- 1Beirne B. Carter Center for Immunology Research, University of Virginia, Charlottesville, VA, United States
- 2Department of Microbiology, Immunology and Cancer Biology, University of Virginia, Charlottesville, VA, United States
Myeloid-derived suppressor cells (MDSCs) are generated under biological stress such as cancer, inflammatory tissue damage, and viral infection. In recent years, with occurrence of global infectious diseases, new discovery on MDSCs functions has been significantly expanded during viral infection and COVID-19. For a successful viral infection, pathogens viruses develop immune evasion strategies to avoid immune recognition. Numerous viruses induce the differentiation and expansion of MDSCs in order to suppress host immune responses including natural killer cells, antigen presenting cells, and T-cells. Moreover, MDSCs play an important role in regulation of immunopathogenesis by balancing viral infection and tissue damage. In this review article, we describe the overview of immunomodulation and genetic regulation of MDSCs during viral infection in the animal model and human studies. In addition, we include up-to-date review of role of MDSCs in SARS-CoV-2 infection and COVID-19. Finally, we discuss potential therapeutics targeting MDSCs.
Introduction
Coronavirus Induced Disease 2019 (COVID-19) caused by Severe Acute Respiratory Syndrome Corona Virus (SARS-CoV)-2 started in the Hubei province, China in 2019 (1). In the past two years, despite the efforts of many countries in response to the global pandemic situation, more than 260 million COVID-19 cases have been confirmed and over 5.2 million deaths have been reported to the World Health Organization (2). Patients infected with SARS-CoV-2 show various pathologies ranging from asymptomatic to mild, moderate, severe, and fulminant symtoms. Among them, critical pathology is followed by complications such as respiratory failure, myocarditis, sepsis, and various organ failures (3, 4).
It has been reported that the onset of COVID is not only directly affected by the virus, but also affected by immune responses such as cytokine storm syndrome, neutropenia, and lymphopenia (5, 6). However, the main potential targets for therapeutic purposes in relation to these immune mechanisms still remain challenging. Reduced function and depletion of CD8+ T cells were observed in patients with severe symptoms of COVID-19 (7, 8), CD4+ T cells have been reported to be important for patient recovery and protective immunity against SARS-CoV-2 (9, 10).
So far, immunological studies for COVID-19 individuals have mainly focused on innate and adaptive immune responses. Several studies report how the induction of MDSC and its role affect the progression of COVID-19 (11–14). Accordingly, studies confirm the role of the MDSC subset in severity of COVID-19 progression and provide potential therapeutic targets for COVID-19 (15–17). Further research identifying new biomarkers could be critical in developing therapeutics for disease prevention and amelioration. In this review, we discuss the latest studies focusing on the immunoregulating properties of MDSC and with biomarkers that may influence the intervention in treatment of COVID-19.
Dysregulated Immune Response in COVID-19
Since the outbreak of COVID-19, numerous publications related to immune responses and immunopathogenesis have been reported. The pathophysiology of COVID-19 has been listed as a complex state according to age, sex, pregnancy, presence of underlying disease, etc. (18–20). In the innate immune system, similar to Middle East respiratory syndrome (MERS)-CoV, SARS-CoV-2 modifies the signaling of TRAF3 and RNA sensor adapter molecules (MAVS) through proteins such as PLpro and inhibits type I interferon (IFN-1) production (21, 22). Antagonism of IFN-1 production aids viral replication, promotes release of pyroptotic products, and induces additional inflammatory responses (23). Pyroptosis is a form of programmed cell death within inflammatory cells (24) and is mediated by the production of IL-1β during SARS-CoV-2 infection (25).
Severe COVID-19 patients showed impaired IFN-1 signaling compared to mild patients, and developed an inappropriate inflammatory state due to early delay in IFN-1 expression and activation of pro-inflammatory cytokines (IL-1, IL-6, IL-8, MCP-1, and CXCL-10) (26–28). In addition, there were high viral titers and accumulation of monocyte-derived macrophages and neutrophils in the lungs. This condition leads to a systemic inflammatory response and cytokine storm syndrome via a massive release of cytokines. In addition, the risk of COVID-19 may increase or decrease differently depending on the number and activity of natural killer (NK) cells. Healthy children have been reported to have more NK cells than adults and the elderly (29), which might explain why children are expected to have a better defense against SARS-CoV-2. According to a recent study, the number of NK cells in adults with severe COVID-19 was reported to be low, and the activated form of CD56low NK cells increased to generate cytokines (30, 31).
In terms of the adaptive immune system, inefficient innate immune responses in SARS-CoV-2 infection lead to dampen adaptive immune responses and exacerbate inflammation (32). Pro-inflammatory cytokines induce the expansion of CD4+ and CD8+ T cells, decrease regulatory T, and lead to activation of Th1-type and B cells. When patients with underlying severe disease are infected with SARS-CoV-2, the number of lymphocytes is decreased while the blood levels of CD4, CD8, and regulatory T cells were also significantly lower than those in patients with mild disease. At this time, monocytes, macrophages, and T cells are accumulated in the lungs, and T cells migrate from the blood to these tissues to regulate the depletion of blood lymphocytes (23). In addition, a recent study showed that the lymphocyte counts of children infected with SARS-CoV-2 remained at a steady normal level compared to adults, and thus had less negative effect on immunomodulation (33).
Extensive studies on analyzing immune responses in COVID-19 patients show that the number of T or B lymphocytes, DC, NK cells, and HLA-DRhigh cells are reduced in patients with severe symptoms (34–36). Additionally, severe COVID-19-associated hyperinflammatory syndromes have been reported that they originate from a host innate immune response (37). Studies of transcriptome, proteomic, and epigenomics have revealed a wide range of functional impairments, including marked neutrophil hyperactivation symptoms in severe COVID-19 (38–41). Collectively, COVID-19 caused by SARS-CoV-2 is associated with a failure of innate and adaptive immune system regulation due to changes in other immune cells associated with a decrease in adaptive T cells. Although the link between the immune systems is still only partially explained, studies on MDSC, have been risen significantly and may provide explanation for dysregulated immune responses in COVID-19.
MDSC’s Immunoregulatory Function in Viral Infection
MDSC Phenotypes
Myeloid-derived suppressor cells (MDSCs) are defined as a heterogeneous population of immature bone marrow cells that suppress T cell responses, and was first described in a mouse model of lung cancer in 1987. Together with myeloid progenitor cells, they have the ability to suppress the immune responses at the forefront of viral infection (42). It has been reported that these cells have changed research fields related to cancer, inflammation, and immune response over the past 30 years, and even serve as a marker for distinguishing disease progression (43–45). MDSCs are mainly classified into two distinct group–neutrophilic/granulocytic (PMN)-MDSCs and monocytic (M)-MDSCs (46, 47) (Table 1). Granulocytic MDSCs have multi-lobed nuclei similar to polymorphonuclear cells, and monocytic MDSCs have a single, round nucleus; therefore, they look similar to monocytes (48). The morphological heterogeneity of these cells depends on the expression of Gr1, and the Gr1-specific antibody binds to both Ly6G and Ly6C, which are myeloid lineage differentiation antigens. Granulocyte and monocyte MDSCs of mice have phenotypes of CD11b+Ly6G+Ly6Clow and CD11b+Ly6G-Ly6Chigh, respectively (49). Human MDSCs are mainly identified as phenotypic markers Lin−HLA-DR−CD33+ or CD11b+CD14−CD33+ (46). In early studies on MDSC, the target of MDSC-mediated suppression was mainly T cells. After that, research on MDSC was gradually expanded, and it was additionally reported that it modulates innate immune cells such as NK cells, dendritic cells (DCs), and macrophages as well as adaptive immune cells such as B cells (50–52).
Immunosuppressive Function of MDSCs
Soluble factors related to MDSC function include reactive oxygen species (ROS), inducible nitric oxide synthase (iNOS), and arginase-1. Each of these key mediators independently attenuates the host immune response. Granulocytic MDSCs mainly use ROS generated by NADPH oxidase to cause immunosuppression. Monocytic MDSCs use iNOS to generate nitric oxide (NO) (48, 53, 54). iNOS nitrosylates T-cell receptor (TCR) together with arginase-1 to generate reactive nitrogen-oxide species that inhibit T-cell or induce apoptosis (55). Interestingly, both granulocytic and monocytic MDSCs utilize the action of arginase-1 to deplete L-arginine, which is required for T cell proliferation and function (56, 57). Effects of T-helper (Th)1 and Th2 cytokines, such as interleukin (IL)-2, IL-4, IL-13, and interferon (IFN)-γ on aginase-1 led to the identification of crosstalk between MDSCs and T cells (58–61).
MDSCs from hepatocellular carcinoma patients have been shown to inhibit NK cell cytotoxicity and IFN-γ release (62). This was an arginase-1 independent, contact-dependent suppression effect that required the expression of NKp30, a receptor for NK cells. In the case of MDSCs expanded from tumor mice, membrane-bound transforming growth factor-β1 (TGF-β1) inhibited NK cell cytotoxicity, IFN-γ production, and the expression of the activating receptor NKG2D (63). In addition, MDSCs cause differentiation of immature DCs in cancer and limit the immune response by inhibiting the antigen uptake ability of DCs (64–66).
During viral infection, similar increases in PMN- and M-MDSCs are initially observed in acute and chronic infection models, but rapidly return to baseline levels in acute cases. In chronic infection, it has been reported that it takes quite a long time to return to the baseline level. In the case of the mouse acute infection model, the inhibitory activity of M-MDSC did not appear at any time point. In the case of chronic infection model, it became more prominent from the 7th day to the 14th day after infection and decreased on the 30th day, but it was still detected. Recently, it has been reported that the ER stress response is essential for the inhibitory activity of M-MDSC in viral infection, and it is known that the acquisition of the most potent inhibitory activity is mediated by IFN-γ signaling (67).
