- 1Nephrology, Department of Internal Medicine, Leiden University Medical Centre, Leiden, Netherlands
- 2Transplant Centre of the Leiden University Medical Centre, Leiden University Medical Centre, Leiden, Netherlands
- 3Nuffield Department of Surgical Sciences, University of Oxford, Oxford, United Kingdom
Hypothermic machine perfusion (HMP) has become the new gold standard in clinical donor kidney preservation and a promising novel strategy in higher risk donor livers in several countries. As shown by meta-analysis for the kidney, HMP decreases the risk of delayed graft function (DGF) and improves graft survival. For the liver, HMP immediately prior to transplantation may reduce the chance of early allograft dysfunction (EAD) and reduce ischemic sequelae in the biliary tract. Ischemia-reperfusion injury (IRI), unavoidable during transplantation, can lead to massive cell death and is one of the main causes for DGF, EAD or longer term impact. Molecular mechanisms that are affected in IRI include levels of hypoxia inducible factor (HIF), induction of cell death, endothelial dysfunction and immune responses. In this review we have summarized and discussed mechanisms on how HMP can ameliorate IRI. Better insight into how HMP influences IRI in kidney and liver transplantation may lead to new therapies and improved transplant outcomes.
Introduction
Several countries use hypothermic machine perfusion (HMP) as standard preservation method for kidney transplantation instead of storing the organ on ice. It has been shown by several clinical studies that HMP is superior to static cold storage (SCS), but the underlying mechanisms are still unknown. Ischemia-reperfusion injury (IRI) is unavoidable during transplantation and is one of the main causes for delayed graft function (DGF) (1). Since HMP is known to affect DGF, it is possible that this positive effect is due to its influence on IRI. To gain a better insight into the effect of HMP on ameliorating IRI. In this review we evaluated the effect of HMP on four main aspects that are involved in the pathophysiology of IRI to gain a better insight into the effect of HMP on ameliorating IRI. This was done by analysing studies comparing static cold storage with HMP.
History of Machine Perfusion
Since the early beginning of solid organ transplantation, cold preservation has been the gold standard in kidney preservation. Lowering the temperature of the donor organ below 10°C decreases metabolism by approximately 90%, allowing maintenance of donor organ viability and safe preservation. Initially kidneys were cold perfused on a machine, but when better organ preservation solutions were developed, simple SCS in a box with melting ice became the standard in organ preservation due to its simple and effective way to transport the graft. In the past decades, due to the persistent donor kidney shortage, most centres have increasingly been accepting older and higher risk donor kidneys. These kidneys are from donation after circulatory death (DCD) donors or from donors with increased co-morbidity, i.e. hypertension, diabetes or atherosclerotic disease (2). This change of practice often resulted in compromised function and lower graft survival. Also, it became clear that the conventional method of SCS did not suffice for this type of donor organs and improved strategies in preservation appeared to be necessary. This insight resulted in a revisit of continuous machine perfusion, now using novel technologies in medical engineering including oxygenation, both hypo- and normothermic temperatures and modifications of perfusion solutions. Following initial clinical trials demonstrating better outcomes for higher risk donor kidneys (3), several countries have now implemented HMP as the preferred preservation method. In 2009, the first study was published reporting on the results of an international randomized controlled trial with a paired kidney design. One kidney was preserved with HMP whilst for the contralateral kidney SCS was used. In this trial, the method of HMP overall reduced DGF from 26.5% to 20.8% and increased 1 year graft survival from 90% to 94% (4). When analyzing the different subgroups of donor types (ECD, donation after brain death (DBD), DCD), HMP remained superior in decreasing DGF. Subsequent clinical studies and registry reports have been performed and confirmed the beneficial effect of HMP over SCS. Meta-analysis showed that HMP reduced DGF [relative risk (RR) 0.81 with 0.71-0.92 95% confidence interval (CI)] in all deceased donor types in kidney transplantation (DBD fixed-effects analysis with RR 0.84 with 0.69-1.03 95% CI and DCD random-effects analysis with RR of 0.80 with 0.62-1.04 95% CI) (5). A more recent meta-analysis showed a reduced risk of delayed graft function when kidneys were preserved with HMP versus SCS (RR 0.77, 95% CI 0.67-0.90) as well as a trend towards improved graft survival (6). HMP was also shown to be cost effective, because lower DGF and graft failure rates decreased the need for a return to (chronic) dialysis. Beneficial effects of HMP were also shown in a meta-analysis for the liver, by a lower incidence of early allograft dysfunction (EAD), less biliary complications and ischemic cholangiopathy, as well as lower aspartate aminotransferase levels (7). Although short term outcomes in kidney transplantation have improved over the last decades, long term outcomes are still moderate with 70% of DBD and DCD grafts failing between 5 and 10 years posttransplant (8). Two important factors that influence long term outcome in kidney transplantation are the quality of the graft and the immunosuppression administered (9). HMP may potentially alter immunogenicity of the donor organ, thereby improving the quality of the graft. Also, HMP offers a great platform for therapeutic options to potentially reduce the amount of immune suppressive drugs.
Ischemia-Reperfusion Injury
Ischemia followed by reperfusion is inevitable in the context of organ transplantation. The temporary cut-off of the donor organ from the blood supply at the time of procurement until the recipient operation will cause hypoxia, leading to inhibition of the electron transport chain and subsequent lower ATP production. Lower ATP levels will cause a shift toward anaerobic metabolism with dysfunction of sodium-potassium, calcium and sodium-hydrogen pumps. This will result in an imbalance in cellular osmolarity, with as a consequence cell swelling. Anaerobic metabolism also leads to metabolic acidosis from the increased lactic acid levels; decrease of antioxidative agents; and detachment of ribosomes resulting in less protein synthesis. During reperfusion of the graft, there will be a second wave of injury, further damaging the graft, including the release of reactive oxygen species (ROS). The lower levels of antioxidants are unable to neutralize the ROS, leading to cell death. Subsequently, the innate immune system is activated by the profound release of damage-associated molecular patterns (DAMPs) from dying cells. A more detailed review about IRI can be found elsewhere (10). In this review IRI is divided into three main phases: hypoxia, reoxygenation and reperfusion. Although all three phases can be characterized by distinct molecular mechanisms, they are also closely interrelated (Figure 1).
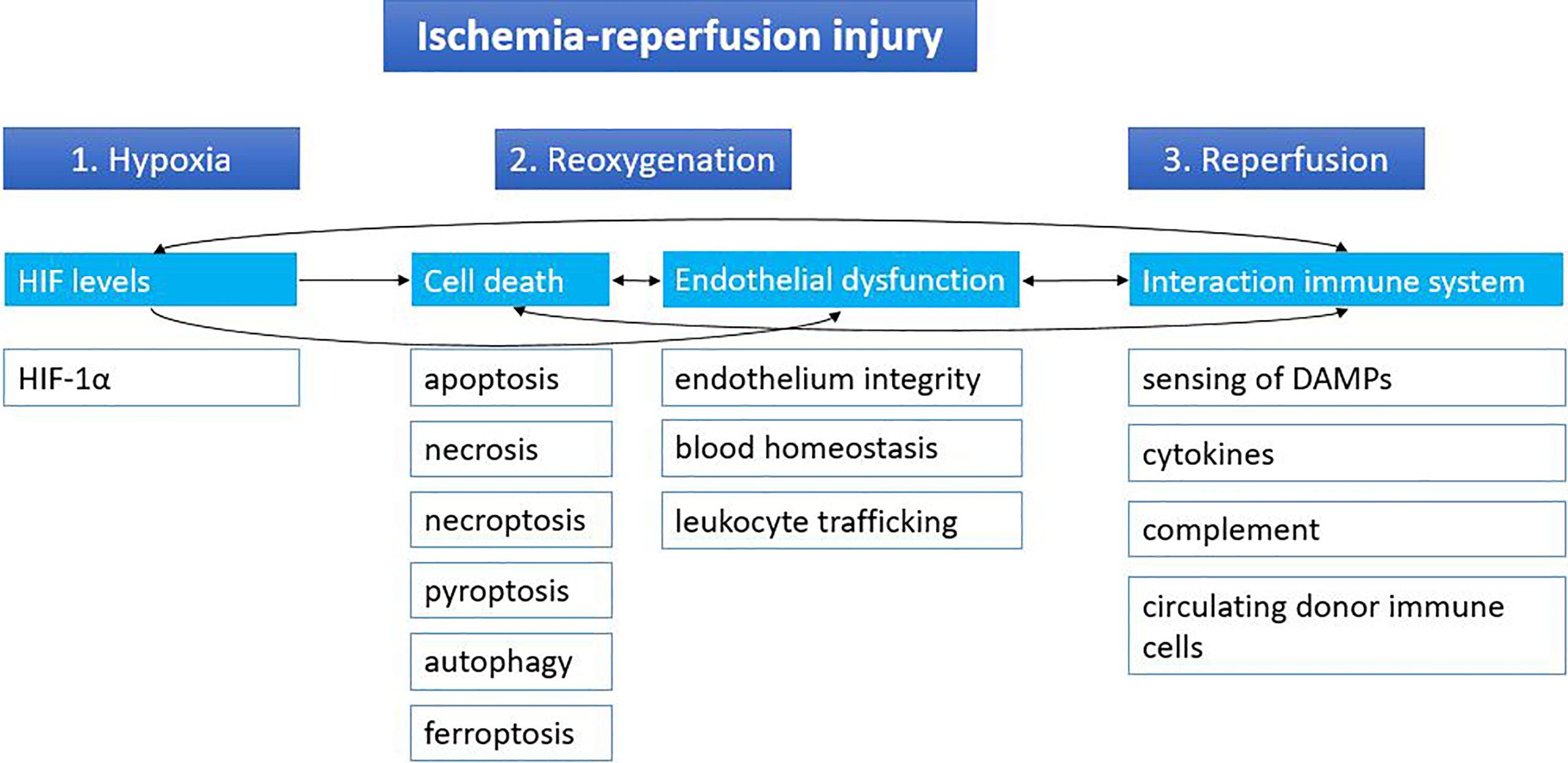
Figure 1 Stages of ischemia-reperfusion injury. Ischemia-reperfusion injury can be divided into three stages based on distinct molecular mechanisms: hypoxia, reoxygenation and reperfusion. Cut-off of the donor organ leads to hypoxia, which leads to changes in HIF levels. During reoxygenation, reactive oxygen species lead to massive cell death and endothelial dysfunction. When the graft is reperfused in the recipient, the immune system of the recipient can react to the graft and its immune cells and vice versa. Arrows indicate the relations between the different mechanisms. DAMPs, damage-associated molecular patterns; HIF, hypoxia inducible factor.
Influence on Hypoxia Inducible Factor Levels
The first phase of ischemia-reperfusion injury is hypoxia. When oxygen levels are normal, hypoxia inducible factor (HIF) α proteins (HIF-1α, HIF-2α) are rapidly produced, but also degraded. Prolyl hydroxylases (PHD) enzymes use oxygen as a cofactor to mark HIF - α proteins for degradation by the proteasome (Figure 2). During hypoxia, PHD enzymes are no longer able to trigger the break down, resulting in increasing HIF-α levels. HIF-α translocates to the nucleus where it binds to the HIF-1β subunit. Together the HIF protein binds to HIF response elements on the DNA, thereby influencing many genes that regulate angiogenesis, metabolism, cell growth and survival (11, 12).
