- 1Department of Infectious Diseases, School of Immunology and Microbial Sciences, King’s College London, London, United Kingdom
- 2Immunoregulation Laboratory, Francis Crick Institute, London, United Kingdom
Type III interferons (IFNs), or IFNλs, are cytokines produced in response to microbial ligands. They signal through the IFNλ receptor complex (IFNLR), which is located on epithelial cells and select immune cells at barrier sites. As well as being induced during bacterial or viral infection, type III IFNs are produced in response to the microbiota in the lung and intestinal epithelium where they cultivate a resting antiviral state. While the multiple anti-viral activities of IFNλs have been extensively studied, their roles in immunity against bacteria are only recently emerging. Type III IFNs increase epithelial barrier integrity and protect from infection in the intestine but were shown to increase susceptibility to bacterial superinfections in the respiratory tract. Therefore, the effects of IFNλ can be beneficial or detrimental to the host during bacterial infections, depending on timing and biological contexts. This duality will affect the potential benefits of IFNλs as therapeutic agents. In this review, we summarize the current knowledge on IFNλ induction and signaling, as well as their roles at different barrier sites in the context of anti-bacterial immunity.
1 Type III Interferon Induction and Signaling
1.1 Type III Interferons and Their Receptor
Type III interferons (IFNs), also called IFNλs, are the most recent addition to the IFN family. These class II cytokines include two more members: type I and II IFNs. Type I IFNs include IFNαs and IFNβ and are functionally similar to IFNλs. They are produced by many cell types and signal through the IFNα Receptor (IFNAR), which is expressed on nearly every nucleated cell (1, 2). Type II IFN only includes IFNγ and is the most phylogenetically and functionally distinct (3, 4). The type III IFN family is comprised of 4 members in humans: IFNλ1 (IL-29), IFNλ2 (IL-28A), IFNλ3 (IL-28B), and the most recently identified IFNλ4. Mice express IFNλ2 and IFNλ3, but murine IFNλ1 and IFNλ4 are pseudogenes (5). Type III IFNs are induced by both epithelial and immune cells (6, 7), in particular dentritic cells (DC) (8–11). Type I and III IFNs are produced in response to the detection of Microbe-Associated Molecular Patterns (MAMPs) by host Pattern Recognition Receptors (PRRs) (12–17). Secreted IFNλs signal in an autocrine and paracrine manner through a common heterodimeric receptor, IFNLR, composed of the high affinity type III IFN receptor (IFNLR1, also known as IL-28Ra) and the Interleukin 10 Receptor 2 (IL-10R2) (18). IFNLR is mostly expressed at barrier sites and was initially thought to be only present on epithelial cells (19–21). However, increasing evidence has demonstrated the expression of IFNLR on multiple immune cell subsets such as neutrophils (22), macrophages (23), plasmacytoid dentritic cells (pDCs) (8, 24) and lymphocytes (5, 25, 26). Human natural killer (NK) cells do not appear to express IFNLR (27), whereas mouse NK cells express IFNLR1 and potently respond to IFNλ (28). This selective distribution of IFNLR distinguishes type III IFNs from the ubiquitously sensed type I IFNs (1, 29).
1.2 Production of Type III IFNs in Response to Bacterial Ligands
MAMPs are conserved microbe-specific structures that are produced by both pathogenic and non-pathogenic microorganisms. They include nucleic acids and bacterial cell components like flagellin, present in the flagella of motile bacteria (30); peptidoglycan, found in the cell wall of most bacteria (31); or lipopolysaccharide (LPS) from the outer membrane of Gram-negative bacteria (32). Detection of both Gram-positive and Gram-negative bacteria by epithelial and innate immune cells was shown to induce type III IFNs to similar or greater levels than type I IFNs (13, 16, 33–36). IFNλs are induced via stimulation of all PRRs that induce type I IFN (37), including the cytosolic DNA and RNA sensors cGAS (38–43) RIG-I-like receptors (RLRs) (44) and endosomal Toll-like receptors (TLRs) (16, 35, 45). However, several signaling pathways have been shown to preferentially drive IFNλ expression (16, 33, 35, 46). For example, following detection of LPS by TLR4, IFNβ and IFNλ are induced via distinct mechanisms. While the induction of IFNβ does not occur until TLR4 reaches endosomes, and is independent of the adaptor MyD88 (47–49), IFNλ production occurs from the plasma membrane and requires MyD88 (16). Similarly, TLR2 and TLR5 that detect bacterial components from the plasma membrane do not strongly induce type I IFNs (16, 50–52), but potently induce type III IFN expression (16). Therefore, IFNλs seem to be preferentially induced in response to ligands of bacterial origin, hinting at possible roles of these IFNs in antibacterial immunity.
1.3 IFNλ-Mediated Signaling and Anti-Bacterial ISGs
Although type I and type III IFNs bind unique receptors, the signaling pathways downstream of IFNLR and IFNAR activation are similar. In both cases, phosphorylated Janus kinase (JAK) family proteins activate Signal Transducer and Activator of Transcription 1 (STAT1) and STAT2. Activated STAT1/2 form a complex with the IFN regulatory factor 9 (IRF9), called the IFN stimulated gene factor 3 (ISGF3). ISGF3 translocates into the nucleus where it binds IFN-stimulated response elements (ISREs) located in the promoters of IFN-stimulated genes (ISGs) (19, 29). Despite these similarities, the combination of JAK kinases activated by type I and III IFNs may be different. Some studies have suggested that JAK2 may mediate IFNLR, but not IFNAR, signaling (22, 35, 53). In addition, Tyrosine kinase 2 (TYK2) is required for type I IFN signaling, but seems to be dispensable for IFNλ signaling (54–56).
Hundreds of ISGs are expressed in response to IFNs and can modulate innate and adaptive immunity to promote microbial clearance. The ISGs expressed in response to type I and type III IFNs overlap greatly, however the kinetics of their production differ. Type I IFNs typically induce a strong, rapid expression of ISGs, whereas the type III IFN response is slower and of a lesser magnitude (19, 29, 57–61). Although the functions of IFNλ and ISGs have been predominantly studied in the context of viral infection (62, 63), emerging evidence indicates a protective role of some ISGs in bacterial infection. For example, the anti-viral IFN induced transmembrane (IFITM) proteins have been shown to restrict Mycobacterium tuberculosis intracellular growth (64) and the ISG Viperin inhibits the entry of Shigella into epithelial cells (65). Additionally, guanylate-binding proteins (GBPs) bind the LPS of Gram-negative bacteria, facilitating activation of the non-canonical inflammasome (66–69). GBPs also interfere with actin-based motility of Shigella flexneri, hindering bacterial dissemination (70, 71). Given their emerging antibacterial roles, ISGs are attractive targets for bacterial virulence factors (72). This is exemplified by the Shigella ubiquitin ligase IpaH9.8, which targets GBPs for proteosomal degradation (69, 71, 73).
The production of IFNλs and ISGs in response to bacterial ligands, in conjunction with the privileged localisation of IFNLR, raises interest regarding the function of IFNλ at barrier sites. Here, we summarize the roles of IFNλ in mucosal epithelia during disease and homeostasis.
