expression (Figure 5D). Taken together, it is likely that NF-κB p65 activation contributes to IL-1β-mediated expression of IL-8, but not p105, in canine cardiac fibroblasts.
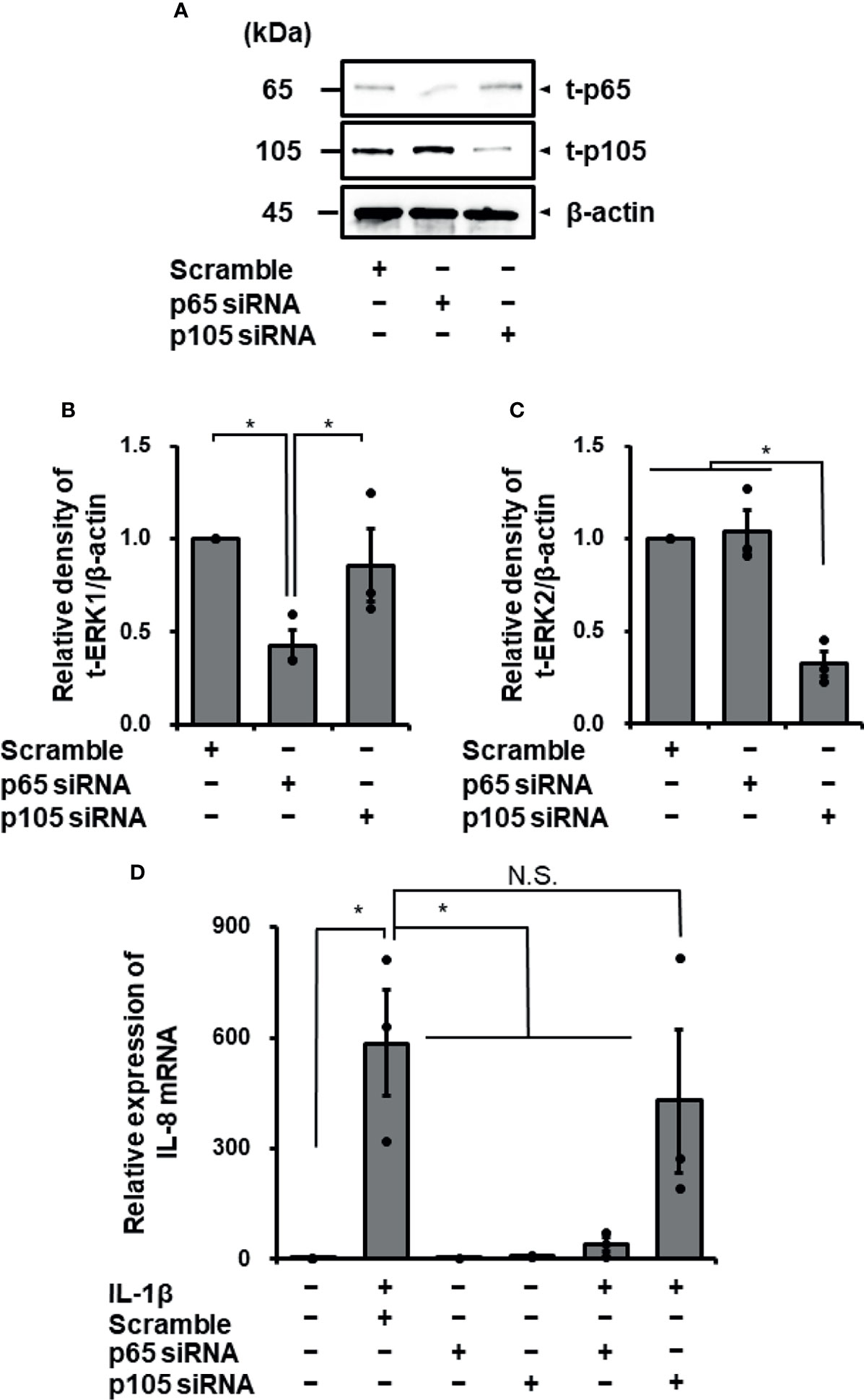
Figure 5 Transfection with p65 siRNA attenuated IL-1β-mediated IL-8 mRNA expression in canine cardiac fibroblasts but not that with p105 siRNA. In canine cardiac fibroblasts transfected with p65, p105, and scrambled siRNAs, expression of t-p65, t-105, and β-actin was detected by western blotting. The expression of p65 or p105 was reduced in fibroblasts transfected with p65 or p105 siRNA, respectively, but not in fibroblasts transfected with scrambled siRNA. β-actin was used as an internal standard. Representative results (A) and relative density of protein expression of t-p65 (B) or t-p105 (C) in siRNA-transfected fibroblasts compared with those in scrambled siRNA-transfected fibroblasts are depicted. (D) Cardiac fibroblasts transfected with p65, p105 and scrambled siRNAs were incubated with or without IL-1β (100 pM) for 24 h After the incubation, IL-8 mRNA expression was determined. HRPT1 was used as an internal standard. Transfection with p65 siRNA resulted in reduction of IL-1β-mediated IL-8 mRNA expression, while p105 and scrambled siRNA-transfection did not. Results have been represented as mean ± standard error (SE) from biological triplicates. *P <0.05; N.S, Not Significant.
