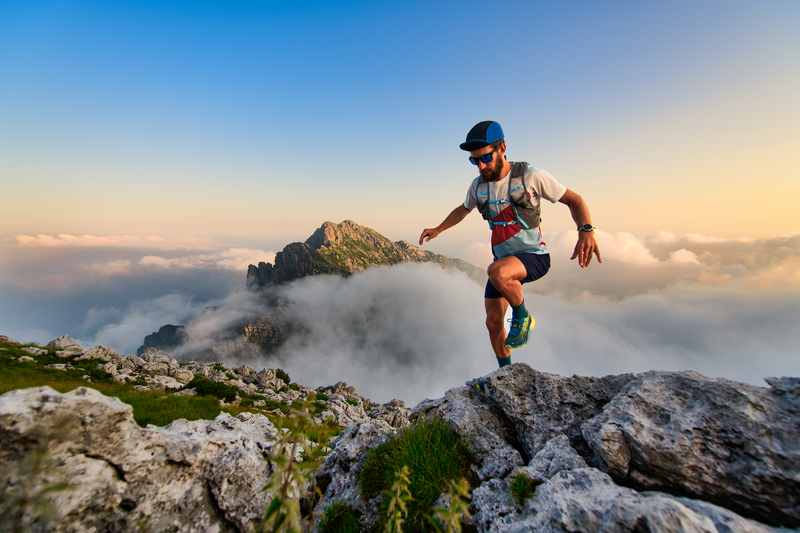
94% of researchers rate our articles as excellent or good
Learn more about the work of our research integrity team to safeguard the quality of each article we publish.
Find out more
REVIEW article
Front. Immunol. , 08 April 2022
Sec. Alloimmunity and Transplantation
Volume 13 - 2022 | https://doi.org/10.3389/fimmu.2022.846032
This article is part of the Research Topic Progress of Allo- and Xeno-transplantation Facilitating the Initial Xeno-Kidney and Islet Clinical Trials View all 16 articles
Organ transplantation has evolved rapidly in recent years as a reliable option for patients with end-stage organ failure. However, organ shortage, surgical risks, acute and chronic rejection reactions and long-term immunosuppressive drug applications and their inevitable side effects remain extremely challenging problems. The application of nanotechnology in medicine has proven highly successful and has unique advantages for diagnosing and treating diseases compared to conventional methods. The combination of nanotechnology and transplantation brings a new direction of thinking to transplantation medicine. In this article, we provide an overview of the application and progress of nanotechnology in kidney and islet transplantation, including nanotechnology for renal pre-transplantation preservation, artificial biological islets, organ imaging and drug delivery.
As a reliable survival choice for patients with end-stage organ failure, organ transplantation has progressed markedly in the era of modern medicine. With progress in surgical approaches, anesthesia, nursing and the addition of immunosuppressants, the survival of grafts has markedly improved. However, with the extension of human life expectancy and the increasing incidence of chronic diseases, the demand rate for organ transplantation may also increase, which will further aggravate the serious imbalance between organ demand and supply, the most important issue in transplantation medicine (1–3).
In addition to organ shortages, other challenges, such as the risk of transplantation, immune rejection, long-term immunosuppressants and their inevitable side effects, will limit the application of transplantation technology (2, 4–6). Fortunately, the combination of emerging technologies with transplantation approaches is influencing traditional transplantation methods (7), such as single-cell sequencing technology being used to determine cell heterogeneity and new molecular characteristics for immune rejection and CRISPR/cas9 gene-editing technology being used to generate transgenic pigs to resolve issues of organ shortage (8, 9).
Nanotechnology has developed rapidly in the past few decades and has its applications in many fields, including medicine. Nanomaterials are usually divided into metallic, organic and semiconducting particles, with sizes ranging from 1 to 100 nm. The main purpose of nano medicine is to diagnose and treat diseases at the nano level using the properties and properties of nano materials (10). For example, nanomaterials are used as carriers to achieve drug targeting and controlled release (5, 10), improve pharmacokinetics (11) and reduce drug toxicity and side effects (12, 13). They have also been used for direct tumor treatment (14) and molecular imaging (15). Nanomedicine has unlimited potential. Its application in transplantation medicine overcomes the obstacles of many traditional transplantation methods, such as optimizing the pharmacokinetics of immunosuppressive drugs, improving organ or tissue preservation, aiding in the generation of artificial biological organs and improving organ imaging (5, 16–18).
We focused on the outcomes of combining nanotechnology with kidney transplantation and islet transplantation and summarized the current popular applications and latest progress in this field.
Kidney transplantation is the most promising way to cure renal failure, and dialysis is one way to extend the survival of patients who must wait because of the scarcity of kidney sources. However, dialysis inevitably transforms the daily lives of patients.
The combination of silicon nanotechnology and tissue engineering has given birth to a device that has changed the current situation: the IAK. The HemoCartridge of the IAK is responsible for filtration, and the IAK also possesses a bioreactor to culture renal tubular cell epithelium (BioCartridge). Benefiting from silicon nanotechnology, each microchip in the HemoCartridge forms a filtration channel resembling a glomerular slit diaphragm that can block molecules such as albumin and allow waste filtration of small molecules. The device, which is implanted surgically, uses blood pressure for filtering power, not relying on an electric pump and dialysate, and simulates the natural kidney function (Figure 1). This technology is of great significance for patients with chronic kidney disease. The implantation of an artificial kidney can greatly reduce the urgent need for renal transplantation and alleviate the current pressure of renal scarcity (19–24).
Figure 1 Arterial blood (red) is filtered through the Iak and the generated urine passes through a tubing (yellow) into the bladder. Filtered blood flows back into the vein (blue).
An additional way to alleviate the pressure associated with the kidney transplant donor shortage is the acceptance of less-ideal organs, such as the gradually increasing number of kidneys that are donated after circulatory death (DCD). The issue with DCD kidneys is their longer warm ischemic time, leading to injury. The effects of such injury are diverse. The kidney is particularly prone to acute tubular necrosis, resulting in a delay in the renal function recovery, while the pancreas is more prone to graft thrombosis and islet loss (25–27). However, these should not be reasons for abandoning DCD organs. The experience with the UK DCD kidney transplantation program supports the utilization of DCD organs to help alleviate the global shortage of kidneys from deceased donors (17, 28).
Organ preservation is an important step before transplantation. At present, the main goal is to preserve the function of organs after they are removed from the body and reduce the injury of ischemia and reperfusion (29). SCS, which is easy to operate and inexpensive, has become the main method of organ preservation in solid organ transplantation. However, in the era of the increasing use of DCD organs, the preservation effect has been unsatisfactory.
Organ damage due to hypoxia as well as reperfusion is particularly pronounced in DCD organs. This has necessitated revisiting organ preservation methods that predate SCS, with a renewed emphasis on organ preservation to reduce organ damage from ischemia-reperfusion injury(IRI). A prospective study on HMP carried out by Moers et al. pulled the prelude to the use of mechanical perfusion for clinical organ preservation. Their team randomly divided the kidneys of 336 deceased donors into HMP and SCS groups and then transplanted the kidneys into 672 subjects. After one year of follow-up, it was found that perfusion reduced the incidence and extended the duration of a delayed graft function. The one-year graft survival was also better than that in the SCS group (30).
Since HMP does not completely mimic the organ circulatory environment in a physiological state, the effect of cold ischemia on graft injury as well as adverse graft outcomes has been noted. Therefore, NMP, which lacks the disadvantages of cold ischemia, has attracted widespread interest (31).
Arnaud et al. performed orthotopic transplantation after NMP of isolated rabbit kidney, suggesting that this method for organ preservation may be ideal for improving the current state of DCD organ preservation (32). Not only do normothermic perfused kidneys suffer less endothelial cell damage, but the partial damage induced by cold ischemia can be reversed, and the metabolic function of the organ can be restored as well, thereby improving the final transplantation outcome (33–35). Given the good results obtained in animal trials, NMP has been used in clinical trials with marginal kidneys, further demonstrating the superiority of this approach (36, 37).
The exploration of the utility of NMP continues. After Kaths et al. found that prolonged NMP in isolated porcine kidneys resulted in better graft outcomes than either SCS alone or that combined with short-term NMP (38). AnnemarieWeissenbacher’s group were further explored in human kidney with developing an automated normothermic perfusion system. This research group found a trend toward improvement in kidney quality after prolonged perfusion(24 hours) (39).
Hypoxia impairs the endothelial cell function, promotes inflammation and procoagulant activity and leads to vascular convergence, which is considered key to IRI (40–42). Although these processes can be avoided to some degree with the use of unfractionated heparin, the risk of systemic bleeding must still be considered. Semisynthetic antiplatelet and anticoagulants (APACs) have been found to protect the kidney from moderate and severe IRI by reducing adaptive immune activation and vascular injury, but the systemic risk of systemic administration still needs attention (43, 44). The MP, particularly NMP is more than a preservation benefit to the graft, and more importantly, treatment and handling of the graft before transplantation can be performed during the perfusion period, further improving graft efficacy (45). It may be a more effective way to use mechanical perfusion as a vehicle combined with drugs targeting endothelial cells(ECs) to help reduce the local IR response. Hamaoui et al. used thrombalexin (TLN) for local anticoagulation during HMP, which confirmed the feasibility of HMP as a vehicle. This method promoted the adhesion between TLN and its target to improve organ perfusion (46).
A rather promising direction for combination nanotherapeutics during machine perfusion. Machine perfusion provides delivery motivation for nanotherapeutics, whereas nanotherapeutics can accomplish treatment of more targeted pretransplant grafts during machine perfusion.
Devalliere et al. used biodegradable PLGA polymer nanoparticles to wrap microRNA-132, which can promote angiogenesis. At the same time, this complex was combined with cyclic RGD (cRGD) peptides to promote its uptake by ECs, and was then implanted into ECs before transplantation, and then the collagen-fibronectin-based scaffold was used to control the transplantation site of ECs. The slowly released RNA then continuously promotes angiogenesis at the target site (47).
Nanoparticles will combine with proteins in organisms to form a coating called protein corona on the surface. This reaction will greatly hinder the normal function of nanoparticles, and may also cause the death of endothelial cells (48). The use of NPs to treat grafts during mechanical perfusion can help prevent the aggregation of NPs in the macrophage system and the “protein corona” caused by serum proteins. Even so, there is still room for improving the targeting ability of NPs. Ensuring that NPs accumulate in target cells to influence perfusion remains challenging. Tietjen et al. bound the anti-human CD31 antibody to NPs and applied it to human kidneys during the machine perfusion phase. The experimental results showed that, compared with non-targeted NPs, targeted NPs had a significantly enhanced enrichment effect, but this effect was weakened by nonspecific aggregation. The nonspecific aggregation of NPs is particularly significant in areas with insufficient blood perfusion, which is a key factor hindering the role of therapeutic NPs. But, however, it can provide direction for predicting the therapeutic effect of NPs or evaluating organ function in the future (49).
Improving the targeting effect of NPs during NMP requires not only modifying NPs but also improving the microenvironmental status of the organ itself. Areas of hypoperfusion were found to have significant microvascular obstruction. Indeed, DiRito et al. found that the microvascular obstruction in the kidney was due to fibrin (ogen) from the proximal tubular epithelium, and treatment with a tPA+plasminogen regimen prior to transplantation resulted in improved microvascular obstruction and vascular targeting capability of the NPs (50). During NMP, a large number of nanoparticles are released, including kidney-derived extracellular vesicles (EVs). These nanoparticles can help clarify the status of the kidney and are a promising means of conducting a evaluating the renal function before transplantation (51).
As an effective means of improving the outcome of transplantation, nanotherapy before transplantation has attracted increasing attention. Zhu et al. used micelles to encapsulate rapamycin and modified the particles to enable targeting of endothelial and epithelial cells. The addition of targeted rapamycin micelles (TRAMs) to standard organ preservation solutions as a pre-transplant preservation regimen helps prevent downstream organ dysfunction and protects cells in vitro while preventing the vascular injury and fibrosis resulting from chronic rejection in vivo (52). PACE nanoparticles loaded with siRNA targeting CIITA were able to be delivered into organs via machine perfusion. Those nanoparticles enabled the efficient encapsulation and controlled release of siRNA, thereby facilitating the prolonged ongoing silencing of proteins of interest to protect organs from acute rejection after transplantation (53).
