- 1Nephrology and Kidney Transplantation Unit, “Maggiore della Carità” University Hospital, Department of Translational Medicine, Translational Research on Autoimmune and Allergic Disease (CAAD), University of Piemonte Orientale (UPO), Novara, Italy
- 2Department of Medical Sciences, University of Torino, Torino, Italy
- 3Nephrology, Dialysis and Transplantation Unit, Department of Emergency and Organ Transplantation, University of Bari, Bari, Italy
- 4Nephrology, Dialysis and Kidney Transplantation Unit, Fondazione IRCCS Ca’ Granda Ospedale Maggiore Policlinico, University of Milan, Milan, Italy
- 5Laboratory of Physiology, Department of Translational Medicine, Translational Research on Autoimmune and Allergic Disease (CAAD), University of Piemonte Orientale, Novara, Italy
- 6Department of Anesthesiology and Intensive Care, University of Torino, Torino, Italy
Acute kidney injury is a frequent complication of hospitalized patients and significantly increases morbidity and mortality, worsening costs and length of hospital stay. Despite this impact on healthcare system, treatment still remains only supportive (dialysis). Stem cell-derived extracellular vesicles are a promising option as they recapitulate stem cells properties, overcoming safety issues related to risks or rejection or aberrant differentiation. A growing body of evidence based on pre-clinical studies suggests that extracellular vesicles may be effective to treat acute kidney injury and to limit fibrosis through direct interference with pathogenic mechanisms of vascular and tubular epithelial cell damage. We herein analyze the state-of-the-art knowledge of therapeutic approaches with stem cell-derived extracellular vesicles for different forms of acute kidney injury (toxic, ischemic or septic) dissecting their cytoprotective, regenerative and immunomodulatory properties. We also analyze the potential impact of extracellular vesicles on the mechanisms of transition from acute kidney injury to chronic kidney disease, with a focus on the pivotal role of the inhibition of complement cascade in this setting. Despite some technical limits, nowadays the development of therapies based on stem cell-derived extracellular vesicles holds promise as a new frontier to limit acute kidney injury onset and progression.
Introduction: Definition and Classification of Acute Kidney Injury
The term Acute kidney injury (AKI) indicates a sudden worsening of renal function due to acute renal damage, with consequent accumulation of nitrogenous waste products and alteration of hydrosaline and acid-base homeostasis. In the past decades, several criteria have been proposed in order to uniform the definition of AKI: the recommendation statements of 2012 Kidney Disease Improving Global Outcomes (KDIGO) Clinical Practice Guideline define AKI as a rise in the serum creatinine (sCr) level by 0.3 mg/dl within 48 hours, or a 1.5-fold increase from baseline within prior 7 days; or oliguria (<0.5 ml/kg/h for 6 hours). In addition, AKI is staged for severity – from mild stage 1 to most severe stage 3 – according to serum creatinine values and urine output, usually classifying patients requiring renal replacement therapy (RRT) as stage 3 KDIGO. Several causes may induce AKI in patients with or without underlying chronic kidney disease (CKD); a potential classification is based on pathophysiological mechanisms of renal injury such as kidney hypoperfusion (pre-renal AKI), parenchymal kidney diseases (intra-renal or parenchymal AKI, which includes acute tubular necrosis, ATN) and obstruction of the urinary tract (post-renal AKI). Incomplete recovery of an AKI event due to persistence of renal pathophysiologic process can lead to AKI-CKD transition, especially in patient with some degree of pre-existent CKD (Figure 1). As for AKI epidemiology, incidence and prevalence are not well defined due to different AKI definitions. Despite these limitations, a metanalysis of Susantitaphong et al. (1) observed that in-hospital AKI incidence was 22% – using 2012 KDIGO AKI definition – while it reached 57% in intensive care units (ICU) according to the multinational AKI-EPI study (2). Overall AKI incidence seems to be rising in the United States and it is associated with higher health care costs, greater long-term care, increased risk of CKD and hospital mortality (3, 4). This increase especially affects Afro-American population, due to genetic factors which also condition a reduced number of nephrons. A large metanalysis (5) and recent studies (6) confirmed that Black race is an independent risk factor for AKI.
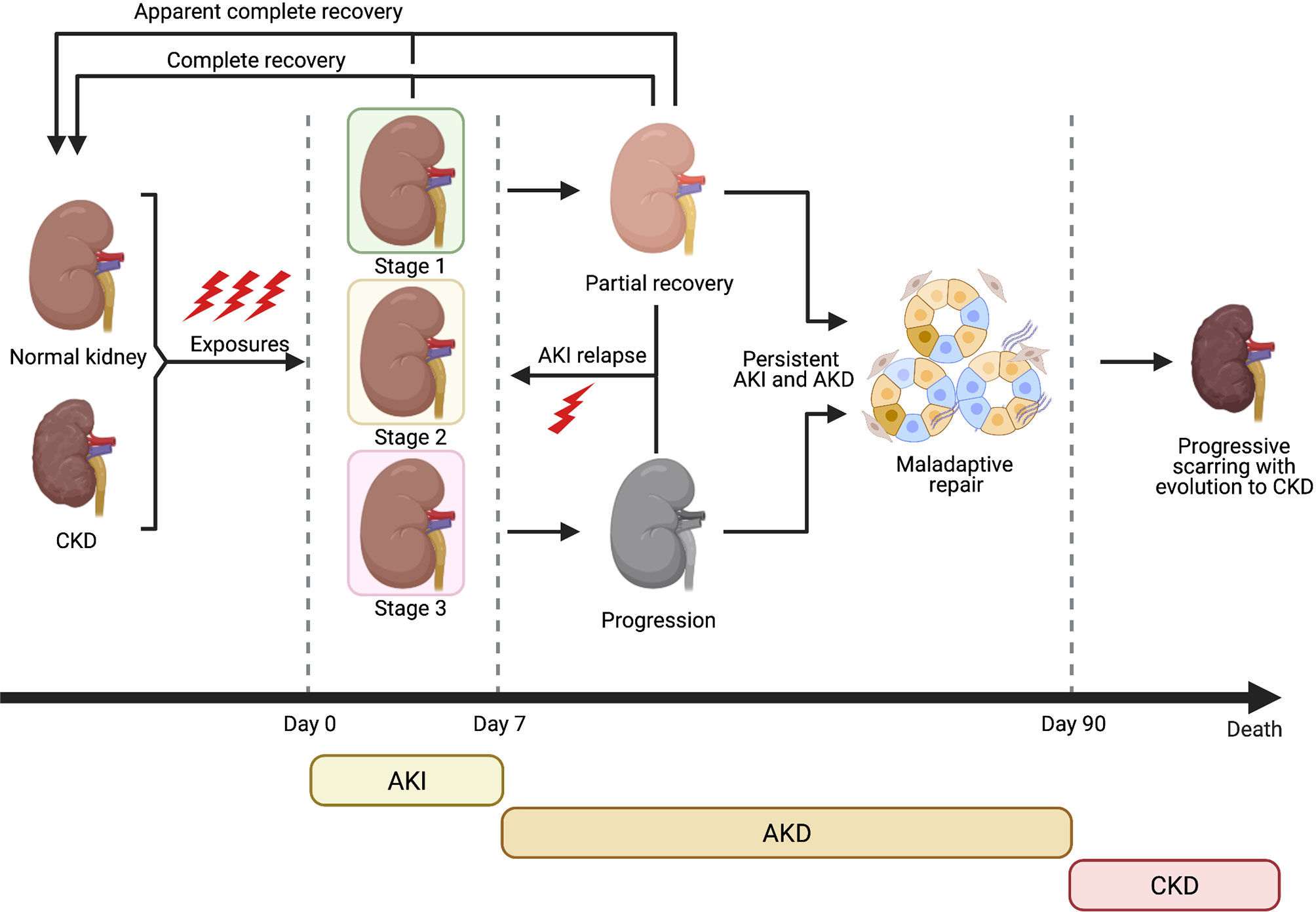
Figure 1 Clinical course of AKI and evolution towards CKD (Created with BioRender.com).
Similar to CKD, other factors associated to the rise of AKI incidence are older age, increasing burden of comorbidities (e.g. hypertension, diabetes mellitus, CKD, heart failure, sepsis, cancer), improved clinician’s awareness (leading to inclusion of less severe forms), growing use of nephrotoxic drugs and increasing frequency of surgical and angiographic procedures (7, 8). Among these, older age is strongly associated with AKI incidence through multifactorial mechanisms. Renal senescence reduces nephron number and functional reserve, predisposing to relapsing AKI episodes and also to maladaptive repair, incomplete recovery and AKI-CKD transition. This process can be considered as a form of accelerated renal senescence and will be analysed in a specific Section (9, 10).
Different causes related to ischemia-reperfusion injury due to hypovolemia and/or hypoperfusion, administration of nephrotoxic drugs and the presence of sepsis/septic shock have been identified as hallmarks for AKI development. Recent studies have also provided new insights into complex AKI pathophysiology, with remarkable progress especially in the field of sepsis-associated AKI (s-AKI).
Sepsis is a dysregulated immune response to infection that causes multiple organ dysfunction: the immune response after a septic insult is characterized by unbalanced hyperinflammation and immune suppression (11). Sepsis-associated excessive inflammation is sustained by several cell types including leukocytes (neutrophils, macrophages, natural killer cells), endothelial cells (EC), cytokines, complement products, and the coagulation system (12). The widely used concept of “cytokine storm” refers to the release of proinflammatory cytokines such as TNF-alpha, IL-1b, IL-12, and IL-18 that may contribute to organ injury (12). However, a complex network of several mediators is embedded in sepsis-associated multiple organ dysfunction such as the release of extracellular traps by neutrophils (NETs) (13), the complement activation resulting in the release of the anaphylatoxins C3a and C5a, the immunothrombosis in the microvasculature mainly triggered by tissue factor that initiates blood coagulation by forming a complex with clotting factor (F) VIIa, thereby inciting blood coagulation by activating FX and FIX (14). These alterations have been also recently described in COVID-19-associated AKI (15).
Sepsis represents nowadays the most frequent cause of AKI in critically ill patients admitted to Intensive Care Units (ICU) with pathogenic mechanisms similar to those described above for immune dysfunction. Indeed, experimental and clinical studies aimed to evaluate kidney perfusion clearly demonstrated that during sepsis, AKI can develop in the presence of a normal or even increased renal blood flow: this finding suggested the presence of other key mechanisms of tissue injury such as microvascular derangement, endothelial dysfunction, inflammation, metabolic reprogramming and sub-lethal injury of tubular epithelial cells, overthrowing the simplistic old paradigm based on kidney hypoperfusion (16, 17). Recent works have further shed light on these etiopathogenetic mechanisms: for example, interaction of damage-associated molecular pattern (DAMPs) and pathogen- associated molecular pattern (PAMPs) molecules with Toll-like Receptors (TLRs) expressed on renal tubular epithelial cells (RTEC) and other renal resident cells can activate several pro-inflammatory pathways (18) and mitochondrial DNA (mDNA) damage due to oxidative stress also plays a key role in inducing RTEC dysfunction (19). Basically, PAMPs and DAMPs can be freely filtered by glomeruli, a process favored by sepsis-induced increased permeability, thus reaching the tubular lumen and inducing functional alterations of RTECs; moreover, microvascular damage of ECs located in the peritubular capillaries may enhance metabolic alterations of RTECs (20).
Innate and adaptive immunity activation during AKI have been increasingly recognized to play an important role in AKI. Cross-talk between RTECs and immune cells through a network of cytokines seems to be essential to induce T cell phenotype changes which affect AKI evolution (21). In this setting an important role of IL-15 has been delineated. RTECs express IL 15 and its receptors and intrarenal IL 15 levels inversely correlate with AKI severity in experimental models. Inhibition of RTECs apoptosis during pathological stress appears to explain IL 15 protective effects (22) Furthermore, IL 15 can reduce extracellular matrix (ECM) synthesis by myofibroblasts and monocyte chemoattractant protein (MCP-1) release by macrophages and also inhibits Transforming Growth Factor-β (TGF-β) 1-induced RTEC epithelial-mesenchymal transition (EMT): these multiple anti-fibrotic effects suggest a role in the prevention of AKI-CKD transition (23, 24).
Despite progress in understanding AKI pathophysiology, however, efforts to develop targeted therapies has not led to robust results yet (16) and treatment is still mainly supportive (e.g. hemodynamic stabilization, dose adjustment or discontinuation of nephrotoxic drugs, antibiotic therapy in s-AKI) (25–28). Also in s-AKI, neither pharmacological approaches nor extracorporeal blood purification therapies aimed at removing PAMPs and DAMPs have led to a significant improvement of in-hospital AKI incidence and mortality (16).
