- 1Committee on Immunology, The University of Chicago, Chicago, IL, United States
- 2Committee on Cancer Biology, The University of Chicago, Chicago, IL, United States
- 3Department of Pathology, The University of Chicago, Chicago, Chicago, IL, United States
- 4Department of Medicine, Section of Hematology/Oncology, The University of Chicago, Chicago, IL, United States
- 5Center for Discovery and Innovation, Hackensack University Medical Center, Nutley, NJ, United States
- 6Department of Molecular Hematology, Lund University, Lund, Sweden
- 7Department of Biochemistry and Molecular Genetics, Northwestern University, Chicago, IL, United States
T lymphocyte acute lymphoblastic leukemia (T-ALL) is a heterogeneous disease affecting T cells at multiple stages of their development and is characterized by frequent genomic alterations. The transcription factor LEF1 is inactivated through mutation in a subset of T-ALL cases but elevated LEF1 expression and activating mutations have also been identified in this disease. Here we show, in a murine model of T-ALL arising due to E2a inactivation, that the developmental timing of Lef1 mutation impacts its ability to function as a cooperative tumor suppressor or oncogene. T cell transformation in the presence of LEF1 allows leukemic cells to become addicted to its presence. In contrast, deletion prior to transformation both accelerates leukemogenesis and results in leukemic cells with altered expression of genes controlling receptor-signaling pathways. Our data demonstrate that the developmental timing of Lef1 mutations impact its apparent oncogenic or tumor suppressive characteristics and demonstrate the utility of mouse models for understanding the cooperation and consequence of mutational order in leukemogenesis.
Introduction
T acute lymphoblastic leukemia (T-ALL) is an aggressive malignancy that accounts for approximately 10-15% of pediatric and 25% of adult leukemia (1). This disease arises in thymocytes leading to an enlarged thymus and respiratory distress; however, leukemic cells can disseminate through the blood and infiltrate tissues. Treatment involves intensive chemotherapy but 25% of pediatric cases and nearly 50% of adult cases show therapy resistance or relapse within 5 years (1). T-ALL is a consequence of transformation of cells at multiple stages of T cell differentiation (2, 3) and distinct subsets of T-ALL can be identified by their unique gene expression signatures, genetic alterations, and response to therapy (1, 4, 5). Recent analysis further highlights that clonal evolution and progressive mutation contribute to disease evolution but much remains to be learned about the consequences of individual mutations and mutational order on transformation (6). Understanding the molecular pathogenesis of T-ALL is crucial for the improved development of prognostic markers and tailored therapeutic approaches.
The most broadly occurring mutations in T-ALL affect the Notch signaling pathway. Mutations in the NOTCH1 gene occur in > 60% of cases (7). Mutations occur within the heterodimerization domain, resulting in ligand independent activation, and the intracellular PEST domain, resulting in stabilization of the transcriptionally active form (intracellular Notch1/ICN). In another 15% of T-ALL, mutations occur in FBXW7, which encodes an ubiquitin ligase involved in the PEST-domain dependent degradation of ICN (8–10). Further, epigenetic alterations affecting the Notch1 gene impact the site of transcription initiation and splicing and result in ligand independent activation (11–13). Consistent with the oncogenic role of Notch1, ectopic expression of constitutively active forms of Notch1 in mouse T cell progenitors leads to their transformation (14).
Activation of the TAL1 and LYL1 genes is also associated with subsets of T-ALL (4). Tal1 and Lyl1 are basic helix-loop-helix proteins that bind DNA in association with the E protein transcription factors (15), which are critical for T cell development (16). While Tal1:E protein and Lyl1:E protein dimers have targets implicated in T-ALL (17–21), their ability to inhibit E protein homodimer formation is sufficient to promote T cell transformation as revealed by the development of T-ALL like disease in E2a-/- mice and in mice ectopically expressing inhibitors of E protein DNA binding (22–25). E2a-/- leukemias are characterized by recurrent mutations in the Notch1 PEST domain and altered Notch1 splicing and transcription initiation leading to ligand independence (11, 26).
A critical target of Notch1 in many cases of T-ALL is c-myc (27–29). However, in E2a-/- leukemias Notch1 signaling is essential but it regulates expression of Lef1, encoding a TCF1-related transcription factor that is an effector of the Wnt signaling pathway (26, 30, 31). LEF1 appears essential for the survival of E2a-/- leukemias since siRNA-mediated knockdown of Lef1 causes cell cycle arrest and the death of leukemias in vitro (30). Other murine models of T-ALL, including those arising in Tcf7-/- and Ikzf1-/- mice also show increased expression of Lef1 (32, 33). Recently, a study of childhood ALL, including 28 patients with T-ALL, revealed a positive prognostic value to high LEF1 expression and a second study confirmed this for specific LEF1 isoforms (34, 35). However, in a study of adult T-ALL, 25% of patients had elevated expression of LEF1 that was associated with a poor prognosis (36). In 4 patients, mutations in LEF1 (K86E and P106L) were found to augment the transcriptional capacity of LEF1. In contrast to these findings of increased LEF1 expression or function, a subset of human T-ALL (18-27%) have inactivating mutations within the LEF1 gene (5, 37). These observations suggest that LEF1 can function as both a pro- and anti-leukemia factor but a molecular understanding of the basis for these distinct functions is currently unknown.
To gain insight into the role of LEF1 in T-ALL we investigated its requirement in the generation of E2a-/- T cell leukemias. We demonstrate that E2a-/- leukemias that arose in mice sufficient for LEF1 became dependent on this transcription factor for their survival. However, deletion of Lef1 prior to T cell transformation resulted in significant alterations in T cell development and a reduced latency to leukemic morbidity. Leukemic cells arising in the latter context resembled E2a-/- leukemias in that they had mutations in Notch1 and dependence on the Notch signaling pathway as well as frequent trisomy of chromosome 15, and hence increased c-myc expression. However, they differed from E2a-/- leukemias in that they had a CD4loCD8lo phenotype with increased peripheral cell numbers at the time of sacrifice. Cell lines generated from these leukemias reveal differences in expression of multiple genes associated with monocarboxylic transport and hedgehog signaling that could also impact T cell receptor signaling. Our study describes novel models for studying LEF1 function in T-ALL and indicate that LEF1 is a modulator of leukemic transformation providing both addictive and inhibitory functions depending on its availability during transformation.
Methods and Materials
Mice
Mice were backcrossed onto an FvB/NJ background for at least 8 generations. All experiments were performed in compliance with the University of Chicago Institutional Animal Care and Use Committee. E2a-/-, Lef1f/f, and Lck-Cre mice and genotyping protocols were described previously (38). FvBn/J mice and Lck-Cre mice were purchased from the Jackson Laboratory.
Flow Cytometry
Thymocytes or splenocytes were dissected and dispersed using frosted glass slides followed by filtration through a 100 mM cell strainer. Cells were stained at a concentration of 2 x 107 cells/ml in FACS buffer (PBS + 5% FCS +.02% azide) after incubation with FcBlock. Intracellular staining was performed using the FoxP3/Transcription Factor Staining Kit. Antibodies were purchased from BioLegend, eBiosciences or Fischer Scientific and specific antibody clones and fluorochromes are available upon request. Antibodies used: Lef1, CD4, CD8, CD117, CD25, CD44, TCRb, CD11b, CD11c, DX5, B220, Gr1. Data was acquired on an LSRII or Fortessa and analyzed using FlowJo (TreeStar).
Cell Lines
Leukemia cell lines were generated by culturing thymic cells from moribund mice in OPTI-MEM media containing 10% FCS, 2-mercaptoethanol and Pen/Strep/Glu for greater than 2 weeks. All established lines were frozen in 5% DMSO/50%FCS in liquid nitrogen for long term storage.
In Vitro Culture
OP9-DL1 stromal cells were maintained in OPTI-MEM and plated 1 day before used to achieve a near confluent monolayer of cells. Multipotent progenitors were isolated as Ter119-Gr1- cells from e13 fetal liver and cultured on OP9-DL1 in the presence of 5 ng/ml Flt3 ligand, IL-7 and CD117 ligand.
Retroviral Transduction
The retroviral vectors MigR1, MigR1-Cre, MigR1-DNMAML were described previously (39). Retroviral plasmid DNA was isolated using CsCl. Retroviral supernatants were produced by transfecting plasmid DNA into Phoenix cells using Ca2PO4 precipitation and cells were transduced with retrovirus as previously described (40).
RNA Extraction, Microarray, Sequencing and Analysis
RNA was extracted using Trizol Reagent. For microarray analysis, RNA was converted to cDNA that was used to probe Affymetrix MOE 430_2 arrays as previously described (41). Raw array data were normalized with RMAexpress (http://rmaexpress.bmbolstad.com/) and analyzed by dChip (http://www.biostat.harvard.edu/complab/dchip/). Probe set annotation was obtained from Affymetrix. For RNA-seq analysis, RNA was sequenced on a Next-Seq500 and analyzed as described (42). Raw sequence reads were trimmed using Trimmomatic v0.33 (TRAILING:30 MINLEN:20) and then aligned to mouse genome assembly mm10 with TopHat v 2.1.0. Reads were assigned to genes using the htseq-count tool from HTSeq v 0.6.1 and gene annotations from Ensembl release 78. The R package EdgeR was used to normalize the gene counts and to calculate differential expression statistics for each gene for each pairwise comparison of sample groups. Metascape analysis was performed on differential gene expression lists (https://metascape.org) (43). Genes were considered differentially expressed at Log2FC with an adj. p-valule of <0.01.
Quantitative PCR
RNA was reverse transcribed with Superscript III (Invitrogen). Quantitative RT-PCR was performed in an iCycler (Bio-Rad Laboratories) with SYBR Green (Bio-Rad Laboratories). Expression values were normalized to Hprt and were calculated by the ΔCT method. Primer sequences are available on request.
Spectral Karyotyping Analysis
To characterize the cytogentic pattern of T cell leukemias from E2a-/-Lef1 Δ/Δ mice, SKY analysis was performed using the ASI SkyPaintTM assay for mouse chromosomes as described previously (44) on cell lines or fresh leukemic cells from moribund mice with leukemia (10 metaphase cells were analyzed per case). Karyotype results are in Table S2.