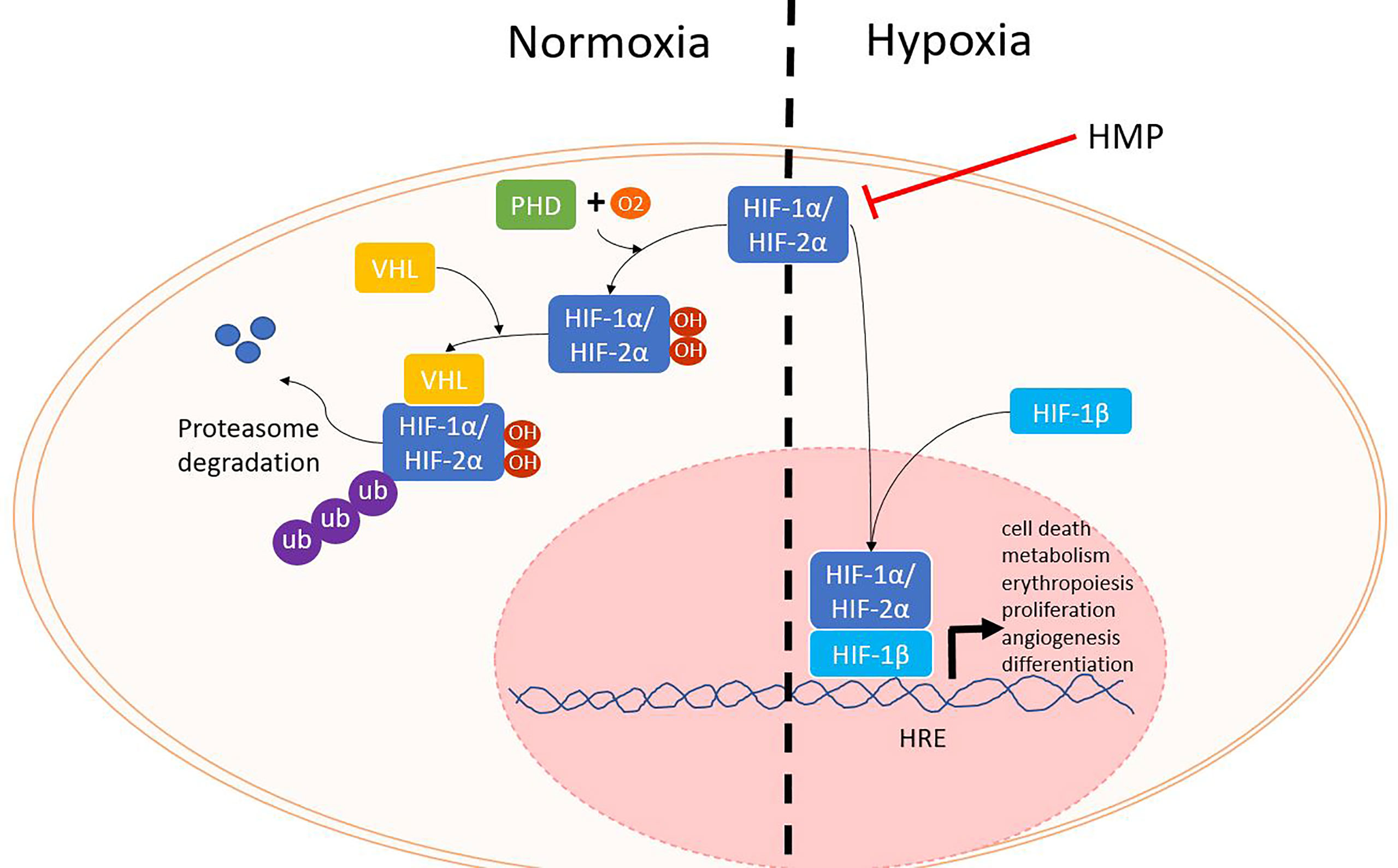
Figure 2 HIF proteins during normoxia and hypoxia. When oxygen levels are sufficient, PHD enzymes use oxygen as a cofactor to hydroxylate HIF-α. Next, VHL will mark HIF-α for degradation by the proteasome. During hypoxia, HIF-α is not degraded and can translocate with HIF-1β to the nucleus where it can bind to HRE and lead to the activation of several different genes. HIF, hypoxia inducible factor; HRE, HIF response element; PHD, prolyl hydroxylase; VHL, von Hippel Lindau.
Most studies reported lower levels of HIF proteins (HIF-1α, HIF-2α, HIF-3α and HIF-1β) in HMP-stored donor organs compared with SCS (13–18). Besides lower levels of HIF proteins, improvements on transplant outcome (less damage, better kidney function) were also observed when HMP was applied. However, there is a lot of variability between these studies. Studies were performed with canine, porcine or human organs with HMP duration varying between 3 and 24h. Organs that were subjected to HMP or SCS were liver, limb or kidney. Also, two studies were found where higher levels of HIF-1α and HIF-2α (and downstream factor vascular endothelial growth factor A) were found in HMP perfused human livers. Burlage et al. (19) showed in a human liver model better endothelial cell function with increased HIF-1α levels as measured by Krüppel-like factor 2 (Klf2), endothelial nitric oxide synthase (eNOS), nitric oxide (NO) and thrombomodulin (19). Ito et al. showed in a human liver model that HIF-1α expression increased after HMP (20). They also analysed HIF-1α levels in clinical liver patients and found that higher levels were associated with significantly better graft survival. Unfortunately, to date only an abstract is available of this study, so details about this study are lacking. HIF upregulation that occurs during hypoxia can be explained as a protective mechanism to better handle the ischemic injury (20–23). Studies reporting lower levels of HIF proteins focus more on the hypoxia part, suggesting that lower levels of HIF mean less hypoxia. The two studies reporting higher HIF levels can be compared at best with the studies of Guarrera et al. (17) and Henry et al. (18) that also use a human liver model. Unfortunately, the first study only provides an abstract and does not include the duration of HMP, making it difficult to compare with other studies. The study of Henry et al. provided both clinical outcomes (beneficial for HMP with a shorter hospital stay and a trend towards less EAD and analysis of biopsies and serum (less oxidative stress, inflammatory markers, adhesion molecules and cellular infiltration in the HMP group). Taking along the previously mentioned studies that reported lower levels of HIF, it is likely that HMP reduces the level of HIF proteins despite the great heterogeneity between studies. Nevertheless, these results illustrate the complexity of interpreting data on HIF levels, next to the different molecular consequences of HIF.
As mentioned before, HIF can regulate expression levels of many different genes. Those gene products can have contradictory effects, making it difficult to predict the effect in changing HIF levels. For example, HIF activation can lead to upregulation of both pro- and anti-apoptotic genes. A study by Ravall et al. showed that in a renal cell carcinoma cell line there were opposing effects of HIF-1α and HIF-2α (24). When HIF-1α was expressed, HIF-2α was suppressed and tumour growth was inhibited. When HIF-2α was expressed, HIF-1α was suppressed and tumour growth was increased.. HIF-1α regulates many glycolytic enzymes and is expressed in many different tissues whereas HIF-2α regulates more broadly hypoxia inducible genes like cyclin D, transforming growth factor (TGF)-α and matrix metalloprotease 2 and its expression is more regulated (25, 26). The HIF-3α unit is less studied, but it is thought to inhibit HIF-1α and HIF-2α (26). The expression of the different HIF proteins can vary between different tissues, which could result in different hypoxia responses. In the kidney HIF-1α is expressed in the tubular epithelium, while HIF-2α is expressed in glomerular cells and peritubular interstitial cells (27, 28). HIF-2 has been associated with regulating erythropoietin synthesis (29). Kapitsinou et al. showed in a mouse model that HIF-2α endothelial inactivation resulted in increased expression of cellular infiltration and renal injury markers (27). This was associated with elevated Vcam1 expression. HIF-2 appears to protect against IRI and could therefore be a potential therapeutic target.
As stated earlier, the different phases of IRI are overlapping. HIF activation has been linked to increased thrombotic factors (30), fibrosis (31) and activating the innate immune cells (32). A rat study showed the effects of changing HIF levels on cell death and inflammation in a hepatic IRI model (33). Overexpression of HIF-1α lead to a protective effect by reducing necrosis, apoptosis, neutrophil infiltration and inflammatory cytokines IL-6 and TNF-α. Inhibition of HIF-1α had the opposite effect and aggravated the IRI injury. These results show the diversity of effects of changing HIF levels, where the protective effect was seen with increasing levels, whereas HMP mainly showed that a decrease lead to better outcomes. Future studies with a paired kidney design, where one kidney is put on HMP while the other is preserved SCS, both with similar cold ischemia times, could provide better insight into changing HIF levels.
Influence on Cell Death
During the second phase of IRI the reoxygenation leads to the release of ROS, leading to massive cell death. There are different forms of cell death (i.e. necroptosis, pyroptosis, autophagy), but all result in the release of DAMPs that can activate an immune response. However, different forms of cell death could still lead to different DAMPs being released, leading to a different immune response. Apoptosis is generally considered not to activate the immune system. Macrophages that engulf apoptotic cells are stimulated to secrete anti-inflammatory TGF-β and IL-10 (34). Nevertheless, DAMPs can still be released during this form of regulated cell death, as macrophages can also be stimulated to secrete high mobility group box 1 (HMGB1), a well-known DAMP (35, 36). Also, the release during apoptosis of oxidized mitochondrial DNA has been shown to initiate an immune response by activating the nucleotide binding domain and leucine rich repeat (NLR) pyrin domain containing 3 (NLRP3) (37). Necrosis - on the other hand - is also a form of cell death where many DAMPs are released. Necrosis is characterized by organ swelling and membrane rupture, leading to release of the cellular content (10). When comparing the effects of HMP with SCS, the amount of cell death by apoptosis and necrosis was reduced in HMP (Figure 3) (38–45). Less cell death most likely translates to less release of DAMPs, leading to less activation of the immune system. Therefore this could contribute to better outcomes with hypothermic machine perfused organs versus static cold stored ones. The ratio of anti-apoptotic Bcl2/pro-apoptotic Bax increased when organs were perfused with HMP versus SCS (41, 42). A higher Bcl2/Bax ratio will prevent the occurrence of apoptosis (46). Apoptosis is initiated from an extrinsic pathway that activates caspase 8 and 10 or via an intrinsic pathway activating caspase 9. Once activated, the cascade of caspases leads to a regulated dismantling of the cell. Caspases also play a role in inflammation by recognizing bacterial products such as lipopolysaccharide, leading to pyroptosis and activating pro-inflammatory cytokines by cleavage. It has been suggested that caspase 4 and 5 can directly recognize lipopolysaccharide in the cytosol (47). Caspase 3 has been found to be downregulated after reperfusion while caspase 12 was found upregulated when organs were perfused with HMP versus SCS (18, 38–40, 42, 48–51). Caspase 3 is an essential effector caspase that plays a role in both the intrinsic and extrinsic pathway. Caspase 12, on the contrary, is known as a negative regulator of inflammation by inhibition of caspase 1, which is responsible for cleavage of pro-IL-1β and IL-18 to their active form (47, 52).
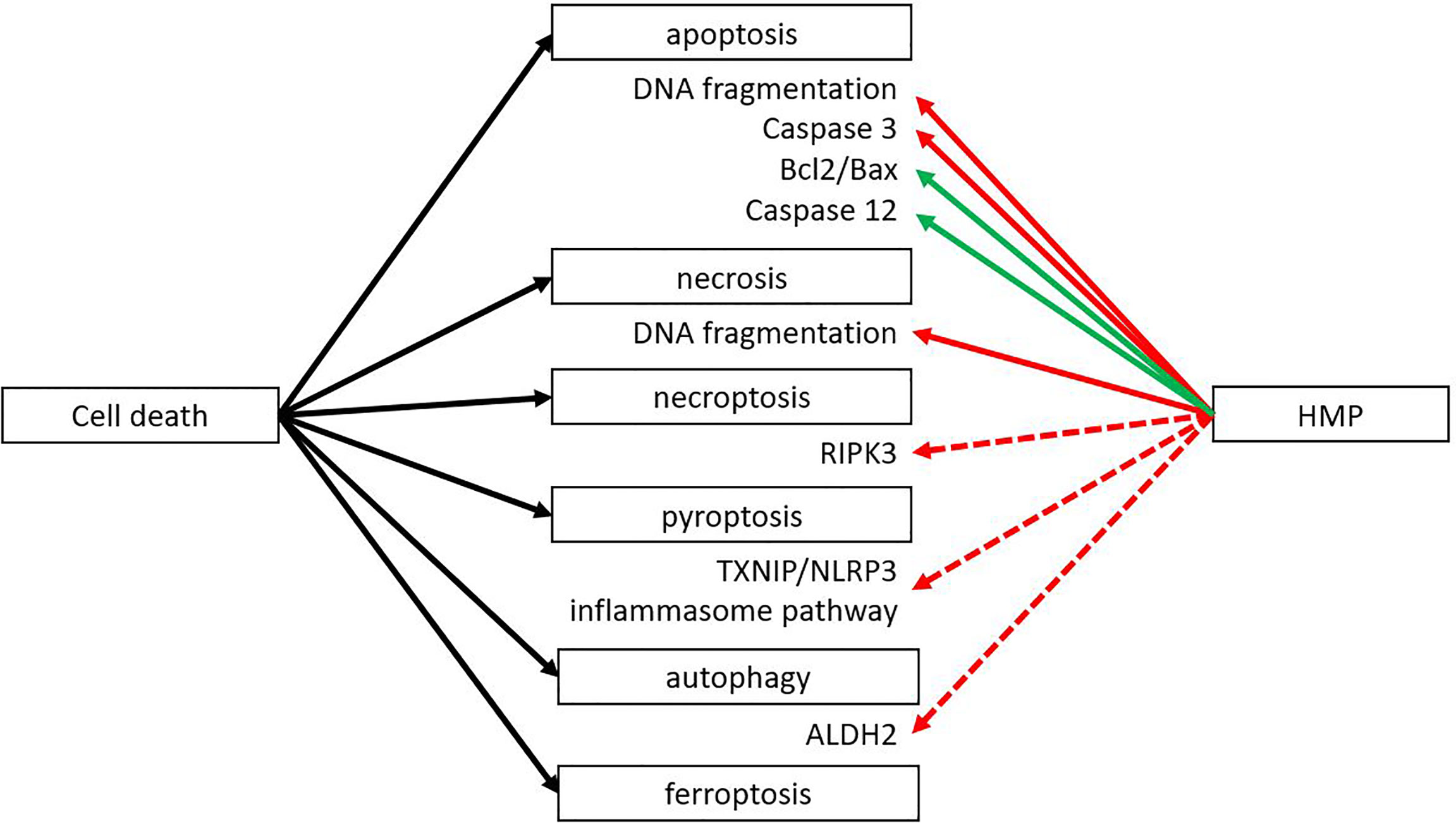
Figure 3 HMP reduces several forms of cell death. Different forms of cell death can occur after ischemia-reperfusion injury. HMP has been found to have an inhibiting effect on apoptosis and necrosis at the end of machine perfusion and after reperfusion in a NMP setting or autotransplantation (red line). The inhibiting effect on apoptosis is via inhibition of pro-apoptotic processes and increase of anti-apoptotic factors (green line). There are studies suggesting HMP could also have an inhibiting effect on necroptosis, pyroptosis and autophagy, but more research is needed (red dotted line). The effect of HMP on ferroptosis is yet unclear. ALDH2, aldehyde dehydrogenase; RIPK, receptor-interacting protein kinase; TXNIP/NLRP3, thioredoxin-interacting protein/NOD-like receptor protein.