The importance of graft treatment before transplantation is self-evident, especially for DCD organs. We should thus seek out new renal-related nanoparticles that are more useful for evaluating the renal quality during perfusion, improving the microcirculation according to the evaluation results and then conducting NMP combined with NPs for targeted pre-transplantation treatment in order to further improve the graft prognosis.
T1DM is a heterogeneous autoimmune disease characterized by a decrease in the number of pancreatic β cells, resulting in insulin secretion dysfunction (54). For such patients, long-term glycemic control, disease management and complication prevention are important. Long-term use of exogenous insulin is an effective way to control blood glucose in such patients; however, while several options are currently available, adverse effects, such as injection site pain, inflammation and hypoglycemia, cannot be avoided (55). In contrast, islet transplantation is believed to be promising for refining physiological insulin secretion and glycemic control, thereby avoiding long-term dependence on exogenous insulin and associated complications; furthermore, it is less demanding than pancreas transplantation, as patients do not need to undergo major surgery (56, 57).
The Edmonton protocol confirmed islet transplantation as a promising approach for restoring endogenous insulin secretion in T1DM to control blood glucose; however, this regimen was unable to maintain insulin independence in the long term and still required the administration of strong immunosuppressive therapy (58). The side effects of long-term immunotherapy cannot be ignored, so how to further reduce the graft’s immune response within the recipient is the primary issue to address in order to improve the outcomes of islet transplantation.
Encapsulation of islets with semipermeable membranes for immunosuppressive sequestration is a promising approach to achieving immunosuppressant-free transplantation (59). Lim and Sun were the first to use sodium alginate to encapsulate pancreatic islets, and their method prevented the direct contact of immune components with the islet cells without the use of immunosuppressive agents (ISAs), thereby reducing the immune response while not affecting the nutrient uptake by the pancreatic islets (60). Most subsequent studies achieved control over the cyst wall thickness and microcapsule size, improved the overall durability and reduced toxicity of the material itself for the islets of Langerhans by optimizing the encapsulated material (61–63). However, due to biocompatibility issues, while direct immune reactions with cells are avoided, the body’s nonspecific foreign body reaction to the microcapsules can lead to the proliferation of fibers, which can lead to disorders in the communication of the islets with external nutrients and eventually cause necrosis of the islets within the microcapsules (64, 65).
Anti-inflammatory-related drugs have benefits in reducing fibroproliferation, but the extent to which different drugs are effective is unknown, and adverse drug reactions caused by the way in which they are administered systemically are difficult to avoid. To this end, Dang et al. performed an intravital subcutaneous screen of 16 anti-inflammatory drugs and found that dexamethasone and curcumin had the strongest inhibitory effect on early inflammatory proteases and reactive oxygen species (ROS). Therefore, co-encapsulating the drug with the islets in alginate microcapsules, confirming that localized drug release inhibited fibrosis around the microcapsules can improve the control of blood glucose by the microencapsulated islets (64). This issue was similarly explored by Azadi et al., who designed an alginate/dextran-spermine microcapsule encapsulating pancreatic islets along with pentoxifylline (PTX), an anti-inflammatory drug. This therapeutic structure was not only able to effectively shield the islet cells inside the microcapsules but also alleviated the inflammatory response around the microcapsules and reduced fibroproliferation (66).
Approaches to reduce the immunoinflammatory response around microcapsules, such as by optimizing the material to improve biocompatibility or by adding anti-inflammatory-related drugs, are reassuring for improving the outcomes of microcystic islet transplantation. Nevertheless, a large number of empty microcapsules may be left behind during islet microencapsulation, which increases the overall graft volume and leads to a stronger host immunoinflammatory response.
Espona et al. combined superparamagnetic iron oxide nanoparticles (SPIONs) with microfluidic technology to purify magnetically labeled pseudoislet-containing microcapsules in order to achieve automated screening of empty microcapsules, thereby reducing the probability of technical errors, and the microcysts that remained after screening greatly reduced the graft size (by over 75%) (67). Large volumes of capsules containing islets still need to be invested in order to achieve therapeutic purposes. The retrieval of these capsules after graft failure or loss of the graft function is an issue that needs to be urgently addressed. Delcassian developed a new encapsulated cell therapy with capsules that could be retrieved magnetically after transplantation by loading carboxylated iron oxide nanoparticles (NP-COOH) into encapsulated islet alginate hydrogels. This strategy preserved the encapsulated functionality (i.e. no need for immunosuppressive therapy for six to eight weeks) while guaranteeing the functionality of the transplanted islets, and no long-term leaching of the nanoparticles was noted (68).
Although microencapsulation has proven effective in islet encapsulation, the issue of an excessive volume of microcapsules remains difficult to overcome due to the fabrication process (69, 70). An excessive microcyst volume can affect islet cell metabolic processes and limits the choice of transplant site, such as preventing transplantation to the most desirable site—the portal vein—and forcing the selection of sites such as the peritoneal cavity, where blood supply is not abundant (71), ultimately risking graft failure due to hypoxia. A much more refined encapsulation strategy is therefore needed to solve this problem.
LBL polymer self-assembly enables the preparation of nano-sized thin film coating and is a reasonable approach for downsizing encapsulated islets, and can effectively block the attack of immune components (Figure 2). Wilson et al. constructed a nano coating rich in PEG through LBL self-assembly of poly(L-lysine)-g-poly (ethylene glycol) (biotin) (PPB) and streptavidin (SA) and found that this coating did not cause a loss of islets while guaranteeing the immune isolation of islet cells. At the same time, nutrient exchange was not affected, nor was the islet viability or function (72). This encapsulation method can be performed in the nanometer range without concerns regarding diffusion barriers while at the same time greatly reducing the volume of transplanted islets, thereby allowing transplantation to the portal vein.
Figure 2 The combined application of LBL structure and immune preparation can effectively realize the destruction of islet cells by immunity.
The feasibility of LBL islets was first validated in an NHP model by Haque et al. They covalently grafted three layers of PEG onto the surface of pancreatic islets via LBL technology, achieving a nano-shielding effect. The shielded islets were subsequently transplanted, and studies showed that the LBL islets were less immunogenic, did not have their activity or function affected, had a higher survival rate than non-LBL islets and suffered relatively little immune damage after the application of a glucocorticoid-free immunosuppressive drug regimen, demonstrating the feasibility of this transplantation strategy (73).
To improve the transplanted islet function, Kizilel constructed a nano thin multilayer film of biotin-PEG-NHS/SA/biotin-PEG-GLP-1 via the LBL method. This biofunctional multilayer film immobilized GLP-1 on the surface of pancreatic islets and not only improved the islet survival but also promoted insulin secretion, and the response time to glucose was not affected. This coating may reduce the number of islets required, which may help mitigate issues associated with the shortage of islet donors, while also decreasing the volume of the graft and thereby reducing the risk of an elevated portal pressure after transplantation (74).
The instant blood-mediated inflammatory reaction (IBMIR) is a major cause of early and massive loss of intraportal transplanted islets and must be addressed in order to successfully initiate intraportal islet transplantation. This problem attracts the attention of a wide range of researchers, and many protocols are proved to be effective in reducing inflammatory injury (Table 1). Im et al. used 8-arm-PEG-catechol (PEG8) and N-hydroxysuccinimidyl-linked unfractionated heparin (UFH-NHS) to construct a dual-islet shielding system that effectively inhibited the transplanted islet mediated immune response and IBMIR, an effect that was further strengthened by adding the immunosuppressive agent FK506 (75). Haque et al. used polyethylene glycol plus heparin (heparin nano-shielded islets; HNSIs) in an LBL fashion to shield NHP islets, a protocol that not only blocks islet damage by immune cells but also functions in combination with most current immunosuppressive regimens, demonstrating its reliability in NHP models (76).
Islets remain vulnerable for most of the early period after transplantation due to the loss of their microenvironment. Restoring this microenvironment would thus be conducive to the islet survival and functional recovery. Therefore, Haque et al. constructed a hyperbranched polyethylene glycol (hb-PEG)/Heparin(Hep) nano-encapsule to promote the islet cell survival by shielding them from immune attack, a strategy that reduced IBMIR injury by mitigating complement activation and proinflammatory cytokine generation. This regimen in combination with immunosuppressive drugs had an even stronger effect of preserving the graft survival. The effect of hb-PEG/Hep nano-encapsules on the reestablishment of the islet microenvironment is not a direct effect, instead indirectly helping the islets recreate their own microenvironment by supporting the islet survival (77). Unlike the native environment under physiological conditions, transplanted islets are often in an inflammatory microenvironment, which directly affects the islet transplant survival. Izadi et al. developed a method for modifying the islet surface by coupling NHS groups on PEG to primary amines in the Jagged-1 (JAG-1) structure to immobilize JAG-1 on the surface of islets PEGylated, and these JAG1-PEG islets showed a greater ability to activate the Notch signaling pathway than untreated islets. Increasing CD4+, CD25+ and FOXP3+ cells as well as the IL-10 and TGF-β production while decreasing the INF-γ and TNF-α generation, which reverses the inflammatory microenvironment to an anti-inflammatory one, promotes the formation of an immunotolerant microenvironment that protects pancreatic islets from inflammatory injury (78).
Kozlovskaya et al. used a non-ionic hydrogen-bonded LBL technique to produce a novel and versatile cytoprotective coating that is more efficient than the conventional ionic LBL-based method. This method can be performed in a physiological state, and the resulting islets are uniformly coated with a non-toxic and non-immunogenic layer. They can stably exist in vitro without affecting the islet function or viability, supporting the modification of functional molecules and also playing a role in inhibiting proinflammatory cytokine synthesis (79).
An insufficient antioxidant capacity of islet cells and oxidative stress damage after transplantation are important causes of a poor function of transplanted islets as well as their death. Abuid et al. generated a nanoscale antioxidant coating through LBL assembly of cerium oxide nanoparticles (CONPs) and alginate hydrogels, a strategy that effectively and durably protected the encapsulated cells from ROS-mediated damage while preserving the cellular metabolism and ability to respond to glucose stimuli. The effect of protection correlates with the number of layers, and it has been shown that 12 layers of CONP/alginate hydrogels coatings have a stronger effect than 2 or 6 layers (80).
Syed et al. used electrostatic bonding technology to deposit two differently charged polymers (positively charged chitosan and negatively charged polystyrene sulfonate sodium salts [PSS]) for the multi-layer-by-layer (MLBL) encapsulation of islets. The MLBL islet activity and function were unaffected, and cells were protected from inflammatory cytokine damage. The transplanted islets showed an improved glucose tolerance and had a significant ability to reduce and stabilize blood glucose levels. A longer time after transplantation can also ensure the survival and morphology of islets and reduce the infiltration of immune cells (81).
The LBL method for constructing pancreatic islet cell coatings often utilizes electrostatic interaction, a promising and helpful approach, but the long-term stability and biocompatibility are issues that remain to be resolved. Gattás-Asfura et al. explored the engineering covalently-stabilized LBL (CLBL) coating approach, which used triethoxysilane with a functionalized poly (amino amides) (PAMAM) dendrimer to enhance the coating stability. Encapulation via the CLBL approach allowed for islet engraftment at sites such as the kidney capsule, with no significant difference from untreated islets in the ability to restore normoglycemia or stabilize blood glucose levels and also support islet re revascularization (82).
Despite continuous advances in technology for islet encapsulation, the paucity of islet donors remains a barrier to the widespread use of artificial islets. Porcine insulin is structurally very similar to human insulin, and the porcine islet supply is not an issue at present, as access is very easy to achieve, making porcine islets a reliable replacement for human ones (83, 84). Porcine islets are derived from high-quality donors, unlike human donors, who are often elderly or deceased, and porcine islets can be modified by genetic engineering to reduce the immune response, resulting in an improved transplant success (85).
However, immune rejection remains a major obstacle to porcine islet xenotransplantation. Matsumoto et al. used alginate to wrap neonatal porcine islets and implanted them intraperitoneally in patients with T1DM in an experimental group. Their findings suggested undetected porcine endogenous retrovirus (PERV) infection in all subjects, with the HbA1c level maintained at <7%, thus significantly reducing hypoglycemic events. Longer-term maintenance was feasible, as no ISAs were applied, suggesting that immune shielding of porcine islets is an effective approach to address xenogeneic rejection (86). Lew et al. constructed exenatidel-loaded poly (lactic-co-glycolic acid) microspheres after determining the suitable drug-loading capacity and coencapsulated them in alginate microcapsules with porcine islets. The addition of exenatide reduced the rate of islet cell death and improved the stimulatory response to glucose, further enhancing the transplantation success of microencapsulated porcine islets (87).