Western Blot
Total protein extracts were prepared and analyzed by Western blot analysis as described previously (30). Primary antibodies used were anti-Notch1 antibody (V1744) reactive with the cleaved cytoplasmic domain (Cell Signaling Technology) and anti-actin (Abcam).
Data Sharing Statement
For original data please contact bkee@bsd.uchicago.edu. RNA-sequencing data can be accessed in the Gene Expression Omnibus under GSE186420. Microarray data is under GSE196391.
Results
LEF1 Is Required for the Survival of E2A-Deficient T Cell Leukemias
Our previous studies revealed that Notch1 is mutated in E2a-/- T cell leukemias and required for their survival (26). We identified Lef1 as a target of the Notch pathway in these cells and demonstrated that siRNA directed against Lef1 reduced the viability of these leukemic cells (30). Here, using flow cytometry, we found that LEF1 and TCF1 protein are detected in E2a-/- leukemias and that LEF1, but not TCF1, was reduced after treatment of cells with a γ-secretase inhibitor, which antagonizes Notch signaling (Figures 1A, B). To rigorously demonstrate that LEF1 was required for growth of these leukemias, we created E2a-/- mice that were homozygous for alleles of Lef1 with loxp sites flanking the DNA binding domain (32). We generated 2 lines from the leukemias arising in these mice and used a retrovirus producing Cre to delete the DNA binding domain of LEF1 (Figure 1C). The retrovirus produced GFP in addition to Cre and therefore we could track Cre expressing cells by their expression of GFP (Figure 1C, D). LEF1 was lost from a subset of cells after transduction with MigR1-Cre and the frequency of LEF1 negative cells mirrored the frequency of GFP+ cells (Figure 1D). QPCR analysis of sorted GFP+ cells from MigR1 or MigR1-Cre transduced cells revealed decreased Lef1 mRNA after transduction of E2a-/-Lef1f/f but not E2a-/- leukemias with MigR1-Cre (Figure 1E). We tracked the fate of cells with deletion of Lef1 by following the frequency of GFP+ cells. GFP+ cells in MigR1-Cre expressing E2a-/-Lef1f/f cells declined steadily over time consistent with the loss of cells that lacked LEF1 (Figure 1F). An E2a-/- leukemia line that was heterozygous for the Lef1f allele (E2a-/-Lef1f/+) and transduced with MigR1-Cre also showed reduced representation of GFP+ cells with time in culture, although not to the degree of E2A-/-Lef1f/f leukemias (Figure 1F). In contrast, the same cell lines transduced with MigR1, which does not promote deletion of Lef1, demonstrated a stable frequency of GFP+ cells over time (Figures 1D–F). Similarly, E2a-/- lines transduced with either MigR1 or MigR1-Cre showed stable GFP expression (Figure 1F). To further examine the impact of LEF1-deletion on these leukemic cells, we analyzed the transcriptome of GFP+ cells 72 hours after transduction with MigR1 or MigR1-Cre. We found decreased expression of multiple signaling-associate genes (Id3, Syk, Sgk, Rasgrp1) and increased expression of Cdkn1a, encoding the cell cycle inhibitor p21 (Figure 1G and Table S1). The increased expression of p21 could contribute to the reduced expansion of LEF1-deleted leukemias. These data demonstrate that LEF1 is required for the maintenance of E2a-/- T cell leukemia lines in vitro and are consistent with our previous studies using Lef1 siRNA (30).
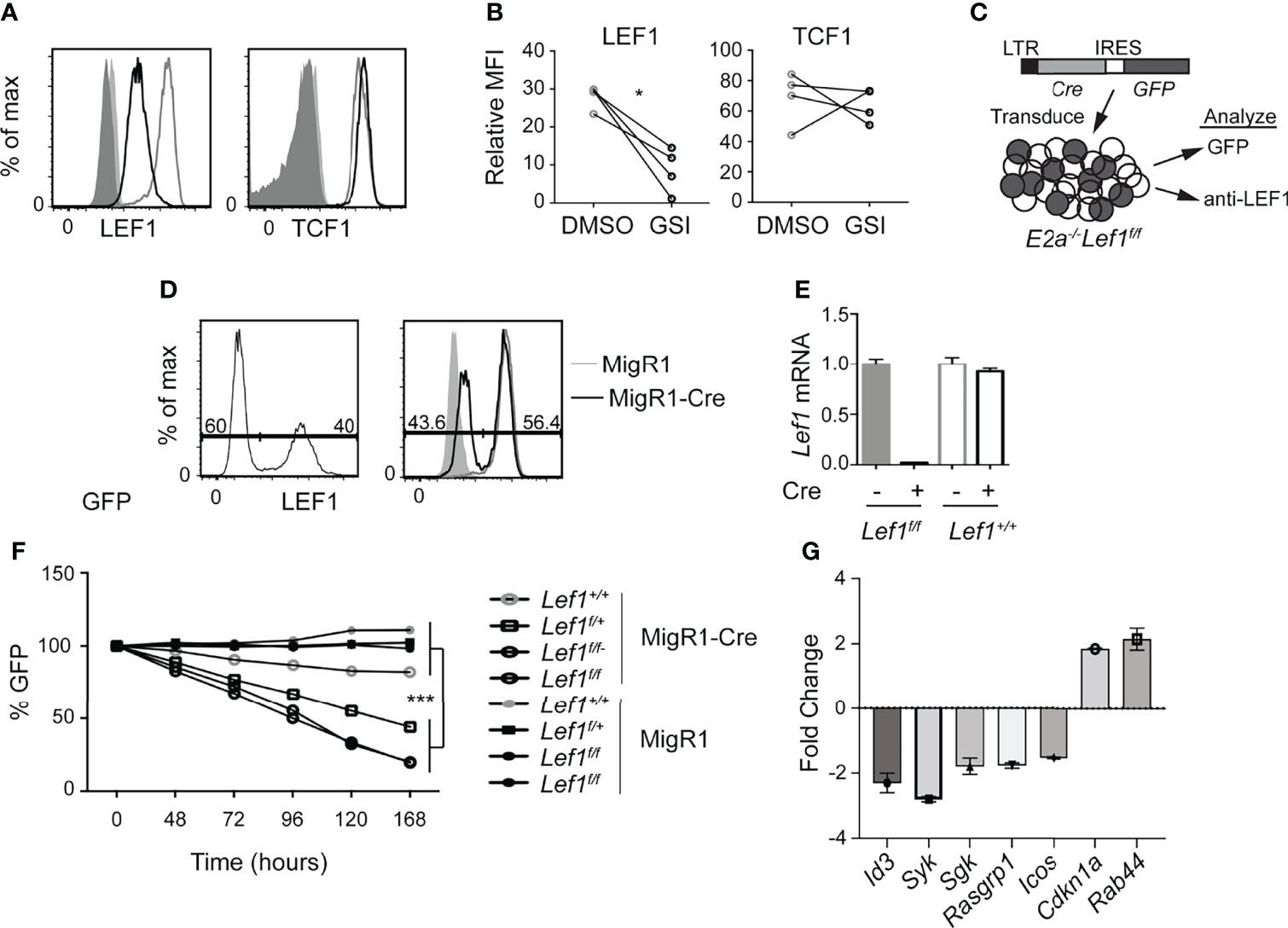
Figure 1 LEF1 is required for the survival of established E2a-/- T cell leukemias. (A) Flow cytometry for LEF1 (left) or TCF1 (right) in an E2a-/- T cell leukemia line treated with DMSO (grey) or the Notch1 inhibitor GSI (black). Shaded histograms are isotype control stainings. n = 4 (B) Summary of the MFI for LEF1 or TCF1 in multiple E2a-/- leukemia lines. *p < 0.05, Students t-test. (C) Schematic representation of experiment to inactivate Lef1 in E2a-/-Lef1f/f T cell leukemias. (D) Expression of GFP (left) or LEF1 (right) in E2a-/-Lef1f/f leukemias transduced with MigR1-Cre (black). For LEF1 staining, MigR1 transduced cells (grey) and isotype controls (shaded histograms) are also shown. (E) qRT-PCR analysis for Lef1 mRNA in GFP+ cells isolated from E2a-/- leukemias with Lef1f/f or Lef1+/+ 96 hours after transduction with MigR1 or MigR1-Cre. Error bars represent standard deviation. (F) The relative percent of GFP expressing cells with time in culture after retroviral transduction of E2a-/-Lef1+/+, E2a-/-Lef1f/+ or E2a-/-Lef1f/f leukemias with MigR1 or MigR1-Cre retrovirus. Data are representative of n = 4 (A, B), n = 2 (D, E) and n = 3 (F) experiments. ***p < 0.005. Students t-test on E2a-/-Lef1F/F and E2a-/-Lef1F/+ compared to E2a-/-Lef1+/+ leukemias at t = 168 hours. (G) RNA from a GFP+ E2A-/-Lef1F/F leukemia transduced with MigR1 or MigR1-Cre was analyzed at t = 72 hours by microarray. The graph depicts the FC between MigR1 and MigR1-Cre transduced cells for selected genes and 95% confidence.
LEF1 Is Increased in E2a-/- DN3 Thymocytes and Promotes Their Differentiation
To determine whether the increased expression of LEF1 occurs in pre-leukemic mice, we examined the Lineage- population of the thymus for expression of LEF1. As expected, there were fewer DN3 thymocytes in E2a-/- mice compared to control with an increased frequency of CD117loCD25int cells, previously shown to be innate lymphoid cells (Figure 2A) (45, 46). The E2a-/- DN3 cells expressed substantially more LEF1 than control DN3 thymocytes (Figure 2B). By qRT-PCR we found increased expression of Lef1 mRNA in E2a-/- DN3 thymocytes (Figure 2C). DN3 thymocytes fail to develop from E2a-/- multipotent progenitors cultured in vitro on OP9-DL1 (45, 47), indicating that these cells are compromised with respect to T cell differentiation. To determine whether LEF1 might provide an advantage to these cells, we used a retrovirus to force multipotent progenitor (MPP) cells to ectopically express LEF1. E2a-/- MPPs transduced with the MigR1 retrovirus, which produce GFP only, failed to generate DN3 cells in vitro, as expected (Figures 2D, E). In contrast, E2a-/- MPPs transduced with LEF1 producing retrovirus generated DN3 cells and an increased frequency of DN2 cells. Notably, even control MPPs transduced with LEF1 producing retrovirus showed increased generation of DN2 and DN3 cells (Figures 2D, E). This propensity to promote differentiation of control and E2a-/- MPPs was not dependent on the presence of the β-catenin interaction domain (CAT) as a retrovirus producing a mutant form of LEF1 lacking the CAT domain also supported differentiation (Figures 2D, E). These data lead us to hypothesize that the increased expression of LEF1 in E2a-/- DN3 thymocytes aids in their differentiation from more immature progenitors and that LEF1’s essential functions are independent of its interaction with β-catenin.