Most research about the effect of HMP on cell death is focused on apoptosis and necrosis. Other forms of cell death such as necroptosis, pyroptosis or autophagy have not yet been studied extensively, although it is known that different forms of cell death may activate different immune pathways. Necroptosis has been associated with IRI in liver transplantation (53), ischemic brain injury (54) and renal IRI (55, 56). A study performed in rabbit kidneys showed that the expression of receptor-interacting protein kinase 3 (RIPK3) was significantly lower in the HMP group versus the SCS group both at the protein and mRNA level (51). The RIPK1-RIPK3 complex is an important regulator of necroptosis by forming the necroptosis-inducing complex called the necrosome (57).
The influence of HMP on pyroptosis has been investigated in liver perfusion in a rat study (58). The investigators studied the effect of hypothermic oxygenated machine perfusion (HOPE) following a period of SCS on the inflammasome pathway that plays a role in pyroptosis. The HOPE group showed less pyroptosis, likely by blocking the interaction between thioredoxin-interacting protein and NLRP3. The role of decreasing pyroptosis on transplant outcome was studied by Noda et al. in lung perfusion. They showed that lung perfusion of rat heart-lung blocks showed significantly better lung function and lower IL-6 levels when perfused with a leukocyte filter (59). Leukocytes trapped in the filter were analysed for cell death and they found that 26% of the cells were pyroptotic (caspase 1 positive, 7AAD negative), 16% apoptotic (Annexin V positive, 7AAD negative) and 40% necrotic (Annexin V positive, 7AAD positive). Administering a caspase-1 inhibitor during perfusion to inhibit pyroptosis showed better lung function and lower mRNA levels of proinflammatory cytokines IL-6, TNF-α and IL-1β, comparable with perfusion with a leukocyte filter. A limitation of this study is that only mRNA levels of cytokines were measured instead of protein levels. Caspase 1 plays an important role in activation and secretion of IL-1β and has also been linked to TNF-α and IL-6 secretion from macrophages (60).Therefore, it may be of interest to study whether the effects of blocking pyroptosis are similar on the protein level as found to be on the mRNA level.
The role of autophagy during IRI has also been studied in various organs (61–63). According to van Erp et al. the degree of autophagy in the donor can also be influenced by age and gender (64). During the first ischemic phase, it is proposed that autophagy acts as a protection mechanism whereas during the reperfusion stage excessive autophagy results in cell death (65). A study in rabbits showed higher levels of phosphorylated aldehyde dehydrogenase 2 (ALDH2) in HMP-perfused kidneys versus SCS (63). ALDH2 influences autophagy via expression of 4-HNE that regulates the Akt/mTOR autophagy pathway, suggesting that under HMP conditions, autophagy increases when compared to SCS. Administering an ALDH2 agonist to enhance autophagy resulted in (i) better kidney function as shown by lower serum creatinine, (ii) lower oxidative stress levels measured by malonaldehyde and superoxide dismutase 2, and (iii) better inflammatory profile as demonstrated by lower levels of TNF-α, IL-6 and higher levels of IL-10. Administering an antagonist, to decrease autophagy, lead to the opposite results. A study by Zeng et al. looked into the role of HOPE in upregulating autophagy to alleviate liver IRI in a rat model (44). HOPE increased expression of autophagy-related proteins and was associated with better liver function as measured by alanine transaminase, aspartate transaminase and lactate dehydrogenase compared with SCS. Administration of the autophagy inhibitor 3-MA attenuated the protective effect. Perfusion with 100% nitrogen showed similar results as under SCS conditions, indicating that the effect of HOPE treatment was not due to a washout during perfusion. It also demonstrated that a minimal oxygen level is required for the protective effect of HMP. According to Boteon et al. autophagy is important for removal of harmful substances and providing energy during cell stress (66). Normothermic machine perfusion (NMP) has been suggested to increase autophagy by maintaining normal calcium levels and by providing shear stress. Although more research is needed to evaluate the effect of HMP on autophagy, it is likely similar to NMP. During HMP the perfusate contains calcium and there are also low levels of shear stress detectable.
Another form of cell death is ferroptosis, which is iron-dependent. Excessive amounts of iron can lead to the generation of ROS through the Fenton reaction (67). Several studies showed that ferroptosis could play a role in IRI in the liver and kidney (68–70). Ferroptosis was also shown in several cell lines exposed to continuous cold stress, therefore it could be interesting to look into the role of ferroptosis during machine perfusion at subnormothermic (25-35°C) or normothermic (37°C) temperatures as well (71). Currently, no studies have looked into the role of HMP on ferroptosis.
Most studies use a TUNEL (Terminal deoxynucleotidyl transferase dUTP nick end labelling) assay to measure apoptosis via detection of DNA fragmentation. However, this assay can also detect necrosis, pyroptosis and possibly other forms of cell death (72–74). Knowing which forms of cell death are affected and which are not, is also important to identify specific targets to reduce IRI. A combination of multiple forms of cell death could also be possible. Recently, a new protein complex was identified as the PANoptosome (75). It drives the three main forms of programmed cell death, namely pyroptosis, apoptosis and necroptosis. The PANoptosome contains RIPK1, caspase 8, NLRP3 and apoptosis-associated speck-like protein (ASC) containing a caspase recruitment domain. Therefore, it contains molecules that are critical for programmed cell death. The different pathways can be activated together or separately and crosstalk between pathways occurs (75, 76).
In conclusion, it appears that HMP reduces the amount of cell death by decreasing apoptosis and necrosis. It is possible that HMP also reduces other forms of cell death like necroptosis, autophagy, pyroptosis and ferroptosis, but so far not many studies have looked into this. The effect of HMP on cell death will likely affect other mechanisms as well. Cell death plays an important role in activating the innate immune system via the release of DAMPs and caspases are critical in cleaving several cytokines into their active form. If cell death occurs in endothelial cells, this will disrupt the glycocalyx.
Influence on Endothelial Dysfunction
The endothelium plays a major role in inflammation with leukocyte adhesion and vascular health. Multiple studies have shown that IRI leads to the upregulation of adhesion molecules like E-cadherin and intercellular adhesion molecule 1 (ICAM-1) that enable leukocyte adhesion and neutrophil infiltration (77–79). Following HMP and reperfusion, many studies showed better endothelial function as measured by less transmigration of immune cells (Figure 4). In addition, less fibrosis after kidney preservation was measured by Sirius Red staining as well as by lower levels of pro-fibrotic transforming growth factor β (39, 80–82). A porcine model was used to study the effects of HMP on fibrosis, with the exception of Liu et al. (82) who used a rabbit model. Fibrosis is significantly associated with chronic graft dysfunction (11). Endothelial dysfunction has been mentioned as an important initiator and maintainer of fibrosis (83, 84). Therefore, we suggest that the level of fibrosis may indicate endothelial dysfunction. P-selectin is important for the rolling of leukocytes to ultimately invade the tissue and ICAM-1 is important for adhesion of leukocytes to endothelial cells. Lower expression of P-selectin and ICAM-1 were observed when HMP was applied versus SCS (13, 17, 18, 43, 49, 85). Reduced leukocyte rolling and adhesion with HMP was shown in liver models in rat (49), mouse (43), dog (13) and human (17, 18, 85). Most studies showed lower expression of P-selectin and ICAM-1 after perfusion (13, 17, 18, 85) or reperfusion (13, 43, 49), while Henry et al. (18) also showed a decrease in P-selectin levels after transplantation. Less cellular infiltration was also shown by a reduction in invading neutrophils and monocytes as measured by myeloperoxidase, CD68, Ly6G and reduced levels of monocyte chemoattractant protein-1 (18, 43, 80, 86, 87). A reduction in cellular infiltration was seen across various organisms (mouse, rabbit, human, pig) after perfusion, in reperfusion models and after transplantation. This was supported by a decrease in chemokines CXCL14 and IL-8 as shown after perfusion in a canine or human liver (13, 18, 85). Henry et al. (18) showed both after perfusion and transplantation an downregulation of CXCL14 and IL-8. Endothelial-to-mesenchymal transition (EMT) was observed less frequently as measured by lower levels of EMT marker vimentin after perfusion of porcine kidneys (80, 81). EMT is an important factor that contributes to fibrosis and chronic graft failure (88). A downregulation of the thrombotic von Willebrand-factor was also observed after reperfusion in liver transplantation in mice or pigs (44, 45). The vascular tone of the endothelium is important for sufficient flow. Vasodilation can be regulated by eNOS phosphorylation, leading to an increase in NO levels, which were found to be upregulated after reperfusion of HMP-treated organs (19, 89–91). This was true for both kidneys and livers and in different organisms (pig, rat, human). For the porcine kidney transplantation, higher levels of eNOS were also found after transplantation (90). In conclusion, a beneficial effect of HMP on endothelial integrity and function has been demonstrated at different levels.
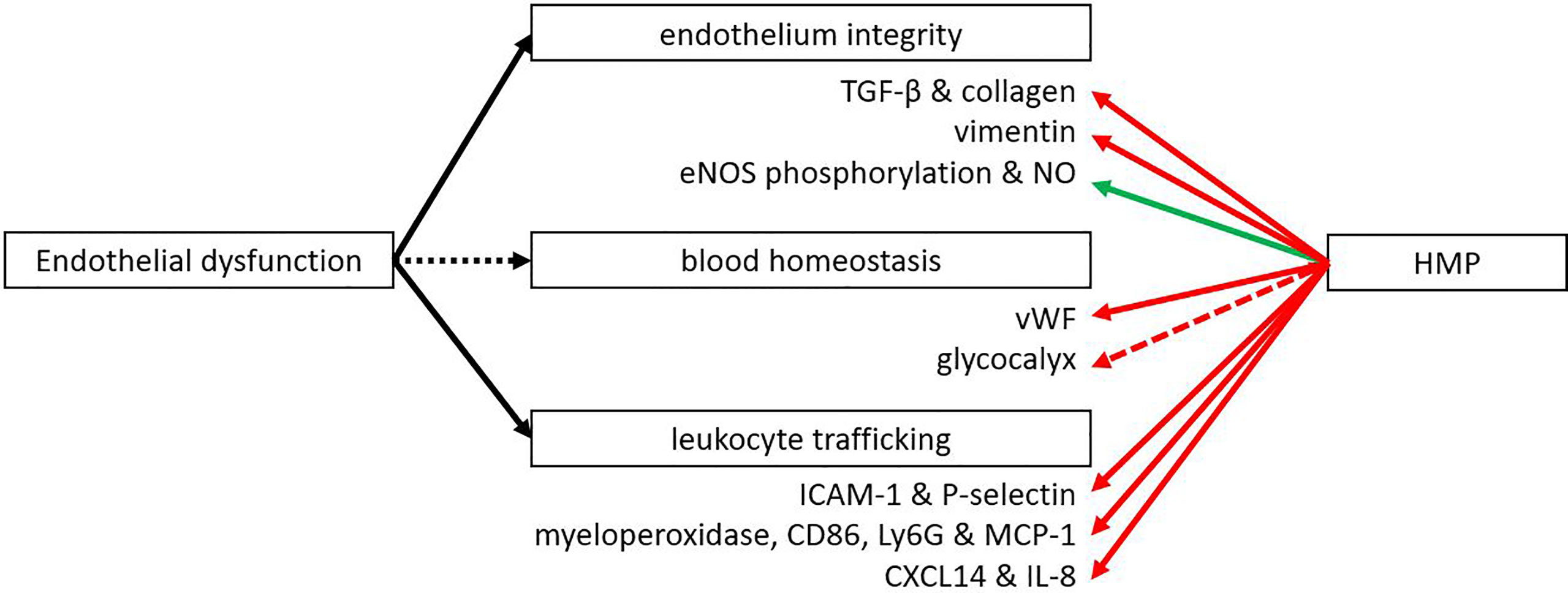
Figure 4 HMP influences several functions of the endothelium. After HMP or reperfusion (NMP or autotransplantation) there is less leukocyte trafficking compared to SCS treated organs (red line). Also less thrombotic factors, fibrosis and EMT were observed. However, the vascular tone was improved when organs were treated with HMP instead of SCS (green line). More studies are needed to confirm the effect of HMP on the glycocalyx (red dotted line). eNOS, endothelial nitric oxide synthase; ICAM, intercellular adhesion molecule; Ly6G, lymphocyte antigen 6 complex locus G6D; MCP-1, monocyte chemoattractant protein-1; NO, nitric oxide; TGF-β, transforming growth factor β; vWF, von Willebrand factor.