Discussion
In this study, we demonstrated that IL-1β mediates IL-8 production in canine cardiac fibroblasts. IL-8 is a critical inflammatory mediator that functions as a chemotactic factor for neutrophils and lymphocytes, and an angiogenic factor (39–41). In human patients with myocardial infarction, which is associated with an intense inflammatory response that ultimately leads to healing and scar formation, a significant increase in serum IL-8 levels has been reported (42–44). An increase in serum IL-8 concentrations has also been reported in human patients undergoing cardiac transplantation (45, 46). Ischemia followed by reperfusion (I/R) induces a complex series of inflammatory reactions, resulting in myocardial injury in animals (47). In a rabbit and rat model of myocardial I/R injury, an increase in serum IL-8 levels was observed (48, 49). In a dog treated with I/R, IL-8 mRNA expression in the myocardium was enhanced (50). These observations suggested that IL-8 is involved in cardiac inflammation and myocardial injury. Recombinant canine IL-8 markedly increases the adhesion of neutrophils to isolated canine cardiac myocytes in vitro, but the effect of IL-8 is blocked by antibodies directed against IL-8 (50). In a rabbit model of I/R injury, neutralization of IL-8 using a monoclonal antibody against IL-8 resulted in a reduction in the degree of necrosis (51). In a model of I/R injury, treatment with pterostilbene, a candidate anti-inflammatory substance, attenuated serum IL-8 levels, which was associated with a reduction in infarct size and myocardial apoptosis (49). Therefore, it is likely that IL-8 mediates inflammation via I/R injury.
IL-1β is a pro-inflammatory mediator in acute and chronic inflammation, which provokes the synthesis and expression of a variety of secondary inflammatory mediators (52, 53). In experimental mouse models, IL-1β was reported to be highly expressed in cardiac tissues following acute myocardial infarction (54).
The blockade of IL-1 signaling attenuates heart failure after acute myocardial infarction in both mice and humans (55, 56). These observations suggest that IL-1β plays an important role in MI pathogenesis in myocardial infarction. IL-1β is considered important for recruiting leukocytes, especially neutrophils and monocytes, to the infarcted area after myocardial infarction (54, 55). Therefore, IL-1β-mediated IL-8 expression and release in cardiac fibroblasts may contribute to inflammation.