Factors Involved on MDSCs Generation
Selective mediators have been shown to be responsible for the generation of MDSCs. Prostaglandin E2 (PGE2) exerts numerous biological actions, including anti-inflammatory and pro-inflammatory and is a key mediator for MDSC generation. Administration of PGE2 blocks DC differentiation and allows myeloid progenitor cells to acquire the characteristics of MDSC (68). In addition, anti-inflammatory mediators such as NOS2, iNOS, indolamine-2,3-deoxygenase (IDO), and IL-10 are secreted between PGE2 and cyclooxygenase 2 (COX-2), and their function as MDSCs regulating immunosuppression has been reported (69, 70). MDSCs suppress T cell effector function via co-expression of arginase1 and NOS1. When they are added to the co-culture of MDSC and activated T cells, T cell function is completely restored (71). Since the expression level of PGE2 is elevated when tumors form, the inhibitory effect of COX-2 is considered to partially affect the reduction in MDSC production. Among the many factors that can induce MDSC production, IL-1β further stimulates the recruitment of MDSCs in the non-tumor state (72). S100A9 increases the immunosuppressive ability of MDSCs by increasing the expression of nuclear factor-κB (NF-κB) dependent arginase by binding to the receptor for advanced glycation end products (RAGE) (46, 73).
To date, MDSCs have also been reported in numerous non-cancer pathologies, including viral, parasitic, bacterial, and fungal infections (74, 75). The functional role of accumulated MDSCs in most infectious diseases is to inhibit host defense and regulate inflammatory cytokines such as TNF-α, IL-1β, and IL-6 (76). Some studies have also mentioned a detrimental role of MDSC, but the study model, pathogen, disease stage, and T cell ratio show different results (77–79). In relation to the study of MDSC viral infection, induction of MDSC expansion of viruses such as hepatitis B or C virus, Epstein-Barr virus, human papillomavirus, influenza, and SARS-CoV-2 has been reported, and these induce virus persistence; nonetheless, evidence of MDSC’s tissue damage protective role has also been reported in other studies (80–82). In these various inhibitory mechanisms, the activity of MDSCs effectively interferes with enhancing tumor and non-tumor immune responses (Figure 1).
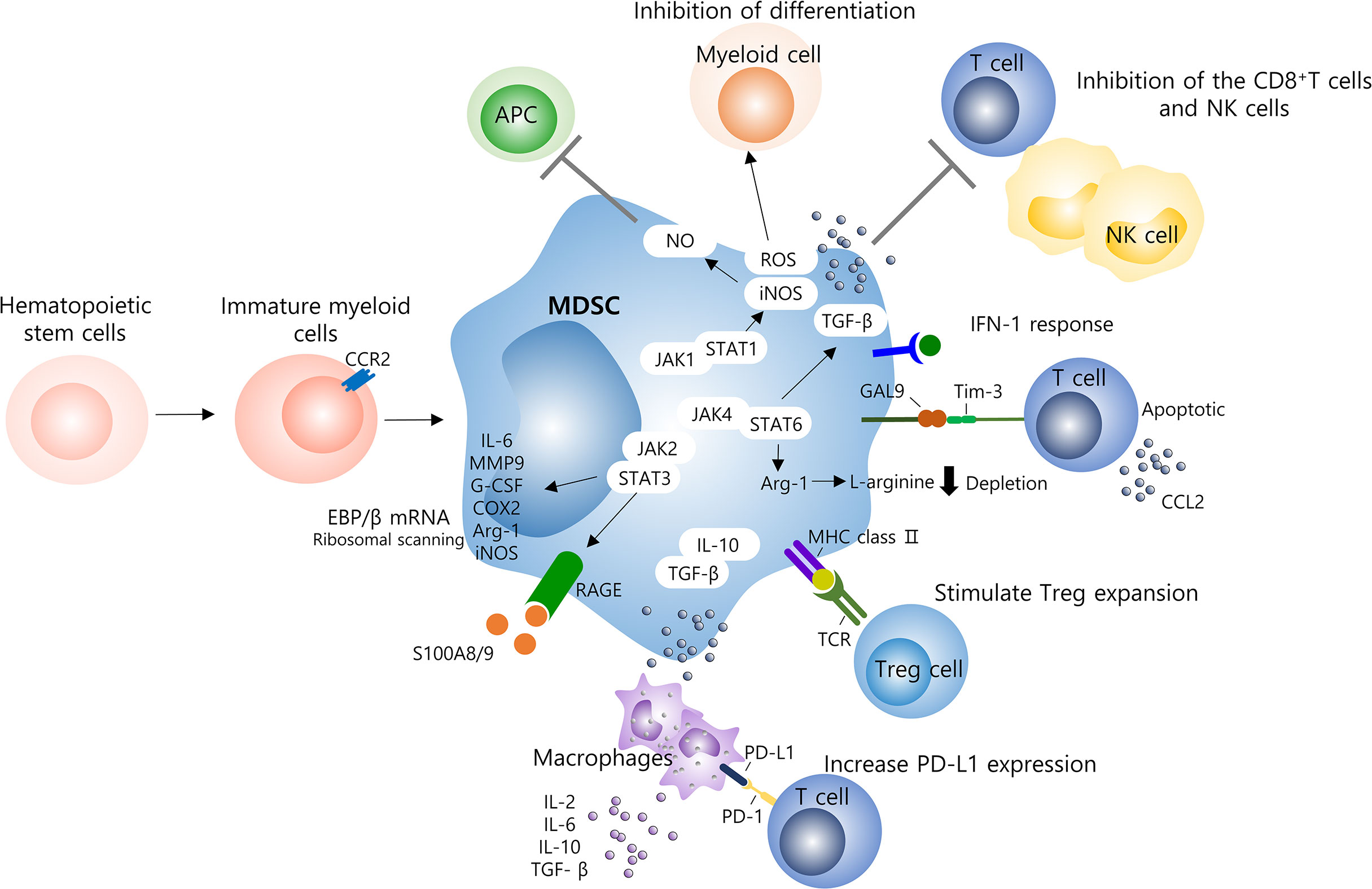
Figure 1 Molecular mechanisms of MDSCs on the immune system. Several mechanisms contribute to MDSC-induced immune suppression and hyperinflammatory activation in viral infection and including those with COVID-19 patients. Specially, MDSCs are able to suppress T cells, NK cells, and other myeloid cells function. T cells are mainly inhibited through the production of ROS or depletion of L-arginine, and the delayed production of IFN-1 seems to result in the continuous accumulation of MDSCs into the lungs. Several signaling pathways, such as STAT1/3/6, are involved, to increase levels of immunosuppressive factors such as ROS, iNOS, NO, and Arg-1, which inhibit T cell responses. High levels of PD-L1 found in MDSCs and macrophages can reduce the activation of antigen-specific T cells by binding to the PD-1 receptor on T cells. In addition, the release of IL-10 and TGF-β by MDSC induces additional inflammatory system of macrophages by recruiting lymphocytes and granulocytes as well as inflammatory monocytes. MDSCs, myeloid derived suppressor cells; NK, natural killer cell; ROS, reactive oxygen species; TGF-β, transforming growth factor-β; APC, antigen presenting cells; NO, nitric oxide; STAT, signal transducer and activator of transcription; JAK, janus activated kinase; IL, interleukin; MMP, matrix metalloporteinases; G-CSF, granulocyte colony stimulating factor; COX-2, cyclooxygenase 2; Arg, arginine.
The factors involved in the production and suppression of MDSC in various disease states include stem cell factor (SCF), HIF-1α, IL-6, macrophage colony stimulating factor (M-CSF), signal transducer and activator of transcription 3 (STAT3), myeloid-related protein S100A9, and IL-1β (51, 52, 70, 83, 84). In these microenvironmental factors, MDSCs go through a journey to the site of immune response to exert an immunosuppressive effect. In particular, the efflux from the blood to the tumor depends on CXCR4 and also affects the chemotaxis of mature myeloid cells (85). Another function of MDSC is epigenetic regulation. Histone deacetylase-2 (HDAC-2), which has been studied for a long time as a cancer treatment agent, converted monocyte MDSC to granulocyte MDSC, and the mechanism was suggested. In addition, HDAC-11 suppressed the expression of IL-10, an immunosuppressive cytokine, in chromosomal modification due to the action of tumor-derived factors (TDF) infiltrating MDSC (86). Meanwhile, the DNA methylation inhibitor zebularine decreased the expression of IDO, a potent immunosuppressive mediator of MDSC (87). This may support the epigenetic function of MDSCs regardless of acetylation or methylation.
MDSCs Function and Biomarkers in COVID-19
MDSCs are innate immune cells that can be increased in activity by infection-causing factors as previously described for various viral, parasitic, and bacterial infections, and also regulate the adaptive immune system. Several studies related to COVID-19 have reported that the high frequency of MDSC is associated with symptoms of severe disease and appears in the form of myeloid cell compartments that are difficult to control. Expansion of MDSC that occur in blood of severe COVID-19 patients had a close effect on lymphopenia and enhanced arginase activity (88). In particular, the ratio of MDSC to effector CD8+ T cells was increased in patients with severe pneumonia accompanied by acute respiratory distress syndrome (ARDS). MDSC frequencies in total circulating mononuclear cells ranging from mild to severe cases were recorded at a maximum of 25% and 90%, respectively (89). In addition, MDSC is involved in not only the inhibitory effect on T cell proliferation and activation, but also functional impairment of NK cells, B cell inhibition, Treg expansion induction, and downregulation of cytokine production by macrophages (89, 90). Markers suggesting granulocytes, such as eosinophils, neutrophils, and basophils, were highly expressed in COVID-19 patients, predicting the activity of granulocytic MDSCs. Decrease in the expression of granulocytes identified by the integrin CD11b, increase in the number of neutrophils identified in CD15+CD16+, and down regulation of Th2-related CRTH2 in eosinophils and basophils were established as the signature of COVID-19. In addition, the appearance of PD-L1 checkpoint expression in eosinophils and basophils was found to be related to severity (91). In the immune cell metabolism program of COVID-19 patients, voltage-dependent anion channel 1 (VDAC1) was expressed in the T cell population, which is associated with mitochondrial dysfunction and apoptosis. This may provide a means to predict disease severity, follow-up, and design metabolic therapy regimens (92).