Many of these beneficial effects on the endothelium are most likely caused by the activation of Klf2, which gets activated upon flow-mediated shear stress. Besides regulation by flow, Klf2 can also be negatively regulated by proinflammatory cytokines such as TNF-α and H2O2 resulting from oxidative stress (92). Klf2 can regulate many different processes in the endothelium like angiogenesis, vascular tone, thrombosis, inflammation, immune regulation and oxidative stress. It is known to downregulate HIF-1α, vascular endothelial growth factor, endothelin-1, vascular cell adhesion molecule and E-selectin, while thrombomodulin and eNOS are some of the upregulated markers (92, 93). It has already been shown by multiple studies that Klf2 gets upregulated during HMP by the shear stress created from the pump (82, 94, 95). The upregulation of eNOS phosphorylation is important for NO levels. NO is an important vasodilator, allowing for sufficient blood flow through the organ. Besides regulating vascular tone, NO also plays a role in endothelial cell migration, proliferation, angiogenesis and it has anti-inflammatory properties by inhibiting leukocyte adherence (96).
When discussing the endothelium, another essential factor is the glycocalyx, a thin layer consisting of proteoglycans that covers the endothelium. This cover is important for leukocyte and platelet adhesion, coagulation and transferring shear stress to endothelial cells (97). The glycocalyx is also an important place where several enzymatic reactions take place due to the docking function of the glycosaminoglycans. Damage to the glycocalyx is a direct consequence of IRI, as demonstrated in several studies (98–100). A large part of the damage to the glycocalyx happens during the reperfusion phase (101). One study looked in more detail into the glycocalyx degradation during human liver transplantation and found that syndecan-1, a biomarker of glycocalyx degradation, was released during reperfusion (102). However, heparan-sulphate levels, another biomarker for glycocalyx degradation, were lower in effluent veins compared with portal venous blood, suggesting binding or uptake of heparan-sulphates. This uptake might suggest repair of the damaged graft. This is supported by a study in kidney transplantation, where the thickness of the glycocalyx increased in time after reperfusion (103). Therefore, it could be beneficial to perfuse donor grafts for a longer period of time to give the glycocalyx time to repair itself, although more research is needed to confirm this. One study in a porcine model of brain death showed that HMP could potentially be used as a platform to restore the glycocalyx by infusion of corline heparin conjugate, heparin molecules that strongly bind to tissue with heparin affinity. By labelling the heparin molecules, binding to the damaged endothelium could be demonstrated (104). Unfortunately, no results on function or outcome were reported.
All in all, there appear to be many functions of the endothelium that are influenced by HMP. Most studies focussed on the infiltration of immune cells and fibrosis and noticed a reduction when using HMP versus SCS. Endothelial dysfunction also affects other mechanisms of IRI. ICAM-1 has also been studied in cancer cells, where they found that an upregulation of ICAM-1 increased cell survival (105). For P-selectin it was found that inhibition of P-selectin reduced apoptosis in endotoxin-induced liver injury in a mouse model (106). Besides leukocyte recruitment, activation of the coagulation system can also lead to an immune response. Components of the clotting system like fibrin can enhance adherence of immune cells and facilitate migration (107). Cross-talk between the complement system and the coagulation system can further activate the immune system (108).
Influence on Innate Immune Response
Both the innate and adaptive immune system play an important role in transplantation. An eligible donor-recipient match has to be found, immunosuppressive drugs have to be taken daily and there is the risk of allograft rejection of the donor organ. The innate immune system plays an important role in IRI. Toll like receptor 4 (TLR4), one of the best characterised TLRs, is able to sense DAMPs that are released during IRI. HMBG1 is one of the most described DAMPs and it has been found to play a role in IRI by activating TLR4 (109). HMGB1 normally resides within the nucleus, where it plays a role in transcription and chromatin modelling (110). It can be actively released by immune cells like dendritic cells or macrophages or passively released upon cell death. Extracellular HMGB1 can bind to TLR4, TLR2 or the receptor for advanced glycation end-products (RAGE) to promote inflammation (110, 111). Several TLRs have been found upregulated in ischemia, mostly TLR2 and TLR4. Activation of TLR4 leads to increased expression of proinflammatory cytokines and adhesion molecules, attraction of neutrophils and macrophages, and activation of circulating immune cells (11, 112–114). Several studies have shown that TLR4 expression strongly correlates with renal graft dysfunction in rats and that TLR4 knockout mice are protected against IRI (14). Both TLR4 and HMGB1 were found to be downregulated when comparing HMP versus SCS (Figure 5) (41, 44, 45, 51). Besides TLR4 and HMGB1, pro-inflammatory cytokines TNF-α, IL-1β, IL-6 and IL-2 were reported to be downregulated after HMP treatment (17, 18, 42, 43, 45, 51, 85, 86, 91). The possibility of further reducing pro-inflammatory cytokine levels during HMP was shown in a study that compared porcine kidneys in a reperfusion model with or without a cytokine filter (115). Kidneys that were perfused with a cytokine filter showed lower levels of IL-6 and IL-8 and higher blood flow. No effect on kidney function based on creatinine clearance was found, but it might be that more processes play a role in improving kidney function. For instance, a cytokine filter is non-specific which means that anti-inflammatory cytokines are removed as well. A shortcoming of most studies reporting on changes in the immune system after HMP is that many only look at pro-inflammatory cytokines while anti-inflammatory cytokines like IL-10 are not as often investigated.
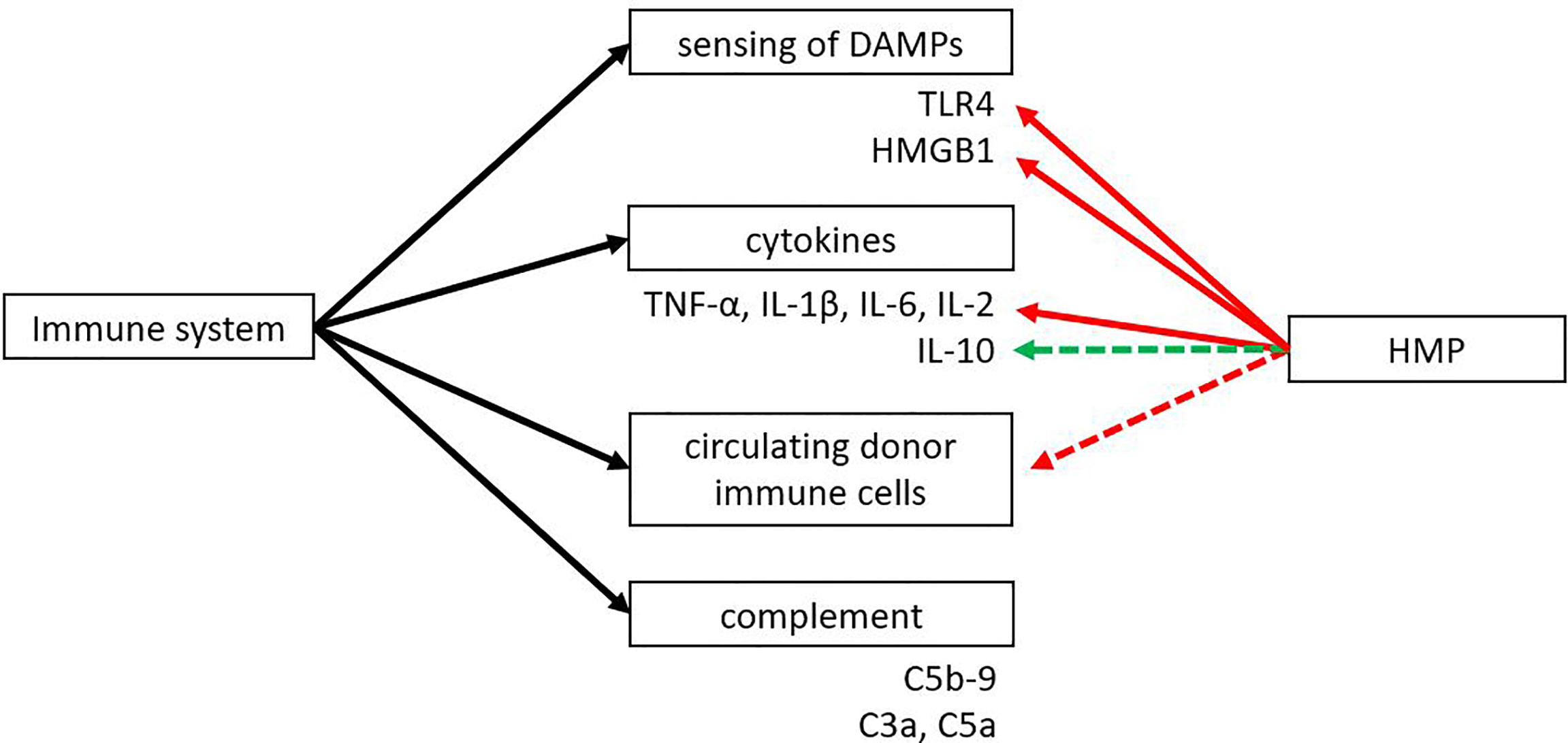
Figure 5 The effect of HMP on dampening the immune response. HMP has shown to reduce sensing of DAMPs and pro-inflammatory cytokines compared to SCS (red line). Some studies suggest there might be an upregulation of anti-inflammatory cytokines and a reduction in circulating donor immune cells (green and red dotted line). The effect of HMP on the complement system is still unknown. DAMPs, damage-associated molecular patterns; HMGB1, high-mobility group box 1; TLR, Toll-like receptor.
Next to the release of DAMPs or inflammatory mediators, molecular alterations in the donor organ following IRI can also result in the activation of innate immunity via the complement system. The complement system can be activated via the classical, lectin or alternative pathway. Activation of the complement system by DAMPs released from IRI can lead to the cleavage of C3 and C5 and the formation of the membrane attack complex. Cleavage products C3a and C5a can act as anaphylatoxins to activate immune cells (116, 117). A complexity in complement research is that activation of the complement system can vary between organs. IRI in the liver leads to activation of the classical pathway, while IRI in the heart activates the classical and lectin pathway (117). Therefore, more studies should look into the effect of hypothermic machine perfusion on complement activation.
Probably due to the short time of perfusion and the cold temperature not many studies have looked into the effect of HMP on the adaptive immune system. Several studies have looked into the circulating cell types during machine perfusion and found large amounts of immune cells with flow cytometry (118, 119). Although the consequence of circulating immune cells during machine perfusion is not clear yet, it could have a beneficial effect by immunodepletion of the graft.
Just like the other mechanisms, immune activation also influences the previous discussed mechanisms involved in IRI. Jantsch et al. (120) showed that TLR activation on mouse dendritic cells can also lead to stabilization of HIF-1α under normoxic conditions. This increase in HIF-1α resulted in the transcription of inflammatory target genes Ptgs2 and Nos2, whereas increase of HIF-1α by hypoxia lead to increased transcription of HIF-1α target genes Glut1 and Pgk1. Besides regulation by PHD, pro-inflammatory cytokines have also been reported to regulate HIF-1α (121). TNF-α and IL-1β have been proposed to increase HIF-1α levels via various mechanisms at pre- and posttranscriptional levels. However, most information is obtained from cell studies, so further investigations in transplantation models are needed to confirm this. Immune activation can also lead to cell death and endothelial dysfunction. Activation of the complement system leading to the membrane attack complex can induce cell death via lysis (117). In an IRI mouse model it was also shown that activation of the terminal complement pathway lead to shedding of the glycocalyx, indicated by accumulation of glycocalyx components syndecan-1, hyaluronan and heparan sulphate (122).
