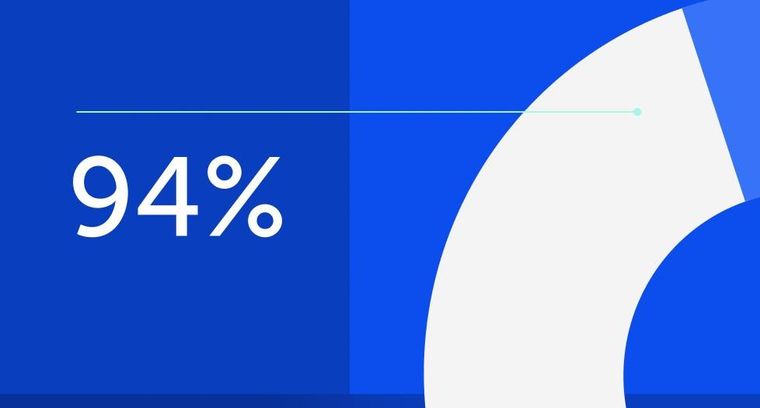
94% of researchers rate our articles as excellent or good
Learn more about the work of our research integrity team to safeguard the quality of each article we publish.
Find out more
REVIEW article
Front. Immunol., 25 February 2022
Sec. Inflammation
Volume 13 - 2022 | https://doi.org/10.3389/fimmu.2022.842858
This article is part of the Research TopicThe Immunological Consequences of Regulated Cell Death in Infection and InflammationView all 12 articles
Iron metabolism is vital for the survival of both humans and microorganisms. Heme oxygenase-1 (HO-1) is an essential stress-response enzyme highly expressed in the lungs, and catabolizes heme into ferrous iron, carbon monoxide (CO), and biliverdin (BV)/bilirubin (BR), especially in pathological conditions which cause oxidative stress and inflammation. Ferrous iron (Fe2+) is an important raw material for the synthesis of hemoglobin in red blood cells, and patients with iron deficiency are often associated with decreased cellular immunity. CO and BR can inhibit oxidative stress and inflammation. Thus, HO-1 is regarded as a cytoprotective molecule during the infection process. However, recent study has unveiled new information regarding HO-1. Being a highly infectious pathogenic bacterium, Mycobacterium tuberculosis (MTB) infection causes acute oxidative stress, and increases the expression of HO-1, which may in turn facilitate MTB survival and growth due to increased iron availability. Moreover, in severe cases of MTB infection, excessive reactive oxygen species (ROS) and free iron (Fe2+) due to high levels of HO-1 can lead to lipid peroxidation and ferroptosis, which may promote further MTB dissemination from cells undergoing ferroptosis. Therefore, it is important to understand and illustrate the dual role of HO-1 in tuberculosis. Herein, we critically review the interplay among HO-1, tuberculosis, and the host, thus paving the way for development of potential strategies for modulating HO-1 and iron metabolism.
Tuberculosis (TB), a highly infectious disease caused by Mycobacterium tuberculosis (MTB), is spread from person to person predominantly through air by droplet contact (1). After inhalation, the bacteria are phagocytosed by alveolar macrophages (2). During their contact with the invading pathogens, macrophages are activated to produce cytokines and high levels of reactive oxygen species (ROS) (3). These inflammatory responses are a major defense mechanism against invading pathogens; however, an excessive inflammatory response can result in accumulation of ROS and extensive tissue and organ damage (4). An imbalance in oxidant/antioxidant levels may lead to oxidative stress, which is likely to be a major mechanism causing cell and tissue injury, and even massive blood loss in TB patients (3). As a result, iron deficiency anemia is commonly observed among TB patients, which, in turn, is associated with decreased cellular immunity. It has been reported that tissue damage is associated with high levels of oxidation in tuberculosis patients (3, 5). ROS are highly reactive major cellular oxidants generated as byproducts of oxygen metabolism, and the accumulation of the ROS within cells may initiate cell necrosis (6, 7). Ferroptosis is a recently identified form of programmed cell death, differs from other cell death mechanisms, and results in an iron-dependent intracellular accumulation of ROS and lipid peroxides (8, 9). Amaral et al., reported in a recent study that excessive iron accumulation in MTB-infected cells can induce necrotic cell death via ferroptosis, which promotes tissue damage and facilitates bacterial dissemination (10).
Heme oxygenase-1 (HO-1) is a major antioxidant which is highly expressed in the lungs, and is activated by a variety of stress signals such as ROS and inflammatory mediators (11, 12). As a critical cytoprotective enzyme, HO-1 degrades free heme into free iron, carbon monoxide (CO), and biliverdin (BV), which, in turn, may be further converted to bilirubin (BR) (13). CO is known to possess anti-inflammatory, anti-oxidative, and anti-apoptotic effects (14). Otterbein et al., have demonstrated that CO exerts anti-inflammatory effects, in part, by increasing macrophage IL-10 production (15). Moreover, overexpression of exogenous HO-1 in the macrophages ensures a high level of endogenous IL-10 production by these cells (16) (Figure 1). BV, together with bilirubin, plays a protective role through their anti-inflammatory and antioxidant activities (17, 18). The precise role of HO-1 in the host response to MTB infection and disease progression is controversial. On the one hand, some studies suggest that HO-1 induction improves disease status in TB patients (3, 19, 20). The importance of HO-1 has been demonstrated in HO-1 knockout mice and human genetic HO-1 deficiency cases (21–25). Pro-inflammatory cytokines (IL-1β, IL-6, and TNF-α) secreted by macrophages in knockout mice are significantly increased, and these mice go on to develop a progressive inflammatory state (23). Previous studies have shown that proinflammatory cytokines such as IL-1β, IL-6, and TNF-α are key intermediaries of an over-responsive host-response reaction (26–28). IL-10 is a pleiotropic cytokine recognized for its inhibitory activity on a variety of immune functions (29). IL-10 exerts anti-inflammatory effects on macrophages and dendritic cells by suppressing production of inflammatory cytokines such as IL-1β, IL-6, and TNF-α (30) (Figure 1). Moreover, other studies have observed cytoprotective properties of HO-1, suggesting that modulation of HO-1 expression and activity could have potential therapeutic value (24, 25). On the other hand, several recent studies suggest that HO-1 might increase ferrous iron levels, which is associated with ferroptosis, and facilitate MTB survival and growth under specified disease conditions (31–33). In this review, we present information regarding recent advances with respect to HO-1, and critically discuss the role of HO-1 in human tuberculosis infection, paving the way for development of potential strategies to modulate HO-1 and iron metabolism.
Figure 1 Potential mechanisms of the dual role of HO-1 in TB. It is generally assumed that this HO-1 and its products may act as a crucial regulator in inflammatory processes, and regulates the balance between proinflammatory and anti-inflammatory mediators. IL-10 is responsible for its anti-inflammatory properties; IL-1β, IL-6, and TNF-α are pro-inflammatory cytokines secreted by macrophages to initiate and regulate the progression of inflammation. MTB, Mycobacterium tuberculosis; HO-1, heme oxygenase-1; ROS, reactive oxygen species; BV, biliverdin; BR, bilirubin; FtH, ferritin heavy chain; CO, carbon monoxide; Fe, free iron.
The HO protein family is an endoplasmic reticulum-associated enzyme group that is involved in the selective catabolism of free circulating heme. Three isoforms of HO are found in mammalian cells: an inductive form, HO-1, and two constitutive forms, HO-2 and HO-3 (13, 34, 35). The two main isoforms, HO-1 and HO-2, are products of two different genes, HMOX1 and HMOX2, which are located on chromosome 22 and chromosome 16, respectively (36). HO-3 is considered to be a pseudogene derived from HO-2 transcripts, and its function remains unclear (34). HO-1 and HO-2 differ in structure and tissue distribution (36). The enzyme HO-2 is a 36 kilodalton (kDa) protein that is highly detected in the brain, testis, and endothelial and smooth muscle cells in cerebral blood vessels (37). HO-1, also known as heat shock protein 32 (HSP32), is a 32kDa protein expressed in all mammalian tissues at basal levels, and is highly expressed in the lung, spleen, liver, bone marrow, and in senescent red blood cells (38).
The promoter region of the HMOX1 gene contains an antioxidant response element (ARE), which is important for the expression of HO-1, and consists of the BTB and CNC Homology 1 (Bach1) transcription factor, together with one of 3 small musculoaponeurotic fibrosarcoma oncogene homolog (Maf) proteins (MafF, MafG or MafK). Under normal conditions, BACH1 forms heterodimers with Maf, which binds Maf recognition elements (MAREs) within the promoter region of target genes to repress transcription (39). Under conditions of excess intracellular heme, heme binds to the heme-binding region of BACH1, which induces the dissociation of Bach1 from a heterodimeric repressor complex. The BACH1 molecule is then translocated into the cytoplasm, where it undergoes ubiquitination, followed by proteasomal degradation (40). This event allows Maf to form a dimer with nuclear factor erythroid-related factor 2 (Nrf2) via the same ARE region, resulting in transcriptional activation (41) (Figure 2). This process can be initiated by oxidative stress, which occurs, for example, when excessive ROS is produced (42).