In this study, we demonstrated that NF-κB p65 contributes to IL-1β-mediated IL-8 expression in canine cardiac fibroblasts. In the absence of stimuli, p65 exists in the cytoplasm in a heterodimeric form with the p50 subunit or a homodimeric form in the inactivated state bound to an inhibitory IκB protein, such as IκBα, inhibiting translocation of NF-κB into the nucleus (57–60). In cells stimulated with cytokines, including IL-1β, the IκB protein is degraded by the proteasome, and both dimeric forms of NF-κB are translocated into the nucleus to induce the expression of immune and inflammatory genes by binding to their promoters (23, 47, 61). We demonstrated that IκBα was degraded in cells stimulated with IL-1β. Degradation of IκB is regulated by IκB kinases (23, 47, 61). In our study, IL-1β-mediated IL-8 mRNA expression and protein release in cells treated with the IκB kinase inhibitor TPCA-1 (62). Therefore, it is likely that IκB kinase activation is an important upstream of the activation of p65 in canine cardiac fibroblasts. In our study, IL-1β stimulated the phosphorylation of p105. p105 is a precursor protein that generates the p50. Phosphorylation of p105 is involved in signal-induced p105 processing to p50 (63–67). However, IL-1β-mediated IL-8 mRNA expression occurred in the p105-knockdown cells. Therefore, it is unlikely that p50/p105 is involved in the IL-1β-mediated IL-8 mRNA expression. NF-κB p65 homodimers preferentially bind to the promoter regions of the IL-8 gene (59, 68). Thus, it is conceivable that the p65 homodimer functions as a regulator of IL-8 expression in canine cardiac fibroblasts stimulated with IL-1β. In this study, it is still unclear how IL-8 gene and its transcription are regulated in a genomic context in IL-1β-cardiac fibroblasts. Elucidation of genome-wide regulation in this pathway is indeed our next research subject.
In conclusion, IL-1β mediates IL-8 expression in canine cardiac fibroblasts, and activation of NF-κB p65 contributes to this IL-1β-mediated IL-8 expression. These results support the idea that cardiac fibroblasts are involved in the inflammatory processes of heart diseases as sentinel cells of the cardiac tissue (6, 7).
Data Availability Statement
The original contributions presented in the study are included in the article/Supplementary Material. Further inquiries can be directed to the corresponding author.
Author Contributions
MasM, R.N., H.S., and M.U. designed and planned the experiments. MasM, R.N., S.N., MoeM, N.Y. and K.K. prepared, measured, and analyzed the samples. MasM, R.N., H.S., and M.U. interpreted the data and wrote the paper. All authors listed have made a substantial, direct, and intellectual contribution to the work and approved it for publication.
Funding
This work was supported in part by a Grant-in-Aid for Scientific Research (grant no. 18K14594, RN) from the Ministry of Education, Science, Sports, and Culture of Japan (https://www.jsps.go.jp/j-grantsinaid/). The funders had no role in the study design, data collection and analysis, decision to publish, or preparation of the manuscript.
Conflict of Interest
The authors declare that the research was conducted in the absence of any commercial or financial relationships that could be construed as a potential conflict of interest.
Publisher’s Note
All claims expressed in this article are solely those of the authors and do not necessarily represent those of their affiliated organizations, or those of the publisher, the editors and the reviewers. Any product that may be evaluated in this article, or claim that may be made by its manufacturer, is not guaranteed or endorsed by the publisher.