Interventions During Hypothermic Machine Perfusion
Machine perfusion provides an ideal platform to use for interventions. Current interventions that are being exploited are RNA silencing, stem cell therapy and complement blockade (123). One study looked into the use of lentiviral vectors encoding short hairpin RNAs that target the β2-microglobulin of the major histocompatibility complex 1 during sub-normothermic ex vivo rat kidney perfusion (124). They found decreased transcription levels of β2-microglobulin and pro-inflammatory cytokine levels, while increased levels of anti-inflammatory cytokines were found. Genetic modification showed no additional cell death, showing feasibility of this technique.
Mesenchymal stromal cells (MSCs) are also an emerging topic in this field. Due to the immune modulating properties of MSCs both the innate and adaptive immune response could be controlled. MSCs could also be beneficial for tissue repair (125). The TRITON study was a single centre randomized prospective study where MSCs were infused 6 and 7 weeks after renal transplantation in combination with reduced immunosuppressive drugs. It showed that MSC therapy was safe and feasible and also showed higher numbers of regulatory T cells in peripheral blood (126). Due to the size of the MSCs they will get stuck in the lung capillaries when given to the recipient systemically via the blood stream. Therefore, ex vivo machine perfusion could be an attractive way to give MSC therapy. In a renal porcine autotransplantation model it was shown by the MePEP consortium that giving MSC therapy during NMP was safe and feasible and MSCs ended up in the renal cortex (127). However, in the short follow-up time of 14 days no beneficial effects on function could be shown despite prolonged warm and cold ischemia times. Thompson et al. (128) added multipotent adult progenitor cells added during NMP of discarded human kidneys. They showed that this therapy may increase urine production and microvascular perfusion, upregulate anti-inflammatory cytokines and downregulate injury markers and pro-inflammatory cytokines (128).
Activation of the complement system already occurs early-on in deceased donors as shown by Damman et al., as elevated C5b-9 levels were found in the plasma of deceased donors (129). The elevated levels were also associated with biopsy-proven acute rejection. A phase 1 study where complement is inhibited using C1-INH is being conducted to try to decrease systemic inflammation and DGF incidence in expanded criteria donors as a donor pre-treatment strategy (NCT02435732). Complement inhibitor C1-INH is a serine protease that can regulate the classical, lectin and alternative pathway (130). Machine perfusion could provide an ideal environment to target the complement system specifically in the graft instead of the whole body. The EMPIRIKAL trial was the first study to look into the administration of complement inhibitor Mirococept at time of transplantation to prevent DGF (ISRCTN49958194) (131, 132). The inhibitor is given during a 15min flush of the kidney while the organ is on ice slush. Mirococept is designed to inhibit the complement system at the C3 level. Because the first dose of 10mg did not show a significant difference from the control, the study was stopped to conduct a dose study first. A dose finding study was initiated in normothermic machine perfused porcine kidneys showing that 80mg of Mirococept was the optimal dose (132). It showed to be safe as minimal washout into the circulation occurred and no detrimental effects on flow parameters or histology were observed, opening the path to further clinical development. In this study the inhibitor was not given during HMP, but during a short flush. It would be interesting to study if Mirococept, when given during HMP where would be circulating through the organ for several hours, could be administered in a lower dose.
New Areas of Interest
With HMP being implemented and clinically used in kidney and liver preservation in several countries, research into machine perfusion has emerged. Due to novel technology, nowadays also ex-vivo NMP has become feasible, although its clinical implementation is still in its infancy. The potential advantages of NMP over HMP are its ability to restore cellular function, upregulate protective repair mechanisms and allow better assessment of function. At the same time it provides a platform for cell therapy i.e. administration of mesenchymal stem cells (133). The first clinical trial performed by Hosgood and Nicholson et al. showed that 1-hour normothermic perfusion of human donor kidneys with subsequent transplantation is safe and feasible (134). Due to better assessment of human DCD kidneys, that were declined for transplantation, this group was able to reverse that decision and successfully transplant several kidneys, thereby increasing the donor pool.
A topic of interest in current HMP research is the presumed positive effect of addition of oxygen during cold machine perfusion. Due to the low temperature (approximately 10°C), the metabolism of the graft is reduced by 90%. Initially, it was thought that addition of oxygen was not necessary at that level of reduced metabolism and might actually increase detrimental ROS formation. New insight obtained by pre-clinical work in kidney and liver (135–137) showed improved recovery of transplanted organs when oxygenated perfusion had been used. Recently, the COMPARE trial by the COPE consortium showed in a multicentre clinical context that oxygenated HMP of older DCD donor kidneys was better than non-oxygenated HMP in terms of kidney function, graft survival and rejection rate (ISRCTN32967929) (138). The POMP trial of the COPE consortium compared in higher-risk ECD kidneys conventional SCS to a preservation of first SCS, then followed by a brief period of oxygenated HMP. This large clinical study did not detect any difference in function or survival. This suggests that a brief period of 4.5h oxygenated HMP at the end of preservation is not sufficient for oxygenated HMP to have beneficial effects. It might be best to start oxygenated HMP as soon as possible after organ procurement (139). To date, several clinical trials investigating hypothermic oxygenated machine perfusion in liver and kidney transplantation are ongoing.
As in many other fields, studies looking into machine perfusion are still mainly performed in animal models. Choosing the right animal model however is of importance to be able to translate the newfound knowledge to the human situation. Lerink et al. showed that there is still a big translational gap in many preclinical IRI models (140). This was also shown in the above mentioned EMPERIKAL trial that was first tested in a rat model. Based on those results, the dose range for humans was decided. However, when tested in the pig it was shown that the human dose should have been 12 times higher than estimated from the rat study (132). The porcine transplantation model appears to be the best simulation of human conditions due to similar physiology, size and immune system. Several groups have started to use slaughterhouse pig organs to test various aspects of organ perfusion which reduces the need for animal house pigs n the early exploratory stages (141). In a next step, including experimental transplantation, animal house models will be required before phase 1 studies in humans can be ethically justified.
Summary
In conclusion, machine perfusion and in particular HMP appears to influence many different pathways involved in IRI. The goal of this review was to compare HMP with the old gold standard SCS. Studies are now available including clinical evidence that HMP has beneficial effects on outcomes such as immediate function and survival but also on important mechanisms that are involved in IRI: HIF levels, cell death, endothelial dysfunction and the innate immune response. To obtain better insight in the mechanisms of injury and repair, future studies should focus on analysis of the effects of HMP on all four mechanisms. This will allow the discovery of underlying relationships and clinically relevant pathways. It will also lead to the development of targeted interventions to increase viability whilst possibly modulating the graft and rendering it less immunogenic. This may help to reach the goal to enhance function and prolong survival avoiding chronic graft dysfunction after 5-10 years due to progressive scarring of the transplanted organ.
Author Contributions
LK, CK, and RP came up with the conception and design. LK wrote the manuscript and CK and RP revised the manuscript. All authors have read and agreed to the published version of the manuscript.
Conflict of Interest
The authors declare that the research was conducted in the absence of any commercial or financial relationships that could be construed as a potential conflict of interest.
Publisher’s Note
All claims expressed in this article are solely those of the authors and do not necessarily represent those of their affiliated organizations, or those of the publisher, the editors and the reviewers. Any product that may be evaluated in this article, or claim that may be made by its manufacturer, is not guaranteed or endorsed by the publisher.
References
1. Schröppel B, Legendre C. Delayed Kidney Graft Function: From Mechanism to Translation. Kidney Int (2014) 86:251–8. doi: 10.1038/ki.2014.18
2. Aubert O, Kamar N, Vernerey D, Viglietti D, Martinez F, Duong-Van-huyen JP, et al. Long Term Outcomes of Transplantation Using Kidneys From Expanded Criteria Donors: Prospective, Population Based Cohort Study. BMJ (2015) 351:18. doi: 10.1136/BMJ.H3557
3. Jochmans I, O’Callaghan JM, Pirenne J, Ploeg RJ. Hypothermic Machine Perfusion of Kidneys Retrieved From Standard and High-Risk Donors. Transplant Int (2015) 28:665–76. doi: 10.1111/tri.12530
4. Moers C, Smits JM, Maathuis M-HJ, Treckmann J, van Gelder F, Napieralski BP, et al. Machine Perfusion or Cold Storage in Deceased-Donor Kidney Transplantation. New Engl J Med (2009) 360:7–19. doi: 10.1056/NEJMoa0802289
5. O’Callaghan JM, Morgan RD, Knight SR, Morris PJ. Systematic Review and Meta-Analysis of Hypothermic Machine Perfusion Versus Static Cold Storage of Kidney Allografts on Transplant Outcomes. Br J Surg (2013) 100:991–1001. doi: 10.1002/bjs.9169
6. Tingle SJ, Figueiredo RS, Moir JAG, Goodfellow M, Thompson ER, Ibrahim IK, et al. Hypothermic Machine Perfusion is Superior to Static Cold Storage in Deceased Donor Kidney Transplantation: A Meta-Analysis. Clin Transplant (2020) 34:e13814. doi: 10.1111/ctr.13814
7. Jia J, Nie Y, Li J, Xie H, Zhou L, Yu J, et al. A Systematic Review and Meta-Analysis of Machine Perfusion vs. Static Cold Storage of Liver Allografts on Liver Transplantation Outcomes: The Future Direction of Graft Preservation. Front Med (2020) 7:135. doi: 10.3389/fmed.2020.00135
8. Schaapherder A, Wijermars LGM, de Vries DK, de Vries APJ, Bemelman FJ, van de Wetering J, et al. Equivalent Long-Term Transplantation Outcomes for Kidneys Donated After Brain Death and Cardiac Death: Conclusions From a Nationwide Evaluation. EClinicalMedicine (2018) 4–5:25–31. doi: 10.1016/J.ECLINM.2018.09.007
9. Legendre C, Canaud G, Martinez F. Factors Influencing Long-Term Outcome After Kidney Transplantation. Transplant Int (2014) 27:19–27. doi: 10.1111/TRI.12217
10. Wu MY, Yiang GT, Liao WT, Tsai APY, Cheng YL, Cheng PW, et al. Current Mechanistic Concepts in Ischemia and Reperfusion Injury. Cell Physiol Biochem (2018) 46:1650–67. doi: 10.1159/000489241
11. Nieuwenhuijs-Moeke GJ, Pischke SE, Berger SP, Sanders JSF, Pol RA, Struys MMRF, et al. Ischemia and Reperfusion Injury in Kidney Transplantation: Relevant Mechanisms in Injury and Repair. J Clin Med (2020) 9:253. doi: 10.3390/jcm9010253
12. Strowitzki M, Cummins E, Taylor C. Protein Hydroxylation by Hypoxia-Inducible Factor (HIF) Hydroxylases: Unique or Ubiquitous? Cells (2019) 8:384. doi: 10.3390/cells8050384
13. Zhao DF, Dong Q, Zhang T. Effects of Static Cold Storage and Hypothermic Machine Perfusion on Oxidative Stress Factors, Adhesion Molecules, and Zinc Finger Transcription Factor Proteins Before and After Liver Transplantation. Ann Transplant (2017) 22:96–100. doi: 10.12659/AOT.901897
14. Krezdorn N, Sakthivel D, Turk M, Aycart MA, Tasigiorgos S, Bueno EM, et al. Reduced Hypoxia-Related Genes in Porcine Limbs in Ex Vivo Hypothermic Perfusion Versus Cold Storage. J Surg Res (2018) 232:137–45. doi: 10.1016/j.jss.2018.05.067
15. Wszola M, Kwiatkowski A, Latek M, Ostrowski K, Domagala P, Ciszek M, et al. Long Term Medical and Economical Benefit of Machine Perfusion (MP) Kidney Storage in Comparison to Cold Storage (CS). Available at: http://www.annalsoftransplantation.com/fulltxt.php?ICID=883854.
16. Darius T, Vergauwen M, Smith TB, Patel K, Craps J, Joris V, et al. Influence of Different Partial Pressures of Oxygen During Continuous Hypothermic Machine Perfusion in a Pig Kidney Ischemia-Reperfusion Autotransplant Model. Transplantation (2020) 104:731–43. doi: 10.1097/TP.0000000000003051
17. Guarrera J, Bae C, Henry S. Hypothermic Machine Preservation Reduces Inflammatory, Hypoxic and Apoptotic Injuries in Human Liver Transplantation. Am J Transplant (2015) 15 (suppl 3).
