- 1Pediatric Scientist Development Program, University of Pittsburgh Medical Center (UPMC) Children’s Hospital of Pittsburgh, Pittsburgh, PA, United States
- 2Division of Allergy/Immunology, University of Pittsburgh Medical Center (UPMC) Children’s Hospital of Pittsburgh, Pittsburgh, PA, United States
- 3Medical Scientist Training Program, University of Pittsburgh, Pittsburgh, PA, United States
- 4Division of Pediatric Infectious Diseases, University of Pittsburgh Medical Center (UPMC) Children’s Hospital of Pittsburgh, Pittsburgh, PA, United States
Respiratory tract infections are a leading cause of morbidity and mortality in newborns, infants, and young children. These early life infections present a formidable immunologic challenge with a number of possibly conflicting goals: simultaneously eliminate the acute pathogen, preserve the primary gas-exchange function of the lung parenchyma in a developing lung, and limit long-term sequelae of both the infection and the inflammatory response. The latter has been most well studied in the context of childhood asthma, where multiple epidemiologic studies have linked early life viral infection with subsequent bronchospasm. This review will focus on the clinical relevance of respiratory syncytial virus (RSV), human metapneumovirus (HMPV), and rhinovirus (RV) and examine the protective and pathogenic host responses within the neonate.
Respiratory Viral Infections in the Neonatal Population
Global studies estimate that over 100 million lower respiratory tract infections occur annually in children under the age of 5, accounting for 700,000-900,000 deaths per year in this age group (1). The estimated RSV and HMPV lower respiratory tract infection burden in this age group is approximately 33.1 million and 14.2 million cases per year, respectively (2, 3). For context, estimates of influenza lower respiratory tract infection in children under the age of 5 is 10.1 million cases per year (4). RV lower respiratory tract infection burden has not been quantified on a global scale; however, at least in hospitalized children, RV has been shown to be a common cause of bronchiolitis (5–7).
RSV is a negative-sense single-stranded RNA virus in the Pneumoviridae family (8). RSV cases typically peak in the winter months in temperate climates (9). RSV is a common pathogen in young children, with one study estimating the infection rate as 68.8/100 children under the age of 1 year with near ubiquitous exposure by 2 years (10). RSV infection typically presents with upper respiratory symptoms, with progression to lower respiratory symptoms in approximately 40% of cases (11, 12). While progression to bronchiolitis requiring hospitalization is relatively rare (1-2% of all RSV cases), RSV accounts for nearly 70% of all hospitalizations for bronchiolitis in the United States (13, 14). Global estimates of RSV disease are staggering, with 3.2 million hospital admissions and 59,600 in-hospital deaths in 2015; 45% of hospitalizations (~1.4 million) and deaths (~27,300) were in infants under the age of 6 months (2). Premature infants are at especially high risk, with one meta-analysis indicating 3-fold higher risk of hospitalization in premature compared to term infants (15).
HMPV is a negative-sense single-stranded RNA virus first isolated from children with lower respiratory tract infection by Dutch investigators in 2001 (16). HMPV is also a member of the Pneumoviridae family (16–18). HMPV largely occurs in the winter and early spring months in the United States, typically 1-2 months after the peak of RSV season (19–22). Most children are infected with HMPV within the first years of life, although re-infection can occur frequently with either heterologous or homologous strains of HMPV (22, 23). HMPV infection most commonly presents in children with fever, rhinorrhea, and cough, while wheezing on presentation has been reported in approximately 50% of pediatric cases (22, 24, 25). One prospective multicenter surveillance study demonstrated that HMPV causes 7% of sick clinic and emergency department visits for children <5 years of age (26). In 2018, HMPV infections globally in children under the age of 5 years contributed to approximately 500,000 hospital admissions and 11,300 deaths (3). Infants had a disproportionately high risk of hospitalization and death—a trend that was magnified in low-income or low-middle-income countries (3). Infants with history of prematurity also have increased susceptibility to hospitalization and severe HMPV disease (26–29).
Human rhinoviruses are positive-sense single-stranded RNA virus in the family Picornaviridae and genus Enterovirus (30–32). Rhinovirus (RV) infection most commonly occurs in the fall with a smaller peak in the spring (33, 34). The mean age for first symptomatic RV infection is approximately 6 months, with re-infection via heterologous strains occurring frequently (35, 36). RV infection has a range of presentations: asymptomatic (occurring frequently in young children), upper respiratory tract infection (known colloquially as the ‘common cold’), and lower respiratory tract infection (37–42). However, RV is a frequent cause of lower respiratory tract infection in infants and toddlers, causing common presentations of bronchiolitis, pneumonia, and wheezing episodes (43–50). Hospitalization among children with RV was disproportionately seen in infants <6 months, with RV infection accounting for ~5 out of every 1,000 hospitalizations in this age range (51). Like RSV and HMPV, RV infection can cause severe disease in premature infants; in fact, nosocomial outbreaks of RV have been described in neonatal intensive care units (52–54).
Collectively, RSV, HMPV, and RV represent three leading causes of respiratory morbidity and mortality in young children, highlighting the need to understand host-pathogen interactions in the lung that put these patients at higher risk of poor outcomes. The unique immunologic functions of the neonatal lung in homeostasis shape the responses to these three pathogens.
Lung Development and Prenatal Immunity
Structurally, the lungs in utero undergo three major developmental stages characterized by histologic appearance: pseudoglandular, canalicular, and terminal saccular stages (55). When the lungs spring open with the newborn’s first cry, the primary functions of ventilation and oxygenation begin as residual amniotic fluid is absorbed. However, for the next 4 weeks in mice and the next 1-2 years in humans, the last stage of development, alveolarization, occurs. This process is marked by increased branching and budding of alveoli, exponentially increasing the surface area of cells capable of performing gas-exchange (55).
As infectious threats to the newborn lung can be present from that first breath, it is unsurprising that development of the immune system within the lung occurs early in embryonic development (56). Immune cells, particularly embryonic macrophages and dendritic cells, can be found in the lung as early as embryonic day(E) 9.5 in mice and E35 in humans (57, 58). NK cells are present early in fetal development in the murine liver and spleen at E14.5 and 15.5, respectively (59). Shortly after, γδ T cells develop in the embryonic thymus and are important sources of prenatal IL-17 (60, 61). Proteins like mucins (e.g. Muc5b) and surfactants (e.g. SP-A) are released from the prenatal lung into the amniotic fluid and both substances have immunomodulatory properties (62–66).
Overview of Lung Innate Immunity in the Neonate
Shortly after birth, the composition of the innate immune system in the lung changes. Single-cell RNA sequencing of post-natal day 1 mice demonstrate the presence of 5 unique subsets of macrophages/monocytes and 3 subsets of dendritic cells (DCs), as well as mast cells, basophils, and neutrophils (67). Interestingly, the monocytes present in the murine lung prenatally differentiate into long-lived alveolar macrophages within the first week of life in a GM-CSF-dependent fashion (68). Following LPS stimulation, murine neonatal macrophages have increased production of IL-10 (an anti-inflammatory cytokine); IL-1, IL-6 and TNF production were significantly decreased following LPS stimulation, potentially due to decreased TLR2 and TLR4 expression (Figure 1) (69). Between postnatal day 3-21 in mice, macrophages in the neonatal lung show increased polarization towards an M2-like macrophage phenotype, which is temporally associated with alveolarization (70). In contrast, phagocytic function and bactericidal capabilities of neonatal macrophages isolated from human cord blood are comparable to that of adult cells (71, 72). Human cord blood derived macrophages stimulated with LPS do not decrease their oxygen consumption, indicating an impaired ability to modulate metabolism and effectively activate macrophages, while adult macrophages downregulate oxidative phosphorylation, thus shifting to Warburg metabolism (73).
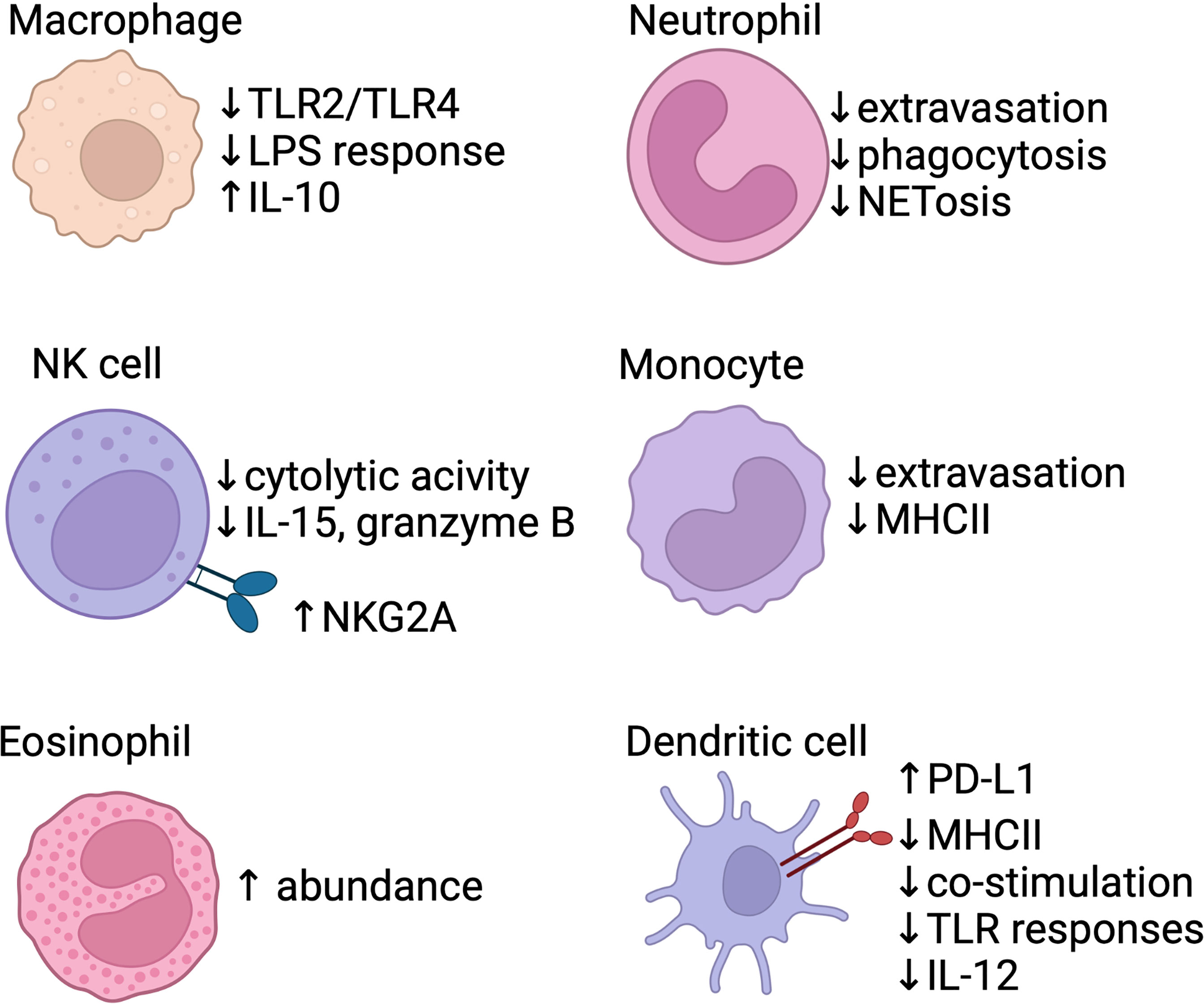
Figure 1 Baseline differences in the neonatal innate immune system. Several areas of the innate response are blunted in neonates, including macrophage response to TLR signals, neutrophil/monocyte extravasation, and poor function of antigen-presenting cells (monocytes/dendritic cells). Anti-inflammatory signals are also produced by the innate system, with IL-10 production via macrophages. Dendritic cells express PD-L1, a co-inhibitory receptor, shortly after birth and produce less pro-inflammatory IL-12. NK cells show reduced effector function and increased expression of inhibitory receptors (e.g. NKG2A). Eosinophils accumulate in the lung within the first two weeks of life in mice. Created with biorender.com.
Human cord blood derived macrophages and monocytes are also less responsive to IFN-γ compared to adult cells (74). In humans, circulating monocytes in the newborn exhibit decreased MHC class II expression, which leads to impaired antigen presenting capacity in tissues (75). Cord blood monocytes have an intrinsic impairment in extravasation under homeostatic conditions when compared to adult monocytes, although activation of endothelial cells by inflammation can improve neonatal monocyte migration (76).
In the newborn mouse, absolute number and function of DCs are decreased compared to the adult lung (77, 78). Neonatal lung DCs in mice promote tolerance by upregulating PD-L1 expression shortly after birth in a microbiota-dependent fashion (79). Human cord blood derived DCs have decreased expression of MHC class II and co-stimulatory molecules such as CD25, CD83, and CD86 and exhibit blunted responses to LPS (80, 81). Following LPS stimulation, human neonatal conventional DCs (cDCs) have impaired transcription of IL12-p35, a subunit of the Th1-potentiating cytokine IL-12p70, although this expression was restored by exogenous IFN-γ administration (82, 83). Similarly, stimulation of human cord blood DCs with TLR7 and TLR9 agonists demonstrated defective type I interferon responses (84, 85). Interestingly, murine neonatal DCs may have a constrained response to TLR stimulation in part due to IL-10 production by CD5+ B cells, demonstrating the complex interplay of neonatal innate and adaptive immunity (86, 87). In a human cohort followed prospectively, IFN-α production by DCs increased to adult levels by 1 year of age, IL-10 production declined to adult levels by 2 years, while IL12-p70 secretion remained decreased at 2 years (88). While similarities in decreased co-stimulatory molecule expression and blunted inflammatory responses in DCs have been characterized, other differences between murine and human DCs responses to specific stimulations have been reviewed elsewhere (89). More recently, fate-mapping and sequencing based techniques in mice have demonstrated that cDC2s derived early in life have differing responses to pathogens compared to the adult cDC2s due to an altered cytokine milieu in the lung, rather than inherent pre-programmed differences (90).
Although absolute numbers of neutrophils are comparable to that of older children and adults, neonatal neutrophils exhibit poor chemotaxis, endothelial adhesion, and phagocytic function (91–93). Human neonatal neutrophils neither produce neutrophil extracellular traps (NETs) nor respond to Fas-mediated apoptosis as effectively as adult neutrophils (94–97). One study also identified a unique group of myeloid suppressor cells with a neutrophilic/granulocytic phenotype (Gr-MDSC) in human cord blood; this cell population was found to suppress NK cell activation and T cell polarization to effector subsets (98).
NK cells in the human lung exhibit a selective tolerogenic phenotype where they retain their ability to generate strong antibody-dependent cell-mediated cytotoxicity (ADCC) responses similarly to adult NK cells, but are unable to mount a response against cells displaying non-self peptide or lacking MHC class I receptor (99). Analysis of human cord blood revealed that NK cells have increased expression of inhibitory receptors (e.g. NKG2A/CD94) and decreased expression of activation receptors (e.g. leukocyte immunoglobulin-like receptor (LIR)-1), leading to impaired recognition of MHC-I negative cells (100–102). Human cord blood derived NK cells also had decreased toll-like receptor 3 (TLR3) expression and failed to mount a response to polyinosinic-polycytidylic acid (poly(I:C)), a synthetic viral double-stranded RNA viral pathogen-associated molecular pattern, but paradoxically had increased IFN-γ release with TLR8 stimulation (103, 104). NK cells isolated from cord blood also had a 3-fold lower capacity for cytolytic activity and decreased degranulation and cytokine production (e.g. IL-15, IL-2, granzyme B, and IFN-γ) compared to adult NK cells (100, 105–107). Maturation of neonatal murine NK cells into adult-like NK cells was constrained by TGF-β signaling (108).
While comparing murine-derived data from lung tissue with peripherally acquired human cells presents a challenge, a pattern of anti-inflammatory and tolerogenic responses from neonatal innate immune cells emerges. Stimuli that would normally initiate a robust inflammatory response in adult models, such as LPS or other pathogen-associated molecular patterns, have blunted effects on neonatal dendritic cells and macrophages (69, 73, 82–86). Further, innate immune cells in the neonate contribute to the local anti-inflammatory milieu by production of IL-10 with suppression of ‘typical’ inflammatory cytokines (e.g. IL-12), upregulation of co-inhibitory receptor (e.g. PD-L1) expression, and reduced co-stimulatory receptor expression (69, 79–83, 88).. Collectively, these alterations not only stifle an inflammatory innate response in the neonatal lung, but also effect downstream adaptive immunity as well.