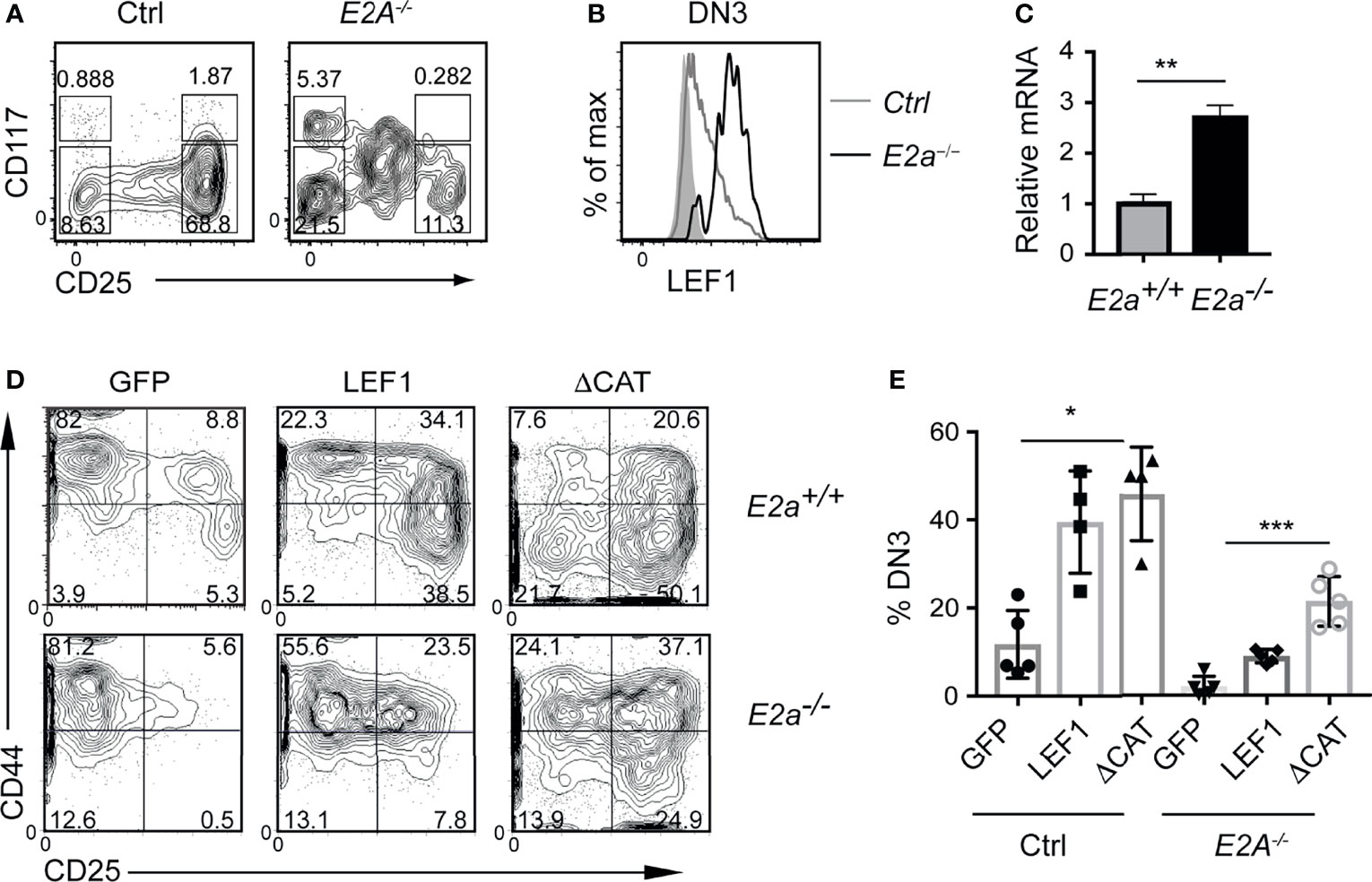
Figure 2 LEF1 is highly expressed in E2a-/- DN3 cells and promotes their development from MPPs. (A) Flow cytometry showing CD117 and CD25 on Lineage- (CD4, CD8, CD11b, Ter119) thymocytes from Ctrl (left) and E2a-/- (right) mice at 5 weeks of age. (B) LEF1 expression in DN3 thymocytes from Ctrl (grey) and E2a-/- (black) mice. (C) Summary of Lef1 mRNA relative to Hprt mRNA in Ctrl (grey) and E2a-/- (black) DN3 thymocytes. Data are representative of mice at 5-6 weeks of age. **p < 0.01 Student’s t-test. (D) Flow cytometry of MPPs isolated from Ctrl (top) or E2a-/- (bottom) embryos (16 days p.c.) cultured in vitro for 10 days after retroviral transduction with MigR1 (GFP), MigR1-LEF1 or MigR1-LEF1ΔCAT. Data are gated on GFP+ cells. (E) Summary of the %DN3 cells in the Lineage- population of MPPs cultured in vitro as in (D) from the indicated strains. Data are representative of (A, B) n = 3, (C) n = 2, (D, E) n = 4-6 experiments. *p < 0.05, **p < 0.01 and ***p < 0.005 ANOVA.
T Cell Specific Deletion of Lef1 in E2a-/- Mice Abrogates DN3 Development
To test the hypothesis that LEF1 is essential for the development of E2a-/- DN3 cells and for leukemic transformation, we generated Lck-Cre E2a-/-Lef1f/f mice, which express Cre starting at the DN2 stage. Lck-Cre+ E2a-/-Lef1f/f mice (DKO) and E2a-/- mice had similar numbers of thymocytes and Lineage- thymocytes (Figures 3A–C). However, the Lineage- population of DKO mice showed a reduced frequency of DN3 thymocytes (Figures 3B, D). The number of DN3 thymocytes was also significantly decreased in DKO compared to E2a-/- mice (Figure 3E). In contrast Lck-Cre+ Lef1f/f (Lef1Δ/Δ) mice had thymocyte and Lineage- thymocyte numbers, as well as DN3 frequencies that were similar to Control mice (Figure 3D), indicating that Lef1 deletion did not have a major impact on these cells.
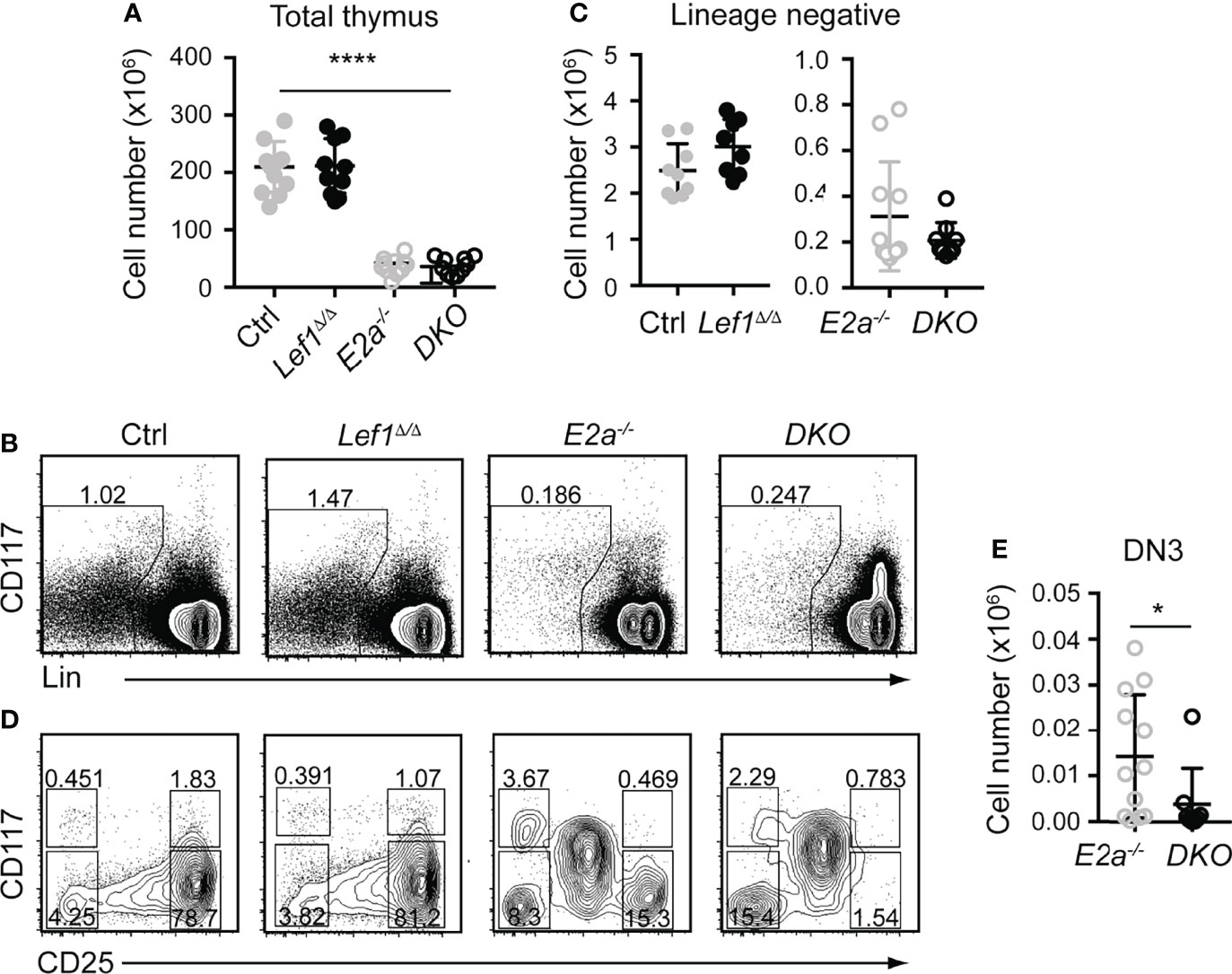
Figure 3 T cell specific deletion of Lef1 from E2a-/- mice does not impact thymocyte numbers but results in a loss of DN3 cells. (A) Total thymocyte numbers from mice of the indicated genotype. ****p < 0.001, Anova for multiple comparisons. (B) Flow cytometry showing Lineage markers (CD8, TCRβ, TCRγδ, CD11b, CD11c, NK1.1) and CD117 and the gating strategy for Lin- cells. (C) Lin- thymocyte numbers in mice of the indicated genotypes. (D) Flow cytometry showing CD25 and CD117 on Lin- thymocytes. (E) Summary of DN3 thymocyte numbers in mice of the indicated genotypes. Data is representative on more than 8 experiments *p < 0.05, Students t-test. Mice were between 5-7 weeks of age.