Figure 2 Regulation of HO-1 gene expression via the redox-dependent Keap1-Nrf2 system. HO-1 gene expression is regulated via inactivation of Bach1 and activation of Nrf2, which have counter-regulatory functions. When cellular heme levels are high, and in response to stress stimuli, Bach1 is removed from the HO-1 promoter. In addition, stress stimuli cause dissociation of Nrf2 from Keap1, which activates HO-1 gene expression after nuclear translocation via binding to HO-1 ARE. ARE, antioxidant response element; Keap1, Kelch-like ECH-associated protein 1; HMOX1, HO-1 gene; Bach1, BTB and CNC homologue 1; Nrf2, nuclear factor erythroid-related factor 2; ROS; reactive oxygen species.
Activation of Nrf2 requires dissociation from its partner, Kelch-like ECH-associated protein 1 (Keap1) (Figure 2). The Nrf2-Keap1 complex acts as a cellular sensor of xenobiotics, drugs, and radiation-induced ROS/electrophilic stress (43). Under oxidative stress, Nrf2 is released from its cytosolic inhibitor, Keap1, and translocates to the nucleus (44). It is also worth noting that the Keap1/Nrf2 module may not only be regulated by pro-oxidant stimuli. It is not clear whether Nrf2-dependent induction of HO-1 is part of a general Nrf2-regulated antioxidant protective response that includes other Keap1/Nrf2-regulated genes such as NAD(P)H: oxidoreductase or thioredoxin reductase-1 (45). Thus, the redox-dependent Keap1/Nrf2 system plays a central role in HO-1 induction in response to oxidative stress.
In general, heme iron is more bioavailable than free iron in mammals, and is the form in which iron is stored in the body (46). However, under various pathophysiological conditions, including sickle cell disease and malaria, excessive release of heme from liberated hemoglobin has pro-oxidant and cytotoxic effects (47). Malaria is a hemolytic disease characterized by high levels of free heme, and in responding to this stress, the expression of HO-1 is induced, which catabolizes excess heme into biliverdin, CO, and Fe2+ through degradation of the protoporphyrin IX ring of heme (48). Moreover, HO-1 also upregulates the iron-binding protein, ferritin, and the iron transporter, ferroportin (FPN), which exports labile iron to the extracellular milieu, resulting in reduced intracellular iron and oxidative stress (49, 50). Body iron is stored in the liver, in the form of ferritin and hemosiderin. When cellular iron exceeds requirements, the excessive iron is stored in a bioavailable form as ferritin, which protects cells from potentially toxic reactions catalyzed by iron (51). Ferritin thus has a dual function in facilitating both iron detoxification and acting as a reserve. Ferritin is a hetero-polymeric protein consisting of 24 subunits of two types, i.e., heavy (FtH, 21 kDa) chains and light (FtL, 19 kDa) chains (52). Ferritin-stored iron is believed to be utilized when cells become iron deficient; however, the precise mechanisms underlying the extraction of iron from ferritin have yet to be comprehensively elucidated (53). HO-1 has been reported to promote neutralization and detoxication of intracellular iron overload through inducing the FtH enzyme (54).
Given the essential functions of iron, and the toxicity associated with iron excess, humans have evolved specialized proteins and tightly regulated homeostatic mechanisms for the uptake, transport, storage, and export of iron, to provide adequate iron for essential biological processes, but also to limit the toxicity of iron excess (55). The major avenues for regulating systemic iron balance are in controlling dietary iron uptake, and iron release from hepatocytes and recycling macrophages. Dietary iron taken up by enterocytes can be stored largely in the form of ferritin (56). This allows iron to be stored in an inert form, and allows for a more controlled delivery of systemic iron. The majority of iron is delivered to the bone marrow for red blood cell (RBC) production, with smaller amounts being distributed to other tissues for fundamental cellular processes, and any excess iron is transported to the liver for storage (57). Iron recycling macrophages are a major storage site for iron in addition to liver hepatocytes, and provide a major source of daily iron. Systemic iron homeostasis is maintained predominantly by recycling iron from RBCs via reticuloendothelial macrophages. This specialized macrophage population phagocytoses old and damaged RBCs. RBCs are lysed, and iron is released from hemoglobin by HO-1. Iron can then be stored in ferritin and exported to the bloodstream by FPN, the only known mammalian iron exporter, which has been suggested to be a major gatekeeper by controlling iron entry into the bloodstream (58). The hormone, hepcidin, is a negative regulator of iron metabolism, and binds to FPN, inducing its internalization and degradation, and is involved in pathological regulation of iron in response to infection, inflammation, hypoxia, and anemia (59). Hepcidin controls the absorption of dietary iron as well as the distribution of iron between intracellular stores and extracellular fluids, including plasma (60). HO-1 contribution to iron homeostasis has been postulated. It has been reported that the patient with HO-1 deficiency exhibits both signs of inflammation and dysregulation of body iron homeostasis, including anemia and liver and kidney hemosiderosis, both induce hepcidin (61–63). Surprisingly, Kartikasari et al., observed low hepcidin levels in HO-1-deficient patients, suggesting increased need for iron in the bone marrow due to anemia (63). The decreased iron recycling, because of HO-1 deficiency, and subsequently, lower serum iron-transferrin levels may, in part, have contributed to the observed low hepcidin levels (63).. HO-activity was not observed to have a direct modulating effect on expression of HAMP, the gene that encodes for hepcidin (63). Charlebois et al., confirmed that hepatocellular HO-1 has no physiological effect on hepcidin regulation via the inflammatory pathway (64). The preceding findings indicate that dysregulation of iron homeostasis in HO-1 deficiency is the result of both defective iron recycling and erythroid activity-associated inhibition of hepcidin expression.
Inflammation is a primary immune system reaction, and as the first response of host defense, free radicals are produced by inflammatory cells to eliminate pathogens (65, 66). Tuberculosis, like other chronic inflammatory diseases, is characterized by production of free radicals such as ROS (67). ROS play an important role in host defenses against MTB, and are produced by macrophages (68). However, ROS accumulation is considered potentially harmful due to their contribution to oxidative stress and tissue damage, resulting in damage to host immunity, especially in people with impaired antioxidant capacity, such as patients with HIV infection (69). Free radical generation in excess of the antioxidant capacity of the host may lead to oxidative stress, which can be amplified by tissue damage and cell death (67, 70). Cell damage and death leads to increased free radical production and decreased antioxidant capacity, and eventually a vicious cycle is established (71).
The clinical manifestations of tuberculosis in humans and animals are proportional to the severity of pulmonary and extra-pulmonary inflammation (67, 72). The tuberculous lesion is primarily composed of macrophages and granulocytes during the early stages of MTB infection (67). Jack et al., showed that free oxygen radicals are released from polymorphonuclear leukocytes and macrophages during inflammation (73). A recent study by Scharn et al., demonstrated that the development of tuberculous pulmonary lesion necrosis correlates with an increase in macrophages and granulocyte influx into the lung (33). In addition to co-localization of inflammatory cells to sites of necrosis, these cells eventually release their cytoplasmic contents, thus resulting in extracellular accumulation of iron and copper, both of which catalyze the production of free radicals (74, 75).
It is also known that inflammatory mediators and immunity-related factors can directly or indirectly produce red cell hemolysis, leading to increased extracellular hemoglobin (68, 76). Excess free heme, which is released from heme proteins under oxidative stress, is highly cytotoxic via the production of free radicals (77–79). Furthermore, free heme sensitizes non-hematopoietic cells to undergo programmed cell death, particularly in the course of infection by intracellular pathogens such as Mycobacterium tuberculosis (54, 68, 80). This deleterious effect of free heme is most probably driven by Fe (81, 82); however, it is not clear whether Fe must first be released from heme or must still be contained within the protoporphyrin ring of heme for cytotoxicity to occur, or whether both of the preceding scenarios are able to induce cytotoxicity (81, 82).
It is well known that hemoptysis and pulmonary hemorrhage, which frees heme, are typical clinical features of acute human TB (80). Hence, acute elevation in plasma free heme levels may overwhelm the antioxidant function of HO-1, and contribute to the immunopathology of TB by dysregulation of oxidative, inflammatory, and iron homeostasis (3, 80). In a cross-sectional study by Madebo et al., the markers of free radical damage in the peripheral circulation of patients with active tuberculosis were found to be elevated (83). These results were further confirmed by Taha et al., as active pulmonary tuberculosis was associated with oxidative stress (84). Moreover, oxidative stress is associated with the pathogenesis of lung fibrosis and dysfunction in tuberculosis patients even following effective antimicrobial therapy (73, 83).
MTB is transmitted to human hosts via the respiratory route and persists primarily within lung alveolar macrophages as a facultative intracellular pathogen (85, 86). Within the macrophage, MTB creates the environment required to establish infection by disrupting phagolysosome maturation, which is an iron-dependent process (82, 86). Iron is an essential nutrient for humans and almost all pathogenic microorganisms (82). MTB may utilize multiple pathways to obtain host iron (87–91). It is an important survival strategy for MTB to produce a diffusion-and-capture system to capture iron, where MTB expresses siderophores known as mycobactins, which diffuse out of phagosomes, chelates iron, and then re-enters the phagosome (88). Luo et al., revealed that MTB also can acquire iron via endocytosis of transferrin (Tf), the main iron carrier in plasma, which means that the intracellular pool of host iron can be accessed by MTB (87). Moreover, MTB can manipulate host cell iron homeostasis to support its growth by attenuating expression of the iron export protein FPN to increase intracellular iron content (87).Recent evidence has revealed that MTB employs the heme/hemoglobin uptake system of the host macrophage to obtain host iron in early endosomal phagosomes (89–91).