Acknowledgments
We would like to thank Editage (www.editage.jp) for English language editing.
Supplementary Material
The Supplementary Material for this article can be found online at: https://www.frontiersin.org/articles/10.3389/fimmu.2022.863309/full#supplementary-material
References
1. Camelliti P, Borg TK, Kohl P. Structural and Functional Characterisation of Cardiac Fibroblasts. Cardiovasc Res (2005) 65:40–51. doi: 10.1016/j.cardiores.2004.08.020
2. Souders CA, Bowers SL, Baudino TA. Cardiac Fibroblast: The Renaissance Cell. Circ Res (2009) 105:1164–76. doi: 10.1161/CIRCRESAHA.109.209809
3. MacKenna D, Summerour SR, Villarreal FJ. Role of Mechanical.Factors in Modulating Cardiac Fibroblast Function and Extracellular Matrix Synthesis. Cardiovasc Res (2000) 46:257–63. doi: 10.1016/S0008-6363(00)00030-4
4. Sun Y, Kiani MF, Postlethwaite AE, Weber KT. Infarct Scar as Living Tissue. Basic Res Cardiol (2002) 97:343–7. doi: 10.1007/s00395-002-0365-8
5. Kohl P, Noble D. Mechanosensitive Connective Tissue: Potential Influence on Heart Rhythm. Cardiovasc Res (1996) 32:62–8. doi: 10.1016/S0008-6363(95)00224-3
6. Díaz-Araya G, Vivar R, Humeres C, Boza P, Bolivar S, Muñoz C. Cardiac Fibroblasts as Sentinel Cells in Cardiac Tissue: Receptors, Signaling Pathways and Cellular Functions. Pharmacol Res (2015) 101:30–40. doi: 10.1016/j.phrs.2015.07.001
7. Turner NA, Porter KE. Function and Fate of Myofibroblasts After Myocardial Infarction. Fibrogenes Tissue Repair (2013) 6:5. doi: 10.1186/1755-1536-6-5
8. Baggiolini M, Clark-Lewis I. Interleukin-8, a Chemotactic and Inflammatory Cytokine. FEBS Lett (1992) 307:97–101. doi: 10.1016/0014-5793(92)80909-Z
9. Harada A, Sekido N, Akahoshi T, Wada T, Mukaida N, Matsushima K. Essential Involvement of Interleukin-8 (IL-8) in Acute Inflammation. J Leukoc Biol (1994) 56:559–64. doi: 10.1002/jlb.56.5.559
10. Li A, Dubey S, Varney ML, Dave BJ, Singh RK. IL-8 Directly Enhanced Endothelial Cell Survival, Proliferation, and Matrix Metalloproteinases Production and Regulated Angiogenesis. J Immunol (2003) 170:3369–76. doi: 10.4049/jimmunol.170.6.3369
11. Rosenkilde MM, Schwartz TW. The Chemokine System – a Major Regulator of Angiogenesis in Health and Disease. APMIS (2004) 112:481–95. doi: 10.1111/j.1600-0463.2004.apm11207-0808.x
12. Peveri P, Walz A, Dewald B, Baggiolini M. A Novel Neutrophil-Activating Factor Produced by Human Mononuclear Phagocytes. J Exp Med (1988) 167:1547–59. doi: 10.1084/jem.167.5.1547
13. Strieter RM, Kunkel SL, Showell HJ, Remick DG, Phan SH, Ward PA, et al. Endothelial Cell Gene Expression of a Neutrophil Chemotactic Factor by TNF-α, LPS, and IL-1β. Science (1989) 243:1467–9. doi: 10.1126/science.2648570
14. Larsen CG, Anderson AO, Oppenheim JJ, Matsushima K. Production of Interleukin-8 by Human Dermal Fibroblasts and Keratinocytes in Response to Interleukin-1 or Tumour Necrosis Factor. Immunol (1989) 68:31–6.
15. Namba S, Nakano R, Kitanaka T, Kitanaka N, Nakayama T, Sugiya H. ERK2 and JNK1 Contribute to TNF-α-Induced IL-8 Expression in Synovial Fibroblasts. PloS One (2017) 12:e0182923. doi: 10.1371/journal.pone.0182923
16. Naruke A, Nakano R, Nunomura J, Suwabe Y, Nakano M, Namba S, et al. Tpl2 Contributes to IL-1β-Induced IL-8 Expression via ERK1/2 Activation in Canine Dermal Fibroblasts. PloS One (2021) 16:e0259489. doi: 10.1371/journal.pone.0259489
17. Hoffmann E, Dittrich-Breiholz O, Holtmann H, Kracht M. Multiple Control of Interleukin-8 Gene Expression. J Leukoc Biol (2002) 72:847–55.