PMN-MDSCs in COVID-19
PMN-MDSC was expanded during COVID-19, especially in patients requiring intensive care. A positive correlation was found between PMN-MDSC and the concentration level of inflammatory cytokines (IL-1β, IL-6, IL-8, and TNF-α) in the blood (93, 94). Inflammatory cytokines play a central role in inducing the expansion of MDSCs (46). The expression level of lectin-type oxidized LDL receptor 1 (LOX-1) was suggested as a marker to distinguish a subset of MDSCs with strong immunosuppressive ability in patients suffering from ARDS (16). In addition, a significant increase in hexokinase II+ PMN-MDSC was confirmed in severe COVID-19 patients with moderate or severe disease. In mild COVID-19 patients, IFN-stimulating inflammatory HLA-DRhiCD11chi monocytes increased, and IFN-1 deficiency was confirmed in severe patients. On the other hand, the high frequency of monocytes in HLA-DRlow and neutrophils in CD10lowCD101CXCR4+/- suggest emergent myelopoiesis as immunosuppressive markers in the blood and lungs of severe patients (95–101). Reductions in MDSCs during the COVID-19 recovery phase were associated with increases in inflammatory cytokines in the patient’s blood, including decreases in TGF-β (88). A multivariate regression analysis showed an association between the PMN-MDSC rate and fatal disease state, and the frequency of PMN-MDSC was higher in the non-survivors group than that in the recovered group (93). A recent study reported a significant correlation between C-reactive protein, ferritin, and lactate dehydrogenase levels and MDSC in patients with COVID-19. This indicates that immature PMN-MDSCs are associated with disease severity (102). Expansion of PMN-MDSCs and immature neutrophils in severe COVID-19 conditions indicates Th1 cell suppression and an increase in the frequency of Th17 cells due to strong polar migration to Th17 cells (103).
M-MDSC in COVID-19
M-MDSCs that have been mainly identified in PBMCs from acute COVID-19 patients, are associated with disease severity, and also suppress T cell responses (17). They are characterized by expressing VDAC and carnitine palmitoyltransferase I. M-MDSC isolated from COVID-19 patient inhibited T cell proliferation and IFN-γ production through an Arg-1-dependent mechanism, and increased Arg-1 and IL-6 levels (104). In an in vitro study that tested T cell proliferation, arginine supplementation helped restore T cell proliferation in patients with COVID-19, which had been reduced (88). In addition, the monocyte distribution width (MDW), which has recently emerged as a promising early biomarker of sepsis, has been considered as a key mediator of hyperinflammatory disorders in severe COVID-19 conditions. High MDW values have been reported to be associated with prognostic lethal outcome in COVID-19 patients (105).
The presence of neutrophils and macrophages was confirmed in the bronchoalveolar lavage fluid (BALF) from patients with severe COVID-19, while large amounts of cytokines and chemokines were secreted. Among the gene signatures identified in single-cell RNA sequencing (scRNAseq) data, gene sinatures such as H3F3B, IFITM1/2, SAT1, and S100A8 are associated with neutrophils, and CCL2/3/8, KLF6, and SPP1 are associated with macrophages (106, 107). This was the similar result as the high level of S100A8/9 found in the plasma of severe patients (98). High levels of proinflammatory cytokines and chemokines such as CXCL8, IL-6, and IL-10 were associated with upregulation of the monocyte compartment (108, 109). Another additional serum chemokines and cytokines (IL-6, IFN-λ3, IP-10, CXCL9, CXCR1/2/4 and CCL17), virus-sensing TLRs, HIF1α, and several genes involved in various metabolic regulation were identified in COVID-19 (110, 111). Furthermore, it was reported that soluble triggering receptor expressed on myeloid cells and an IL-6-based algorithm could serve as a very sensitive marker for early discrimination among patients with adverse reactions among COVID-19 patients (112). Although more research is needed, one of the markers that can predict the correct mortality rate among COVID-19 ICU patients is mid-regional pro-adrenomedullin (MR-proADM): it presented as high levels in non-survivors (113). In addition, several recent studies have shown new markers such as neutrophil-to-lymphocyte ratio, neutrophil-to-platelet ratio, uric acid level, total antioxidant capacity, eosinophil/PMN ratio, high-density lipoprotein, and apoprotein A1 (114–119).
In summary, monocytes and segmented neutrophils from peripheral blood migrate to immature myeloid cell candidates due to the elevation of cytokines and pro-inflammatory mediators during COVID-19, demonstrating the generation of emergency myeloid cells. Bone marrow cells identified in severe COVID-19 conditions are a subset of the primary immune cells that have initiated their activity, and studies on their ratio, inflammation, and identification of chemotactic genes will lead to potential diagnostics and therapeutics. Many studies related to COVID-19 have addressed the roles of MDSCs and their subsets, suggesting their selection as biomarkers for immune dysregulation in COVID-19 (120). This is clearly clinically meaningful given its correlation with disease. Candidates for newly added biomarkers related to COVID-19 are shown in Table 2.
Potential Therapeutics Targeting MDSC in COVID-19
Based on the expansion of MDSC in COVID-19, several molecular mechanisms for MDSC differentiation have been elucidated, making it possible to target and develop therapeutic agents. As cancer therapy, MDSC removal is beneficial to boost anti-tumor immunity such that chemotherapy reduces MDSC-mediated inhibition of T cells (125, 126). Rather this therapy was able to concert MDSCs into pro-inflammatory cells and disrupt tumor growth (127). Given the immunophenotype and suppression mechanism of the existing tumor microenvironment (TME) are diverse, it is challenging to target various human MDSC types (128). By using information gained from cancer studies, MDSCs-target therapeutic strategies can be applied to COVID-19 (70, 129). Table 3 summarizes the core of MDSCs targeting strategies for disease control and inhibition of MDSCs activity identified so far and the candidates applicable to COVID-19. Various types of drugs have been reported as 1) drugs that differentiate MDSCs into mature myeloid cells, 2) drugs that interfere with MDSC maturation from cell precursors, 3) drugs that reduce MDSC accumulation in peripheral organs, and 4) drugs that affect MDSC inhibitory function (120).
First, as a potential MDSC target, COX-2 inhibitors are useful because of significant role of the PGE-COX-2 axis in MDSC generation. COX-2 inhibitors inhibit the migration of MDSCs to tumor sites and reduce the incidence of several cancers by regulating transcriptomes (137–139). Prostaglandin D2 (PGD2) plays a role as a key mediator for lymphopenia in a recent COVID-19 study, and induces upregulation of M-MDSC through the DP2 receptor in group 2 innate lymphoid cells. Targeting PGD2/DP2 signaling using a related receptor antagonist (ramatroban) has been consedered as a therapy to address immune dysfunction and lymphopenia in COVID-19 (153).
Second, a phosphodiesterase-5 inhibitor (tadalafil) approved by the Federal Drug Administration (FDA) can inhibit MDSC by inducing downregulation of Arg1 and iNOS activity in several preclinical models (140–142). In a study using an animal model, tadalafil decreased the levels of glutamic oxaloacetic transaminase, an enzyme that promotes carbohydrate and protein metabolism in aflatoxin-induced liver cancer cells (154). Similarly, all-trans retinoic acid (ATRA), which has been previously used as a treatment for acute promyelocytic leukemia, induces MDSD differentiation, allowing NKT cells to mature into a state where they can be helped. ATRA also induces the expression of glutathione synthase, which leads to the production of glutathione, a ROS neutralizing agent (130). 1α,25-dihydroxyvitamin D3 (calcitriol) is able to inhibit IL-6-stimulated MDSC proliferation in a mouse esophageal squamous cell carcinoma model (131). This treatment can be extended to treatment of other diseases. Strong pathogen molecular patterns, such as CpG oligonucleotides and paclitaxel, have also been shown to differentiate MDSCs into mature myeloid cells (132, 133).
Third, potential drug can be designed toward aiming at the migration/recruitment of myeloid cells among treatment strategies in order to recover COVID-19-related hyperinflammation and the resulting immunomodulatory disorders (155). CXCR2 and CCR2/5 inhibitors are known to decrease the migration of MDSCs from the bone marrow to the circulation (143, 144). The importance of inhibiting MDSC proliferation and migration to TME with strategies such as anti-CXCR2 monoclonal antibody has also been reported (145). Leronlimab (CCR5 blocking antibody) decreased plasma IL-6 levels, restored the CD4/CD8 ratio, and induced a decrease in SARS-CoV-2 plasma viremia (156). The previously mentioned alamin S100A8 is strongly induced in COVID-19 patients and SARS-CoV-2 infected models. Paquinimod, known as an inhibitor of S100A8/9, induced a decrease in viral load in mice infected with SARS-CoV-2, resulting in relief from pneumonia (151). Although the number of neutrophils increases in the onset of COVID-19 and is accompanied by uncontrollable pathological damage, paquinimod decreased the number of neutrophils. The treatment of this drug can be suggested as a method for therapeutic purposes while simultaneously detecting abnormal changes in S100A8/9 and neutrophils in the COVID-19 state (151).
Lastly, drug can be designed to regulate metabolism of MDSCs. The inhibition of arginase-1 or supplementation of arginine in severe COVID-19 patients could be a treatment to restore depleted arginine and impaired T cell function (157). Moreover, reprogramming of MDSCs targeting epigenetics based on immune metabolism is also expected to solve the pulmonary inflammatory state of COVID-19 (158). This diverse list of drugs capable of manipulating MDSC populations could play an important role in MDSC-related treatment modalities in chronic inflammation, cancer, as well as in COVID-19.
Conclusions
MDSCs plays a pivotal role in regulating the innate immunity and adaptive immunity. Dysregulated immune response and MDSCs expansion have been reported in COVID-19 and other viral infections. The removal of MDSC leads to an increase in the immune response against the viral infection. Thereby, active research has been conducted to identify various MDSC phenotypic markers and discover therapeutic agents targeting MDSCs. Despite of the detrimental role of MDSCs in human inflammatory diseases, MDSCs are resistant to allograft transplantation and autoimmune diseases, limits the inflammatory damaging, and shows a tendency to return to a non-inflammatory state by homeostasis. There are still few research reports that MDSC directly inhibits hyperinflammation and helps clinical recovery, but additional studies are needed because the possibility cannot be ruled out. In this regard, it is necessary to keep the tolerogenic properties of MDSC in mind to develop MDSC-targeted therapeutics (81).