Since the number of thymocytes was similar in E2a-/- and DKO mice we examined the phenotype of more mature thymocytes. As previously reported, E2a-/- thymocytes had a reduced frequency of CD4+CD8+ (DP) thymocytes with an increased frequency of CD4+ and CD8+ cells. The CD4 by CD8 profile of DKO thymocytes revealed a slightly increased frequency of CD4+CD8+ (DP) cells and a decreased frequency of CD4+ of CD8+ single positive thymocytes compared to E2a-/- mice (Figure 4A). E2a-/- mice also have an increased frequency of TCRβ+ thymocytes, although the number of these cells is lower than in Ctrl mice (Figures 4A, B). The frequency and number of TCRβ+ cells was reduced in DKO mice compared to E2a-/- mice, although it was still higher than in Ctrl or Lef1Δ/Δ mice (Figures 4A, B). These data suggest that deletion of LEF1 had a subtle but significant impact on the differentiation of thymocytes in the absence of E2a. Interestingly, a substantial portion of DKO Lineage+ thymocytes, which are primarily DP cells, expressed CD25 (Figures 4C, D). The frequency of Lineage+CD25+ cells was also higher in E2a-/- thymocytes than in Ctrl or Lef1Δ/Δ thymocytes but was not significantly elevated in number (Figures 4C, D). Indeed, a direct comparison of DP thymocytes revealed a substantial increase CD25 on DKO compared to E2a-/- thymocytes (Figure 4E). Given that CD25 is a known Notch1 target gene (48), we tested the hypothesis that Notch1 was expressed in DP thymocytes from DKO mice. By QPCR analysis we observed mRNA for Notch1 and the Notch1 target gene Nrarp in DP thymocytes from DKO but not E2a-/- or Ctrl mice (Figure 4F). These data indicate that Notch1 is activated in DKO DP thymocytes.
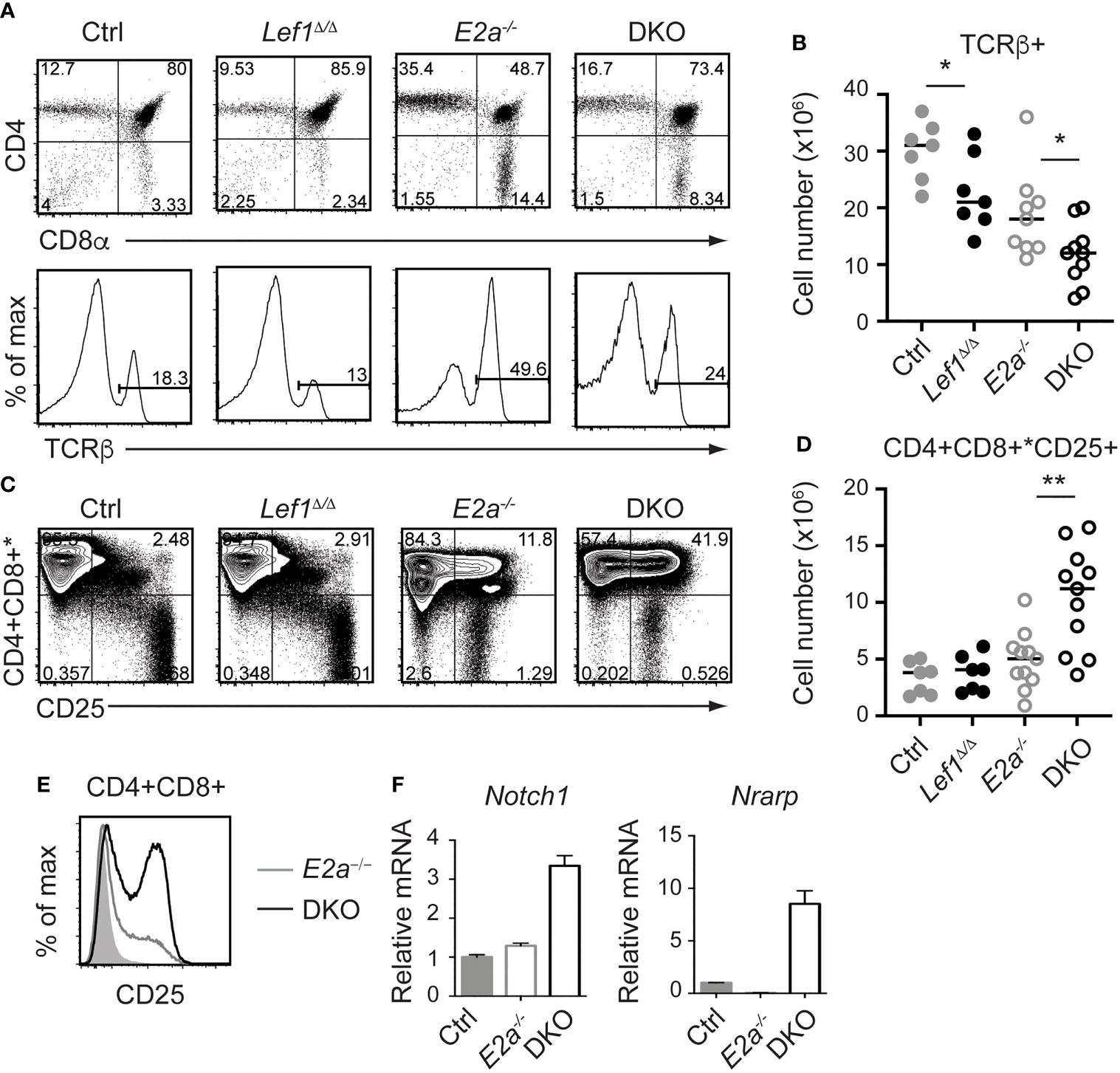
Figure 4 LEF1 restrains expression of CD25, Notch1, and Nrarp in E2a-/- CD4+CD8+ thymocytes. (A) Flow cytometry showing CD4 and CD8 (top) or TCRβ (bottom) on thymocytes from mice of the indicated genotype. (B) Summary of the number of TCRβ+ thymocytes in the indicated strains. (C) Flow cytometry showing expression of CD4 and CD8 (* indicates CD11b, CD11c, CD3e were also in this panel) and CD25. *p < 0.05, Student’s t-test. (D) Total number of CD4+CD8+*CD25+ cells in mice of the indicated genotype. **p < 0.01, Student’s t-test. (E) Flow cytometry of CD25 on CD4+CD8+ thymocytes from WT (shaded), E2a-/- (grey) and DKO (black) mice. (F) qRT-PCR for Notch1 and Nrarp on sorted CD4+CD8+ thymocytes from mice of the indicated genotype. Data is representative of >6 mice (A-D) or 2 experiments (E, F). Mice were 5-7 weeks of age.
DKO Mice Develop T Cell Leukemia With Reduced Latency
To determine whether deletion of Lef1 impacted the transformation potential of E2a-/- thymocytes, we allowed the mice to age and monitored them for signs of leukemia. Surprisingly, DKO mice became moribund with an average latency of 100 days (range 80-130 days) whereas the average latency for E2a-/- mice was 130 days (range 100-170 days), and notably, all mice that we followed developed disease (Figure 5A). At sacrifice, leukemia was confirmed by counting thymocyte numbers and by flow cytometry of multiple tissues. Cell numbers were similar in the thymus of moribund E2a-/- or DKO mice, although the range was greater for the DKO cells (Figure 5B). However, splenic lymphocyte numbers were elevated in the moribund DKO as compared to E2a-/- mice (Figure 5C). Primary leukemic cells in DKO mice were frequently low for CD4 and CD8 whereas E2a-/- leukemias were CD4hiCD8hi or contained SP cells (Figures 5D, E). The DKO leukemias also expressed CD25 without CD44, a phenotype that is distinct from that of the majority of E2a-/- leukemias (Figure 5E). Taken together, these data demonstrate that, despite the reduced number of DN3 cells, DKO mice developed T cell leukemia with reduced latency compared to E2a-/- mice and these leukemias had a distinct surface receptor phenotype.