18. Dinarello CA. A Clinical Perspective of IL-1β as the Gatekeeper of Inflammation. Eur J Immunol (2011) 41:1203–17. doi: 10.1002/eji.201141550
19. Mantovani A, Dinarello CA, Molgora M, Garlanda C. Interleukin-1 and Related Cytokines in the Regulation of Inflammation and Immunity. Immunity (2019) 50:778–95. doi: 10.1016/j.immuni.2019.03.012
20. Rathanaswami P, Hachicha M, Wong WL, Schall TJ, McColl SR. Synergistic Effect of Interleukin-1β and Tumor Necrosis Factor α on Interleukin-8 Gene Expression in Synovial Fibroblasts. Evidence That Interleukin-8 is the Major Neutrophil-Activating Chemokine Released in Response to Monokine Activation. Arthritis Rheumatol (1993) 36:1295–304. doi: 10.1002/art.1780360914
21. Sodin-Semrl S, Taddeo B, Tseng D, Varga J, Fiore S. Lipoxin A4 Inhibits IL-1β-Induced IL-6, IL-8, and Matrix Metalloproteinase-3 Production in Human Synovial Fibroblasts and Enhances Synthesis of Tissue Inhibitors of Metalloproteinases. J Immunol (2000) 164:2660–6. doi: 10.4049/jimmunol.164.5.2660
22. Lawrence T. The Nuclear Factor NF-κb Pathway in Inflammation. Cold Spring Harbor Pespect Biol (2009) 1:a001651. doi: 10.1101/cshperspect.a001651
23. Hayden MS, Ghosh S. NF-κb, the First Quarter-Century: Remarkable Progress and Outstanding Questions. Genes Dev (2012) 26:203–34. doi: 10.1101/gad.183434.111
24. Mitchell S, Vargas J, Hoffmann A. Signaling via the Nfκb System. Wiley Interdiscip Rev Syst Biol Med (2016) 8:227–41. doi: 10.1002/wsbm.1331
25. Nakano R, Edamura K, Nakayama T, Teshima K, Asano K, Narita T, et al. Differentiation of Canine Bone Marrow Stromal Cells Into Voltage- and Glutamate-Responsive Neuron-Like Cells by Basic Fibroblast Growth Factor. J Vet Med Sci (2015) 77:27–35. doi: 10.1292/jvms.14-0284
26. Nakano R, Edamura K, Nakayama T, Narita T, Okabayashi K, Sugiya H. Fibroblast Growth Factor Receptor-2 Contributes to the Basic Fibroblast Growth Factor-Induced Neuronal Differentiation in Canine Bone Marrow Stromal Cells via Phosphoinositide 3-Kinase/Akt Signaling Pathway. PloS One (2015) 10:e0141581. doi: 10.1371/journal.pone.0141581
27. Tsuchiya H, Nakano R, Konno T, Okabayashi K, Narita T, Sugiya H. Activation of MEK/ERK Pathways Through NF-κb Activation Is Involved in Interleukin-1β-Induced Cyclooxygenease-2 Expression in Canine Dermal Fibroblasts. Vet Immunol Immunopathol (2015) 168:223–32. doi: 10.1016/j.vetimm.2015.10.003
28. Konno T, Nakano R, Mamiya R, Tsuchiya H, Kitanaka T, Namba S, et al. Expression and Function of Interleukin-1β-Induced Neutrophil Gelatinase-Associated Lipocalin in Renal Tubular Cells. PloS One (2016) 11:e0166707. doi: 10.1371/journal.pone.0166707
29. Kitanaka T, Nakano R, Kitanaka N, Kimura T, Okabayashi K, Narita T, et al. JNK Activation is Essential for Activation of MEK/ERK Signaling in IL-1β-Induced COX-2 Expression in Synovial Fibroblasts. Sci Rep (2017) 7:39914. doi: 10.1038/srep39914
30. Nakano R, Kitanaka T, Namba S, Kitanaka N, Sugiya H. Protein Kinase Cϵ Regulates Nuclear Translocation of Extracellular Signal-Regulated Kinase, Which Contributes to Bradykinin-Induced Cyclooxygenase-2 Expression. Sci Rep (2018) 8:8535. doi: 10.1038/s41598-018-26473-7
31. Kitanaka N, Nakano R, Kitanaka T, Namba S, Konno T, Nakayama T, et al. NF-κb P65 and P105 Implicate in Interleukin 1β-Mediated COX-2 Expression in Melanoma Cells. PloS One (2018) 13:e0208955. doi: 10.1371/journal.pone.0208955