In patients infected with COVID-19, both PMN-MDSC and M-MDSC accumulation and their expansion have been confirmed. Thereby, various markers for identifying MDSCs are important in the development of diagnostic systems. It can also open up several possibilities for treatment by targeting immunosuppressive function of MDSCs. The correlation between BALF and serum MDSC frequency and clinical biomarkers will facilitate the consideration and selection of future therapeutics. In many of these studies, the severity of COVID-19 was clinically evaluated using metabolites, cytokines, chemokines, and several proteins related to various mechanisms such as inflammation and apoptosis. Candidates considered as therapeutic agents for COVID-19 were typically presented as specific cytokine inhibitors or immunomodulatory agents. Although official approval of future treatments will be necessary, reports on various therapeutic approaches and treatment prognosis for approved drugs will still be required.
Author Contributions
S-JP contributed to manuscript research and writing. D-eN contributed to manuscript research and writing. HS contributed to manuscript writing and review. YH contribute to manuscript supervision, writing, and review. All authors contributed to the article and approved the submitted version.
Funding
This work was supported by NIH Grant (DK122737 to YH).
Conflict of Interest
The authors declare that the research was conducted in the absence of any commercial or financial relationships that could be construed as a potential conflict of interest.
Publisher’s Note
All claims expressed in this article are solely those of the authors and do not necessarily represent those of their affiliated organizations, or those of the publisher, the editors and the reviewers. Any product that may be evaluated in this article, or claim that may be made by its manufacturer, is not guaranteed or endorsed by the publisher.
Acknowledgments
This review article is dedicated to the memory of Hugo Rosen, MD who has done pioneering studies on chronic liver diseases and liver immunology. We also thank the former members of the Hahn lab (Drs. Robert Tacke, Celeste Goh) for initiating first MDSCs studies in viral infection and elucidating the immunosuppressive function of MDSCs on T cells and NK cell responses.
References
1. Li Q, Guan X, Wu P, Wang X, Zhou L, Tong Y, et al. Early Transmission Dynamics in Wuhan, China, of Novel Coronavirus–Infected Pneumonia. N Engl J Med (2020) 382:1199–207. doi: 10.1056/NEJMoa2001316
2. W. H. O. Covid-19. World Health Organiazation (2021). Available at: http://www.who.int.
3. Grant MC, Geoghegan L, Arbyn M, Mohammed Z, McGuinness L, Clarke EL, et al. The Prevalence of Symptoms in 24,410 Adults Infected by the Novel Coronavirus (SARS-CoV-2; COVID-19): A Systematic Review and Meta-Analysis of 148 Studies From 9 Countries. PLoS One (2020) 15:e0234765. doi: 10.1371/journal.pone.0234765
4. Irabien-Ortiz Á, Carreras-Mora J, Sionis A, Pàmies J, Montiel J, Tauron M. Fulminant Myocarditis Due to COVID-19. Rev Esp Cardiol Engl Ed (2020) 73:503–4. doi: 10.1016/j.rec.2020.04.005
5. Moore JB, June CH. Cytokine Release Syndrome in Severe COVID-19. Science (2020) 368:473–4. doi: 10.1126/science.abb8925
6. Chen G, Wu D, Guo W, Cao Y, Huang D, Wang H, et al. Clinical and Immunological Features of Severe and Moderate Coronavirus Disease 2019. J Clin Invest (2020) 130:2620–9. doi: 10.1172/JCI137244
7. Zheng M, Gao Y, Wang G, Song G, Liu S, Sun D, et al. Functional Exhaustion of Antiviral Lymphocytes in COVID-19 Patients. Cell Mol Immunol (2020) 17:533–5. doi: 10.1038/s41423-020-0402-2
8. Mazzoni A, Salvati L, Maggi L, Capone M, Vanni A, Spinicci M, et al. Impaired Immune Cell Cytotoxicity in Severe COVID-19 Is IL-6 Dependent. J Clin Invest (2020) 130:4694–703. doi: 10.1172/JCI138554
9. Weiskopf D, Schmitz KS, Raadsen MP, Grifoni A, Okba NMA, Endeman H, et al. Phenotype and Kinetics of SARS-CoV-2–Specific T Cells in COVID-19 Patients With Acute Respiratory Distress Syndrome. Sci Immunol (2020) 5:eabd2071. doi: 10.1126/sciimmunol.abd2071
10. Grifoni A, Weiskopf D, Ramirez SI, Mateus J, Dan JM, Moderbacher CR, et al. Targets of T Cell Responses to SARS-CoV-2 Coronavirus in Humans With COVID-19 Disease and Unexposed Individuals. Cell (2020) 181:1489–501.e15. doi: 10.1016/j.cell.2020.05.015
11. Liao M, Liu Y, Yuan J, Wen Y, Xu G, Zhao J, et al. Single-Cell Landscape of Bronchoalveolar Immune Cells in Patients With COVID-19. Nat Med (2020) 26:842–4. doi: 10.1038/s41591-020-0901-9
12. Zhou Y, Fu B, Zheng X, Wang D, Zhao C, qi Y, et al. Pathogenic T-Cells and Inflammatory Monocytes Incite Inflammatory Storms in Severe COVID-19 Patients. Nat Sci Rev (2020) 7:998–1002. doi: 10.1093/nsr/nwaa041
13. Chen M-F, Kuan F-C, Yen T-C, Lu M-S, Lin P-Y, Chung Y-H, et al. IL-6-Stimulated CD11b+CD14+HLA-DR– Myeloid-Derived Suppressor Cells, Are Associated With Progression and Poor Prognosis in Squamous Cell Carcinoma of the Esophagus. Oncotarget (2014) 5:8716–28. doi: 10.18632/oncotarget.2368
14. Tomić S, Joksimović B, Bekić M, Vasiljević M, Milanović M, Čolić M, et al. Prostaglanin-E2 Potentiates the Suppressive Functions of Human Mononuclear Myeloid-Derived Suppressor Cells and Increases Their Capacity to Expand IL-10-Producing Regulatory T Cell Subsets. Front Immunol (2019) 10:475:475. doi: 10.3389/fimmu.2019.00475
15. Tomić S, Đokić J, Stevanović D, Ilić N, Gruden-Movsesijan A, Dinić M, et al. Reduced Expression of Autophagy Markers and Expansion of Myeloid-Derived Suppressor Cells Correlate With Poor T Cell Response in Severe COVID-19 Patients. Front Immunol (2021) 12:614599:614599. doi: 10.3389/fimmu.2021.614599
16. Coudereau R, Waeckel L, Cour M, Rimmele T, Pescarmona R, Fabri A, et al. Emergence of Immunosuppressive LOX-1+ PMN-MDSC in Septic Shock and Severe COVID-19 Patients With Acute Respiratory Distress Syndrome. J Leukoc Biol (2021) 0:JLB.4COVBCR0321-129R. doi: 10.1002/JLB.4COVBCR0321-129R
17. Thompson EA, Cascino K, Ordonez AA, Zhou W, Vaghasia A, Hamacher-Brady A, et al. Metabolic Programs Define Dysfunctional Immune Responses in Severe COVID-19 Patients. Cell Rep (2021) 34:108863. doi: 10.1016/j.celrep.2021.108863
18. Chen G, Liao Q, Ai J, Yang B, Bai H, Chen J, et al. Immune Response to COVID-19 During Pregnancy. Front Immunol (2021) 12:675476:675476. doi: 10.3389/fimmu.2021.675476
19. Abobaker A, Raba AA. Does COVID-19 Affect Male Fertility? World J Urol (2021) 39:975–6. doi: 10.1007/s00345-020-03208-w
20. Hansen CB, Jarlhelt I, Pérez-Alós L, Hummelshøj Landsy L, Loftager M, Rosbjerg A, et al. SARS-CoV-2 Antibody Responses Are Correlated to Disease Severity in COVID-19 Convalescent Individuals. J Immunol (2021) 206:109–17. doi: 10.4049/jimmunol.2000898
21. de Wit E, van Doremalen N, Falzarano D, Munster VJ. SARS and MERS: Recent Insights Into Emerging Coronaviruses. Nat Rev Microbiol (2016) 14:523–34. doi: 10.1038/nrmicro.2016.81
22. Gordon DE, Jang GM, Bouhaddou M, Xu J, Obernier K, O’Meara MJ, et al. A SARS-CoV-2-Human Protein-Protein Interaction Map Reveals Drug Targets and Potential Drug-Repurposing. Syst Biol (2020) 583:459–68. doi: 10.1101/2020.03.22.002386
23. Tay MZ, Poh CM, Rénia L, MacAry PA, Ng LFP. The Trinity of COVID-19: Immunity, Inflammation and Intervention. Nat Rev Immunol (2020) 20:363–74. doi: 10.1038/s41577-020-0311-8
24. Fink SL, Cookson BT. Apoptosis, Pyroptosis, and Necrosis: Mechanistic Description of Dead and Dying Eukaryotic Cells. Infect Immun (2005) 73:1907–16. doi: 10.1128/IAI.73.4.1907-1916.2005
25. Huang C, Wang Y, Li X, Ren L, Zhao J, Hu Y, et al. Clinical Features of Patients Infected With 2019 Novel Coronavirus in Wuhan, China. Lancet (2020) 395:497–506. doi: 10.1016/S0140-6736(20)30183-5
26. Channappanavar R, Fehr AR, Vijay R, Mack M, Zhao J, Meyerholz DK, et al. Dysregulated Type I Interferon and Inflammatory Monocyte-Macrophage Responses Cause Lethal Pneumonia in SARS-CoV-Infected Mice. Cell Host Microbe (2016) 19:181–93. doi: 10.1016/j.chom.2016.01.007