2 Type III IFNs in Anti-Bacterial Mucosal Immunity
2.1 Type III IFNs and Epithelial Barriers
2.1.1 Intestinal Epithelial Barriers
Compartmentalisation by epithelial barriers is critical in the intestine, as they not only protect the host from potential pathogens but also separate the underlying tissue from foreign material ingested by the host. IFNλs protect intestinal epithelial barrier integrity (16, 74, 75). In a mouse model of colitis induced by dextran sulfate sodium (DSS), IFNλs were shown to control the proliferation of intestinal epithelial cells and accelerate intestinal mucosal healing, which reduced epithelial cell damage (15, 22, 76).
The protective role of IFNλ in intestinal epithelia extends to the context of bacterial infection. Treatment with IFNλ1 was shown to increase trans-epithelial electrical resistance (TEER) in an in vitro model of barrier integrity using polarized T84 colonic epithelial cells (16). Invasive enteric pathogens such as S. flexneri and Salmonella enterica serovar Typhimurium disrupt epithelial barriers to aid bacterial dissemination. While TEER dropped upon infection with these bacteria in control conditions, it was maintained following IFNλ1 pre-treatment. In addition, IFNλ treatment prevented S. flexneri and S. Typhimurium transmigration, showing that IFNλ protects intestinal epithelial barriers from infection and damage mediated by invasive bacteria in vitro (16) (Figure 1). The molecular mechanism of action of IFNλs-mediated protection of barriers has not been uncovered, but intracellular tight junction proteins like claudin-1 were shown to be upregulated by IFNλ2 (75). Whether these observations in cell culture extend to in vivo models of bacterial infection remains to be determined.
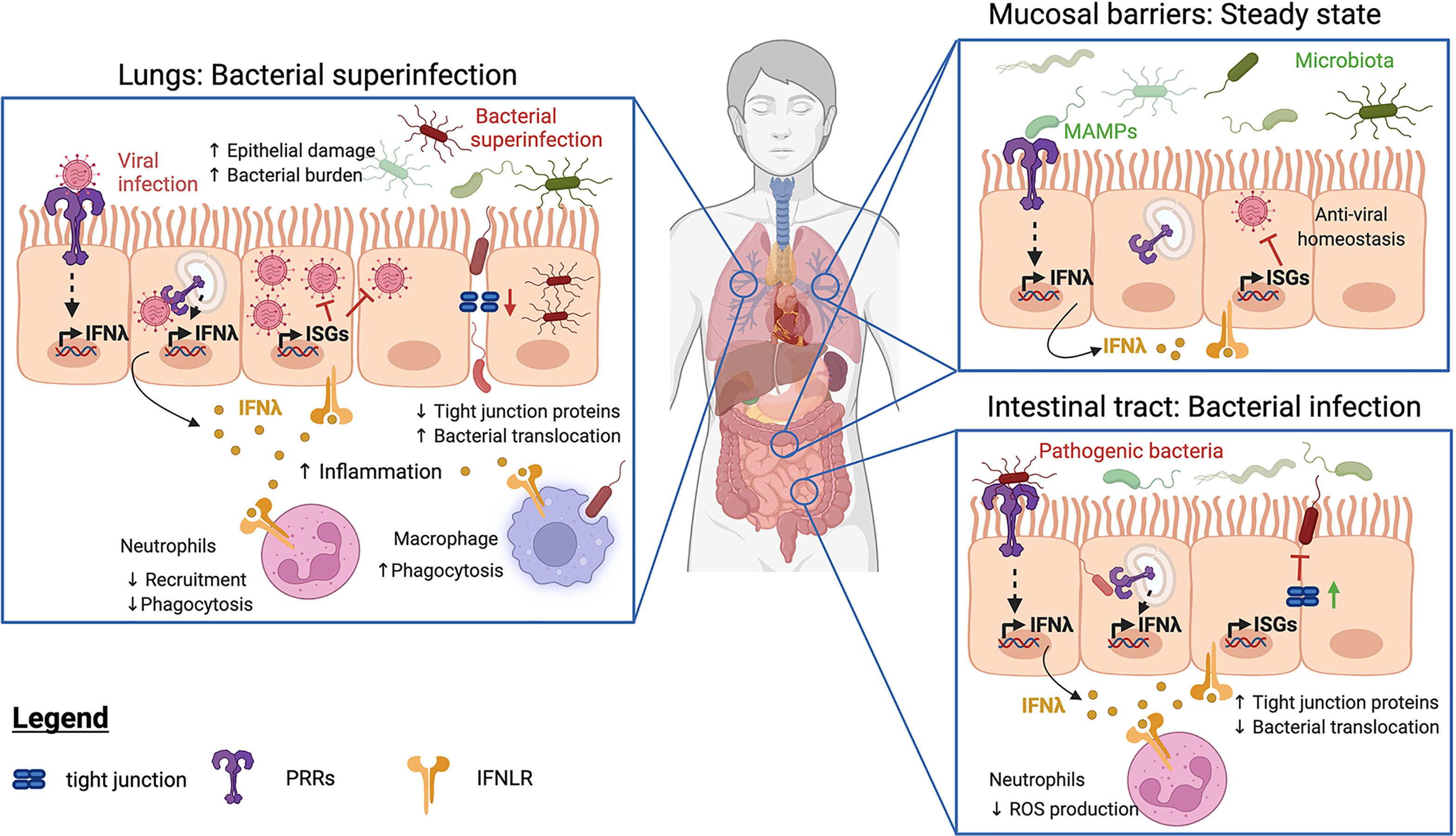
Figure 1 Functions of IFNλ at barrier sites at steady state and during bacterial infection. At barrier sites, detection of Microbe-Associated Molecular Patterns (MAMPs) by Pattern Recognition Receptors (PRRs) triggers IFNλ production (in yellow) and subsequent expression of IFN-stimulated genes (ISGs). IFNλ can enhance (green lines) or inhibit (red lines) host immune responses. In infected or inflamed lungs (left panel), IFNλ and ISGs restrict neutrophil recruitment, resulting in increased bacterial burdens. IFNλ also decreases tight junction protein production, facilitating bacterial translocation and promoting bacterial superinfections. In contrast, IFNλ promotes the phagocytic activity of macrophages. During bacterial infections of the intestinal tract (lower right panel), IFNλ strengthens tight junction proteins, preventing bacterial transmigration. IFNλ also impairs neutrophil recruitment and reactive oxygen species (ROS) production to limit tissue destruction. At both sites (top right panel), steady-state detection of the bacterial microbiota induces the production of IFNλs, whose signaling drives homeostatic expression of ISGs. The resulting basal ISG response protects against viral infections.
2.1.2 Airways Epithelial Barriers
While type III IFNs appear to protect against enteric bacterial infections, their roles in the airway epithelium are unclear. At steady state, IFNλs modulate immune cell responses to decrease inflammation (24, 25, 77, 78). However, during infection, the activities of type III IFNs on lung epithelial cells were also shown to increase inflammation and compromise barrier function (79–82) (Figure 1). During infection with Klebsiella pneumoniae, IFNλ treatment lowered epithelial barrier integrity in vitro, facilitating neutrophil transmigration and bacterial translocation. Moreover, Ifnlr1-/- mice were protected from Klebsiella-induced pneumonia (79). Similarly, IFNλ exacerbated lung inflammatory pathology in a mouse model of Bordetella pertussis infection (80). In this study, antibody neutralization of IFNλ, as well as deletion of IFNLR, led to reduced lung inflammatory pathology (80), congruent with a detrimental role of type III IFN in bacteria-infected lungs.