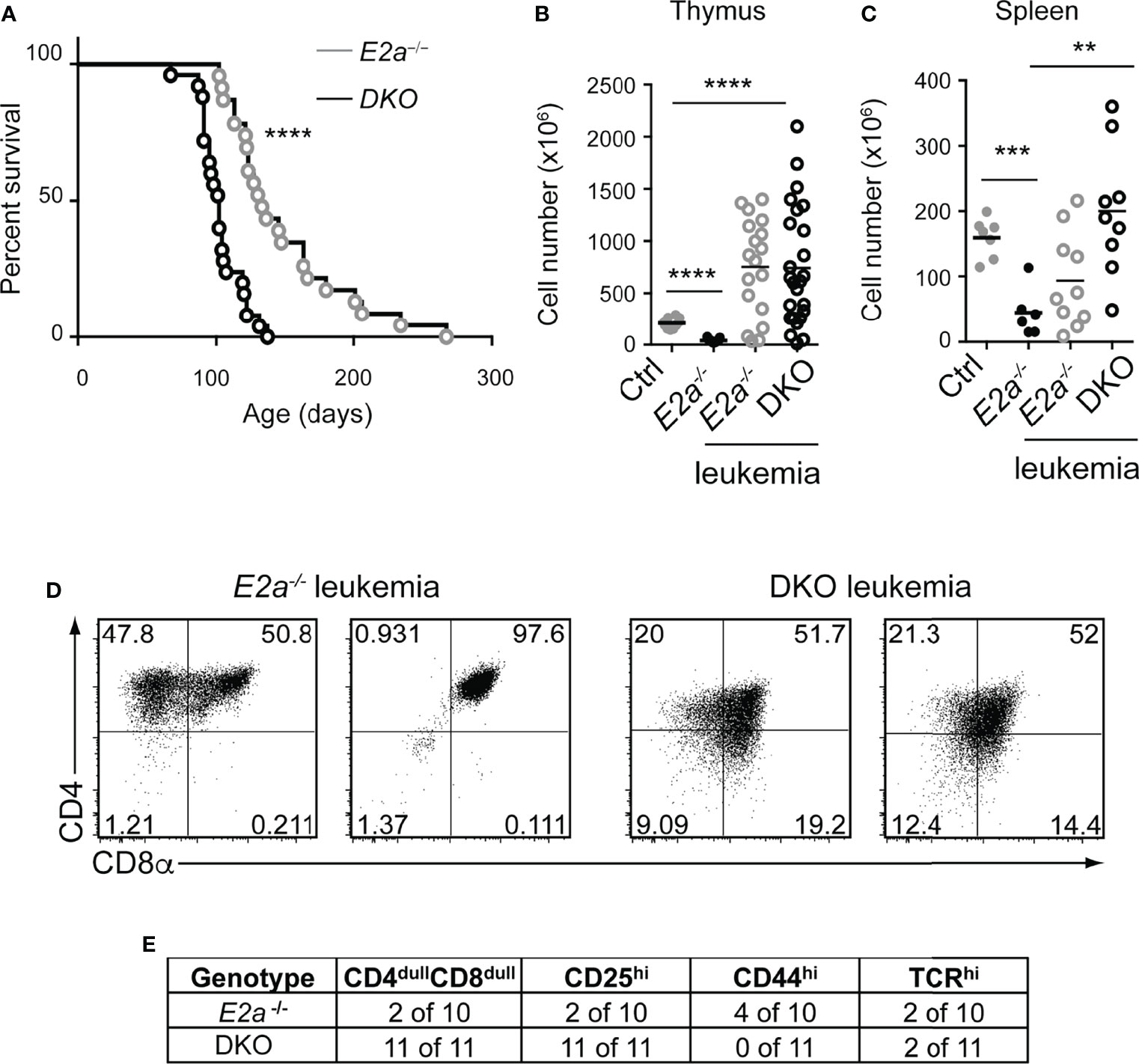
Figure 5 DKO mice have an accelerated onset of T cell leukemia. (A) Kaplan-Meier plot of leukemia incidence in E2a-/- and DKO mice. ****p < 0.0001, Mantel-Cox Test (B) Total thymocyte numbers and (C) spleen lymphocyte numbers at time of morbidity in E2a-/- and DKO mice compared to age matched Ctrl and non-moribund E2a-/- mice. ** p< 0.01, ***p < 0.005, ****p < 0.001. Student’s t-test for pairwise comparisons. (D) Flow cytometry showing CD4 and CD8 on two representative E2a-/- and DKO thymic leukemias. (E) Summary of phenotype of E2a-/- and DKO leukemias.
DKO Leukemias Have Notch1 Mutations and Require Notch Signaling
E2a-/- leukemias have mutations in the Notch1 gene and they are dependent on Notch signaling for their survival (26). To determine whether DKO leukemias also had mutations in the Notch1 gene, we PCR amplified the 3’ portion of Notch1 and performed sequencing on 3 DKO cell lines that we established in culture. Notably, we found insertions that resulted in out of frame translation of the PEST domain of Notch1 in all of the DKO lines (Figure 6A). These mutations are predicted to stabilize ICN1 and, indeed, ICN1 protein could be detected in these leukemias by western blot analysis (Figure 6B). To determine whether these leukemias were dependent on Notch1 signaling we used retroviral transduction to ectopically express a dominant negative version of the Notch1 co-activator MAML in these cells (39). The frequency of cells expressing DN-MAML, identified by their expression of GFP, declined over time in culture regardless of whether they were E2a-/- or DKO leukemias (Figure 6C). In contrast, the frequency of GFP+ cells remained stable in cultures transduced with the control MigR1 retrovirus (Figure 6C). Cytogenetic analysis using spectral karyotyping of three DKO primary leukemias revealed trisomy for chromosome 15 containing the c-Myc locus, a feature that is also observed in E2a-/- leukemias (Figures 6C, D and Table S2) (24). Consistent with this observation, E2a-/- and DKO cell lines had similar levels of c-Myc mRNA (Figure 6E). These data demonstrate that DKO leukemias, like E2a-/- leukemias, required Notch signaling for their survival and had mutations impacting the stability of ICN1 expression of c-Myc.
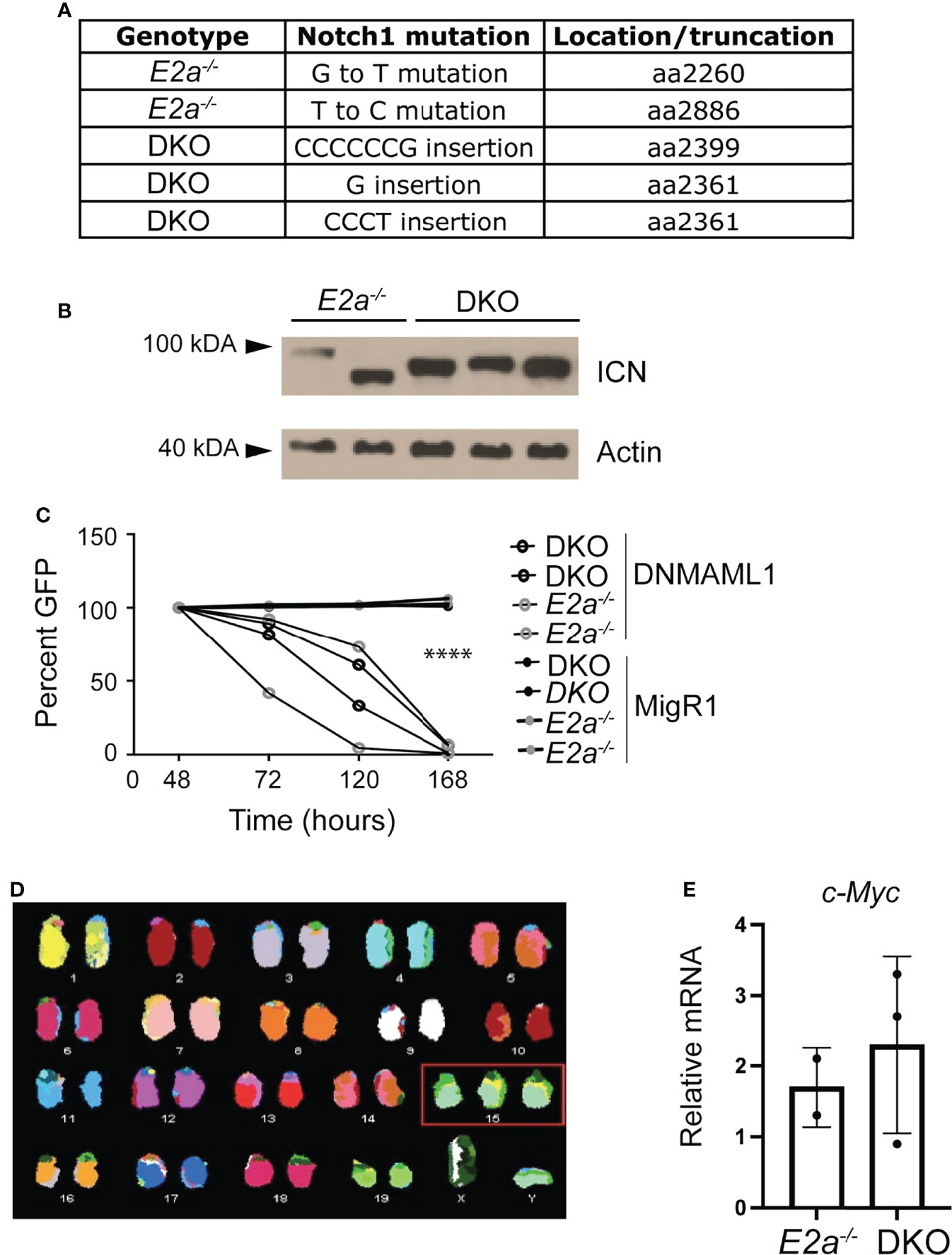
Figure 6 DKO leukemias require Notch1 signaling and have amplification of chromosome 15. (A) Identification of mutations in the Notch1 gene in two E2a-/- and 3 DKO leukemias. (B) Western Blot Analysis for cleaved ICN1 in the indicated leukemia lines. Actin is shown as a loading control. (C) Relative GFP expression in leukemias of the indicated genotype after transduction with MigR1 or MigR1-DNMAML. The frequency of GFP+ cells was assessed by flow cytometry at the indicated times after transduction. ****p < 0.0001 Students t-test on MigR1 versus DNMAML infected cells (D) Spectral karyotyping analysis of metaphase cells isolated from a DKO leukemia. (E) qRT-PCR analysis for c-Myc in 2 E2a-/- and 3 DKO leukemias.