27. Bastard P, Rosen LB, Zhang Q, Michailidis E, Hoffmann H-H, Zhang Y, et al. Autoantibodies Against Type I IFNs in Patients With Life-Threatening COVID-19. Science (2020) 370:eabd4585. doi: 10.1126/science.abd4585
28. Hadjadj J, Yatim N, Barnabei L, Corneau A, Boussier J, Smith N, et al. Impaired Type I Interferon Activity and Inflammatory Responses in Severe COVID-19 Patients. Science (2020) 369:718–24. doi: 10.1126/science.abc6027
29. Yabuhara A, Kawal H, Komiyama A. Development of Natural Killer Cytotoxicity During Childhood: Marked Increases in Number of Natural Killer Cells With Adequate Cytotoxic Abilities During Infancy to Early Childhood. Pediatr Res (1990) 28:316–21. doi: 10.1203/00006450-199010000-00002
30. Wang F, Nie J, Wang H, Zhao Q, Xiong Y, Deng L, et al. Characteristics of Peripheral Lymphocyte Subset Alteration in COVID-19 Pneumonia. J Infect Dis (2020) 221:1762–9. doi: 10.1093/infdis/jiaa150
31. Maucourant C, Filipovic I, Ponzetta A, Aleman S, Cornillet M, Hertwig L, et al. Natural Killer Cell Immunotypes Related to COVID-19 Disease Severity. Sci Immunol (2020) 5:eabd6832. doi: 10.1126/sciimmunol.abd6832
32. Paces J, Strizova Z, Smrz D, Cerny J. COVID-19 and the Immune System. Physiol Res (2020) 69:379–88. doi: 10.33549/physiolres.934492
33. Du W, Yu J, Wang H, Zhang X, Zhang S, Li Q, et al. Clinical Characteristics of COVID-19 in Children Compared With Adults in Shandong Province, China. Infection (2020) 48:445–52. doi: 10.1007/s15010-020-01427-2
34. Wang F, Hou H, Yao Y, Wu S, Huang M, Ran X, et al. Systemically Comparing Host Immunity Between Survived and Deceased COVID-19 Patients. Cell Mol Immunol (2020) 17:875–7. doi: 10.1038/s41423-020-0483-y
35. Wang C, Xu J, Wang S, Pan S, Zhang J, Han Y, et al. Imaging Mass Cytometric Analysis of Postmortem Tissues Reveals Dysregulated Immune Cell and Cytokine Responses in Multiple Organs of COVID-19 Patients. Front Microbiol (2020) 11:600989:600989. doi: 10.3389/fmicb.2020.600989
36. Bozzano F, Dentone C, Perrone C, Di Biagio A, Fenoglio D, Parodi A, et al. Extensive Activation, Tissue Trafficking, Turnover and Functional Impairment of NK Cells in COVID-19 Patients at Disease Onset Associates With Subsequent Disease Severity. PLoS Pathog (2021) 17:e1009448. doi: 10.1371/journal.ppat.1009448
37. Gustine JN, Jones D. Immunopathology of Hyperinflammation in COVID-19. Am J Pathol (2021) 191:4–17. doi: 10.1016/j.ajpath.2020.08.009
38. Hargadon KM. A Call for Discovery: Re-Envisioning The Cancer Genome Atlas as a Blueprint for a TCGA2.0—The COVID-19 Genome Atlas. Clin Transl Discov (2021) 1:e7. doi: 10.1002/ctd2.7
39. Li C-X, Gao J, Zhang Z, Chen L, Li X, Zhou M, et al. Multiomics Integration-Based Molecular Characterizations of COVID-19. Brief Bioinform (2021) 239:bbab485. doi: 10.1093/bib/bbab485
40. Tan HW, Xu Y-M, Lau ATY. Human Bronchial-Pulmonary Proteomics in Coronavirus Disease 2019 (COVID-19) Pandemic: Applications and Implications. Expert Rev Proteomics (2021) 14789450:2021.2010549. doi: 10.1080/14789450.2021.2010549
41. Spijkerman R, Bongers SH, Bindels BJJ, Tinnevelt GH, Giustarini G, Jorritsma NKN, et al. Flow Cytometric Evaluation of the Neutrophil Compartment in COVID-19 at Hospital Presentation: A Normal Response to an Abnormal Situation. J Leukoc Biol (2021) 109:99–114. doi: 10.1002/JLB.5COVA0820-520RRR
42. Young MR, Newby M, Wepsic HT. Hematopoiesis and Suppressor Bone Marrow Cells in Mice Bearing Large Metastatic Lewis Lung Carcinoma Tumors. Cancer Res (1987) 47:100–5 https://cancerres.aacrjournals.org/content/47/1/100.article-info.
43. Cole S, Montero A, Garret-Mayer E, Onicescu G, Vandenberg T, Hutchens S, et al. Elevated Circulating Myeloid Derived Suppressor Cells (MDSC) Are Associated With Inferior Overall Survival (OS) and Correlate With Circulating Tumor Cells (CTC) in Patients With Metastatic Breast Cancer. Poster Session Abstracts Am Assoc Cancer Res (2009) 69:4135–5. doi: 10.1158/0008-5472.SABCS-09-4135
44. Diaz-Montero CM, Salem ML, Nishimura MI, Garrett-Mayer E, Cole DJ, Montero AJ. Increased Circulating Myeloid-Derived Suppressor Cells Correlate With Clinical Cancer Stage, Metastatic Tumor Burden, and Doxorubicin–Cyclophosphamide Chemotherapy. Cancer Immunol Immunother (2009) 58:49–59. doi: 10.1007/s00262-008-0523-4
45. Hegde S, Leader AM, Merad M. MDSC: Markers, Development, States, and Unaddressed Complexity. Immunity (2021) 54:875–84. doi: 10.1016/j.immuni.2021.04.004
46. Gabrilovich DI, Nagaraj S. Myeloid-Derived Suppressor Cells as Regulators of the Immune System. Nat Rev Immunol (2009) 9:162–74. doi: 10.1038/nri2506
47. Bronte V, Brandau S, Chen S-H, Colombo MP, Frey AB, Greten TF, et al. Recommendations for Myeloid-Derived Suppressor Cell Nomenclature and Characterization Standards. Nat Commun (2016) 7:12150. doi: 10.1038/ncomms12150
48. Ostrand-Rosenberg S, Sinha P. Myeloid-Derived Suppressor Cells: Linking Inflammation and Cancer. J Immunol (2009) 182:4499–506. doi: 10.4049/jimmunol.0802740
49. Youn J-I, Nagaraj S, Collazo M, Gabrilovich DI. Subsets of Myeloid-Derived Suppressor Cells in Tumor-Bearing Mice. J Immunol (2008) 181:5791–802. doi: 10.4049/jimmunol.181.8.5791
50. Serafini P, Mgebroff S, Noonan K, Borrello I. Myeloid-Derived Suppressor Cells Promote Cross-Tolerance in B-Cell Lymphoma by Expanding Regulatory T Cells. Cancer Res (2008) 68:5439–49. doi: 10.1158/0008-5472.CAN-07-6621
51. Veglia F, Perego M, Gabrilovich D. Myeloid-Derived Suppressor Cells Coming of Age. Nat Immunol (2018) 19:108–19. doi: 10.1038/s41590-017-0022-x
52. Ostrand-Rosenberg S, Fenselau C. Myeloid-Derived Suppressor Cells: Immune-Suppressive Cells That Impair Antitumor Immunity and Are Sculpted by Their Environment. J Immunol (2018) 200:422–31. doi: 10.4049/jimmunol.1701019
53. Dietlin TA, Hofman FM, Lund BT, Gilmore W, Stohlman SA, van der Veen RC. Mycobacteria-Induced Gr-1 + Subsets From Distinct Myeloid Lineages Have Opposite Effects on T Cell Expansion. J Leukoc Biol (2007) 81:1205–12. doi: 10.1189/jlb.1006640
54. Peranzoni E, Zilio S, Marigo I, Dolcetti L, Zanovello P, Mandruzzato S, et al. Myeloid-Derived Suppressor Cell Heterogeneity and Subset Definition. Curr Opin Immunol (2010) 22:238–44. doi: 10.1016/j.coi.2010.01.021
55. Kusmartsev S, Gabrilovich DI. STAT1 Signaling Regulates Tumor-Associated Macrophage-Mediated T Cell Deletion. J Immunol (2005) 174:4880–91. doi: 10.4049/jimmunol.174.8.4880
56. Cuervo H, Guerrero NA, Carbajosa S, Beschin A, De Baetselier P, Gironès N, et al. Myeloid-Derived Suppressor Cells Infiltrate the Heart in Acute Trypanosoma Cruzi Infection. J Immunol (2011) 187:2656–65. doi: 10.4049/jimmunol.1002928
57. Highfill SL, Rodriguez PC, Zhou Q, Goetz CA, Koehn BH, Veenstra R, et al. Bone Marrow Myeloid-Derived Suppressor Cells (MDSCs) Inhibit Graft-Versus-Host Disease (GVHD) via an Arginase-1–Dependent Mechanism That Is Up-Regulated by Interleukin-13. Blood (2010) 116:5738–47. doi: 10.1182/blood-2010-06-287839
58. Rodriguez PC, Ernstoff MS, Hernandez C, Atkins M, Zabaleta J, Sierra R, et al. Arginase I–Producing Myeloid-Derived Suppressor Cells in Renal Cell Carcinoma Are a Subpopulation of Activated Granulocytes. Cancer Res (2009) 69:1553–60. doi: 10.1158/0008-5472.CAN-08-1921
59. Bronte V, Serafini P, De Santo C, Marigo I, Tosello V, Mazzoni A, et al. IL-4-Induced Arginase 1 Suppresses Alloreactive T Cells in Tumor-Bearing Mice. J Immunol (2003) 170:270–8. doi: 10.4049/jimmunol.170.1.270
60. Rutschman R, Lang R, Hesse M, Ihle JN, Wynn TA, Murray PJ. Cutting Edge: Stat6-Dependent Substrate Depletion Regulates Nitric Oxide Production. J Immunol (2001) 166:2173–7. doi: 10.4049/jimmunol.166.4.2173