Considering the absolute requirement for iron by almost all human pathogens, nutritional immunity aims to limit iron availability to invading microorganisms, and plays an important role in the innate immune system (81, 92). To limit MTB iron acquisition, pro-inflammatory cytokines, such as IFN-gamma, downregulate transferrin receptors of macrophages, which decrease iron availability in late endosomes (93). Additionally, macrophages synthesize hepcidin in response to infectious agents, allowing for modulation of iron availability at the infectious focus (59, 94, 95). For intracellular agents, such as MTB, the hepcidin-ferroportin axis may play an important role to promote outflow of iron from the infected cell and deprive MTB of the iron required for it to divide and multiply (96).
Major hepcidin regulators include iron, erythropoietic drive, and inflammation (55). In particular, iron loading stimulates hepcidin expression, which can sequester iron from pathogenic microorganisms. Conversely, iron deficiency states such as anemias, inhibit hepcidin as a feedback mechanism to maintain normal body iron levels; however, this also leads to hypoferremia and iron restricted erythropoiesis in chronic inflammatory diseases (42). These results were observed by Chinta et al., where pulmonary hemorrhage and excessive iron deposition were observed in pulmonary TB patients (3).
HO-1, as a major antioxidant which is highly expressed in lung tissue, plays a controversial role in human tuberculosis (3, 97). Previous studies have shown that the induction of HO-1 exerts protective effects in cells (48, 97, 98). Conversely, Costa et al., reported that pharmacological inhibition of HO-1 contributes to a substantial reduction of MTB burden, implying that HO-1 plays a pathogenic role rather than a protective in TB (32).
HO-1 is commonly regarded as a cytoprotective molecule during the infection process. HO-1 levels have been shown to distinguish active TB from latent TB and successfully-treated TB patients, and the increased HO-1 levels observed in plasma derives from injured tissues, and is strongly associated with bacterial burden (99, 100). HO-1 levels of latent MTB carriers were found to be comparable to healthy people, while high levels of serum HO-1 were found in patients with active tuberculosis, and HO-1 levels returned to baseline following successful treatment (97, 99). Andrade et al., showed that HO-1 level is a biomarker of active disease, and can be used to monitor the clinical condition of TB patients after receiving chemotherapy (99). Additionally, HO-1 levels positively correlate with plasma IL-10 levels and negatively correlate with TNF-alpha levels, suggesting that increased plasma levels of HO-1 may be involved in the regulation of inflammatory responses in active pulmonary tuberculosis (97, 101).
HO-1 plays a critical protective role in oxidant-induced acute lung injury (ALI), which is known to be a potent pro-oxidant and pro-inflammatory state (102). Studies have shown that oxidative stress biomarkers such as total oxidative status, malondialdehyde, and lipid peroxidation increase in pulmonary tuberculosis patients (3, 67). HO-1 catalyzes the oxidation of heme to generate iron, CO, and biliverdin, which have important anti-oxidant, anti-inflammatory, and anti-apoptotic properties (13). In addition, an increase in the intracellular free heme concentration due to massive hemorrhage is observed during active TB, and the cytoprotective role of HO-1 induction can be explained by the removal of pro-oxidant free heme in human TB patients (3, 97). Recent studies have revealed that decreased HO-1 levels positively correlate with significantly elevated ROS/RNS in neutrophils and macrophages isolated from severely damaged regions compared to healthy regions, strongly suggesting a cytoprotective role for HO-1 (3). Furthermore, HO-1 deficient mice are more susceptible to MTB infection, have increased bacterial loads, and higher mortality rates, whereas up-regulation of HO-1 has been shown to offer protection against infection, indicating the critical importance of HO-1 in controlling infection by MTB (20–23).
However, several groups have reported that treatment with SnPPIX (an HO-1 enzyme inhibitor) enhanced control of bacterial replication in vitro and decreased production of the anti-inflammatory cytokine, IL-10 (31–33). Additionally, they believe that HO-1 expression increases iron availability in activated macrophages, which is beneficial for growth of MTB (32, 103). Heme can be utilized as an abundant source of iron for replication by intracellular pathogens, directly enhancing their survival and growth inside host cells, including phagocytes (90). Although HO-1 inhibition has resulted in decreased bacterial burdens, no increase in survival has been observed (32). With respect to the preceding study, the period allocated for follow-up post-infection bacterial counts was relatively short, and it may require a longer period of follow-up to accurately determine the full effect of treatment, since TB is a chronic disease. Another limitation of the Costa et al., study is a lack of corresponding pathological data that could be utilized to relate the MTB bacterial load results to specific alterations in patient pathological states.
The effects of HO-1 are dependent on cellular iron homoeostasis (Figure 1). Ferrous iron, a product of HO-1-mediated heme degradation, is generally considered to be a pro-inflammatory agent, and an essential nutrient for MTB (104). The increased ferrous iron is diverted from the pro-oxidant Fenton reaction because it is sequestered by cellular ferritin, which is co-induced with HO-1 during the early stages of the disease (54). Recently, Reddy et al., observed that hemosiderin deposits are seen in granulomatous lesions via iron van Gieson staining in human tuberculous lungs, suggesting that there may be little free iron available for MTB growth in tuberculous lungs (80). Thus, when the human host is able to establish an iron-deprived environment for MTB at the early stage of infection, HO-1 induction occurs as a protective factor against oxidant-mediated cellular injury (Figures 1, 3).
Figure 3 The role of HO-1 in MTB infection. Free heme is highly cytotoxic via the production of free radicals. Accordingly, when HO-1 is activated moderately, HO-1 exerts a cytoprotective effect by neutralizing ROS. Conversely, the large amounts of excessive free heme released during the late stages of tuberculosis overwhelms the cytoprotective effects of HO-1, thereby contributing to oxidative stress and excessive ROS production. The over-activation of HO-1 becomes detrimental due to the excessive labile Fe2+, along with ROS overload, leading to oxidative-cell death (ferroptosis), which contributes to the spread of infection. MTB, Mycobacterium tuberculosis; HO-1, heme oxygenase-1; ROS, reactive oxygen species; Fe, free iron.
Although there is no direct evidence to support clear causality, the distribution of iron within human lung tissue shows that iron homeostasis of distinct microanatomic locations is severely disrupted by MTB infection, and this may contribute to lung immunopathology, suggesting that the ability of the host cell to maintain intracellular iron homeostasis is disrupted (3, 75). The large amounts of hemoglobin and heme released during the late stages of tuberculosis, and the presence of excessive free heme, overwhelms the cytoprotective effects of HO-1, thereby contributing to oxidative stress and excessive ROS production (3, 80). Moreover, over-activation of HO-1 increases labile Fe2+, and the binding capacity of ferritin is disturbed by iron-catalyzed oxidative stress, causing an uncontrolled release of iron, finally resulting in enhancement of lipid peroxidation and cell death, which accelerates the spread of infection. Iron produced by the decomposition of heme by HO-1 further promotes pro-oxidizer-mediated inflammation, which leads to a vicious circle of HO-1 over activation resulting in labile Fe2+, which, in turn, results in excessive ROS, leading to oxidative cell death and a proinflammatory response. All components of this vicious circle thus exacerbates the progression of immunopathology and disease in chronic tuberculosis (75, 105) (Figure 1).
It is a well-established fact that oxidative stress is a major inducer of HO-1 expression and is closely linked to inflammation (61). However, other stressful conditions also been reported to trigger HMOX1 transcription, such as pathogen-associated molecular patterns (PAMPs), danger-associated molecular patterns (DAMPs), and several different upstream signaling molecules and transcription factors (106, 107).
Previous reports have indicated that mitogen-activated protein kinases (MAPKs) are commonly activated in response to the stimuli referred to above (61, 106, 107), and play a critical role in HO-1 up-regulation (106–108). Three major subfamilies of MAPK, including p38 MAP kinase, the extracellular regulated kinases (ERK), and c-Jun N-terminal protein kinase (JNK), have been regarded as important pathways for the induction of HO-1 gene expression (108). PI3K signaling is known to regulate cellular proliferation and growth, and play a critical role in triggering inflammatory reactions through the activation of the downstream protein kinase, Akt (109). Zhuang et al., have reported that HO-1 expression may be up-regulated by rhein to protect IEC-6 cells against oxidative damage, via the PI3K/Akt pathway (110). In addition, Chen et al., found that PI3K/Akt activation not only up-regulates HO-1 gene expression, but also that the protective effects of this pathway might be linked to the beneficial effects of HO-1 (111). Previous reports have shown that other signaling pathways, such as the protein kinase A (PKA) pathway and the protein kinase C (PKC) pathway, have been associated with HO-1 up-regulation (112). It is important to point out that, in the regulation of HO-1, these signaling cascades are probably dependent on the specific cell types and specific inducers.