Overview of Lung Adaptive Immunity in the Neonate
Although once thought to be in a relative state of immunosuppression due to poor adaptive immunity, recent studies clearly demonstrate the presence of a more nuanced adaptive immune response in the neonate (109–112). Single cell RNA sequencing demonstrated T cell and B cell numbers increase in the murine neonatal lung on the first day of life (67, 113). This early arrival in the lungs is not necessarily in response to a stimulus, but reflective of the fact that neonatal naïve T cells extravasate into end-organ sites to a higher degree than adult naïve T cells (114–116). Following trafficking to the lung, the adaptive immune system of the neonate has strikingly different properties than adult counterparts (117, 118). This concept has been described as a ‘layered immunity,’ as neonatal B and T cells have functional differences that phenotypically distinguish these cells (119–123)..
While CD8+ T cells are present in the murine newborn lung at a relatively low abundance, exposure to pathogens can lead to rapid induction of CD8+ T cells (67, 113, 124, 125). Neonatal CD8+ T cells demonstrate greater proliferative capabilities with rapid terminal differentiation at the expense of memory formation (Figure 2) (123, 126–128). From a functional perspective, neonatal CD8+ T cells demonstrate decreased cytotoxicity but increased innate-like characteristics, such as antimicrobial peptide production and reactive oxygen species formation (118, 129). Furthermore, antigen-naïve murine CD8+ T cells in the neonate can secrete IFN-γ in response to cytokine stimulation alone, suggesting less reliance on TCR signaling (118, 123). IL-12 in particular appears to be a key cytokine in changing the epigenetic landscape of human neonatal CD8+ T cells, shifting functionality towards a more ‘adult’ phenotype (130, 131).
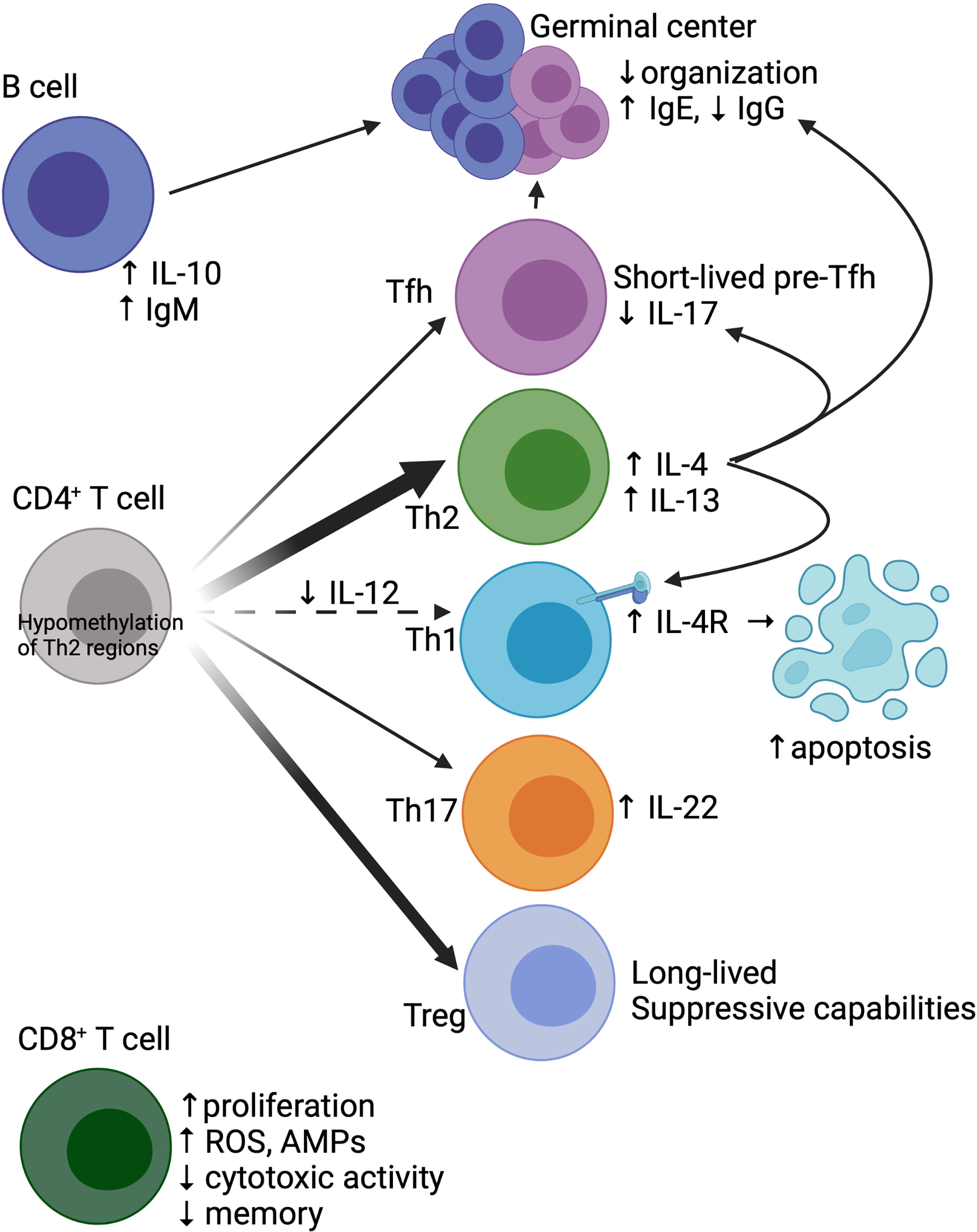
Figure 2 Baseline differences in the neonatal adaptive immune system. The neonatal CD4+ T cell compartment is skewed towards Th2 (due to hypomethylation of critical Th2 regulatory regions) and Treg development. There is less differentiation towards Th1 due to reduced IL-12 in the milieu, coupled with increased Th1 apoptosis due to IL-4 signaling. Tfh cells, while stimulated by IL-4 to differentiate, have arrested development, with generation of short-lived pre-Tfh cells. IL-4 signaling on Tfh cells also limits IL-17 production and skews the humoral response towards IgE production. Both neonatal Tfh and B cells have poor migration to germinal centers, which structurally demonstrate poor organization. B cells also have increased production of IL-10 and spontaneous secretion of IgM. CD8+ T cells show radically different properties in neonates compared to adults, with increased proliferation, generation of reactive oxygen species (ROS) and antimicrobial peptides (AMPs), and reduced cytotoxicity and memory formation. Created with biorender.com.
Similar to the findings of neonatal CD8+ T cells, neonatal CD4+ T cells have greater proliferative capabilities (117, 132), a more restrictive T cell receptor (TCR) repertoire with bias towards self-reactive TCRs (133–135), and are more likely to become terminally differentiated rather than form a memory population (136). However, neonatal CD4+ T cells have significant heterogeneity in differentiation states (e.g. subsets) and effector function. Compared to adult cells, murine CD4+ T cells found after birth skew towards Th2 and away from Th1 cells (Figure 2) (137–139). Neonatal CD4+ T cells produce an abundance of IL-4 and IL-13 after activation, in part due to hypomethylation of a key murine Th2 cytokine regulatory region (CNS-1) and an open IL-13 locus resistant to transcriptional repression in human cells (140–142). This epigenetic pattern in T cells in mice changes rapidly post-birth, reaching adult-like epigenetic patterns within 6 days of life and thus contributing to this notion of a “critical window” of Th2 bias (143). In murine Th1 cells, lack of IL-12 in the milieu leads to upregulation of IL-13Rα1, allowing for IL-4 signaling through the IL-4 receptor which results in induction of apoptosis, furthering the imbalance of Th2/Th1 cells (144–146).
In addition to favoring the Th2 subset, naïve T cells from the neonate preferentially differentiate into regulatory T cells (Tregs) (117, 132). Using a fate-mapping Foxp3 mouse, Tregs generated early in life in the lung demonstrated suppressive activity that was maintained into adulthood (147). Similarly, in humans, Tregs directed against maternal alloantigens could be detected in children 7-17 years old, suggesting a long-lived functionality of neonatal Tregs (148). To a lesser extent than Th2/Treg skewing, one murine fate-mapping model showed increased neonatal T cell differentiation towards Th17 cells (149). Human cord blood derived T cells cultured in Th17-polarizing conditions produced higher levels of IL-22, an anti-inflammatory cytokine designed at maintaining epithelial barriers, when compared to adult T cells (150).
T follicular helper (Tfh) cells, a Bcl6-dependent CD4+ T cell subset that specializes in facilitating germinal center reactions and B cell development, also show altered function in neonates (151). In studies of neonatal immunization, induction and migration of Tfh cells were impaired compared to adult mice (152, 153). Further, impaired Tfh cell function in neonates led to reduced germinal center quantity, less differentiation of B cells towards plasma cells, and reduced antigen-specific IgG production when compared to adult mice (152). While neonatal Tfh cells demonstrate some degree of intrinsic dysfunction, the extrinsic milieu in the neonate also plays a factor; for instance, IL-4 seems to promote Tfh cell development and localization to germinal centers, while simultaneously constraining IL-17 production by Tfh cells and skewing the humoral response towards IgE production (152, 153). Using microarray, a more recent study demonstrated that neonatal Tfh cells can initiate the transcriptional program associated with Tfh development, but preferentially differentiate into short-lived pre-Tfh cells more so than adult counterparts (154). Further, these neonatal Tfh cells also expressed a signature more classically associated with Th2 cells, such as increased expression of IL-13 and transcription factors associated with Th2 development (154).
While the diminished function of Tfh cells in neonates contributes to the changes in the humoral response, there are also key differences between neonatal and adult B cells. The murine and human neonatal B cell compartment is largely composed of a first layer of B-1 B cells arising from the embryonic yolk sac and fetal liver, compared to mature B-2 cells in the adult (120). Murine B-1 cells, unlike B-2 cells, do not require IL-7 or B cell activating factor (BAFF) for development and are more responsive to TSLP (155, 156). Neonatal B cells in mice also have low levels of activation induced cytidine deaminase (AID) leading to lower affinity antibody responses (157). Human cord blood derived B-1 cells have a more restricted immunoglobulin repertoire, spontaneously secrete IgM, and exhibit limited somatic hypermutation (158). Passive humoral immunity, though, is provided via transplacental transfer of high-titer maternal antibodies, which occurs in humans during the third trimester of pregnancy (159–161).
Taken together, these studies not only indicate that the neonatal lung has a coordinated first layer of adaptive immunity at baseline, but are also identifying the mechanistic differences between neonatal and adult adaptive immunity. The evolutionary reasons for these altered responses remains an active question. Considering the altered CD4+ T cell response as a prototypical example, the Th2-predominance may be critical for lung development, as evidenced by the temporal association of polarization of M2-like macrophages and recruitment of eosinophils with alveolarization and primary septation, respectively (70, 162). Additionally, there is a theological argument to be made for limiting a potentially inflammatory and cytotoxic Th1 response in area of active development. While sustaining a milieu favoring Th2 vs. Th1 cells in the neonatal lung under physiologic conditions presumably confers some evolutionary advantage to the host, understanding this baseline difference—along with the others discussed in these last two sections—informs how the neonatal immune system responds in the face of a viral infection.
Neonatal Immune Responses to Respiratory Viral Infection in Preclinical Models
Several neonatal animal models exist to study the host-pathogen interactions of respiratory viruses, with the most extensive body of literature surrounding neonatal RSV infection.
RSV infection of newborn mice resulted in prolonged viral replication when compared to adult mice, although neonates are capable of controlling and clearing infection (163, 164). Neonatal epithelial cells release IL-33 and TSLP in greater magnitude following RSV infection compared to adult mice (Figure 3) (165, 166). Early mediators of antiviral response such as recruitment of plasmacytoid DCs (pDCs) and production of IFN-α and IFN-β were deficient in neonatal mice infected with RSV (167). cDC1s were adequately recruited to the lung, but failed to respond to type I IFNs and upregulate co-stimulatory molecules (e.g. CD80/CD86) required for proficient CD8+ T cell response following RSV infection in mice (168). cDCs from human cord blood infected with RSV similarly had poor co-stimulatory molecule expression (168). Antigen-specific CD8+ T cells were induced in neonatal mice following RSV infection but were less likely to produce IFN-γ (169). This lack of IFN-γ production leads to a delayed and blunted classically activated macrophage response in neonatal mice (163). TLR4 or TLR9 agonist treatment increased co-stimulatory molecule expression on murine cDCs and restored CD8+ T cell responses to adult levels (170). Interestingly, the CD8+ T cell repertoire following RSV infection is significantly different in neonatal mice with variations in epitope-specific Vβ repertoire usage and lower functional avidity, a finding that resolves within 2 weeks of life, highlighting a critical window for altered CD8+ T cell responses (164).
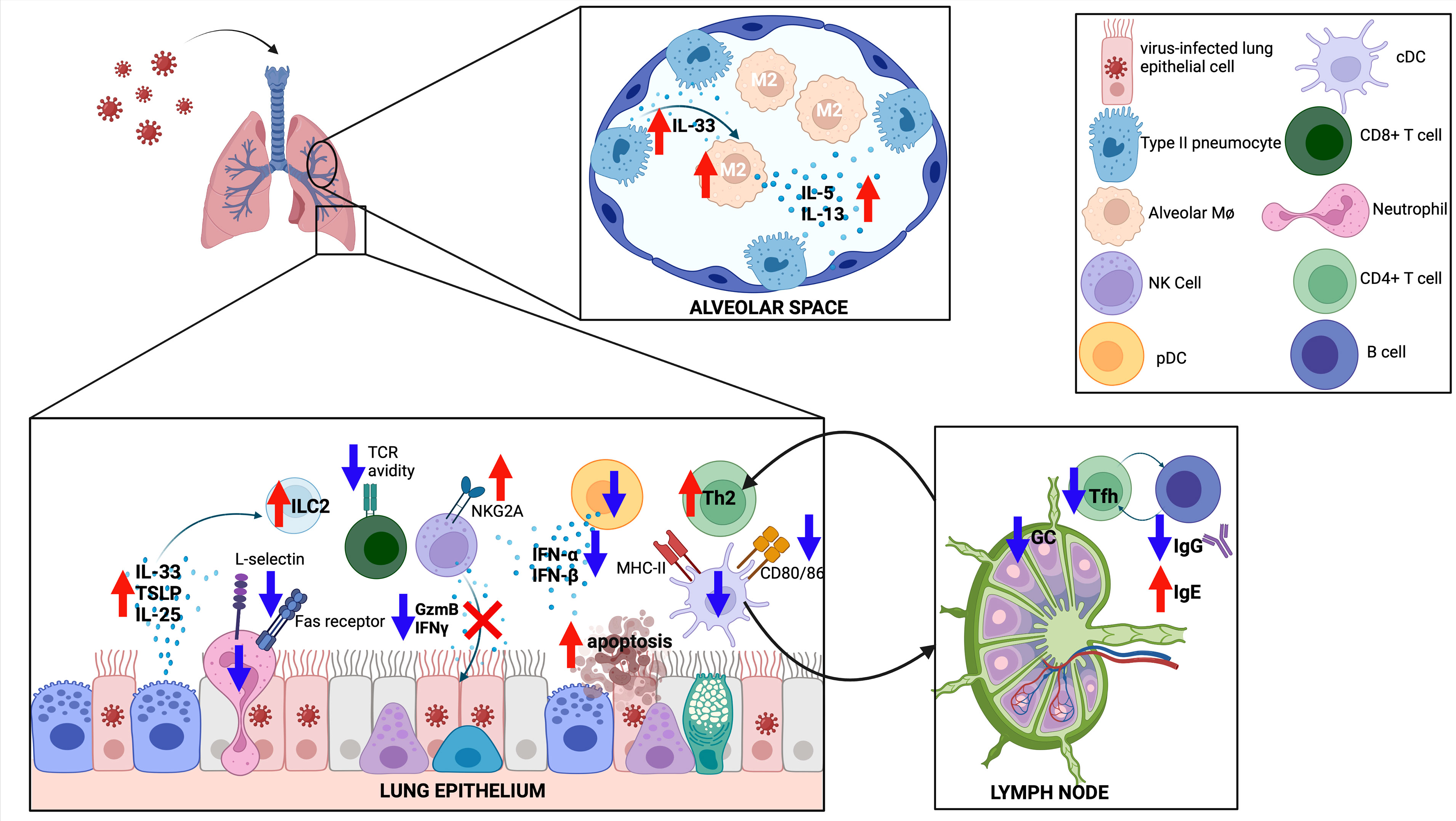
Figure 3 The immune response to respiratory viruses in neonates. The neonatal response favors a type II response, with increased IL-33, IL-25 and TSLP released from the epithelium, increased type 2 innate lymphoid cells (ILC2), increased type 2 helper T cells (Th2), and differentiation of M2-like alveolar macrophages (M2). CD8 T cells have reduced T cell receptor (TCR) avidity, while both CD8 T cells and natural killer (NK) cells show reduced effector functions. Germinal center (GC) reactions are diminished in neonates, with less T follicular helper (Tfh) cell differentiation and less IgG production from GC B cells. Created with biorender.com.