61. Liscovsky MV, Ranocchia RP, Gorlino CV, Alignani DO, Morón G, Maletto BA, et al. Interferon-γ Priming Is Involved in the Activation of Arginase by Oligodeoxinucleotides Containing CpG Motifs in Murine Macrophages. Immunology (2009) 128:e159–69. doi: 10.1111/j.1365-2567.2008.02938.x
62. Hoechst B, Voigtlaender T, Ormandy L, Gamrekelashvili J, Zhao F, Wedemeyer H, et al. Myeloid Derived Suppressor Cells Inhibit Natural Killer Cells in Patients With Hepatocellular Carcinoma Via the NKp30 Receptor. Hepatology (2009) 50:799–807. doi: 10.1002/hep.23054
63. Li H, Han Y, Guo Q, Zhang M, Cao X. Cancer-Expanded Myeloid-Derived Suppressor Cells Induce Anergy of NK Cells Through Membrane-Bound TGF-β1. J Immunol (2009) 182:240–9. doi: 10.4049/jimmunol.182.1.240
64. Cheng P, Corzo CA, Luetteke N, Yu B, Nagaraj S, Bui MM, et al. Inhibition of Dendritic Cell Differentiation and Accumulation of Myeloid-Derived Suppressor Cells in Cancer Is Regulated by S100A9 Protein. J Exp Med (2008) 205:2235–49. doi: 10.1084/jem.20080132
65. Ma Y, Shurin GV, Peiyuan Z, Shurin MR. Dendritic Cells in the Cancer Microenvironment. J Cancer (2013) 4:36–44. doi: 10.7150/jca.5046
66. Ostrand-Rosenberg S, Sinha P, Beury DW, Clements VK. Cross-Talk Between Myeloid-Derived Suppressor Cells (MDSC), Macrophages, and Dendritic Cells Enhances Tumor-Induced Immune Suppression. Semin Cancer Biol (2012) 22:275–81. doi: 10.1016/j.semcancer.2012.01.011
67. Tcyganov EN, Hanabuchi S, Hashimoto A, Campbell D, Kar G, Slidel TWF, et al. Distinct Mechanisms Govern Populations of Myeloid-Derived Suppressor Cells in Chronic Viral Infection and Cancer. J Clin Invest (2021) 131:e145971. doi: 10.1172/JCI145971
68. Obermajer N, Muthuswamy R, Lesnock J, Edwards RP, Kalinski P. Positive Feedback Between PGE2 and COX2 Redirects the Differentiation of Human Dendritic Cells Toward Stable Myeloid-Derived Suppressor Cells. Blood (2011) 118:5498–505. doi: 10.1182/blood-2011-07-365825
69. Harden JL, Egilmez NK. Indoleamine 2,3-Dioxygenase and Dendritic Cell Tolerogenicity. Immunol Invest (2012) 41:738–64. doi: 10.3109/08820139.2012.676122
70. Veglia F, Sanseviero E, Gabrilovich DI. Myeloid-Derived Suppressor Cells in the Era of Increasing Myeloid Cell Diversity. Nat Rev Immunol (2021) 21:485–98. doi: 10.1038/s41577-020-00490-y
71. Raber P, Ochoa AC, Rodríguez PC. Metabolism of L-Arginine by Myeloid-Derived Suppressor Cells in Cancer: Mechanisms of T Cell Suppression and Therapeutic Perspectives. Immunol Invest (2012) 41:614–34. doi: 10.3109/08820139.2012.680634
72. Elkabets M, Ribeiro VSG, Dinarello CA, Ostrand-Rosenberg S, Di Santo JP, Apte RN, et al. IL-1β Regulates a Novel Myeloid-Derived Suppressor Cell Subset That Impairs NK Cell Development and Function. Eur J Immunol (2010) 40:3347–57. doi: 10.1002/eji.201041037
73. Wang L, Chang EWY, Wong SC, Ong S-M, Chong DQY, Ling KL. Increased Myeloid-Derived Suppressor Cells in Gastric Cancer Correlate With Cancer Stage and Plasma S100A8/A9 Proinflammatory Proteins. J Immunol Baltim Md 1950 (2013) 190:794–804. doi: 10.4049/jimmunol.1202088
74. Medina E, Hartl D. Myeloid-Derived Suppressor Cells in Infection: A General Overview. J Innate Immun (2018) 10:407–13. doi: 10.1159/000489830
75. Yaseen MM, Abuharfeil NM, Darmani H. Myeloid-Derived Suppressor Cells and the Pathogenesis of Human Immunodeficiency Virus Infection. Open Biol (2021) 11:210216. doi: 10.1098/rsob.210216
76. Tengesdal IW, Dinarello A, Powers NE, Burchill MA, Joosten LAB, Marchetti C, et al. Tumor NLRP3-Derived IL-1β Drives the IL-6/STAT3 Axis Resulting in Sustained MDSC-Mediated Immunosuppression. Front Immunol (2021) 12:661323:661323. doi: 10.3389/fimmu.2021.661323
77. Ferrer G, Jung B, Chiu PY, Aslam R, Palacios F, Mazzarello AN, et al. Myeloid-Derived Suppressor Cell Subtypes Differentially Influence T-Cell Function, T-Helper Subset Differentiation, and Clinical Course in CLL. Leukemia (2021) 35:3163–75. doi: 10.1038/s41375-021-01249-7
78. Priceputu E, Cool M, Bouchard N, Caceres-Cortes JR, Lowell CA, Hanna Z, et al. HIV-1 Nef Induces Hck/Lyn-Dependent Expansion of Myeloid-Derived Suppressor Cells Associated With Elevated Interleukin-17/G-CSF Levels. J Virol (2021) 95:1–22. doi: 10.1128/JVI.00471-21
79. Vanhaver C, van der Bruggen P, Bruger AM. MDSC in Mice and Men: Mechanisms of Immunosuppression in Cancer. J Clin Med (2021) 10:2872. doi: 10.3390/jcm10132872
80. Jiménez-Cortegana C, Liró J, Palazón-Carrión N, Salamanca E, Sojo-Dorado J, de la Cruz-Merino L, et al. Increased Blood Monocytic Myeloid Derived Suppressor Cells But Low Regulatory T Lymphocytes in Patients With Mild COVID-19. Viral Immunol (2021) 34:639–45. doi: 10.1089/vim.2021.0044
81. Goh C, Narayanan S, Hahn YS. Myeloid-Derived Suppressor Cells: The Dark Knight or the Joker in Viral Infections? Immunol Rev (2013) 255:210–21. doi: 10.1111/imr.12084
82. Ginex T, Luque FJ. Searching for Effective Antiviral Small Molecules Against Influenza A Virus: A Patent Review. Expert Opin Ther Pat (2021) 31:53–66. doi: 10.1080/13543776.2020.1831471
83. Condamine T, Gabrilovich DI. Molecular Mechanisms Regulating Myeloid-Derived Suppressor Cell Differentiation and Function. Trends Immunol (2011) 32:19–25. doi: 10.1016/j.it.2010.10.002
84. Rieber N, Brand A, Hector A, Graepler-Mainka U, Ost M, Schäfer I, et al. Flagellin Induces Myeloid-Derived Suppressor Cells: Implications for Pseudomonas Aeruginosa Infection in Cystic Fibrosis Lung Disease. J Immunol (2013) 190:1276–84. doi: 10.4049/jimmunol.1202144
85. Obermajer N, Muthuswamy R, Odunsi K, Edwards RP, Kalinski P. PGE 2 -Induced CXCL12 Production and CXCR4 Expression Controls the Accumulation of Human MDSCs in Ovarian Cancer Environment. Cancer Res (2011) 71:7463–70. doi: 10.1158/0008-5472.CAN-11-2449
86. Youn J-I, Kumar V, Collazo M, Nefedova Y, Condamine T, Cheng P, et al. Epigenetic Silencing of Retinoblastoma Gene Regulates Pathologic Differentiation of Myeloid Cells in Cancer. Nat Immunol (2013) 14:211–20. doi: 10.1038/ni.2526
87. Liu H, Xue Z, Sjögren H-O, Salford LG, Widegren B. Low Dose Zebularine Treatment Enhances Immunogenicity of Tumor Cells. Cancer Lett (2007) 257:107–15. doi: 10.1016/j.canlet.2007.07.013
88. Reizine F, Lesouhaitier M, Gregoire M, Pinceaux K, Gacouin A, Maamar A, et al. SARS-CoV-2-Induced ARDS Associates With MDSC Expansion, Lymphocyte Dysfunction, and Arginine Shortage. J Clin Immunol (2021) 41:515–25. doi: 10.1007/s10875-020-00920-5
89. Agrati C, Sacchi A, Bordoni V, Cimini E, Notari S, Grassi G, et al. Expansion of Myeloid-Derived Suppressor Cells in Patients With Severe Coronavirus Disease (COVID-19). Cell Death Differ (2020) 27:3196–207. doi: 10.1038/s41418-020-0572-6
90. Sacchi A, Grassi G, Notari S, Gili S, Bordoni V, Tartaglia E, et al. Expansion of Myeloid Derived Suppressor Cells Contributes to Platelet Activation by L-Arginine Deprivation During SARS-CoV-2 Infection. Cells (2021) 10:2111. doi: 10.3390/cells10082111
91. Vitte J, Diallo AB, Boumaza A, Lopez A, Michel M, Allardet-Servent J, et al. A Granulocytic Signature Identifies COVID-19 and Its Severity. J Infect Dis (2020) 222:1985–96. doi: 10.1093/infdis/jiaa591
92. Thompson EA, Cascino K, Ordonez AA, Zhou W, Vaghasia A, Hamacher-Brady A, et al. Metabolic Programs Define Dysfunctional Immune Responses in Severe COVID-19 Patients. Cell Rep (2021) 34:108863. doi: 10.1016/j.celrep.2021.108863
93. Sacchi A, Grassi G, Bordoni V, Lorenzini P, Cimini E, Casetti R, et al. Early Expansion of Myeloid-Derived Suppressor Cells Inhibits SARS-CoV-2 Specific T-Cell Response and may Predict Fatal COVID-19 Outcome. Cell Death Dis (2020) 11:921. doi: 10.1038/s41419-020-03125-1
94. Takano T, Matsumura T, Adachi Y, Terahara K, Moriyama S, Onodera T, et al. Myeloid Cell Dynamics Correlating With Clinical Outcomes of Severe COVID-19 in Japan. Int Immunol (2021) 33:241–7. doi: 10.1093/intimm/dxab005
95. Schulte-Schrepping J, Reusch N, Paclik D, Baßler K, Schlickeiser S, Zhang B, et al. Severe COVID-19 Is Marked by a Dysregulated Myeloid Cell Compartment. Cell (2020) 182:1419–40.e23. doi: 10.1016/j.cell.2020.08.001