In this setting, stem-cell (SC) therapy and SC-derived extracellular vesicles (EVs) represents a new frontier in the treatment of acute and chronic kidney disorders, because of their anti-inflammatory, immunomodulatory and regenerative properties. Recent studies observed the beneficial therapeutic properties of Mesenchymal Stromal Cells (MSCs) in ischemic AKI, renal transplantation, lupus nephritis and diabetic nephropathy (29, 30). These actions are mainly paracrine and mostly mediated by transfer of EVs containing microRNAs, mRNAs, and proteins that reprogram cell functions via immunomodulatory and regenerative effects (31), as detailed in the following section.
In this review, we summarize the state-of-the-art knowledge on EVs derived from different types of stem cells (SCs) as therapy of AKI, focusing on impact of EVs on different pathophysiological mechanisms underlying toxic, ischemic and s-AKI (29–31) and AKI-CKD transition.
General Features of Stem Cells and Extracellular Vesicles (EVs)
SCs are unspecialized cells with self-renewal capacity, which can potentially differentiate into any cell type of organism. Their therapeutic potential is revolutionizing regenerative medicine and is providing promising applications also in the field of Nephrology (32).
Among progenitor cells, MSCs represent the most studied type over the last decades: MSCs are adult multipotent stromal cells with a high proliferative potential, derived from non-hematopoietic precursors. They can differentiate into mesenchymal (osteocytes, adipocytes and chondroblasts) and non-mesenchymal lineages (33). Initially found in bone marrow (BM) (34), they were subsequently isolated from multiple fetal and adult tissues such as adipose tissue (AD-MSCs), umbilical cord blood (UC-MSCs), fetal membrane (FM-MSCs) and human placenta (hP-MSCs) (35–37). This makes them one of the most accessible SC type and an attractive candidate source to develop products for cell therapies (38). Due to the ease of preparation, MSCs remain the most common option among cellular therapies and have already proven to be safe and effective in reducing AKI in experimental models and clinical trials, displaying cytoprotective, regenerative and immunomodulatory properties (39, 40).
Other types of SCs have been recently investigated as a therapy for AKI, including inducible Pluripotent Stem Cells (iPSCs). These are derived from differentiated adult cells (e.g. keratinocytes, fibroblasts) which are induced into pluripotency by exposing them to specific reprogramming factors through viral vectors and directed towards renal lineages such as podocyte progenitors (41). Administration of iPSC has proved to be effective in a rat model of AKI, reducing oxidative stress and inflammation (42).
Spermatogonial stem cells (SCCs) have also been shown to be capable of differentiating into pluripotent stem cell lines, converting into embryonic-like SCs and differentiating into renal tubular-like cells. Also, this type of SC has shown promise in restoring kidney function after AKI (43).
Endothelial progenitor cells (EPCs), a BM-derived progenitor type able to circulate in the bloodstream, play a major role in vascular integrity by protecting ECs and promoting angiogenesis and recovery also in AKI models (44).
Of interest, the beneficial effects of all these SC types are predominantly mediated by paracrine/endocrine actions, via secretion of growth factors and especially EVs. The latter represent crucial components of cellular secretome and mediate intercellular communication through transfer of bioactive molecules between originator and recipient cells, especially mRNAs and microRNAs (miRNAs), modifying phenotype and function of target cells (45, 46). As their parental cells, EVs are not immunogenic and may successfully activate regenerative processes in injured cells and tissues (47, 48).
Recently, EVs released from renal cells themselves have been investigated as therapy for AKI: kidney-derived MSCs (49), glomerular and tubular renal progenitors (50), renal tubular epithelial cells (RTEC) (51) and even urinary EVs (uEVs) from healthy subjects have shown initial promising results in this setting (52). The efficacy of SC-derived EVs has been demonstrated in different settings including numerous studies showing an interesting cross-kingdom communication: EVs from different eukaryotic and prokaryotic kingdoms are involved in many processes including host-pathogen interactions and modulation of cellular functions (53). Of note, some studies showed that exogenous dietary RNAs of plant and animal origin are protected from food processing and gut microenvironment through encapsulation within EVs. EV-carried RNAs (in particular miRNAs) are able to exert biological activities between the host and gut microbiota influencing organ function in the recipient after ingestion (54). Our group has already demonstrated the horizontal transfer of mRNAs and miRNAs from SC-derived EVs of human origin in rat cells: indeed, in a rat model of anti-Thy1.1-induced mesangioproliferative glomerulonephritis, EVs released from human Endothelial Progenitor Cells (EPCs) horizontally transferred to rat mesangial cells distinct mRNAs coding for Factor H, CD55 and CD59, thus inhibiting complement-induced apoptosis and C5b-9/C3 mesangial cell deposition (55). Moreover, the same type of EVs protected the kidney from ischemia-reperfusion injury in rats by delivering their RNA content, the miRNA cargo of which was shown to contribute to reprogramming hypoxic renal endothelial and tubular epithelial cells to a regenerative program (56).
EV-based therapeutic approach has some advantages compared to cell-therapy. First, EVs exhibit a superior efficacy profile as they pass through the blood-tissue barriers and efficiently reach injured cells (57). Second, no adverse immune responses have been reported in patients undergoing allogeneic administration of SC-EVs and no evidence of oncogenic potential of SC-EVs has been reported. In fact, they can inhibit tumor growth by interfering with cell cycle and inducing apoptosis and/or necrosis of cancer cells (58, 59). Thus, EVs represent a feasible, cell-free therapeutic alternative and their role has recently been investigated in several renal diseases (45, 46), especially in AKI. This is the setting in which therapeutic properties of SC-EVs and the rationale for their employment has been better defined and represents the main focus of this Review.
Rationale of Therapy With EVs in AKI
A growing body of pre-clinical studies indicates a potential therapeutic role of EVs derived from MSCs and other progenitor cell types especially in IRI-induced AKI. EVs can shuttle miRNAs and other genetic material into injured renal cells – such as RTEC and ECs – and epigenetically re-programme them. This leads to activation of signaling pathways, which exerts multiple beneficial effects within three main areas (60, 61) (Figure 2).
● renal protection: inhibition of apoptosis/necrosis, oxidative stress, senescence and fibrogenesis; promotion of autophagy (62).
● renal regeneration: stimulation of cell proliferation, migration, tubular dedifferentiation, angiogenesis (63).
● immunomodulation: anti-inflammatory and immunosuppressive effects, mainly through induction of M2 macrophages and T-regulatory cells (Treg) (64) and modulation of NK cell activity (65).
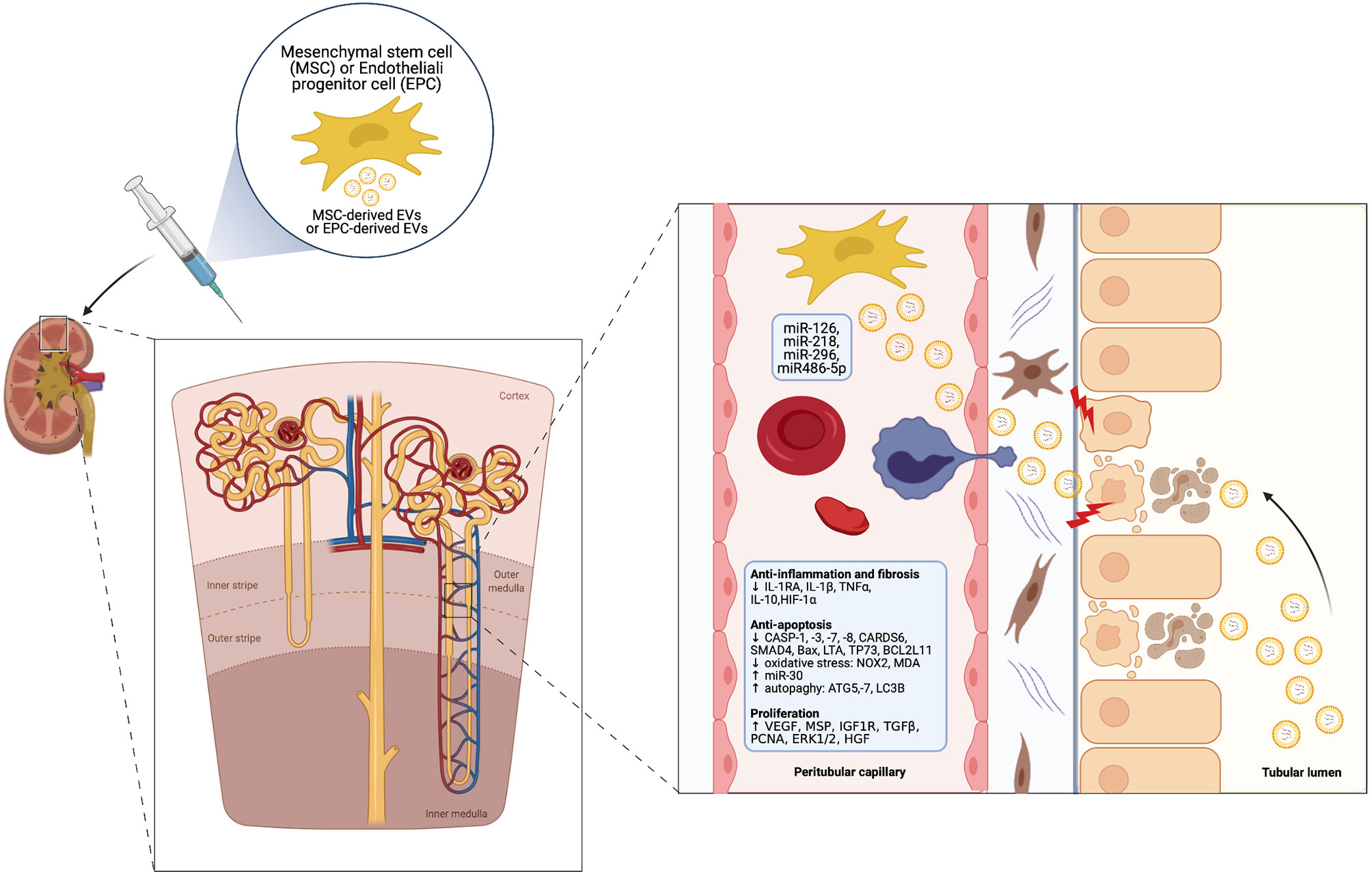
Figure 2 Cytoprotective, regenerative and immunomodulatory effects of MSC-derived EVs in the setting of AKI (Created with BioRender.com).
The combination of these effects can heal injured RTECs and ECs, thus promoting regression of AKI (66). Of note, pre-treatment with RNAase consistently abolished these effects, indicating that mRNA and miRNA transfer from SCs to injured renal cells is crucial in this healing process (67). Ferguson et al. identified 23 top-miRNAs which account for over 79% of total miRNAs in MSC-derived exosomes and seem to mediate the predominant effects, targeting 5481 genes (68). A comprehensive review of miRNA repertoire carried by SC-derived EVs for AKI treatment has been recently published and it is beyond the scope of this work (69).
Therapy With SC-Derived EVs in Different Forms of AKI
As previously mentioned, while most studies were performed with EVs derived from BM-MSCs, UC-MSCs and AD-MSC, other EV sources have been recently employed, including kidney resident populations (50, 70) and uEVs (71). Potential mechanisms of actions and main mediators, with a focus on miRNAs, will be briefly analyzed. A summary of the main studies on EVs as therapy of AKI is outlined in Table 1, in which they are categorised according to type of originating cell type (49–51, 56, 65–67, 72–96).
Nephrotoxic AKI
Several cellular sources of SCs have been tested in models of toxic AKI. Bruno et al. first demonstrated that BM-MSC-derived EVs facilitated morphological and functional renal recovery in a model of glycerol-induced AKI mimicking rhabdomyolysis-associated tubular damage (66). Human liver stem cells (HLSCs) cells were then tested in the same experimental model with similar results. A single intravenous injection of HLSCs facilitated histological and functional renal recovery through the induction of RTEC proliferation and inhibition of apoptosis. Of interest, EVs were the main component of HLSC-derived conditioned medium capable of promoting regeneration (72).
Furthermore, uEVs have been recently employed in the same mouse model of glycerol-induced toxic AKI. Intravenously injected uEVs stimulated RTEC proliferation, reduced expression of inflammatory markers and restored endogenous Klotho. Interestingly, murine uEVs derived from Klotho-null mice lost these reno-protective effects, suggesting a key-role of Klotho in mediating uEVs beneficial effects on RTECs (52).
