- 1Cell Biology and Immunology, Wageningen University and Research, Wageningen, Netherlands
- 2School of Human Development and Health, Faculty of Medicine, University of Southampton, Southampton, United Kingdom
- 3National Institute for Health Research (NIHR) Southampton Biomedical Research Centre, University Hospital Southampton National Health Service (NHS) Foundation Trust and University of Southampton, Southampton, United Kingdom
- 4Nutrileads BV, Wageningen, Netherlands
- 5Research & Development, FrieslandCampina, Amersfoort, Netherlands
Respiratory infections place a heavy burden on the health care system, particularly in the winter months. Individuals with a vulnerable immune system, such as very young children and the elderly, and those with an immune deficiency, are at increased risk of contracting a respiratory infection. Most respiratory infections are relatively mild and affect the upper respiratory tract only, but other infections can be more serious. These can lead to pneumonia and be life-threatening in vulnerable groups. Rather than focus entirely on treating the symptoms of infectious disease, optimizing immune responsiveness to the pathogens causing these infections may help steer towards a more favorable outcome. Nutrition may have a role in such prevention through different immune supporting mechanisms. Nutrition contributes to the normal functioning of the immune system, with various nutrients acting as energy sources and building blocks during the immune response. Many micronutrients (vitamins and minerals) act as regulators of molecular responses of immune cells to infection. It is well described that chronic undernutrition as well as specific micronutrient deficiencies impair many aspects of the immune response and make individuals more susceptible to infectious diseases, especially in the respiratory and gastrointestinal tracts. In addition, other dietary components such as proteins, pre-, pro- and synbiotics, and also animal- and plant-derived bioactive components can further support the immune system. Both the innate and adaptive defense systems contribute to active antiviral respiratory tract immunity. The initial response to viral airway infections is through recognition by the innate immune system of viral components leading to activation of adaptive immune cells in the form of cytotoxic T cells, the production of neutralizing antibodies and the induction of memory T and B cell responses. The aim of this review is to describe the effects of a range different dietary components on anti-infective innate as well as adaptive immune responses and to propose mechanisms by which they may interact with the immune system in the respiratory tract.
Introduction
Due to the increasing population density and the worldwide trend of urbanization, people are living closer together, thus providing a favorable environment for transmission of respiratory viruses and other infective organisms. Contacts with animals such as chickens and pigs, but also with wild animals, increase the risk of pandemic zoonotic disease outbreaks. Examples include the 2009 swine influenza pandemic and the current severe acute respiratory syndrome coronavirus 2 (SARS-CoV-2) pandemic. COVID-19, caused by SARS-CoV-2, is a reminder of the impact that respiratory viral infections can have on human health and wider society.
Respiratory infections are common in the elderly and in very young children and place a heavy burden on the health care system, especially in the winter months. In addition to the direct impact of respiratory infections, there are causal links between respiratory infections and the development and worsening of chronic respiratory diseases such as asthma in children (1–3), long COVID in children and adults (4–6), COVID-19 induced pulmonary fibrosis in adults and the elderly (7), and chronic obstructive pulmonary disease (COPD) in the elderly (8).
It is therefore highly relevant from a societal perspective, in addition to developing effective vaccines and new antiviral treatments, to understand more about possible preventive, lifestyle-related approaches, including those related to nutrition. Therefore, it is important to understand the possible role that nutrition can play in reducing the occurrence and in dampening the severity of these infections. This role has been largely neglected to date, even though there are indications that nutrition in general, and specific nutrients and other food components in particular, may improve resistance against viral infections. In this review we will describe which population subgroups are susceptible to respiratory infections, which immunological mechanisms contribute to antiviral immunity, how nutritional components can modulate antiviral immune responses and infections, and finally we will propose how these components that are ingested through the gastrointestinal tract can have effects on immunity in the respiratory tract.
Immune Vulnerability: The Young and Old
Both young children (0-4 years) and the elderly (> 65 years) are more susceptible to respiratory and gastrointestinal infections than older children and young and middle aged adults (9, 10) as their immune system is less effective (11). The underlying reasons for this differ between young children and the elderly. In young children, lower immune responsiveness results from a less matured adaptive immune system, especially in the first 2-3 years of life, which cannot always respond well to external stimuli. Moreover, the type of adaptive immune response in early life is more focused on the prevention of inflammation, as a result of which Th1 immunity and pro-inflammatory responses are not yet well developed [(11, 12) and Figure 1]. The innate immune system, however, is already developed in young children. Pan-pathogen receptors such as toll-like receptors (TLRs) and other pattern recognition receptors (PRRs) are present soon after birth, enabling recognition and responses by innate immune cells in the form of antiviral cytokine production after infection (11, 12). Protection of infants against infection is further provided by transplacental transport of immunoglobulins during pregnancy, and by breastfeeding after birth. In addition to immunoglobulins, breastmilk contains a wide range of immune-related components including micronutrients, oligosaccharides, antimicrobial proteins, and immunoregulatory cytokines that support immune maturation and regulation (13–15), as well as the development of the microbiota in early life [(16, 17) and Figure 1].