Several redox-sensitive transcription factors can be indirectly modulated by the various proteins mentioned above, such as MAPKs (61), PI3K (109), and other protein kinases (108), under a wide range of stressful conditions, leading to HO-1 regulation. As mentioned previously (41) the Nrf2 signaling pathway can regulate HO-1 gene transcription by targeting AREs. Nrf2/HO-1 activation induced by 4-Ketopinoresinol may be suppressed by inhibition of the PI3K-Akt pathway using chemical inhibitors or RNA interference, suggesting that the cytoprotective effect against oxidative damage may be eliminated (111). Other transcription factors, such as heat-shock factors (HSFs), activator protein-1 (AP-1), nuclear factor-kappa B (NF-kB), and hypoxia-induced factor 1 alpha (HIF1α) have also been reported to regulate HO-1 expression (108, 113).
Anti-tuberculosis drug-induced hepatotoxicity is one of the leading adverse drug reactions during the course of tuberculosis treatment, and poses a considerable challenge to clinicians and researchers (114). Previous studies suggest that anti-TB drugs may cause hepatotoxicity by generating toxic intermediaries via drug metabolism reactions (115). These reactive metabolites could induce the production of excessive reactive oxygen species (ROS), leading to lipid peroxidation and cell death (115). Otterbein et al., have demonstrated that co-administration of Isoniazid (INH) and rifampin (RIF) to HepG2 cells could induce expression of the cytoplasmic HO-1 protein during the early hours of drug treatment, with minimum loss of cell viability and cell death, and induction of HO-1 by hemin chloride could reverse the drug induced liver injury (116). This could be a potential target for a future therapeutic option to counteract INH-RIF drug-induced liver injury. Moreover, Lee et al., reported that rifampicin effectively protects the liver against cellular oxidative stress by activating AMPKα-induced activation of the Nrf2 transcription factor, with subsequent upregulation of HO-1 (117).
Several plant-based extracts and drugs have been reported to induce HO-1 expression in a variety of cells (118). The Sagittaria sagittifolia L-polysaccharide (SSP) is a purified form of a homogeneous polysaccharide isolated from the root tubers of S. sagittifolia. Wang et al., showed that, in mice, SSP significantly alleviates the hepatotoxicity induced by co-administration of INH and RIF, which is mainly attributable to an increase in expression of Nrf2 and its downstream enzyme HO-1, induced by SSP (119). Curcumin, a polyphenolic compound isolated from turmeric, has also been found to exert significant anti-inflammatory activity as a non-cytotoxic HO-1 inducer in vascular smooth muscle cells (VSMCs), endothelial cells, astrocytes, and macrophages (120, 121). Kundu et al., studied the effect of the coumarin derivative, fraxetin, on HO-1 expression in HaCaT cells, and found that fraxetin induced HO-1 expression through activation of the Akt/Nrf2 or the AMPK/Nrf2 pathways (122). Martin et al., has demonstrated the antioxidant effect of the herb-derived phenol, carnosol, which induced HO-1 expression at both mRNA and protein levels. Moreover, carnosol increased the nuclear levels of Nrf2, a transcription factor regulating AREs (123). Several HO-1-inducing compounds derived from natural sources have been also been reported to treat inflammatory conditions, including quercetin (124), resveratrol (125), anthocyanins (126), celastrol (127), and sulforaphane (128). Moreover, older repurposed drugs with novel uses have been reported. Dunigan-Russell et al., found that auranofin, an FDA-approved drug for rheumatoid arthritis, which was used to inhibit thioredoxin reductase-1 (TrxR1), was observed to activate Nrf2 responses to augment gene expression of HO-1, and thus prevent lung injury in acute respiratory distress syndrome (ARDS) (129). Phorbol myristate acetate (PMA), a strong inducer of NF-kB activity, has been reported by Naidu et al., to induce expression of HO-1 in murine primary astrocytes (130). Thus, using active components from medicinal plants and the use of repurposed old drugs have been shown to induce the expression of HO-1, and could possibly lead to new therapeutic strategies for oxidative stress-related diseases (61, 106). Over the past decade, the beneficial effects of HO-1 induction have been extensively studied and reported, as shown in Table 1.
Table 1 Natural source, evaluated model of diseases, reported effect, and pathway of natural compounds and drugs.
Current anti-TB antibiotic therapy mainly targets actively replicating bacteria, and accelerates bacterial clearance; however, they have little effect on improving disease pathology. In addition, poor patient compliance caused by the prolonged treatment duration can lead to treatment failure, and induce the emergence of multi-drug and drug resistant Mycobacterium tuberculosis strains (132). Therefore, the identification and study of effective pharmacological targets to improve the pathology related to tuberculosis can be an important and beneficial adjunct to the limited pharmacological treatment strategies for TB that are currently available. As a component of the nutrition of the human body and the nutritional immunity of host, iron homeostasis of host cells can withhold iron from Mycobacterium tuberculosis (49, 54). HO-1 plays an important role in iron homeostasis and the antioxidant system as a cytoprotective enzyme to control oxidative stress-induced cellular damage. However, the dual role of HO-1 in tuberculosis may depend on different pathological conditions (Figure 3): On the one hand, HO-1 plays a mainly cytoprotective role in the early stages of the disease, and on the other hand, HO-1 can potentially be cytotoxic due to accumulation of large amounts of heme, and the human host may have an impaired ability to maintain iron homeostasis during the later stages of Mycobacterium tuberculosis infection. HO-1 is highly inducible in response to a variety of stimuli, such as oxidative stress, hypoxia, bacterial lipopolysaccharide (LPS), cytokines, and its substrate heme. Targeted induction of this powerful enzyme may be a useful therapeutic strategy to supplement conventional pharmaceutical anti-TB therapy, and to help serve a protective function by improving oxidative stress disorders, to decrease lesional inflammatory cell infiltration, to further support anti-inflammatory activity, and to induce tissue repair at later stages of MTB infection.
SY and JO wrote the initial draft of the manuscript. YL and VH critically revised the manuscript. YC conceived and designed the study, and provided critical revision of the manuscript. YC supervised the work. All authors contributed to the article and agreed to the published version of the manuscript.
This work was supported by Chongqing Talent Cultivation Program (cstc2021ycjh-bgzxm0275), the Joint Medical Research Projects of Chongqing Municipal Health Committee and Chongqing Municipal Science and Technology Bureau (2020MSXM097, 2022QNXM032), the medical scientific research project of Chongqing Health Commission(2022WSJK006) and Scientific Research Project of Chongqing Public Health Medical Center (2022QNKYXM01).
The authors declare that the research was conducted in the absence of any commercial or financial relationships that could be construed as a potential conflict of interest.
All claims expressed in this article are solely those of the authors and do not necessarily represent those of their affiliated organizations, or those of the publisher, the editors and the reviewers. Any product that may be evaluated in this article, or claim that may be made by its manufacturer, is not guaranteed or endorsed by the publisher.