LBL technology-based nanofilms as well as islet surface modification are an additional option for porcine islet xenotransplantation. Haque et al. combined SH-6-arm-peg-lipid and gelatin-catechol to construct a bilayer structured artificial extracellular matrix for encapsulating and stabilizing porcine islets against cell detachment. In parallel, the use of PEG-derivative encapsulation for islet camouflage reduced the overall immunogenicity and adhesion of serum albumin, fibronectin and immunoglobulin G (IgG). A cocktail immunosuppressant treatment regimen has been adopted after xenoimplantation, which can further inhibit the activation of immunity (83).
Neonatal porcine islet-like cell clusters (NPCCs) are popular because of their low price, ease of isolation and proliferative capacity, but they cause early loss of the graft and subsequent graft failure due to a transplant immune response, such as IBMIR or hyperacute rejection (88, 89). Nanoencapsulation can cope well with these problems, but it is still inescapable for damage in NPCCs encapsulation. Lee et al. focused on how to optimize nanoencapsulation for NPCCs to guarantee the quality of encapsulated islets and avoid unnecessary islet loss. They obtained more stable nanocapsules by inducing cross−linking between bifunctional psomes (NHS-/NH2 psomes) and also found that entrapment in F-10 medium at pH 7.3 could reduce the NPCC damage, while a higher yield of NPCCs could be achieved after adding 0.25% bovine serum albumin (90).
Nanotechnology attempts in optimizing islet transplantation are multidirectional and multiangular. Bilirubin is a powerful antioxidant, and its intravenous administration has been shown to reduce apoptosis and levels of inflammatory mediators in order to increase the graft survival in a murine transplant model. However, bilirubin is not readily soluble in water under physiological conditions and has a low bioavailability, and its repeated intravenous injections to maintain effective concentrations carry a risk of toxicity (91–93). Fullagar et al. encapsulated bilirubin into Pluronic F127-chitosan NPs to construct bilirubin nanoparticles. This nanodrug delivery strategy increased the bilirubin uptake and bioavailability by pancreatic islet cells and provided protection to pancreatic islet cells during times of oxidative stress (94). Huang et al. tried to control the insulin secretion process from the implanted islets. They entrapped islets using SA-PEI-melanin composite threadlike hydrogels, an approach that has good biocompatibility and enables effective immune shielding. The introduction of melanin nanoparticles can stimulate insulin secretion and further regulate insulin secretion via near-infrared (NIR), achieving a better control of blood glucose levels. Furthermore, they also have retrievable functions (95). Cheng et al. developed an efficient method of islet cryopreservation by combining microfluidic encapsulation systems, cold-responsive nanocapsules (CR-NCS) as well as calcium alginate hydrogel (CAH) embedding. Calcium alginate substitutes traditional toxic CPAs as islet cell cryoprotectants. CAH is responsible for protecting cells during the freeze-thaw process while providing a barrier to the transplanted islets. The islet cell function was preserved under this strategy, and a good glucose-lowering effect was still seen after implantation into diabetic rats. This method is expected to aid in the establishment of islet tissue banks, solve the issue of time lag between donors and recipients and help further mitigate the donor shortage (16).
Furthermore, pancreatic tissue engineering is a promising area for islet transplantation. Elham hoveizi et al. obtained nanofibrous PLA/Cs scaffold by electrospinning technique and incorporated zinc oxide nanoparticles (nZnO) into this 3D culture structure. The results demonstrate that this strategy can provide a favorable nano environment for Endometrial stem cells(EnCSs) differentiation into insulin-producing cells (IPCs) (96). Yung Chih Kuo’s group then found that pancreatic islet cells could be generated from IPCs in activin A-grafted gelatin-PLGA NP scaffold by controlling appropriate concentrations of activin A, LY294002, and retinoic acid (RA) (97).
For patients with chronic pancreatitis, on the one hand, long-term chronic pain seriously affects the quality of life of patients. On the other hand, chronic inflammation leads to progressive destruction of pancreatic islets and finally causes diabetes. Total-pancreatectomy (TP) with intraportal-islet-auto-transplantation (IAT) is one of the options for the treatment of chronic pancreatitis. This treatment modality provides pain relief and preserves meaningful islet function (98–100). The introduction of nanotechnology to improve the effect of autologous islet transplantation is a worthy direction for further optimization of this therapeutic approach.
From the use of alginate as a representative microencapsulation tool to the application of LBL technology for successful transplantation from allogeneic to xenografts, from NHP models to humans, from the optimization of encapsulation materials to emphasizing the encapsulation process, optimization of islets themselves, and islet preservation techniques, the continuous advancement and optimization of artificial biological islets have made islet transplantation an important strategy for the treatment of T1DM.
The rejection between donor and recipient of transplanted organs and the functional quality defects of transplanted islets may eventually lead to the failure of the whole transplant treatment program. Therefore, it is necessary to perform timely intervention according to the real-time state of the transplanted islets.
Real-time monitoring of the activity and function of the transplanted islets is critical for the treatment of T1DM. At present, the evaluation of the islet graft function mainly depends on the measurement of clinical biochemical indexes, including C-peptide levels, fasting and stimulated glucose levels and oral/intravenous glucose tolerance testing (101). However, these indexes can only indirectly reflect the functional status of islet grafts. Therefore, the establishment of a non-invasive real-time quantitative technique for monitoring the islet graft survival is important for the clinical application of islet transplantation (Table 2).
MRI is a reliable non-invasive imaging method for monitoring the efficiency of islet transplantation. It has outstanding clinical benefits, such as no harm to the patient due to ionizing radiation, a high representativeness of reuse, a high imaging resolution, deep tissue penetration and strong tomographic imaging ability. It has been widely used in the imaging-based monitoring of islet transplantation (102).
Because of the similar density of pancreas and liver on MRI, it is difficult to observe islet cells transplanted through the hepatic vein, so contrast agent is needed to distinguish them. Although islet cell labeling requires an effective uptake of MRI contrast agents, common commercial magnetic nanoparticles cannot be effectively introduced into cells, which has a great impact on imaging.
SPIO has become a widely used MRI contrast agent in clinical settings because of its low toxicity and high sensitivity (103). Koblas et al. showed that the labeling of islet cells with SPIO had no effect on the survival rate, activity or function of transplanted islets. Subsequently, Toso et al. confirmed for the first time in clinical practice that real-time imaging monitoring of islet cells by MRI with SPIO labeling was completely safe and feasible (104). Koichi et al. developed six kinds of magnetic iron oxide nanoparticles coated with different dextrans. The results suggested that these new positively charged nanoparticles might be useful MRI contrast agents for monitoring the islet quality after transplantation, thus confirming that MRI using SPIO nanoparticles contrast agent is a viable tool for in vivo monitoring of the islet quality (105).
Some studies on MRI have shown that, after labeling islet cells with these new positively charged nanoparticles, the T1- and T2-weighted imaging signals of islet transplantation sites in mice decreased significantly. However, this result is caused by the transplantation of islets into the subcapsular space of the kidney. If the islets are placed in the porta hepatis commonly used in clinical settings, more artifacts will occur due to the high iron content in the liver (106). This is an urgent problem to be solved in the application of this new positively charged nanoparticle technology in MRI.
In the study of Toso et al., the intensity of the images of three patients before islet transplantation was normal, and the iron-loaded islets were recognizable as low-intensity spots in the liver after transplantation. Another patient had a diffuse low-signal image on baseline liver MRI, so transplant-related changes could not be observed. Iron overload (spontaneous or induced) is the main obstacle to MRI with iron-containing contrast medium (104). Researchers have thus conducted a large number of studies on the modification, improvement and replacement of SPIO contrast media, with some breakthroughs made, and a large number of new SPIO contrast agents have been developed and put into clinical use.
IBMIR is one of the main inflammatory reactions that occurs after pancreatic islet transplantation through the hepatic portal vein, leading to the activation of platelets as well as the coagulation and complement systems. This platelet-amplified reaction creates activated platelets bind to the islets and contribute to a continuous fibrin formation, which can damage the transplanted islets, potentially causing an early function loss, and poses a serious challenge for clinical islet cell replacement therapy. To prevent such islet transplantation failure, a new type of heparin-immobilized HSPIO nanoparticles with anticoagulant activity was developed, and its chemical coupling can be used to camouflage the surface of islet cells. Yong et al. found that HSPIO nanoparticles were able to prevent IBMIR in vivo and in vitro, and the implantation of such labeled islets significantly reduced the blood glucose level of diabetic animals (107). Such HSPIO was also shown to be quickly eliminated after portal vein injection, did not accumulate in the liver, and was able to be used to track fixed islets, showing both MRI sensitivity and anticoagulant activity. It therefore seems to be a promising clinical choice for a new type of SPIO contrast agent with remarkable future prospects.
The biggest difference between USPIO and SPIO lies in their diameters: the diameter of USPIO is less than 50 nm, while that of SPIO is more than 50 nm. As a biodegradable preparation, USPIO is safer than SPIO and non-toxic, showing a long blood half-life, good biocompatibility, and an ability to be swallowed by macrophages without cell activation.
However, most reports thus far on USPIO have focused on the evaluation of kidney transplantation. Stoumpos et al. found that ferumoxytol-enhanced magnetic resonance angiography (FeMRA) was feasible and practical for vascular imaging in patients with advanced renal transplantation. It has the advantage of obtaining arteriography and venography findings at the same time without inducing nephrotoxicity (108). Maryam et al. found that ferumoxytol was not retained in renal allografts in patients with acute rejection, and that renal allografts with acute rejection showed a prolonged T2 * value compared with non-rejection allografts (109).
It was recently reported for the first time that the USPIO nanoparticle Ferumoxtran-10 can be used in the clinical treatment of islet inflammation in patients with T1DM. In addition, it is a feasible contrast agent for the commercial use of USPIO, receiving approval from the US Food and Drug Administration (FDA) in 2005 (110). Bin et al. found that using a new type of Bcl-2-functionalized PEG-USPIO as a molecular imaging agent had great potential for monitoring islets or other cells in vivo (111). Sang-Man et al. found that the labeling effect of USPIO-based MRI was not directly suitable for clinical islet transplantation, so they evaluated the feasibility of ferumoxytol in islet MRI by multi-layer surface modification. They found that multi-layer islet surface modification was a promising choice for portal vein concave islet MRI (112). Therefore, after proper surface modification, the ordinary commercially available USPIO should be able to be used to evaluate the status of islet transplant patients as a promising new contrast agent.
The term “therapeutic diagnostics” was first proposed by Funkhoer et al. in 2002 (113). Treatment diagnostic imaging is combined with MRI diagnostic imaging and related intervention therapy. It is used to identify specific targets by imaging, design relevant reagents for the targets and visualize them and monitor the treatment response, so as to minimize any effects on normal tissue (114). It can analyze specific information by combining the genome and proteome, enabling the design of a personalized treatment for organ transplantation, which has great prospects and significance for clinical applications in the future.
Wang et al. designed an MR probe by coupling therapeutic siRNA with glucan-coated SPIO and incubated the probe with it before islet transplantation. The results showed that islets transplanted under the renal capsule had a better survival by reducing the expression of caspase-3 in the MN-siCaspase-3 group, while hyperglycemia caused by T cell attack in the MN-siB2M group was significantly delayed (102, 103). Ping et al. synthesized a nano-therapeutic agent composed of magnetic nanoparticles (MNs) coupled with siRNA molecules targeting genes harmful to islet grafts. The combined therapeutic effect provided by an RNA interference technique with an in vivo MRI diagnosis is expected to improve the results of islet transplantation significantly in patients with T1DM (115).
Recently, a new kind of nanoparticle was synthesized: MNs targeting phosphatase and tensin homologue (PTEN) coupled to miR-216a. These nanoparticles can down-regulate the expression of PTEN to promote the proliferation of islet β-cells in T1DM animal models (116). However, while these new nanoparticles have the advantage of penetrating the biofilm barrier, particle aggregation trends hinder their large-scale synthesis (117).
MPI is a newly emerging real-time monitoring and imaging method that can directly perform imaging using magnetized iron oxide nanoparticles. It has strong specificity, high sensitivity and safe translation (118). The sensitivity of MPI is 108 times higher than that of the proton magnetization seen in MRI (119). At the same time, MPI is safe and translatable because its carrier iron oxide nanoparticles are biocompatible (120) and do not produce ionizing radiation, and magnetic fields can be used with no threat to patient safety (121).