Neonatal RSV infection in mice was associated with recruitment of CD4+ T cells with a Th2 bias (171). Compared to adult mice, the CD4+ T cell compartment of neonatal mice showed increased proliferative capacity, expression of IL-4Rα, and differentiation into Th2 cells following RSV infection (172). Using a lamb model, neonatal RSV infection further increased Th2 cytokine production in the setting of an elevated baseline Th2 milieu (173). This skewing towards type 2 inflammation (e.g. increased ILC2s and Th2s) was due to rapid and increased production of IL-33 in neonatal mice (165, 174). Consistent with a Th1/Th2 imbalance, neonatal mice had fewer Th1 CD4+ cells in the bronchoalveolar lavage fluid when compared to adult mice (171).
RSV infection of 7-day-old mice resulted in long-standing pathophysiologic changes such as reduced lung function, chronic inflammation, and airway remodeling; synergistic changes were noted in mice sensitized to OVA after prior neonatal RSV infection (175). Re-infection 4-6 weeks after initial neonatal infection led to an exaggerated Th2 response and increased airway hyperresponsiveness when compared to weanling-aged or adult-aged mice infected twice with RSV (172, 176). Mechanistically, neonatal mice with repeated exposure to RSV demonstrated a break in tolerance to an antigen (OVA) present in maternal breastmilk, increasing susceptibility to allergic airway disease (177). It should also be noted that RSV strain virulence could also play a role in mediating pathology, as a chimeric RSV A2 strain carrying the F protein from a clinical isolate induced a more robust Th2 response and subsequent lung pathology (178).
Murine Th2 responses following neonatal RSV infection contribute to pathology. CD4+ T cell-specific deletion of IL-4Rα led to reduced Th2 responses and reduced airway hyperresponsiveness following secondary exposure (172). Similarly, administration of an IL-4Rα antisense oligonucleotide during the initial neonatal infection mitigated Th2-mediated pathology with subsequent RSV exposure (179). Interestingly, administration of recombinant IFN-α during primary infection led to reduced pathology with re-infection, in part by limiting increased IL-4Rα on Th2 cells (167). Amelioration of altered airway responses after secondary infection could also be achieved with administration of an IL-13Rα2 fusion protein, blockade of IgE, blockade of IL-33 signaling, or inhibition of STAT6 during the primary infection (165, 176, 180, 181).
The lack of a strong Th1 response in neonates also may contribute to pathology. RSV-infected human cord blood DCs produce increased TGF-β, an anti-inflammatory cytokine that in this context limits IL-12p70 production (and subsequent Th1 differentiation), compared to RSV-infected DCs derived from adults (182). Recombinant IFN-γ therapy diminished the number of GATA3+ CD4+ T cells (Th2) and increased antigen-specific CD8+ T cell recruitment to BAL fluid (171). Recombinant IFN-γ treatment in mice activates classically activated and alveolar macrophages and leads to improved RSV clearance (163, 183). Likewise, neonatal infection with a recombinant RSV strain expressing IFN-γ led to improved viral clearance with primary infection and reduced pathology upon secondary infection (184). Collectively, these studies suggest that diminishing an exuberant Th2 response or enhancement of a deficient Th1 response can limit RSV-induced pathology.
Type 17 immunity has been implicated in protection and pathology in neonatal RSV infection. To recapitulate the clinical phenomenon of infants with CX3CR1 gene variants having worse outcomes with RSV infection, Das et al. evaluated neonatal RSV infection in mice lacking this chemokine receptor (185, 186). Cx3cr1 knockout mice also had worsened pathology dependent on increased IL-17 from γδ T cells (185). Administration of IL-22, a cytokine produced by Th17 cells, Th22 cells, and others to neonatal mice infected with RSV led to faster reduction in RSV burden by limiting RSV-mediated subversion of autophagy (187). RSV infection of a subgroup neonatal B cells named B regulatory cells (nBregs) derived from human cord blood occurs through interaction with the chemokine receptor CX3CR1; infection of nBregs led to secretion of IL-10, further blunting the Th1 response (188).
From a humoral perspective, neonatal mice produced RSV-specific IgE after infection, consistent with a Th2 bias (181). However, neonatal mice had a less robust RSV-specific neutralizing IgG response compared to adult mice (189). Interestingly, depletion of CD4 and CD8 T+ cells, NK cells, or IFN-γ blockade led to enhanced RSV-specific antibody production in the neonates (189). The poor antibody response in neonates was the result of poor germinal center activity and deficient differentiation of CD4+ T cells to the follicular helper (Tfh) subset (190). Mice lacking Tfh cells had increased pathology with re-exposure to RSV, while blockade of IL-2 led to increased Tfh number, reduced RSV-driven pathology, and increased RSV-specific IgG production (190). Interestingly, administration of recombinant IFN-α during neonatal RSV infection led to increased B cell trafficking to the lung, increased B-cell activating factor (BAFF) and a proliferation-inducing ligand (APRIL) expression, and increased RSV-specific IgA (191).
Another member of the Pneumoviridae family, pneumonia virus of mice (PVM), has been utilized as a murine model of early life lower respiratory tract infection (192, 193). Unlike RSV or HMPV, however, PVM is a natural murine pathogen; this allows for the investigation of infection dynamics and the resultant immune response in context of a natural host-pathogen dyad (192). Although kinetics of PVM infection were similar between neonatal and adult mice, neonatal mice had markedly reduced pro-inflammatory mediator production and leukocyte recruitment (194). In a model of severe bronchiolitis using IRF7-deficienct mice, neonatal infection with PVM led to release of IL-33 and HMGB1 (high-mobility group box 1), another nuclear alarmin, both of which contributed to ILC2 proliferation, type 2 inflammation, and airway remodeling (195). Similar to neonatal models of RSV, early life PVM coupled with exposure to an allergen led to Th2-driven phenotype mirroring asthma (196, 197).
The relationship between neonatal infection and type 2 inflammation has also been well characterized in a neonatal RV model. RV infection in mice at DOL7 led to detectable viral RNA for 7 days, accompanied by inflammatory cell infiltration and upregulation of IL-13 in the acute phase (198). However, pathology persisted 4 weeks after initial exposure, as mice with neonatal RV infection had exaggerated airway hyperresponsiveness compared to age-matched uninfected controls and RV-infected adult mice (198). Interestingly, these late-phase pathologic effects were mitigated by anti-IL-13 treatment in the immediate post-infectious period (198). Neonatal RV infection resulted in induction of IL-33, IL-25, and TSLP–all of which are epithelial derived cytokines implicated in asthma pathogenesis–and in this model contributed to type 2 innate lymphoid cells (ILC2) expansion (199–201). Neonatal infection with RV followed by a challenge with a heterologous strain of RV lead to increased expansion of this pathologic ILC2 population, perhaps serving as a murine model of the clinical response in children with frequent RV re-exposure (202). Additionally, sensitization to an unrelated antigen (e.g. OVA) followed by antigen challenge led to worsening airway hyperresponsiveness in mice with a history of neonatal RV infection (198). There were strain differences observed as well, as neonatal RV-C infection led to an enhanced type 2 response when compared to RV-A infection, in part due to poor inflammasome activation and decreased IL-1β production in the RV-C infected animals (203). Mitigation of this strong type 2 inflammatory response could be achieved with recombinant IFN-γ treatment, demonstrating a reciprocal relationship between type 1 and type 2 immunity (204).
Limited preclinical studies exist on neonatal HMPV infection, although unpublished data generated in our laboratory suggest neonatal mice are capable of HMPV clearance similar to RSV models. In children less than <3 years of age with a documented HMPV infection, nasal secretions showed a relative increase in proteins associated with Th1 responses but not Th2 responses; this deviation in Th1/Th2 balance was abrogated in patients with a history of prematurity (205). In contrast, a second study evaluating nasal protein levels found infants with HMPV infection had a decrease in IFN-γ/IL-4 ratio (e.g. Th2-skewing) when compared to RSV and influenza (206).
In summary, the preclinical neonatal models of respiratory viral infection demonstrated an amplification of the baseline neonatal immune response: dampened early antiviral pro-inflammatory mediators, reduced co-stimulatory help via antigen-presenting cells, poor organization of Tfh/B cell interactions, and reinforced dominance of type 2 immunity. The latter is driven by epithelial-derived cytokines like IL-33, IL-25, and TSLP and contributes to longstanding pathophysiologic changes in some models. Addition of another antigen or allergen synergistically contributed to asthma-like pathology. Collectively, these models demonstrate a skewed, but protective, immune response in neonates compared to adult animals, and help establish a mechanistic link between respiratory viral infection early in life and long-term sequelae like asthma.
Long-Term Clinical Ramifications of Early Life Respiratory Viral Infection
Several epidemiologic studies have supported the clinically observed phenomenon of development of asthma after an early respiratory viral infection, with a particular focus on RV and RSV (207, 208). Interestingly, first-time wheeze in young children was predominantly associated with RV (44, 209). A prospective study of children showed that wheezing with a RV infection in infancy was associated with a significantly higher risk of wheezing at age 3 and a 10-fold increase in risk of diagnosis of asthma at the age of 6 (210, 211). Similarly, a prospective study of children presenting with bronchiolitis used machine learning clustering to identify risk factors for recurrent wheeze; RV detection was the strongest single predictor (212). A prospective study found RV-C bronchiolitis in infancy, but not RV-A or -B, was associated with recurrent wheeze and IgE-sensitization compared to infants with RSV bronchiolitis (213). Genetic-environment interactions play a role in this process, as RV-related recurrent wheeze (but not RSV) was associated with polymorphisms in the 17q21 locus, a well-established susceptibility locus for development of childhood asthma (214, 215).
Similar to RV, an RSV illness with wheeze early in life also showed a significant 3-fold increase in risk of subsequent asthma development (209, 211). Children with RSV-bronchiolitis that went on to develop asthma were more likely to have an elevated IgE, higher birth weight, or delivery via caesarean section (216). At age 18, children with a history of RSV bronchiolitis within the first year of life had an increased risk of asthma, allergy, and sensitization to perennial allergens compared to controls; these differences were magnified when accounting for parental history of asthma (217). Further endotyping of children with RSV bronchiolitis found those with parental asthma, RV co-infection, IgE sensitization, a Moraxella-dominant airway microbiome, and high IFN-γ responses had the highest risk of subsequent asthma development, highlighting the myriad factors contributing to this outcome (218). One study of Danish twins found hospitalization with RSV was associated with increased short-term risk of admission for asthma; asthma admission was also associated with severe RSV, demonstrating a bidirectional association, emphasizing a genetic component (219). A second twin study found that RSV hospitalization in early childhood may not directly cause asthma but may indicate a genetic predisposition for subsequent asthma development (220). Large systematic reviews of studies evaluating risk of asthma after RSV infection during infancy demonstrate a higher prevalence of asthma throughout childhood years (221).
Like RSV and RV, HMPV in childhood is associated with development of asthma. Children followed prospectively after a HMPV lower respiratory tract infection were found to have a shorter duration of time between wheezing episodes (both with and without a subsequent viral trigger) when compared to controls without evidence of HMPV infection (222). Furthermore, patients with a history of HMPV bronchiolitis were much more likely to have asthma by age 5 (odds ratio=5.21) compared to patients without HMPV bronchiolitis (223). Additionally, HMPV lower respiratory tract infection in premature infants was associated with abnormal lung function at one year of age (224). In regards to upstream mediators of asthma-like inflammation, HMPV has been shown to induce in vitro expression of IL-33 and TSLP in human alveolar epithelial cells (225). Clinically, serum levels of TSLP in children with wheeze during HMPV infection were elevated, further potentiating a link between TSLP production and HMPV infection (226).
While specific pathogens have been evaluated, Bønnelykke et al. recently demonstrated that any lower respiratory tract infection (e.g. viral or bacterial) in the first years of life and the frequency of infections were variables associated with increased risk of asthma at age 7 (227). Additionally, while the focus of this review is on infectious triggers of asthma, childhood-onset asthma represents a multifactorial disease (e.g. genetic, exposures, atopy, microbiome etc.) contributing to pathogenesis (228).
Treatment/Prevention
There is a relative dearth of antiviral agents directed against the respiratory viruses reviewed here. Aerosolized ribavirin has been studied in severely ill children with RSV but has demonstrated minimal efficacy (229). No licensed therapeutic options currently exist for HMPV or RV (18, 30).
Given the lack of effective therapies, the focus has turned to prevention. Vaccination against these respiratory pathogens has been an area of particular emphasis. However, the turbulent experiences of the formalin-inactivated RSV vaccine in the 1960’s delayed progress (230). Infants and toddlers who received the formalin-inactivated RSV vaccine demonstrated worsened outcomes of RSV infection, with 80% requiring hospitalization and two succumbing to infection (231–233). Studies have demonstrated a role for Th2-bias leading to the pathology of this enhanced respiratory disease (ERD); this theory was recently strengthened further after transcriptomic analysis of autopsy specimens from the two fatal cases of ERD in toddlers showed a Th2-signature and low-affinity antibodies causing complement deposition (169, 234–237). Several candidate vaccines, including live-attenuated, inactivated, particle-based, and subunit based, are in preclinical development or clinical trials (233, 238). An alternative approach has focused on maternal immunization with passive immunity conferred to the infant; a nanoparticle protein-based vaccine administered in the third trimester showed a significant reduction in hospitalization rate with RSV in the first 90 days of the infant’s life (239). This would, however, be of less utility for premature infants delivered prior to vaccine administration. HMPV vaccines, including protein-based vaccines, live attenuated viruses, and virus-like particles, have shown promise in animal models (240–247). However, one clinical trial of a live attenuated HMPV vaccine showed only modest induction of a neutralizing antibody response in 30% of participants (248). While RV vaccines have proven difficult given the number of serotypes, several approaches are being explored in preclinical models (30, 249, 250).
A degree of prevention has been achieved against RSV with the use of monoclonal antibodies. Palivizumab, a humanized monoclonal antibody directed against the F protein of RSV, decreased hospitalizations in high-risk infants (e.g., prematurity, congenital heart disease, immunodeficiency) when administered monthly (251–253). However, cost limits the widespread use of palivizumab (251, 254). Several other anti-RSV monoclonal antibodies are in development, including a long-acting monoclonal (nirsevimab) capable of offering protection for 5 months (230, 255). One recent study estimated that a strategic switch from monthly monoclonal injections to either a maternal immunization strategy and/or use of long-acting monoclonals would afford significant cost-savings while providing a similar degree of benefit (256).
Analogous to palivizumab, human monoclonal antibodies against HMPV have been developed; in preclinical models, these monoclonals have been shown to have preventative and therapeutic potential (257, 258). Interestingly, certain HPMV-derived monoclonals show a degree of cross-protection against other Pneumoviridae family members, including RSV (259, 260). Development of monoclonal antibodies directed against RV has been studied, but face similar hurdles as RV vaccine development (261).