1. He W, Chen BD, Lv Y, Zhou Z, Xu JP, Lv PX, et al. Use of Low-Dose Computed Tomography to Assess Pulmonary Tuberculosis Among Healthcare Workers in a Tuberculosis Hospital. Infect Dis Poverty (2017) 6:68. doi: 10.1186/s40249-017-0274-6
2. Ryan RC, O’Sullivan MP, Keane J. Mycobacterium Tuberculosis Infection Induces Non-Apoptotic Cell Death of Human Dendritic Cells. BMC Microbiol (2011) 11:237. doi: 10.1186/1471-2180-11-237
3. Chinta KC, Rahman MA, Saini V, Glasgow JN, Reddy VP, Lever JM, et al. Microanatomic Distribution of Myeloid Heme Oxygenase-1 Protects Against Free Radical-Mediated Immunopathology in Human Tuberculosis. Cell Rep (2018) 25:1938–52.e5. doi: 10.1016/j.celrep.2018.10.073
4. Chai J, Luo L, Hou F, Fan X, Yu J, Ma W, et al. Agmatine Reduces Lipopolysaccharide-Mediated Oxidant Response via Activating PI3K/Akt Pathway and Up-Regulating Nrf2 and HO-1 Expression in Macrophages. PloS One (2016) 11:e0163634. doi: 10.1371/journal.pone.0163634
5. Teskey G, Abrahem R, Cao R, Gyurjian K, Islamoglu H, Lucero M, et al. Glutathione as a Marker for Human Disease. Adv Clin Chem (2018) 87:141–59. doi: 10.1016/bs.acc.2018.07.004
6. Scherz-Shouval R, Elazar Z. Regulation of Autophagy by ROS: Physiology and Pathology. Trends Biochem Sci (2011) 36:30–8. doi: 10.1016/j.tibs.2010.07.007
7. Le Belle JE, Orozco NM, Paucar AA, Saxe JP, Mottahedeh J, Pyle AD, et al. Proliferative Neural Stem Cells Have High Endogenous ROS Levels That Regulate Self-Renewal and Neurogenesis in a PI3K/Akt-Dependant Manner. Cell Stem Cell (2011) 8:59–71. doi: 10.1016/j.stem.2010.11.028
8. Lee J, Repasy T, Papavinasasundaram K, Sassetti C, Kornfeld H. Mycobacterium Tuberculosis Induces an Atypical Cell Death Mode to Escape From Infected Macrophages. PloS One (2011) 6:e18367. doi: 10.1371/journal.pone.0018367
9. Moraco AH, Kornfeld H. Cell Death and Autophagy in Tuberculosis. Semin Immunol (2014) 26:497–511. doi: 10.1016/j.smim.2014.10.001
10. Amaral EP, Costa DL, Namasivayam S, Riteau N, Kamenyeva O, Mittereder L, et al. A Major Role for Ferroptosis in Mycobacterium Tuberculosis-Induced Cell Death and Tissue Necrosis. J Exp Med (2019) 216:556–70. doi: 10.1084/jem.20181776
11. Kassovska-Bratinova S, Yang G, Igarashi K, Dennery PA. Bach1 Modulates Heme Oxygenase-1 Expression in the Neonatal Mouse Lung. Pediatr Res (2009) 65:145–9. doi: 10.1203/PDR.0b013e318191eedc
12. Yamada N, Yamaya M, Okinaga S, Nakayama K, Sekizawa K, Shibahara S, et al. Microsatellite Polymorphism in the Heme Oxygenase-1 Gene Promoter is Associated With Susceptibility to Emphysema. Am J Hum Genet (2000) 66:187–95. doi: 10.1086/302729
13. Wilks A. Heme Oxygenase: Evolution, Structure, and Mechanism. Antioxid Redox Signal (2002) 4:603–14. doi: 10.1089/15230860260220102
14. Ryter SW, Alam J, Choi AM. Heme Oxygenase-1/Carbon Monoxide: From Basic Science to Therapeutic Applications. Physiol Rev (2006) 86:583–650. doi: 10.1152/physrev.00011.2005
15. Otterbein LE, Bach FH, Alam J, Soares M, Tao Lu H, Wysk M, et al. Carbon Monoxide has Anti-Inflammatory Effects Involving the Mitogen-Activated Protein Kinase Pathway. Nat Med (2000) 6:422–8. doi: 10.1038/74680
16. Vergadi E, Chang MS, Lee C, Liang OD, Liu X, Fernandez-Gonzalez A, et al. Early Macrophage Recruitment and Alternative Activation are Critical for the Later Development of Hypoxia-Induced Pulmonary Hypertension. Circulation (2011) 123:1986–95. doi: 10.1161/CIRCULATIONAHA.110.978627
17. Jansen T, Daiber A. Direct Antioxidant Properties of Bilirubin and Biliverdin. Is There a Role for Biliverdin Reductase? Front Pharmacol (2012) 3:30. doi: 10.3389/fphar.2012.00030
18. Wegiel B, Otterbein LE. Go Green: The Anti-Inflammatory Effects of Biliverdin Reductase. Front Pharmacol (2012) 3:47. doi: 10.3389/fphar.2012.00047
19. Regev D, Surolia R, Karki S, Zolak J, Montes-Worboys A, Oliva O, et al. Heme Oxygenase-1 Promotes Granuloma Development and Protects Against Dissemination of Mycobacteria. Lab Invest (2012) 92:1541–52. doi: 10.1038/labinvest.2012.125
20. Silva-Gomes S, Appelberg R, Larsen R, Soares MP, Gomes MS. Heme Catabolism by Heme Oxygenase-1 Confers Host Resistance to Mycobacterium Infection. Infect Immun (2013) 81:2536–45. doi: 10.1128/IAI.00251-13
21. Kovtunovych G, Eckhaus MA, Ghosh MC, Ollivierre-Wilson H, Rouault TA. Dysfunction of the Heme Recycling System in Heme Oxygenase 1-Deficient Mice: Effects on Macrophage Viability and Tissue Iron Distribution. Blood (2010) 116:6054–62. doi: 10.1182/blood-2010-03-272138
22. Yachie A, Niida Y, Wada T, Igarashi N, Kaneda H, Toma T, et al. Oxidative Stress Causes Enhanced Endothelial Cell Injury in Human Heme Oxygenase-1 Deficiency. J Clin Invest (1999) 103:129–35. doi: 10.1172/JCI4165
23. Kapturczak MH, Wasserfall C, Brusko T, Campbell-Thompson M, Ellis TM, Atkinson MA, et al. Heme Oxygenase-1 Modulates Early Inflammatory Responses: Evidence From the Heme Oxygenase-1-Deficient Mouse. Am J Pathol (2004) 165:1045–53. doi: 10.1016/S0002-9440(10)63365-2
24. Slebos DJ, Ryter SW, Choi AM. Heme Oxygenase-1 and Carbon Monoxide in Pulmonary Medicine. Respir Res (2003) 4:7. doi: 10.1186/1465-9921-4-7
25. Constantin M, Choi AJ, Cloonan SM, Ryter SW. Therapeutic Potential of Heme Oxygenase-1/Carbon Monoxide in Lung Disease. Int J Hypertens (2012) 2012:859235. doi: 10.1155/2012/859235
26. Ghosh CC, Ramaswami S, Juvekar A, Vu HY, Galdieri L, Davidson D, et al. Gene-Specific Repression of Proinflammatory Cytokines in Stimulated Human Macrophages by Nuclear IkappaBalpha. J Immunol (2010) 185:3685–93. doi: 10.4049/jimmunol.0902230
27. Hoesel LM, Neff TA, Neff SB, Younger JG, Olle EW, Gao H, et al. Harmful and Protective Roles of Neutrophils in Sepsis. Shock (2005) 24:40–7. doi: 10.1097/01.shk.0000170353.80318.d5
28. Kasama T, Miwa Y, Isozaki T, Odai T, Adachi M, Kunkel SL. Neutrophil-Derived Cytokines: Potential Therapeutic Targets in Inflammation. Curr Drug Targets Inflammation Allergy (2005) 4:273–9. doi: 10.2174/1568010054022114
29. Endale M, Aksoylar HI, Hoebe K. Central Role of Gimap5 in Maintaining Peripheral Tolerance and T Cell Homeostasis in the Gut. Mediators Inflamm (2015) 2015:436017. doi: 10.1155/2015/436017
30. Cruz AH, Mendonca RZ, Petricevich VL. Crotalus Durissus Terrificus Venom Interferes With Morphological, Functional, and Biochemical Changes in Murine Macrophage. Mediators Inflamm (2005) 2005:349–59. doi: 10.1155/MI.2005.349
31. Abdalla MY, Ahmad IM, Switzer B, Britigan BE. Induction of Heme Oxygenase-1 Contributes to Survival of Mycobacterium Abscessus in Human Macrophages-Like THP-1 Cells. Redox Biol (2015) 4:328–39. doi: 10.1016/j.redox.2015.01.012