EVs obtained from BM-MSCs were also tested in a lethal cisplatin-induced AKI model, in which they stimulated RTEC proliferation and conferred them resistance to apoptosis in vitro, resulting in improvement of renal function and morphology in an in vivo SCID mouse model (73). Similar results were reported employing UC-MSCs-derived EVs in the same experimental setting (74).
Last, BM-derived MSC repaired but did not prevent gentamycin-induced AKI (97).
Ischemic AKI
SC-derived EVs have also been studied in several models of ischemia/reperfusion injury (IRI)-associated AKI, as outlined in Table 1. In all of these experimental models, administration of EVs derived from different cell types after IRI accelerated recovery of renal function and/or decreased histological tubular injury through multiple mechanisms (61).
Gatti S et al. demonstrated a beneficial effect of BM-MSC-derived EVs in favouring recovery from ischemic AKI and the need for multiple injections to achieve renal function normalization. This effect was mainly due to upregulation of anti-apoptotic genes in injured RETCs and prevented transition from AKI to CKD (67).
Of interest is the fact that i.v. administered human MSC-derived EVs were effective in alleviating renal damage even in rats which had received kidney transplant after cardiac death, a setting characterized by severe IRI (93).
Another study highlighted the role of specific miRNAs within the cargo of MSC-derived EVs (miR-148b-3p, miR-410, miR-495, miR-548c-5p, miR-886-3p) in protecting RTECs from IRI in an in vitro model induced by ATP-depletion. Down-regulation of miRNAs involved in apoptosis, hypoxia and cytoskeletal reorganization mediated this effect (75).
Other studies brought out a wide spectrum of beneficial actions of BM-MSC-derived EVs in ischemic AKI: they can induce RTEC dedifferentiation and growth via hepatocyte growth factor (HGF) induction (76) and prevent apoptosis through transfer of miR-21 (98) and miR-223 (77); they inhibit CXC3CL1, blunting evolution towards fibrosis (90); finally, they are enriched in chemokine receptor type 2 (CCR-2), which enables them to buffer extracellular free chemokine ligand 2 (CCL-2), suppressing macrophage functions (91).
Of note, several EVs biological activities are specifically related to aerobic metabolism; for example, MSC-derived EVs can carry respiratory complexes, supporting an independent aerobic metabolism when mitochondrial respiratory capacity is impaired (99); they can inhibit mitochondrial fission through miR-30 transfer (83) and re-establish adequate intracellular ATP levels, with beneficial epigenetic changes such as reversion of histone H2 and H2B up-regulation, a typical feature of apoptotic cells (100); they can attenuate mitochondrial damage in RTECs by stabilising mitochondrial DNA (mDNA) previously depleted by oxidative stress (101).
Furthermore, they might contribute to the anti-oxidant potential of injured cells, for example down-regulating calnexin, a nicotinamide adenine dinucleotide phosphate (NADPH)-oxidase 4 (NOX4)-interacting protein (102).
Similarly, Zhang et al. reported that human Warton Jelly (hWG) MSC-derived EVs can reduce expression of NADPH-oxidase 2 (NOX2) and stimulate the nuclear factor eryhroid 2-related factor 2 (NRF-2) and anti-oxidant response element pathway (81, 82). All these actions result in an overall reduction of reactive oxygen species (ROS) formation and could limit tubular cell death and senescence after re-oxygenation.
EVs derived from iPSC (103) have also been shown to protect mitochondrial function and regulate several genes associated with oxidative stress. Interestingly, iPSC-EVs showed a higher efficiency in renal protection than AD-EVs (104, 105).
Another aspect which contributes to reparative effects of EVs is their capacity to promote angiogenesis, counteracting renal hypoxia. EPCs administration determined increased tubular proliferation and reduction in capillary rarefaction, glomerulosclerosis and tubulointerstitial fibrosis in a rat model of IRI-associated AKI, suggesting protection against post-IRI fibrosis. In this study, pro-angiogenic miR-126 and miR-296 shuttled by EPC-derived EVs to ECs located in peritubular and glomerular capillaries accounted for this effect, as treatment with RNAase or specific antagomiRs abolished it (56). Other studies subsequently proved that similar pro-angiogenetic effects were mediated by EVs released from other human SC types, such as kidney-derived MSCs (49, 50), endothelial colony-forming cells (ECFCs) (85, 86), vascular progenitor cells derived from renal arteries (87) and BM-MSCs (79, 88, 89).
Interestingly, the study of Zou X et al. showed that hWJ-MSC-derived EVs upregulated vascular endothelial growth factor (VEGF) and downregulated hypoxia-inducible factor 1 (HIF-1α) in a rat model of IRI and that VEGF was through directly transferred by EVs (89).
Recent studies have shown that hypoxia preconditioning (HPC) can improve and differentiate EVs regenerative properties compared with EVs released under normal oxygenation, as secreted EVs convey the metabolic state of originating cell, including trophic factors protecting against hypoxia (106).
In the study by Collino et al. peculiar anti-apoptotic, anti-oxidative, mitochondrial energy-supply and pro-angiogenic pathways were activated by hypoxic AD-MSC derived EVs and induced a distinct proteomic pattern is in RTECs (107). Four effects were specifically enhanced in hypoxic EVs: downregulation of fibroblast growth factor receptor 1 (FGFR-1) and reduction of Transforming Growth Factor β-1 (TGFβ-1)-induced epithelial-to-mesenchimal transition (EMT) (108); promotion of angiogenesis through vascular endothelial growth factor (VEGF), blunting renal microvasculature rarefaction (109); translocation of Nrf-2 into the nucleus, activating antioxidant genes including Heme-oxigenase-1 (HO-1) (110); downregulation of IL6, reducing macrophage infiltration and polarization towards a M2 phenotype (111).
Hypoxic conditions have also been shown to promote angiogenic potential of iPSC-derived EVs (112).
An interesting aspect is that injured RTECs can themselves, especially when treated with HPC (113, 114), release EVs with anti-oxidant properties (94) and have the potential to modulate phenotypic and functional features of SCs, stimulating them to differentiate into normal RTEC. This effect may be ascribed to the release of a specific EV phenotype by de-differentiated tubular cells (57).
In another study, Dominguez JM et al. harvested hypoxic human RTECs and derived EVs from kidneys declined for transplantation and demonstrated that, after injecting them into nude rats exposed to bilateral renal ischemia, they both preserved renal function; however, EVs proved superior in maintaining renal vascular and epithelial networks, preventing oxidative stress and blunting pro-inflammatory and fibrogenic pathways. Proteomic analysis demonstrated broad ischemia-induced alterations at all cell levels and prevention of major drift in transcriptome by EV infusion (377 out of 628 altered proteins were “corrected” by EVs). This resulted in a reduced risk of evolution towards fibrosis and CKD (51).
On this basis, association of normoxic and hypoxic EVs has been proposed with the rationale of integrating respective peculiar effects (92, 115).
Finally, a recent study by Liu et al. showed that encapsulation of EVs isolated from human placenta (hP)-derived MSCs in a collagen matrix improved their retention in an AKI murine model, remarkably enhancing their therapeutic effects (inhibition of RTEC proliferation and of endoplasmic reticulum stress, stimulation of angiogenesis) compared with hP-MSC-derived EVs alone (116).
Taken together, all these studies indicate that SC-derived EVs have a multi-level therapeutic potential and that different type of EVs may specifically target pathophysiological aspects involved in ischemic AKI, either preventing it or accelerating its recovery.
Sepsis-Associated AKI (s-AKI)
As described above, sepsis is a common and life-threatening systemic disorder often leading to AKI in a clinical scenario of multiple organ failure due to the maladaptive host response to infection. S-AKI is not merely a consequence of ischemic damage due to hypoperfusion (renal overflow is in fact often normal or even increased) but recognizes a more complex pathogenesis. This includes microvascular damage and intrarenal redistribution of renal blood flow, activation of immune cells and complement with massive release of inflammatory molecules causing RTEC dysfunction (autophagy and mitophagy; arrest of cell cycle; dedifferentiation), endocrine dysregulation (16).
Transfer of miRNAs, mRNAs and proteins from activated immune and ECs through EVs may play a pivotal pathogenetic role in these processes but, on the other hand, it may also represent a therapeutic option for the use of SC-derived EVs (117). For example, EPC-derived EVs carrying mi-RNA-93 5p conferred renal protection in a LPS-induced mouse model of S-AKI, also alleviating multiple organ injury and vascular leakage (118) and blunted LPS-induced HK2 cell injury in another study (119).
During infections, MSC-derived EVs have been shown to eliminate pathogens and to regulate immune response through the secretion of antimicrobial factors, both inhibiting the replication of pathogens and activating the phagocytic function of macrophages (120). In a mouse model in which Escherichia Coli-derived outer membrane vesicles were intraperitoneally injected to establish sepsis, MSC-derived EVs significantly suppressed cytokine release into the systemic circulation, as well as PMN and monocyte infiltration in the peritoneum, by upregulating IL-10 production (121). In experimental models of sepsis obtained by LPS administration or by cecal ligation and puncture (CLP) MSC-derived EVs inhibited the development of disease by downregulating JMJD3 and inactivating the NF-κB signaling pathway through the selected transfer of miR-27b (122). EVs isolated from AD-MSCs have been shown to attenuate inflammation and protect from organ dysfunction by regulating the Notch-miR148a-3p signaling axis and decreasing macrophage polarization to M1 (123). In experimental s-AKI, the administration of EVs isolated from AD-MSCs exerted a renal protective effect through SIRT1 signaling pathway (124). In another CLP model of s-AKI, EVs obtained from UC-MSCs decreased IRAK1 expression through the up-regulation of miR-146b level, inhibited NF-κB activity and limited AKI and mortality (125). These results suggest an important immunomodulatory effect induced by MSC-EV administration in sepsis and a specific protective effect from AKI.
Last, EVs derived from mice pre-treated with remote ischemic preconditioning, elicited by brief periods of IRI in femoral arteries, appears to protect against s-AKI through miR-21, which integrates into RTECs and targets the downstream PDCD4/NF-κB and PTEN/AKT pathway (126).
The Process of AKI toCKD Transition
Recent studies have demonstrated that maladaptive repair after an AKI episode can predispose to evolution towards CKD and end-stage renal disease (ESRD) (127–129). Different factors appear to contribute to maladaptive repair during AKI, including oxidative stress, DNA damage, microvascular rarefaction, tubular loss, early fibrosis induced by endothelial-to-mesenchymal transition (EndMT) and pericyte-to-mesenchymal transition (PMT), lymphocyte infiltrates, inflammatory cytokine storm (128, 129). Pathophysiology of AKI-CKD transition has been the focus of research and complement system activation is increasingly recognised as having an essential role in this inflammatory scenario, which is closely related to renal senescence (130). These aspects and the potential of EVs as a tool to treat AKI and prevent AKI-CKD transition will be analysed in the following sections.
The Role of Complement in Renal Senescence and AKI-CKD Transition
In addition to liver synthesis, complement components can derive from renal cells (131) and complement C3 can be expressed by proximal RTECs, ECs, glomerular epithelial and mesangial cells after IRI (132, 133). Recent evidence indicates a crucial role of complement activation in renal tubules and vessels in AKI secondary to rhabdomyolysis (134) and trauma (135).
An aberrant complement activation and a high number of senescent cells also characterise both renal senescence and AKI-CKD transition, which is currently viewed as an accelerated form of kidney aging (136–138) and shares the same pathway and intracellular mediators (139, 140). Thus, there is actually a tight relationship between AKI itself and mechanism of senescence activation (129). From a molecular point of view, senescence refers to a well-defined program associated with cell cycle arrest, apoptosis inhibition and a pro-inflammatory “senescence-associated secretory phenotype” (SASP) (141). The SASP secretome relies on the production of a wide range of pro-inflammatory cytokines, chemokines, growth factors and matrix degrading factors promoting spread of senescence and fibrosis (142). Chronic accumulation of SASP cells leads to “inflammaging”, a persistent, low-grade inflammatory state which causes tissue deterioration (143, 144). Renal senescent cells can be detected by several markers, including loss of key nephroprotective factors such as Klotho. This transmembrane protein, expressed mainly on proximal RTECs where it interacts with the fibroblast growth factor receptor (FGF-23), regulates phosphate homeostasis (145) and exerts anti-fibrotic and anti-inflammatory actions through its 65-kDa soluble form, released into the bloodstream and urine (146). Disruption of Klotho gene determines shortened life span due to premature arteriosclerosis in mice (147) and AKI-induced Klotho deficiency accelerates renal fibrogenesis, retards renal tissue regeneration and promotes AKI-CKD transition (148, 149). Aberrant complement activation during AKI triggers inflammaging and represents an important link between AKI and CKD. Complement activation is involved in two key processes leading to CKD lesions: endothelial-to-mesenchymal transition (EndMT) and pericyte-to-myofibroblast transition (PMT) (130). In EndMT, complement drives ECs to acquire a myofibroblast phenotype, contributing to vascular damage and early fibrosis (150), as demonstrated in preclinical models of AKI induced by LPS and I/R (151). Similarly, complement also promotes PMT and enhances renal fibrogenesis. The loss of pericytes, which play a key role in angiogenesis and vascular homeostasis, is a hallmark of AKI and correlates with the decline of kidney function (152–154). Another interesting aspect is the role of C3 and C1q complement components in macrophages polarization, an essential factor in AKI evolution. While classically activated M1 macrophages contribute to initial injury, conversion to M2 anti-inflammatory macrophages during the recovery phase is critical in resolving inflammation and restoring tubular function. Strikingly, their differentiation into the M1 or M2 phenotype is regulated by C3 and C1q (155, 156). Overall, available evidence supports a critical role of complement in accelerating the process of premature aging which characterizes AKI-CKD transition. This phenomenon is more marked in the elderly, increasing susceptibility to accumulate chronic irreversible lesions after AKI events (157).