Ping et al. described the first application of MPI in the monitoring of transplanted islets. SPIO was used to label islets separated from baboons (Papio hamadryas). The results showed that MPI was an imaging method without depth attenuation or a background tissue signal, which is suitable for imaging transplanted islet grafts. However, MPI lacks the background support of physiology and anatomy depictions, which is the main issue limiting its clinical application (122).
The simple use of an imaging mode has obvious limitations, and the observed research results cannot be completely scientifically representative. For example, performing MRI just once results in a low sensitivity, its contrast medium is somewhat toxic, islets must be prelabeled before transplantation, and it is difficult to distinguish between living and dead islets. In contrast, Bioluminescence Imaging (BLI) and Fluorescence Imaging (FI)have disadvantages of poor tissue penetration, a short elimination period, low spatial resolution, propensity to be affected by the internal environment, and a tendency to show weak signals (102). These issues lead to a bottleneck in the clinical promotion of single imaging techniques and fail to objectively reflect the real situation. Therefore, it is very necessary to develop a multi-mode imaging platform that combines the advantages of different imaging methods and overcomes the limitations of individual methods.
Barnett et al. labeled human islet organs with PFOB and PFPE nanoparticles and visualized the islets under the renal capsule of mice and rabbits by MRI, ultrasound and computed tomography (123). The combination of specific and quantitative Fmur19 MRI and sensitive and convenient optical imaging can provide supplementary information to support the distribution and survival of islet grafts. Based on poly (lactic acid-glycolic acid) (PLGA-NPs) wrapped in perfluoro-15-crown-5-ether and NIR fluorescent dye indocyanine green, Gálisová successfully developed a three-mode imaging platform for the in vivo examination of islet transplantation (124).However, most of these multi-mode nanoparticles are more complex and expensive than conventional single modality nanoparticles, and the effects of these nanoparticles on transplanted islet cells and human body and their stability need to be explored.
To improve the success rate of organ cell transplantation, patients need to take ISAs to alleviate the immune response. However, systemic administration of ISAs is associated with serious side effects; for example, the oral and intravenous administration of tacrolimus may lead to systemic toxicity, which greatly reduces the long-term success rate of transplantation (125). The emerging method of nanodelivery may improve the disadvantages of systemic drug delivery via the targeted delivery of ISAs to specific organs and tissues.
For drugs with a narrow therapeutic index or low bioavailability, intervention with nanoparticle technology can potentially optimize the pharmacokinetics and delivery efficiency. In the early stage of developing transport platforms for ISAS, Edgar et al. found that cyclosporine was needed after renal transplantation, and the pharmacokinetics of cyclosporine were affected by a high lipid value, suggesting the relatively minor influence of a fat-rich meal on the absorption of cyclosporine from Sandimmune Neoral is advantageous (126).. Indeed, Ritschel et al. found that the bioavailability of cyclosporine was significantly improved when it was used as a microemulsion particle in clinical trials of kidney and liver transplant recipients (127).
At the same time, some studies have found that the release of the mTOR inhibitor rapamycin from poly(ethylene glycol)-b-poly(ϵ-caprolactone) (PEG-PCL) micelles in vitro has remarkable benefits (128). A large number of studies have shown that the pharmacokinetic characteristics and delivery efficiency of ISAs can be adjusted by changing the size, shape, surface charge and composition of nanoparticles (129–131). The size of nanoparticles is of great significance in guiding the delivery of drugs to specific transplanted organs (132). After intravenous injection, nanoparticles of different diameters are quickly removed by the kidney, liver and spleen (133–135) or preferentially gathered in the liver and spleen (136, 137). Studies have shown that nanoparticles are injected into the arterial blood supply of organs before transplantation, so the selection of an appropriate particle size will directly affect the intraorgan penetrance (138). Nanoparticles with an appropriate diameter can better meet their target, thereby improving the delivery efficiency of ISAs.
The shape of nanoparticles is also a key factor for determining the half-life of nanoparticles in the circulation. Gentile et al. found that discoidal particles are more likely to marginalize and adhere to the endothelium than spherical particles because of their specific tumbling and edge dynamics (139). Geng et al. found that the circulation time of phyllo-micelles nanoparticles in the body is longer than that of spherical nanoparticles. At the same time, some studies have shown that nanoparticles can affect the internalization of cells due to the change in the phagocytosis rate depending on their external shape, thus improving the pharmacokinetic characteristics and delivery efficiency of ISAs (134).
However, the most important parameter is the surface composition of nanoparticles. Poly(lactic-co-glycolic acid) (PLGA) is a family of biodegradable copolymers, the ultimate degradation products are lactic acid and glycolic acid and easily metabolized by the body. Therefore, it is frequently used clinically as nanoparticles carrying ISAs. ISAs encapsulated within them have the advantages of stability, long circulation time and guided drug release (140). Studies have shown that encapsulating ISAs, such as cyclosporine, tacrolimus and rapamycin, in liposome nanoparticles, such as polyethylene glycol-PLGA (PEG-PLGA), for a controlled release can stabilize the inherent variability of their pharmacokinetics (127, 141–143). High-density lipoproteins (HDLs) can also be used for the surface composition of small, dynamic nanoparticles carrying low doses of statins in order to alter the activity of the immune response through the internalization of macrophages (144). Nanogels coated with intracellular adhesion molecule-1 (ICAM-1) antibodies consisting of a mixture of dextran and lysozyme were found to provide effective drug delivery in vitro and in vivo (145).
Although we can improve the pharmacokinetics and delivery efficiency of ISAs by artificially setting the above parameters, it is extremely important to ensure the stability of these parameters during drug delivery. Results thus far have shown that the chemical composition of nanoparticles can be maintained using inert metal, graphene oxide or composite surfactant (146–148). As nanoparticles tend to target out-of-organ aggregation after collision, which greatly reduces their delivery efficiency, we need to reduce the aggregation effect in order to improve the stability of nanoparticles (149). These limitations associated with the clinical application are difficult problems that must be resolved in the future.
Nanoparticles are often used as movement-targeting agents because they can deliver drugs directly to different locations in tissues and/or cells. Since endogenous autoantigen presentation may have a synergistic effect with targeted inhibition, nanoparticles that deliver ISAs to the target site are a feasible therapeutic strategy. Nanoparticles can target phagocytes (such as dendritic cells) while carrying ISAs or genetic material to achieve therapeutic effects. Alternatively, drug therapy can be achieved by exploring the interaction of ligand-T cell receptor (TCR), which targeting non-phagocytic cells (such as T cells). Nanoparticles can also be used to facilitate the continuous release of autoantigens or to target antigen-presenting cells (APCs) through surface coupling of ligands. In the absence of inflammatory signals, nanoparticles can induce the presentation of self-antigens to APCs, resulting in T cell anergy, failure or tolerance (Figure 3). In addition, nanoparticles can transfer antigens and ISAs through surface delivery and/or soluble delivery, resulting in antigen-specific immune regulation (150, 151). Shirali et al. upregulated PD-L1 on dendritic cells via the targeted delivery of mycophenolic acid by nanoparticles to prolong the survival time of mouse allografts (152). Shah et al. used an allogeneic skin transplantation model to optimize the transplantation tolerance of the PLG nanoparticle-peptide delivery platform, suggesting that the design of perfect targeted nanoparticles requires suitable immune cell populations (153). Studies have shown that carboxylated 500-nm biodegradable PLG nanoparticles (surface-coupled or encapsulated homologous diabetic peptides) can quickly and effectively restore the tolerance of non-obese diabetic (NOD) mice (154). Nanoparticles have been used to transmit tolerance-causing molecules and cellular antigens, thereby inducing tolerance phenotypes of dendritic cells and promoting the formation of Treg cells in vivo (155). Based on the injectable dual-sized microparticle (MP) platform, the immune system can be reeducated in an antigen-specific manner. These particles can be subcutaneously injected into NOD mice to prevent diabetes (156).
Figure 3 Immunomodulatory nanoparticles (A) Nanoparticles target non-phagocytic cells such as T cells in transplanted organs (pancreas, etc.) by exploring ligand-T cell receptor (TCR) interactions. (B) Nanoparticles target antigen-presenting cell (APC) through the continuous release of their own antigens or the surface coupling of ligands, and induce antigen presentation to APC. The combination of the two modalities can quickly and effectively activate the target organ to produce regulatory T cells in the absence of inflammatory signals, and recruit a large number of T cells. At the same time, under the combined action of ISAs released by nanoparticles, resulting in T cell anergy, exhaustion or tolerance.
In addition, the binding of antigen peptides and red blood cells to antibody fragments via nanoparticles promoted antigen processing by scavenging apoptotic cells mediated by bionic red blood cells in a mouse T1DM model (157). Thomas et al. protected islet donors from non-human primates (NHPs; baboons) by silencing a gene responsible for inducing apoptosis (Caspase3), which was connected to a small interference RNA on MNs (158). Targeted antigen transmission can induce immune tolerance, and nanoparticles can be used to improve the effect of antigen therapy. However, antigen transmission alone may not be sufficient to overcome strong immune rejection and chronic inflammation after replacement with organ cells. The administration of tolerance-inducing drugs in the absence of antigens may lead to non-specific immunosuppression, while the administration of antigens alone may not be effective for treating multi-antigen heterogeneous diseases, such as T1DM. Nanoparticles can be designed to deliver antigens presented on MHC molecules to promote effective antigen presentation and thereby improve specificity and induce inhibition of the T cell phenotype. In the absence of any supportive costimulatory ligands, the treatment of NOD mice with these nanoparticles can inhibit the occurrence of diabetic symptoms via the interaction between particles and self-reactive T cells.
Continuous advances in transplantation technology are improving the survival of patients with end-stage organ failure, and the addition of nanotechnology is undoubtedly exciting, given the quandary faced by transplantation technology. Whether it be in combination with pretransplant organ preservation via machine perfusion, or as artificial biological islets undergoing constant optimization, or through nanoimaging for the in vivo survival assessment of transplanted organs, or in the optimization of traditional immunosuppressive drug pharmacokinetics, nanotechnology has been intimately involved in addressing the problems faced by traditional transplantation, and these applications are constantly proving to be extremely promising.
We herein reported advances made in nanotechnology that we feel are inspirational, mainly by discussing the applications of nanotechnology in kidney transplantation as well as pancreatic islet transplantation. However, at present, the application of these findings in the clinical setting is not yet feasible, and the long-term efficacy and safety of nanotechnology need further observation and discussion. The development of nanomaterials and continuous advances in the strategies used for transplantation will ultimately lead to a more favorable prognosis for transplant patients.
All authors contributed to the writing and editing of the manuscript, contributed to the article, and approved the submitted version.
This work was supported in part by Medical Science and Technology Project of Zhejiang Province (2021PY083), Program of Taizhou Science and Technology Grant (20ywb29), Major Research Program of Taizhou Enze Medical Center Grant (19EZZDA2), Open Project Program of Key Laboratory of Minimally Invasive Techniques and Rapid Rehabilitation of Digestive System Tumor of Zhejiang Province (21SZDSYS01, 21SZDSYS09) and Key Technology Research and Development Program of Zhejiang Province (2019C03040).
The authors declare that the research was conducted in the absence of any commercial or financial relationships that could be construed as a potential conflict of interest.
All claims expressed in this article are solely those of the authors and do not necessarily represent those of their affiliated organizations, or those of the publisher, the editors and the reviewers. Any product that may be evaluated in this article, or claim that may be made by its manufacturer, is not guaranteed or endorsed by the publisher.