32. Costa DL, Namasivayam S, Amaral EP, Arora K, Chao A, Mittereder LR, et al. Sher A, Pharmacological Inhibition of Host Heme Oxygenase-1 Suppresses Mycobacterium Tuberculosis Infection In Vivo by a Mechanism Dependent on T Lymphocytes. mBio (2016) 7(5):e01675–16. doi: 10.1128/mBio.01675-16
33. Scharn CR, Collins AC, Nair VR, Stamm CE, Marciano DK, Graviss EA, et al. Heme Oxygenase-1 Regulates Inflammation and Mycobacterial Survival in Human Macrophages During Mycobacterium Tuberculosis Infection. J Immunol (2016) 196:4641–9. doi: 10.4049/jimmunol.1500434
34. Hayashi S, Omata Y, Sakamoto H, Higashimoto Y, Hara T, Sagara Y, et al. Characterization of Rat Heme Oxygenase-3 Gene. Implication of Processed Pseudogenes Derived From Heme Oxygenase-2 Gene. Gene (2004) 336:241–50. doi: 10.1016/j.gene.2004.04.002
35. McCoubrey WK Jr., Huang TJ, Maines MD. Isolation and Characterization of a cDNA From the Rat Brain That Encodes Hemoprotein Heme Oxygenase-3. Eur J Biochem (1997) 247:725–32. doi: 10.1111/j.1432-1033.1997.00725.x
36. Kutty RK, Kutty G, Rodriguez IR, Chader GJ, Wiggert B. Chromosomal Localization of the Human Heme Oxygenase Genes: Heme Oxygenase-1 (HMOX1) Maps to Chromosome 22q12 and Heme Oxygenase-2 (HMOX2) Maps to Chromosome 16p13.3. Genomics (1994) 20:513–6. doi: 10.1006/geno.1994.1213
37. Chen YH, Yet SF, Perrella MA. Role of Heme Oxygenase-1 in the Regulation of Blood Pressure and Cardiac Function. Exp Biol Med (Maywood) (2003) 228:447–53. doi: 10.1177/15353702-0322805-03
38. Gottlieb Y, Truman M, Cohen LA, Leichtmann-Bardoogo Y, Meyron-Holtz EG. Endoplasmic Reticulum Anchored Heme-Oxygenase 1 Faces the Cytosol. Haematologica (2012) 97:1489–93. doi: 10.3324/haematol.2012.063651
39. Zhang X, Guo J, Wei X, Niu C, Jia M, Li Q, et al. Bach1: Function, Regulation, and Involvement in Disease. Oxid Med Cell Longev (2018) 2018:1347969. doi: 10.1155/2018/1347969
40. Zenke-Kawasaki Y, Dohi Y, Katoh Y, Ikura T, Ikura M, Asahara T, et al. Heme Induces Ubiquitination and Degradation of the Transcription Factor Bach1. Mol Cell Biol (2007) 27:6962–71. doi: 10.1128/MCB.02415-06
41. Reichard JF, Motz GT, Puga A. Heme Oxygenase-1 Induction by NRF2 Requires Inactivation of the Transcriptional Repressor BACH1. Nucleic Acids Res (2007) 35:7074–86. doi: 10.1093/nar/gkm638
42. Kobayashi A, Kang MI, Watai Y, Tong KI, Shibata T, Uchida K, et al. Oxidative and Electrophilic Stresses Activate Nrf2 Through Inhibition of Ubiquitination Activity of Keap1. Mol Cell Biol (2006) 26:221–9. doi: 10.1128/MCB.26.1.221-229.2006
43. Niture SK, Khatri R, Jaiswal AK. Regulation of Nrf2-An Update. Free Radic Biol Med (2014) 66:36–44. doi: 10.1016/j.freeradbiomed.2013.02.008
44. Lai TH, Shieh JM, Tsou CJ, Wu WB. Gold Nanoparticles Induce Heme Oxygenase-1 Expression Through Nrf2 Activation and Bach1 Export in Human Vascular Endothelial Cells. Int J Nanomed (2015) 10:5925–39. doi: 10.2147/IJN.S88514
45. Gall T, Balla G, Balla J. Heme, Heme Oxygenase, and Endoplasmic Reticulum Stress-A New Insight Into the Pathophysiology of Vascular Diseases. Int J Mol Sci (2019) 20(15):3675. doi: 10.3390/ijms20153675
46. Monsen ER. Iron Nutrition and Absorption: Dietary Factors Which Impact Iron Bioavailability. J Am Diet Assoc (1988) 88:786–90. doi: 10.1016/S0002-8223(21)07902-5
47. Immenschuh S, Vijayan V, Janciauskiene S, Gueler F. Heme as a Target for Therapeutic Interventions. Front Pharmacol (2017) 8:146. doi: 10.3389/fphar.2017.00146
48. Gozzelino R, Jeney V, Soares MP. Mechanisms of Cell Protection by Heme Oxygenase-1. Annu Rev Pharmacol Toxicol (2010) 50:323–54. doi: 10.1146/annurev.pharmtox.010909.105600
49. Lanceta L, Li C, Choi AM, Eaton JW. Haem Oxygenase-1 Overexpression Alters Intracellular Iron Distribution. Biochem J (2013) 449:189–94. doi: 10.1042/BJ20120936
50. Paiva CN, Feijo DF, Dutra FF, Carneiro VC, Freitas GB, Alves LS, et al. Oxidative Stress Fuels Trypanosoma Cruzi Infection in Mice. J Clin Invest (2012) 122:2531–42. doi: 10.1172/JCI58525
51. Kurz T, Gustafsson B, Brunk UT. Cell Sensitivity to Oxidative Stress is Influenced by Ferritin Autophagy. Free Radic Biol Med (2011) 50:1647–58. doi: 10.1016/j.freeradbiomed.2011.03.014
52. Mehlenbacher M, Poli M, Arosio P, Santambrogio P, Levi S, Chasteen ND, et al. Iron Oxidation and Core Formation in Recombinant Heteropolymeric Human Ferritins. Biochemistry (2017) 56:3900–12. doi: 10.1021/acs.biochem.7b00024
53. Asano T, Komatsu M, Yamaguchi-Iwai Y, Ishikawa F, Mizushima N, Iwai K. Distinct Mechanisms of Ferritin Delivery to Lysosomes in Iron-Depleted and Iron-Replete Cells. Mol Cell Biol (2011) 31:2040–52. doi: 10.1128/MCB.01437-10
54. Cheng HT, Yen CJ, Chang CC, Huang KT, Chen KH, Zhang RY, et al. Ferritin Heavy Chain Mediates the Protective Effect of Heme Oxygenase-1 Against Oxidative Stress. Biochim Biophys Acta (2015) 1850:2506–17. doi: 10.1016/j.bbagen.2015.09.018
55. Dev S, Babitt JL. Overview of Iron Metabolism in Health and Disease. Hemodial Int (2017) 21 Suppl 1:S6–S20. doi: 10.1111/hdi.12542
56. Arosio P, Carmona F, Gozzelino R, Maccarinelli F, Poli M. The Importance of Eukaryotic Ferritins in Iron Handling and Cytoprotection. Biochem J (2015) 472:1–15. doi: 10.1042/BJ20150787
57. Zumerle S, Mathieu JR, Delga S, Heinis M, Viatte L, Vaulont S, et al. Targeted Disruption of Hepcidin in the Liver Recapitulates the Hemochromatotic Phenotype. Blood (2014) 123:3646–50. doi: 10.1182/blood-2014-01-550467
58. Ganz T. Systemic Iron Homeostasis. Physiol Rev (2013) 93:1721–41. doi: 10.1152/physrev.00008.2013
59. Nemeth E, Tuttle MS, Powelson J, Vaughn MB, Donovan A, Ward DM, et al. Hepcidin Regulates Cellular Iron Efflux by Binding to Ferroportin and Inducing Its Internalization. Science (2004) 306:2090–3. doi: 10.1126/science.1104742
60. Ganz T, Nemeth E. Hepcidin and Disorders of Iron Metabolism. Annu Rev Med (2011) 62:347–60. doi: 10.1146/annurev-med-050109-142444
61. Costa DL, Amaral EP, Andrade BB, Sher A. Modulation of Inflammation and Immune Responses by Heme Oxygenase-1: Implications for Infection With Intracellular Pathogens. Antioxid (Basel) (2020) 9(12):1205. doi: 10.3390/antiox9121205
62. Bednarz A, Lipinski P, Starzynski RR, Tomczyk M, Kraszewska I, Herman S, et al. Exacerbation of Neonatal Hemolysis and Impaired Renal Iron Handling in Heme Oxygenase 1-Deficient Mice. Int J Mol Sci (2020) 21(20):7754. doi: 10.3390/ijms21207754
63. Kartikasari AE, Wagener FA, Yachie A, Wiegerinck ET, Kemna EH, Swinkels DW. Hepcidin Suppression and Defective Iron Recycling Account for Dysregulation of Iron Homeostasis in Heme Oxygenase-1 Deficiency. J Cell Mol Med (2009) 13:3091–102. doi: 10.1111/j.1582-4934.2008.00494.x
64. Charlebois E, Fillebeen C, Pantopoulos K. Hepatocellular Heme Oxygenase 1 Deficiency Does Not Affect Inflammatory Hepcidin Regulation in Mice. PloS One (2019) 14:e0219835. doi: 10.1371/journal.pone.0219835