DKO Leukemias Have an Altered Transcriptome Implicating Monocarboxylic Acid Transport and Hedgehog Signaling
To gain further insight into the differences between E2a-/- and DKO leukemias we performed RNA-sequencing on multiple E2a-/- and DKO leukemia lines. There was a large amount of variation in the gene programs of these leukemias but they were resolved into distinct populations by principle component analysis through PC1 and PC3 (Table S3 and Figure 7A). We identified 89 genes that were generally decreased in DKO as compared to the E2a-/- lines and 70 genes that were increased (Log2FC, adj. p-value <0.01) (Figure 7B). Tcf7 mRNA appeared to be increased in DKO leukemias but TCF1 protein, unlike LEF1 protein, was expressed similarly in E2a-/- and DKO lines when evaluated by flow cytometry (Figure S1). Analysis of the differentially expressed genes by Metascape revealed that the genes that decreased in the DKO leukemias were enriched for genes involved in IL-4 production whereas those that increased were enriched for genes in the monocarboxylic acid transport (MCT) and the Hedgehog signaling pathways (Figures 7C, D). Genes in the MCT pathway included Fabp5, Pla2g12a, Syk and Hoxa13 (Figures 7B, E). Genes in the Hedgehog signaling pathway included Axin2, Cdkn1a, Prkch and Rasgrp1 (Figures 7B, F). Taken together, these data indicate that DKO and E2a-/- leukemia lines share many common gene expression features but differ in a few key genes that could impact their metabolic requirements or response to external signals.
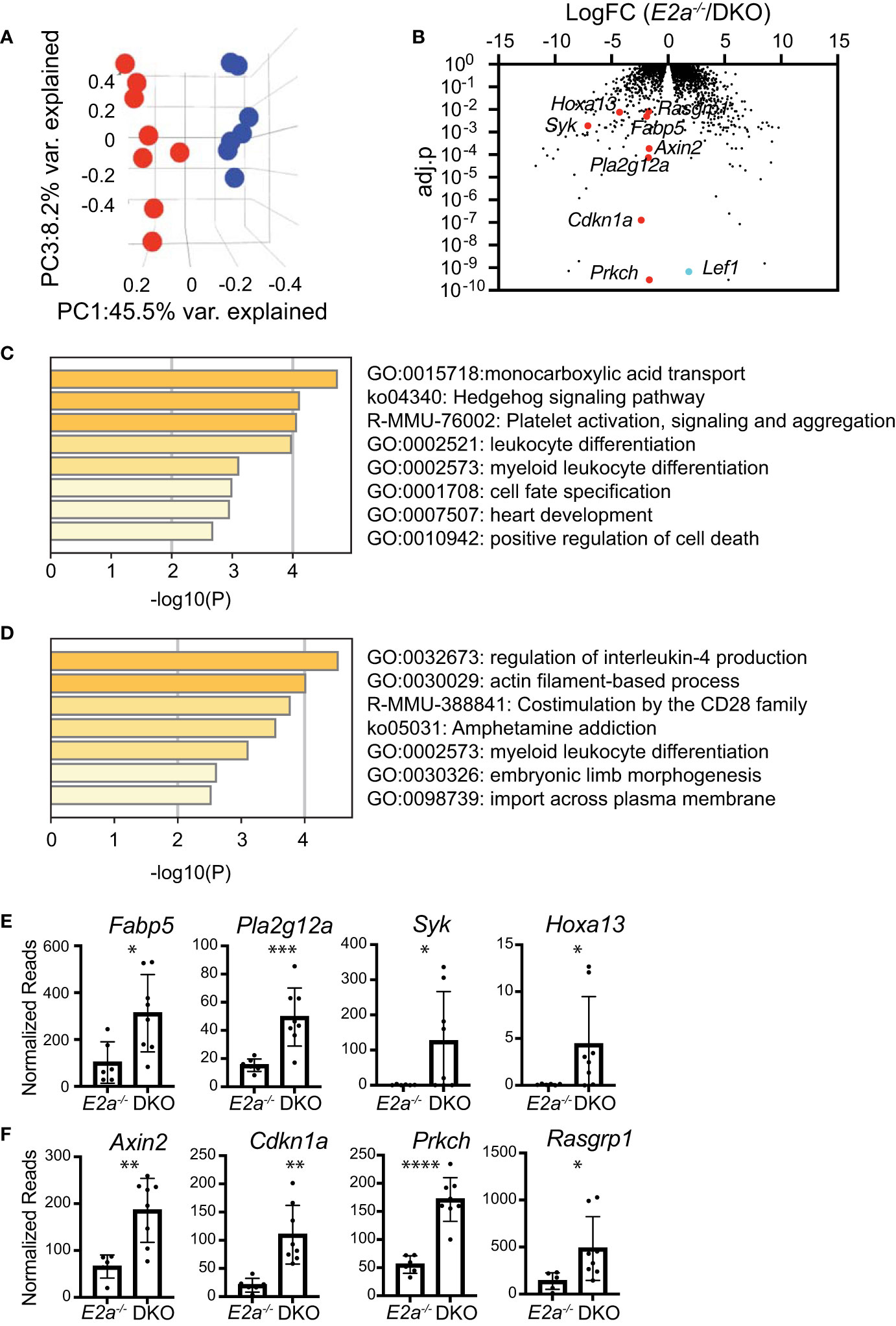
Figure 7 RNA-sequencing of E2a-/- and DKO leukemia lines reveals differences in multiple genes associated with signaling and monocarboxylic acid transport. (A) Principal Component Analysis of E2a-/- (blue) and DKO (red) leukemias based on differential gene expression in RNA-seq analysis. adj.p based on FDR. (B) Volcano plot showing Log2FC by adj.p-value comparing RNA-seq from E2a-/- and DKO leukemias. Lef1 and genes on interest that were higher in DKO as compared to E2a-/- leukemias are indicated. (C) Pathway analysis for genes that are increased in DKO compared to E2a-/- leukemias. (D) Pathway analysis of genes that are decreased in DKO compared to E2a-/- leukemias. (E) Normalized reads for individual E2a-/- and DKO leukemia samples for selected genes in the monocarboxylic acid transport pathway or (F) the Hedgehog Signaling Pathway. *p < 0.05, **p < 0.01, ***p < 0.005, and ****p < 0.0001 by student t-test of individual normalized reads.
Discussion
Over the past 10 years, analysis of gene expression and mutations in T-ALL have revealed both positive and negative associations with LEF1 (34, 36, 37). Here, we investigated the role of LEF1 in T cell transformation in a murine model of T-ALL. We used conditional alleles of Lef1 in E2a-/- T lymphocytes, that were deleted either before or after leukemic transformation and found that LEF1 can function as either an oncogene or a tumor suppressor depending on the context in which it is deleted. When LEF1 is present during the transformation process, leukemias can become addicted to its presence. In contrast, when Lef1 is deleted prior to transformation, early T cell development is altered and there is a more rapid onset of leukemogenesis. We note that leukemias arising in DKO mice had some of the characteristics of human T-ALL with LEF1 inactivating mutations including mutations in the Notch1 signaling pathway and gain of chromosome 15 (37), although these characteristics are shared with E2a-/- leukemias (24, 26). However, DKO leukemias had a more a rapid onset than E2a-/- leukemias mirroring the early development of T-ALL with Lef1 inactivating mutations (37). In addition to providing insight into how LEF1 can be both a positive and negative regulator of leukemogenesis, our data indicate that the order of acquisition of specific mutations in T cell progenitors can impact the phenotype and latency of T-ALL.
The leukemic cells that arise in DKO mice have very dim expression of CD4 and CD8 and express CD25, which distinguishes them from E2a-/- leukemias. Cell lines established from DKO mice also showed some differences in gene expression from E2a-/- leukemias. In particular, genes associated with the MCT and Hedgehog signaling pathway, were elevated relative to E2a-/- leukemias. Among these genes were Rasgrp1, Pla2g12a and Syk, which are also involved in T cell receptor signaling and their increased expression may be related to the dim expression of CD4 and CD8, which canonically identifies cells undergoing strong TCR signaling or negative selection (49). The increased expression of Fabp5 also raises the possibility that DKO and E2a-/- leukemias may have different metabolic requirements. While there was quite a bit of variability in the gene programs of individual leukemia lines, these observations raise the possibility that leukemias with different mutation profiles may have unique susceptibilities that could be exploited for therapy.
Lef1 is increased in expression in multiple mouse models of T-ALL. Tcf7-/- mice also have increased Lef1 in DN thymocytes but develop an ETP-like T-ALL (32). TCF1 was shown to repress Lef1 and indeed, TCF1 binds to a cluster of sites in the Lef1 gene and represses transcription from this region in a reporter construct transduced into DN thymocytes. TCF1 is expressed in E2a-/- thymocytes at levels indistinguishable from control mice indicating that the increased LEF1 is not a consequence of TCF1-deficiency (45, 46). We showed here that unlike LEF1, TCF1 is not regulated by Notch signaling in E2a-/- leukemias and therefore we hypothesize that Notch1 regulates Lef1 directly and independent of TCF1. Deletion of Lef1 in Tcf7-/- thymocytes had surprisingly little impact on DN thymocyte development but prevented β-selection and abrogated leukemogenesis (32). In contrast, deletion of Lef1 in E2a-/- mice did not prevent leukemogenesis. Therefore, TCF1 appears sufficient in E2a-/- mice to support T cell transformation. TCF1 protein was expressed equivalently in E2a-/- and DKO leukemias suggesting that LEF1 does not promote leukemogenesis by repressing Tcf7. Given that E2a-/- leukemias are not ETP-like, we do not think that the major function of LEF1 is simply antagonism of TCF1. However, it is possible that high levels of LEF1 impact TCF1 function by competing with TCF1 for binding to TCF1/LEF1 binding sites, either promoting or inhibiting TCF1-like functions. In this scenario, LEF1 may antagonize TCF1 functions that prevent transformation while promoting, or leaving intact, TCF1 functions that support T cell differentiation. Indeed, we note that Cdkn1a is increased in both DKO leukemias and E2a-/-Lef1F/F leukemias after deletion of Lef1 compared to E2a-/- leukemias suggesting that Cdkn1a could be a TCF1 target that is repressed by LEF1. In contrast, Syk and Rasgrp1 are increased in DKO compared to E2A-/- leukemias but decreased after deletion of Lef1 from E2a-/-Lef1F/F leukemias. Thus, we could speculate that Syk and Rasgrp1 are positively regulated by LEF1 or TCF1, gaining a dependence on high levels of LEF1 in E2a-/-Lef1F/F leukemias and TCF1 in DKO leukemias. Further studies are required to understand the mechanisms by which LEF1 and TCF1 function in these different contexts.