IAK, implantable artificial kidney; DCD, donated after circulatory death; HMP, hypothermic machine perfusion; SCS, static cold storage; NMP, normothermic machine perfusion; APACs, antiplatelet and anticoagulants; IRI, ischemia-reperfusion injury; EVLP, ex vivo lung perfusion; ECs, endothelial cells; TLN, thrombalexin; cRGD, cyclic RGD; EVs, extracellular vesicles; TRAMs, targeted rapamycin micelles; ISAs, immunosuppressive agents; ROS, reactive oxygen species; PTX, pentoxifylline; SPIONs, superparamagnetic iron oxide nanoparticles; LBL, layer-by-layer; PEG8, 8-arm-PEG-catechol; UFH-NHS, N-hydroxysuccinimidyl-linked unfractionated heparin; HNSIs, heparin nano-shielded islets; JAG-1, Jagged-1; CONPs, cerium oxide nanoparticles; MLBL, multi-layer-by-layer; CLBL, covalently-stabilized LBL; PERV, porcine endogenous retrovirus; NPCCs, neonatal porcine islet-like cell clusters; CR-NCS, cold-responsive nanocapsules; CAH, calcium alginate hydrogel; mTOR, mammalian target of rapamycin; PEG-PCL, poly(ethylene glycol)-b-poly(ϵ-caprolactone); PLGA, Poly (lactic-co-glycolic acid); PEG-PLGA, poly(ethylene glycol)- Poly (lactic-co-glycolic acid); HDLs, high density lipoproteins; ICAM-1, intracellular adhesion molecule-1; TCR, T cell receptor; APC, antigen presenting cells; PD-L1, programmed cell death-Ligand 1; PLG, poly (D,L-lactide-co-glycolide); NOD, non-obese diabetic; MP, microparticle; T1DM, type 1 diabetes mellitus; NHP, non-human primates; MN, magnetic nanoparticles; MHC, major histocompatibility complex; MRI, magnetic resonance imaging; SPIO, superparamagnetic iron oxide; IBMIR, immediate blood-mediated inflammatory response; USPIO, ultra-miniature superparamagnetic iron oxide; FeMRA, ferumoxytol-enhanced magnetic resonance angiography; FDA, food and drug administration; PTEN, phosphatase and tensin homologue; MPI, magnetic particle imaging; BLI, bioluminescence Imaging; FI, fluorescence Imaging; PFOB, perfluorooctylbromide; PFPE, perfluoropolyethers.
1. Bezinover D, Saner F. Organ Transplantation in the Modern Era. BMC Anesthesiol (2019) 19(1):32. doi: 10.1186/s12871-019-0704-z
2. Jenssen T, Hartmann A. Post-Transplant Diabetes Mellitus in Patients With Solid Organ Transplants. Nat Rev Endocrinol (2019) 15(3):172–88. doi: 10.1038/s41574-018-0137-7
3. Edgar L, Pu T, Porter B, Aziz JM, La Pointe C, Asthana A, et al. Regenerative Medicine, Organ Bioengineering and Transplantation. Br J Surg (2020) 107(7):793–800. doi: 10.1002/bjs.11686
4. Humar A, Michaels M. American Society of Transplantation Recommendations for Screening, Monitoring and Reporting of Infectious Complications in Immunosuppression Trials in Recipients of Organ Transplantation. Am J Transplant (2006) 6(2):262–74. doi: 10.1111/j.1600-6143.2005.01207.x
5. Tasciotti E, Cabrera FJ, Evangelopoulos M, Martinez JO, Thekkedath UR, Kloc M, et al. The Emerging Role of Nanotechnology in Cell and Organ Transplantation. Transplantation (2016) 100(8):1629–38. doi: 10.1097/tp.0000000000001100
6. Jenssen T, Hartmann A. Emerging Treatments for Post-Transplantation Diabetes Mellitus. Nat Rev Nephrol (2015) 11(8):465–77. doi: 10.1038/nrneph.2015.59
7. Dangi A, Yu S, Luo X. Emerging Approaches and Technologies in Transplantation: The Potential Game Changers. Cell Mol Immunol (2019) 16(4):334–42. doi: 10.1038/s41423-019-0207-3
8. Kemter E, Denner J, Wolf E. Will Genetic Engineering Carry Xenotransplantation of Pig Islets to the Clinic? Curr Diabetes Rep (2018) 18(11):103. doi: 10.1007/s11892-018-1074-5
9. Cooper DKC, Hara H, Iwase H, Yamamoto T, Li Q, Ezzelarab M, et al. Justification of Specific Genetic Modifications in Pigs for Clinical Organ Xenotransplantation. Xenotransplantation (2019) 26(4):e12516. doi: 10.1111/xen.12516
10. Kim BY, Rutka JT, Chan WC. Nanomedicine. N Engl J Med (2010) 363(25):2434–43. doi: 10.1056/NEJMra0912273
11. Kim TY, Kim DW, Chung JY, Shin SG, Kim SC, Heo DS, et al. Phase I and Pharmacokinetic Study of Genexol-PM, a Cremophor-Free, Polymeric Micelle-Formulated Paclitaxel, in Patients With Advanced Malignancies. Clin Cancer Res (2004) 10(11):3708–16. doi: 10.1158/1078-0432.Ccr-03-0655
12. Prencipe G, Tabakman SM, Welsher K, Liu Z, Goodwin AP, Zhang L, et al. PEG Branched Polymer for Functionalization of Nanomaterials With Ultralong Blood Circulation. J Am Chem Soc (2009) 131(13):4783–7. doi: 10.1021/ja809086q
13. Libutti S, Paciotti G, Myer L, Haynes R, Gannon W, Eugeni M, et al. Preliminary Results of a Phase I Clinical Trial of CYT-6091: A Pegylated Colloidal Gold-TNF Nanomedicine. J Clin Oncol (2007) 25(18_suppl):3603–3. doi: 10.1200/jco.2007.25.18_suppl.3603
14. Hirsch LR, Stafford RJ, Bankson JA, Sershen SR, Rivera B, Price RE, et al. Nanoshell-Mediated Near-Infrared Thermal Therapy of Tumors Under Magnetic Resonance Guidance. Proc Natl Acad Sci USA (2003) 100(23):13549–54. doi: 10.1073/pnas.2232479100
15. Hedlund A, Ahrén M, Gustafsson H, Abrikossova N, Warntjes M, Jönsson JI, et al. Gd₂O₃ Nanoparticles in Hematopoietic Cells for MRI Contrast Enhancement. Int J Manomed (2011) 6:3233–40. doi: 10.2147/ijn.S23940
16. Cheng Y, Yu Y, Zhang Y, Zhao G, Zhao YJS. Cold-Responsive Nanocapsules Enable the Sole-Cryoprotectant-Trehalose Cryopreservation of β Cell–Laden Hydrogels for Diabetes Treatment. Small (2019) 15(50). doi: 10.1002/smll.201904290
17. Yao CG, Martins PN. Nanotechnology Applications in Transplantation Medicine. Transplantation (2020) 104(4):682–93. doi: 10.1097/tp.0000000000003032
18. Pan W, Zheng X, Chen G, Su L, Luo S, Wang W, et al. Nanotechnology’s Application in Type 1 Diabetes. Wiley Interdiscip Rev Nanomed Nanobiotechnol (2020) 12(6):e1645. doi: 10.1002/wnan.1645
19. Westover AJ, Buffington DA, Humes HD. Enhanced Propagation of Adult Human Renal Epithelial Progenitor Cells to Improve Cell Sourcing for Tissue-Engineered Therapeutic Devices for Renal Diseases. J Tissue Eng Regen Med (2012) 6(8):589–97. doi: 10.1002/term.471
20. Kensinger C, Karp S, Kant R, Chui BW, Goldman K, Yeager T, et al. First Implantation of Silicon Nanopore Membrane Hemofilters. ASAIO J (Am Soc Artif Inter Organs: 1992) (2016) 62(4):491–5. doi: 10.1097/mat.0000000000000367
21. Muthusubramaniam L, Lowe R, Fissell WH, Li L, Marchant RE, Desai TA, et al. Hemocompatibility of Silicon-Based Substrates for Biomedical Implant Applications. Ann Biomed Eng (2011) 39(4):1296–305. doi: 10.1007/s10439-011-0256-y
22. Fissell WH, Dubnisheva A, Eldridge AN, Fleischman AJ, Zydney AL, Roy S. High-Performance Silicon Nanopore Hemofiltration Membranes. J Membr Sci (2009) 326(1):58–63. doi: 10.1016/j.memsci.2008.09.039
23. Kanani DM, Fissell WH, Roy S, Dubnisheva A, Fleischman A, Zydney AL. Permeability - Selectivity Analysis for Ultrafiltration: Effect of Pore Geometry. J Membr Sci (2010) 349(1-2):405. doi: 10.1016/j.memsci.2009.12.003
24. Iqbal Z, Moses W, Kim S, Kim EJ, Fissell WH, Roy S. Sterilization Effects on Ultrathin Film Polymer Coatings for Silicon-Based Implantable Medical Devices. Journal of Biomedical Materials Research Part B. Appl Biomater (2018) 106(6):2327–36. doi: 10.1002/jbm.b.34039
25. Hosgood SA, Shah K, Patel M, Nicholson ML. The Effect of Prolonged of Warm Ischaemic Injury on Renal Function in an Experimental Ex Vivo Normothermic Perfusion System. J Trans Med (2015) 13:207. doi: 10.1186/s12967-015-0571-4
26. Harper SJ, Hosgood SA, Waller HL, Yang B, Kay MD, Goncalves I, et al. The Effect of Warm Ischemic Time on Renal Function and Injury in the Isolated Hemoperfused Kidney. Transplantation (2008) 86(3):445–51. doi: 10.1097/TP.0b013e31817fe0cd
27. Toso C, Oberholzer J, Ris F, Triponez F, Bucher P, Demirag A, et al. Factors Affecting Human Islet of Langerhans Isolation Yields. Transplant Proc (2002) 34(3):826–7. doi: 10.1016/s0041-1345(01)02925-6
28. Summers DM, Watson CJ, Pettigrew GJ, Johnson RJ, Collett D, Neuberger JM, et al. Kidney Donation After Circulatory Death (DCD): State of the Art. Kidney Int (2015) 88(2):241–9. doi: 10.1038/ki.2015.88
29. Weissenbacher A, Vrakas G, Nasralla D, Ceresa CDL. The Future of Organ Perfusion and Re-Conditioning. Transplant Int (2019) 32(6):586–97. doi: 10.1111/tri.13441
30. Moers C, Pirenne J, Paul A, Ploeg RJ. Machine Perfusion or Cold Storage in Deceased-Donor Kidney Transplantation. N Engl J Med (2012) 366(8):770–1. doi: 10.1056/NEJMc1111038
31. Debout A, Foucher Y, Trébern-Launay K, Legendre C, Kreis H, Mourad G, et al. Each Additional Hour of Cold Ischemia Time Significantly Increases the Risk of Graft Failure and Mortality Following Renal Transplantation. Kidney Int (2015) 87(2):343–9. doi: 10.1038/ki.2014.304
32. Arnaud FG, Khirabadi BS, Fahy GM. Normothermic Blood Perfusion of Isolated Rabbit Kidneys. II. In Vitro Evaluation of Renal Function Followed by Orthotopic Transplantation. ASAIO J (Am Soc Artif Intern Organs: 1992) (2000) 46(6):707–18. doi: 10.1097/00002480-200011000-00011
33. Bagul A, Hosgood SA, Kaushik M, Kay MD, Waller HL, Nicholson ML. Experimental Renal Preservation by Normothermic Resuscitation Perfusion With Autologous Blood. Br J Surg (2008) 95(1):111–8. doi: 10.1002/bjs.5909
34. Mayfield KB, Ametani M, Southard JH, Belzer FO. Mechanism of Action of Ex Vivo Blood Rescue in Six-Day Preserved Kidneys. Transplant Proc (1987) 19(1 Pt 2):1367–8.