65. Pham-Huy LA, He H, Pham-Huy C. Free Radicals, Antioxidants in Disease and Health. Int J BioMed Sci (2008) 4:89–96.
66. Rahal A, Kumar A, Singh V, Yadav B, Tiwari R, Chakraborty S, et al. Oxidative Stress, Prooxidants, and Antioxidants: The Interplay. BioMed Res Int (2014) 2014:761264. doi: 10.1155/2014/761264
67. Palanisamy GS, Kirk NM, Ackart DF, Shanley CA, Orme IM, Basaraba RJ. Evidence for Oxidative Stress and Defective Antioxidant Response in Guinea Pigs With Tuberculosis. PloS One (2011) 6:e26254. doi: 10.1371/journal.pone.0026254
68. Vijayan V, Wagener F, Immenschuh S. The Macrophage Heme-Heme Oxygenase-1 System and its Role in Inflammation. Biochem Pharmacol (2018) 153:159–67. doi: 10.1016/j.bcp.2018.02.010
69. Zicari S, Sessa L, Cotugno N, Ruggiero A, Morrocchi E, Concato C, et al. Immune Activation, Inflammation, and Non-AIDS Co-Morbidities in HIV-Infected Patients Under Long-Term ART. Viruses (2019) 11(3):200. doi: 10.3390/v11030200
70. Betteridge DJ. What is Oxidative Stress? Metabolism (2000) 49:3–8. doi: 10.1016/s0026-0495(00)80077-3
71. Sies H. Oxidative Stress: A Concept in Redox Biology and Medicine. Redox Biol (2015) 4:180–3. doi: 10.1016/j.redox.2015.01.002
72. Kumar NP, Moideen K, Nancy A, Viswanathan V, Shruthi BS, Sivakumar S, et al. Plasma Chemokines Are Biomarkers of Disease Severity, Higher Bacterial Burden and Delayed Sputum Culture Conversion in Pulmonary Tuberculosis. Sci Rep (2019) 9:18217. doi: 10.1038/s41598-019-54803-w
73. Jack CI, Jackson MJ, Hind CR. Circulating Markers of Free Radical Activity in Patients With Pulmonary Tuberculosis. Tuber Lung Dis (1994) 75:132–7. doi: 10.1016/0962-8479(94)90042-6
74. Wolschendorf F, Ackart D, Shrestha TB, Hascall-Dove L, Nolan S, Lamichhane G, et al. Copper Resistance is Essential for Virulence of Mycobacterium Tuberculosis. Proc Natl Acad Sci USA (2011) 108:1621–6. doi: 10.1073/pnas.1009261108
75. Phelan JJ, Basdeo SA, Tazoll SC, McGivern S, Saborido JR, Keane J. Modulating Iron for Metabolic Support of TB Host Defense. Front Immunol (2018) 9:2296. doi: 10.3389/fimmu.2018.02296
76. Miao Z, Lu S, Du N, Guo W, Zhang J, Song Y, et al. Hypothermia Induced by Adenosine 5’-Monophosphate Attenuates Acute Lung Injury Induced by LPS in Rats. Mediators Inflamm (2012) 2012:459617. doi: 10.1155/2012/459617
77. Fortes GB, Alves LS, de Oliveira R, Dutra FF, Rodrigues D, Fernandez PL, et al. Heme Induces Programmed Necrosis on Macrophages Through Autocrine TNF and ROS Production. Blood (2012) 119:2368–75. doi: 10.1182/blood-2011-08-375303
78. Deshmukh R, Trivedi V. Phagocytic Uptake of Oxidized Heme Polymer is Highly Cytotoxic to Macrophages. PloS One (2014) 9:e103706. doi: 10.1371/journal.pone.0103706
79. Prestes EB, Alves LS, Rodrigues DAS, Dutra FF, Fernandez PL, Paiva CN, et al. Mitochondrial Reactive Oxygen Species Participate in Signaling Triggered by Heme in Macrophages and Upon Hemolysis. J Immunol (2020) 205:2795–805. doi: 10.4049/jimmunol.1900886
80. Reddy VP, Chinta KC, Saini V, Glasgow JN, Hull TD, Traylor A, et al. Ferritin H Deficiency in Myeloid Compartments Dysregulates Host Energy Metabolism and Increases Susceptibility to Mycobacterium Tuberculosis Infection. Front Immunol (2018) 9:860. doi: 10.3389/fimmu.2018.00860
81. Gozzelino R, Arosio P. Iron Homeostasis in Health and Disease. Int J Mol Sci (2016) 17(1):130. doi: 10.3390/ijms17010130
82. Cassat JE, Skaar EP. Iron in Infection and Immunity. Cell Host Microbe (2013) 13:509–19. doi: 10.1016/j.chom.2013.04.010
83. Madebo T, Lindtjorn B, Aukrust P, Berge RK. Circulating Antioxidants and Lipid Peroxidation Products in Untreated Tuberculosis Patients in Ethiopia. Am J Clin Nutr (2003) 78:117–22. doi: 10.1093/ajcn/78.1.117
84. Taha DA, Thanoon IA. Antioxidant Status, C-Reactive Protein and Iron Status in Patients With Pulmonary Tuberculosis. Sultan Qaboos Univ Med J (2010) 10:361–9.
85. Lenaerts A, Barry CE 3rd, Dartois V. Heterogeneity in Tuberculosis Pathology, Microenvironments and Therapeutic Responses. Immunol Rev (2015) 264:288–307. doi: 10.1111/imr.12252
86. Pacl HT, Reddy VP, Saini V, Chinta KC, Steyn AJC. Host-Pathogen Redox Dynamics Modulate Mycobacterium Tuberculosis Pathogenesis. Pathog Dis (2018) 76(5):fly036. doi: 10.1093/femspd/fty036
87. Banerjee S, Farhana A, Ehtesham NZ, Hasnain SE. Iron Acquisition, Assimilation and Regulation in Mycobacteria. Infect Genet Evol (2011) 11:825–38. doi: 10.1016/j.meegid.2011.02.016
88. Luo M, Fadeev EA, Groves JT. Mycobactin-Mediated Iron Acquisition Within Macrophages. Nat Chem Biol (2005) 1:149–53. doi: 10.1038/nchembio717
89. Tullius MV, Harmston CA, Owens CP, Chim N, Morse RP, McMath LM, et al. Discovery and Characterization of a Unique Mycobacterial Heme Acquisition System. Proc Natl Acad Sci USA (2011) 108:5051–6. doi: 10.1073/pnas.1009516108
90. Jones CM, Niederweis M. Mycobacterium Tuberculosis can Utilize Heme as an Iron Source. J Bacteriol (2011) 193:1767–70. doi: 10.1128/JB.01312-10
91. Wells RM, Jones CM, Xi Z, Speer A, Danilchanka O, Doornbos KS, et al. Discovery of a Siderophore Export System Essential for Virulence of Mycobacterium Tuberculosis. PloS Pathog (2013) 9:e1003120. doi: 10.1371/journal.ppat.1003120
92. Skaar EP. The Battle for Iron Between Bacterial Pathogens and Their Vertebrate Hosts. PloS Pathog (2010) 6:e1000949. doi: 10.1371/journal.ppat.1000949
93. Olakanmi O, Schlesinger LS, Ahmed A, Britigan BE. Intraphagosomal Mycobacterium Tuberculosis Acquires Iron From Both Extracellular Transferrin and Intracellular Iron Pools. Impact of Interferon-Gamma and Hemochromatosis. J Biol Chem (2002) 277:49727–34. doi: 10.1074/jbc.M209768200
94. Muckenthaler MU, Galy B, Hentze MW. Systemic Iron Homeostasis and the Iron-Responsive Element/Iron-Regulatory Protein (IRE/IRP) Regulatory Network. Annu Rev Nutr (2008) 28:197–213. doi: 10.1146/annurev.nutr.28.061807.155521
95. Peyssonnaux C, Zinkernagel AS, Datta V, Lauth X, Johnson RS, Nizet V. TLR4-Dependent Hepcidin Expression by Myeloid Cells in Response to Bacterial Pathogens. Blood (2006) 107:3727–32. doi: 10.1182/blood-2005-06-2259
96. Abreu R, Quinn F, Giri PK. Role of the Hepcidin-Ferroportin Axis in Pathogen-Mediated Intracellular Iron Sequestration in Human Phagocytic Cells. Blood Adv (2018) 2:1089–100. doi: 10.1182/bloodadvances.2017015255
97. Rockwood N, Costa DL, Amaral EP, Du Bruyn E, Kubler A, Gil-Santana L, et al. Mycobacterium Tuberculosis Induction of Heme Oxygenase-1 Expression Is Dependent on Oxidative Stress and Reflects Treatment Outcomes. Front Immunol (2017) 8:542. doi: 10.3389/fimmu.2017.00542
98. Funes SC, Rios M, Fernandez-Fierro A, Covian C, Bueno SM, Riedel CA, et al. Naturally Derived Heme-Oxygenase 1 Inducers and Their Therapeutic Application to Immune-Mediated Diseases. Front Immunol (2020) 11:1467. doi: 10.3389/fimmu.2020.01467
99. Andrade BB, Pavan Kumar N, Mayer-Barber KD, Barber DL, Sridhar R, Rekha VV, et al. Plasma Heme Oxygenase-1 Levels Distinguish Latent or Successfully Treated Human Tuberculosis From Active Disease. PloS One (2013) 8:e62618. doi: 10.1371/journal.pone.0062618
100. Andrade BB, Pavan Kumar N, Amaral EP, Riteau N, Mayer-Barber KD, Tosh KW, et al. Heme Oxygenase-1 Regulation of Matrix Metalloproteinase-1 Expression Underlies Distinct Disease Profiles in Tuberculosis. J Immunol (2015) 195:2763–73. doi: 10.4049/jimmunol.1500942
101. Lee TS, Chau LY. Heme Oxygenase-1 Mediates the Anti-Inflammatory Effect of Interleukin-10 in Mice. Nat Med (2002) 8:240–6. doi: 10.1038/nm0302-240
102. Dunigan-Russell K, Silverberg M, Lin VY, Li R, Wall SB, Li Q, et al. Club Cell Heme Oxygenase-1 Deletion: Effects in Hyperoxia-Exposed Adult Mice. Oxid Med Cell Longev (2020) 2020:2908271. doi: 10.1155/2020/2908271
103. Costa DL, Amaral EP, Namasivayam S, Mittereder LR, Fisher L, Bonfim CC, et al. Heme Oxygenase-1 Inhibition Promotes IFNgamma- and NOS2-Mediated Control of Mycobacterium Tuberculosis Infection. Mucosal Immunol (2021) 14:253–66. doi: 10.1038/s41385-020-00342-x