32. Kitanaka N, Nakano R, Sugiura K, Kitanaka T, Namba S, Konno T, et al. Interleukin-1β Promotes Interleulin-6 Expression via ERK1/2 Signaling Pathway in Canine Dermal Fibroblasts. PloS One (2019) 14:e0220262. doi: 10.1371/journal.pone.0220262
33. Kitanaka N, Nakano R, Sakai M, Kitanaka T, Namba S, Konno T, et al. ERK1/ATF-2 Signaling Axis Contributes to Interleukin-1β-Induced MMP-3 Expression in Dermal Fibroblasts. PloS One (2019) 14:e0222869. doi: 10.1371/journal.pone.0222869
34. Nakano R, Kitanaka T, Namba S, Kitanaka N, Sato M, Shibukawa Y, et al. All-Trans Retinoic Acid Induces Reprogramming of Canine Dedifferentiated Cells Into Neuron-Like Cells. PloS One (2020) 15:e0229892. doi: 10.1371/journal.pone.0229892
35. Nakano R, Kitanaka T, Namba S, Kitanaka N, Suwabe Y, Konno T, et al. Non-Transcriptional and Translational Function of Canonical NF-κb Signaling in Activating ERK1/2 in IL-1β-Induced COX-2 Expression in Synovial Fibroblasts. Front Immunol (2020) 11:579266. doi: 10.3389/fimmu.2020.579266
36. Suwabe Y, Nakano R, Namba S, Yachiku N, Kuji M, Sugimura M, et al. Involvement of GLUT1 and GLUT3 in the Growth of Canine Melanoma Cells. PloS One (2021) 16:e0243859. doi: 10.1371/journal.pone.0243859
37. Bradford MM. A Rapid and Sensitive Method for the Quantitation of Microgram Quantities of Protein Utilizing the Principle of Protein-Dye Binding. Anal Biochem (1976) 72:248–54. doi: 10.1016/0003-2697(76)90527-3
38. Kanda Y. Investigation of the Freely-Available Easy-to-Use Software “EZR” (Easy R) for Medical Statistics. Bone Marrow Transpl (2013) 48:452–8. doi: 10.1038/bmt.2012.244
39. Hammond ME, Lapointe GR, Feucht PH, Hilt S, Gallegos CA, Gordon CA, et al. IL-8 Induces Neutrophil Chemotaxis Predominantly via Type I IL-8 Receptors. J Immunol (1995) 155:1428–33.
40. Taub DD, Anver M, Oppenheim JJ, Longo DL, Murphy WJ. T Lymphocyte Recruitment by Interleukin-8 (IL-8). IL-8-Induced Degranulation of Neutrophils Releases Potent Chemoattractants for Human T Lymphocytes Both In Vitro and In Vivo. J Clin Invest (1996) 97:1931–41. doi: 10.1172/JCI118625
41. Heidemann J, Ogawa H, Dwinell MB, Rafiee P, Maaser C, Gockel HR, et al. Angiogenic Effects of Interleukin 8 (CXCL8) in Human Intestinal Microvascular Endothelial Cells are Mediated by CXCR2. J Biol Chem (2003) 278:8508–15. doi: 10.1074/jbc.M208231200
42. Abe Y, Kawakami M, Kuroki M, Yamamoto T, Fujii M, Kobayashi H, et al. Transient Rise in Serum Interleukin-8 Concentration During Acute Myocardial Infarction. Br Heart J (1993) 70:132–4. doi: 10.1136/hrt.70.2.132
43. Riesenberg K, Levy R, Katz A, Galkop S, Schlaeffer F. Neutrophil Superoxide Release and Interleukin 8 in Acute Myocardial Infarction: Distinction Between Complicated and Uncomplicated States. Eur J Clin Invest (1997) 27:398–404. doi: 10.1046/j.1365-2362.1997.1270667.x
44. Zarrouk-Mahjoub S, Zaghdoudi M, Amira Z, Chebi H, Khabouchi N, Finsterer J, et al. Pro- and Anti-Inflammatory Cytokines in Post-Infarction Left Ventricular Remodeling. Int J Cardiol (2016) 221:632–6. doi: 10.1016/j.ijcard.2016.07.073
45. Oz MC, Liao H, Naka Y, Seldomridge A, Becker DN, Michler RE, et al. Ischemia Induced Interleukin-8 Release After Human Heart Transplantation. A Potential Role for Endothelial Cells. Circulation (1995) 92:II428–32. doi: 10.1161/01.CIR.92.9.428