Compromised barrier functions and increased inflammation during viral infection in the lungs can result in complications such as bacterial superinfection (81–84). Infection with Influenza A virus (IAV) or intratracheal instillation of poly (I:C), a synthetic ligand that mimics viral dsRNA, were shown to up-regulate IFNλ2 and IFNλ3. This led to compromised barrier function and promoted superinfection by Staphylococcus aureus (81) and S. pneumoniae (82). Greater barrier damage and higher bacterial burdens were found in mice treated with poly (I:C), a phenotype reversed in mice lacking IFNLR. Moreover, the co-administration of IFNλ and R848 (a TLR7 agonist that mimics viral ssRNA), but not IFNλ alone, increased sensitivity to S. aureus infection. This suggests that IFNλ promotes superinfections in inflamed lungs by driving inflammation and compromising barrier function (81) (Figure 1).
IFNλ was also shown to impair neutrophil recruitment and phagocytosis during recovery from IAV infection (85). This enhanced superinfections with methicillin-resistant S. aureus (MRSA) or S. pneumoniae (82, 83). Consequently, mice lacking IFNLR that were infected with IAV were less susceptible to superinfections (82, 83).
Although type III IFNs can be protective in the intestinal epithelium and healthy lung, it is evident that they can have a detrimental role in inflamed airways. These findings emphasize that biological context is crucial, and further research is required to understand the nuanced effects of IFNs at epithelial barriers.
2.2 Type III IFNs and the Microbiota
Mucosal barriers serve to protect the host in a multitude of ways. As well as forming a physical barrier, they maintain a tolerance to the commensal microorganisms that make up the microbiota. This population benefits the host by inhibiting infection by pathogenic species, as well as contributing to host metabolism (86).
2.2.1 Intestinal Microbiota
At steady-state, ISGs are detected in mouse and human intestinal epithelial cells (87–90). The expression of ISGs is dependent on PRR-mediated sensing of the microbiota, particularly in the ileum, and expression of specific ISGs was found to be ablated following antibiotic treatment to eliminate the microbiota (87, 90). Interestingly, homeostatic ISG expression was shown to control enteric viral infections (89), which benefits the host (Figure 1).
2.2.2 Airway Microbiota
Although the pulmonary microbiota is poorly understood (91–93), a homeostatic ISG response has also been found in mouse lungs (89, 94). Emerging evidence demonstrates that IFNλ is also important for regulating the microbiota and influencing infection at this mucosal barrier in humans (Figure 1).
One study investigated the microbiota composition using random sampling of bronchoalveolar lavage fluid (BALF) from lung transplant recipients. Post-transplant lung microbiota were categorised into four ‘pneumotypes’. In balanced pneumotype, BALF samples analysis of differential expression of host genes, revealed that Ifnlr1 was upregulated, relative to the other groups. This balanced pneumotype was associated with the lowest risk of infection and allogenic responses, resulting in a lower clinical risk (95). Moreover, in a study assessing the contribution of nasal commensal bacteria in antiviral defense against IAV infection in the upper respiratory tract, the commensal bacterium Staphylococcus epidermidis was shown to reduce host susceptibility to IAV infection by inducing the expression of IFNλ (96).
2.3 Type III IFNs and Immune Cells
It was initially believed that the IFNLR was solely expressed by epithelial cells, but it has since been demonstrated to be present on immune cells. Neutrophils (22), macrophages (23), pDCs (8, 24), T cells, B cells (5, 25, 26) and mouse NK cells (28) express IFNLR. These cells exhibit limited basal expression of IFNLR, but can rapidly increase expression upon stimulation of TLRs (10, 22, 28), T cell receptor (TCR) (23, 26) or B cell receptor (BCR) (26).
2.3.1 Neutrophils
Neutrophils are phagocytes that can engulf foreign materials and pathogens. They also potently produce microbicidal reactive oxygen species (ROS) and promote inflammation via degranulation. However, excessive or prolonged neutrophil activation is a hallmark of many inflammatory pathologies. IFNLR is expressed at higher levels in human and mouse neutrophils than other immune or epithelial cells (22, 25, 78, 97), and its expression in neutrophils is further upregulated by bacterial ligands such as LPS (22). While IFNβ elicits pro-inflammatory activities in neutrophils (78), IFNλs cultivate an anti-inflammatory state by inhibiting neutrophil recruitment (22, 25, 85). Crucially, IFNλ treatment of bone marrow derived neutrophils diminished ROS production and degranulation but did not affect protective cytokine production or phagocytosis. This was protective in the intestine in a mouse model of DSS-induced colitis (22).
Neutrophils are efficient phagocytes and are essential to the resolution of infection. Despite their crucial roles, the functions of IFNλ in neutrophils in the context of bacterial infections have seldom been addressed. In models of bacterial superinfection in murine lungs, IFNλ was shown to reduce the phagocytic abilities of neutrophils which impaired bacterial clearance, exposing the host to infection (85). However, during infection with Pseudomonas aeruginosa, an IFNλ2-mediated decrease in neutrophil recruitment was protective and resulted in less epithelial damage (98) (Figure 1). As such, the balance between inhibiting neutrophil-mediated inflammatory damage and promoting bacterial clearance should be carefully considered when evaluating the therapeutic potential of IFNλ.
2.3.2 Macrophages
Macrophages are phagocytes that engulf and destroy bacteria. They can also stimulate the adaptive immune system via the presentation of foreign antigens to T cells. Exposure to IFNλ increases their phagocytic activity and the production of proinflammatory cytokines and chemokines (23). This promotes bacterial clearance during infection; IFNλ1 was shown to enhance S. aureus uptake in macrophages, and increase bacterial killing (99) (Figure 1).
Interestingly, when monocytes are incubated with Granulocyte Macrophage Colony-Stimulating Factor (GM-CSF) or Macrophage Colony-Stimulating Factor (M-CSF) to respectively produce M1- or M2-shifted macrophages, the differentiated macrophages respond differently to IFNλ3. GM-CSF differentiated macrophages demonstrated greater IFNLR1 expression and had increased levels of STAT1 phosphorylation and ISG expression in response to IFNλ3 than M-CSF differentiated macrophages (23). Moreover, GM-CSF differentiated macrophages exhibited a pro-inflammatory profile and were more potent at recruiting leukocytes and NK cells upon IFNλ3 stimulation. Interestingly, when stimulated with type I IFN, these macrophages did not exhibit a pro-inflammatory phenotype and were incapable of recruiting leukocyte and NK cells (23).
2.3.3 Other Immune Cells
IFNλ also alters the function of pDCs (8, 24), T and B lymphocytes (5, 26) and mouse NK cells (28). However, whether these effects have an impact on bacterial infections has yet to be explored. pDCs are antigen presenting cells which secrete cytokines that recruit and activate cells of the adaptive immune system. IFNλ3 was shown to prolong the survival of human pDCs in vitro, as well as increase their immunoreactivity and ISG response (100). IFNλ was also demonstrated to enhance activation of, and antibody production by, human B lymphocytes (101, 102) and skew the T helper 1 (Th1)/Th2 balance to a Th1-pro-inflammatory response (103–105). Finally, in an in vivo model of bacterial-induced inflammation, IFNλ signaling in mouse NK cells was shown to induce IFNγ production, which promoted inflammation. Although enhanced activation of immune cells may promote bacterial clearance, the dangers of prolonged inflammation and tissue destruction must be considered. As such, studying the role of IFNλ in the resolution of inflammatory responses to infection is essential to uncover whether it causes any detrimental effects.