We anticipated that LEF1 would be required for the differentiation of ETPs to the DN3 stage in the absence of E2A and our in vitro experiments supported this hypothesis. Indeed, deletion of Lef1 in E2a-/- mice resulted in a loss of DN3 thymocytes. However, total thymocyte numbers were not impacted by deletion of Lef1 suggesting that LEF1 was not required for T cell development in the absence of E2a. Given that we observed increased CD25 and Notch1 signaling in DP thymocytes after deletion of Lef1, we hypothesize that LEF1 prevented differentiation of DN3 cells into DP cells. Whether these cells are truly DP thymocytes or DN3 thymocytes that express CD4 and CD8 remains to be investigated. Thus, the altered latency of transformation in DKO mice could be related to differences in the intrinsic susceptibility of thymocytes at different stages of differentiation to transformation in the absence of E2A or to an altered environment in which the transforming progenitors reside.
Taken together, our data demonstrate that LEF1 impacts the developmental trajectory of E2a-/- T cell progenitors and can act as a tumor suppressor or oncogene depending on its availability during the transformation process. Moreover, our data support the utility of mouse models for understanding the cooperativity and consequence of mutational order on leukemogenesis.
Data Availability Statement
The datasets presented in this study can be found in online repositories. The names of the repository/repositories and accession number(s) can be found below: https://www.ncbi.nlm.nih.gov/geo/, GSE186420 and GSE196391.
Ethics Statement
The animal study was reviewed and approved by Institutional Animal Care and Use Committee, University of Chicago.
Author Contributions
TC, SM, SD, and MV designed and performed experiments and interpreted data. ML supervised the SKY analysis, HX provided the Lef1F/F mice, MS supervised the microarray experiments, EB performed bioinformatics analysis, BK designed and performed experiments, interpreted data, and wrote the manuscript. All authors contributed to the article and approved the submitted version.
Funding
This study was supported, in part, by funding from NIAID (R21 AI119894, R21 AI096530 and R01 AI079213) to BK, and (P30 CA014599) to the University of Chicago Comprehensive Cancer Center.
Conflict of Interest
BK is on the Scientific Advisory Board for Century Therapeutics.
The remaining authors declare that the research was conducted in the absence of any commercial or financial relationships that could be construed as a potential conflict of interest.
The reviewer PN declared a shared affiliation, with no collaboration, with the author MS to the handling editor at the time of review.
Publisher’s Note
All claims expressed in this article are solely those of the authors and do not necessarily represent those of their affiliated organizations, or those of the publisher, the editors and the reviewers. Any product that may be evaluated in this article, or claim that may be made by its manufacturer, is not guaranteed or endorsed by the publisher.
Acknowledgments
We thank members of the Kee Lab, Warren Pear and Adolfo Ferrando for helpful discussions. Christina Spaulding, Grant van der Voort, Simon Liang, Samantha Cuthbert, Renee de Pooter, Emma Hegermiller and Elizabeth M. Davis for technical assistance and Warren Pear for the DN-MAML and Cre producing MigR1 constructs. We are also grateful to the University of Chicago Cytometry and Antibody Technology facility (RRID: SCR_017760) and the Functional Genomics Facility (RRID: SCR_019196) and the Animal Resource Center.
Supplementary Material
The Supplementary Material for this article can be found online at: https://www.frontiersin.org/articles/10.3389/fimmu.2022.845488/full#supplementary-material
Supplementary Figure 1 | Expression of LEF1 and TCF1 in E2a-/- and DKO leukemias. Normalized Reads for Lef1 (A) and Tcf7 (B) from RNA-sequencing data using RNA isolated from E2a-/- or DKO leukemia lines. Each dot represents the normalized reads from on line. Flow cytometry for (C) LEF1 and (D) TCF1 in Ctrl thymocytes (left panels) or an E2a-/- (middle panels) or DKO (right panels) leukemia. The shaded histogram is isotype control staining. ***p < 0.005.
References
1. Belver L, Ferrando A. The Genetics and Mechanisms of T Cell Acute Lymphoblastic Leukaemia. Nat Rev Cancer (2016) 16:494–507. doi: 10.1038/nrc.2016.63
2. Bene MC, Castoldi G, Knapp W, Ludwig WD, Matutes E, Orfao A, et al. Proposals for the Immunological Classification of Acute Leukemias. European Group for the Immunological Characterization of Leukemias (EGIL). Leukemia (1995) 9:1783–6.
3. Coustan-Smith E, Mullighan CG, Onciu M, Behm FG, Raimondi SC, Pei D, et al. Early T-Cell Precursor Leukaemia: A Subtype of Very High-Risk Acute Lymphoblastic Leukaemia. Lancet Oncol (2009) 10:147–56. doi: 10.1016/S1470-2045(08)70314-0
4. Ferrando AA, Look AT. Gene Expression Profiling in T-Cell Acute Lymphoblastic Leukemia. Semin Hematol (2003) 40:274–80. doi: 10.1016/S0037-1963(03)00195-1
5. Noronha EP, Marques LVC, Andrade FG, Thuler LCS, Terra-Granado E, Pombo-de-Oliveira MS. Brazilian Collaborative Study Group of Acute. The Profile of Immunophenotype and Genotype Aberrations in Subsets of Pediatric T-Cell Acute Lymphoblastic Leukemia. Front Oncol (2019) 9:316. doi: 10.3389/fonc.2019.00316
6. Ferrando AA, Lopez-Otin C. Clonal Evolution in Leukemia. Nat Med (2017) 23:1135–45. doi: 10.1038/nm.4410
7. Weng AP, Ferrando AA, Lee W, t. Morris JP, Silverman LB, Sanchez-Irizarry C, et al. Activating Mutations of NOTCH1 in Human T Cell Acute Lymphoblastic Leukemia. Science (2004) 306:269–71. doi: 10.1126/science.1102160
8. Malyukova A, Brown S, Papa R, O’Brien R, Giles J, Trahair TN, et al. FBXW7 Regulates Glucocorticoid Response in T-Cell Acute Lymphoblastic Leukaemia by Targeting the Glucocorticoid Receptor for Degradation. Leukemia (2013) 27:1053–62. doi: 10.1038/leu.2012.361
9. Thompson BJ, Buonamici S, Sulis ML, Palomero T, Vilimas T, Basso G, et al. The SCFFBW7 Ubiquitin Ligase Complex as a Tumor Suppressor in T Cell Leukemia. J Exp Med (2007) 204:1825–35. doi: 10.1084/jem.20070872
10. King B, Trimarchi T, Reavie L, Xu L, Mullenders J, Ntziachristos P, et al. The Ubiquitin Ligase FBXW7 Modulates Leukemia-Initiating Cell Activity by Regulating MYC Stability. Cell (2013) 153:1552–66. doi: 10.1016/j.cell.2013.05.041
11. Gomez-del Arco P, Kashiwagi M, Jackson AF, Naito T, Zhang J, Liu F, et al. Alternative Promoter Usage at the Notch1 Locus Supports Ligand-Independent Signaling in T Cell Development and Leukemogenesis. Immunity (2010) 33:685–98. doi: 10.1016/j.immuni.2010.11.008
12. Ashworth TD, Pear WS, Chiang MY, Blacklow SC, Mastio J, Xu L, et al. Deletion-Based Mechanisms of Notch1 Activation in T-ALL: Key Roles for RAG Recombinase and a Conserved Internal Translational Start Site in Notch1. Blood (2010) 116:5455–64. doi: 10.1182/blood-2010-05-286328
13. Jeannet R, Mastio J, Macias-Garcia A, Oravecz A, Ashworth T, Geimer Le Lay AS, et al. Oncogenic Activation of the Notch1 Gene by Deletion of Its Promoter in Ikaros-Deficient T-ALL. Blood (2010) 116:5443–54. doi: 10.1182/blood-2010-05-286658
14. Allman D, Karnell FG, Punt JA, Bakkour S, Xu L, Myung P, et al. Separation of Notch1 Promoted Lineage Commitment and Expansion/Transformation in Developing T Cells. J Exp Med (2001) 194:99–106. doi: 10.1084/jem.194.1.99
15. de Pooter RF, Kee BL. E Proteins and the Regulation of Early Lymphocyte Development. Immunol Rev (2010) 238:93–109. doi: 10.1111/j.1600-065X.2010.00957.x
16. Anderson MK. At the Crossroads: Diverse Roles of Early Thymocyte Transcriptional Regulators. Immunol Rev (2006) 209:191–211. doi: 10.1111/j.0105-2896.2006.00352.x
17. Liau WS, Tan SH, Ngoc PCT, Wang CQ, Tergaonkar V, Feng H, et al. Aberrant Activation of the GIMAP Enhancer by Oncogenic Transcription Factors in T-Cell Acute Lymphoblastic Leukemia. Leukemia (2017) 31:1798–807. doi: 10.1038/leu.2016.392
18. Mansour MR, Sanda T, Lawton LN, Li X, Kreslavsky T, Novina CD, et al. The TAL1 Complex Targets the FBXW7 Tumor Suppressor by Activating Mir-223 in Human T Cell Acute Lymphoblastic Leukemia. J Exp Med (2013) 210:1545–57. doi: 10.1084/jem.20122516