While the search for a safe and effective vaccine and further monoclonal antibodies continues, the COVID-19 pandemic has also illustrated the efficacy of non-pharmaceutical public health measures. From 2020 to 2021, strategies to mitigate COVID-19 such as masking and distancing effectively reduced the case burden of RSV and HMPV (262). With loosening of these restrictions in the summer of 2021, there was an anomalous increase in pediatric RSV cases in the summer months (262). Collectively, these findings illustrate that public health approaches represent a cost-effective and, in the absence of a pandemic, a possibly underutilized approach towards protecting infants against respiratory viruses.
Conclusions and Future Directions
Respiratory pathogens, such as RSV, HMPV, and RV, are major contributors to morbidity and mortality in the neonatal population. This may be in part due to the unique immunologic milieu of the neonatal lung, which differs from an adult immune response in practically every cell type. Type 2 immunity predominates in the neonatal lung and, in animal models, contributes to long-term pathology. This is mirrored clinically, as infants exposed to these viruses are at increased risk of development of long-term sequelae such as asthma. However, deeper understanding of the underlying immunologic differences in neonates has the potential to impact how clinicians consider these pathologies, both in the acute and long-term settings. For instance, understanding the pre-existing imbalance of type 1 and type 2 immunity in the neonate could lead to development of immunomodulatory therapeutics to boost the former or suppress the latter. Several pre-clinical models have inhibited type 2 immune factors during the acute immune response and mitigated long-term pathology. Preventing an increased risk of asthma in infants with lower respiratory tract viral infections is a lofty but worthy aspiration. From a basic science perspective, there are many new avenues of neonatal lung biology to explore, such as the roles of the neonatal microbiome (lung and gut) and the use of broad -omics based techniques to elucidate novel aspects of neonatal immunity. While the clinical realm awaits the furthered characterization and promise of translation of these findings, respiratory viral infections will continue to present a significant challenge to neonates and infants. Far too many young children still succumb to these infections, with disproportionate mortality in resource-limited areas of the world, highlighting the need for cost-effective interventions. Although therapeutic options are limited at present, advances in vaccination and monoclonal antibody prophylactics are hoped to translate to increased prevention in neonates.
Author Contributions
TE - drafted and revised manuscript, generated figures. OP - drafted a section of manuscript, helped generate figures, and revised manuscript. JW - drafting/writing of manuscript. All authors contributed to the article and approved the submitted version.
Funding
Research reported in this publication was supported by the Eunice Kennedy Shriver National Institute Of Child Health & Human Development of the National Institutes of Health (K12 HD000850, TE), the National Institute of General Medicacal Sciences T32 GM008208 (OP), the National Institute of Allergy and Infectious Diseases (R01 AI085062, JW), and the Henry L. Hillam Foundation (JW).
Conflict of Interest
The remaining authors declare that the research was conducted in the absence of any commercial or financial relationships that could be construed as a potential conflict of interest.
The handling editor KE declared a shared affiliation with the author OP at the time of review.
Publisher’s Note
All claims expressed in this article are solely those of the authors and do not necessarily represent those of their affiliated organizations, or those of the publisher, the editors and the reviewers. Any product that may be evaluated in this article, or claim that may be made by its manufacturer, is not guaranteed or endorsed by the publisher.
References
1. McAllister DA, Liu L, Shi T, Chu Y, Reed C, Burrows J, et al. Global, Regional, and National Estimates of Pneumonia Morbidity and Mortality in Children Younger Than 5 Years Between 2000 and 2015: A Systematic Analysis. Lancet Glob Health (2019) 7:e47–57. doi: 10.1016/S2214-109X(18)30408-X
2. Shi T, McAllister DA, O’Brien KL, Simoes EAF, Madhi SA, Gessner BD, et al. Global, Regional, and National Disease Burden Estimates of Acute Lower Respiratory Infections Due to Respiratory Syncytial Virus in Young Children in 2015: A Systematic Review and Modelling Study. Lancet (2017) 390:946–58. doi: 10.1016/S0140-6736(17)30938-8
3. Wang X, Li Y, Deloria-Knoll M, Madhi SA, Cohen C, Ali A, et al. Global Burden of Acute Lower Respiratory Infection Associated With Human Metapneumovirus in Children Under 5 Years in 2018: A Systematic Review and Modelling Study. Lancet Glob Health (2021) 9:e33–43. doi: 10.1016/S2214-109X(20)30393-4
4. Wang X, Li Y, O’Brien KL, Madhi SA, Widdowson M-A, Byass P, et al. Global Burden of Respiratory Infections Associated With Seasonal Influenza in Children Under 5 Years in 2018: A Systematic Review and Modelling Study. Lancet Glob Health (2020) 8:e497–510. doi: 10.1016/S2214-109X(19)30545-5
5. Mansbach JM, Piedra PA, Teach SJ, Sullivan AF, Forgey T, Clark S, et al. Prospective Multicenter Study of Viral Etiology and Hospital Length of Stay in Children With Severe Bronchiolitis. Arch Pediatr Adolesc Med (2012) 166:700–6. doi: 10.1001/archpediatrics.2011.1669
6. Calvo C, Pozo F, García-García ML, Sanchez M, Lopez-Valero M, Pérez-Breña P, et al. Detection of New Respiratory Viruses in Hospitalized Infants With Bronchiolitis: A Three-Year Prospective Study. Acta Paediatr (2010) 99:883–7. doi: 10.1111/j.1651-2227.2010.01714.x
7. Marguet C, Lubrano M, Gueudin M, Le Roux P, Deschildre A, Forget C, et al. In Very Young Infants Severity of Acute Bronchiolitis Depends on Carried Viruses. PloS One (2009) 4:e4596. doi: 10.1371/journal.pone.0004596
8. Borchers AT, Chang C, Gershwin ME, Gershwin LJ. Respiratory Syncytial Virus–a Comprehensive Review. Clin Rev Allergy Immunol (2013) 45:331–79. doi: 10.1007/s12016-013-8368-9
9. Li Y, Reeves RM, Wang X, Bassat Q, Brooks WA, Cohen C, et al. Global Patterns in Monthly Activity of Influenza Virus, Respiratory Syncytial Virus, Parainfluenza Virus, and Metapneumovirus: A Systematic Analysis. Lancet Glob Health (2019) 7:e1031–45. doi: 10.1016/S2214-109X(19)30264-5
10. Glezen WP, Taber LH, Frank AL, Kasel JA. Risk of Primary Infection and Reinfection With Respiratory Syncytial Virus. Am J Dis Child (1986) 140:543–6. doi: 10.1001/archpedi.1986.02140200053026
11. Openshaw PJM, Tregoning JS. Immune Responses and Disease Enhancement During Respiratory Syncytial Virus Infection. Clin Microbiol Rev (2005) 18:541–55. doi: 10.1128/CMR.18.3.541-555.2005
12. Wright M, Piedimonte G. Respiratory Syncytial Virus Prevention and Therapy: Past, Present, and Future. Pediatr Pulmonol (2011) 46:324–47. doi: 10.1002/ppul.21377
13. Henrickson KJ, Hoover S, Kehl KS, Hua W. National Disease Burden of Respiratory Viruses Detected in Children by Polymerase Chain Reaction. Pediatr Infect Dis J (2004) 23:S11–8. doi: 10.1097/01.inf.0000108188.37237.48
14. Leader S, Kohlhase K. Respiratory Syncytial Virus-Coded Pediatric Hospitalizations, 1997 to 1999. Pediatr Infect Dis J (2002) 21:629–32. doi: 10.1097/00006454-200207000-00005
15. Stein RT, Bont LJ, Zar H, Polack FP, Park C, Claxton A, et al. Respiratory Syncytial Virus Hospitalization and Mortality: Systematic Review and Meta-Analysis. Pediatr Pulmonol (2017) 52:556–69. doi: 10.1002/ppul.23570
16. van den Hoogen BG, de Jong JC, Groen J, Kuiken T, de Groot R, Fouchier RA, et al. A Newly Discovered Human Pneumovirus Isolated From Young Children With Respiratory Tract Disease. Nat Med (2001) 7:719–24. doi: 10.1038/89098
17. van den Hoogen BG, Bestebroer TM, Osterhaus ADME, Fouchier RAM. Analysis of the Genomic Sequence of a Human Metapneumovirus. Virology (2002) 295:119–32. doi: 10.1006/viro.2001.1355
18. Schildgen V, van den Hoogen B, Fouchier R, Tripp RA, Alvarez R, Manoha C, et al. Human Metapneumovirus: Lessons Learned Over the First Decade. Clin Microbiol Rev (2011) 24:734–54. doi: 10.1128/CMR.00015-11
19. Aberle JH, Aberle SW, Redlberger-Fritz M, Sandhofer MJ, Popow-Kraupp T. Human Metapneumovirus Subgroup Changes and Seasonality During Epidemics. Pediatr Infect Dis J (2010) 29:1016–8. doi: 10.1097/INF.0b013e3181e3331a
20. Baer G, Schaad UB, Heininger U. Clinical Findings and Unusual Epidemiologic Characteristics of Human Metapneumovirus Infections in Children in the Region of Basel, Switzerland. Eur J Pediatr (2008) 167:63–9. doi: 10.1007/s00431-007-0427-x
21. Robinson JL, Lee BE, Bastien N, Li Y. Seasonality and Clinical Features of Human Metapneumovirus Infection in Children in Northern Alberta. J Med Virol (2005) 76:98–105. doi: 10.1002/jmv.20329
22. Williams JV, Harris PA, Tollefson SJ, Halburnt-Rush LL, Pingsterhaus JM, Edwards KM, et al. Human Metapneumovirus and Lower Respiratory Tract Disease in Otherwise Healthy Infants and Children. N Engl J Med (2004) 350:443–50. doi: 10.1056/NEJMoa025472
23. Williams JV, Wang CK, Yang C-F, Tollefson SJ, House FS, Heck JM, et al. The Role of Human Metapneumovirus in Upper Respiratory Tract Infections in Children: A 20-Year Experience. J Infect Dis (2006) 193:387–95. doi: 10.1086/499274
24. Esper F, Martinello RA, Boucher D, Weibel C, Ferguson D, Landry ML. Kahn JS. A 1-Year Experience With Human Metapneumovirus in Children Aged <5 Years. J Infect Dis (2004) 189:1388–96. doi: 10.1086/382482
25. Døllner H, Risnes K, Radtke A, Nordbø SA. Outbreak of Human Metapneumovirus Infection in Norwegian Children. Pediatr Infect Dis J (2004) 23:436–40. doi: 10.1097/01.inf.0000126401.21779.74
26. Edwards KM, Zhu Y, Griffin MR, Weinberg GA, Hall CB, Szilagyi PG, et al. Burden of Human Metapneumovirus Infection in Young Children. N Engl J Med (2013) 368:633–43. doi: 10.1056/NEJMoa1204630
27. Maitre NL, Williams JV. Human Metapneumovirus in the Preterm Neonate: Current Perspectives. RRN (2016) 6:41–9. doi: 10.2147/RRN.S76270
28. Arruda E, Jones MH, Escremim de Paula F, Chong D, Bugarin G, Notario G, et al. The Burden of Single Virus and Viral Coinfections on Severe Lower Respiratory Tract Infections Among Preterm Infants: A Prospective Birth Cohort Study in Brazil. Pediatr Infect Dis J (2014) 33:997–1003. doi: 10.1097/INF.0000000000000349
29. García-Garcia ML, González-Carrasco E, Quevedo S, Muñoz C, Sánchez-Escudero V, Pozo F, et al. Clinical and Virological Characteristics of Early and Moderate Preterm Infants Readmitted With Viral Respiratory Infections. Pediatr Infect Dis J (2015) 34:693–9. doi: 10.1097/INF.0000000000000718
30. Jacobs SE, Lamson DM, St George K, Walsh TJ. Human Rhinoviruses. Clin Microbiol Rev (2013) 26:135–62. doi: 10.1128/CMR.00077-12
31. Palmenberg AC, Spiro D, Kuzmickas R, Wang S, Djikeng A, Rathe JA, et al. Sequencing and Analyses of All Known Human Rhinovirus Genomes Reveal Structure and Evolution. Science (2009) 324:55–9. doi: 10.1126/science.1165557
32. Palmenberg AC, Rathe JA, Liggett SB. Analysis of the Complete Genome Sequences of Human Rhinovirus. J Allergy Clin Immunol (2010) 125:1190–9. doi: 10.1016/j.jaci.2010.04.010
33. Gwaltney JM, Hendley JO, Simon G, Jordan WS. Rhinovirus Infections in an Industrial Population. I. The Occurrence of Illness. N Engl J Med (1966) 275:1261–8. doi: 10.1056/NEJM196612082752301
34. Winther B, Hayden FG, Hendley JO. Picornavirus Infections in Children Diagnosed by RT-PCR During Longitudinal Surveillance With Weekly Sampling: Association With Symptomatic Illness and Effect of Season. J Med Virol (2006) 78:644–50. doi: 10.1002/jmv.20588
35. Jartti T, Lee WM, Pappas T, Evans M, Lemanske RF, Gern JE. Serial Viral Infections in Infants With Recurrent Respiratory Illnesses. Eur Respir J (2008) 32:314–20. doi: 10.1183/09031936.00161907
36. Regamey N, Kaiser L, Roiha HL, Deffernez C, Kuehni CE, Latzin P, et al. Swiss Paediatric Respiratory Research Group. Viral Etiology of Acute Respiratory Infections With Cough in Infancy: A Community-Based Birth Cohort Study. Pediatr Infect Dis J (2008) 27:100–5. doi: 10.1097/INF.0b013e31815922c8
37. Singleton RJ, Bulkow LR, Miernyk K, DeByle C, Pruitt L, Hummel KB, et al. Viral Respiratory Infections in Hospitalized and Community Control Children in Alaska. J Med Virol (2010) 82:1282–90. doi: 10.1002/jmv.21790
38. Nokso-Koivisto J, Kinnari TJ, Lindahl P, Hovi T, Pitkäranta A. Human Picornavirus and Coronavirus RNA in Nasopharynx of Children Without Concurrent Respiratory Symptoms. J Med Virol (2002) 66:417–20. doi: 10.1002/jmv.2161
39. van Benten I, Koopman L, Niesters B, Hop W, van Middelkoop B, de Waal L, et al. Predominance of Rhinovirus in the Nose of Symptomatic and Asymptomatic Infants. Pediatr Allergy Immunol (2003) 14:363–70. doi: 10.1034/j.1399-3038.2003.00064.x