3 Concluding Remarks
IFNλs are crucial mediators of inflammation. They are produced by a variety of cell types in response to both commensal and pathogenic microorganisms. Although the IFNLR receptor is found on a restricted subset of cells, the localisation of these cells at barrier sites makes them optimally suited as gatekeepers of immune responses (summarized in Figure 1). Homeostatic IFNλ production in response to the microbiota promotes a resting antiviral state and strengthens epithelial barrier integrity in the intestines and the lungs. IFNλ has diverse effects in health and disease, with the outcome depending on the biological context. In the intestine, IFNλ appears to be protective during bacterial infection, inhibiting the invasion of enteric pathogens in vitro. In contrast, in the respiratory tract, IFNλ production during viral infection promotes inflammation and can lead to bacterial superinfections. In addition to the lungs and intestinal tract, mucosal epithelial barriers are also present in the genitourinary tract. IFNλ is produced and protective against viral infection in the vaginal and cervical epithelium (106–108). Whether type III IFNs are protective against genitourinary bacterial infections warrants further investigation.
IFNλ also has inverse effects on different immune cells. While it dampens neutrophil recruitment and phagocytosis, it enhances bacterial uptake and killing by macrophages. As they are intrinsically involved in innate and adaptive immune responses, it is important that IFNλs are not studied in isolation. Although IFNλ and the other IFN family members are functionally distinct, they exert antagonistic or synergistic influences on each other (109–111). For example, epithelial cells lacking type III IFN signaling were shown to be more responsive to type I IFN. Conversely, depletion of type I IFN signaling negatively regulates the sensitivity of cells to IFNλ (109). Finally, defining the bidirectional relationship that occurs between the gut microbiota and the lung IFN response will be key to our understanding of anti-bacterial immunity at barrier sites. This review illustrates the need for further research into the functions of IFNλ at barrier sites in the context of bacterial infection and mandates further exploration into this field.
Author Contributions
All authors contributed to the article and figure and approved the submitted version.
Funding
NA is supported by a studentship from the King’s College London/Francis Crick Institute partnership which receives its core funding from Cancer Research UK (FC001206), the UK Medical Research Council (FC001206) and the Wellcome Trust (FC001206). RD is supported by a studentship from the UK Medical Research Council (MR/N013700/1). AA is supported by a studentship from the Ministry of Higher Education in the Kingdom of Saudi Arabia. CO is supported by a Sir Henry Dale Fellowship from the Royal Society and the Wellcome Trust (206200/Z/17/Z). For the purpose of open access, the author has applied a CC BY public copyright licence to any Author Accepted Manuscript version arising from this submission.
Conflict of Interest
The authors declare that the research was conducted in the absence of any commercial or financial relationships that could be construed as a potential conflict of interest.
Publisher’s Note
All claims expressed in this article are solely those of the authors and do not necessarily represent those of their affiliated organizations, or those of the publisher, the editors and the reviewers. Any product that may be evaluated in this article, or claim that may be made by its manufacturer, is not guaranteed or endorsed by the publisher.
References
1. Pott J, Mahlakõiv T, Mordstein M, Duerr CU, Michiels T, Stockinger S, et al. IFN-λ Determines the Intestinal Epithelial Antiviral Host Defense. Proc Natl Acad Sci USA (2011) 108:7944–9. doi: 10.1073/pnas.1100552108
2. Boxx GM, Cheng G. The Roles of Type I Interferon in Bacterial Infection. Cell Host Microbe (2016) 19:760–9. doi: 10.1016/j.chom.2016.05.016
3. Sheppard P, Kindsvogel W, Xu W, Henderson K, Schlutsmeyer S, Whitmore TE, et al. IL-28, IL-29 and Their Class II Cytokine Receptor IL-28r. Nat Immunol (2002) 4:63–8. doi: 10.1038/ni873
4. Kotenko SV, Gallagher G, Baurin VV, Lewis-Antes A, Shen M, Shah NK, et al. IFN-λs Mediate Antiviral Protection Through a Distinct Class II Cytokine Receptor Complex. Nat Immunol (2003) 4:69–77. doi: 10.1038/ni875
5. Lasfar A, Lewis-Antes A, Smirnov SV, Anantha S, Abushahba W, Tian B, et al. Characterization of the Mouse IFN-λ Ligand-Receptor System: IFN-λs Exhibit Antitumor Activity Against B16 Melanoma. Cancer Res (2006) 66:4468–77. doi: 10.1158/0008-5472.CAN-05-3653
6. Donnelly RP, Kotenko SV. Interferon-Lambda: A New Addition to an Old Family. J Interf Cytokine Res (2010) 30:555–64. doi: 10.1089/jir.2010.0078
7. Durbin RK, Kotenko SV, Durbin JE. Interferon Induction and Function at the Mucosal Surface. Immunol Rev (2013) 255:25–39. doi: 10.1111/imr.12101
8. Wolk K, Witte K, Witte E, Proesch S, Schulze-Tanzil G, Nasilowska K, et al. Maturing Dendritic Cells Are an Important Source of IL-29 and IL-20 That May Cooperatively Increase the Innate Immunity of Keratinocytes. J Leukoc Biol (2008) 83:1181–93. doi: 10.1189/jlb.0807525
9. Lauterbach H, Bathke B, Gilles S, Traidl-Hoffmann C, Luber CA, Fejer G, et al. Mouse Cd8α+ DCs and Human BDCA3+ DCs Are Major Producers of IFN-λ in Response to Poly IC. J Exp Med (2010) 207:2703–17. doi: 10.1084/jem.20092720
10. Yin Z, Dai J, Deng J, Sheikh F, Natalia M, Shih T, et al. Type III IFNs Are Produced by and Stimulate Human Plasmacytoid Dendritic Cells. J Immunol (2012) 189:2735–45. doi: 10.4049/jimmunol.1102038