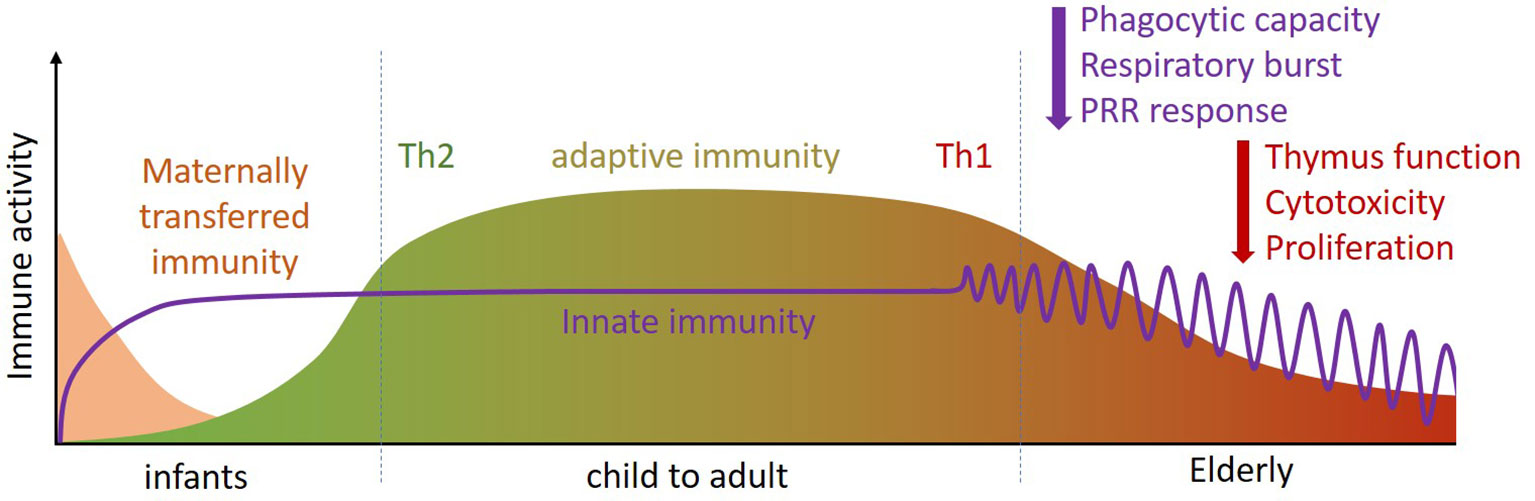
Figure 1 Throughout the life course (infant, child to adult, elderly) the immune system changes in composition and activity. Innate immunity is already present at birth and quickly matures (purple line). For adaptive immune defense infants rely on placental transfer and mothers’ milk for their first period (pink section). During development, the adaptive immune system phenotype changes from Th2 dominant [i.e. tolerant (green)] to Th1 dominant [i.e. inflammatory (red)]. At later age, specific functions of innate and adaptive immunity become less efficient (purple and red arrows). Th, T helper.
The reduced immune responsiveness/efficacy in the elderly, termed immune senescence, is characterized in particular by a less flexible adaptive immune response [(18–22) and Figure 1]. One contributor to immune senescence is likely to be the decreased output of immune cells from bone marrow, the site of origin of all immune cells, with increasing age. Furthermore, involution of the thymus with age decreases output of naive T cells, resulting in reduced capacity to respond to new antigens. In addition to altered numbers of immune cells in the circulation, their function is often impaired in older people. For example, neutrophils show impaired phagocytosis, respiratory burst and bacterial killing; natural killer (NK) cells have impaired cytotoxicity towards virally-infected and tumor cells; dendritic cells (DCs) have impaired responsiveness to immune signals. T cells have reduced ability to proliferate and to produce important cytokines like interleukin (IL)-2 and interferon (IFN)-γ; Cytotoxic T cell activity is reduced and antibody production by B cells is altered. Hence, older people can show a broad range of immune impairments, making them more susceptible to infectious diseases. This includes respiratory illnesses caused by viruses. Immune senescence also impairs responses to vaccination, including to the seasonal influenza vaccine (23, 24).
The reduced adaptive immune response to vaccination and to viral infection in the elderly may resemble the reduced adaptive immune response in young children, but it has a different cause. In infants and toddlers up to 2-3 years of age, T-cell responses and the induction of specific antibodies are reduced because of immaturity of the immune system; in elderly, there is a bias towards generation of memory T cells, and the ability to mount naive T cell responses to new antigens, as well as vaccines, is reduced.