40. Monto AS, Sullivan KM. Acute Respiratory Illness in the Community. Frequency of Illness and the Agents Involved. Epidemiol Infect (1993) 110:145–60. doi: 10.1017/S0950268800050779
41. Arruda E, Pitkäranta A, Witek TJ, Doyle CA, Hayden FG. Frequency and Natural History of Rhinovirus Infections in Adults During Autumn. J Clin Microbiol (1997) 35:2864–8. doi: 10.1128/jcm.35.11.2864-2868.1997
42. Mackay IM. Human Rhinoviruses: The Cold Wars Resume. J Clin Virol (2008) 42:297–320. doi: 10.1016/j.jcv.2008.04.002
43. Vandini S, Biagi C, Fischer M, Lanari M. Impact of Rhinovirus Infections in Children. Viruses (2019) 11(6):521. doi: 10.3390/v11060521
44. Turunen R, Koistinen A, Vuorinen T, Arku B, Söderlund-Venermo M, Ruuskanen O, et al. The First Wheezing Episode: Respiratory Virus Etiology, Atopic Characteristics, and Illness Severity. Pediatr Allergy Immunol (2014) 25:796–803. doi: 10.1111/pai.12318
45. Kusel MMH, de Klerk NH, Holt PG, Kebadze T, Johnston SL, Sly PD. Role of Respiratory Viruses in Acute Upper and Lower Respiratory Tract Illness in the First Year of Life: A Birth Cohort Study. Pediatr Infect Dis J (2006) 25:680–6. doi: 10.1097/01.inf.0000226912.88900.a3
46. Juvén T, Mertsola J, Waris M, Leinonen M, Meurman O, Roivainen M, et al. Etiology of Community-Acquired Pneumonia in 254 Hospitalized Children. Pediatr Infect Dis J (2000) 19:293–8. doi: 10.1097/00006454-200004000-00006
47. Papadopoulos NG, Moustaki M, Tsolia M, Bossios A, Astra E, Prezerakou A, et al. Association of Rhinovirus Infection With Increased Disease Severity in Acute Bronchiolitis. Am J Respir Crit Care Med (2002) 165:1285–9. doi: 10.1164/rccm.200112-118BC
48. Malcolm E, Arruda E, Hayden FG, Kaiser L. Clinical Features of Patients With Acute Respiratory Illness and Rhinovirus in Their Bronchoalveolar Lavages. J Clin Virol (2001) 21:9–16. doi: 10.1016/s1386-6532(00)00180-3
49. Korppi M, Kotaniemi-Syrjänen A, Waris M, Vainionpää R, Reijonen TM. Rhinovirus-Associated Wheezing in Infancy: Comparison With Respiratory Syncytial Virus Bronchiolitis. Pediatr Infect Dis J (2004) 23:995–9. doi: 10.1097/01.inf.0000143642.72480.53
50. Kieninger E, Fuchs O, Latzin P, Frey U, Regamey N. Rhinovirus Infections in Infancy and Early Childhood. Eur Respir J (2013) 41:443–52. doi: 10.1183/09031936.00203511
51. Miller EK, Lu X, Erdman DD, Poehling KA, Zhu Y, Griffin MR, et al. Rhinovirus-Associated Hospitalizations in Young Children. J Infect Dis (2007) 195:773–81. doi: 10.1086/511821
52. Steiner M, Strassl R, Straub J, Böhm J, Popow-Kraupp T, Berger A. Nosocomial Rhinovirus Infection in Preterm Infants. Pediatr Infect Dis J (2012) 31:1302–4. doi: 10.1097/INF.0b013e31826ff939
53. Miller EK, Bugna J, Libster R, Shepherd BE, Scalzo PM, Acosta PL, et al. Human Rhinoviruses in Severe Respiratory Disease in Very Low Birth Weight Infants. Pediatrics (2012) 129:e60–7. doi: 10.1542/peds.2011-0583
54. van Piggelen RO, van Loon AM, Krediet TG, Verboon-Maciolek MA. Human Rhinovirus Causes Severe Infection in Preterm Infants. Pediatr Infect Dis J (2010) 29:364–5. doi: 10.1097/INF.0b013e3181c6e60f
55. Warburton D, El-Hashash A, Carraro G, Tiozzo C, Sala F, Rogers O, et al. Lung Organogenesis. Curr Top Dev Biol (2010) 90:73–158. doi: 10.1016/S0070-2153(10)90003-3
56. Torow N, Marsland BJ, Hornef MW, Gollwitzer ES. Neonatal Mucosal Immunology. Mucosal Immunol (2017) 10:5–17. doi: 10.1038/mi.2016.81
57. McCarthy KM, Gong JL, Telford JR, Schneeberger EE. Ontogeny of Ia+ Accessory Cells in Fetal and Newborn Rat Lung. Am J Respir Cell Mol Biol (1992) 6:349–56. doi: 10.1165/ajrcmb/6.3.349
58. Blackwell TS, Hipps AN, Yamamoto Y, Han W, Barham WJ, Ostrowski MC, et al. NF-κb Signaling in Fetal Lung Macrophages Disrupts Airway Morphogenesis. J Immunol (2011) 187:2740–7. doi: 10.4049/jimmunol.1101495
59. Tang Y, Peitzsch C, Charoudeh HN, Cheng M, Chaves P, Jacobsen SEW, et al. Emergence of NK-Cell Progenitors and Functionally Competent NK-Cell Lineage Subsets in the Early Mouse Embryo. Blood (2012) 120:63–75. doi: 10.1182/blood-2011-02-337980
60. Haas JD, Ravens S, Düber S, Sandrock I, Oberdörfer L, Kashani E, et al. Development of Interleukin-17-Producing γδ T Cells Is Restricted to a Functional Embryonic Wave. Immunity (2012) 37:48–59. doi: 10.1016/j.immuni.2012.06.003
61. Ramond C, Berthault C, Burlen-Defranoux O, de Sousa AP, Guy-Grand D, Vieira P, et al. Two Waves of Distinct Hematopoietic Progenitor Cells Colonize the Fetal Thymus. Nat Immunol (2014) 15:27–35. doi: 10.1038/ni.2782
62. Roy MG, Rahmani M, Hernandez JR, Alexander SN, Ehre C, Ho SB, et al. Mucin Production During Prenatal and Postnatal Murine Lung Development. Am J Respir Cell Mol Biol (2011) 44:755–60. doi: 10.1165/rcmb.2010-0020oc
63. Roy MG, Livraghi-Butrico A, Fletcher AA, McElwee MM, Evans SE, Boerner RM, et al. Muc5b Is Required for Airway Defence. Nature (2014) 505:412–6. doi: 10.1038/nature12807
64. Agrawal V, Smart K, Jilling T, Hirsch E. Surfactant Protein (SP)-A Suppresses Preterm Delivery and Inflammation via TLR2. PloS One (2013) 8:e63990. doi: 10.1371/journal.pone.0063990
65. Condon JC, Jeyasuria P, Faust JM, Mendelson CR. Surfactant Protein Secreted by the Maturing Mouse Fetal Lung Acts as a Hormone That Signals the Initiation of Parturition. Proc Natl Acad Sci USA (2004) 101:4978–83. doi: 10.1073/pnas.0401124101
66. Wu H, Kuzmenko A, Wan S, Schaffer L, Weiss A, Fisher JH, et al. Surfactant Proteins A and D Inhibit the Growth of Gram-Negative Bacteria by Increasing Membrane Permeability. J Clin Invest (2003) 111:1589–602. doi: 10.1172/JCI16889
67. Domingo-Gonzalez R, Zanini F, Che X, Liu M, Jones RC, Swift MA, et al. Diverse Homeostatic and Immunomodulatory Roles of Immune Cells in the Developing Mouse Lung at Single Cell Resolution. Elife (2020) 9:1–39. doi: 10.7554/eLife.56890
68. Guilliams M, De Kleer I, Henri S, Post S, Vanhoutte L, De Prijck S, et al. Alveolar Macrophages Develop From Fetal Monocytes That Differentiate Into Long-Lived Cells in the First Week of Life via GM-CSF. J Exp Med (2013) 210:1977–92. doi: 10.1084/jem.20131199
69. Chelvarajan RL, Collins SM, Doubinskaia IE, Goes S, Van Willigen J, Flanagan D, et al. Defective Macrophage Function in Neonates and Its Impact on Unresponsiveness of Neonates to Polysaccharide Antigens. J Leukoc Biol (2004) 75:982–94. doi: 10.1189/jlb.0403179
70. Jones CV, Williams TM, Walker KA, Dickinson H, Sakkal S, Rumballe BA, et al. M2 Macrophage Polarisation Is Associated With Alveolar Formation During Postnatal Lung Development. Respir Res (2013) 14:41. doi: 10.1186/1465-9921-14-41
71. Speer CP, Gahr M, Wieland M, Eber S. Phagocytosis-Associated Functions in Neonatal Monocyte-Derived Macrophages. Pediatr Res (1988) 24:213–6. doi: 10.1203/00006450-198808000-00015
72. Dreschers S, Ohl K, Schulte N, Tenbrock K, Orlikowsky TW. Impaired Functional Capacity of Polarised Neonatal Macrophages. Sci Rep (2020) 10:624. doi: 10.1038/s41598-019-56928-4
73. Maoldomhnaigh C, Cox DJ, Phelan JJ, Malone FD, Keane J, Basdeo SA. The Warburg Effect Occurs Rapidly in Stimulated Human Adult But Not Umbilical Cord Blood Derived Macrophages. Front Immunol (2021) 12:657261. doi: 10.3389/fimmu.2021.657261
74. Maródi L, Goda K, Palicz A, Szabó G. Cytokine Receptor Signalling in Neonatal Macrophages: Defective STAT-1 Phosphorylation in Response to Stimulation With IFN-Gamma. Clin Exp Immunol (2001) 126:456–60. doi: 10.1046/j.1365-2249.2001.01693.x
75. Jones CA, Holloway JA, Warner JO. Phenotype of Fetal Monocytes and B Lymphocytes During the Third Trimester of Pregnancy. J Reprod Immunol (2002) 56:45–60. doi: 10.1016/s0165-0378(02)00022-0
76. Sanchez-Schmitz G, Morrocchi E, Cooney M, Soni D, Khatun R, Palma P, et al. Neonatal Monocytes Demonstrate Impaired Homeostatic Extravasation Into a Microphysiological Human Vascular Model. Sci Rep (2020) 10:17836. doi: 10.1038/s41598-020-74639-z
77. Ruckwardt TJ, Malloy AMW, Morabito KM, Graham BS. Quantitative and Qualitative Deficits in Neonatal Lung-Migratory Dendritic Cells Impact the Generation of the CD8+ T Cell Response. PloS Pathog (2014) 10:e1003934. doi: 10.1371/journal.ppat.1003934
78. Dakic A, Shao Q, D’Amico A, O’Keeffe M, Chen W, Shortman K, et al. Development of the Dendritic Cell System During Mouse Ontogeny. J Immunol (2004) 172:1018–27. doi: 10.4049/jimmunol.172.2.1018
79. Gollwitzer ES, Saglani S, Trompette A, Yadava K, Sherburn R, McCoy KD, et al. Lung Microbiota Promotes Tolerance to Allergens in Neonates via PD-L1. Nat Med (2014) 20:642–7. doi: 10.1038/nm.3568
80. Langrish CL, Buddle JC, Thrasher AJ, Goldblatt D. Neonatal Dendritic Cells Are Intrinsically Biased Against Th-1 Immune Responses. Clin Exp Immunol (2002) 128:118–23. doi: 10.1046/j.1365-2249.2002.01817.x
81. Aksoy E, Albarani V, Nguyen M, Laes J-F, Ruelle J-L, De Wit D, et al. Interferon Regulatory Factor 3-Dependent Responses to Lipopolysaccharide Are Selectively Blunted in Cord Blood Cells. Blood (2007) 109:2887–93. doi: 10.1182/blood-2006-06-027862
82. Goriely S, Vincart B, Stordeur P, Vekemans J, Willems F, Goldman M, et al. Deficient IL-12(P35) Gene Expression by Dendritic Cells Derived From Neonatal Monocytes. J Immunol (2001) 166:2141–6. doi: 10.4049/jimmunol.166.3.2141
83. Zaghouani H, Hoeman CM, Adkins B. Neonatal Immunity: Faulty T-Helpers and the Shortcomings of Dendritic Cells. Trends Immunol (2009) 30:585–91. doi: 10.1016/j.it.2009.09.002
84. De Wit D, Olislagers V, Goriely S, Vermeulen F, Wagner H, Goldman M, et al. Blood Plasmacytoid Dendritic Cell Responses to CpG Oligodeoxynucleotides Are Impaired in Human Newborns. Blood (2004) 103:1030–2. doi: 10.1182/blood-2003-04-1216
85. Danis B, George TC, Goriely S, Dutta B, Renneson J, Gatto L, et al. Interferon Regulatory Factor 7-Mediated Responses Are Defective in Cord Blood Plasmacytoid Dendritic Cells. Eur J Immunol (2008) 38:507–17. doi: 10.1002/eji.200737760
86. Sun C-M, Deriaud E, Leclerc C, Lo-Man R. Upon TLR9 Signaling, CD5+ B Cells Control the IL-12-Dependent Th1-Priming Capacity of Neonatal DCs. Immunity (2005) 22:467–77. doi: 10.1016/j.immuni.2005.02.008
87. Walker WE, Goldstein DR. Neonatal B Cells Suppress Innate Toll-Like Receptor Immune Responses and Modulate Alloimmunity. J Immunol (2007) 179:1700–10. doi: 10.4049/jimmunol.179.3.1700
88. Corbett NP, Blimkie D, Ho KC, Cai B, Sutherland DP, Kallos A, et al. Ontogeny of Toll-Like Receptor Mediated Cytokine Responses of Human Blood Mononuclear Cells. PloS One (2010) 5:e15041. doi: 10.1371/journal.pone.0015041
89. Willems F, Vollstedt S, Suter M. Phenotype and Function of Neonatal DC. Eur J Immunol (2009) 39:26–35. doi: 10.1002/eji.200838391
90. Papaioannou NE, Salei N, Rambichler S, Ravi K, Popovic J, Küntzel V, et al. Environmental Signals Rather Than Layered Ontogeny Imprint the Function of Type 2 Conventional Dendritic Cells in Young and Adult Mice. Nat Commun (2021) 12:464. doi: 10.1038/s41467-020-20659-2
91. Nussbaum C, Gloning A, Pruenster M, Frommhold D, Bierschenk S, Genzel-Boroviczény O, et al. Neutrophil and Endothelial Adhesive Function During Human Fetal Ontogeny. J Leukoc Biol (2013) 93:175–84. doi: 10.1189/jlb.0912468
92. Simon AK, Hollander GA, McMichael A. Evolution of the Immune System in Humans From Infancy to Old Age. Proc Biol Sci (2015) 282:20143085. doi: 10.1098/rspb.2014.3085
93. Filias A, Theodorou GL, Mouzopoulou S, Varvarigou AA, Mantagos S, Karakantza M. Phagocytic Ability of Neutrophils and Monocytes in Neonates. BMC Pediatr (2011) 11:29. doi: 10.1186/1471-2431-11-29
94. Yost CC, Cody MJ, Harris ES, Thornton NL, McInturff AM, Martinez ML, et al. Impaired Neutrophil Extracellular Trap (NET) Formation: A Novel Innate Immune Deficiency of Human Neonates. Blood (2009) 113:6419–27. doi: 10.1182/blood-2008-07-171629
95. Basha S, Surendran N, Pichichero M. Immune Responses in Neonates. Expert Rev Clin Immunol (2014) 10:1171–84. doi: 10.1586/1744666X.2014.942288
96. Luo D, Schowengerdt KO, Stegner JJ, May WS, Koenig JM. Decreased Functional Caspase-3 Expression in Umbilical Cord Blood Neutrophils Is Linked to Delayed Apoptosis. Pediatr Res (2003) 53:859–64. doi: 10.1203/01.PDR.0000059747.52100.2E
97. Hanna N, Vasquez P, Pham P, Heck DE, Laskin JD, Laskin DL, et al. Mechanisms Underlying Reduced Apoptosis in Neonatal Neutrophils. Pediatr Res (2005) 57:56–62. doi: 10.1203/01.PDR.0000147568.14392.F0
98. Rieber N, Gille C, Köstlin N, Schäfer I, Spring B, Ost M, et al. Neutrophilic Myeloid-Derived Suppressor Cells in Cord Blood Modulate Innate and Adaptive Immune Responses. Clin Exp Immunol (2013) 174:45–52. doi: 10.1111/cei.12143
99. Ivarsson MA, Loh L, Marquardt N, Kekäläinen E, Berglin L, Björkström NK, et al. Differentiation and Functional Regulation of Human Fetal NK Cells. J Clin Invest (2013) 123:3889–901. doi: 10.1172/JCI68989
100. Wang Y, Xu H, Zheng X, Wei H, Sun R, Tian Z. High Expression of NKG2A/CD94 and Low Expression of Granzyme B Are Associated With Reduced Cord Blood NK Cell Activity. Cell Mol Immunol (2007) 4:377–82.