18. Henry SD, Nachber E, Tulipan J, Stone J, Bae C, Reznik L, et al. Hypothermic Machine Preservation Reduces Molecular Markers of Ischemia/Reperfusion Injury in Human Liver Transplantation. Am J Transplant (2012) 12:2477–86. doi: 10.1111/j.1600-6143.2012.04086.x
19. Burlage LC, Karimian N, Westerkamp AC, Visser N, Matton APM, van Rijn R, et al. Oxygenated Hypothermic Machine Perfusion After Static Cold Storage Improves Endothelial Function of Extended Criteria Donor Livers. HPB (2017) 19:538–46. doi: 10.1016/j.hpb.2017.02.439
20. Ito M, Tanaka T, Ishii T, Wakashima T, Fukui K, Nangaku M. Prolyl Hydroxylase Inhibition Protects the Kidneys From Ischemia via Upregulation of Glycogen Storage. Kidney Int (2020) 97:687–701. doi: 10.1016/j.kint.2019.10.020
21. Tekin D, Dursun AD, Xi L. Hypoxia Inducible Factor 1 (HIF-1) and Cardioprotection. Acta Pharmacologica Sin (2010) 31:1085–94. doi: 10.1038/aps.2010.132
22. Zhang Z, Yao L, Yang J, Wang Z, Du G. PI3K/Akt and HIF-1 Signaling Pathway in Hypoxia-Ischemia (Review). Mol Med Rep (2018) 18:3547–54. doi: 10.3892/mmr.2018.9375
23. Li J, Zhou W, Chen W, Wang H, Zhang Y, Yu T. Mechanism of the Hypoxia Inducible Factor 1/Hypoxic Response Element Pathway in Rat Myocardial Ischemia/Diazoxide Post–Conditioning. Mol Med Rep (2020) 21:1527–36. doi: 10.3892/mmr.2020.10966
24. Raval RR, Lau KW, Tran MGB, Sowter HM, Mandriota SJ, Li J-L, et al. Contrasting Properties of Hypoxia-Inducible Factor 1 (HIF-1) and HIF-2 in Von Hippel-Lindau-Associated Renal Cell Carcinoma. Mol Cell Biol (2005) 25:5675–86. doi: 10.1128/mcb.25.13.5675-5686.2005
25. Tennant D, Howell NJ. The Role of HIFs in Ischemia-Reperfusion Injury. Hypoxia (2014) 2:107. doi: 10.2147/HP.S49720
26. Majmundar AJ, Wong WJ, Simon MC. Molecular Cell Hypoxia-Inducible Factors and the Response to Hypoxic Stress. Mol Cell (2010) 40:294–309. doi: 10.1016/j.molcel.2010.09.022
27. Kapitsinou PP, Sano H, Michael M, Kobayashi H, Davidoff O, Bian A, et al. Endothelial HIF-2 Mediates Protection and Recovery From Ischemic Kidney Injury. J Clin Invest (2014) 124:2396–409. doi: 10.1172/JCI69073
28. Bernhardt WM, Schmitt R, Rosenberger C, Münchenhagen PM, Gröne HJ, Frei U, et al. Expression of Hypoxia-Inducible Transcription Factors in Developing Human and Rat Kidneys. Kidney Int (2006) 69:114–22. doi: 10.1038/SJ.KI.5000062
29. Haase VH. Hypoxia-Inducible Factors in the Kidney. Am J Physiol Renal Physiol (2006) 291:F271. doi: 10.1152/AJPRENAL.00071.2006
30. Evans CE. Hypoxia and HIF Activation as a Possible Link Between Sepsis and Thrombosis. Thromb J (2019) 17:1–4. doi: 10.1186/S12959-019-0205-9
31. Goodwin J, Choi H, Hsieh MH, Neugent ML, Ahn JM, Hayenga HN, et al. Targeting Hypoxia-Inducible Factor-1a/Pyruvate Dehydrogenase Kinase 1 Axis by Dichloroacetate Suppresses Bleomycin-Induced Pulmonary Fibrosis. Am J Respir Cell Mol Biol (2018) 58:216–31. doi: 10.1165/RCMB.2016-0186OC/SUPPL_FILE/DISCLOSURES.PDF
32. Harris AJ, Thompson AR, Whyte MK, Walmsley SR. HIF-Mediated Innate Immune Responses: Cell Signaling and Therapeutic Implications. Hypoxia (2014) 2:47. doi: 10.2147/HP.S50269
33. Zhang X, Du P, Luo K, Li Y, Liu Z, Wang W, et al. Hypoxia-Inducible Factor-1alpha Protects the Liver Against Ischemia-Reperfusion Injury by Regulating the A2B Adenosine Receptor. Bioengineered (2021) 12:3737. doi: 10.1080/21655979.2021.1953217
34. Szondy Z, Sarang Z, Kiss B, Garabuczi É, Köröskényi K. Anti-Inflammatory Mechanisms Triggered by Apoptotic Cells During Their Clearance. Front Immunol (2017) 8:909. doi: 10.3389/FIMMU.2017.00909
35. Yatim N, Cullen S, Albert ML. Dying Cells Actively Regulate Adaptive Immune Responses. Nat Rev Immunol (2017) 17:262–75. doi: 10.1038/nri.2017.9
36. Qin S, Wang H, Yuan R, Li H, Ochani M, Ochani K, et al. Role of HMGB1 in Apoptosis-Mediated Sepsis Lethality. J Exp Med (2006) 203:1637–42. doi: 10.1084/jem.20052203
37. Shimada K, Crother TR, Karlin J, Dagvadorj J, Chiba N, Chen S, et al. Oxidized Mitochondrial DNA Activates the NLRP3 Inflammasome During Apoptosis. Immunity (2012) 36:401–14. doi: 10.1016/j.immuni.2012.01.009
38. Zhang Y, Fu Z, Zhong Z, Wang R, Hu L, Xiong Y, et al. Hypothermic Machine Perfusion Decreases Renal Cell Apoptosis During Ischemia/Reperfusion Injury via the Ezrin/AKT Pathway. Artif Organs (2016) 40:129–35. doi: 10.1111/aor.12534
39. Lázaro A, Humanes B, Jado JC, Mojena M, González-Nicolás MÁ, del Cañizo JF, et al. Beneficial Effect of Short Pretransplant Period of Hypothermic Pulsatile Perfusion of the Warm-Ischemic Kidney After Cold Storage: Experimental Study. BioMed Res Int (2016) 2016. doi: 10.1155/2016/2518626
40. Urbanellis P, Hamar M, Kaths JM, Kollmann D, Linares I, Mazilescu L, et al. Normothermic Ex Vivo Kidney Perfusion Improves Early DCD Graft Function Compared With Hypothermic Machine Perfusion and Static Cold Storage. Transplantation (2020) 104:947–55. doi: 10.1097/TP.0000000000003066
41. Xue S, He W, Zeng X, Tang Z, Feng S, Zhong Z, et al. Hypothermic Machine Perfusion Attenuates Ischemia/Reperfusion Injury Against Rat Livers Donated After Cardiac Death by Activating the Keap1/Nrf2-ARE Signaling Pathway. Mol Med Rep (2018) 18:815–26. doi: 10.3892/mmr.2018.9065
42. Zhong Z, Hu Q, Fu Z, Wang R, Xiong Y, Zhang Y, et al. Increased Expression of Aldehyde Dehydrogenase 2 Reduces Renal Cell Apoptosis During Ischemia/Reperfusion Injury After Hypothermic Machine Perfusion. Artif Organs (2016) 40:596–603. doi: 10.1111/aor.12607
43. Zeng X, Li M, Fan X, Xue S, Liang W, Fang Z, et al. Hypothermic Oxygenated Machine Perfusion Alleviates Donation After Circulatory Death Liver Injury Through Regulating P-Selectin-Dependent and -Independent Pathways in Mice. Transplantation (2019) 103:918–28. doi: 10.1097/TP.0000000000002621
44. Zeng X, Wang S, Li S, Yang Y, Fang Z, Huang H, et al. Hypothermic Oxygenated Machine Perfusion Alleviates Liver Injury in Donation After Circulatory Death Through Activating Autophagy in Mice. Artif Organs (2019) 43:E320–32. doi: 10.1111/aor.13525
45. Schlegel A, de Rougemont O, Graf R, Clavien PA, Dutkowski P. Protective Mechanisms of End-Ischemic Cold Machine Perfusion in DCD Liver Grafts. J Hepatol (2013) 58:278–86. doi: 10.1016/j.jhep.2012.10.004
46. Oltval ZN, Milliman CL, Korsmeyer SJ. Bcl-2 Heterodimerizes In Vivo With a Conserved Homolog, Bax, That Accelerates Programed Cell Death. Cell (1993) 74:609–19. doi: 10.1016/0092-8674(93)90509-O
47. García de la Cadena S, Massieu L. Caspases and Their Role in Inflammation and Ischemic Neuronal Death. Focus on Caspase-12. Apoptosis (2016) 21:763–77. doi: 10.1007/s10495-016-1247-0
48. He N, Li JH, Jia JJ, Xu KD, Zhou YF, Jiang L, et al. Hypothermic Machine Perfusion’s Protection on Porcine Kidney Graft Uncovers Greater Akt-Erk Phosphorylation. Transplant Proc (2017) 49:1923–9. doi: 10.1016/j.transproceed.2017.05.011
49. Minor T, Manekeller S, Sioutis M, Dombrowski F. Endoplasmic and Vascular Surface Activation During Organ Preservation: Refining Upon the Benefits of Machine Perfusion. Am J Transplant (2006) 6:1355–66. doi: 10.1111/j.1600-6143.2006.01338.x
50. Manekeller S, Schuppius A, Stegemann J, Hirner A, Minor T. Role of Perfusion Medium, Oxygen and Rheology for Endoplasmic Reticulum Stress-Induced Cell Death After Hypothermic Machine Preservation of the Liver. Transplant Int (2008) 21:169–77. doi: 10.1111/j.1432-2277.2007.00595.x
51. Yang Z, Zhong Z, Li M, Xiong Y, Wang Y, Peng G, et al. Hypothermic Machine Perfusion Increases A20 Expression Which Protects Renal Cells Against Ischemia/Reperfusion Injury by Suppressing Inflammation, Apoptosis and Necroptosis. Int J Mol Med (2016) 38:161–71. doi: 10.3892/ijmm.2016.2586
52. Galluzzi L, López-Soto A, Kumar S, Kroemer G. Caspases Connect Cell-Death Signaling to Organismal Homeostasis. Immunity (2016) 44:221–31. doi: 10.1016/j.immuni.2016.01.020
53. Baidya R, Crawford DHG, Gautheron J, Wang H, Bridle KR. Necroptosis in Hepatosteatotic Ischaemia-Reperfusion Injury. Int J Mol Sci (2020) 21:1–29. doi: 10.3390/ijms21165931
54. Degterev A, Huang Z, Boyce M, Li Y, Jagtap P, Mizushima N, et al. Chemical Inhibitor of Nonapoptotic Cell Death With Therapeutic Potential for Ischemic Brain Injury. Nat Chem Biol (2005) 1:112–9. doi: 10.1038/nchembio711
55. Shen B, Mei M, Pu Y, Zhang H, Liu H, Tang M, et al. Necrostatin-1 Attenuates Renal Ischemia and Reperfusion Injury via Meditation of HIF-1α/Mir-26a/TRPC6/PARP1 Signaling. Mol Ther - Nucleic Acids (2019) 17:701–13. doi: 10.1016/j.omtn.2019.06.025
56. Liu S, Chen Y, Wang S, Yu Q. Protective Effect of Dabrafenib on Renal Ischemia-Reperfusion Injury In Vivo and In Vitro. Biochem Biophys Res Commun (2020) 522:395–401. doi: 10.1016/j.bbrc.2019.11.105