Potential Therapeutic Role of EVs in AKI-CKD Transition
Can EVs play a therapeutic role in reducing the risk of AKI-CKD transition? Although no study has specifically focused on this endpoint, there is some evidence from some of the previously mentioned studies that EV pleiotropic actions (Table 1) could inhibit AKI-CKD transition (51, 67). Activation of specific mediators such as Sox 9 (95) and transfer of miRNAs such as mi-R29b, which modulates Angiotensin 2-induced EMT of RTECs (158), are examples of effects which could be exploited to inhibit mechanisms leading to irreversible renal damage. Furthermore, as already mentioned, uEVs from healthy subjects can carry Klotho protein and transfer it to RTECs, restoring normal intra-tubular levels with beneficial effects on recovery from AKI (70).
Another interesting approach to prevent fibrosis after AKI is through complement blockade (159). A few studies suggest that EVs may exert an anti-complement activity through transfer of specific complement inhibitors. Cantaluppi et al. demonstrated that EPC-derived EVs could protect from complement mediated injury in experimental anti-Thy1.1 glomerulonephritis by transferring mRNAs coding for Factor H, CD55 and CD59 and related proteins to mesangial cell, thus inhibiting antibody/complement-induced apoptosis and C5b-9/C3 mesangial cell deposition (55). Similarly, EPC-derived EVs were able to preserve glomerular EC and podocyte integrity from complement-induced damage in a co-culture model mimicking the glomerular filtration barrier (160).
Although this setting is completely different from AKI, it is tempting to speculate that this mechanism of action might also explain some of the beneficial effects of EVs in AKI-CKD transition. Initial evidence indicates that human MSCs can ameliorate complement-induced inflammatory cascade and improve renal function at very early stages in experimental ischemic AKI, suggesting an immunomodulatory capacity possibly mediated by EVs (161). Finally, preliminary results from our group showed that EPC-derived EVs may limit ischemic AKI through complement inhibition (data not shown). Further studies are needed to investigate the potential of EVs as anti-complement therapy in order to prevent AKI-CKD transition. However, the potential of EV therapy to limit AKI development and AKI-CKD progression based on the horizontal transfer of proteins, receptors, bioactive lipids and different types of RNAs represents a great incentive for future research in this field.
Limits and Perspectives of EV-Based Therapy
The use of SC-derived EVs as a therapeutic tool to deliver growth factors, proteins and genetic material to injured renal resident cells is promising in different AKI fields: however, several obstacles still limit their translation to clinic and have been recently reviewed (162, 163).
Biochemical composition is not defined and can vary depending on parental cell but also on surrounding milieu (e.g. inflammation, hypoxia). EVs released from the same cell-type may even have contradictory effects: for example, hypoxic RTECs have proved beneficial in alleviating tubular damage and fibrosis but injured RTECs can also release EVs which contribute to amplify inflammation (164). Moreover, focusing on SC-derived EVs, a different phenotype may depend on donor characteristics (autologous vs. heterologous, age, gender, presence of comorbidities or transient inflammatory states, etc.)
Furthermore, EV production or uptake mechanisms by kidney resident cells or infiltrating inflammatory cells are not completely defined and an intact glomerular filtration barrier could prevent EVs from reaching podocytes and tubular cells (165).
Finally, lack of good manufacturing practice standards and high-scale EV production hinder clinical application. At the moment, the clinical use of EVs is not classified as cell therapy and their mechanisms of action look like more to administration of a drug rather than a real cell therapy. Moreover, despite the development of new isolation procedures, cGMP production of SC-derived EVs for clinical application seems still to depend on an ultracentrifugation step to be performed within a cell factory.
Despite these limits, EV-based therapy has many strengths and is opening new therapeutic perspectives for a condition currently treated only with supportive therapy.
In general, EV lipid and surface protein composition (e.g.CD47) limits phagocytosis by circulating monocytes and prolongs blood half-life if compared to liposomes or other nanoparticles employed to carry drugs. EVs usually express integrins and adhesion molecules which allow to enhance their homing to inflamed or injured tissue: moreover, EVs protect RNA from degradation after their intravenous administration and at tissue level.
Technologies such as tangential flow filtration (TFF) appears to allow large-scale production of high-quality, reproducible EVs from AD-MSCs, paving the way for potential widespread clinical application in AKI (166). Other technological advances may potentiate EV qualitative therapeutic properties. For example, EV encapsulation could make their therapeutic content (e.g. miRNAs, mRNAs, proteins) more protected and stable. Nanomedicine techniques may help engineering EV features (size, shape, surface charge) in order to enable them to pass specific biological barriers, including glomeruli. Decoy exosomes have been proposed to antagonize inflammatory mediators (167). Another therapeutic approach is that of transfecting MSCs with specific miRNA mimics in order to enrich them with selected miRNAs. These enriched EVs proved to be more effective than naïve ones, potentially allowing the use of a lower amount of them (168). Combination therapy of pulsed focused ultrasound (pFUS) and EVs has proved more effective than either approach alone in reversing AKI-related inflammation through suppression of heat shock protein 70 – mediated NLPR3 inflammasome (169).
An interesting tool to increase therapeutic potential of MSCs is the adoption of three-dimensional (3D) culture of human placental MSC (hPMSCs), which proved to be more effective than two-dimension (2D) culture in preventing renal damage when injected in a mouse model of IRI-induced AKI (170). MSC 3D spheroid structures enable increased cell-cell interactions and enhance MSC trophic and immunomodulatory functions, with more reproducible clinical outcomes in many preclinical models (171) including cisplatin-induced AKI (172).
We have already discussed the potential of hypoxia and collagen matrix encapsulation to enhance EV protective effects, paving the way to new possibilities of therapeutic manipulation (106, 107, 116). Of note, the use of EVs avoid the possible adverse effects associated with whole cell therapies such as pulmonary embolism, vascular thrombosis, maldifferentiation and tumorigenesis (173).
Conclusions
A growing body of evidence based on pre-clinical studies suggests that EVs released from MSCs of different origin and from other SC types could be effective to treat toxic, ischemic and septic AKI through direct interference with multiple etiopathogenetic mechanisms of tubular and endothelial damage. A network of cytoprotective, regenerative and immunomodulatory EV properties is being defined. EV-based therapy could prevent renal fibrosis and AKI-CKD transition, also through inhibition of complement-mediated processes such as EndMT and PMT. Hypoxia-conditioned and engineered EVs with enhanced therapeutic properties are promising new tools. Even though some technological hurdles must still be overcome before widespread clinical application, EV-based therapies may become a cornerstone for the treatment of the most common forms of AKI in the near future.
Authors Contributions
MQ and VC designed and wrote the initial manuscript. GM and AC designed Figures (Created with BioRender.com) and Tables and arranged References. SB, AS, RF, EG, and VF reviewed the article focusing on experimental models. GC wrote the paragraph on AKI-CKD transition and all authors critically revised the whole article.
Funding
This study was partially funded by the Italian Ministry of Education, University and Research (MIUR) program “Departments of Excellence 2018-2022”, AGING Project – Department of Translational Medicine, University of Piemonte Orientale (UPO) and by local grants of the University of Piemonte Orientale (UPO, FAR) to M.Q. and V.C.
Conflict of Interest
The authors declare that the research was conducted in the absence of any commercial or financial relationships that could be construed as a potential conflict of interest.
Publisher’s Note
All claims expressed in this article are solely those of the authors and do not necessarily represent those of their affiliated organizations, or those of the publisher, the editors and the reviewers. Any product that may be evaluated in this article, or claim that may be made by its manufacturer, is not guaranteed or endorsed by the publisher.
Glossary
References
1. Susantitaphong P, Cruz DN, Cerda J, Abulfaraj M, Alqahtani F, Koulouridis I, et al. World Incidence of AKI: A Meta-Analysis. Clin J Am Soc Nephrol (2013) 8:1482–93. doi: 10.2215/CJN.00710113
2. Hoste EAJ, Bagshaw SM, Bellomo R, Cely CM, Colman R, Cruz DN, et al. Epidemiology of Acute Kidney Injury in Critically Ill Patients: The Multinational AKI-EPI Study. Intensive Care Med (2015) 41:1411–23. doi: 10.1007/s00134-015-3934-7
3. Pavkov ME, Harding JL, Burrows NR. Trends in Hospitalizations for Acute Kidney Injury - United States, 2000-2014. MMWR Morb Mortal Wkly Rep (2018) 67:289–93. doi: 10.15585/mmwr.mm6710a2
4. Dasta JF, Kane-Gill S. Review of the Literature on the Costs Associated With Acute Kidney Injury. J Pharm Pract (2019) 32:292–302. doi: 10.1177/0897190019852556
5. Grams ME, Sang Y, Ballew SH, Gansevoort RT, Kimm H, Kovesdy CP, et al. A Meta-Analysis of the Association of Estimated GFR, Albuminuria, Age, Race, and Sex With Acute Kidney Injury. Am J Kidney Dis (2015) 66:591–601. doi: 10.1053/j.ajkd.2015.02.337
6. Heung M, Dickinson T, Wu X, Fitzgerald DC, DeLucia A, Paone G, et al. The Role of Race on Acute Kidney Injury Following Cardiac Surgery. Ann Thorac Surg (2021) S0003-4975(21)01978-0. doi: 10.1016/j.athoracsur.2021.10.031
7. Siew ED, Davenport A. The Growth of Acute Kidney Injury: A Rising Tide or Just Closer Attention to Detail? Kidney Int (2015) 87:46–61. doi: 10.1038/ki.2014.293
8. Waikar SS, Liu KD, Chertow GM. The Incidence and Prognostic Significance of Acute Kidney Injury. Curr Opin Nephrol Hypertens (2007) 16:227–36. doi: 10.1097/MNH.0b013e3280dd8c35
9. Ponticelli C, Sala G, Glassock RJ. Drug Management in the Elderly Adult With Chronic Kidney Disease: A Review for the Primary Care Physician. Mayo Clin Proc (2015) 90:633–45. doi: 10.1016/j.mayocp.2015.01.016
10. Frame AA, Wainford RD. Mechanisms of Altered Renal Sodium Handling in Age-Related Hypertension. Am J Physiol Renal Physiol (2018) 315:F1–6. doi: 10.1152/ajprenal.00594.2017
11. Singer M, Deutschman CS, Seymour CW, Shankar-Hari M, Annane D, Bauer M, et al. The Third International Consensus Definitions for Sepsis and Septic Shock (Sepsis-3). JAMA (2016) 315:801–10. doi: 10.1001/jama.2016.0287
12. Hotchkiss RS, Monneret G, Payen D. Sepsis-Induced Immunosuppression: From Cellular Dysfunctions to Immunotherapy. Nat Rev Immunol (2013) 13:862–74. doi: 10.1038/nri3552
13. McDonald B, Davis RP, Kim S-J, Tse M, Esmon CT, Kolaczkowska E, et al. Platelets and Neutrophil Extracellular Traps Collaborate to Promote Intravascular Coagulation During Sepsis in Mice. Blood (2017) 129:1357–67. doi: 10.1182/blood-2016-09-741298