Whereas even at a few weeks of age, infant already have sufficient TLR expression and responsiveness to TLR ligands like single stranded RNA (11) which induce IFN-α, the elderly have a much decreased IFN-α response to influenza viruses (25). This has been shown in particular for TLR7 and TLR8, the receptors that are important in protecting against single stranded RNA viruses, to which most respiratory viruses belong. For example, in older women, the plasmacytoid dendritic cell (pDC) response to TLR7 stimulation with R848 is notably decreased (26). In this context it is interesting to note that in COVID-19 patients with a severe clinical picture, two different TLR7 variants were found to be associated with reduced antiviral function (27).
The immune vulnerability in the very young and the elderly thus illustrates the need for supporting antiviral immune function, for example by nutritional intervention. But let’s first discuss the mechanisms of antiviral immunity.
Mechanisms of Antiviral Immunity
The human immune system comprises an innate and adaptive component. The innate, or inborn, component is rapid (responds minutes to hours after infection), reacts to a large variety of pathogens and is important in the primary protection against infection, but is also limited in effectiveness. The adaptive immune system on the other hand is slow to respond (days to weeks), but highly specific and effective. In fact, the adaptive system is activated by its innate counterpart via the antigen presenting capacity of monocytes, macrophages and DCs.
Both the innate and adaptive defense systems contribute to active antiviral immunity. The initial response against viral airway infections is through sensing of viral replication in infected host cells by cells of the innate immune system via PRRs such as TLR7/8 and RIG-I. PRRs recognize repetitive evolutionarily conserved structures on potential pathogens, such as bacterial cell wall components (lipopolysaccharides, peptidoglycans) and sugars, and also intracellular components of pathogens such as DNA and RNA. The best studied PRRs are the TLRs, of which TLR7 and TLR8 are particularly important against single stranded RNA viruses, such as influenza virus, respiratory syncytial virus (RSV), rhinovirus and SARS-CoV-2. They recognize single stranded RNA that is not found in endosomes under normal metabolic activities of human cells. The recognition of pathogenic structures such as single stranded viral RNA by TLR7 and TLR8 results in induction of anti-viral type I and type III interferons that have a direct inhibitory effect on viral replication. In addition, these interferons (α, β and λ) cause nearby cells to heighten their anti-viral defenses, inducing an antiviral state. The innate defense system includes monocytes, myeloid and plasmacytoid DCs (mDCs and pDCs), macrophages, NK and natural killer T (NKT) cells, and neutrophils, eosinophils, and basophils. Especially the pDCs are high producers of type I interferons that play a key role in antiviral immunity (28–30) to shape the local immunological environment in order to eliminate viruses.
In addition to a first broad response and activation of the adaptive immune system, specific components of the innate immune system can also directly target respiratory pathogens. For example, NKT cells play a direct role in antiviral immunity (31–33). They have receptors that can recognize lipid when presented on major histocompatibility complex (MHC)-like molecules such as CD1c on innate immune cells. In addition, iNKT cells can inhibit the influenza A-induced immunosuppressive activity of myeloid suppressor cells (34) and iNKT activation can shorten influenza infection (35). The finding that milk contains lipids known as ligands for NKT cells (36) may indicate that the milk lipid fraction also plays a role in antiviral immunity, as well as in intestinal homeostasis as NKT cells also recognize lipids from the intestinal microbiota (37, 38). Plant lipids can also activate NKT cells (39).
Respiratory and gastrointestinal epithelial cells - which together with mucus form a first physical barrier against infection - can also contribute to antiviral immunity. Epithelial cells express TLRs and other PRRs such as RIG I and recognize viral components. In response, both intestinal epithelial and respiratory epithelial cells can produce antiviral IFNs (40–42).
The adaptive defense system consists of the B lymphocytes, which can produce antibodies against viruses, and both the CD4+ and CD8+ T lymphocytes, which can recognize protein fragments of the virus presented by MHC molecules on innate immune cells. This adaptive immune response is crucial in protecting against re-infection with the same pathogen through its long-lasting immunological memory. However, the adaptive immune response starts slowly and it takes a few days to weeks after infection before antibodies become detectable. This results from the process in which innate immune cells such as macrophages or DCs move from the site of infection to nearby specialized immunological compartments such as lymph nodes or the spleen. Monocytes, macrophages and DCs collect pathogenic peptide fragments and present those on MHC molecules - or human leukocyte antigens (HLA) in humans - to T cells that specifically recognize the MHC-peptide complex, resulting in T cell activation and instruction of antigen-specific B cells. After activation, B cells and T cells mature and proliferate (clonal expansion) before they can either start producing antibodies or migrate to the site of infection to kill infected host cells, respectively. Maintenance of virus-specific T and B cells provides a memory giving protection against reinfection. This is the basic principle underlying pathogen-specific protection by adaptive memory induced by vaccination.