46. Wan S, Marchant A, DeSmet JM, Antoine M, Zhang H, Vachiery JL, et al. Human Cytokine Responses to Cardiac Transplantation and Coronary Artery Bypass Grafting. J Thorac Cardiovasc Surg (1996) 111:469–77. doi: 10.1016/S0022-5223(96)70458-0
47. Michael LH, Entman ML, Hartley CJ, Youker KA, Zhu J, Hall SR, et al. Myocardial Ischemia and Reperfusion: A Murine Model. Am J Physiol (1995) 269:H2147–54. doi: 10.1152/ajpheart.1995.269.6.H2147
48. Li Y, Wang Z, Hu Q, Yu D, Gao J, Yang L, et al. Anesthetic Postconditioning Plus Hypothermia Following Cardiopulmonary Resuscitation Protects the Myocardial Ultrastructure by Modulating Inflammatory Events in Rabbits. BioMed Rep (2017) 7:361–4. doi: 10.3892/br.2017.976
49. Hu L, Cai N, Jia H. Pterostilbene Attenuates Myocardial Ischemia-Reperfusion Injury via the Phosphatidylinositol 3’-Kinase-Protein Kinase B Signaling Pathway. Exp Ther Med (2017) 14:5509–14. doi: doi:10.3892/etm.2017.5246
50. Kukielka GL, Smith CW, LaRosa GJ, Manning AM, Mendoza LH, Daly TJ, et al. Interleukin-8 Gene Induction in the Myocardium After Ischemia and Reperfusion In Vivo. J Clin Invest (1995) 95:89–103. doi: 10.1172/JCI117680
51. Boyle EM Jr, Kovacich JC, Hèbert CA, Canty TG Jr, Chi E, Morgan EN, et al. Inhibition of Interleukin-8 Blocks Myocardial Ischemia-Reperfusion Injury. J Thorac Cardiovasc Surg (1998) 116:114–21. doi: 10.1016/S0022-5223(98)70249-1
52. Dinarello CA. Interleukin-1 in the Pathogenesis and Treatment of Inflammatory Diseases. Blood (2011) 117:3720–32. doi: 10.1182/blood-2010-07-273417
53. Dinarello CA, Simon A, van der Meer JW. Treating Inflammation by Blocking Interleukin-1 in a Broad Spectrum of Diseases. Nat Rev Drug Discovery (2012) 11:633–52. doi: 10.1038/nrd3800
54. Sager HB, Heidt T, Hulsmans M, Dutta P, Courties G, Sebas M, et al. Targeting Interleukin-1β Reduces Leukocyte Production After Acute Myocardial Infarction. Circulation (2015) 132:1880–90. doi: 10.1161/CIRCULATIONAHA.115.016160
55. Bujak M, Dobaczewski M, Chatila K, Mendoza LH, Li N, Reddy A, et al. Interleukin-1 Receptor Type I Signaling Critically Regulates Infarct Healing and Cardiac Remodeling. Am J Pathol (2008) 173:57–67. doi: 10.2353/ajpath.2008.070974
56. Abbate A, Kontos MC, Grizzard JD, Biondi-Zoccai GG, Van Tassell BW, Robati R, et al. Interleukin-1 Blockade With Anakinra to Prevent Adverse Cardiac Remodeling After Acute Myocardial Infarction (Virginia Commonwealth University Anakinra Remodeling Trial [VCU-ART] Pilot Study). Am J Cardiol (2010) 105:1371–7. doi: 10.1016/j.amjcard.2009.12.059
57. Beg AA, Ruben SM, Scheinman RI, Haskill S, Rosen CA, Baldwin AS Jr. Iκb Interacts With the Nuclear Localization Sequences of the Subunits of NF-κb: A Mechanism for Cytoplasmic Retention. Genes Dev (1992) 6:1899–913. doi: 10.1101/gad.6.10.1899
58. Ganchi PA, Sun SC, Greene WC, Ballard DW. Iκb/MAD-3 Masks the Nuclear Localization Signal of NF-κb P65 and Requires the Transactivation Domain to Inhibit NF-κb P65 DNA Binding. Mol Biol Cell (1992) 3:1339–52. doi: 10.1091/mbc.3.12.1339
59. Ferrari D, Wesselborg S, Bauer MK, Schulze-Osthoff K. Extracellular ATP Activates Transcription Factor NF-κb Through the P2Z Purinoreceptor by Selectively Targeting NF-κb P65. J Cell Biol (1997) 139:1635–43. doi: 10.1083/jcb.139.7.1635