57. Vandenabeele P, Galluzzi L, vanden Berghe T, Kroemer G. Molecular Mechanisms of Necroptosis: An Ordered Cellular Explosion. Nat Rev Mol Cell Biol (2010) 11:700–14. doi: 10.1038/nrm2970
58. He W, Ye S, Zeng C, Xue S, Hu X, Zhang X, et al. Hypothermic Oxygenated Perfusion (HOPE) Attenuates Ischemia/Reperfusion Injury in the Liver Through Inhibition of the TXNIP/NLRP3 Inflammasome Pathway in a Rat Model of Donation After Cardiac Death. Faceb J. (2018) 32:6212–27. doi: 10.1096/fj.201800028RR
59. Noda K, Tane S, Haam SJ, D’Cunha J, Hayanga AJ, Luketich JD, et al. Targeting Circulating Leukocytes and Pyroptosis During Ex Vivo Lung Perfusion Improves Lung Preservation. Transplantation (2017) 101:2841–9. doi: 10.1097/TP.0000000000001798
60. Bergsbaken T, Fink SL, Cookson BT. Pyroptosis: Host Cell Death and Inflammation. Nat Rev Microbiol (2009) 7:99. doi: 10.1038/NRMICRO2070
61. Ma S, Wang Y, Chen Y, Cao F. The Role of the Autophagy in Myocardial Ischemia/Reperfusion Injury. Biochim Biophys Acta - Mol Basis Dis (2015) 1852:271–6. doi: 10.1016/j.bbadis.2014.05.010
62. Cursio R, Colosetti P, Gugenheim J. Autophagy and Liver Ischemia-Reperfusion Injury. BioMed Res Int (2015) 2015. doi: 10.1155/2015/417590
63. Lin D, Xiang T, Qiu Q, Leung J, Xu J, Zhou W, et al. Aldehyde Dehydrogenase 2 Regulates Autophagy via the Akt-mTOR Pathway to Mitigate Renal Ischemia-Reperfusion Injury in Hypothermic Machine Perfusion. Life Sci (2020) 253:117705. doi: 10.1016/j.lfs.2020.117705
64. van Erp AC, Hoeksma D, Rebolledo RA, Ottens PJ, Jochmans I, Monbaliu D, et al. The Crosstalk Between ROS and Autophagy in the Field of Transplantation Medicine. Oxid Med Cell Longevity (2017) 2017. doi: 10.1155/2017/7120962
65. Zhang T, Guo J, Gu J, Chen K, Li H, Wang J. Protective Role of mTOR in Liver Ischemia/Reperfusion Injury: Involvement of Inflammation and Autophagy. Oxid Med Cell Longevity (2019) 2019. doi: 10.1155/2019/7861290
66. Boteon YL, Laing R, Mergental H, Reynolds GM, Mirza DF, Afford SC, et al. Mechanisms of Autophagy Activation in Endothelial Cell and Their Targeting During Normothermic Machine Liver Perfusion. World J Gastroenterol (2017) 23:8443–51. doi: 10.3748/wjg.v23.i48.8443
67. Valko M, Jomova K, Rhodes CJ, Kuča K, Musílek K. Redox- and Non-Redox-Metal-Induced Formation of Free Radicals and Their Role in Human Disease. Arch Toxicol (2015) 90:1–37. doi: 10.1007/S00204-015-1579-5
68. Linkermann A, Skouta R, Himmerkus N, Mulay SR, Dewitz C, de ZF, et al. Synchronized Renal Tubular Cell Death Involves Ferroptosis. Proc Natl Acad Sci (2014) 111:16836–41. doi: 10.1073/PNAS.1415518111
69. Angeli JPF, Schneider M, Proneth B, Tyurina YY, Tyurin VA, Hammond VJ, et al. Inactivation of the Ferroptosis Regulator Gpx4 Triggers Acute Renal Failure in Mice. Nat Cell Biol (2014) 16:1180–91. doi: 10.1038/ncb3064
70. Yamada N, Karasawa T, Wakiya T, Sadatomo A, Ito H, Kamata R, et al. Iron Overload as a Risk Factor for Hepatic Ischemia-Reperfusion Injury in Liver Transplantation: Potential Role of Ferroptosis. Am J Transplant (2020) 20:1606–18. doi: 10.1111/AJT.15773
71. Hattori K, Ishikawa H, Sakauchi C, Takayanagi S, Naguro I, Ichijo H. Cold Stress-Induced Ferroptosis Involves the ASK1-P38 Pathway. EMBO Rep (2017) 18:2067–78. doi: 10.15252/EMBR.201744228
72. Yang M, Antoine DJ, Weemhoff JL, Jenkins RE, Farhood A, Park BK, et al. Biomarkers Distinguish Apoptotic and Necrotic Cell Death During Hepatic Ischemia/Reperfusion Injury in Mice. Liver Transplant (2014) 20:1372–82. doi: 10.1002/lt.23958
73. Kraupp BG, Ruttkay-Nedecky B, Koudelka H, Bukowska K, Bursch W, Schulte-Hermann R. In Situ Detection of Fragmented Dna (Tunel Assay) Fails to Discriminate Among Apoptosis, Necrosis, and Autolytic Cell Death: A Cautionary Note. Hepatology (1995) 21:1465–8. doi: 10.1002/hep.1840210534
74. Jiménez-Castro MB, Cornide-Petronio ME, Gracia-Sancho J, Peralta C. Inflammasome-Mediated Inflammation in Liver Ischemia-Reperfusion Injury. Cells (2019) 8:1131. doi: 10.3390/cells8101131
75. Samir P, Malireddi RKS, Kanneganti TD. The PANoptosome: A Deadly Protein Complex Driving Pyroptosis, Apoptosis, and Necroptosis (PANoptosis). Front Cell Infect Microbiol (2020) 10:238. doi: 10.3389/fcimb.2020.00238
76. Kaushal GP. Shah S V. Autophagy in Acute Kidney Injury. Kidney Int (2016) 89:779–91. doi: 10.1016/j.kint.2015.11.021
77. Yang Q, He GW, Underwood MJ, Yu CM. Cellular and Molecular Mechanisms of Endothelial Ischemia/Reperfusion Injury: Perspectives and Implications for Postischemic Myocardial Protection(2016) (Accessed April 19, 2021).
78. Nishiwaki A, Ueda T, Ugawa S, Shimada S, Ogura Y. Upregulation of P-Selectin and Intercellular Adhesion Molecule-1 After Retinal Ischemia-Reperfusion Injury. Invest Ophthalmol Visual Sci (2003) 44:4931–5. doi: 10.1167/iovs.02-1324
79. Pernow J. The Role of the Endothelium for Reperfusion Injury. Eur Heart J Suppl (2001) 3:C22–7. doi: 10.1016/S1520-765X(01)90026-4
80. Vaziri N, Thuillier R, Favreau FD, Eugene M, Milin S, Chatauret NP, et al. Analysis of Machine Perfusion Benefits in Kidney Grafts: A Preclinical Study. J Trans Med (2011) 9:15. doi: 10.1186/1479-5876-9-15
81. Thuillier R, Codas R, Marchand E, Lathelize H, Page O, Valagier A, et al. Chronic Renoprotective Effect of Pulsatile Perfusion Machine RM3 and IGL-1 Solution in a Preclinical Kidney Transplantation Model. J Trans Med (2012) 10:233. doi: 10.1186/1479-5876-10-233
82. Liu Z, Zhong Z, Lan J, Li M, Wang W, Yang J, et al. Mechanisms of Hypothermic Machine Perfusion to Decrease Donation After Cardiac Death Graft Inflammation: Through the Pathway of Upregulating Expression of KLF2 and Inhibiting TGF-β Signaling. Artif Organs (2017) 41:82–8. doi: 10.1111/aor.12701
83. Guerrot D, Dussaule JC, Kavvadas P, Boffa JJ, Chadjichristos CE, Chatziantoniou C. Progression of Renal Fibrosis: The Underestimated Role of Endothelial Alterations. Fibrogenesis Tissue Repair (2012) 5:1–6. doi: 10.1186/1755-1536-5-S1-S15/FIGURES/1
84. Perry HM, Okusa MD. Endothelial Dysfunction in Renal Interstitial Fibrosis. Nephron (2016) 134:167–71. doi: 10.1159/000447607
85. Guarrera JV, Henry SD, Chen SWC, Brown T, Nachber E, Arrington B, et al. Hypothermic Machine Preservation Attenuates Ischemia/Reperfusion Markers After Liver Transplantation: Preliminary Results. J Surg Res (2011) 167:e365–73. doi: 10.1016/j.jss.2010.01.038
86. Fu Z, Ye Q, Zhang Y, Zhong Z, Xiong Y, Wang Y, et al. Hypothermic Machine Perfusion Reduced Inflammatory Reaction by Downregulating the Expression of Matrix Metalloproteinase 9 in a Reperfusion Model of Donation After Cardiac Death. Artif Organs (2016) 40:E102–11. doi: 10.1111/aor.12658
87. Tulipan JE, Stone J, Samstein B, Kato T, Emond JC, Henry SD, et al. Molecular Expression of Acute Phase Mediators is Attenuated by Machine Preservation in Human Liver Transplantation: Preliminary Analysis of Effluent, Serum, and Liver Biopsies. Surgery (2011) 150:352–60. doi: 10.1016/j.surg.2011.06.003
88. Bedi S, Vidyasagar A, Djamali A. Epithelial-To-Mesenchymal Transition and Chronic Allograft Tubulointerstitial Fibrosis. Transplant Rev (2008) 22:1–5. doi: 10.1016/j.trre.2007.09.004
89. Chatauret N, Coudroy R, Delpech PO, Vandebrouck C, Hosni S, Scepi M, et al. Mechanistic Analysis of Nonoxygenated Hypothermic Machine Perfusion’s Protection on Warm Ischemic Kidney Uncovers Greater eNOS Phosphorylation and Vasodilation. Am J Transplant (2014) 14:2500–14. doi: 10.1111/ajt.12904
90. Gallinat A, Efferz P, Paul A, Minor T. One or 4 H of “In-House” Reconditioning by Machine Perfusion After Cold Storage Improve Reperfusion Parameters in Porcine Kidneys. Transplant Int (2014) 27:1214–9. doi: 10.1111/tri.12393
91. Hu X, Wang W, Zeng C, He W, Zhong Z, Liu Z, et al. Appropriate Timing for Hypothermic Machine Perfusion to Preserve Livers Donated After Circulatory Death. Mol Med Rep (2020) 22:2003–11. doi: 10.3892/mmr.2020.11257
92. Nayak L, Lin Z, Jain MK. “‘Go With the Flow’”: How Krüppel-Like Factor 2 Regulates the Vasoprotective Effects of Shear Stress. Antioxidants Redox Signaling (2011) 15:1449–61. doi: 10.1089/ars.2010.3647
93. Parmar KM, Larman HB, Dai G, Zhang Y, Wang ET, Moorthy SN, et al. Integration of Flow-Dependent Endothelial Phenotypes by Kruppel-Like Factor 2. J Clin Invest (2006) 116:49–58. doi: 10.1172/JCI24787
94. Burlage L. Oxygenated Machine Perfusion of Donor Livers and Limbs: Studies on Endothelial Activation and Function (2019). Available at: www.designyourthesis.com (Accessed February 4, 2021).