11. Zhang S, Kodys K, Li K, Szabo G. Human Type 2 Myeloid Dendritic Cells Produce Interferon-λ and Amplify Interferon-α in Response to Hepatitis C Virus Infection. Gastroenterology (2013) 144:414–25. doi: 10.1053/j.gastro.2012.10.034
12. Levy DE, García-Sastre A. The Virus Battles: IFN Induction of the Antiviral State and Mechanisms of Viral Evasion. Cytokine Growth Factor Rev (2001) 12:143–56. doi: 10.1016/S1359-6101(00)00027-7
13. Bierne H, Travier L, Mahlakõiv T, Tailleux L, Subtil A, Lebreton A, et al. Activation of Type III Interferon Genes by Pathogenic Bacteria in Infected Epithelial Cells and Mouse Placenta. PloS One (2012) 7:1–11. doi: 10.1371/journal.pone.0039080
14. Taylor KE, Mossman KL. Recent Advances in Understanding Viral Evasion of Type I Interferon. Immunology (2013) 138:190–7. doi: 10.1111/imm.12038
15. Rauch I, Rosebrock F, Hainzl E, Heider S, Majoros A, Wienerroither S, et al. Noncanonical Effects of IRF9 in Intestinal Inflammation: More Than Type I and Type III Interferons. Mol Cell Biol (2015) 35:2332–43. doi: 10.1128/mcb.01498-14
16. Odendall C, Voak AA, Kagan JC. Type III Interferons Are Commonly Induced by Bacteria-Sensing TLRs, and Reinforce Epithelial Barriers During Infection. J Immunol (2017) 176:139–48. doi: 10.4049/jimmunol.1700250
17. Rojas JM, Alejo A, Martín V, Sevilla N. Viral Pathogen-Induced Mechanisms to Antagonize Mammalian Interferon (IFN) Signaling Pathway. Cell Mol Life Sci (2021) 78:1423–44. doi: 10.1007/s00018-020-03671-z
18. Yoon S, Jones BC, Logsdon NJ, Harris BD, Deshpande A, Radaeva S, et al. Structure and Mechanism of Receptor Sharing by the IL-10r2 Common Chain. Structure (2010) 18:638–48. doi: 10.1016/j.str.2010.02.009
19. Zhou Z, Hamming OJ, Ank N, Paludan SR, Nielsen AL, Hartmann R. Type III Interferon (IFN) Induces a Type I IFN-Like Response in a Restricted Subset of Cells Through Signaling Pathways Involving Both the Jak-STAT Pathway and the Mitogen-Activated Protein Kinases. J Virol (2007) 81:7749–58. doi: 10.1128/jvi.02438-06
20. Sommereyns C, Paul S, Staeheli P, Michiels T. IFN-Lambda (IFN-λ) Is Expressed in a Tissue-Dependent Fashion and Primarily Acts on Epithelial Cells In Vivo. PloS Pathog (2008) 4:1–12. doi: 10.1371/journal.ppat.1000017
21. Mordstein M, Neugebauer E, Ditt V, Jessen B, Rieger T, Falcone V, et al. Lambda Interferon Renders Epithelial Cells of the Respiratory and Gastrointestinal Tracts Resistant to Viral Infections. J Virol (2010) 84:5670–7. doi: 10.1128/jvi.00272-10
22. Broggi A, Tan Y, Granucci F, Zanoni I. IFN-λ Suppresses Intestinal Inflammation by Non-Translational Regulation of Neutrophil Function. Nat Immunol (2017) 18:1084–93. doi: 10.1038/ni.3821
23. Read SA, Wijaya R, Ramezani-Moghadam M, Tay E, Schibeci S, Liddle C, et al. Macrophage Coordination of the Interferon Lambda Immune Response. Front Immunol (2019) 10:2674. doi: 10.3389/fimmu.2019.02674
24. Koltsida O, Hausding M, Stavropoulos A, Koch S, Tzelepis G, Übel C, et al. IL-28a (IFN-λ2) Modulates Lung DC Function to Promote Th1 Immune Skewing and Suppress Allergic Airway Disease. EMBO Mol Med (2011) 3:348–61. doi: 10.1002/emmm.201100142
25. Blazek K, Eames HL, Weiss M, Byrne AJ, Perocheau D, Pease JE, et al. IFN-λ Resolves Inflammation via Suppression of Neutrophil Infiltration and IL-1β Production. J Exp Med (2015) 212:845–53. doi: 10.1084/jem.20140995
26. Santer DM, Minty GES, Golec DP, Lu J, May J, Namdar A, et al. Differential Expression of Interferon-Lambda Receptor 1 Splice Variants Determines the Magnitude of the Antiviral Response Induced by Interferon-Lambda 3 in Human Immune Cells. PloS Pathog (2020) 16:1–26. doi: 10.1371/journal.ppat.1008515
27. Morrison MH, Keane C, Quinn LM, Kelly A, O’Farrelly C, Bergin C, et al. IFNL Cytokines do Not Modulate Human or Murine NK Cell Functions. Hum Immunol (2014) 75:996–1000. doi: 10.1016/j.humimm.2014.06.016
28. Souza-Fonseca-Guimaraes F, Young A, Mittal D, Martinet L, Bruedigam C, Takeda K, et al. NK Cells Require IL-28R for Optimal In Vivo Activity. Proc Natl Acad Sci USA (2015) 112:E2376–84. doi: 10.1073/pnas.1424241112
29. Pervolaraki K, Rastgou Talemi S, Albrecht D, Bormann F, Bamford C, Mendoza JL, et al. Differential Induction of Interferon Stimulated Genes Between Type I and Type III Interferons Is Independent of Interferon Receptor Abundance. PloS Pathog (2018) 14:1–31. doi: 10.1371/journal.ppat.1007420
30. Ramos HC, Rumbo M, Sirard JC. Bacterial Flagellins: Mediators of Pathogenicity and Host Immune Responses in Mucosa. Trends Microbiol (2004) 12:509–17. doi: 10.1016/j.tim.2004.09.002
31. Dziarski R. Peptidoglycan Recognition Proteins (PGRPs). Mol Immunol (2004) 40:877–86. doi: 10.1016/j.molimm.2003.10.011
32. Bertani B, Ruiz N. Function and Biogenesis of Lipopolysaccharides. EcoSal Plus (2018) 8:1–25. doi: 10.1128/ecosalplus.ESP-0001-2018.Function
33. Pietilä TE, Latvala S, Österlund P, Julkunen I. Inhibition of Dynamin-Dependent Endocytosis Interferes With Type III IFN Expression in Bacteria-Infected Human Monocyte-Derived DCs. J Leukoc Biol (2010) 88:665–74. doi: 10.1189/jlb.1009651
34. Lebreton A, Lakisic G, Job V, Fritsch L, Tham TN, Camejo A, et al. A Bacterial Protein Targets the BAHD1 Chromatin Complex to Stimulate Type III Interferon Response. Science (2011) 331:1319–21. doi: 10.1126/science.1200120
35. Odendall C, Dixit E, Stavru F, Bierne H, Franz KM, Durbin AF, et al. Diverse Intracellular Pathogens Activate Type III Interferon Expression From Peroxisomes. Nat Immunol (2014) 15:717–26. doi: 10.1038/ni.2915
36. Peignier A, Planet PJ, Parker D. Differential Induction of Type I and III Interferons by. Infect Immun (2020) 88:1–14. doi: 10.1128/IAI.00352-20
37. Savitsky D, Tamura T, Yanai H, Taniguchi T. Regulation of Immunity and Oncogenesis by the IRF Transcription Factor Family. Cancer Immunol Immunother (2010) 59:489–510. doi: 10.1007/s00262-009-0804-6