14. Keragala CB, Draxler DF, McQuilten ZK, Medcalf RL. Haemostasis and Innate Immunity - a Complementary Relationship: A Review of the Intricate Relationship Between Coagulation and Complement Pathways. Br J Haematol (2018) 180:782–98. doi: 10.1111/bjh.15062
15. Legrand M, Bell S, Forni L, Joannidis M, Koyner JL, Liu K, et al. Pathophysiology of COVID-19-Associated Acute Kidney Injury. Nat Rev Nephrol (2021) 17:751–64. doi: 10.1038/s41581-021-00452-0
16. Dellepiane S, Marengo M, Cantaluppi V. Detrimental Cross-Talk Between Sepsis and Acute Kidney Injury: New Pathogenic Mechanisms, Early Biomarkers and Targeted Therapies. Crit Care (2016) 20:61. doi: 10.1186/s13054-016-1219-3
17. Peerapornratana S, Manrique-Caballero CL, Gómez H, Kellum JA. Acute Kidney Injury From Sepsis: Current Concepts, Epidemiology, Pathophysiology, Prevention and Treatment. Kidney Int (2019) 96:1083–99. doi: 10.1016/j.kint.2019.05.026
18. Jha AK, Gairola S, Kundu S, Doye P, Syed AM, Ram C, et al. Toll-Like Receptor 4: An Attractive Therapeutic Target for Acute Kidney Injury. Life Sci (2021) 271:119155. doi: 10.1016/j.lfs.2021.119155
19. van der Slikke EC, Star BS, van Meurs M, Henning RH, Moser J, Bouma HR. Sepsis Is Associated With Mitochondrial DNA Damage and a Reduced Mitochondrial Mass in the Kidney of Patients With Sepsis-AKI. Crit Care (2021) 25:36. doi: 10.1186/s13054-020-03424-1
20. Gómez H, Kellum JA, Ronco C. Metabolic Reprogramming and Tolerance During Sepsis-Induced AKI. Nat Rev Nephrol (2017) 13:143–51. doi: 10.1038/nrneph.2016.186
21. Dellepiane S, Leventhal JS, Cravedi P. T Cells and Acute Kidney Injury: A Two-Way Relationship. Front Immunol (2020) 11:1546. doi: 10.3389/fimmu.2020.01546
22. Eini H, Tejman-Yarden N, Lewis EC, Chaimovitz C, Zlotnik M, Douvdevani A. Association Between Renal Injury and Reduced Interleukin-15 and Interleukin-15 Receptor Levels in Acute Kidney Injury. J Interferon Cytokine Res (2010) 30:1–8. doi: 10.1089/jir.2009.0005
23. Devocelle A, Lecru L, François H, Desterke C, Gallerne C, Eid P, et al. Inhibition of TGF-β1 Signaling by IL-15: A Novel Role for IL-15 in the Control of Renal Epithelial-Mesenchymal Transition: IL-15 Counteracts TGF-β1-Induced EMT in Renal Fibrosis. Int J Cell Biol (2019) 2019:9151394. doi: 10.1155/2019/9151394
24. Devocelle A, Lecru L, Ferlicot S, Bessede T, Candelier J-J, Giron-Michel J, et al. IL-15 Prevents Renal Fibrosis by Inhibiting Collagen Synthesis: A New Pathway in Chronic Kidney Disease? Int J Mol Sci (2021) 22:11698. doi: 10.3390/ijms222111698
25. Khwaja A. KDIGO Clinical Practice Guidelines for Acute Kidney Injury. Nephron Clin Pract (2012) 120:c179–184. doi: 10.1159/000339789
26. Moore PK, Hsu RK, Liu KD. Management of Acute Kidney Injury: Core Curriculum 2018. Am J Kidney Dis (2018) 72:136–48. doi: 10.1053/j.ajkd.2017.11.021
27. Meersch M, Schmidt C, Hoffmeier A, Van Aken H, Wempe C, Gerss J, et al. Prevention of Cardiac Surgery-Associated AKI by Implementing the KDIGO Guidelines in High Risk Patients Identified by Biomarkers: The PrevAKI Randomized Controlled Trial. Intensive Care Med (2017) 43:1551–61. doi: 10.1007/s00134-016-4670-3
28. Bagshaw SM, Lapinsky S, Dial S, Arabi Y, Dodek P, Wood G, et al. Acute Kidney Injury in Septic Shock: Clinical Outcomes and Impact of Duration of Hypotension Prior to Initiation of Antimicrobial Therapy. Intensive Care Med (2009) 35:871–81. doi: 10.1007/s00134-008-1367-2
29. Perico N, Casiraghi F, Remuzzi G. Clinical Translation of Mesenchymal Stromal Cell Therapies in Nephrology. J Am Soc Nephrol (2018) 29:362–75. doi: 10.1681/ASN.2017070781
30. Bochon B, Kozubska M, Surygała G, Witkowska A, Kuźniewicz R, Grzeszczak W, et al. Mesenchymal Stem Cells-Potential Applications in Kidney Diseases. Int J Mol Sci (2019) 20(10):2462. doi: 10.3390/ijms20102462
31. Tsuji K, Kitamura S, Wada J. Immunomodulatory and Regenerative Effects of Mesenchymal Stem Cell-Derived Extracellular Vesicles in Renal Diseases. Int J Mol Sci (2020) 21(3):756. doi: 10.3390/ijms21030756
32. Zakrzewski W, Dobrzyński M, Szymonowicz M, Rybak Z. Stem Cells: Past, Present, and Future. Stem Cell Res Ther (2019) 10:68. doi: 10.1186/s13287-019-1165-5
33. Dominici M, Le Blanc K, Mueller I, Slaper-Cortenbach I, Marini F, Krause D, et al. Minimal Criteria for Defining Multipotent Mesenchymal Stromal Cells. The International Society for Cellular Therapy Position Statement. Cytotherapy (2006) 8:315–7. doi: 10.1080/14653240600855905
34. Pittenger MF, Mackay AM, Beck SC, Jaiswal RK, Douglas R, Mosca JD, et al. Multilineage Potential of Adult Human Mesenchymal Stem Cells. Science (1999) 284:143–7. doi: 10.1126/science.284.5411.143
35. Hass R, Kasper C, Böhm S, Jacobs R. Different Populations and Sources of Human Mesenchymal Stem Cells (MSC): A Comparison of Adult and Neonatal Tissue-Derived MSC. Cell Commun Signal (2011) 9:12. doi: 10.1186/1478-811X-9-12
36. Morigi M, Benigni A. Mesenchymal Stem Cells and Kidney Repair. Nephrol Dial Transplant (2013) 28:788–93. doi: 10.1093/ndt/gfs556
37. Tsuda H, Yamahara K, Otani K, Okumi M, Yazawa K, Kaimori J, et al. Transplantation of Allogenic Fetal Membrane-Derived Mesenchymal Stem Cells Protects Against Ischemia/Reperfusion-Induced Acute Kidney Injury. Cell Transplant (2014) 23:889–99. doi: 10.3727/096368913X665594
38. Bellavia M, Altomare R, Cacciabaudo F, Santoro A, Allegra A, Concetta Gioviale M, et al. Towards an Ideal Source of Mesenchymal Stem Cell Isolation for Possible Therapeutic Application in Regenerative Medicine. BioMed Pap Med Fac Univ Palacky Olomouc Czech Repub (2014) 158:356–60. doi: 10.5507/bp.2013.051
39. Barnes CJ, Distaso CT, Spitz KM, Verdun VA, Haramati A. Comparison of Stem Cell Therapies for Acute Kidney Injury. Am J Stem Cells (2016) 5:1–10.
40. Liu Y, Fang J. Mesenchymal Stem Cells as Therapeutic Agents and Novel Carriers for the Delivery of Candidate Genes in Acute Kidney Injury. Stem Cells Int (2020) 2020:8875554. doi: 10.1155/2020/8875554
41. Thatava T, Armstrong AS, De Lamo JG, Edukulla R, Khan YK, Sakuma T, et al. Successful Disease-Specific Induced Pluripotent Stem Cell Generation From Patients With Kidney Transplantation. Stem Cell Res Ther (2011) 2:48. doi: 10.1186/scrt89
42. Lee P-Y, Chien Y, Chiou G-Y, Lin C-H, Chiou C-H, Tarng D-C. Induced Pluripotent Stem Cells Without C-Myc Attenuate Acute Kidney Injury via Downregulating the Signaling of Oxidative Stress and Inflammation in Ischemia-Reperfusion Rats. Cell Transplant (2012) 21:2569–85. doi: 10.3727/096368912X636902
43. De Chiara L, Fagoonee S, Ranghino A, Bruno S, Camussi G, Tolosano E, et al. Renal Cells From Spermatogonial Germline Stem Cells Protect Against Kidney Injury. J Am Soc Nephrol (2014) 25:316–28. doi: 10.1681/ASN.2013040367
44. Ozkok A, Yildiz A. Endothelial Progenitor Cells and Kidney Diseases. Kidney Blood Press Res (2018) 43:701–18. doi: 10.1159/000489745
45. Karpman D, Ståhl A-L, Arvidsson I. Extracellular Vesicles in Renal Disease. Nat Rev Nephrol (2017) 13:545–62. doi: 10.1038/nrneph.2017.98
46. Lv L-L, Feng Y, Tang T-T, Liu B-C. New Insight Into the Role of Extracellular Vesicles in Kidney Disease. J Cell Mol Med (2019) 23:731–9. doi: 10.1111/jcmm.14101
47. Maas SLN, Breakefield XO, Weaver AM. Extracellular Vesicles: Unique Intercellular Delivery Vehicles. Trends Cell Biol (2017) 27:172–88. doi: 10.1016/j.tcb.2016.11.003
48. Bruno S, Chiabotto G, Favaro E, Deregibus MC, Camussi G. Role of extracellular vesicles in stem cell biology. Am J Physiol Cell Physiol (2019) 317:C303–13. doi: 10.1152/ajpcell.00129.2019
49. Choi HY, Moon SJ, Ratliff BB, Ahn SH, Jung A, Lee M, et al. Microparticles From Kidney-Derived Mesenchymal Stem Cells Act as Carriers of Proangiogenic Signals and Contribute to Recovery From Acute Kidney Injury. PLoS One (2014) 9:e87853. doi: 10.1371/journal.pone.0087853
50. Ranghino A, Bruno S, Bussolati B, Moggio A, Dimuccio V, Tapparo M, et al. The Effects of Glomerular and Tubular Renal Progenitors and Derived Extracellular Vesicles on Recovery From Acute Kidney Injury. Stem Cell Res Ther (2017) 8:24. doi: 10.1186/s13287-017-0478-5
51. Dominguez JM, Dominguez JH, Xie D, Kelly KJ. Human Extracellular Microvesicles From Renal Tubules Reverse Kidney Ischemia-Reperfusion Injury in Rats. PLoS One (2018) 13:e0202550. doi: 10.1371/journal.pone.0202550
52. Grange C, Papadimitriou E, Dimuccio V, Pastorino C, Molina J, O’Kelly R, et al. Urinary Extracellular Vesicles Carrying Klotho Improve the Recovery of Renal Function in an Acute Tubular Injury Model. Mol Ther (2020) 28:490–502. doi: 10.1016/j.ymthe.2019.11.013
53. Woith E, Melzig MF. Extracellular Vesicles From Fresh and Dried Plants-Simultaneous Purification and Visualization Using Gel Electrophoresis. Int J Mol Sci (2019) 20:E357. doi: 10.3390/ijms20020357
54. Del Pozo-Acebo L, López de Las Hazas M-C, Margollés A, Dávalos A, García-Ruiz A. Eating microRNAs: Pharmacological Opportunities for Cross-Kingdom Regulation and Implications in Host Gene and Gut Microbiota Modulation. Br J Pharmacol (2021) 178:2218–45. doi: 10.1111/bph.15421
55. Cantaluppi V, Medica D, Mannari C, Stiaccini G, Figliolini F, Dellepiane S, et al. Endothelial Progenitor Cell-Derived Extracellular Vesicles Protect From Complement-Mediated Mesangial Injury in Experimental Anti-Thy1.1 Glomerulonephritis. Nephrol Dial Transplant (2015) 30:410–22. doi: 10.1093/ndt/gfu364
56. Cantaluppi V, Gatti S, Medica D, Figliolini F, Bruno S, Deregibus MC, et al. Microvesicles Derived From Endothelial Progenitor Cells Protect the Kidney From Ischemia-Reperfusion Injury by microRNA-Dependent Reprogramming of Resident Renal Cells. Kidney Int (2012) 82:412–27. doi: 10.1038/ki.2012.105
57. Zhao L, Hu C, Zhang P, Jiang H, Chen J. Genetic Communication by Extracellular Vesicles Is an Important Mechanism Underlying Stem Cell-Based Therapy-Mediated Protection Against Acute Kidney Injury. Stem Cell Res Ther (2019) 10:119. doi: 10.1186/s13287-019-1227-8
58. Bruno S, Collino F, Deregibus MC, Grange C, Tetta C, Camussi G. Microvesicles Derived From Human Bone Marrow Mesenchymal Stem Cells Inhibit Tumor Growth. Stem Cells Dev (2013) 22:758–71. doi: 10.1089/scd.2012.0304
59. Večerić-Haler Ž, Cerar A, Perše M. (Mesenchymal) Stem Cell-Based Therapy in Cisplatin-Induced Acute Kidney Injury Animal Model: Risk of Immunogenicity and Tumorigenicity. Stem Cells Int (2017) 2017:7304643. doi: 10.1155/2017/7304643
60. Fatima F, Ekstrom K, Nazarenko I, Maugeri M, Valadi H, Hill AF, et al. Non-Coding RNAs in Mesenchymal Stem Cell-Derived Extracellular Vesicles: Deciphering Regulatory Roles in Stem Cell Potency, Inflammatory Resolve, and Tissue Regeneration. Front Genet (2017) 8:161. doi: 10.3389/fgene.2017.00161
61. Tsuji K, Kitamura S, Wada J. Secretomes From Mesenchymal Stem Cells Against Acute Kidney Injury: Possible Heterogeneity. Stem Cells Int (2018) 2018:8693137. doi: 10.1155/2018/8693137
62. Kaushal GP, Shah SV. Autophagy in Acute Kidney Injury. Kidney Int (2016) 89:779–91. doi: 10.1016/j.kint.2015.11.021
63. Grange C, Skovronova R, Marabese F, Bussolati B. Stem Cell-Derived Extracellular Vesicles and Kidney Regeneration. Cells (2019) 8(10):1240. doi: 10.3390/cells8101240
64. Maccario R, Podestà M, Moretta A, Cometa A, Comoli P, Montagna D, et al. Interaction of Human Mesenchymal Stem Cells With Cells Involved in Alloantigen-Specific Immune Response Favors the Differentiation of CD4+ T-Cell Subsets Expressing a Regulatory/Suppressive Phenotype. Haematologica (2005) 90:516–25.