95. Gallinat A, Paul A, Efferz P, Lüer B, Kaiser G, Wohlschlaeger J, et al. Hypothermic Reconditioning of Porcine Kidney Grafts by Short-Term Preimplantation Machine Perfusion. Transplantation (2012) 93:787–93. doi: 10.1097/TP.0b013e3182492201
96. Kolluru GK, Siamwala JH, Chatterjee S. ENOS Phosphorylation in Health and Disease. Biochimie (2010) 92:1186–98. doi: 10.1016/j.biochi.2010.03.020
97. Mathis S, Putzer G, Schneeberger S, Martini J. The Endothelial Glycocalyx and Organ Preservation—From Physiology to Possible Clinical Implications for Solid Organ Transplantation. Int J Mol Sci (2021) 22:4019. doi: 10.3390/IJMS22084019
98. Chappell D, Jacob M, Hofmann-Kiefer K, Rehm M, Welsch U, Conzen P, et al. Antithrombin Reduces Shedding of the Endothelial Glycocalyx Following Ischaemia/Reperfusion. Cardiovasc Res (2009) 83:388–96. doi: 10.1093/CVR/CVP097
99. Bruegger D, Rehm M, Jacob M, Chappell D, Stoeckelhuber M, Welsch U, et al. Exogenous Nitric Oxide Requires an Endothelial Glycocalyx to Prevent Postischemic Coronary Vascular Leak in Guinea Pig Hearts. Crit Care (2008) 12:1–11. doi: 10.1186/CC6913
100. van Golen R, Reiniers M, Vrisekoop N, Zuurbier C, Olthof P, van Rheenen J, et al. The Mechanisms and Physiological Relevance of Glycocalyx Degradation in Hepatic Ischemia/Reperfusion Injury. Antioxid Redox Signal (2014) 21:1098–118. doi: 10.1089/ARS.2013.5751
101. Abassi Z, Armaly Z, Heyman SN. Glycocalyx Degradation in Ischemia-Reperfusion Injury. Am J Pathol (2020) 190:752–67. doi: 10.1016/J.AJPATH.2019.08.019
102. Passov A, Schramko A, Mäkisalo H, Nordin A, Andersson S, Pesonen E, et al. Graft Glycocalyx Degradation in Human Liver Transplantation. PloS One (2019) 14:e0221010. doi: 10.1371/JOURNAL.PONE.0221010
103. Snoeijs MG, Vink H, Voesten N, Christiaans MH, Daemen J-WH, Peppelenbosch AG, et al. Acute Ischemic Injury to the Renal Microvasculature in Human Kidney Transplantation. Am J Physiol Renal Physiol (2010) 299:1134–40. doi: 10.1152/AJPRENAL.00158.2010
104. Sedigh A, Larsson R, Brännström J, Magnusson P, Larsson E, Tufveson G, et al. Modifying the Vessel Walls in Porcine Kidneys During Machine Perfusion. J Surg Res (2014) 191:455–62. doi: 10.1016/J.JSS.2014.04.006
105. Chen YC, Lu PH, Hsu JL, Yu CC, Guh JH. ICAM-1 and AMPK Regulate Cell Detachment and Apoptosis by N-Methyl-N′-Nitro-N-Nitrosoguanidine, a Widely Spread Environmental Chemical, in Human Hormone-Refractory Prostate Cancers. Toxicol Appl Pharmacol (2011) 257:412–9. doi: 10.1016/J.TAAP.2011.09.025
106. Klintman D, Li X, Thorlacius H. Important Role of P-Selectin for Leukocyte Recruitment, Hepatocellular Injury, and Apoptosis in Endotoxemic Mice. Clin Diagn Lab Immunol (2004) 11:56. doi: 10.1128/CDLI.11.1.56-62.2004
107. Antoniak S. The Coagulation System in Host Defense. Res Pract Thromb Haemostasis (2018) 2:549. doi: 10.1002/rth2.12109
108. Kenawy HI, Boral I, Bevington A. Complement-Coagulation Cross-Talk: A Potential Mediator of the Physiological Activation of Complement by Low Ph. Front Immunol (2015) 6:215/BIBTEX. doi: 10.3389/FIMMU.2015.00215/BIBTEX
109. Wu H, Ma J, Wang P, Corpuz TM, Panchapakesan U, Wyburn KR, et al. HMGB1 Contributes to Kidney Ischemia Reperfusion Injury. J Am Soc Nephrol (2010) 21:1878–90. doi: 10.1681/ASN.2009101048
110. Leventhal JS, Schröppel B. Toll-Like Receptors in Transplantation: Sensing and Reacting to Injury. Kidney Int (2012) 81:826–32. doi: 10.1038/ki.2011.498
111. Zhao G, Fu C, Wang L, Zhu L, Yan Y, Xiang Y, et al. Down-Regulation of Nuclear HMGB1 Reduces Ischemia-Induced HMGB1 Translocation and Release and Protects Against Liver Ischemia-Reperfusion Injury. Sci Rep (2017) 7:1–11. doi: 10.1038/srep46272
112. Arslan F, Keogh B, McGuirk P, Parker AE. TLR2 and TLR4 in Ischemia Reperfusion Injury. Mediators Inflamm (2010) 2010. doi: 10.1155/2010/704202
113. Wu H, Chen G, Wyburn KR, Yin J, Bertolino P, Eris JM, et al. TLR4 Activation Mediates Kidney Ischemia/Reperfusion Injury. J Clin Invest (2007) 117:2847–59. doi: 10.1172/JCI31008
114. Bergler T, Hoffmann U, Bergler E, Jung B, Banas MC, Reinhold SW, et al. Toll-Like Receptor 4 in Experimental Kidney Transplantation: Early Mediator of Endogenous Danger Signals. Nephron - Exp Nephrol (2013) 121:e59–70. doi: 10.1159/000343566
115. Hosgood SA, Moore T, Kleverlaan T, Adams T, Nicholson ML. Haemoadsorption Reduces the Inflammatory Response and Improves Blood Flow During Ex Vivo Renal Perfusion in an Experimental Model. J Trans Med (2017) 15:216. doi: 10.1186/s12967-017-1314-5
116. Dunkelberger JR, Song WC. Complement and its Role in Innate and Adaptive Immune Responses. Cell Res (2010) 20:34–50. doi: 10.1038/cr.2009.139
117. Danobeitia JS, Djamali A, Fernandez LA. The Role of Complement in the Pathogenesis of Renal Ischemia-Reperfusion Injury and Fibrosis. Fibrogenesis Tissue Repair (2014) 7:16. doi: 10.1186/1755-1536-7-16
118. de Vries RJ, Pendexter CA, Cronin SEJ, Marques B, Hafiz EOA, Muzikansky A, et al. Cell Release During Perfusion Reflects Cold Ischemic Injury in Rat Livers. Sci Rep (2020) 10:1–14. doi: 10.1038/s41598-020-57589-4
119. Stone JP, Ball AL, Critchley WR, Major T, Edge RJ, Amin K, et al. Ex Vivo Normothermic Perfusion Induces Donor-Derived Leukocyte Mobilization and Removal Prior to Renal Transplantation. Kidney Int Rep (2016) 1:230–9. doi: 10.1016/J.EKIR.2016.07.009
120. Jantsch J, Wiese M, Schödel J, Castiglione K, Gläsner J, Kolbe S, et al. Toll-Like Receptor Activation and Hypoxia Use Distinct Signaling Pathways to Stabilize Hypoxia-Inducible Factor 1α (HIF1A) and Result in Differential HIF1A-Dependent Gene Expression. J Leukocyte Biol (2011) 90:551–62. doi: 10.1189/JLB.1210683
121. Malkov MI, Lee CT, Taylor CT. Regulation of the Hypoxia-Inducible Factor (HIF) by Pro-Inflammatory Cytokines. Cells (2021) 10:2340. doi: 10.3390/CELLS10092340
122. Bongoni AK, Lu B, McRae JL, Salvaris EJ, Toonen EJM, Vikstrom I, et al. Complement-Mediated Damage to the Glycocalyx Plays a Role in Renal Ischemia-Reperfusion Injury in Mice. Transplant Direct (2019) 5:e341. doi: 10.1097/TXD.0000000000000881
123. Carlson K, Barbas A, Goldaracena N, Fernandez L, Al-Adra DP. Immunological Organ Modification During Ex Vivo Machine Perfusion: The Future of Organ Acceptance. Transplant Rev (2021) 35:100586. doi: 10.1016/J.TRRE.2020.100586
124. Yuzefovych Y, Valdivia E, Rong S, Hack F, Rother T, Schmitz J, et al. Genetic Engineering of the Kidney to Permanently Silence MHC Transcripts During Ex Vivo Organ Perfusion. Front Immunol (2020) 0:265. doi: 10.3389/FIMMU.2020.00265
125. Pool M, Eertman T, Parraga JS, Hart N‘t, Rhijn MR, Eijken M, et al. Infusing Mesenchymal Stromal Cells Into Porcine Kidneys During Normothermic Machine Perfusion: Intact MSCs Can Be Traced and Localised to Glomeruli. Int J Mol Sci (2019) 20:3607. doi: 10.3390/IJMS20143607
126. Reinders M, Groeneweg K, Hendriks S, Bank J, Dreyer G, de Vries A, et al. Autologous Bone Marrow-Derived Mesenchymal Stromal Cell Therapy With Early Tacrolimus Withdrawal: The Randomized Prospective, Single-Center, Open-Label TRITON Study. Am J Transplant (2021) 21:3055–65. doi: 10.1111/AJT.16528
127. Lohmann S, Pool M, Rozenberg K, Keller A, Moers C, Møldrup U, et al. Mesenchymal Stromal Cell Treatment of Donor Kidneys During Ex Vivo Normothermic Machine Perfusion: A Porcine Renal Autotransplantation Study. Am J Transplant (2021) 21:2348–59. doi: 10.1111/AJT.16473
128. Thompson ER, Bates L, Ibrahim IK, Sewpaul A, Stenberg B, McNeill A, et al. Novel Delivery of Cellular Therapy to Reduce Ischemia Reperfusion Injury in Kidney Transplantation. Am J Transplant (2021) 21:1402–14. doi: 10.1111/AJT.16100
129. Damman J, Seelen MA, Moers C, Daha MR, Rahmel A, Leuvenink HG, et al. Systemic Complement Activation in Deceased Donors is Associated With Acute Rejection After Renal Transplantation in the Recipient. Transplantation (2011) 92:163–9. doi: 10.1097/TP.0b013e318222c9a0
130. Jager NM, Poppelaars F, Daha MR, Seelen MA. Complement in Renal Transplantation: The Road to Translation. Mol Immunol (2017) 89:22–35. doi: 10.1016/J.MOLIMM.2017.05.014
131. Kassimatis T, Qasem A, Douiri A, Ryan EG, Rebollo-Mesa I, Nichols LL, et al. A Double-Blind Randomised Controlled Investigation Into the Efficacy of Mirococept (APT070) for Preventing Ischaemia Reperfusion Injury in the Kidney Allograft (EMPIRIKAL): Study Protocol for a Randomised Controlled Trial. Trials (2017) 18:255. doi: 10.1186/s13063-017-1972-x
132. Kassimatis T, Greenlaw R, Hunter JP, Douiri A, Flach C, Rebollo-Mesa I, et al. Ex Vivo Delivery of Mirococept: A Dose-Finding Study in Pig Kidney After Showing a Low Dose is Insufficient to Reduce Delayed Graft Function in Human Kidney. Am J Transplant (2020) 21:1021–26. doi: 10.1111/ajt.16265
133. Jochmans I, Nicholson ML, Hosgood SA. Kidney Perfusion. Curr Opin Organ Transplant (2017) 22:260–6. doi: 10.1097/MOT.0000000000000405
134. Hosgood SA, Thompson E, Moore T, Wilson CH, Nicholson ML. Normothermic Machine Perfusion for the Assessment and Transplantation of Declined Human Kidneys From Donation After Circulatory Death Donors. Br J Surg (2018) 105:388–94. doi: 10.1002/bjs.10733
135. Minor T, Lüer B, von Horn C, Paul A, Gallinat A. Effect of Oxygen Concentration in Anterograde Liver Persufflation on High Energy Phosphates and Graft Function After Ischemic Preservation. Cryobiology (2020) 92:248–50. doi: 10.1016/J.CRYOBIOL.2020.01.020
136. Kron P, Schlegel A, Muller X, Gaspert A, Clavien P, Dutkowski P. Hypothermic Oxygenated Perfusion: A Simple and Effective Method to Modulate the Immune Response in Kidney Transplantation. Transplantation (2019) 103:e128–36. doi: 10.1097/TP.0000000000002634
137. Thuillier R, Allain G, Celhay O, Hebrard W, Barrou B, Badet L, et al. Benefits of Active Oxygenation During Hypothermic Machine Perfusion of Kidneys in a Preclinical Model of Deceased After Cardiac Death Donors. J Surg Res (2013) 184:1174–81. doi: 10.1016/j.jss.2013.04.071
138. Jochmans I, Brat A, Davies L, Hofker HS, van de Leemkolk FEM, Leuvenink HGD, et al. Oxygenated Versus Standard Cold Perfusion Preservation in Kidney Transplantation (COMPARE): A Randomised, Double-Blind, Paired, Phase 3 Trial. Lancet (2020) 396:1653–62. doi: 10.1016/S0140-6736(20)32411-9
139. Husen P, Boffa C, Jochmans I, Krikke C, Davies L, Mazilescu L, et al. Oxygenated End-Hypothermic Machine Perfusion in Expanded Criteria Donor Kidney Transplant: A Randomized Clinical Trial. J Am Med Assoc Surg (2021) 156:517–25. doi: 10.1001/JAMASURG.2021.0949
140. Lerink LJS, de Kok MJC, Mulvey JF, le Dévédec SE, Markovski AA, Wüst RCI, et al. Preclinical Models Versus Clinical Renal Ischemia Reperfusion Injury: A Systematic Review Based on Metabolic Signatures. Am J Transplant (2021) 00:1–27. doi: 10.1111/AJT.16868
Keywords: hypothermic machine perfusion, ischemia-reperfusion injury, HIF, cell death, endothelial dysfunction, immune response
Citation: Knijff LWD, van Kooten C and Ploeg RJ (2022) The Effect of Hypothermic Machine Perfusion to Ameliorate Ischemia-Reperfusion Injury in Donor Organs. Front. Immunol. 13:848352. doi: 10.3389/fimmu.2022.848352
Received: 04 January 2022; Accepted: 04 April 2022;
Published: 29 April 2022.
Edited by:
Stefan Schneeberger, Innsbruck Medical University, AustriaReviewed by:
Luuk Hilbrands, Radboud University Nijmegen, NetherlandsRoss Francis, Princess Alexandra Hospital, Australia
Copyright © 2022 Knijff, van Kooten and Ploeg. This is an open-access article distributed under the terms of the Creative Commons Attribution License (CC BY). The use, distribution or reproduction in other forums is permitted, provided the original author(s) and the copyright owner(s) are credited and that the original publication in this journal is cited, in accordance with accepted academic practice. No use, distribution or reproduction is permitted which does not comply with these terms.
*Correspondence: Rutger J. Ploeg, cnV0Z2VyLnBsb2VnQG5kcy5veC5hYy51aw==; Cees van Kooten, Yy52YW5fa29vdGVuQGx1bWMubmw=