38. Burdette DL, Monroe KM, Sotelo-Troha K, Iwig JS, Eckert B, Hyodo M, et al. STING Is a Direct Innate Immune Sensor of Cyclic Di-GMP. Nature (2011) 478:515–8. doi: 10.1038/nature10429
39. Davies BW, Bogard RW, Young TS, Mekalanos JJ. Coordinated Regulation of Accessory Genetic Elements Produces Cyclic Di-Nucleotides for V. Cholerae Virulence. Cell (2012) 149:358–70. doi: 10.1016/j.cell.2012.01.053
40. Gao D, Wu J, Wu Y, Du F, Aroh C, Yan N, et al. Cyclic GMP-AMP Synthase Is an Innate Immune Sensor of HIV and Other Retroviruses. Science (2013) 341:903–7. doi: 10.1126/science.1240933
41. Li X-D, Wu J, Gao D, Wang H, Sun L, Chen ZJ. Pivotal Roles of cGAS-cGAMP Signaling in Antiviral Defense and Immune Adjuvant Effects. Science (2013) 341:1–10. doi: 10.1126/science.1244040
42. Cohen D, Melamed S, Millman A, Shulman G, Oppenheimer-Shaanan Y, Kacen A, et al. Cyclic GMP–AMP Signalling Protects Bacteria Against Viral Infection. Nature (2019) 574:691–5. doi: 10.1038/s41586-019-1605-5
43. Morehouse BR, Govande AA, Millman A, Keszei AFA, Lowey B, Ofir G, et al. STING Cyclic Dinucleotide Sensing Originated in Bacteria. Nature (2020) 586:429–33. doi: 10.1038/s41586-020-2719-5
44. Ishikawa H, Barber GN. STING Is an Endoplasmic Reticulum Adaptor That Facilitates Innate Immune Signalling. Nature (2008) 455:674–8. doi: 10.1038/nature07317
45. Honda K, Yanai H, Negishi H, Asagiri M, Sato M, Mizutani T, et al. IRF-7 Is the Master Regulator of Type-I Interferon-Dependent Immune Responses. Nature (2005) 434:772–7. doi: 10.1038/nature03419.1
46. Love AC, Schwartz I, Petzke MM. Borrelia Burgdorferi RNA Induces Type I and III Interferons via Toll-Like Receptor 7 and Contributes to Production of NF-κb-Dependent Cytokines. Infect Immun (2014) 82:2405–16. doi: 10.1128/IAI.01617-14
47. Jiang Z, Georgel P, Du X, Shamel L, Sovath S, Mudd S, et al. CD14 Is Required for MyD88-Independent LPS Signaling. Nat Immunol (2005) 6:565–70. doi: 10.1038/ni1207
48. Broad A, Kirby JA, Jones DEJ. Toll-Like Receptor Interactions: Tolerance of MyD88-Dependent Cytokines But Enhancement of MyD88-Independent Interferon-β Production. Immunology (2007) 120:103–11. doi: 10.1111/j.1365-2567.2006.02485.x
49. Zanoni I, Tan Y, Gioia M, Broggi A, Ruan J, Shi J, et al. An Endogenous Caspase-11 Ligand Elicits Interleukin-1 Release From Living Dendritic Cells. Science (2016) 352:1232–6. doi: 10.1126/science.aaf3036
50. Barbalat R, Lau L, Locksley RM, Barton GM. Toll-Like Receptor 2 on Inflammatory Monocytes Induces Type I Interferon in Response to Viral But Not Bacterial Ligands. Nat Immunol (2009) 10:1200–7. doi: 10.1038/ni.1792
51. Kawasaki T, Kawai T. Toll-Like Receptor Signaling Pathways. Front Immunol (2014) 5:461. doi: 10.3389/fimmu.2014.00461
52. Stack J, Doyle SL, Connolly DJ, Reinert LS, O’Keeffe KM, McLoughlin RM, et al. TRAM Is Required for TLR2 Endosomal Signaling to Type I IFN Induction. J Immunol (2014) 193:6090–102. doi: 10.4049/jimmunol.1401605
53. Lee S-J, Kim W-J, Moon S-K. Role of the P38 MAPK Signaling Pathway in Mediating Interleukin-28A-Induced Migration of UMUC-3 Cells. Int J Mol Med (2012) 30:945–52. doi: 10.3892/ijmm.2012.1064
54. Karaghiosoff M, Neubauer H, Lassnig C, Kovarik P, Schindler H, Pircher H, et al. Partial Impairment of Cytokine Responses in Tyk2-Deficient Mice. Immunity (2000) 13:549–60. doi: 10.1016/S1074-7613(00)00054-6
55. Kreins AY, Ciancanelli MJ, Okada S, Kong XF, Ramírez-Alejo N, Kilic SS, et al. Human TYK2 Deficiency: Mycobacterial and Viral Infections Without Hyper-IgE Syndrome. J Exp Med (2015) 212:1641–62. doi: 10.1084/jem.20140280
56. Schnepf D, Crotta S, Thamamongood T, Stanifer M, Polcik L, Ohnemus A, et al. Selective Janus Kinase Inhibition Preserves Interferon-{Lambda}-Mediated Antiviral Responses. Sci Immunol (2021) 6:eabd5318. doi: 10.1126/sciimmunol.abd5318
57. Marcello T, Grakoui A, Barba-Spaeth G, Machlin ES, Kotenko SV, Macdonald MR, et al. Interferons α and λ Inhibit Hepatitis C Virus Replication With Distinct Signal Transduction and Gene Regulation Kinetics. Gastroenterology (2006) 131:1887–98. doi: 10.1053/j.gastro.2006.09.052
58. Bolen CR, Ding S, Robek MD, Kleinstein SH. Dynamic Expression Profiling of Type I and Type III Interferon-Stimulated Hepatocytes Reveals a Stable Hierarchy of Gene Expression. Hepatology (2014) 59:1262–72. doi: 10.1002/hep.26657
59. Jilg N, Lin W, Hong J, Schaefer EA, Wolski D, Meixong J, et al. Kinetic Differences in the Induction of Interferon Stimulated Genes by Interferon-α and Interleukin 28B Are Altered by Infection With Hepatitis C Virus. Hepatology (2014) 59:1250–61. doi: 10.1002/hep.26653
60. Bhushal S, Wolfsmüller M, Selvakumar TA, Kemper L, Wirth D, Hornef MW, et al. Cell Polarization and Epigenetic Status Shape the Heterogeneous Response to Type III Interferons in Intestinal Epithelial Cells. Front Immunol (2017) 8:671. doi: 10.3389/fimmu.2017.00671
61. Pervolaraki K, Stanifer ML, Münchau S, Renn LA, Albrecht D, Kurzhals S, et al. Type I and Type III Interferons Display Different Dependency on Mitogen-Activated Protein Kinases to Mount an Antiviral State in the Human Gut. Front Immunol (2017) 8:459. doi: 10.3389/fimmu.2017.00459
62. Schoggins JW. Interferon-Stimulated Genes: Roles in Viral Pathogenesis. Curr Opin Virol (2014) 6:40–6. doi: 10.1016/j.coviro.2014.03.006
63. Zhou JH, Wang YN, Chang QY, Ma P, Hu Y, Cao X. Type III Interferons in Viral Infection and Antiviral Immunity. Cell Physiol Biochem (2018) 51:173–85. doi: 10.1159/000495172
64. Ranjbar S, Haridas V, Jasenosky LD, Falvo JV, Goldfeld AE. A Role for IFITM Proteins in Restriction of Mycobacterium Tuberculosis Infection. Cell Rep (2015) 13:874–83. doi: 10.1016/j.celrep.2015.09.048
65. Helbig KJ, Teh MY, Crosse KM, Monson EA, Smith M, Tran EN, et al. The Interferon Stimulated Gene Viperin, Restricts Shigella. Flexneri In Vitro. Sci Rep (2019) 9:1–12. doi: 10.1038/s41598-019-52130-8