96. Wilk AJ, Lee MJ, Wei B, Parks B, Pi R, Martínez-Colón GJ, et al. Multi-Omic Profiling Reveals Widespread Dysregulation of Innate Immunity and Hematopoiesis in COVID-19. J Exp Med (2021) 218:e20210582. doi: 10.1084/jem.20210582
97. Reyes M, Filbin MR, Bhattacharyya RP, Sonny A, Mehta A, Billman K, et al. Plasma From Patients With Bacterial Sepsis or Severe COVID-19 Induces Suppressive Myeloid Cell Production From Hematopoietic Progenitors In Vitro. Sci Transl Med (2021) 13:eabe9599. doi: 10.1126/scitranslmed.abe9599
98. Silvin A, Chapuis N, Dunsmore G, Goubet A-G, Dubuisson A, Derosa L, et al. Elevated Calprotectin and Abnormal Myeloid Cell Subsets Discriminate Severe From Mild COVID-19. Cell (2020) 182:1401–18.e18. doi: 10.1016/j.cell.2020.08.002
99. Peruzzi B, Bencini S, Capone M, Mazzoni A, Maggi L, Salvati L, et al. Quantitative and Qualitative Alterations of Circulating Myeloid Cells and Plasmacytoid DC in SARS-CoV-2 Infection. Immunology (2020) 161:345–53. doi: 10.1111/imm.13254
100. Benlyamani I, Venet F, Coudereau R, Gossez M, Monneret G. Monocyte HLA-DR Measurement by Flow Cytometry in COVID -19 Patients: An Interim Review. Cytom A (2020) 97:1217–21. doi: 10.1002/cyto.a.24249
101. Arunachalam PS, Wimmers F, Mok CKP, Perera RAPM, Scott M, Hagan T, et al. Systems Biological Assessment of Immunity to Mild Versus Severe COVID-19 Infection in Humans. Science (2020) 369:1210–20. doi: 10.1126/science.abc6261
102. Emsen A, Sumer S, Tulek B, Cizmecioglu H, Vatansev H, Goktepe MH, et al. Correlation of Myeloid-Derived Suppressor Cells With C-Reactive Protein, Ferritin and Lactate Dehydrogenase Levels in Patients With Severe COVID-19. Scand J Immunol (2021) 95:e13108. doi: 10.1111/sji.13108
103. Parackova Z, Bloomfield M, Klocperk A, Sediva A. Neutrophils Mediate Th17 Promotion in COVID-19 Patients. J Leukoc Biol (2021) 109:73–6. doi: 10.1002/JLB.4COVCRA0820-481RRR
104. Falck-Jones S, Vangeti S, Yu M, Falck-Jones R, Cagigi A, Badolati I, et al. Functional Monocytic Myeloid-Derived Suppressor Cells Increase in Blood But Not Airways and Predict COVID-19 Severity. J Clin Invest (2021) 131:e144734. doi: 10.1172/JCI144734
105. Riva G, Castellano S, Nasillo V, Ottomano AM, Bergonzini G, Paolini A, et al. Monocyte Distribution Width (MDW) as Novel Inflammatory Marker With Prognostic Significance in COVID-19 Patients. Sci Rep (2021) 11:12716. doi: 10.1038/s41598-021-92236-6
106. Shaath H, Vishnubalaji R, Elkord E, Alajez NM. Single-Cell Transcriptome Analysis Highlights a Role for Neutrophils and Inflammatory Macrophages in the Pathogenesis of Severe COVID-19. Cells (2020) 9:2374. doi: 10.3390/cells9112374
107. Xu G, Qi F, Li H, Yang Q, Wang H, Wang X, et al. The Differential Immune Responses to COVID-19 in Peripheral and Lung Revealed by Single-Cell RNA Sequencing. Cell Discov (2020) 6:73. doi: 10.1038/s41421-020-00225-2
108. Reyes M, Filbin MR, Bhattacharyya RP, Sonny A, Mehta A, Billman K, et al. Plasma From Patients With Bacterial Sepsis or Severe COVID-19 Induces Suppressive Myeloid Cell Production From Hematopoietic Progenitors In Vitro. Sci Transl Med (2021) 13:eabe9599. doi: 10.1126/scitranslmed.abe9599
109. Park JH, Lee HK. Re-Analysis of Single Cell Transcriptome Reveals That the NR3C1-CXCL8-Neutrophil Axis Determines the Severity of COVID-19. Front Immunol (2020) 11:2145:2145. doi: 10.3389/fimmu.2020.02145
110. Sugiyama M, Kinoshita N, Ide S, Nomoto H, Nakamoto T, Saito S, et al. Serum CCL17 Level Becomes a Predictive Marker to Distinguish Between Mild/Moderate and Severe/Critical Disease in Patients With COVID-19. Gene (2021) 766:145145. doi: 10.1016/j.gene.2020.145145
111. Taniguchi-Ponciano K, Vadillo E, Mayani H, Gonzalez-Bonilla CR, Torres J, Majluf A, et al. Increased Expression of Hypoxia-Induced Factor 1α mRNA and Its Related Genes in Myeloid Blood Cells From Critically Ill COVID-19 Patients. Ann Med (2021) 53:197–207. doi: 10.1080/07853890.2020.1858234
112. Van Singer M, Brahier T, Ngai M, Wright J, Weckman AM, Erice C, et al. COVID-19 Risk Stratification Algorithms Based on sTREM-1 and IL-6 in Emergency Department. J Allergy Clin Immunol (2021) 147:99–106.e4. doi: 10.1016/j.jaci.2020.10.001
113. Montrucchio G, Sales G, Rumbolo F, Palmesino F, Fanelli V, Urbino R, et al. Effectiveness of Mid-Regional Pro-Adrenomedullin (MR-proADM) as Prognostic Marker in COVID-19 Critically Ill Patients: An Observational Prospective Study. PLoS One (2021) 16:e0246771. doi: 10.1371/journal.pone.0246771
114. López-Escobar A, Madurga R, Castellano JM, Ruiz de Aguiar S, Velázquez S, Bucar M, et al. Hemogram as Marker of in-Hospital Mortality in COVID-19. J Investig Med (2021) 69:962–9. doi: 10.1136/jim-2021-001810
115. Li G, Wu X, Zhou C, Wang Y, Song B, Cheng X, et al. Uric Acid as a Prognostic Factor and Critical Marker of COVID-19. Sci Rep (2021) 11:17791. doi: 10.1038/s41598-021-96983-4
116. Yaghoubi N, Youssefi M, Jabbari Azad F, Farzad F, Yavari Z, Zahedi Avval F. Total Antioxidant Capacity as a Marker of Severity of COVID-19 Infection: Possible Prognostic and Therapeutic Clinical Application. J Med Virol (2021) 69:jmv.27500. doi: 10.1002/jmv.27500
117. Outh R, Boutin C, Gueudet P, Suzuki M, Saada M, Aumaître H. Eosinopenia <100/μl as a Marker of Active COVID-19: An Observational Prospective Study. J Microbiol Immunol Infect (2021) 54:61–8. doi: 10.1016/j.jmii.2020.12.005
118. Agouridis AP, Pagkali A, Zintzaras E, Rizos EC, Ntzani EE. High-Density Lipoprotein Cholesterol: A Marker of COVID-19 Infection Severity? Atheroscler Plus (2021) 44:1–9. doi: 10.1016/j.athplu.2021.08.007
119. Tronko MD, Cherviakova SA, Pushkarev VV, Belchina YB, Kovzun OI, Pushkarev VM, et al. Apolipoprotein A1 Level in Plasma of Patients With Diabetes and Diabetic Patients With COVID-19 as a Possible Marker of Disease. Rep Natl Acad Sci Ukr (2021) 2021:110–3. doi: 10.15407/dopovidi2021.04.110
120. Rowlands M, Segal F, Hartl D. Myeloid-Derived Suppressor Cells as a Potential Biomarker and Therapeutic Target in COVID-19. Front Immunol (2021) 12:697405:697405. doi: 10.3389/fimmu.2021.697405
121. Laing AG, Lorenc A, del Molino del Barrio I, Das A, Fish M, Monin L, et al. A Dynamic COVID-19 Immune Signature Includes Associations With Poor Prognosis. Nat Med (2020) 26:1623–35. doi: 10.1038/s41591-020-1038-6
122. Li Y, Zhao K, Wei H, Chen W, Wang W, Jia L, et al. Dynamic Relationship Between D-Dimer and COVID-19 Severity. Br J Haematol (2020) 190:e1–38. doi: 10.1111/bjh.16811
123. Liu F, Li L, Xu M, Wu J, Luo D, Zhu Y, et al. Prognostic Value of Interleukin-6, C-Reactive Protein, and Procalcitonin in Patients With COVID-19. J Clin Virol (2020) 127:104370. doi: 10.1016/j.jcv.2020.104370
124. Chiang C-C, Korinek M, Cheng W-J, Hwang T-L. Targeting Neutrophils to Treat Acute Respiratory Distress Syndrome in Coronavirus Disease. Front Pharmacol (2020) 11:572009:572009. doi: 10.3389/fphar.2020.572009
125. Tongu M, Harashima N, Monma H, Inao T, Yamada T, Kawauchi H, et al. Metronomic Chemotherapy With Low-Dose Cyclophosphamide Plus Gemcitabine can Induce Anti-Tumor T Cell Immunity In Vivo. Cancer Immunol Immunother (2013) 62:383–91. doi: 10.1007/s00262-012-1343-0
126. Bunt SK, Mohr AM, Bailey JM, Grandgenett PM, Hollingsworth MA. Rosiglitazone and Gemcitabine in Combination Reduces Immune Suppression and Modulates T Cell Populations in Pancreatic Cancer. Cancer Immunol Immunother (2013) 62:225–36. doi: 10.1007/s00262-012-1324-3
127. Hong E-H, Chang S-Y, Lee B-R, Pyun A-R, Kim J-W, Kweon M-N, et al. Intratumoral Injection of Attenuated Salmonella Vaccine can Induce Tumor Microenvironmental Shift From Immune Suppressive to Immunogenic. Vaccine (2013) 31:1377–84. doi: 10.1016/j.vaccine.2013.01.006