65. Zou X, Gu D, Zhang G, Zhong L, Cheng Z, Liu G, et al. NK Cell Regulatory Property Is Involved in the Protective Role of MSC-Derived Extracellular Vesicles in Renal Ischemic Reperfusion Injury. Hum Gene Ther (2016) 27:926–35. doi: 10.1089/hum.2016.057
66. Bruno S, Grange C, Deregibus MC, Calogero RA, Saviozzi S, Collino F, et al. Mesenchymal Stem Cell-Derived Microvesicles Protect Against Acute Tubular Injury. J Am Soc Nephrol (2009) 20:1053–67. doi: 10.1681/ASN.2008070798
67. Gatti S, Bruno S, Deregibus MC, Sordi A, Cantaluppi V, Tetta C, et al. Microvesicles Derived From Human Adult Mesenchymal Stem Cells Protect Against Ischaemia-Reperfusion-Induced Acute and Chronic Kidney Injury. Nephrol Dial Transplant (2011) 26:1474–83. doi: 10.1093/ndt/gfr015
68. Ferguson SW, Wang J, Lee CJ, Liu M, Neelamegham S, Canty JM, et al. The microRNA Regulatory Landscape of MSC-Derived Exosomes: A Systems View. Sci Rep (2018) 8:1419. doi: 10.1038/s41598-018-19581-x
69. Wang S-Y, Hong Q, Zhang C-Y, Yang Y-J, Cai G-Y, Chen X-M. miRNAs in Stem Cell-Derived Extracellular Vesicles for Acute Kidney Injury Treatment: Comprehensive Review of Preclinical Studies. Stem Cell Res Ther (2019) 10:281. doi: 10.1186/s13287-019-1371-1
70. Bussolati B, Camussi G. Therapeutic Use of Human Renal Progenitor Cells for Kidney Regeneration. Nat Rev Nephrol (2015) 11:695–706. doi: 10.1038/nrneph.2015.126
71. Sun IO, Kwon SH. Extracellular Vesicles: A Novel Window Into Kidney Function and Disease. Curr Opin Nephrol Hypertens (2020) 29:613–9. doi: 10.1097/MNH.0000000000000641
72. Herrera Sanchez MB, Bruno S, Grange C, Tapparo M, Cantaluppi V, Tetta C, et al. Human Liver Stem Cells and Derived Extracellular Vesicles Improve Recovery in a Murine Model of Acute Kidney Injury. Stem Cell Res Ther (2014) 5:124. doi: 10.1186/scrt514
73. Bruno S, Grange C, Collino F, Deregibus MC, Cantaluppi V, Biancone L, et al. Microvesicles Derived From Mesenchymal Stem Cells Enhance Survival in a Lethal Model of Acute Kidney Injury. PLoS One (2012) 7:e33115. doi: 10.1371/journal.pone.0033115
74. Zhou Y, Xu H, Xu W, Wang B, Wu H, Tao Y, et al. Exosomes Released by Human Umbilical Cord Mesenchymal Stem Cells Protect Against Cisplatin-Induced Renal Oxidative Stress and Apoptosis In Vivo and In Vitro. Stem Cell Res Ther (2013) 4:34. doi: 10.1186/scrt194
75. Lindoso RS, Collino F, Bruno S, Araujo DS, Sant’Anna JF, Tetta C, et al. Extracellular Vesicles Released From Mesenchymal Stromal Cells Modulate miRNA in Renal Tubular Cells and Inhibit ATP Depletion Injury. Stem Cells Dev (2014) 23:1809–19. doi: 10.1089/scd.2013.0618
76. Ju G, Cheng J, Zhong L, Wu S, Zou X, Zhang G, et al. Microvesicles Derived From Human Umbilical Cord Mesenchymal Stem Cells Facilitate Tubular Epithelial Cell Dedifferentiation and Growth via Hepatocyte Growth Factor Induction. PLoS One (2015) 10:e0121534. doi: 10.1371/journal.pone.0121534
77. Yuan X, Wang X, Chen C, Zhou J, Han M. Bone Mesenchymal Stem Cells Ameliorate Ischemia/Reperfusion-Induced Damage in Renal Epithelial Cells via microRNA-223. Stem Cell Res Ther (2017) 8:146. doi: 10.1186/s13287-017-0599-x
78. Chen W, Yan Y, Song C, Ding Y, Du T. Microvesicles Derived From Human Wharton’s Jelly Mesenchymal Stem Cells Ameliorate Ischemia-Reperfusion-Induced Renal Fibrosis by Releasing From G2/M Cell Cycle Arrest. Biochem J (2017) 474:4207–18. doi: 10.1042/BCJ20170682
79. Zhu G, Pei L, Lin F, Yin H, Li X, He W, et al. Exosomes From Human-Bone-Marrow-Derived Mesenchymal Stem Cells Protect Against Renal Ischemia/Reperfusion Injury via Transferring miR-199a-3p. J Cell Physiol (2019) 234:23736–49. doi: 10.1002/jcp.28941
80. Wang R, Lin M, Li L, Li L, Qi G, Rong R, et al. [Bone Marrow Mesenchymal Stem Cell-Derived Exosome Protects Kidney Against Ischemia Reperfusion Injury in Rats]. Zhonghua Yi Xue Za Zhi (2014) 94:3298–303. doi: 10.3760/cma.j.issn.0376-2491.2014.42.005
81. Zhang G, Zou X, Miao S, Chen J, Du T, Zhong L, et al. The Anti-Oxidative Role of Micro-Vesicles Derived From Human Wharton-Jelly Mesenchymal Stromal Cells Through NOX2/gp91(phox) Suppression in Alleviating Renal Ischemia-Reperfusion Injury in Rats. PLoS One (2014) 9:e92129. doi: 10.1371/journal.pone.0092129
82. Zhang G, Zou X, Huang Y, Wang F, Miao S, Liu G, et al. Mesenchymal Stromal Cell-Derived Extracellular Vesicles Protect Against Acute Kidney Injury Through Anti-Oxidation by Enhancing Nrf2/ARE Activation in Rats. Kidney Blood Press Res (2016) 41:119–28. doi: 10.1159/000443413
83. Gu D, Zou X, Ju G, Zhang G, Bao E, Zhu Y. Mesenchymal Stromal Cells Derived Extracellular Vesicles Ameliorate Acute Renal Ischemia Reperfusion Injury by Inhibition of Mitochondrial Fission Through miR-30. Stem Cells Int (2016) 2016:2093940. doi: 10.1155/2016/2093940
84. Wang C, Zhu G, He W, Yin H, Lin F, Gou X, et al. BMSCs Protect Against Renal Ischemia-Reperfusion Injury by Secreting Exosomes Loaded With miR-199a-5p That Target BIP to Inhibit Endoplasmic Reticulum Stress at the Very Early Reperfusion Stages. FASEB J (2019) 33:5440–56. doi: 10.1096/fj.201801821R
85. Burger D, Viñas JL, Akbari S, Dehak H, Knoll W, Gutsol A, et al. Human Endothelial Colony-Forming Cells Protect Against Acute Kidney Injury: Role of Exosomes. Am J Pathol (2015) 185:2309–23. doi: 10.1016/j.ajpath.2015.04.010
86. Viñas JL, Burger D, Zimpelmann J, Haneef R, Knoll W, Campbell P, et al. Transfer of microRNA-486-5p From Human Endothelial Colony Forming Cell-Derived Exosomes Reduces Ischemic Kidney Injury. Kidney Int (2016) 90:1238–50. doi: 10.1016/j.kint.2016.07.015
87. Pang P, Abbott M, Chang SL, Abdi M, Chauhan N, Mistri M, et al. Human Vascular Progenitor Cells Derived From Renal Arteries Are Endothelial-Like and Assist in the Repair of Injured Renal Capillary Networks. Kidney Int (2017) 91:129–43. doi: 10.1016/j.kint.2016.07.037
88. Liang X, Zhang L, Wang S, Han Q, Zhao RC. Exosomes Secreted by Mesenchymal Stem Cells Promote Endothelial Cell Angiogenesis by Transferring miR-125a. J Cell Sci (2016) 129:2182–9. doi: 10.1242/jcs.170373
89. Zou X, Gu D, Xing X, Cheng Z, Gong D, Zhang G, et al. Human Mesenchymal Stromal Cell-Derived Extracellular Vesicles Alleviate Renal Ischemic Reperfusion Injury and Enhance Angiogenesis in Rats. Am J Transl Res (2016) 8:4289–99.