66. Santos JC, Dick MS, Lagrange B, Degrandi D, Pfeffer K, Yamamoto M, et al. LPS Targets Host Guanylate-Binding Proteins to the Bacterial Outer Membrane for Non-Canonical Inflammasome Activation. EMBO J (2018) 37:1–19. doi: 10.15252/embj.201798089
67. Santos JC, Boucher D, Schneider LK, Demarco B, Dilucca M, Shkarina K, et al. Human GBP1 Binds LPS to Initiate Assembly of a Caspase-4 Activating Platform on Cytosolic Bacteria. Nat Commun (2020) 11:1–15. doi: 10.1038/s41467-020-16889-z
68. Kutsch M, Coers J, Sistemich L, Lesser CF, Goldberg MB, Herrmann C. Direct Binding of Polymeric GBP1 to LPS Disrupts Bacterial Cell Envelope Functions. Embo (2020) 39:1–22. doi: 10.15252/embj.2020104926
69. Wandel MP, Kim B, Park E, Boyle KB, Nayak K, Lagrange B, et al. Guanylate-Binding Proteins Convert Cytosolic Bacteria Into Caspase-4 Signaling Platforms. Nat Immunol (2020) 21:1–13. doi: 10.1038/s41590-020-0697-2
70. Piro AS, Hernandez D, Luoma S, Feeley EM, Finethy R, Yirga A, et al. Detection of Cytosolic Shigella flexneri via a C-Terminal Triple-Arginine Motif of GBP1 Inhibits Actin-Based Motility. mBio (2017). 8:1–16. doi: 10.1128/mBio.01979-17
71. Wandel MP, Pathe C, Werner EI, Ellison CJ, Boyle KB, von der Malsburg A, et al. GBPs Inhibit Motility of Shigella Flexneri But Are Targeted for Degradation by the Bacterial Ubiquitin Ligase Ipah9.8. Cell Host Microbe (2017) 22:507–18.e5. doi: 10.1016/j.chom.2017.09.007
72. Alphonse N, Dickenson RE, Odendall C. Interferons: Tug of War Between Bacteria and Their Host. Front Cell Infect Microbiol (2021) 11:624094. doi: 10.3389/fcimb.2021.624094
73. Li P, Jiang W, Yu Q, Liu W, Zhou P, Li J, et al. Ubiquitination and Degradation of GBPs by a Shigella Effector to Suppress Host Defence. Nature (2017) 551:378–83. doi: 10.1038/nature24467
74. Lazear HM, Daniels BP, Pinto AK, Huang AC, Vick SC, Doyle SE, et al. Interferon-λ Restricts West Nile Virus Neuroinvasion by Tightening the Blood-Brain Barrier. Sci Transl Med (2015) 7:1–14. doi: 10.1126/scitranslmed.aac6108
75. Li L, Zhou C, Li T, Xiao W, Yu M, Yang H. Interleukin-28A Maintains the Intestinal Epithelial Barrier Function Through Regulation of Claudin-1. Ann Transl Med (2021) 9:365–5. doi: 10.21037/atm-20-5494
76. Chiriac MT, Buchen B, Wandersee A, Hundorfean G, Günther C, Bourjau Y, et al. Activation of Epithelial Signal Transducer and Activator of Transcription 1 by Interleukin 28 Controls Mucosal Healing in Mice With Colitis and Is Increased in Mucosa of Patients With Inflammatory Bowel Disease. Gastroenterology (2017) 153:123–38.e8. doi: 10.1053/j.gastro.2017.03.015
77. Davidson S, McCabe TM, Crotta S, Gad HH, Hessel EM, Beinke S, et al. IFN λ Is a Potent Anti-Influenza Therapeutic Without the Inflammatory Side Effects of IFN α. EMBO Mol Med (2016) 8:1099–112. doi: 10.15252/emmm.201606413
78. Galani IE, Triantafyllia V, Eleminiadou EE, Koltsida O, Stavropoulos A, Manioudaki M, et al. Interferon-λ Mediates Non-Redundant Front-Line Antiviral Protection Against Influenza Virus Infection Without Compromising Host Fitness. Immunity (2017) 46:875–90.e6. doi: 10.1016/j.immuni.2017.04.025
79. Ahn D, Wickersham M, Riquelme S, Prince A. The Effects of IFN-λ on Epithelial Barrier Function Contribute to Klebsiella Pneumoniae ST258 Pneumonia. Am J Respir Cell Mol Biol (2018) 60:158–66. doi: 10.1165/rcmb.2018-0021OC
80. Ardanuy J, Scanlon K, Skerry C, Fuchs SY, Carbonetti NH. Age-Dependent Effects of Type I and Type III IFNs in the Pathogenesis of Bordetella Pertussis Infection and Disease. J Immunol (2020) 204:2192–202. doi: 10.4049/jimmunol.1900912
81. Broggi A, Ghosh S, Sposito B, Spreafico R, Balzarini F, Lo Cascio A, et al. Type III Interferons Disrupt the Lung Epithelial Barrier Upon Viral Recognition. Science (2020) 369:706–12. doi: 10.1126/science.abc3545
82. Major J, Crotta S, Llorian M, McCabe TM, Gad HH, Hartmann R, et al. Type I and III Interferons Disrupt Lung Epithelial Repair During Recovery From Viral Infection. Science (2020) 369:712–7. doi: 10.1126/science.abc2061
83. Planet PJ, Parker D, Cohen TS, Smith H, Leon JD, Ryan C, et al. Lambda Interferon Restructures the Nasal Microbiome and Increases Susceptibility to Staphylococcus Aureus Superinfection. mBio (2016) 7:1–12. doi: 10.1128/mBio.01939-15
84. Krammer F, Smith GJD, Fouchier RAM, Peiris M, Kedzierska K, Doherty PC, et al. Influenza. Nat Rev Dis Prim (2018) 4:1–21. doi: 10.1038/s41572-018-0002-y
85. Rich HE, McCourt CC, Zheng WQ, McHugh KJ, Robinson KM, Wang J, et al. Interferon Lambda Inhibits Bacterial Uptake During Influenza Superinfection. Infect Immun (2019) 87:1–12. doi: 10.1128/IAI.00114-19
86. Krajmalnik-Brown R, Ilhan ZE, Kang DW, DiBaise JK. Effects of Gut Microbes on Nutrient Absorption and Energy Regulation. Nutr Clin Pract (2012) 27:201–14. doi: 10.1177/0884533611436116
87. Stockinger S, Duerr CU, Fulde M, Dolowschiak T, Pott J, Yang I, et al. TRIF Signaling Drives Homeostatic Intestinal Epithelial Antimicrobial Peptide Expression. J Immunol (2014) 193:4223–34. doi: 10.4049/jimmunol.1302708
88. Baldridge MT, Nice TJ, McCune BT, Yokoyama CC, Kambal A, Wheadon M, et al. Commensal Microbes and Interferon-λ Determine Persistence of Enteric Murine Norovirus Infection. Science (2015) 347:266–9. doi: 10.1126/science.1258025
89. Baldridge MT, Lee S, Brown JJ, McAllister N, Urbanek K, Dermody TS, et al. Expression of Ifnlr1 on Intestinal Epithelial Cells Is Critical to the Antiviral Effects of Interferon Lambda Against Norovirus and Reovirus. J Virol (2017) 91:1–14. doi: 10.1128/jvi.02079-16
90. Van Winkle JA, Peterson ST, Kennedy EA, Wheadon MJ, Ingle H, Desai C, et al. A Homeostatic Interferon-Lambda Response to Bacterial Microbiota Stimulates Preemptive Antiviral Defense Within Discrete Pockets of Intestinal Epithelium. Elife (2021) 11:1–30. doi: 10.1101/2021.06.02.446828