128. Fultang L, Panetti S, Ng M, Collins P, Graef S, Rizkalla N, et al. MDSC Targeting With Gemtuzumab Ozogamicin Restores T Cell Immunity and Immunotherapy Against Cancers. EBioMedicine (2019) 47:235–46. doi: 10.1016/j.ebiom.2019.08.025
129. Pawelec G, Verschoor CP, Ostrand-Rosenberg S. Myeloid-Derived Suppressor Cells: Not Only in Tumor Immunity. Front Immunol (2019) 10:1099:1099. doi: 10.3389/fimmu.2019.01099
130. Nefedova Y, Fishman M, Sherman S, Wang X, Beg AA, Gabrilovich DI. Mechanism of All-Trans Retinoic Acid Effect on Tumor-Associated Myeloid-Derived Suppressor Cells. Cancer Res (2007) 67:11021–8. doi: 10.1158/0008-5472.CAN-07-2593
131. Chen P-T, Hsieh C-C, Wu C-T, Yen T-C, Lin P-Y, Chen W-C, et al. 1α,25-Dihydroxyvitamin D3 Inhibits Esophageal Squamous Cell Carcinoma Progression by Reducing IL6 Signaling. Mol Cancer Ther (2015) 14:1365–75. doi: 10.1158/1535-7163.MCT-14-0952
132. Shirota Y, Shirota H, Klinman DM. Intratumoral Injection of CpG Oligonucleotides Induces the Differentiation and Reduces the Immunosuppressive Activity of Myeloid-Derived Suppressor Cells. J Immunol Baltim Md 1950 (2012) 188:1592–9. doi: 10.4049/jimmunol.1101304
133. Michels T, Shurin GV, Naiditch H, Sevko A, Umansky V, Shurin MR. Paclitaxel Promotes Differentiation of Myeloid-Derived Suppressor Cells Into Dendritic Cells In Vitro in a TLR4-Independent Manner. J Immunotoxicol (2012) 9:292–300. doi: 10.3109/1547691X.2011.642418
134. Daurkin I, Eruslanov E, Vieweg J, Kusmartsev S. Generation of Antigen-Presenting Cells From Tumor-Infiltrated CD11b Myeloid Cells With DNA Demethylating Agent 5-Aza-2′-Deoxycytidine. Cancer Immunol Immunother (2010) 59:697–706. doi: 10.1007/s00262-009-0786-4
135. Wang H, Li W, Guo R, Sun J, Cui J, Wang G, et al. An Intragenic Long Noncoding RNA Interacts Epigenetically With the RUNX1 Promoter and Enhancer Chromatin DNA in Hematopoietic Malignancies: RUNX1 Intragenic Noncoding RNA. Int J Cancer (2014) 135:2783–94. doi: 10.1002/ijc.28922
136. Mabuchi S, Sasano T, Komura N. Targeting Myeloid-Derived Suppressor Cells in Ovarian Cancer. Cells (2021) 10:329. doi: 10.3390/cells10020329
137. Sivak-Sears NR, Schwartzbaum JA, Miike R, Moghadassi M, Wrensch M. Case-Control Study of Use of Nonsteroidal Antiinflammatory Drugs and Glioblastoma Multiforme. Am J Epidemiol (2004) 159:1131–9. doi: 10.1093/aje/kwh153
138. Sandler RS, Halabi S, Baron JA, Budinger S, Paskett E, Keresztes R, et al. A Randomized Trial of Aspirin to Prevent Colorectal Adenomas in Patients With Previous Colorectal Cancer. N Engl J Med (2003) 348:883–90. doi: 10.1056/NEJMoa021633
139. Fujita M, Kohanbash G, Fellows-Mayle W, Hamilton RL, Komohara Y, Decker SA, et al. COX-2 Blockade Suppresses Gliomagenesis by Inhibiting Myeloid-Derived Suppressor Cells. Cancer Res (2011) 71:2664–74. doi: 10.1158/0008-5472.CAN-10-3055
140. Serafini P, Meckel K, Kelso M, Noonan K, Califano J, Koch W, et al. Phosphodiesterase-5 Inhibition Augments Endogenous Antitumor Immunity by Reducing Myeloid-Derived Suppressor Cell Function. J Exp Med (2006) 203:2691–702. doi: 10.1084/jem.20061104
141. Lin S, Wang J, Wang L, Wen J, Guo Y, Qiao W, et al. Phosphodiesterase-5 Inhibition Suppresses Colonic Inflammation-Induced Tumorigenesis via Blocking the Recruitment of MDSC. Am J Cancer Res (2017) 7:41–52 https://pubmed.ncbi.nlm.nih.gov/28123846/.
142. Yu SJ, Ma C, Heinrich B, Brown ZJ, Sandhu M, Zhang Q, et al. Targeting the Crosstalk Between Cytokine-Induced Killer Cells and Myeloid-Derived Suppressor Cells in Hepatocellular Carcinoma. J Hepatol (2019) 70:449–57. doi: 10.1016/j.jhep.2018.10.040
143. Stadtmann A, Zarbock A. CXCR2: From Bench to Bedside. Front Immunol (2012) 3:263. doi: 10.3389/fimmu.2012.00263
144. Lu Z, Zou J, Li S, Topper MJ, Tao Y, Zhang H, et al. Epigenetic Therapy Inhibits Metastases by Disrupting Premetastatic Niches. Nature (2020) 579:284–90. doi: 10.1038/s41586-020-2054-x
145. Highfill SL, Cui Y, Giles AJ, Smith JP, Zhang H, Morse E, et al. Disruption of CXCR2-Mediated MDSC Tumor Trafficking Enhances Anti-PD1 Efficacy. Sci Transl Med (2014) 6:237ra67. doi: 10.1126/scitranslmed.3007974
146. Gabrilovich DI, Ostrand-Rosenberg S, Bronte V. Coordinated Regulation of Myeloid Cells by Tumours. Nat Rev Immunol (2012) 12:253–68. doi: 10.1038/nri3175
147. Najjar YG, Finke JH. Clinical Perspectives on Targeting of Myeloid Derived Suppressor Cells in the Treatment of Cancer. Front Oncol (2013) 3:49. doi: 10.3389/fonc.2013.00049
148. Farshidpour M, Ahmed M, Junna S, Merchant JL. Myeloid-Derived Suppressor Cells in Gastrointestinal Cancers: A Systemic Review. World J Gastrointest Oncol (2021) 13:1–11. doi: 10.4251/wjgo.v13.i1.1
149. Groth C, Weber R, Utikal J, Umansky V. Depletion and Maturation of Myeloid-Derived Suppressor Cells in Murine Cancer Models. In: Brandau S, Dorhoi A, editors. Myeloid-Derived Suppressor Cells. Methods in Molecular Biology. New York, NY: Springer US (2021). p. 67–75. doi: 10.1007/978-1-0716-1060-2_7
150. Tobin RP, Jordan KR, Kapoor P, Spongberg E, Davis D, Vorwald VM, et al. IL-6 and IL-8 Are Linked With Myeloid-Derived Suppressor Cell Accumulation and Correlate With Poor Clinical Outcomes in Melanoma Patients. Front Oncol (2019) 9:1223:1223. doi: 10.3389/fonc.2019.01223
151. Guo Q, Zhao Y, Li J, Liu J, Yang X, Guo X, et al. Induction of Alarmin S100A8/A9 Mediates Activation of Aberrant Neutrophils in the Pathogenesis of COVID-19. Cell Host Microbe (2021) 29:222–35.e4. doi: 10.1016/j.chom.2020.12.016
152. Diaz-Montero CM, Finke J, Montero AJ. Myeloid-Derived Suppressor Cells in Cancer: Therapeutic, Predictive, and Prognostic Implications. Semin Oncol (2014) 41:174–84. doi: 10.1053/j.seminoncol.2014.02.003
153. Gupta A, Chander Chiang K. Prostaglandin D2 as a Mediator of Lymphopenia and a Therapeutic Target in COVID-19 Disease. Med Hypotheses (2020) 143:110122. doi: 10.1016/j.mehy.2020.110122
154. Divya R, Raj Kumar K. Tadalafil Inhibits Elevated Glutamic Oxaloacetic Transaminase During Alcohol Aflatoxin Induced Hepatocellular Carcinoma in Rats. Int J Immunother Cancer Res (2020) 6:010–3. doi: 10.17352/2455-8591.000022
155. Mann ER, Menon M, Knight SB, Konkel JE, Jagger C, Shaw TN, et al. Longitudinal Immune Profiling Reveals Key Myeloid Signatures Associated With COVID-19. Sci Immunol (2020) 5:eabd6197. doi: 10.1126/sciimmunol.abd6197
156. Patterson BK, Seethamraju H, Dhody K, Corley MJ, Kazempour K, Lalezari J, et al. Disruption of the CCL5/RANTES-CCR5 Pathway Restores Immune Homeostasis and Reduces Plasma Viral Load in Critical COVID-19. Infect Dis (Except HIV/AIDS) (2020). doi: 10.1101/2020.05.02.20084673
157. Dean MJ, Ochoa JB, Sanchez-Pino M, Zabaleta J, Garai J, Del Valle L, et al. Transcriptome and Functions of Granulocytic Myeloid-Derived Suppressor Cells Determine Their Association With Disease Severity of COVID-19. Allergy Immunol (2021). doi: 10.1101/2021.03.26.21254441
Keywords: MDSC, COVID-19, immune regulation, biomarkers, therapeutics
Citation: Park S-J, Nam D-e, Seong HC and Hahn YS (2022) New Discovery of Myeloid-Derived Suppressor Cell’s Tale on Viral Infection and COVID-19. Front. Immunol. 13:842535. doi: 10.3389/fimmu.2022.842535
Received: 23 December 2021; Accepted: 17 January 2022;
Published: 03 February 2022.
Edited by:
Nelita Du Plessis, Stellenbosch University, South AfricaReviewed by:
Ciputra Hartana, Ragon Institute, United StatesJie Zhou, Tianjin Medical University, China
Copyright © 2022 Park, Nam, Seong and Hahn. This is an open-access article distributed under the terms of the Creative Commons Attribution License (CC BY). The use, distribution or reproduction in other forums is permitted, provided the original author(s) and the copyright owner(s) are credited and that the original publication in this journal is cited, in accordance with accepted academic practice. No use, distribution or reproduction is permitted which does not comply with these terms.
*Correspondence: Young S. Hahn, eXNoNWVAdmlyZ2luaWEuZWR1