90. Zou X, Zhang G, Cheng Z, Yin D, Du T, Ju G, et al. Microvesicles Derived From Human Wharton’s Jelly Mesenchymal Stromal Cells Ameliorate Renal Ischemia-Reperfusion Injury in Rats by Suppressing CX3CL1. Stem Cell Res Ther (2014) 5:40. doi: 10.1186/scrt428
91. Shen B, Liu J, Zhang F, Wang Y, Qin Y, Zhou Z, et al. CCR2 Positive Exosome Released by Mesenchymal Stem Cells Suppresses Macrophage Functions and Alleviates Ischemia/Reperfusion-Induced Renal Injury. Stem Cells Int (2016) 2016:1240301. doi: 10.1155/2016/1240301
92. Lin K-C, Yip H-K, Shao P-L, Wu S-C, Chen K-H, Chen Y-T, et al. Combination of Adipose-Derived Mesenchymal Stem Cells (ADMSC) and ADMSC-Derived Exosomes for Protecting Kidney From Acute Ischemia-Reperfusion Injury. Int J Cardiol (2016) 216:173–85. doi: 10.1016/j.ijcard.2016.04.061
93. Wu X, Yan T, Wang Z, Wu X, Cao G, Zhang C, et al. Micro-Vesicles Derived From Human Wharton’s Jelly Mesenchymal Stromal Cells Mitigate Renal Ischemia-Reperfusion Injury in Rats After Cardiac Death Renal Transplantation. J Cell Biochem (2018) 119:1879–88. doi: 10.1002/jcb.26348
94. Dominguez JH, Liu Y, Gao H, Dominguez JM, Xie D, Kelly KJ. Renal Tubular Cell-Derived Extracellular Vesicles Accelerate the Recovery of Established Renal Ischemia Reperfusion Injury. J Am Soc Nephrol (2017) 28:3533–44. doi: 10.1681/ASN.2016121278
95. Zhu F, Chong Lee Shin OLS, Pei G, Hu Z, Yang J, Zhu H, et al. Adipose-Derived Mesenchymal Stem Cells Employed Exosomes to Attenuate AKI-CKD Transition Through Tubular Epithelial Cell Dependent Sox9 Activation. Oncotarget (2017) 8:70707–26. doi: 10.18632/oncotarget.19979
96. Zhang K, Chen S, Sun H, Wang L, Li H, Zhao J, et al. In Vivo Two-Photon Microscopy Reveals the Contribution of Sox9+ Cell to Kidney Regeneration in a Mouse Model With Extracellular Vesicle Treatment. J Biol Chem (2020) 295:12203–13. doi: 10.1074/jbc.RA120.012732
97. Reis LA, Borges FT, Simões MJ, Borges AA, Sinigaglia-Coimbra R, Schor N. Bone Marrow-Derived Mesenchymal Stem Cells Repaired But did Not Prevent Gentamicin-Induced Acute Kidney Injury Through Paracrine Effects in Rats. PLoS One (2012) 7:e44092. doi: 10.1371/journal.pone.0044092
98. Song N, Zhang T, Xu X, Lu Z, Yu X, Fang Y, et al. miR-21 Protects Against Ischemia/Reperfusion-Induced Acute Kidney Injury by Preventing Epithelial Cell Apoptosis and Inhibiting Dendritic Cell Maturation. Front Physiol (2018) 9:790. doi: 10.3389/fphys.2018.00790
99. Panfoli I, Ravera S, Podestà M, Cossu C, Santucci L, Bartolucci M, et al. Exosomes From Human Mesenchymal Stem Cells Conduct Aerobic Metabolism in Term and Preterm Newborn Infants. FASEB J (2016) 30:1416–24. doi: 10.1096/fj.15-279679
100. Visvanathan A, Ahmed K, Even-Faitelson L, Lleres D, Bazett-Jones DP, Lamond AI. Modulation of Higher Order Chromatin Conformation in Mammalian Cell Nuclei Can Be Mediated by Polyamines and Divalent Cations. PLoS One (2013) 8:e67689. doi: 10.1371/journal.pone.0067689
101. Zhao M, Liu S, Wang C, Wang Y, Wan M, Liu F, et al. Mesenchymal Stem Cell-Derived Extracellular Vesicles Attenuate Mitochondrial Damage and Inflammation by Stabilizing Mitochondrial DNA. ACS Nano (2021) 15:1519–38. doi: 10.1021/acsnano.0c08947
102. Prior K-K, Wittig I, Leisegang MS, Groenendyk J, Weissmann N, Michalak M, et al. The Endoplasmic Reticulum Chaperone Calnexin Is a NADPH Oxidase NOX4 Interacting Protein. J Biol Chem (2016) 291:7045–59. doi: 10.1074/jbc.M115.710772
103. Barreca MM, Cancemi P, Geraci F. Mesenchymal and Induced Pluripotent Stem Cells-Derived Extracellular Vesicles: The New Frontier for Regenerative Medicine? Cells (2020) 9:E1163. doi: 10.3390/cells9051163
104. Collino F, Lopes JA, Tapparo M, Tortelote GG, Kasai-Brunswick TH, Lopes GMC, et al. Extracellular Vesicles Derived From Induced Pluripotent Stem Cells Promote Renoprotection in Acute Kidney Injury Model. Cells (2020) 9:E453. doi: 10.3390/cells9020453
105. Tarng D-C, Tseng W-C, Lee P-Y, Chiou S-H, Hsieh S-L. Induced Pluripotent Stem Cell-Derived Conditioned Medium Attenuates Acute Kidney Injury by Downregulating the Oxidative Stress-Related Pathway in Ischemia-Reperfusion Rats. Cell Transplant (2016) 25:517–30. doi: 10.3727/096368915X688542
106. Duan P, Tan J, Miao Y, Zhang Q. Potential Role of Exosomes in the Pathophysiology, Diagnosis, and Treatment of Hypoxic Diseases. Am J Transl Res (2019) 11:1184–201.
107. Collino F, Lopes JA, Corrêa S, Abdelhay E, Takiya CM, Wendt CHC, et al. Adipose-Derived Mesenchymal Stromal Cells Under Hypoxia: Changes in Extracellular Vesicles Secretion and Improvement of Renal Recovery After Ischemic Injury. Cell Physiol Biochem (2019) 52:1463–83. doi: 10.33594/000000102
108. Lovisa S, LeBleu VS, Tampe B, Sugimoto H, Vadnagara K, Carstens JL, et al. Epithelial-To-Mesenchymal Transition Induces Cell Cycle Arrest and Parenchymal Damage in Renal Fibrosis. Nat Med (2015) 21:998–1009. doi: 10.1038/nm.3902
109. Han Y, Ren J, Bai Y, Pei X, Han Y. Exosomes From Hypoxia-Treated Human Adipose-Derived Mesenchymal Stem Cells Enhance Angiogenesis Through VEGF/VEGF-R. Int J Biochem Cell Biol (2019) 109:59–68. doi: 10.1016/j.biocel.2019.01.017
110. Shokeir AA, Hussein AM, Barakat N, Abdelaziz A, Elgarba M, Awadalla A. Activation of Nuclear Factor Erythroid 2-Related Factor 2 (Nrf2) and Nrf-2-Dependent Genes by Ischaemic Pre-Conditioning and Post-Conditioning: New Adaptive Endogenous Protective Responses Against Renal Ischaemia/Reperfusion Injury. Acta Physiol (Oxf) (2014) 210:342–53. doi: 10.1111/apha.12164
111. Su H, Lei C-T, Zhang C. Interleukin-6 Signaling Pathway and Its Role in Kidney Disease: An Update. Front Immunol (2017) 8:405. doi: 10.3389/fimmu.2017.00405
112. Andrade AC, Wolf M, Binder H-M, Gomes FG, Manstein F, Ebner-Peking P, et al. Hypoxic Conditions Promote the Angiogenic Potential of Human Induced Pluripotent Stem Cell-Derived Extracellular Vesicles. Int J Mol Sci (2021) 22:3890. doi: 10.3390/ijms22083890
113. Zhang W, Zhou X, Yao Q, Liu Y, Zhang H, Dong Z. HIF-1-Mediated Production of Exosomes During Hypoxia Is Protective in Renal Tubular Cells. Am J Physiol Renal Physiol (2017) 313:F906–13. doi: 10.1152/ajprenal.00178.2017
114. Zhang L, Liu H, Xu K, Ling Z, Huang Y, Hu Q, et al. Hypoxia Preconditioned Renal Tubular Epithelial Cell-Derived Extracellular Vesicles Alleviate Renal Ischaemia-Reperfusion Injury Mediated by the HIF-1α/Rab22 Pathway and Potentially Affected by microRNAs. Int J Biol Sci (2019) 15:1161–76. doi: 10.7150/ijbs.32004
115. Zhang Z-Y, Hou Y-P, Zou X-Y, Xing X-Y, Ju G-Q, Zhong L, et al. Oct-4 Enhanced the Therapeutic Effects of Mesenchymal Stem Cell-Derived Extracellular Vesicles in Acute Kidney Injury. Kidney Blood Press Res (2020) 45:95–108. doi: 10.1159/000504368
116. Liu Y, Cui J, Wang H, Hezam K, Zhao X, Huang H, et al. Enhanced Therapeutic Effects of MSC-Derived Extracellular Vesicles With an Injectable Collagen Matrix for Experimental Acute Kidney Injury Treatment. Stem Cell Res Ther (2020) 11:161. doi: 10.1186/s13287-020-01668-w
117. Humphreys BD, Cantaluppi V, Portilla D, Singbartl K, Yang L, Rosner MH, et al. Targeting Endogenous Repair Pathways After AKI. J Am Soc Nephrol (2016) 27:990–8. doi: 10.1681/ASN.2015030286
118. He Z, Wang H, Yue L. Endothelial Progenitor Cells-Secreted Extracellular Vesicles Containing microRNA-93-5p Confer Protection Against Sepsis-Induced Acute Kidney Injury via the KDM6B/H3K27me3/TNF-α Axis. Exp Cell Res (2020) 395:112173. doi: 10.1016/j.yexcr.2020.112173
119. Li H, Zhang X, Wang P, Zhou X, Liang H, Li C. Knockdown of Circ-FANCA Alleviates LPS-Induced HK2 Cell Injury via Targeting miR-93-5p/OXSR1 Axis in Septic Acute Kidney Injury. Diabetol Metab Syndr (2021) 13:7. doi: 10.1186/s13098-021-00625-8
120. You J, Fu Z, Zou L. Mechanism and Potential of Extracellular Vesicles Derived From Mesenchymal Stem Cells for the Treatment of Infectious Diseases. Front Microbiol (2021) 12:761338. doi: 10.3389/fmicb.2021.761338
121. Park K-S, Svennerholm K, Shelke GV, Bandeira E, Lässer C, Jang SC, et al. Mesenchymal Stromal Cell-Derived Nanovesicles Ameliorate Bacterial Outer Membrane Vesicle-Induced Sepsis via IL-10. Stem Cell Res Ther (2019) 10:231. doi: 10.1186/s13287-019-1352-4
122. Sun J, Sun X, Chen J, Liao X, He Y, Wang J, et al. microRNA-27b Shuttled by Mesenchymal Stem Cell-Derived Exosomes Prevents Sepsis by Targeting JMJD3 and Downregulating NF-κB Signaling Pathway. Stem Cell Res Ther (2021) 12:14. doi: 10.1186/s13287-020-02068-w
123. Bai X, Li J, Li L, Liu M, Liu Y, Cao M, et al. Extracellular Vesicles From Adipose Tissue-Derived Stem Cells Affect Notch-miR148a-3p Axis to Regulate Polarization of Macrophages and Alleviate Sepsis in Mice. Front Immunol (2020) 11:1391. doi: 10.3389/fimmu.2020.01391
124. Gao F, Zuo B, Wang Y, Li S, Yang J, Sun D. Protective Function of Exosomes From Adipose Tissue-Derived Mesenchymal Stem Cells in Acute Kidney Injury Through SIRT1 Pathway. Life Sci (2020) 255:117719. doi: 10.1016/j.lfs.2020.117719
125. Zhang R, Zhu Y, Li Y, Liu W, Yin L, Yin S, et al. Human Umbilical Cord Mesenchymal Stem Cell Exosomes Alleviate Sepsis-Associated Acute Kidney Injury via Regulating microRNA-146b Expression. Biotechnol Lett (2020) 42:669–79. doi: 10.1007/s10529-020-02831-2
126. Pan T, Jia P, Chen N, Fang Y, Liang Y, Guo M, et al. Delayed Remote Ischemic Preconditioning ConfersRenoprotection Against Septic Acute Kidney Injury via Exosomal miR-21. Theranostics (2019) 9:405–23. doi: 10.7150/thno.29832
127. Basile DP, Bonventre JV, Mehta R, Nangaku M, Unwin R, Rosner MH, et al. Progression After AKI: Understanding Maladaptive Repair Processes to Predict and Identify Therapeutic Treatments. J Am Soc Nephrol (2016) 27:687–97. doi: 10.1681/ASN.2015030309
128. Fiorentino M, Grandaliano G, Gesualdo L, Castellano G. Acute Kidney Injury to Chronic Kidney Disease Transition. Contrib Nephrol (2018) 193:45–54. doi: 10.1159/000484962
129. Andrade L, Rodrigues CE, Gomes SA, Noronha IL. Acute Kidney Injury as a Condition of Renal Senescence. Cell Transplant (2018) 27:739–53. doi: 10.1177/0963689717743512
130. Franzin R, Stasi A, Fiorentino M, Stallone G, Cantaluppi V, Gesualdo L, et al. Inflammaging and Complement System: A Link Between Acute Kidney Injury and Chronic Graft Damage. Front Immunol (2020) 11:734. doi: 10.3389/fimmu.2020.00734
131. Zhou W, Marsh JE, Sacks SH. Intrarenal Synthesis of Complement. Kidney Int (2001) 59:1227–35. doi: 10.1046/j.1523-1755.2001.0590041227.x
132. Brooimans RA, Stegmann AP, van Dorp WT, van der Ark AA, van der Woude FJ, van Es LA, et al. Interleukin 2 Mediates Stimulation of Complement C3 Biosynthesis in Human Proximal Tubular Epithelial Cells. J Clin Invest (1991) 88:379–84. doi: 10.1172/JCI115314