91. Hilty M, Burke C, Pedro H, Cardenas P, Bush A, Bossley C, et al. Disordered Microbial Communities in Asthmatic Airways. PloS One (2010) 5:1–9. doi: 10.1371/journal.pone.0008578
92. Huang YJ, Nelson CE, Brodie EL, Desantis TZ, Baek MS, Liu J, et al. Airway Microbiota and Bronchial Hyperresponsiveness in Patients With Sub-Optimally Controlled Asthma. J Allergy Clin Immunol (2011) 127:372–81. doi: 10.1016/j.jaci.2010.10.048
93. Dickson RP, Erb-Downward JR, Freeman CM, Mccloskey L, Falkowski NR, Huffnagle GB, et al. Bacterial Topography of the Healthy Human Lower Respiratory Tract. MBio (2017) 8:1–12. doi: 10.1128/mBio.02287-16
94. Bradley KC, Finsterbusch K, Schnepf D, Crotta S, Llorian M, Davidson S, et al. Microbiota-Driven Tonic Interferon Signals in Lung Stromal Cells Protect From Influenza Virus Infection. Cell Rep (2019) 28:245–56. doi: 10.1016/j.celrep.2019.05.105
95. Das S, Bernasconi E, Koutsokera A, Wurlod DA, Tripathi V, Bonilla-Rosso G, et al. A Prevalent and Culturable Microbiota Links Ecological Balance to Clinical Stability of the Human Lung After Transplantation. Nat Commun (2021) 12:1–17. doi: 10.1038/s41467-021-22344-4
96. Kim HJ, Jo A, Jeon YJ, An S, Lee KM, Yoon SS, et al. Nasal Commensal Staphylococcus Epidermidis Enhances Interferon-λ-Dependent Immunity Against Influenza Virus. Microbiome (2019) 7:1–12. doi: 10.1186/s40168-019-0691-9
97. Espinosa V, Dutta O, McElrath C, Du P, Chang YJ, Cicciarelli B, et al. Type III Interferon Is a Critical Regulator of Innate Antifungal Immunity. Sci Immunol (2017) 2:1–12. doi: 10.1126/sciimmunol.aan5357
98. Broquet A, Besbes A, Martin J, Jacqueline C, Vourc’h M, Roquilly A, et al. Interleukin-22 Regulates Interferon Lambda Expression in a Mice Model of Pseudomonas Aeruginosa Pneumonia. Mol Immunol (2020) 118:52–9. doi: 10.1016/j.molimm.2019.12.003
99. Lan F, Zhong H, Zhang N, Johnston SL, Wen W, Papadopoulos N, et al. Interferon-Lambda1 Enhances Staphylococcus Aureus Clearance in Healthy Nasal Mucosa, Not in Nasal Polyps. J Allergy Clin Immunol (2019) 143:1416–25. doi: 10.1016/j.jaci.2018.09.041
100. Finotti G, Tamassia N, Calzetti F, Fattovich G, Cassatella MA. Endogenously Produced TNF-α Contributes to the Expression of CXCL10/IP-10 in IFN-λ3-Activated Plasmacytoid Dendritic Cells. J Leukoc Biol (2016) 99:107–19. doi: 10.1189/jlb.3vma0415-144r
101. de Groen RA, Groothuismink ZMA, Liu B-S, Boonstra A. IFN- Is Able to Augment TLR-Mediated Activation and Subsequent Function of Primary Human B Cells. J Leukoc Biol (2015) 98:623–30. doi: 10.1189/jlb.3a0215-041rr
102. Syedbasha M, Bonfiglio F, Linnik J, Stuehler C, Wüthrich D, Egli A. Interferon-λ Enhances the Differentiation of Naive B Cells Into Plasmablasts via the Mtorc1 Pathway. Cell Rep (2020) 33:1–12. doi: 10.1016/j.celrep.2020.108211
103. Jordan WJ, Eskdale J, Srinivas S, Pekarek V, Kelner D, Rodia M, et al. Human Interferon Lambda-1 (IFN-λ1/IL-29) Modulates the Th1/Th2 Response. Genes Immun (2007) 8:254–61. doi: 10.1038/sj.gene.6364382
104. Siebler J, Wirtz S, Weigmann B, Atreya I, Schmitt E, Kreft A, et al. IL-28a Is a Key Regulator of T-Cell-Mediated Liver Injury via the T-Box Transcription Factor T-Bet. Gastroenterology (2007) 132:358–71. doi: 10.1053/j.gastro.2006.10.028
105. Gallagher G, Megjugorac NJ, Yu RY, Eskdale J, Gallagher GE, Siegel R, et al. The Lambda Interferons: Guardians of the Immune-Epithelial Interface and the T-Helper 2 Response. J Interf Cytokine Res (2010) 30:603–15. doi: 10.1089/jir.2010.0081
106. Iversen MB, Ank N, Melchjorsen J, Paludan SR. Expression of Type III Interferon (IFN) in the Vaginal Mucosa Is Mediated Primarily by Dendritic Cells and Displays Stronger Dependence on NF-κb Than Type I IFNs. J Virol (2010) 84:4579–86. doi: 10.1128/jvi.02591-09
107. Xu XQ, Guo L, Wang X, Liu Y, Liu H, Zhou RH, et al. Human Cervical Epithelial Cells Release Antiviral Factors and Inhibit HIV Replication in Macrophages. J Innate Immun (2018) 11:29–40. doi: 10.1159/000490586
108. Caine EA, Scheaffer SM, Arora N, Zaitsev K, Artyomov MN, Coyne CB, et al. Interferon Lambda Protects the Female Reproductive Tract Against Zika Virus Infection. Nat Commun (2019) 10:1–12. doi: 10.1038/s41467-018-07993-2
109. Pervolaraki K, Guo C, Albrecht D, Boulant S, Stanifer ML. Type-Specific Crosstalk Modulates Interferon Signaling in Intestinal Epithelial Cells. J Interf Cytokine Res (2019) 39:650–60. doi: 10.1089/jir.2019.0040
110. Perez J. Non-Canonical Roles of Oligoadenylate Synthetase 1 During Viral and Bacterial Infections. (2020).
Keywords: type III interferon, IFNλs, interferon signaling, epithelial barrier, bacterial infection, mucosal barrier
Citation: Alphonse N, Dickenson RE, Alrehaili A and Odendall C (2022) Functions of IFNλs in Anti-Bacterial Immunity at Mucosal Barriers. Front. Immunol. 13:857639. doi: 10.3389/fimmu.2022.857639
Received: 18 January 2022; Accepted: 07 April 2022;
Published: 18 May 2022.
Edited by:
Achille Broggi, U1104 Centre d’immunologie de Marseille-Luminy (CIML) (INSERM), FranceReviewed by:
Sreya Ghosh, Boston Children’s Hospital and Harvard Medical School, United StatesNikaïa Smith, Institut Pasteur, France
Copyright © 2022 Alphonse, Dickenson, Alrehaili and Odendall. This is an open-access article distributed under the terms of the Creative Commons Attribution License (CC BY). The use, distribution or reproduction in other forums is permitted, provided the original author(s) and the copyright owner(s) are credited and that the original publication in this journal is cited, in accordance with accepted academic practice. No use, distribution or reproduction is permitted which does not comply with these terms.
*Correspondence: Charlotte Odendall, Q2hhcmxvdHRlLm9kZW5kYWxsQGtjbC5hYy51aw==