35. Brasile L, Stubenitsky BM, Booster MH, Lindell S, Araneda D, Buck C, et al. Overcoming Severe Renal Ischemia: The Role of Ex Vivo Warm Perfusion. Transplantation (2002) 73(6):897–901. doi: 10.1097/00007890-200203270-00011
36. Hosgood SA, Saeb-Parsy K, Wilson C, Callaghan C, Collett D, Nicholson ML. Protocol of a Randomised Controlled, Open-Label Trial of Ex Vivo Normothermic Perfusion Versus Static Cold Storage in Donation After Circulatory Death Renal Transplantation. BMJ Open (2017) 7(1):e012237. doi: 10.1136/bmjopen-2016-012237
37. Nicholson ML, Hosgood SA. Renal Transplantation After Ex Vivo Normothermic Perfusion: The First Clinical Study. Am J Transplant (2013) 13(5):1246–52. doi: 10.1111/ajt.12179
38. Kaths JM, Cen JY, Chun YM, Echeverri J, Linares I, Ganesh S, et al. Continuous Normothermic Ex Vivo Kidney Perfusion Is Superior to Brief Normothermic Perfusion Following Static Cold Storage in Donation After Circulatory Death Pig Kidney Transplantation. Am J Transplant (2017) 17(4):957–69. doi: 10.1111/ajt.14059
39. Weissenbacher A, Lo Faro L, Boubriak O, Soares MF, Roberts IS, Hunter JP, et al. Twenty-Four-Hour Normothermic Perfusion of Discarded Human Kidneys With Urine Recirculation. Am J Transplant (2019) 19(1):178–92. doi: 10.1111/ajt.14932
40. Sena CM, Leandro A, Azul L, Seiça R, Perry G. Vascular Oxidative Stress: Impact and Therapeutic Approaches. Front Physiol (2018) 9:1668. doi: 10.3389/fphys.2018.01668
41. Aksu K, Donmez A, Keser G. Inflammation-Induced Thrombosis: Mechanisms, Disease Associations and Management. Curr Pharm Des (2012) 18(11):1478–93. doi: 10.2174/138161212799504731
42. Bonventre JV, Yang L. Cellular Pathophysiology of Ischemic Acute Kidney Injury. J Clin Invest (2011) 121(11):4210–21. doi: 10.1172/jci45161
43. Tuuminen R, Jouppila A, Salvail D, Laurent CE, Benoit MC, Syrjälä S, et al. Dual Antiplatelet and Anticoagulant APAC Prevents Experimental Ischemia-Reperfusion-Induced Acute Kidney Injury. Clin Exp Nephrol (2017) 21(3):436–45. doi: 10.1007/s10157-016-1308-2
44. Basile DP. The Endothelial Cell in Ischemic Acute Kidney Injury: Implications for Acute and Chronic Function. Kidney Int (2007) 72(2):151–6. doi: 10.1038/sj.ki.5002312
45. Karimian N, Yeh H. Opportunities for Therapeutic Intervention During Machine Perfusion. Curr Transplant Rep (2017) 4(2):141–8. doi: 10.1007/s40472-017-0144-y
46. Hamaoui K, Gowers S, Boutelle M, Cook TH, Hanna G, Darzi A, et al. Organ Pretreatment With Cytotopic Endothelial Localizing Peptides to Ameliorate Microvascular Thrombosis and Perfusion Deficits in Ex Vivo Renal Hemoreperfusion Models. Transplantation (2016) 100(12):e128–39. doi: 10.1097/tp.0000000000001437
47. Devalliere J, Chang WG, Andrejecsk JW, Abrahimi P, Cheng CJ, Jane-wit D, et al. Sustained Delivery of Proangiogenic microRNA-132 by Nanoparticle Transfection Improves Endothelial Cell Transplantation. FASEB J (2014) 28(2):908–22. doi: 10.1096/fj.13-238527
48. Tenzer S, Docter D, Kuharev J, Musyanovych A, Fetz V, Hecht R, et al. Rapid Formation of Plasma Protein Corona Critically Affects Nanoparticle Pathophysiology. Nat Nanotechnol (2013) 8(10):772–81. doi: 10.1038/nnano.2013.181
49. Tietjen GT, Hosgood SA, DiRito J, Cui J, Deep D, Song E, et al. Nanoparticle Targeting to the Endothelium During Normothermic Machine Perfusion of Human Kidneys. Sci Trans Med (2017) 9(418):eaam6764. doi: 10.1126/scitranslmed.aam6764
50. DiRito JR, Hosgood SA, Reschke M, Albert C, Bracaglia LG, Ferdinand JR, et al. Lysis of Cold-Storage-Induced Microvascular Obstructions for Ex Vivo Revitalization of Marginal Human Kidneys. Am J Transplant (2021) 21(1):161–73. doi: 10.1111/ajt.16148
51. Woud WW, Merino A, Hoogduijn MJ, Boer K, van den Hoogen MWF, Baan CC, et al. Nanoparticle Release by Extended Criteria Donor Kidneys During Normothermic Machine Perfusion. Transplantation (2019) 103(5):e110–1. doi: 10.1097/tp.0000000000002642
52. Zhu P, Atkinson C, Dixit S, Cheng Q, Tran D, Patel K, et al. Organ Preservation With Targeted Rapamycin Nanoparticles: A Pre-Treatment Strategy Preventing Chronic Rejection In Vivo. RSC Adv (2018) 8(46):25909–19. doi: 10.1039/c8ra01555d
53. Cui J, Qin L, Zhang J, Abrahimi P, Li H, Li G, et al. Ex Vivo Pretreatment of Human Vessels With siRNA Nanoparticles Provides Protein Silencing in Endothelial Cells. Nat Commun (2017) 8(1):191. doi: 10.1038/s41467-017-00297-x
54. Eisenbarth G. Metabolism. Update in Type 1 Diabetes. J Clin Endocrinol Metab (2007) 92(7):2403–7. doi: 10.1210/jc.2007-0339
55. DeWitt DE, Hirsch IB. Outpatient Insulin Therapy in Type 1 and Type 2 Diabetes Mellitus: Scientific Review. Jama (2003) 289(17):2254–64. doi: 10.1001/jama.289.17.2254
56. Pathak S, Pham TT, Jeong JH, Byun Y. Immunoisolation of Pancreatic Islets via Thin-Layer Surface Modification. J Control Rel (2019) 305:176–93. doi: 10.1016/j.jconrel.2019.04.034
57. Robertson RP. Islet Transplantation as a Treatment for Diabetes - a Work in Progress. N Engl J Med (2004) 350(7):694–705. doi: 10.1056/NEJMra032425
58. Shapiro AM, Ricordi C, Hering BJ, Auchincloss H, Lindblad R, Robertson RP, et al. International Trial of the Edmonton Protocol for Islet Transplantation. N Engl J Med (2006) 355(13):1318–30. doi: 10.1056/NEJMoa061267
59. de Vos P, Spasojevic M, Faas MM. Treatment of Diabetes With Encapsulated Islets. Adv Exp Med Biol (2010) 670:38–53. doi: 10.1007/978-1-4419-5786-3_5
60. Lim F, Sun AM. Microencapsulated Islets as Bioartificial Endocrine Pancreas. Sci (New York NY) (1980) 210(4472):908–10. doi: 10.1126/science.6776628
61. Wang T, Lacík I, Brissová M, Anilkumar AV, Prokop A, Hunkeler D, et al. An Encapsulation System for the Immunoisolation of Pancreatic Islets. Nat Biotechnol (1997) 15(4):358–62. doi: 10.1038/nbt0497-358
62. Klöck G, Frank H, Houben R, Zekorn T, Horcher A, Siebers U, et al. Production of Purified Alginates Suitable for Use in Immunoisolated Transplantation. Appl Microbiol Biotechnol (1994) 40(5):638–43. doi: 10.1007/bf00173321
63. O’Shea GM, Goosen MF, Sun AM. Prolonged Survival of Transplanted Islets of Langerhans Encapsulated in a Biocompatible Membrane. Biochim Biophys Acta (1984) 804(1):133–6. doi: 10.1016/0167-4889(84)90107-1
64. Dang TT, Thai AV, Cohen J, Slosberg JE, Siniakowicz K, Doloff JC, et al. Enhanced Function of Immuno-Isolated Islets in Diabetes Therapy by Co-Encapsulation With an Anti-Inflammatory Drug. Biomaterials (2013) 34(23):5792–801. doi: 10.1016/j.biomaterials.2013.04.016
65. van Schilfgaarde R, de Vos P. Factors Influencing the Properties and Performance of Microcapsules for Immunoprotection of Pancreatic Islets. J Mol Med (Berlin Germany) (1999) 77(1):199–205. doi: 10.1007/s001090050336
66. Azadi SA, Vasheghani-Farahani E, Hashemi-Najafbabadi S, Godini A. Co-Encapsulation of Pancreatic Islets and Pentoxifylline in Alginate-Based Microcapsules With Enhanced Immunosuppressive Effects. Prog Biomater (2016) 5:101–9. doi: 10.1007/s40204-016-0049-3
67. Espona-Noguera A, Etxebarria-Elezgarai J, Saenz Del Burgo L, Cañibano-Hernández A, Gurruchaga H, Blanco FJ, et al. Type 1 Diabetes Mellitus Reversal via Implantation of Magnetically Purified Microencapsulated Pseudoislets. Int J Pharm (2019) 560:65–77. doi: 10.1016/j.ijpharm.2019.01.058
68. Delcassian D, Luzhansky I, Spanoudaki V, Bochenek M, McGladrigan C, Nguyen A, et al. Magnetic Retrieval of Encapsulated Beta Cell Transplants From Diabetic Mice Using Dual-Function MRI Visible and Retrievable Microcapsules. Adv Mater (Deerfield Beach Fla) (2020) 32(16):e1904502. doi: 10.1002/adma.201904502
69. de Vos P, Faas MM, Strand B, Calafiore R. Alginate-Based Microcapsules for Immunoisolation of Pancreatic Islets. Biomaterials (2006) 27(32):5603–17. doi: 10.1016/j.biomaterials.2006.07.010
70. Wilson JT, Chaikof EL. Challenges and Emerging Technologies in the Immunoisolation of Cells and Tissues. Adv Drug Deliv Rev (2008) 60(2):124–45. doi: 10.1016/j.addr.2007.08.034
71. Bennet W, Sundberg B, Groth CG, Brendel MD, Brandhorst D, Brandhorst H, et al. Incompatibility Between Human Blood and Isolated Islets of Langerhans: A Finding With Implications for Clinical Intraportal Islet Transplantation? Diabetes (1999) 48(10):1907–14. doi: 10.2337/diabetes.48.10.1907
72. Wilson JT, Cui W, Chaikof EL. Layer-By-Layer Assembly of a Conformal Nanothin PEG Coating for Intraportal Islet Transplantation. Nano Lett (2008) 8(7):1940–8. doi: 10.1021/nl080694q
73. Haque MR, Kim J, Park H, Lee HS, Lee KW, Al-Hilal TA, et al. Xenotransplantation of Layer-by-Layer Encapsulated non-Human Primate Islets With a Specified Immunosuppressive Drug Protocol. J Control Rel (2017) 258:10–21. doi: 10.1016/j.jconrel.2017.04.021
74. Kizilel S, Scavone A, Liu X, Nothias JM, Ostrega D, Witkowski P, et al. Encapsulation of Pancreatic Islets Within Nano-Thin Functional Polyethylene Glycol Coatings for Enhanced Insulin Secretion. Tissue Eng Part A (2010) 16(7):2217–28. doi: 10.1089/ten.TEA.2009.0640
75. Im BH, Jeong JH, Haque MR, Lee DY, Ahn CH, Kim JE, et al. The Effects of 8-Arm-PEG-Catechol/Heparin Shielding System and Immunosuppressive Drug, FK506 on the Survival of Intraportally Allotransplanted Islets. Biomaterials (2013) 34(8):2098–106. doi: 10.1016/j.biomaterials.2012.11.028
76. Park H, Haque MR, Park JB, Lee KW, Lee S, Kwon Y, et al. Polymeric Nano-Shielded Islets With Heparin-Polyethylene Glycol in a non-Human Primate Model. Biomaterials (2018) 171:164–77. doi: 10.1016/j.biomaterials.2018.04.028
77. Haque MR, Jeong JH, Lee KW, Shin DY, Kim GS, Kim SJ, et al. Effects of Transplanted Islets Nano-Encapsulated With Hyperbranched Polyethylene Glycol and Heparin on Microenvironment Reconstruction and Glucose Control. Bioconjug Chem (2018) 29(9):2945–53. doi: 10.1021/acs.bioconjchem.8b00364
78. Izadi Z, Hajizadeh-Saffar E, Hadjati J, Habibi-Anbouhi M, Ghanian MH, Sadeghi-Abandansari H, et al. Tolerance Induction by Surface Immobilization of Jagged-1 for Immunoprotection of Pancreatic Islets. Biomaterials (2018) 182:191–201. doi: 10.1016/j.biomaterials.2018.08.017
79. Kozlovskaya V, Zavgorodnya O, Chen Y, Ellis K, Tse HM, Cui W, et al. Ultrathin Polymeric Coatings Based on Hydrogen-Bonded Polyphenol for Protection of Pancreatic Islet Cells. Adv Funct Mater (2012) 22(16):3389–98. doi: 10.1002/adfm.201200138
80. Abuid NJ, Gattás-Asfura KM, Schofield EA, Stabler CL. Layer-By-Layer Cerium Oxide Nanoparticle Coating for Antioxidant Protection of Encapsulated Beta Cells. Adv Healthc Mater (2019) 8(12):e1801493. doi: 10.1002/adhm.201801493