133. Sheerin NS, Zhou W, Adler S, Sacks SH. TNF-Alpha Regulation of C3 Gene Expression and Protein Biosynthesis in Rat Glomerular Endothelial Cells. Kidney Int (1997) 51:703–10. doi: 10.1038/ki.1997.101
134. Boudhabhay I, Poillerat V, Grunenwald A, Torset C, Leon J, Daugan MV, et al. Complement Activation Is a Crucial Driver of Acute Kidney Injury in Rhabdomyolysis. Kidney Int (2021) 99:581–97. doi: 10.1016/j.kint.2020.09.033
135. Messerer DAC, Halbgebauer R, Nilsson B, Pavenstädt H, Radermacher P, Huber-Lang M. Immunopathophysiology of Trauma-Related Acute Kidney Injury. Nat Rev Nephrol (2021) 17:91–111. doi: 10.1038/s41581-020-00344-9
136. Docherty M-H, O’Sullivan ED, Bonventre JV, Ferenbach DA. Cellular Senescence in the Kidney. J Am Soc Nephrol (2019) 30:726–36. doi: 10.1681/ASN.2018121251
137. Sturmlechner I, Durik M, Sieben CJ, Baker DJ, van Deursen JM. Cellular Senescence in Renal Ageing and Disease. Nat Rev Nephrol (2017) 13:77–89. doi: 10.1038/nrneph.2016.183
138. Valentijn FA, Falke LL, Nguyen TQ, Goldschmeding R. Cellular Senescence in the Aging and Diseased Kidney. J Cell Commun Signal (2018) 12:69–82. doi: 10.1007/s12079-017-0434-2
139. Glassock RJ, Rule AD. The Implications of Anatomical and Functional Changes of the Aging Kidney: With an Emphasis on the Glomeruli. Kidney Int (2012) 82:270–7. doi: 10.1038/ki.2012.65
140. Glassock RJ, Delanaye P, Rule AD. Should the Definition of CKD be Changed to Include Age-Adapted GFR Criteria? YES. Kidney Int (2020) 97:34–7. doi: 10.1016/j.kint.2019.08.033
141. Campisi J, d’Adda di Fagagna F. Cellular Senescence: When Bad Things Happen to Good Cells. Nat Rev Mol Cell Biol (2007) 8:729–40. doi: 10.1038/nrm2233
142. Wang W-J, Cai G-Y, Chen X-M. Cellular Senescence, Senescence-Associated Secretory Phenotype, and Chronic Kidney Disease. Oncotarget (2017) 8:64520–33. doi: 10.18632/oncotarget.17327
143. Franceschi C, Garagnani P, Parini P, Giuliani C, Santoro A. Inflammaging: A New Immune-Metabolic Viewpoint for Age-Related Diseases. Nat Rev Endocrinol (2018) 14:576–90. doi: 10.1038/s41574-018-0059-4
144. Kooman JP, Dekker MJ, Usvyat LA, Kotanko P, van der Sande FM, Schalkwijk CG, et al. Inflammation and Premature Aging in Advanced Chronic Kidney Disease. Am J Physiol Renal Physiol (2017) 313:F938–50. doi: 10.1152/ajprenal.00256.2017
145. Nitta K, Nagano N, Tsuchiya K. Fibroblast Growth Factor 23/Klotho Axis in Chronic Kidney Disease. Nephron Clin Pract (2014) 128:1–10. doi: 10.1159/000365787
146. Wang Q, Su W, Shen Z, Wang R. Correlation Between Soluble α-Klotho and Renal Function in Patients With Chronic Kidney Disease: A Review and Meta-Analysis. BioMed Res Int (2018) 2018:9481475. doi: 10.1155/2018/9481475
147. Kuro-o M, Matsumura Y, Aizawa H, Kawaguchi H, Suga T, Utsugi T, et al. Mutation of the Mouse Klotho Gene Leads to a Syndrome Resembling Ageing. Nature (1997) 390:45–51. doi: 10.1038/36285
148. Kuro-O M. The Klotho Proteins in Health and Disease. Nat Rev Nephrol (2019) 15:27–44. doi: 10.1038/s41581-018-0078-3
149. Castellano G, Intini A, Stasi A, Divella C, Gigante M, Pontrelli P, et al. Complement Modulation of Anti-Aging Factor Klotho in Ischemia/Reperfusion Injury and Delayed Graft Function. Am J Transplant (2016) 16:325–33. doi: 10.1111/ajt.13415
150. Curci C, Castellano G, Stasi A, Divella C, Loverre A, Gigante M, et al. Endothelial-To-Mesenchymal Transition and Renal Fibrosis in Ischaemia/Reperfusion Injury Are Mediated by Complement Anaphylatoxins and Akt Pathway. Nephrol Dial Transplant (2014) 29:799–808. doi: 10.1093/ndt/gft516
151. Stasi A, Intini A, Divella C, Franzin R, Montemurno E, Grandaliano G, et al. Emerging Role of Lipopolysaccharide Binding Protein in Sepsis-Induced Acute Kidney Injury. Nephrol Dial Transplant (2017) 32:24–31. doi: 10.1093/ndt/gfw250
152. Shaw I, Rider S, Mullins J, Hughes J, Péault B. Pericytes in the Renal Vasculature: Roles in Health and Disease. Nat Rev Nephrol (2018) 14:521–34. doi: 10.1038/s41581-018-0032-4
153. Castellano G, Franzin R, Stasi A, Divella C, Sallustio F, Pontrelli P, et al. Complement Activation During Ischemia/Reperfusion Injury Induces Pericyte-To-Myofibroblast Transdifferentiation Regulating Peritubular Capillary Lumen Reduction Through pERK Signaling. Front Immunol (2018) 9:1002. doi: 10.3389/fimmu.2018.01002
154. Castellano G, Stasi A, Franzin R, Sallustio F, Divella C, Spinelli A, et al. LPS-Binding Protein Modulates Acute Renal Fibrosis by Inducing Pericyte-To-Myofibroblast Trans-Differentiation Through TLR-4 Signaling. Int J Mol Sci (2019) 20(15):3682. doi: 10.3390/ijms20153682
155. Cui J, Wu X, Song Y, Chen Y, Wan J. Complement C3 Exacerbates Renal Interstitial Fibrosis by Facilitating the M1 Macrophage Phenotype in a Mouse Model of Unilateral Ureteral Obstruction. Am J Physiol Renal Physiol (2019) 317:F1171–82. doi: 10.1152/ajprenal.00165.2019
156. Bohlson SS, O’Conner SD, Hulsebus HJ, Ho M-M, Fraser DA. Complement, C1q, and C1q-Related Molecules Regulate Macrophage Polarization. Front Immunol (2014) 5:402. doi: 10.3389/fimmu.2014.00402
157. Ishani A, Xue JL, Himmelfarb J, Eggers PW, Kimmel PL, Molitoris BA, et al. Acute Kidney Injury Increases Risk of ESRD Among Elderly. J Am Soc Nephrol (2009) 20:223–8. doi: 10.1681/ASN.2007080837
158. Hu H, Hu S, Xu S, Gao Y, Zeng F, Shui H. miR-29b Regulates Ang II-Induced EMT of Rat Renal Tubular Epithelial Cells via Targeting PI3K/AKT Signaling Pathway. Int J Mol Med (2018) 42:453–60. doi: 10.3892/ijmm.2018.3579
159. Danobeitia JS, Ziemelis M, Ma X, Zitur LJ, Zens T, Chlebeck PJ, et al. Complement Inhibition Attenuates Acute Kidney Injury After Ischemia-Reperfusion and Limits Progression to Renal Fibrosis in Mice. PLoS One (2017) 12:e0183701. doi: 10.1371/journal.pone.0183701
160. Medica D, Franzin R, Stasi A, Castellano G, Migliori M, Panichi V, et al. Extracellular Vesicles Derived From Endothelial Progenitor Cells Protect Human Glomerular Endothelial Cells and Podocytes From Complement- and Cytokine-Mediated Injury. Cells (2021) 10:1675. doi: 10.3390/cells10071675
161. Zilberman-Itskovich S, Abu-Hamad R, Zarura R, Sova M, Hachmo Y, Stark M, et al. Human Mesenchymal Stromal Cells Ameliorate Complement Induced Inflammatory Cascade and Improve Renal Functions in a Rat Model of Ischemia-Reperfusion Induced Acute Kidney Injury. PLoS One (2019) 14:e0222354. doi: 10.1371/journal.pone.0222354
162. Tang T-T, Lv L-L, Lan H-Y, Liu B-C. Extracellular Vesicles: Opportunities and Challenges for the Treatment of Renal Diseases. Front Physiol (2019) 10:226. doi: 10.3389/fphys.2019.00226
163. Maumus M, Rozier P, Boulestreau J, Jorgensen C, Noël D. Mesenchymal Stem Cell-Derived Extracellular Vesicles: Opportunities and Challenges for Clinical Translation. Front Bioeng Biotechnol (2020) 8:997. doi: 10.3389/fbioe.2020.00997
164. Li Z-L, Lv L-L, Tang T-T, Wang B, Feng Y, Zhou L-T, et al. HIF-1α Inducing Exosomal microRNA-23a Expression Mediates the Cross-Talk Between Tubular Epithelial Cells and Macrophages in Tubulointerstitial Inflammation. Kidney Int (2019) 95:388–404. doi: 10.1016/j.kint.2018.09.013
165. Kamaly N, He JC, Ausiello DA, Farokhzad OC. Nanomedicines for Renal Disease: Current Status and Future Applications. Nat Rev Nephrol (2016) 12:738–53. doi: 10.1038/nrneph.2016.156
166. Lee JH, Ha DH, Go H-K, Youn J, Kim H-K, Jin RC, et al. Reproducible Large-Scale Isolation of Exosomes From Adipose Tissue-Derived Mesenchymal Stem/Stromal Cells and Their Application in Acute Kidney Injury. Int J Mol Sci (2020) 21:E4774. doi: 10.3390/ijms21134774
167. Duong N, Curley K, Brown A, Campanelli A, Do MA, Levy D, et al. Decoy Exosomes as a Novel Biologic Reagent to Antagonize Inflammation. Int J Nanomedicine (2019) 14:3413–25. doi: 10.2147/IJN.S196975
168. Tapparo M, Bruno S, Collino F, Togliatto G, Deregibus MC, Provero P, et al. Renal Regenerative Potential of Extracellular Vesicles Derived From miRNA-Engineered Mesenchymal Stromal Cells. Int J Mol Sci (2019) 20(10):2381. doi: 10.3390/ijms20102381
169. Ullah M, Liu DD, Rai S, Concepcion W, Thakor AS. HSP70-Mediated NLRP3 Inflammasome Suppression Underlies Reversal of Acute Kidney Injury Following Extracellular Vesicle and Focused Ultrasound Combination Therapy. Int J Mol Sci (2020) 21:E4085. doi: 10.3390/ijms21114085
170. Zhang X, Wang N, Huang Y, Li Y, Li G, Lin Y, et al. Extracellular Vesicles From Three Dimensional Culture of Human Placental Mesenchymal Stem Cells Ameliorated Renal Ischemia/Reperfusion Injury. Int J Artif Organs (2021) 45(2):181–92. doi: 10.1177/0391398820986809
171. Kouroupis D, Correa D. Increased Mesenchymal Stem Cell Functionalization in Three-Dimensional Manufacturing Settings for Enhanced Therapeutic Applications. Front Bioeng Biotechnol (2021) 9:621748. doi: 10.3389/fbioe.2021.621748
172. Cao J, Wang B, Tang T, Lv L, Ding Z, Li Z, et al. Three-Dimensional Culture of MSCs Produces Exosomes With Improved Yield and Enhanced Therapeutic Efficacy for Cisplatin-Induced Acute Kidney Injury. Stem Cell Res Ther (2020) 11:206. doi: 10.1186/s13287-020-01719-2
Keywords: acute kidney injury, acute tubular necrosis, ischemia-reperfusion injury, sepsis, chronic kidney disease, stem cell, extracellular vesicles, regenerative medicine
Citation: Quaglia M, Merlotti G, Colombatto A, Bruno S, Stasi A, Franzin R, Castellano G, Grossini E, Fanelli V and Cantaluppi V (2022) Stem Cell-Derived Extracellular Vesicles as Potential Therapeutic Approach for Acute Kidney Injury. Front. Immunol. 13:849891. doi: 10.3389/fimmu.2022.849891
Received: 06 January 2022; Accepted: 15 February 2022;
Published: 10 March 2022.
Edited by:
Perenlei Enkhbaatar, University of Texas Medical Branch at Galveston, United StatesReviewed by:
Bruno Azzarone, IRCCS Bambino Gesù Children’s Hospital, ItalyDavid Peter Al-Adra, Wisconsin Medical Society, United States
Copyright © 2022 Quaglia, Merlotti, Colombatto, Bruno, Stasi, Franzin, Castellano, Grossini, Fanelli and Cantaluppi. This is an open-access article distributed under the terms of the Creative Commons Attribution License (CC BY). The use, distribution or reproduction in other forums is permitted, provided the original author(s) and the copyright owner(s) are credited and that the original publication in this journal is cited, in accordance with accepted academic practice. No use, distribution or reproduction is permitted which does not comply with these terms.
*Correspondence: Vincenzo Cantaluppi, dmluY2Vuem8uY2FudGFsdXBwaUBtZWQudW5pdXBvLml0