101. Guilmot A, Hermann E, Braud VM, Carlier Y, Truyens C. Natural Killer Cell Responses to Infections in Early Life. J Innate Immun (2011) 3:280–8. doi: 10.1159/000323934
102. Walk J, Westerlaken GHA, van Uden NO, Belderbos ME, Meyaard L, Bont LJ. Inhibitory Receptor Expression on Neonatal Immune Cells. Clin Exp Immunol (2012) 169:164–71. doi: 10.1111/j.1365-2249.2012.04599.x
103. Slavica L, Nordström I, Karlsson MN, Valadi H, Kacerovsky M, Jacobsson B, et al. TLR3 Impairment in Human Newborns. J Leukoc Biol (2013) 94:1003–11. doi: 10.1189/jlb.1212617
104. Eljaafari FM, Takada H, Tanaka T, Doi T, Ohga S, Hara T. Potent Induction of IFN-γ Production From Cord Blood NK Cells by the Stimulation With Single-Stranded RNA. J Clin Immunol (2011) 31:728–35. doi: 10.1007/s10875-011-9528-4
105. Dalle J-H, Menezes J, Wagner E, Blagdon M, Champagne J, Champagne MA, et al. Characterization of Cord Blood Natural Killer Cells: Implications for Transplantation and Neonatal Infections. Pediatr Res (2005) 57:649–55. doi: 10.1203/01.PDR.0000156501.55431.20
106. Lau AS, Sigaroudinia M, Yeung MC, Kohl S. Interleukin-12 Induces Interferon-Gamma Expression and Natural Killer Cytotoxicity in Cord Blood Mononuclear Cells. Pediatr Res (1996) 39:150–5. doi: 10.1203/00006450-199601000-00023
107. Le Garff-Tavernier M, Béziat V, Decocq J, Siguret V, Gandjbakhch F, Pautas E, et al. Human NK Cells Display Major Phenotypic and Functional Changes Over the Life Span. Aging Cell (2010) 9:527–35. doi: 10.1111/j.1474-9726.2010.00584.x
108. Marcoe JP, Lim JR, Schaubert KL, Fodil-Cornu N, Matka M, McCubbrey AL, et al. TGF-β Is Responsible for NK Cell Immaturity During Ontogeny and Increased Susceptibility to Infection During Mouse Infancy. Nat Immunol (2012) 13:843–50. doi: 10.1038/ni.2388
109. Adkins B, Leclerc C, Marshall-Clarke S. Neonatal Adaptive Immunity Comes of Age. Nat Rev Immunol (2004) 4:553–64. doi: 10.1038/nri1394
110. Billingham RE, Brent L, Medawar PB. Actively Acquired Tolerance of Foreign Cells. Nature (1953) 172:603–6. doi: 10.1038/172603a0
111. Ridge JP, Fuchs EJ, Matzinger P. Neonatal Tolerance Revisited: Turning on Newborn T Cells With Dendritic Cells. Science (1996) 271:1723–6. doi: 10.1126/science.271.5256.1723
112. Garcia AM, Fadel SA, Cao S, Sarzotti M. T Cell Immunity in Neonates. Immunol Res (2000) 22:177–90. doi: 10.1385/IR:22:2-3:177
113. Guo M, Du Y, Gokey JJ, Ray S, Bell SM, Adam M, et al. Single Cell RNA Analysis Identifies Cellular Heterogeneity and Adaptive Responses of the Lung at Birth. Nat Commun (2019) 10:37. doi: 10.1038/s41467-018-07770-1
114. Mackay CR, Kimpton WG, Brandon MR, Cahill RN. Lymphocyte Subsets Show Marked Differences in Their Distribution Between Blood and the Afferent and Efferent Lymph of Peripheral Lymph Nodes. J Exp Med (1988) 167:1755–65. doi: 10.1084/jem.167.6.1755
115. Alferink J, Tafuri A, Vestweber D, Hallmann R, Hämmerling GJ, Arnold B. Control of Neonatal Tolerance to Tissue Antigens by Peripheral T Cell Trafficking. Science (1998) 282:1338–41. doi: 10.1126/science.282.5392.1338
116. Mackay CR, Marston WL, Dudler L. Naive and Memory T Cells Show Distinct Pathways of Lymphocyte Recirculation. J Exp Med (1990) 171:801–17. doi: 10.1084/jem.171.3.801
117. Mold JE, Venkatasubrahmanyam S, Burt TD, Michaëlsson J, Rivera JM, Galkina SA, et al. Fetal and Adult Hematopoietic Stem Cells Give Rise to Distinct T Cell Lineages in Humans. Science (2010) 330:1695–9. doi: 10.1126/science.1196509
118. Smith NL, Patel RK, Reynaldi A, Grenier JK, Wang J, Watson NB, et al. Developmental Origin Governs CD8+ T Cell Fate Decisions During Infection. Cell (2018) 174:117–130.e14. doi: 10.1016/j.cell.2018.05.029
119. Rudd BD. Neonatal T Cells: A Reinterpretation. Annu Rev Immunol (2020) 38:229–47. doi: 10.1146/annurev-immunol-091319-083608
120. Montecino-Rodriguez E. Dorshkind K. B-1 B Cell Development in the Fetus and Adult. Immunity (2012) 36:13–21. doi: 10.1016/j.immuni.2011.11.017
121. Herzenberg LA, Herzenberg LA. Toward a Layered Immune System. Cell (1989) 59:953–4. doi: 10.1016/0092-8674(89)90748-4
122. Adkins B. Peripheral CD4+ Lymphocytes Derived From Fetal Versus Adult Thymic Precursors Differ Phenotypically and Functionally. J Immunol (2003) 171:5157–64. doi: 10.4049/jimmunol.171.10.5157
123. Wang J, Wissink EM, Watson NB, Smith NL, Grimson A, Rudd BD. Fetal and Adult Progenitors Give Rise to Unique Populations of CD8+ T Cells. Blood (2016) 128:3073–82. doi: 10.1182/blood-2016-06-725366
124. Sarzotti M, Robbins DS, Hoffman PM. Induction of Protective CTL Responses in Newborn Mice by a Murine Retrovirus. Science (1996) 271:1726–8. doi: 10.1126/science.271.5256.1726
125. Kollmann TR, Reikie B, Blimkie D, Way SS, Hajjar AM, Arispe K, et al. Induction of Protective Immunity to Listeria Monocytogenes in Neonates. J Immunol (2007) 178:3695–701. doi: 10.4049/jimmunol.178.6.3695
126. Reynaldi A, Smith NL, Schlub TE, Venturi V, Rudd BD, Davenport MP. Modeling the Dynamics of Neonatal CD8+ T-Cell Responses. Immunol Cell Biol (2016) 94:838–48. doi: 10.1038/icb.2016.47
127. Smith NL, Wissink E, Wang J, Pinello JF, Davenport MP, Grimson A, et al. Rapid Proliferation and Differentiation Impairs the Development of Memory CD8+ T Cells in Early Life. J Immunol (2014) 193:177–84. doi: 10.4049/jimmunol.1400553
128. Siefker DT, Adkins B. Rapid CD8(+) Function Is Critical for Protection of Neonatal Mice From an Extracellular Bacterial Enteropathogen. Front Pediatr (2016) 4:141. doi: 10.3389/fped.2016.00141
129. Galindo-Albarrán AO, López-Portales OH, Gutiérrez-Reyna DY, Rodríguez-Jorge O, Sánchez-Villanueva JA, Ramírez-Pliego O, et al. CD8+ T Cells From Human Neonates Are Biased Toward an Innate Immune Response. Cell Rep (2016) 17:2151–60. doi: 10.1016/j.celrep.2016.10.056
130. Gutiérrez-Reyna DY, Cedillo-Baños A, Kempis-Calanis LA, Ramírez-Pliego O, Bargier L, Puthier D, et al. IL-12 Signaling Contributes to the Reprogramming of Neonatal CD8+ T Cells. Front Immunol (2020) 11:1089. doi: 10.3389/fimmu.2020.01089
131. McCarron MJ, Reen DJ. Neonatal CD8+ T-Cell Differentiation Is Dependent on Interleukin-12. Hum Immunol (2010) 71:1172–9. doi: 10.1016/j.humimm.2010.09.004
132. Wang G, Miyahara Y, Guo Z, Khattar M, Stepkowski SM, Chen W. “Default” Generation of Neonatal Regulatory T Cells. J Immunol (2010) 185:71–8. doi: 10.4049/jimmunol.0903806
133. Schelonka RL, Raaphorst FM, Infante D, Kraig E, Teale JM, Infante AJ. T Cell Receptor Repertoire Diversity and Clonal Expansion in Human Neonates. Pediatr Res (1998) 43:396–402. doi: 10.1203/00006450-199803000-00015
134. Dong M, Artusa P, Kelly SA, Fournier M, Baldwin TA, Mandl JN, et al. Alterations in the Thymic Selection Threshold Skew the Self-Reactivity of the TCR Repertoire in Neonates. J Immunol (2017) 199:965–73. doi: 10.4049/jimmunol.1602137
135. Mandl JN, Monteiro JP, Vrisekoop N, Germain RN. T Cell-Positive Selection Uses Self-Ligand Binding Strength to Optimize Repertoire Recognition of Foreign Antigens. Immunity (2013) 38:263–74. doi: 10.1016/j.immuni.2012.09.011
136. Zens KD, Chen JK, Guyer RS, Wu FL, Cvetkovski F, Miron M, et al. Reduced Generation of Lung Tissue-Resident Memory T Cells During Infancy. J Exp Med (2017) 214:2915–32. doi: 10.1084/jem.20170521
137. Forsthuber T, Yip HC, Lehmann PV. Induction of TH1 and TH2 Immunity in Neonatal Mice. Science (1996) 271:1728–30. doi: 10.1126/science.271.5256.1728
138. Chen N, Field EH. Enhanced Type 2 and Diminished Type 1 Cytokines in Neonatal Tolerance. Transplantation (1995) 59:933–41. doi: 10.1097/00007890-199504150-00002
139. Adkins B, Bu Y, Guevara P. The Generation of Th Memory in Neonates Versus Adults: Prolonged Primary Th2 Effector Function and Impaired Development of Th1 Memory Effector Function in Murine Neonates. J Immunol (2001) 166:918–25. doi: 10.4049/jimmunol.166.2.918
140. Adkins B, Hamilton K. Freshly Isolated, Murine Neonatal T Cells Produce IL-4 in Response to Anti-CD3 Stimulation. J Immunol (1992) 149:3448–55.
141. Rose S, Lichtenheld M, Foote MR, Adkins B. Murine Neonatal CD4+ Cells Are Poised for Rapid Th2 Effector-Like Function. J Immunol (2007) 178:2667–78. doi: 10.4049/jimmunol.178.5.2667
142. Webster RB, Rodriguez Y, Klimecki WT, Vercelli D. The Human IL-13 Locus in Neonatal CD4+ T Cells Is Refractory to the Acquisition of a Repressive Chromatin Architecture. J Biol Chem (2007) 282:700–9. doi: 10.1074/jbc.M609501200
143. Yoshimoto M, Yoder MC, Guevara P, Adkins B. The Murine Th2 Locus Undergoes Epigenetic Modification in the Thymus During Fetal and Postnatal Ontogeny. PloS One (2013) 8:e51587. doi: 10.1371/journal.pone.0051587
144. Li L, Lee H-H, Bell JJ, Gregg RK, Ellis JS, Gessner A, et al. IL-4 Utilizes an Alternative Receptor to Drive Apoptosis of Th1 Cells and Skews Neonatal Immunity Toward Th2. Immunity (2004) 20:429–40. doi: 10.1016/s1074-7613(04)00072-x
145. Hoeman CM, Dhakal M, Zaghouani AA, Cascio JA, Wan X, Khairallah M-T, et al. Developmental Expression of IL-12rβ2 on Murine Naive Neonatal T Cells Counters the Upregulation of IL-13rα1 on Primary Th1 Cells and Balances Immunity in the Newborn. J Immunol (2013) 190:6155–63. doi: 10.4049/jimmunol.1202207
146. Lee H-H, Hoeman CM, Hardaway JC, Guloglu FB, Ellis JS, Jain R, et al. Delayed Maturation of an IL-12-Producing Dendritic Cell Subset Explains the Early Th2 Bias in Neonatal Immunity. J Exp Med (2008) 205:2269–80. doi: 10.1084/jem.20071371
147. Yang S, Fujikado N, Kolodin D, Benoist C, Mathis D. Immune Tolerance. Regulatory T Cells Generated Early in Life Play a Distinct Role in Maintaining Self-Tolerance. Science (2015) 348:589–94. doi: 10.1126/science.aaa7017
148. Mold JE, Michaëlsson J, Burt TD, Muench MO, Beckerman KP, Busch MP, et al. Maternal Alloantigens Promote the Development of Tolerogenic Fetal Regulatory T CellsIn Utero. Science (2008) 322:1562–5. doi: 10.1126/science.1164511
149. Lei L, Zhang X, Yang X, Su Y, Liu H, Yang H, et al. A Genetic Model Reveals Biological Features of Neonatal CD4 Helper Cells Undergone Homeostasis in Mice. Front Cell Dev Biol (2021) 9:659744. doi: 10.3389/fcell.2021.659744
150. Razzaghian HR, Sharafian Z, Sharma AA, Boyce GK, Lee K, Da Silva R, et al. Neonatal T Helper 17 Responses Are Skewed Towards an Immunoregulatory Interleukin-22 Phenotype. Front Immunol (2021) 12:655027. doi: 10.3389/fimmu.2021.655027
151. Crotty S. T Follicular Helper Cell Biology: A Decade of Discovery and Diseases. Immunity (2019) 50:1132–48. doi: 10.1016/j.immuni.2019.04.011
152. Mastelic B, Kamath AT, Fontannaz P, Tougne C, Rochat A-F, Belnoue E, et al. Environmental and T Cell-Intrinsic Factors Limit the Expansion of Neonatal Follicular T Helper Cells But may be Circumvented by Specific Adjuvants. J Immunol (2012) 189:5764–72. doi: 10.4049/jimmunol.1201143
153. Debock I, Jaworski K, Chadlaoui H, Delbauve S, Passon N, Twyffels L, et al. Neonatal Follicular Th Cell Responses Are Impaired and Modulated by IL-4. J Immunol (2013) 191:1231–9. doi: 10.4049/jimmunol.1203288
154. Mastelic-Gavillet B, Vono M, Gonzalez-Dias P, Ferreira FM, Cardozo L, Lambert P-H, et al. Neonatal T Follicular Helper Cells Are Lodged in a Pre-T Follicular Helper Stage Favoring Innate Over Adaptive Germinal Center Responses. Front Immunol (2019) 10:1845. doi: 10.3389/fimmu.2019.01845
155. Carvalho TL, Mota-Santos T, Cumano A, Demengeot J, Vieira P. Arrested B Lymphopoiesis and Persistence of Activated B Cells in Adult Interleukin 7(-/)- Mice. J Exp Med (2001) 194:1141–50. doi: 10.1084/jem.194.8.1141
156. Montecino-Rodriguez E, Dorshkind K. Formation of B-1 B Cells From Neonatal B-1 Transitional Cells Exhibits NF-κb Redundancy. J Immunol (2011) 187:5712–9. doi: 10.4049/jimmunol.1102416
157. Munguía-Fuentes R, Yam-Puc JC, Silva-Sánchez A, Marcial-Juárez E, Gallegos-Hernández IA, Calderón-Amador J, et al. Immunization of Newborn Mice Accelerates the Architectural Maturation of Lymph Nodes, But AID-Dependent IgG Responses Are Still Delayed Compared to the Adult. Front Immunol (2017) 8:13. doi: 10.3389/fimmu.2017.00013
158. Griffin DO, Holodick NE, Rothstein TL. Human B1 Cells in Umbilical Cord and Adult Peripheral Blood Express the Novel Phenotype CD20+ CD27+ CD43+ CD70-. J Exp Med (2011) 208:67–80. doi: 10.1084/jem.20101499
159. Leach JL, Sedmak DD, Osborne JM, Rahill B, Lairmore MD, Anderson CL. Isolation From Human Placenta of the IgG Transporter, FcRn, and Localization to the Syncytiotrophoblast: Implications for Maternal-Fetal Antibody Transport. J Immunol (1996) 157:3317–22.