104. Wessling-Resnick M. Iron Homeostasis and the Inflammatory Response. Annu Rev Nutr (2010) 30:105–22. doi: 10.1146/annurev.nutr.012809.104804
105. Ganz T, Nemeth E. Hepcidin and Iron Homeostasis. Biochim Biophys Acta (2012) 1823:1434–43. doi: 10.1016/j.bbamcr.2012.01.014
106. Paine A, Eiz-Vesper B, Blasczyk R, Immenschuh S. Signaling to Heme Oxygenase-1 and its Anti-Inflammatory Therapeutic Potential. Biochem Pharmacol (2010) 80:1895–903. doi: 10.1016/j.bcp.2010.07.014
107. Hull TD, Agarwal A, George JF. The Mononuclear Phagocyte System in Homeostasis and Disease: A Role for Heme Oxygenase-1. Antioxid Redox Signal (2014) 20:1770–88. doi: 10.1089/ars.2013.5673
108. Alam J, Cook JL. How Many Transcription Factors Does it Take to Turn on the Heme Oxygenase-1 Gene? Am J Respir Cell Mol Biol (2007) 36:166–74. doi: 10.1165/rcmb.2006-0340TR
109. Patel RK, Mohan C. PI3K/AKT Signaling and Systemic Autoimmunity. Immunol Res (2005) 31:47–55. doi: 10.1385/IR:31:1:47
110. Zhuang S, Yu R, Zhong J, Liu P, Liu Z. Rhein From Rheum Rhabarbarum Inhibits Hydrogen-Peroxide-Induced Oxidative Stress in Intestinal Epithelial Cells Partly Through PI3K/Akt-Mediated Nrf2/HO-1 Pathways. J Agric Food Chem (2019) 67:2519–29. doi: 10.1021/acs.jafc.9b00037
111. Chen HH, Chen YT, Huang YW, Tsai HJ, Kuo CC. 4-Ketopinoresinol, a Novel Naturally Occurring ARE Activator, Induces the Nrf2/HO-1 Axis and Protects Against Oxidative Stress-Induced Cell Injury via Activation of PI3K/AKT Signaling. Free Radic Biol Med (2012) 52:1054–66. doi: 10.1016/j.freeradbiomed.2011.12.012
112. Kronke G, Bochkov VN, Huber J, Gruber F, Bluml S, Furnkranz A, et al. Oxidized Phospholipids Induce Expression of Human Heme Oxygenase-1 Involving Activation of cAMP-Responsive Element-Binding Protein. J Biol Chem (2003) 278:51006–14. doi: 10.1074/jbc.M304103200
113. Medina MV, Sapochnik D, Garcia Sola M, Coso O. Regulation of the Expression of Heme Oxygenase-1: Signal Transduction, Gene Promoter Activation, and Beyond. Antioxid Redox Signal (2020) 32:1033–44. doi: 10.1089/ars.2019.7991
114. Yang M, Zhang H, Tao B, Pan H, Lu L, Yi H, et al. Possible Association of HMOX1 and NQO1 Polymorphisms With Anti-Tuberculosis Drug-Induced Liver Injury: A Matched Case-Control Study. J Clin Pharm Ther (2019) 44:534–42. doi: 10.1111/jcpt.12818
115. Chen R, Wang J, Zhang Y, Tang S, Zhan S. Key Factors of Susceptibility to Anti-Tuberculosis Drug-Induced Hepatotoxicity. Arch Toxicol (2015) 89:883–97. doi: 10.1007/s00204-015-1473-1
116. Bishnu D, Santra S, Dhali GK, Chowdhury A, Santra A. Induction of Heme Oxygenase 1 Protects Hepatocytes From Isoniazid - Rifampicin Induced Cell Death: An In Vitro Study. Trop Gastroenterol (2016) 37:27–36. doi: 10.7869/tg.316
117. Lee EH, Baek SY, Park JY, Kim YW. Rifampicin Activates AMPK and Alleviates Oxidative Stress in the Liver as Mediated With Nrf2 Signaling. Chem Biol Interact (2020) 315:108889. doi: 10.1016/j.cbi.2019.108889
118. Ogborne RM, Rushworth SA, Charalambos CA, O’Connell MA. Haem Oxygenase-1: A Target for Dietary Antioxidants. Biochem Soc Trans (2004) 32:1003–5. doi: 10.1042/BST0321003
119. Wang J, Luo W, Li B, Lv J, Ke X, Ge D, et al. Sagittaria Sagittifolia Polysaccharide Protects Against Isoniazid- and Rifampicin-Induced Hepatic Injury via Activation of Nuclear Factor E2-Related Factor 2 Signaling in Mice. J Ethnopharmacol (2018) 227:237–45. doi: 10.1016/j.jep.2018.09.002
120. Mollazadeh H, Cicero AFG, Blesso CN, Pirro M, Majeed M, Sahebkar A. Immune Modulation by Curcumin: The Role of Interleukin-10. Crit Rev Food Sci Nutr (2019) 59:89–101. doi: 10.1080/10408398.2017.1358139
121. Pae HO, Kim EC, Chung HT. Integrative Survival Response Evoked by Heme Oxygenase-1 and Heme Metabolites. J Clin Biochem Nutr (2008) 42:197–203. doi: 10.3164/jcbn.2008029
122. Kundu J, Chae IG, Chun KS. Fraxetin Induces Heme Oxygenase-1 Expression by Activation of Akt/Nrf2 or AMP-Activated Protein Kinase Alpha/Nrf2 Pathway in HaCaT Cells. J Cancer Prev (2016) 21:135–43. doi: 10.15430/JCP.2016.21.3.135
123. Martin D, Rojo AI, Salinas M, Diaz R, Gallardo G, Alam J, et al. Regulation of Heme Oxygenase-1 Expression Through the Phosphatidylinositol 3-Kinase/Akt Pathway and the Nrf2 Transcription Factor in Response to the Antioxidant Phytochemical Carnosol. J Biol Chem (2004) 279:8919–29. doi: 10.1074/jbc.M309660200
124. Lin HC, Cheng TH, Chen YC, Juan SH. Mechanism of Heme Oxygenase-1 Gene Induction by Quercetin in Rat Aortic Smooth Muscle Cells. Pharmacology (2004) 71:107–12. doi: 10.1159/000076947
125. Hui Y, Chengyong T, Cheng L, Haixia H, Yuanda Z, Weihua Y. Resveratrol Attenuates the Cytotoxicity Induced by Amyloid-Beta1-42 in PC12 Cells by Upregulating Heme Oxygenase-1 via the PI3K/Akt/Nrf2 Pathway. Neurochem Res (2018) 43:297–305. doi: 10.1007/s11064-017-2421-7
126. Song Y, Huang L, Yu J. Effects of Blueberry Anthocyanins on Retinal Oxidative Stress and Inflammation in Diabetes Through Nrf2/HO-1 Signaling. J Neuroimmunol (2016) 301:1–6. doi: 10.1016/j.jneuroim.2016.11.001
127. Luo D, Guo Y, Cheng Y, Zhao J, Wang Y, Rong J. Natural Product Celastrol Suppressed Macrophage M1 Polarization Against Inflammation in Diet-Induced Obese Mice via Regulating Nrf2/HO-1, MAP Kinase and NF-kappaB Pathways. Aging (Albany NY) (2017) 9:2069–82. doi: 10.18632/aging.101302
128. Haodang L, Lianmei Q, Ranhui L, Liesong C, Jun H, Yihua Z, et al. HO-1 Mediates the Anti-Inflammatory Actions of Sulforaphane in Monocytes Stimulated With a Mycoplasmal Lipopeptide. Chem Biol Interact (2019) 306:10–8. doi: 10.1016/j.cbi.2019.04.007
129. Dunigan K, Li Q, Li R, Locy ML, Wall S, Tipple TE. The Thioredoxin Reductase Inhibitor Auranofin Induces Heme Oxygenase-1 in Lung Epithelial Cells via Nrf2-Dependent Mechanisms. Am J Physiol Lung Cell Mol Physiol (2018) 315:L545–52. doi: 10.1152/ajplung.00214.2018
130. Naidu S, Wijayanti N, Santoso S, Kietzmann T, Immenschuh S. An Atypical NF-Kappa B-Regulated Pathway Mediates Phorbol Ester-Dependent Heme Oxygenase-1 Gene Activation in Monocytes. J Immunol (2008) 181:4113–23. doi: 10.4049/jimmunol.181.6.4113
131. Peng X, Dai C, Liu Q, Li J, Qiu J. Curcumin Attenuates on Carbon Tetrachloride-Induced Acute Liver Injury in Mice via Modulation of the Nrf2/HO-1 and TGF-Beta1/Smad3 Pathway. Molecules (2018) 23(1):215. doi: 10.3390/molecules23010215
Keywords: heme oxygenase-1, oxidative stress, iron, Mycobacterium tuberculosis, chemotherapy
Citation: Yang S, Ouyang J, Lu Y, Harypursat V and Chen Y (2022) A Dual Role of Heme Oxygenase-1 in Tuberculosis. Front. Immunol. 13:842858. doi: 10.3389/fimmu.2022.842858
Received: 24 December 2021; Accepted: 08 February 2022;
Published: 25 February 2022.
Edited by:
Bart Tummers, St. Jude Children’s Research Hospital, United StatesReviewed by:
Alexey Victorovich Sokolov, Institute of Experimental Medicine (RAS), RussiaCopyright © 2022 Yang, Ouyang, Lu, Harypursat and Chen. This is an open-access article distributed under the terms of the Creative Commons Attribution License (CC BY). The use, distribution or reproduction in other forums is permitted, provided the original author(s) and the copyright owner(s) are credited and that the original publication in this journal is cited, in accordance with accepted academic practice. No use, distribution or reproduction is permitted which does not comply with these terms.
*Correspondence: Yaokai Chen, eWFva2FpY2hlbkBob3RtYWlsLmNvbQ==
†These authors have contributed equally to this work
Disclaimer: All claims expressed in this article are solely those of the authors and do not necessarily represent those of their affiliated organizations, or those of the publisher, the editors and the reviewers. Any product that may be evaluated in this article or claim that may be made by its manufacturer is not guaranteed or endorsed by the publisher.
Research integrity at Frontiers
Learn more about the work of our research integrity team to safeguard the quality of each article we publish.