60. Marui N, Medford RM, Ahmad M. Activation of RelA Homodimers by Tumour Necrosis Factor α: A Possible Transcriptional Activator in Human Vascular Endothelial Cells. Biochem J (2005) 390:317–24. doi: 10.1042/BJ20041659
61. Karin M. How NF-κb is Activated: The Role of the Iκb Kinase (IKK) Complex. Oncogene (1999) 18:6867–74. doi: 10.1038/sj.onc.1203219
62. Podolin PL, Callahan JF, Bolognese BJ, Li YH, Carlson K, Davis TG, et al. Attenuation of Murine Collagen-Induced Arthritis by a Novel, Potent, Selective Small Molecule Inhibitor of Iκb Kinase 2, TPCA-1 (2-[(Aminocarbonyl)Amino]-5-(4-Fluorophenyl)-3-Thiophenecarboxamide), Occurs via Reduction of Proinflammatory Cytokines and Antigen-Induced T Cell Proliferation. J Pharmacol Exp Ther (2005) 312:373–81. doi: 10.1124/jpet.104.074484
63. Fujimoto K, Yasuda H, Sato Y, Yamamoto K. A Role for Phosphorylation in the Proteolytic Processing of the Human NF-κb1 Precursor. Gene (1995) 165:183–9. doi: 10.1016/0378-1119(95)00507-3
64. Heissmeyer V, Krappmann D, Wulczyn FG, Scheidereit C. NF-κb P105 is a Target of Iκb Kinases and Controls Signal Induction of Bcl-3-P50 Complexes. EMBO J (1999) 18:4766–78. doi: 10.1093/emboj/18.17.4766
65. Heissmeyer V, Krappmann D, Hatada EN, Scheidereit C. Shared Pathways of Iκb Kinase-Induced SCF(βtrcp)-Mediated Ubiquitination and Degradation for the NF-κb Precursor P105 and Iκbα. Mol Cell Biol (2001) 21:1024–35. doi: 10.1128/MCB.21.4.1024-1035.2001
66. Salmerón A, Janzen J, Soneji Y, Bump N, Kamens J, Allen H, et al. Direct Phosphorylation of NF-κb1 P105 by the Iκb Kinase Complex on Serine 927 is Essential for Signal-Induced P105 Proteolysis. J Biol Chem (2001) 276:22215–22. doi: 10.1074/jbc.M101754200
67. Lang V, Janzen J, Fischer GZ, Soneji Y, Beinke S, Salmeron A, et al. βtrcp-Mediated Proteolysis of NF-κb1 P105 Requires Phosphorylation of P105 Serines 927 and 932. Mol Cell Biol (2003) 23:402–13. doi: 10.1128/MCB.23.1.402-413.2003
Keywords: cardiac fibroblasts, inflammation, interleukin 1β, interleukin 8, NF-κB p65
Citation: Mizuno M, Nakano R, Nose S, Matsumura M, Nii Y, Kurogochi K, Sugiya H and Uechi M (2022) Canonical NF-κB p65, but Not p105, Contributes to IL-1β-Induced IL-8 Expression in Cardiac Fibroblasts. Front. Immunol. 13:863309. doi: 10.3389/fimmu.2022.863309
Received: 27 January 2022; Accepted: 15 March 2022;
Published: 20 April 2022.
Edited by:
Nicole Horwood, University of East Anglia, United KingdomReviewed by:
Paramananda Saikia, Milliporesigma, United StatesCeren Ciraci, Istanbul Technical University, Turkey
Copyright © 2022 Mizuno, Nakano, Nose, Matsumura, Nii, Kurogochi, Sugiya and Uechi. This is an open-access article distributed under the terms of the Creative Commons Attribution License (CC BY). The use, distribution or reproduction in other forums is permitted, provided the original author(s) and the copyright owner(s) are credited and that the original publication in this journal is cited, in accordance with accepted academic practice. No use, distribution or reproduction is permitted which does not comply with these terms.
*Correspondence: Hiroshi Sugiya, c3VnaXlhLmhpcm9zaGlAbmlob24tdS5hYy5qcA==
†These authors have contributed equally to this work