160. Crowe JE. Influence of Maternal Antibodies on Neonatal Immunization Against Respiratory Viruses. Clin Infect Dis (2001) 33:1720–7. doi: 10.1086/322971
161. Albrecht M, Arck PC. Vertically Transferred Immunity in Neonates: Mothers, Mechanisms and Mediators. Front Immunol (2020) 11:555. doi: 10.3389/fimmu.2020.00555
162. Loffredo LF, Coden ME, Jeong BM, Walker MT, Anekalla KR, Doan TC, et al. Eosinophil Accumulation in Postnatal Lung Is Specific to the Primary Septation Phase of Development. Sci Rep (2020) 10:4425. doi: 10.1038/s41598-020-61420-5
163. Empey KM, Orend JG, Peebles RS, Egaña L, Norris KA, Oury TD, et al. Stimulation of Immature Lung Macrophages With Intranasal Interferon Gamma in a Novel Neonatal Mouse Model of Respiratory Syncytial Virus Infection. PloS One (2012) 7:e40499. doi: 10.1371/journal.pone.0040499
164. Ruckwardt TJ, Malloy AMW, Gostick E, Price DA, Dash P, McClaren JL, et al. Neonatal CD8 T-Cell Hierarchy Is Distinct From Adults and Is Influenced by Intrinsic T Cell Properties in Respiratory Syncytial Virus Infected Mice. PloS Pathog (2011) 7:e1002377. doi: 10.1371/journal.ppat.1002377
165. Saravia J, You D, Shrestha B, Jaligama S, Siefker D, Lee GI, et al. Respiratory Syncytial Virus Disease Is Mediated by Age-Variable IL-33. PloS Pathog (2015) 11:e1005217. doi: 10.1371/journal.ppat.1005217
166. Han J, Dakhama A, Jia Y, Wang M, Zeng W, Takeda K, et al. Responsiveness to Respiratory Syncytial Virus in Neonates Is Mediated Through Thymic Stromal Lymphopoietin and OX40 Ligand. J Allergy Clin Immunol (2012) 130:1175–86.e9. doi: 10.1016/j.jaci.2012.08.033
167. Cormier SA, Shrestha B, Saravia J, Lee GI, Shen L, DeVincenzo JP, et al. Limited Type I Interferons and Plasmacytoid Dendritic Cells During Neonatal Respiratory Syncytial Virus Infection Permit Immunopathogenesis Upon Reinfection. J Virol (2014) 88:9350–60. doi: 10.1128/JVI.00818-14
168. Lau-Kilby AW, Turfkruyer M, Kehl M, Yang L, Buchholz UJ, Hickey K, et al. Type I IFN Ineffectively Activates Neonatal Dendritic Cells Limiting Respiratory Antiviral T-Cell Responses. Mucosal Immunol (2020) 13:371–80. doi: 10.1038/s41385-019-0234-5
169. Tregoning JS, Yamaguchi Y, Harker J, Wang B, Openshaw PJM. The Role of T Cells in the Enhancement of Respiratory Syncytial Virus Infection Severity During Adult Reinfection of Neonatally Sensitized Mice. J Virol (2008) 82:4115–24. doi: 10.1128/JVI.02313-07
170. Malloy AMW, Ruckwardt TJ, Morabito KM, Lau-Kilby AW, Graham BS. Pulmonary Dendritic Cell Subsets Shape the Respiratory Syncytial Virus-Specific CD8+ T Cell Immunodominance Hierarchy in Neonates. J Immunol (2017) 198:394–403. doi: 10.4049/jimmunol.1600486
171. Eichinger KM, Kosanovich JL, Empey KM. Localization of the T-Cell Response to RSV Infection Is Altered in Infant Mice. Pediatr Pulmonol (2018) 53:145–53. doi: 10.1002/ppul.23911
172. You D, Marr N, Saravia J, Shrestha B, Lee GI, Turvey SE, et al. IL-4rα on CD4+ T Cells Plays a Pathogenic Role in Respiratory Syncytial Virus Reinfection in Mice Infected Initially as Neonates. J Leukoc Biol (2013) 93:933–42. doi: 10.1189/jlb.1012498
173. Démoulins T, Brügger M, Zumkehr B, Oliveira Esteves BI, Mehinagic K, Fahmi A, et al. The Specific Features of the Developing T Cell Compartment of the Neonatal Lung Are a Determinant of Respiratory Syncytial Virus Immunopathogenesis. PloS Pathog (2021) 17:e1009529. doi: 10.1371/journal.ppat.1009529
174. Hijano DR, Vu LD, Kauvar LM, Tripp RA, Polack FP, Cormier SA. Role of Type I Interferon (IFN) in the Respiratory Syncytial Virus (RSV) Immune Response and Disease Severity. Front Immunol (2019) 10:566. doi: 10.3389/fimmu.2019.00566
175. You D, Becnel D, Wang K, Ripple M, Daly M, Cormier SA. Exposure of Neonates to Respiratory Syncytial Virus Is Critical in Determining Subsequent Airway Response in Adults. Respir Res (2006) 7:107. doi: 10.1186/1465-9921-7-107
176. Dakhama A, Park J-W, Taube C, Joetham A, Balhorn A, Miyahara N, et al. The Enhancement or Prevention of Airway Hyperresponsiveness During Reinfection With Respiratory Syncytial Virus Is Critically Dependent on the Age at First Infection and IL-13 Production. J Immunol (2005) 175:1876–83. doi: 10.4049/jimmunol.175.3.1876
177. Krishnamoorthy N, Khare A, Oriss TB, Raundhal M, Morse C, Yarlagadda M, et al. Early Infection With Respiratory Syncytial Virus Impairs Regulatory T Cell Function and Increases Susceptibility to Allergic Asthma. Nat Med (2012) 18:1525–30. doi: 10.1038/nm.2896
178. You D, Siefker DT, Shrestha B, Saravia J, Cormier SA. Building a Better Neonatal Mouse Model to Understand Infant Respiratory Syncytial Virus Disease. Respir Res (2015) 16:91. doi: 10.1186/s12931-015-0244-0
179. Ripple MJ, You D, Honnegowda S, Giaimo JD, Sewell AB, Becnel DM, et al. Immunomodulation With IL-4R Alpha Antisense Oligonucleotide Prevents Respiratory Syncytial Virus-Mediated Pulmonary Disease. J Immunol (2010) 185:4804–11. doi: 10.4049/jimmunol.1000484
180. Srinivasa BT, Restori KH, Shan J, Cyr L, Xing L, Lee S, et al. STAT6 Inhibitory Peptide Given During RSV Infection of Neonatal Mice Reduces Exacerbated Airway Responses Upon Adult Reinfection. J Leukoc Biol (2017) 101:519–29. doi: 10.1189/jlb.4A0215-062RR
181. Dakhama A, Lee Y-M, Ohnishi H, Jing X, Balhorn A, Takeda K, et al. Virus-Specific IgE Enhances Airway Responsiveness on Reinfection With Respiratory Syncytial Virus in Newborn Mice. J Allergy Clin Immunol (2009) 123:138–145.e5. doi: 10.1016/j.jaci.2008.10.012
182. Thornburg NJ, Shepherd B, Crowe JE. Transforming Growth Factor Beta Is a Major Regulator of Human Neonatal Immune Responses Following Respiratory Syncytial Virus Infection. J Virol (2010) 84:12895–902. doi: 10.1128/JVI.01273-10
183. Eichinger KM, Egaña L, Orend JG, Resetar E, Anderson KB, Patel R, et al. Alveolar Macrophages Support Interferon Gamma-Mediated Viral Clearance in RSV-Infected Neonatal Mice. Respir Res (2015) 16:122. doi: 10.1186/s12931-015-0282-7
184. Harker JA, Lee DCP, Yamaguchi Y, Wang B, Bukreyev A, Collins PL, et al. Delivery of Cytokines by Recombinant Virus in Early Life Alters the Immune Response to Adult Lung Infection. J Virol (2010) 84:5294–302. doi: 10.1128/JVI.02503-09
185. Das S, Raundhal M, Chen J, Oriss TB, Huff R, Williams JV, et al. Respiratory Syncytial Virus Infection of Newborn CX3CR1-Deficient Mice Induces a Pathogenic Pulmonary Innate Immune Response. JCI Insight (2017) 2:1–15. doi: 10.1172/jci.insight.94605
186. Amanatidou V, Sourvinos G, Apostolakis S, Tsilimigaki A, Spandidos DA. T280M Variation of the CX3C Receptor Gene Is Associated With Increased Risk for Severe Respiratory Syncytial Virus Bronchiolitis. Pediatr Infect Dis J (2006) 25:410–4. doi: 10.1097/01.inf.0000214998.16248.b7
187. Das S, St Croix C, Good M, Chen J, Zhao J, Hu S, et al. Interleukin-22 Inhibits Respiratory Syncytial Virus Production by Blocking Virus-Mediated Subversion of Cellular Autophagy. iScience (2020) 23:101256. doi: 10.1016/j.isci.2020.101256
188. Zhivaki D, Lemoine S, Lim A, Morva A, Vidalain P-O, Schandene L, et al. Respiratory Syncytial Virus Infects Regulatory B Cells in Human Neonates via Chemokine Receptor CX3CR1 and Promotes Lung Disease Severity. Immunity (2017) 46:301–14. doi: 10.1016/j.immuni.2017.01.010
189. Tregoning JS, Wang BL, McDonald JU, Yamaguchi Y, Harker JA, Goritzka M, et al. Neonatal Antibody Responses Are Attenuated by Interferon-γ Produced by NK and T Cells During RSV Infection. Proc Natl Acad Sci USA (2013) 110:5576–81. doi: 10.1073/pnas.1214247110
190. Pyle CJ, Labeur-Iurman L, Groves HT, Puttur F, Lloyd CM, Tregoning JS, et al. Enhanced IL-2 in Early Life Limits the Development of TFH and Protective Antiviral Immunity. J Exp Med (2021) 218:1–17. doi: 10.1084/jem.20201555
191. Hijano DR, Siefker DT, Shrestha B, Jaligama S, Vu LD, Tillman H, et al. Type I Interferon Potentiates Iga Immunity to Respiratory Syncytial Virus Infection During Infancy. Sci Rep (2018) 8:11034. doi: 10.1038/s41598-018-29456-w
192. Dyer KD, Garcia-Crespo KE, Glineur S, Domachowske JB, Rosenberg HF. The Pneumonia Virus of Mice (PVM) Model of Acute Respiratory Infection. Viruses (2012) 4:3494–510. doi: 10.3390/v4123494
193. Rosenberg HF, Domachowske JB. Pneumonia Virus of Mice: Severe Respiratory Infection in a Natural Host. Immunol Lett (2008) 118:6–12. doi: 10.1016/j.imlet.2008.03.013
194. Bonville CA, Ptaschinski C, Percopo CM, Rosenberg HF, Domachowske JB. Inflammatory Responses to Acute Pneumovirus Infection in Neonatal Mice. Virol J (2010) 7:320. doi: 10.1186/1743-422X-7-320
195. Loh Z, Simpson J, Ullah A, Zhang V, Gan WJ, Lynch JP, et al. HMGB1 Amplifies ILC2-Induced Type-2 Inflammation and Airway Smooth Muscle Remodelling. PloS Pathog (2020) 16:e1008651. doi: 10.1371/journal.ppat.1008651
196. Lynch JP, Werder RB, Simpson J, Loh Z, Zhang V, Haque A, et al. Aeroallergen-Induced IL-33 Predisposes to Respiratory Virus-Induced Asthma by Dampening Antiviral Immunity. J Allergy Clin Immunol (2016) 138:1326–37. doi: 10.1016/j.jaci.2016.02.039
197. Siegle JS, Hansbro N, Herbert C, Rosenberg HF, Domachowske JB, Asquith KL, et al. Early-Life Viral Infection and Allergen Exposure Interact to Induce an Asthmatic Phenotype in Mice. Respir Res (2010) 11:14. doi: 10.1186/1465-9921-11-14
198. Schneider D, Hong JY, Popova AP, Bowman ER, Linn MJ, McLean AM, et al. Neonatal Rhinovirus Infection Induces Mucous Metaplasia and Airways Hyperresponsiveness. J Immunol (2012) 188:2894–904. doi: 10.4049/jimmunol.1101391
199. Han M, Rajput C, Hong JY, Lei J, Hinde JL, Wu Q, et al. The Innate Cytokines IL-25, IL-33, and TSLP Cooperate in the Induction of Type 2 Innate Lymphoid Cell Expansion and Mucous Metaplasia in Rhinovirus-Infected Immature Mice. J Immunol (2017) 199:1308–18. doi: 10.4049/jimmunol.1700216
200. Hong JY, Bentley JK, Chung Y, Lei J, Steenrod JM, Chen Q, et al. Neonatal Rhinovirus Induces Mucous Metaplasia and Airways Hyperresponsiveness Through IL-25 and Type 2 Innate Lymphoid Cells. J Allergy Clin Immunol (2014) 134:429–39. doi: 10.1016/j.jaci.2014.04.020
201. Hammad H, Lambrecht BN. Barrier Epithelial Cells and the Control of Type 2 Immunity. Immunity (2015) 43:29–40. doi: 10.1016/j.immuni.2015.07.007
202. Rajput C, Han M, Ishikawa T, Lei J, Jazaeri S, Bentley JK, et al. Early-Life Heterologous Rhinovirus Infections Induce an Exaggerated Asthma-Like Phenotype. J Allergy Clin Immunol (2020) 146:571–82.e3. doi: 10.1016/j.jaci.2020.03.039
203. Han M, Ishikawa T, Stroupe CC, Breckenridge HA, Bentley JK, Hershenson MB. Deficient Inflammasome Activation Permits an Exaggerated Asthma Phenotype in Rhinovirus C-Infected Immature Mice. Mucosal Immunol (2021) 14:1369–80. doi: 10.1038/s41385-021-00436-0
204. Han M, Hong JY, Jaipalli S, Rajput C, Lei J, Hinde JL, et al. IFN-γ Blocks Development of an Asthma Phenotype in Rhinovirus-Infected Baby Mice by Inhibiting Type 2 Innate Lymphoid Cells. Am J Respir Cell Mol Biol (2017) 56:242–51. doi: 10.1165/rcmb.2016-0056OC
205. Pancham K, Perez GF, Huseni S, Jain A, Kurdi B, Rodriguez-Martinez CE, et al. Premature Infants Have Impaired Airway Antiviral Ifnγ Responses to Human Metapneumovirus Compared to Respiratory Syncytial Virus. Pediatr Res (2015) 78:389–94. doi: 10.1038/pr.2015.113
206. Melendi GA, Laham FR, Monsalvo AC, Casellas JM, Israele V, Polack NR, et al. Cytokine Profiles in the Respiratory Tract During Primary Infection With Human Metapneumovirus, Respiratory Syncytial Virus, or Influenza Virus in Infants. Pediatrics (2007) 120:e410–5. doi: 10.1542/peds.2006-3283
207. Mikhail I, Grayson MH. Asthma and Viral Infections: An Intricate Relationship. Ann Allergy Asthma Immunol (2019) 123:352–8. doi: 10.1016/j.anai.2019.06.020
208. Kenmoe S, Bowo-Ngandji A, Kengne-Nde C, Ebogo-Belobo JT, Mbaga DS, Mahamat G, et al. Association Between Early Viral LRTI and Subsequent Wheezing Development, a Meta-Analysis and Sensitivity Analyses for Studies Comparable for Confounding Factors. PloS One (2021) 16:e0249831. doi: 10.1371/journal.pone.0249831
209. Kusel MMH, de Klerk NH, Kebadze T, Vohma V, Holt PG, Johnston SL, et al. Early-Life Respiratory Viral Infections, Atopic Sensitization, and Risk of Subsequent Development of Persistent Asthma. J Allergy Clin Immunol (2007) 119:1105–10. doi: 10.1016/j.jaci.2006.12.669
210. Lemanske RF, Jackson DJ, Gangnon RE, Evans MD, Li Z, Shult PA, et al. Rhinovirus Illnesses During Infancy Predict Subsequent Childhood Wheezing. J Allergy Clin Immunol (2005) 116:571–7. doi: 10.1016/j.jaci.2005.06.024
211. Jackson DJ, Gangnon RE, Evans MD, Roberg KA, Anderson EL, Pappas TE, et al. Wheezing Rhinovirus Illnesses in Early Life Predict Asthma Development in High-Risk Children. Am J Respir Crit Care Med (2008) 178:667–72. doi: 10.1164/rccm.200802-309OC
212. Makrinioti H, Maggina P, Lakoumentas J, Xepapadaki P, Taka S, Megremis S, et al. Recurrent Wheeze Exacerbations Following Acute Bronchiolitis—A Machine Learning Approach. Front Allergy (2021) 2:728389. doi: 10.3389/falgy.2021.728389