81. Syed F, Bugliani M, Novelli M, Olimpico F, Suleiman M, Marselli L, et al. Conformal Coating by Multilayer Nano-Encapsulation for the Protection of Human Pancreatic Islets: In-Vitro and in-Vivo Studies. Nanomed: Nanotechnol Biol Med (2018) 14(7):2191–203. doi: 10.1016/j.nano.2018.06.013
82. Gattás-Asfura KM, Abuid NJ, Labrada I, Stabler CL. Promoting Dendrimer Self-Assembly Enhances Covalent Layer-By-Layer Encapsulation of Pancreatic Islets. ACS Biomater Sci Eng (2020) 6(5):2641–51. doi: 10.1021/acsbiomaterials.9b01033
83. Haque MR, Jeong JH, Byun Y. Combination Strategy of Multi-Layered Surface Camouflage Using Hyperbranched Polyethylene Glycol and Immunosuppressive Drugs for the Prevention of Immune Reactions Against Transplanted Porcine Islets. Biomaterials (2016) 84:144–56. doi: 10.1016/j.biomaterials.2016.01.039
84. Korsgren O, Buhler LH, Groth CG. Toward Clinical Trials of Islet Xenotransplantation. Xenotransplantation (2003) 10(4):289–92. doi: 10.1034/j.1399-3089.2003.00077.x
85. Chen Y, Stewart JM, Gunthart M, Hawthorne WJ, Salvaris EJ, O’Connell PJ, et al. Xenoantibody Response to Porcine Islet Cell Transplantation Using GTKO, CD55, CD59, and Fucosyltransferase Multiple Transgenic Donors. Xenotransplantation (2014) 21(3):244–53. doi: 10.1111/xen.12091
86. Matsumoto S, Abalovich A, Wechsler C, Wynyard S, Elliott RB. Clinical Benefit of Islet Xenotransplantation for the Treatment of Type 1 Diabetes. EBioMedicine (2016) 12:255–62. doi: 10.1016/j.ebiom.2016.08.034
87. Lew B, Kim IY, Choi H, Kim KK. Sustained Exenatide Delivery via Intracapsular Microspheres for Improved Survival and Function of Microencapsulated Porcine Islets. Drug Deliv Trans Res (2018) 8(3):857–62. doi: 10.1007/s13346-018-0484-x
88. Klymiuk N, Ludwig B, Seissler J, Reichart B, Wolf EJCMBR. Current Concepts of Using Pigs as a Source for Beta-Cell Replacement Therapy of Type 1 Diabetes. Curr Mol Biol Rep (2016) 2(2):73–82. doi: 10.1007/s40610-016-0039-1
89. Zhu HT, Wang WL, Yu L, Wang B. Pig-Islet Xenotransplantation: Recent Progress and Current Perspectives. Front Surg (2014) 1:7. doi: 10.3389/fsurg.2014.00007
90. Lee SH, Kim HO, Kang JT. Optimization of Nano-Encapsulation on Neonatal Porcine Islet-Like Cell Clusters Using Polymersomes. Nanoscale Res Lett (2021) 16(1):53. doi: 10.1186/s11671-021-03512-3
91. Zhu H, Wang J, Jiang H, Ma Y, Pan S, Reddy S, et al. Bilirubin Protects Grafts Against Nonspecific Inflammation-Induced Injury in Syngeneic Intraportal Islet Transplantation. Exp Mol Med (2010) 42(11):739–48. doi: 10.3858/emm.2010.42.11.075
92. Khan NM, Poduval TB. Immunomodulatory and Immunotoxic Effects of Bilirubin: Molecular Mechanisms. J Leukocyte Biol (2011) 90(5):997–1015. doi: 10.1189/jlb.0211070
93. Adin CA, Croker BP, Agarwal A. Protective Effects of Exogenous Bilirubin on Ischemia-Reperfusion Injury in the Isolated, Perfused Rat Kidney. Am J Physiol Renal Physiol (2005) 288(4):F778–84. doi: 10.1152/ajprenal.00215.2004
94. Fullagar B, Rao W, Gilor C, Xu F, He X, Adin CA. Nano-Encapsulation of Bilirubin in Pluronic F127-Chitosan Improves Uptake in β Cells and Increases Islet Viability and Function After Hypoxic Stress. Cell Transplant (2017) 26(10):1703–15. doi: 10.1177/0963689717735112
95. Huang L, Xiang J, Cheng Y, Xiao L, Wang Q, Zhang Y, et al. Regulation of Blood Glucose Using Islets Encapsulated in a Melanin-Modified Immune-Shielding Hydrogel. ACS Appl Mater Interf (2021) 13(11):12877–87. doi: 10.1021/acsami.0c23010
96. Hoveizi E, Mohammadi T. Differentiation of Endometrial Stem Cells Into Insulin-Producing Cells Using Signaling Molecules and Zinc Oxide Nanoparticles, and Three-Dimensional Culture on Nanofibrous Scaffolds. J Mater Sci Mater Med (2019) 30(9):101. doi: 10.1007/s10856-019-6301-3
97. Kuo YC, Liu YC, Rajesh R. Pancreatic Differentiation of Induced Pluripotent Stem Cells in Activin A-Grafted Gelatin-Poly(Lactide-Co-Glycolide) Nanoparticle Scaffolds With Induction of LY294002 and Retinoic Acid. Mater Sci Eng C Mater Biol Appl (2017) 77:384–93. doi: 10.1016/j.msec.2017.03.265
98. Sutherland DE, Radosevich DM, Bellin MD, Hering BJ, Beilman GJ, Dunn TB, et al. Total Pancreatectomy and Islet Autotransplantation for Chronic Pancreatitis. J Am Coll Surg (2012) 214(4):409–24. doi: 10.1016/j.jamcollsurg.2011.12.040
99. Malka D, Hammel P, Sauvanet A, Rufat P, O’Toole D, Bardet P, et al. Risk Factors for Diabetes Mellitus in Chronic Pancreatitis. Gastroenterology (2000) 119(5):1324–32. doi: 10.1053/gast.2000.19286
100. Blondet JJ, Carlson AM, Kobayashi T, Jie T, Bellin M, Hering BJ, et al. The Role of Total Pancreatectomy and Islet Autotransplantation for Chronic Pancreatitis. Surg Clin North Am (2007) 87(6):1477–501. doi: 10.1016/j.suc.2007.08.014
101. Wang P, Medarova Z, Moore A. Molecular Imaging: A Promising Tool to Monitor Islet Transplantation. J Transplant (2011) 2011:202915. doi: 10.1155/2011/202915
102. Zheng L, Wang Y, Yang B, Zhang B, Wu Y. Islet Transplantation Imaging In Vivo. Diabetes Metab Syndr Obes: Targets Ther (2020) 13:3301–11. doi: 10.2147/dmso.S263253
103. Wang YX. Superparamagnetic Iron Oxide Based MRI Contrast Agents: Current Status of Clinical Application. Quant Imaging Med Surg (2011) 1(1):35–40. doi: 10.3978/j.issn.2223-4292.2011.08.03
104. Toso C, Vallee JP, Morel P, Ris F, Demuylder-Mischler S, Lepetit-Coiffe M, et al. Clinical Magnetic Resonance Imaging of Pancreatic Islet Grafts After Iron Nanoparticle Labeling. Am J Transplant (2008) 8(3):701–6. doi: 10.1111/j.1600-6143.2007.02120.x
105. Oishi K, Noguchi H, Saito H, Yukawa H, Miyamoto Y, Ono K, et al. Novel Positive-Charged Nanoparticles for Efficient Magnetic Resonance Imaging of Islet Transplantation. Cell Med (2012) 3(1-3):43–9. doi: 10.3727/215517912x639397
106. Oishi K, Miyamoto Y, Saito H, Murase K, Ono K, Sawada M, et al. In Vivo Imaging of Transplanted Islets Labeled With a Novel Cationic Nanoparticle. PloS One (2013) 8(2):e57046. doi: 10.1371/journal.pone.0057046
107. Hwang YH, Kim MJ, Lee DY. MRI-Sensitive Contrast Agent With Anticoagulant Activity for Surface Camouflage of Transplanted Pancreatic Islets. Biomaterials (2017) 138:121–30. doi: 10.1016/j.biomaterials.2017.05.038
108. Stoumpos S, Hennessy M, Vesey AT, Radjenovic A, Kasthuri R, Kingsmore DB, et al. Ferumoxytol-Enhanced Magnetic Resonance Angiography for the Assessment of Potential Kidney Transplant Recipients. Eur Radiol (2018) 28(1):115–23. doi: 10.1007/s00330-017-4934-5
109. Aghighi M, Pisani L, Theruvath AJ, Muehe AM, Donig J, Khan R, et al. Ferumoxytol Is Not Retained in Kidney Allografts in Patients Undergoing Acute Rejection. Mol Imaging Biol (2018) 20(1):139–49. doi: 10.1007/s11307-017-1084-8
110. Gaglia JL, Guimaraes AR, Harisinghani M, Turvey SE, Jackson R, Benoist C, et al. Noninvasive Imaging of Pancreatic Islet Inflammation in Type 1A Diabetes Patients. J Clin Invest (2011) 121(1):442–5. doi: 10.1172/jci44339
111. Yang B, Cai H, Qin W, Zhang B, Zhai C, Jiang B, et al. Bcl-2-Functionalized Ultrasmall Superparamagnetic Iron Oxide Nanoparticles Coated With Amphiphilic Polymer Enhance the Labeling Efficiency of Islets for Detection by Magnetic Resonance Imaging. Int J Manomed (2013) 8:3977–90. doi: 10.2147/ijn.S52058
112. Jin SM, Lee HS, Haque MR, Kim HN, Kim HJ, Oh BJ, et al. Multi-Layer Surface Modification of Pancreatic Islets for Magnetic Resonance Imaging Using Ferumoxytol. Biomaterials (2019) 214:119224. doi: 10.1016/j.biomaterials.2019.119224
113. Kelkar SS, Reineke TM. Theranostics: Combining Imaging and Therapy. Bioconjug Chem (2011) 22(10):1879–903. doi: 10.1021/bc200151q
114. Penet MF, Chen Z, Kakkad S, Pomper MG, Bhujwalla ZM. Theranostic Imaging of Cancer. Eur J Radiol (2012) 81 Suppl 1(0 1):S124–6. doi: 10.1016/s0720-048x(12)70051-7
115. Wang P, Moore A. In Vivo Magnetic Resonance Imaging of Small Interfering RNA Nanodelivery to Pancreatic Islets. Methods Mol Biol (Clifton NJ) (2016) 1372:25–36. doi: 10.1007/978-1-4939-3148-4_2
116. Wang P, Liu Q, Zhao H, Bishop JO, Zhou G, Olson LK, et al. miR-216a-Targeting Theranostic Nanoparticles Promote Proliferation of Insulin-Secreting Cells in Type 1 Diabetes Animal Model. Sci Rep (2020) 10(1):5302. doi: 10.1038/s41598-020-62269-4
117. Kumari P, Ghosh B, Biswas S. Nanocarriers for Cancer-Targeted Drug Delivery. J Drug Target (2016) 24(3):179–91. doi: 10.3109/1061186x.2015.1051049
118. Gleich B, Weizenecker J. Tomographic Imaging Using the Nonlinear Response of Magnetic Particles. Nature (2005) 435(7046):1214–7. doi: 10.1038/nature03808
119. Goodwill P, Tamrazian A, Croft L, Lu C, Johnson E, Pidaparthi R, et al. Ferrohydrodynamic Relaxometry for Magnetic Particle Imaging. Appl Phys Lett (2011) 98(26):262502. doi: 10.1063/1.3604009
120. Goodwill PW, Saritas EU, Croft LR, Kim TN, Krishnan KM, Schaffer DV, et al. X-Space MPI: Magnetic Nanoparticles for Safe Medical Imaging. Adv Mater (Deerfield Beach Fla) (2012) 24(28):3870–7. doi: 10.1002/adma.201200221
121. Saritas EU, Goodwill PW, Zhang GZ, Conolly SM. Magnetostimulation Limits in Magnetic Particle Imaging. IEEE Trans Med Imaging (2013) 32(9):1600–10. doi: 10.1109/tmi.2013.2260764
122. Wang P, Goodwill PW, Pandit P, Gaudet J, Ross A, Wang J, et al. Magnetic Particle Imaging of Islet Transplantation in the Liver and Under the Kidney Capsule in Mouse Models. Quant Imaging Med Surg (2018) 8(2):114–22. doi: 10.21037/qims.2018.02.06
123. Barnett BP, Ruiz-Cabello J, Hota P, Ouwerkerk R, Shamblott MJ, Lauzon C, et al. Use of Perfluorocarbon Nanoparticles for non-Invasive Multimodal Cell Tracking of Human Pancreatic Islets. Contrast Media Mol Imaging (2011) 6(4):251–9. doi: 10.1002/cmmi.424
124. Gálisová A, Herynek V, Swider E, Sticová E, Pátiková A, Kosinová L, et al. A Trimodal Imaging Platform for Tracking Viable Transplanted Pancreatic Islets In Vivo: F-19 MR, Fluorescence, and Bioluminescence Imaging. Mol Imaging Biol (2019) 21(3):454–64. doi: 10.1007/s11307-018-1270-3
125. Hussain B, Kasinath V, Madsen JC, Bromberg J, Tullius SG, Abdi R. Intra-Organ Delivery of Nanotherapeutics for Organ Transplantation. ACS Nano (2021) 15(11):17124–36. doi: 10.1021/acsnano.1c04707
126. Mueller EA, Kovarik JM, van Bree JB, Grevel J, Lücker PW, Kutz K. Influence of a Fat-Rich Meal on the Pharmacokinetics of a New Oral Formulation of Cyclosporine in a Crossover Comparison With the Market Formulation. Pharm Res (1994) 11(1):151–5. doi: 10.1023/a:1018922517162
127. Ritschel WA. Microemulsion Technology in the Reformulation of Cyclosporine: The Reason Behind the Pharmacokinetic Properties of Neoral. Clin Transplant (1996) 10(4):364–73.