19. Sanda T, Lawton LN, Barrasa MI, Fan ZP, Kohlhammer H, Gutierrez A, et al. Core Transcriptional Regulatory Circuit Controlled by the TAL1 Complex in Human T Cell Acute Lymphoblastic Leukemia. Cancer Cell (2012) 22:209–21. doi: 10.1016/j.ccr.2012.06.007
20. Nagel S, Ehrentraut S, Tomasch J, Lienenklaus S, Schneider B, Geffers R, et al. Transcriptional Activation of Prostate Specific Homeobox Gene NKX3-1 in Subsets of T-Cell Lymphoblastic Leukemia (T-ALL). PLoS One (2012) 7:e40747. doi: 10.1371/journal.pone.0040747
21. Kusy S, Gerby B, Goardon N, Gault N, Ferri F, Gerard D, et al. NKX3.1 is a Direct TAL1 Target Gene That Mediates Proliferation of TAL1-Expressing Human T Cell Acute Lymphoblastic Leukemia. J Exp Med (2010) 207:2141–56. doi: 10.1084/jem.20100745
22. Kim D, Peng XC, Sun XH. Massive Apoptosis of Thymocytes in T-Cell-Deficient Id1 Transgenic Mice. Mol Cell Biol (1999) 19:8240–53. doi: 10.1128/MCB.19.12.8240
23. Morrow MA, Mayer EW, Perez CA, Adlam M, Siu G. Overexpression of the Helix-Loop-Helix Protein Id2 Blocks T Cell Development at Multiple Stages. Mol Immunol (1999) 36:491–503. doi: 10.1016/S0161-5890(99)00071-1
24. Bain G, Engel I, Robanus Maandag EC, te Riele HP, Voland JR, Sharp LL, et al. E2A Deficiency Leads to Abnormalities in Alphabeta T-Cell Development and to Rapid Development of T-Cell Lymphomas. Mol Cell Biol (1997) 17:4782–91. doi: 10.1128/MCB.17.8.4782
25. Yan W, Young AZ, Soares VC, Kelley R, Benezra R, Zhuang Y. High Incidence of T-Cell Tumors in E2A-Null Mice and E2A/Id1 Double-Knockout Mice. Mol Cell Biol (1997) 17:7317–27. doi: 10.1128/MCB.17.12.7317
26. Reschly EJ, Spaulding C, Vilimas T, Graham WV, Brumbaugh RL, Aifantis I, et al. Notch1 Promotes Survival of E2A-Deficient T Cell Lymphomas Through Pre-T Cell Receptor-Dependent and -Independent Mechanisms. Blood (2006) 107:4115–21. doi: 10.1182/blood-2005-09-3551
27. Weng AP, Millholland JM, Yashiro-Ohtani Y, Arcangeli ML, Lau A, Wai C, et al. C-Myc Is an Important Direct Target of Notch1 in T-Cell Acute Lymphoblastic Leukemia/Lymphoma. Genes Dev (2006) 20:2096–109. doi: 10.1101/gad.1450406
28. Sharma VM, Calvo JA, Draheim KM, Cunningham LA, Hermance N, Beverly L, et al. Notch1 Contributes to Mouse T-Cell Leukemia by Directly Inducing the Expression of C-Myc. Mol Cell Biol (2006) 26:8022–31. doi: 10.1128/MCB.01091-06
29. Palomero T, Lim WK, Odom DT, Sulis ML, Real PJ, Margolin A, et al. NOTCH1 Directly Regulates C-MYC and Activates a Feed-Forward-Loop Transcriptional Network Promoting Leukemic Cell Growth. Proc Natl Acad Sci USA (2006) 103:18261–6. doi: 10.1073/pnas.0606108103
30. Spaulding C, Reschly EJ, Zagort DE, Yashiro-Ohtani Y, Beverly LJ, Capobianco A, et al. Notch1 Co-Opts Lymphoid Enhancer Factor 1 for Survival of Murine T-Cell Lymphomas. Blood (2007) 110:2650–8. doi: 10.1182/blood-2007-04-084202
31. Steinke FC, Xue HH. From Inception to Output, Tcf1 and Lef1 Safeguard Development of T Cells and Innate Immune Cells. Immunol Res (2014) 59:45–55. doi: 10.1007/s12026-014-8545-9
32. Yu S, Zhou X, Steinke FC, Liu C, Chen SC, Zagorodna O, et al. The TCF-1 and LEF-1 Transcription Factors Have Cooperative and Opposing Roles in T Cell Development and Malignancy. Immunity (2012) 37:813–26. doi: 10.1016/j.immuni.2012.08.009
33. Geimer Le Lay AS, Oravecz A, Mastio J, Jung C, Marchal P, Ebel C, et al. The Tumor Suppressor Ikaros Shapes the Repertoire of Notch Target Genes in T Cells. Sci Signal (2014) 7:ra28. doi: 10.1126/scisignal.2004545
34. Jia M, Zhao HZ, Shen HP, Cheng YP, Luo ZB, Li SS, et al. Overexpression of Lymphoid Enhancer-Binding Factor-1 (LEF1) Is a Novel Favorable Prognostic Factor in Childhood Acute Lymphoblastic Leukemia. Int J Lab Hematol (2015) 37:631–40. doi: 10.1111/ijlh.12375
35. Erbilgin Y, Hatirnaz Ng O, Can I, Firtina S, Kucukcankurt F, Karaman S, et al. Prognostic Evidence of LEF1 Isoforms in Childhood Acute Lymphoblastic Leukemia. Int J Lab Hematol (2021) 43(5):1093–103. doi: 10.1111/ijlh.13513
36. Guo X, Zhang R, Liu J, Li M, Song C, Dovat S, et al. Characterization of LEF1 High Expression and Novel Mutations in Adult Acute Lymphoblastic Leukemia. PLoS One (2015) 10:e0125429. doi: 10.1371/journal.pone.0125429
37. Gutierrez A, Sanda T, Ma W, Zhang J, Grebliunaite R, Dahlberg S, et al. Inactivation of LEF1 in T-Cell Acute Lymphoblastic Leukemia. Blood (2010) 115:2845–51. doi: 10.1182/blood-2009-07-234377
38. Carr T, Krishnamoorthy V, Yu S, Xue HH, Kee BL, Verykokakis M. The Transcription Factor Lymphoid Enhancer Factor 1 Controls Invariant Natural Killer T Cell Expansion and Th2-Type Effector Differentiation. J Exp Med (2015) 212:793–807. doi: 10.1084/jem.20141849
39. Maillard I, Weng AP, Carpenter AC, Rodriguez CG, Sai H, Xu L, et al. Mastermind Critically Regulates Notch-Mediated Lymphoid Cell Fate Decisions. Blood (2004) 104:1696–702. doi: 10.1182/blood-2004-02-0514
40. Kee BL. Id3 Induces Growth Arrest and Caspase-2-Dependent Apoptosis in B Lymphocyte Progenitors. J Immunol (2005) 175:4518–27. doi: 10.4049/jimmunol.175.7.4518
41. Dias S, Mansson R, Gurbuxani S, Sigvardsson M, Kee BL. E2A Proteins Promote Development of Lymphoid-Primed Multipotent Progenitors. Immunity (2008) 29:217–27. doi: 10.1016/j.immuni.2008.05.015
42. Jacobsen JA, Woodard J, Mandal M, Clark MR, Bartom ET, Sigvardsson M, et al. EZH2 Regulates the Developmental Timing of Effectors of the Pre-Antigen Receptor Checkpoints. J Immunol (2017) 198:4682–91. doi: 10.4049/jimmunol.1700319
43. Zhou Y, Zhou B, Pache L, Chang M, Khodabakhshi AH, Tanaseichuk O, et al. Metascape Provides a Biologist-Oriented Resource for the Analysis of Systems-Level Datasets. Nat Commun (2019) 10:1523. doi: 10.1038/s41467-019-09234-6
44. Le Beau MM, Espinosa R 3rd, Davis EM, Eisenbart JD, Larson RA. And XXXE. D. Green. Cytogenetic and Molecular Delineaton of a Region of Chroosome 7 Commonly Deleted in Malignant Myeloid Diseases. Blood (1996) 88:1930–5.
45. Xu W, Carr T, Ramirez K, McGregor S, Sigvardsson M, Kee BL. E2A Transcription Factors Limit Expression of Gata3 to Facilitate T Lymphocyte Lineage Commitment. Blood (2013) 121:1534–42. doi: 10.1182/blood-2012-08-449447
46. Miyazaki M, Miyazaki K, Chen K, Jin Y, Turner J, Moore AJ, et al. The E-Id Protein Axis Specifies Adaptive Lymphoid Cell Identity and Suppresses Thymic Innate Lymphoid Cell Development. Immunity (2017) 46:818–34.e814. doi: 10.1016/j.immuni.2017.04.022
47. Ikawa T, Kawamoto H, Goldrath AW, Murre C. E Proteins and Notch Signaling Cooperate to Promote T Cell Lineage Specification and Commitment. J Exp Med (2006) 203:1329–42. doi: 10.1084/jem.20060268
48. Gothert JR, Brake RL, Smeets M, Duhrsen U, Begley CG, Izon DJ. NOTCH1 Pathway Activation Is an Early Hallmark of SCL T Leukemogenesis. Blood (2007) 110:3753–62. doi: 10.1182/blood-2006-12-063644
Keywords: E2a, Lef1, leukemia, thymus, lymphocyte
Citation: Carr T, McGregor S, Dias S, Verykokakis M, Le Beau MM, Xue H-H, Sigvardsson M, Bartom ET and Kee BL (2022) Oncogenic and Tumor Suppressor Functions for Lymphoid Enhancer Factor 1 in E2a-/- T Acute Lymphoblastic Leukemia. Front. Immunol. 13:845488. doi: 10.3389/fimmu.2022.845488
Received: 29 December 2021; Accepted: 23 February 2022;
Published: 18 March 2022.
Edited by:
Takashi MaruYama, National Institutes of Health (NIH), United StatesCopyright © 2022 Carr, McGregor, Dias, Verykokakis, Le Beau, Xue, Sigvardsson, Bartom and Kee. This is an open-access article distributed under the terms of the Creative Commons Attribution License (CC BY). The use, distribution or reproduction in other forums is permitted, provided the original author(s) and the copyright owner(s) are credited and that the original publication in this journal is cited, in accordance with accepted academic practice. No use, distribution or reproduction is permitted which does not comply with these terms.
*Correspondence: Barbara L. Kee, YmtlZUBic2QudWNoaWNhZ28uZWR1
†Present address: Stephanie McGregor, Dept. of Pathology and Laboratory Medicine, University of Wisconsin, Madison, WI, United States
Mihalis Verykokakis, Biomedical Sciences Research Center (BSRC) “Aexander Fleming”, Vari, Greece