213. Hasegawa K, Mansbach JM, Bochkov YA, Gern JE, Piedra PA, Bauer CS, et al. Association of Rhinovirus C Bronchiolitis and Immunoglobulin E Sensitization During Infancy With Development of Recurrent Wheeze. JAMA Pediatr (2019) 173:544–52. doi: 10.1001/jamapediatrics.2019.0384
214. Calışkan M, Bochkov YA, Kreiner-Møller E, Bønnelykke K, Stein MM, Du G, et al. Rhinovirus Wheezing Illness and Genetic Risk of Childhood-Onset Asthma. N Engl J Med (2013) 368:1398–407. doi: 10.1056/NEJMoa1211592
215. Moffatt MF, Gut IG, Demenais F, Strachan DP, Bouzigon E, Heath S, et al. A Large-Scale, Consortium-Based Genomewide Association Study of Asthma. N Engl J Med (2010) 363:1211–21. doi: 10.1056/NEJMoa0906312
216. Zhou Y, Tong L, Li M, Wang Y, Li L, Yang D, et al. Recurrent Wheezing and Asthma After Respiratory Syncytial Virus Bronchiolitis. Front Pediatr (2021) 9:649003. doi: 10.3389/fped.2021.649003
217. Sigurs N, Aljassim F, Kjellman B, Robinson PD, Sigurbergsson F, Bjarnason R, et al. Asthma and Allergy Patterns Over 18 Years After Severe RSV Bronchiolitis in the First Year of Life. Thorax (2010) 65:1045–52. doi: 10.1136/thx.2009.121582
218. Raita Y, Pérez-Losada M, Freishtat RJ, Harmon B, Mansbach JM, Piedra PA, et al. Integrated Omics Endotyping of Infants With Respiratory Syncytial Virus Bronchiolitis and Risk of Childhood Asthma. Nat Commun (2021) 12:3601. doi: 10.1038/s41467-021-23859-6
219. Stensballe LG, Simonsen JB, Thomsen SF, Larsen A-MH, Lysdal SH, Aaby P, et al. The Causal Direction in the Association Between Respiratory Syncytial Virus Hospitalization and Asthma. J Allergy Clin Immunol (2009) 123:131–137.e1. doi: 10.1016/j.jaci.2008.10.042
220. Thomsen SF, van der Sluis S, Stensballe LG, Posthuma D, Skytthe A, Kyvik KO, et al. Exploring the Association Between Severe Respiratory Syncytial Virus Infection and Asthma: A Registry-Based Twin Study. Am J Respir Crit Care Med (2009) 179:1091–7. doi: 10.1164/rccm.200809-1471OC
221. Szabo SM, Levy AR, Gooch KL, Bradt P, Wijaya H, Mitchell I. Elevated Risk of Asthma After Hospitalization for Respiratory Syncytial Virus Infection in Infancy. Paediatr Respir Rev (2013) 13 Suppl 2:S9–15. doi: 10.1016/S1526-0542(12)70161-6
222. Coverstone AM, Wilson B, Burgdorf D, Schechtman KB, Storch GA, Holtzman MJ, et al. Recurrent Wheezing in Children Following Human Metapneumovirus Infection. J Allergy Clin Immunol (2018) 142:297–301.e2. doi: 10.1016/j.jaci.2018.02.008
223. García-García ML, Calvo C, Casas I, Bracamonte T, Rellán A, Gozalo F, et al. Human Metapneumovirus Bronchiolitis in Infancy Is an Important Risk Factor for Asthma at Age 5. Pediatr Pulmonol (2007) 42:458–64. doi: 10.1002/ppul.20597
224. Broughton S, Sylvester KP, Fox G, Zuckerman M, Smith M, Milner AD, et al. Lung Function in Prematurely Born Infants After Viral Lower Respiratory Tract Infections. Pediatr Infect Dis J (2007) 26:1019–24. doi: 10.1097/INF.0b013e318126bbb9
225. Lay MK, Céspedes PF, Palavecino CE, León MA, Díaz RA, Salazar FJ, et al. Human Metapneumovirus Infection Activates the TSLP Pathway That Drives Excessive Pulmonary Inflammation and Viral Replication in Mice. Eur J Immunol (2015) 45:1680–95. doi: 10.1002/eji.201445021
226. Gu W, Wang Y, Hao C, Zhang X, Yan Y, Chen Z, et al. Elevated Serum Levels of Thymic Stromal Lymphopoietin in Wheezing Children Infected With Human Metapneumovirus. Jpn J Infect Dis (2017) 70:161–6. doi: 10.7883/yoken.JJID.2016.047
227. Bønnelykke K, Vissing NH, Sevelsted A, Johnston SL, Bisgaard H. Association Between Respiratory Infections in Early Life and Later Asthma Is Independent of Virus Type. J Allergy Clin Immunol (2015) 136:81–86.e4. doi: 10.1016/j.jaci.2015.02.024
228. Di Cicco M, D’Elios S, Peroni DG, Comberiati P. The Role of Atopy in Asthma Development and Persistence. Curr Opin Allergy Clin Immunol (2020) 20:131–7. doi: 10.1097/ACI.0000000000000627
229. van Woensel J, Kimpen J. Therapy for Respiratory Tract Infections Caused by Respiratory Syncytial Virus. Eur J Pediatr (2000) 159:391–8. doi: 10.1007/s004310051295
230. Mazur NI, Higgins D, Nunes MC, Melero JA, Langedijk AC, Horsley N, et al. The Respiratory Syncytial Virus Vaccine Landscape: Lessons From the Graveyard and Promising Candidates. Lancet Infect Dis (2018) 18:e295–311. doi: 10.1016/S1473-3099(18)30292-5
231. Kim HW, Canchola JG, Brandt CD, Pyles G, Chanock RM, Jensen K, et al. Respiratory Syncytial Virus Disease in Infants Despite Prior Administration of Antigenic Inactivated Vaccine. Am J Epidemiol (1969) 89:422–34. doi: 10.1093/oxfordjournals.aje.a120955
232. Chin J, Magoffin RL, Shearer LA, Schieble JH, Lennette EH. Field Evaluation of a Respiratory Syncytial Virus Vaccine and a Trivalent Parainfluenza Virus Vaccine in a Pediatric Population. Am J Epidemiol (1969) 89:449–63. doi: 10.1093/oxfordjournals.aje.a120957
233. Neuzil KM. Progress Toward a Respiratory Syncytial Virus Vaccine. Clin Vaccine Immunol (2016) 23:186–8. doi: 10.1128/CVI.00037-16
234. Eichinger KM, Kosanovich JL, Gidwani SV, Zomback A, Lipp MA, Perkins TN, et al. Prefusion RSV F Immunization Elicits Th2-Mediated Lung Pathology in Mice When Formulated With a Th2 (But Not a Th1/Th2-Balanced) Adjuvant Despite Complete Viral Protection. Front Immunol (2020) 11:1673. doi: 10.3389/fimmu.2020.01673
235. Delgado MF, Coviello S, Monsalvo AC, Melendi GA, Hernandez JZ, Batalle JP, et al. Lack of Antibody Affinity Maturation Due to Poor Toll-Like Receptor Stimulation Leads to Enhanced Respiratory Syncytial Virus Disease. Nat Med (2009) 15:34–41. doi: 10.1038/nm.1894
236. Acosta PL, Caballero MT, Polack FP. Brief History and Characterization of Enhanced Respiratory Syncytial Virus Disease. Clin Vaccine Immunol (2015) 23:189–95. doi: 10.1128/CVI.00609-15
237. Polack FP, Alvarez-Paggi D, Libster R, Caballero MT, Blair RV, Hijano DR, et al. Fatal Enhanced Respiratory Syncytial Virus Disease in Toddlers. Sci Transl Med (2021) 13:eabj7843. doi: 10.1126/scitranslmed.abj7843
238. PATH. RSV Vaccine and mAb Snapshot. (2021) PATH snapshot. Available at: https://www.path.org/resources/rsv-vaccine-and-mab-snapshot/.
239. Madhi SA, Polack FP, Piedra PA, Munoz FM, Trenholme AA, Simões EAF, et al. Respiratory Syncytial Virus Vaccination During Pregnancy and Effects in Infants. N Engl J Med (2020) 383:426–39. doi: 10.1056/NEJMoa1908380
240. Tang RS, Mahmood K, Macphail M, Guzzetta JM, Haller AA, Liu H, et al. A Host-Range Restricted Parainfluenza Virus Type 3 (PIV3) Expressing the Human Metapneumovirus (hMPV) Fusion Protein Elicits Protective Immunity in African Green Monkeys. Vaccine (2005) 23:1657–67. doi: 10.1016/j.vaccine.2004.10.009
241. Palavecino CE, Céspedes PF, Gómez RS, Kalergis AM, Bueno SM. Immunization With a Recombinant Bacillus Calmette-Guerin Strain Confers Protective Th1 Immunity Against the Human Metapneumovirus. J Immunol (2014) 192:214–23. doi: 10.4049/jimmunol.1300118
242. Biacchesi S, Pham QN, Skiadopoulos MH, Murphy BR, Collins PL, Buchholz UJ. Infection of Nonhuman Primates With Recombinant Human Metapneumovirus Lacking the SH, G, or M2-2 Protein Categorizes Each as a Nonessential Accessory Protein and Identifies Vaccine Candidates. J Virol (2005) 79:12608–13. doi: 10.1128/JVI.79.19.12608-12613.2005
243. Buchholz UJ, Biacchesi S, Pham QN, Tran KC, Yang L, Luongo CL, et al. Deletion of M2 Gene Open Reading Frames 1 and 2 of Human Metapneumovirus: Effects on RNA Synthesis, Attenuation, and Immunogenicity. J Virol (2005) 79:6588–97. doi: 10.1128/JVI.79.11.6588-6597.2005
244. Herfst S, de Graaf M, Schrauwen EJA, Sprong L, Hussain K, van den Hoogen BG, et al. Generation of Temperature-Sensitive Human Metapneumovirus Strains That Provide Protective Immunity in Hamsters. J Gen Virol (2008) 89:1553–62. doi: 10.1099/vir.0.2008/002022-0
245. Herfst S, Schrauwen EJA, de Graaf M, van Amerongen G, van den Hoogen BG, de Swart RL, et al. Immunogenicity and Efficacy of Two Candidate Human Metapneumovirus Vaccines in Cynomolgus Macaques. Vaccine (2008) 26:4224–30. doi: 10.1016/j.vaccine.2008.05.052
246. Cox RG, Erickson JJ, Hastings AK, Becker JC, Johnson M, Craven RE, et al. Human Metapneumovirus Virus-Like Particles Induce Protective B and T Cell Responses in a Mouse Model. J Virol (2014) 88:6368–79. doi: 10.1128/JVI.00332-14
247. Lévy C, Aerts L, Hamelin M-È, Granier C, Szécsi J, Lavillette D, et al. Virus-Like Particle Vaccine Induces Cross-Protection Against Human Metapneumovirus Infections in Mice. Vaccine (2013) 31:2778–85. doi: 10.1016/j.vaccine.2013.03.051
248. Talaat KR, Karron RA, Thumar B, McMahon BA, Schmidt AC, Collins PL, et al. Experimental Infection of Adults With Recombinant Wild-Type Human Metapneumovirus. J Infect Dis (2013) 208:1669–78. doi: 10.1093/infdis/jit356
249. Lee S, Nguyen MT, Currier MG, Jenkins JB, Strobert EA, Kajon AE, et al. A Polyvalent Inactivated Rhinovirus Vaccine Is Broadly Immunogenic in Rhesus Macaques. Nat Commun (2016) 7:12838. doi: 10.1038/ncomms12838
250. McLean GR. Developing a Vaccine for Human Rhinoviruses. J Vaccines Immun (2014) 2:16–20. doi: 10.14312/2053-1273.2014-3
251. Andabaka T, Nickerson JW, Rojas-Reyes MX, Rueda JD, Bacic Vrca V, Barsic B. Monoclonal Antibody for Reducing the Risk of Respiratory Syncytial Virus Infection in Children. Cochrane Database Syst Rev (2013) 4:CD006602. doi: 10.1002/14651858.CD006602.pub4
252. Mejías A, Ramilo O. Review of Palivizumab in the Prophylaxis of Respiratory Syncytial Virus (RSV) in High-Risk Infants. Biologics (2008) 2:433–9. doi: 10.2147/BTT.S3104
253. Blanken MO, Rovers MM, Molenaar JM, Winkler-Seinstra PL, Meijer A, Kimpen JLL, et al. Dutch RSV Neonatal Network. Respiratory Syncytial Virus and Recurrent Wheeze in Healthy Preterm Infants. N Engl J Med (2013) 368:1791–9. doi: 10.1056/NEJMoa1211917
254. Mac S, Sumner A, Duchesne-Belanger S, Stirling R, Tunis M, Sander B. Cost-Effectiveness of Palivizumab for Respiratory Syncytial Virus: A Systematic Review. Pediatrics (2019) 143:1–21. doi: 10.1542/peds.2018-4064
255. Griffin MP, Yuan Y, Takas T, Domachowske JB, Madhi SA, Manzoni P, et al. Single-Dose Nirsevimab for Prevention of RSV in Preterm Infants. N Engl J Med (2020) 383:415–25. doi: 10.1056/NEJMoa1913556
256. Nourbakhsh S, Shoukat A, Zhang K, Poliquin G, Halperin D, Sheffield H, et al. Effectiveness and Cost-Effectiveness of RSV Infant and Maternal Immunization Programs: A Case Study of Nunavik, Canada. EClinicalMedicine (2021) 41:101141. doi: 10.1016/j.eclinm.2021.101141
257. Ulbrandt ND, Ji H, Patel NK, Riggs JM, Brewah YA, Ready S, et al. Isolation and Characterization of Monoclonal Antibodies Which Neutralize Human MetapneumovirusIn Vitro and In Vivo. J Virol (2006) 80:7799–806. doi: 10.1128/JVI.00318-06
258. Williams JV, Chen Z, Cseke G, Wright DW, Keefer CJ, Tollefson SJ, et al. A Recombinant Human Monoclonal Antibody to Human Metapneumovirus Fusion Protein That Neutralizes Virus In Vitro and Is Effective Therapeutically In Vivo. J Virol (2007) 81:8315–24. doi: 10.1128/JVI.00106-07
259. Corti D, Bianchi S, Vanzetta F, Minola A, Perez L, Agatic G, et al. Cross-Neutralization of Four Paramyxoviruses by a Human Monoclonal Antibody. Nature (2013) 501:439–43. doi: 10.1038/nature12442
260. Schuster JE, Cox RG, Hastings AK, Boyd KL, Wadia J, Chen Z, et al. A Broadly Neutralizing Human Monoclonal Antibody Exhibits In Vivo Efficacy Against Both Human Metapneumovirus and Respiratory Syncytial Virus. J Infect Dis (2015) 211:216–25. doi: 10.1093/infdis/jiu307
261. Privolizzi R, Solari R, Johnston SL, McLean GR. The Application of Prophylactic Antibodies for Rhinovirus Infections. Antivir Chem Chemother (2014) 23:173–7. doi: 10.3851/IMP2578
262. Centers for Disease Control and Prevention. Health. The National Respiratory and Enteric Virus Surveillance System (NREVSS). In: National Center for Immunization and Respiratory Diseases (NCIRD), Division of Viral Diseases. Washington, DC, USA: Center for Disease Control and prevention. U.S. Department of Health & Human Services (2022). Available at: https://www.cdc.gov/surveillance/nrevss/index.html.
Keywords: neonate, lung, respiratory virus, RSV, rhinovirus, human metapneumovirus
Citation: Eddens T, Parks OB and Williams JV (2022) Neonatal Immune Responses to Respiratory Viruses. Front. Immunol. 13:863149. doi: 10.3389/fimmu.2022.863149
Received: 26 January 2022; Accepted: 23 March 2022;
Published: 14 April 2022.
Edited by:
Kerry M. Empey, University of Pittsburgh, United StatesReviewed by:
Phil Stumbles, University of Western Australia, AustraliaJames Harker, Imperial College London, United Kingdom
Copyright © 2022 Eddens, Parks and Williams. This is an open-access article distributed under the terms of the Creative Commons Attribution License (CC BY). The use, distribution or reproduction in other forums is permitted, provided the original author(s) and the copyright owner(s) are credited and that the original publication in this journal is cited, in accordance with accepted academic practice. No use, distribution or reproduction is permitted which does not comply with these terms.
*Correspondence: John V. Williams, SnZ3QGNocC5lZHU=