128. Forrest ML, Won CY, Malick AW, Kwon GS. In Vitro Release of the mTOR Inhibitor Rapamycin From Poly(Ethylene Glycol)-B-Poly(Epsilon-Caprolactone) Micelles. J Control Rel (2006) 110(2):370–7. doi: 10.1016/j.jconrel.2005.10.008
129. Khongkow M, Yata T, Boonrungsiman S, Ruktanonchai UR, Graham D, Namdee K. Surface Modification of Gold Nanoparticles With Neuron-Targeted Exosome for Enhanced Blood-Brain Barrier Penetration. Sci Rep (2019) 9(1):8278. doi: 10.1038/s41598-019-44569-6
130. Qie Y, Yuan H, von Roemeling CA, Chen Y, Liu X, Shih KD, et al. Surface Modification of Nanoparticles Enables Selective Evasion of Phagocytic Clearance by Distinct Macrophage Phenotypes. Sci Rep (2016) 6:26269. doi: 10.1038/srep26269
131. Guerrini L, Alvarez-Puebla RA, Pazos-Perez N. Surface Modifications of Nanoparticles for Stability in Biological Fluids. Mater (Basel Switzerland) (2018) 11(7):1154. doi: 10.3390/ma11071154
132. Blanco E, Shen H, Ferrari M. Principles of Nanoparticle Design for Overcoming Biological Barriers to Drug Delivery. Nat Biotechnol (2015) 33(9):941–51. doi: 10.1038/nbt.3330
133. Longmire M, Choyke PL, Kobayashi H. Clearance Properties of Nano-Sized Particles and Molecules as Imaging Agents: Considerations and Caveats. Nanomed (Lond Engl) (2008) 3(5):703–17. doi: 10.2217/17435889.3.5.703
134. Zein R, Sharrouf W, Selting K. Physical Properties of Nanoparticles That Result in Improved Cancer Targeting. J Oncol (2020) 2020:5194780. doi: 10.1155/2020/5194780
135. Glassman PM, Myerson JW, Ferguson LT, Kiseleva RY, Shuvaev VV, Brenner JS, et al. Targeting Drug Delivery in the Vascular System: Focus on Endothelium. Adv Drug Deliv Rev (2020) 157:96–117. doi: 10.1016/j.addr.2020.06.013
136. Braet F, Wisse E, Bomans P, Frederik P, Geerts W, Koster A, et al. Contribution of High-Resolution Correlative Imaging Techniques in the Study of the Liver Sieve in Three-Dimensions. Microsc Res Tech (2007) 70(3):230–42. doi: 10.1002/jemt.20408
137. Chen LT, Weiss L. The Role of the Sinus Wall in the Passage of Erythrocytes Through the Spleen. Blood (1973) 41(4):529–37. doi: 10.1182/blood.V41.4.529.529
138. Yu W, Liu R, Zhou Y, Gao H. Size-Tunable Strategies for a Tumor Targeted Drug Delivery System. ACS Cent Sci (2020) 6(2):100–16. doi: 10.1021/acscentsci.9b01139
139. Gentile F, Chiappini C, Fine D, Bhavane RC, Peluccio MS, Cheng MM, et al. The Effect of Shape on the Margination Dynamics of non-Neutrally Buoyant Particles in Two-Dimensional Shear Flows. J Biomech (2008) 41(10):2312–8. doi: 10.1016/j.jbiomech.2008.03.021
140. Alshamsan A, Binkhathlan Z, Kalam MA, Qamar W, Kfouri H, Alghonaim M, et al. Mitigation of Tacrolimus-Associated Nephrotoxicity by PLGA Nanoparticulate Delivery Following Multiple Dosing to Mice While Maintaining its Immunosuppressive Activity. Sci Rep (2020) 10(1):6675. doi: 10.1038/s41598-020-63767-1
141. Tang L, Azzi J, Kwon M, Mounayar M, Tong R, Yin Q, et al. Immunosuppressive Activity of Size-Controlled PEG-PLGA Nanoparticles Containing Encapsulated Cyclosporine a. J Transplant (2012) 2012:896141. doi: 10.1155/2012/896141
142. Alemdar AY, Sadi D, McAlister VC, Mendez I. Liposomal Formulations of Tacrolimus and Rapamycin Increase Graft Survival and Fiber Outgrowth of Dopaminergic Grafts. Cell Transplant (2004) 13(3):263–71. doi: 10.3727/000000004783983936
143. McAlister VC, Keshavamurthy M, Lee TD. Oral Delivery of Liposomal Tacrolimus: Increased Efficacy and Reduced Toxicity. Transplant Proc (1999) 31(1-2):1110. doi: 10.1016/s0041-1345(98)01923-x
144. Duivenvoorden R, Tang J, Cormode DP, Mieszawska AJ, Izquierdo-Garcia D, Ozcan C, et al. A Statin-Loaded Reconstituted High-Density Lipoprotein Nanoparticle Inhibits Atherosclerotic Plaque Inflammation. Nat Commun (2014) 5:3065. doi: 10.1038/ncomms4065
145. Ferrer MC, Shuvaev VV, Zern BJ, Composto RJ, Muzykantov VR, Eckmann DM. Icam-1 Targeted Nanogels Loaded With Dexamethasone Alleviate Pulmonary Inflammation. PloS One (2014) 9(7):e102329. doi: 10.1371/journal.pone.0102329
146. Phan HT, Haes A. Impacts of pH and Intermolecular Interactions on Surface-Enhanced Raman Scattering Chemical Enhancements. J Phys Chem C (2018) 122(26):14846–56. doi: 10.1021/acs.jpcc.8b04019
147. Lu G, Shrestha B, Haes A. Importance of Tilt Angles of Adsorbed Aromatic Molecules on Nanoparticle Rattle SERS Substrates. J Phys Chem C (2016) 120(37):20759–67. doi: 10.1021/acs.jpcc.6b02023
148. Li Y, Zhang H, Wu B, Guo ZJASS. Improving the Oxidation Resistance and Stability of Ag Nanoparticles by Coating With Multilayered Reduced Graphene Oxide. Appl Surf Sci (2017) 425:194–200. doi: 10.1016/j.apsusc.2017.07.054
149. Hotze EM, Phenrat T, Lowry GV. Nanoparticle Aggregation: Challenges to Understanding Transport and Reactivity in the Environment. J Environ Qual (2010) 39(6):1909–24. doi: 10.2134/jeq2009.0462
150. Northrup L, Christopher MA, Sullivan BP, Berkland C. Combining Antigen and Immunomodulators: Emerging Trends in Antigen-Specific Immunotherapy for Autoimmunity. Adv Drug Deliv Rev (2016) 98:86–98. doi: 10.1016/j.addr.2015.10.020
151. Stabler CL, Li Y, Stewart JM, Keselowsky BG. Engineering Immunomodulatory Biomaterials for Type 1 Diabetes. Nat Rev Mater (2019) 4(6):429–50. doi: 10.1038/s41578-019-0112-5
152. Shirali AC, Look M, Du W, Kassis E, Stout-Delgado HW, Fahmy TM, et al. Nanoparticle Delivery of Mycophenolic Acid Upregulates PD-L1 on Dendritic Cells to Prolong Murine Allograft Survival. Am J Transplant (2011) 11(12):2582–92. doi: 10.1111/j.1600-6143.2011.03725.x
153. Shah S, Daneshmandi S, Hughes KR, Yu S, Bedoya AM, Shea LD, et al. Optimizing PLG Nanoparticle-Peptide Delivery Platforms for Transplantation Tolerance Using an Allogeneic Skin Transplant Model. Biomaterials (2019) 210:70–82. doi: 10.1016/j.biomaterials.2019.04.030
154. Prasad S, Neef T, Xu D, Podojil JR, Getts DR, Shea LD, et al. Tolerogenic Ag-PLG Nanoparticles Induce Tregs to Suppress Activated Diabetogenic CD4 and CD8 T Cells. J Autoimmun (2018) 89:112–24. doi: 10.1016/j.jaut.2017.12.010
155. Yeste A, Takenaka MC, Mascanfroni ID, Nadeau M, Kenison JE, Patel B, et al. Tolerogenic Nanoparticles Inhibit T Cell-Mediated Autoimmunity Through SOCS2. Sci Signal (2016) 9(433):ra61. doi: 10.1126/scisignal.aad0612
156. Lewis JS, Stewart JM, Marshall GP, Carstens MR, Zhang Y, Dolgova NV, et al. Dual-Sized Microparticle System for Generating Suppressive Dendritic Cells Prevents and Reverses Type 1 Diabetes in the Nonobese Diabetic Mouse Model. ACS Biomater Sci Eng (2019) 5(5):2631–46. doi: 10.1021/acsbiomaterials.9b00332
157. Kontos S, Kourtis IC, Dane KY, Hubbell JA. Engineering Antigens for in Situ Erythrocyte Binding Induces T-Cell Deletion. Proc Natl Acad Sci USA (2013) 110(1):E60–8. doi: 10.1073/pnas.1216353110
Keywords: nanotechnology, kidney, islet, transplantation, promising field
Citation: Wang W, Teng Y, Xue J-J, Cai H-K, Pan Y-B, Ye X-N, Mao X-L and Li S-W (2022) Nanotechnology in Kidney and Islet Transplantation: An Ongoing, Promising Field. Front. Immunol. 13:846032. doi: 10.3389/fimmu.2022.846032
Received: 30 December 2021; Accepted: 08 March 2022;
Published: 08 April 2022.
Edited by:
Lisha Mou, Shenzhen Second People’s Hospital, ChinaReviewed by:
Shinichi Matsumoto, National Center for Global Health and Medicine, JapanCopyright © 2022 Wang, Teng, Xue, Cai, Pan, Ye, Mao and Li. This is an open-access article distributed under the terms of the Creative Commons Attribution License (CC BY). The use, distribution or reproduction in other forums is permitted, provided the original author(s) and the copyright owner(s) are credited and that the original publication in this journal is cited, in accordance with accepted academic practice. No use, distribution or reproduction is permitted which does not comply with these terms.
*Correspondence: Xin-Li Mao, bGlfc2hhb3dlaTgxQGhvdG1haWwuY29t; Shao-Wei Li, bGlfc2hhb3dlaTgxQGhvdG1haWwuY29t
Disclaimer: All claims expressed in this article are solely those of the authors and do not necessarily represent those of their affiliated organizations, or those of the publisher, the editors and the reviewers. Any product that may be evaluated in this article or claim that may be made by its manufacturer is not guaranteed or endorsed by the publisher.
Research integrity at Frontiers
Learn more about the work of our research integrity team to safeguard the quality of each article we publish.