Passive immunity against virus infections can be provided by antiviral antibodies already circulating in the blood that can neutralize the virus, but there are also small molecules such as complex sugars (oligosaccharides) and oligosaccharide-containing sphingolipids that can also exert such a neutralization role (43–46).
Another way the innate immune system confers protection against infections has been revealed through vaccination with BCG (tuberculosis vaccine). Surprisingly, BCG vaccination was found to also result in better protection against other pathogens (47, 48). Although this can be partly explained by cross-reacting antibodies which can bind to more than one pathogen, it has recently been shown that the innate immune system also has a certain degree of memory. This so-called “innate immune memory” or “trained immunity” appears to result from epigenetic modifications in the DNA conferring innate immune system cells with the ability to respond more strongly to stimulation by pathogens via TLRs and exhibiting some degree of cross-protection (49–52). It has recently been postulated that “trained immunity” may also protect against viral respiratory infections such as COVID-19 (53), and this concept is currently being investigated in multiple studies (54).
Nutrition and the Immune System
Foods and beverages provide macro-and micronutrients, as well as other bioactive components, that contribute to the normal functioning of the immune system, including supporting barrier function. The impact of several food components such as specific vitamins and minerals, and also proteins, flavonoids, and non-digestible polysaccharides, as well as probiotics and short-chain fatty acids, on the immune system has recently been comprehensively reviewed (55, 56). These effects are relevant to anti-viral immunity (57).
Food components act in a variety of ways to influence immune responsiveness against acute upper respiratory tract infections by viruses:
● Macronutrients act as fuels for energy generation by immune cells;
● Macronutrients provide substrate (“building blocks”) for the biosynthesis that is involved in the immune response (e.g. amino acids for immunoglobulins, cytokines, new receptors, acute phase proteins);
● Many micronutrients are regulators of molecular and cellular aspects of the immune response (e.g. iron, zinc, vitamin A, vitamin D);
● Some nutrients are substrates for the synthesis of chemicals involved in the immune response (e.g. arginine and nitric oxide; arachidonic acid and eicosanoids);
● Some micronutrients have specific anti-infection roles (e.g. zinc, vitamin D);
● Some proteins play a direct role in pathogen clearance and immune function (e.g. breast milk proteins like IgG, lactoferrin, and lysozyme);
● Many nutrients and bioactives are involved in protection of the host from the oxidative and inflammatory stress imposed by the immune response (e.g. vitamin C, vitamin E, cysteine, zinc, copper, selenium, flavonoids);
● Many food components contribute to creating a diverse microbiota that supports the immune response (e.g. plant-derived fibers and non-digestible polysaccharides, prebiotic oligosaccharides and human milk oligosaccharides);
● Probiotic bacteria that produce metabolites like short chain fatty acids (SCFAs) and some vitamins, support barrier function and contribute to immune support;
● Some food components act as aryl hydrocarbon receptor ligands, acting to strengthen barrier function (e.g. quercetin).
These considerations suggest multiple sites of interaction of food components with the immune system. Firstly, absorbed food components can act systemically to target the different components of the immune system (e.g. in bone marrow, the thymus, the bloodstream, secondary lymphoid organs, and other organs). Secondly, multiple food components can act to influence the immune system without being absorbed systemically. For example, they could have local actions on epithelial barrier function or on the gut-associated lymphoid system, they could modulate the gut microbiota composition influencing gut microbiota-immune system cross-talk, they could be fermented by the microbiota resulting in metabolites (e.g. short chain fatty acids (SCFAs)) which can act locally on epithelial and immune cells or be absorbed and act systemically, or they could train or prime immune cells involved in surveillance of the luminal contents of the gastrointestinal tract. Although these latter actions are primarily focused on the gastrointestinal tract, because of recirculation of cells from the gut wall to the respiratory tract, effects initiated at the gut level can be enacted at the level of the airways.
Micronutrients: Support of Normal Immune Function and Relevance for Respiratory Infection
Multiple micronutrients (vitamins and minerals) play several roles in supporting the immune system (Table 1), as comprehensively reviewed elsewhere (56, 59–61). People with low intakes or status of these micronutrients show immune impairments and increased susceptibility to infectious disease, especially respiratory and gastrointestinal (56, 60). Amongst the micronutrients, the roles of vitamins A, C and D and the minerals zinc, copper and iron are well explored, but B vitamins, vitamin E, vitamin K, selenium, magnesium and others all have roles.
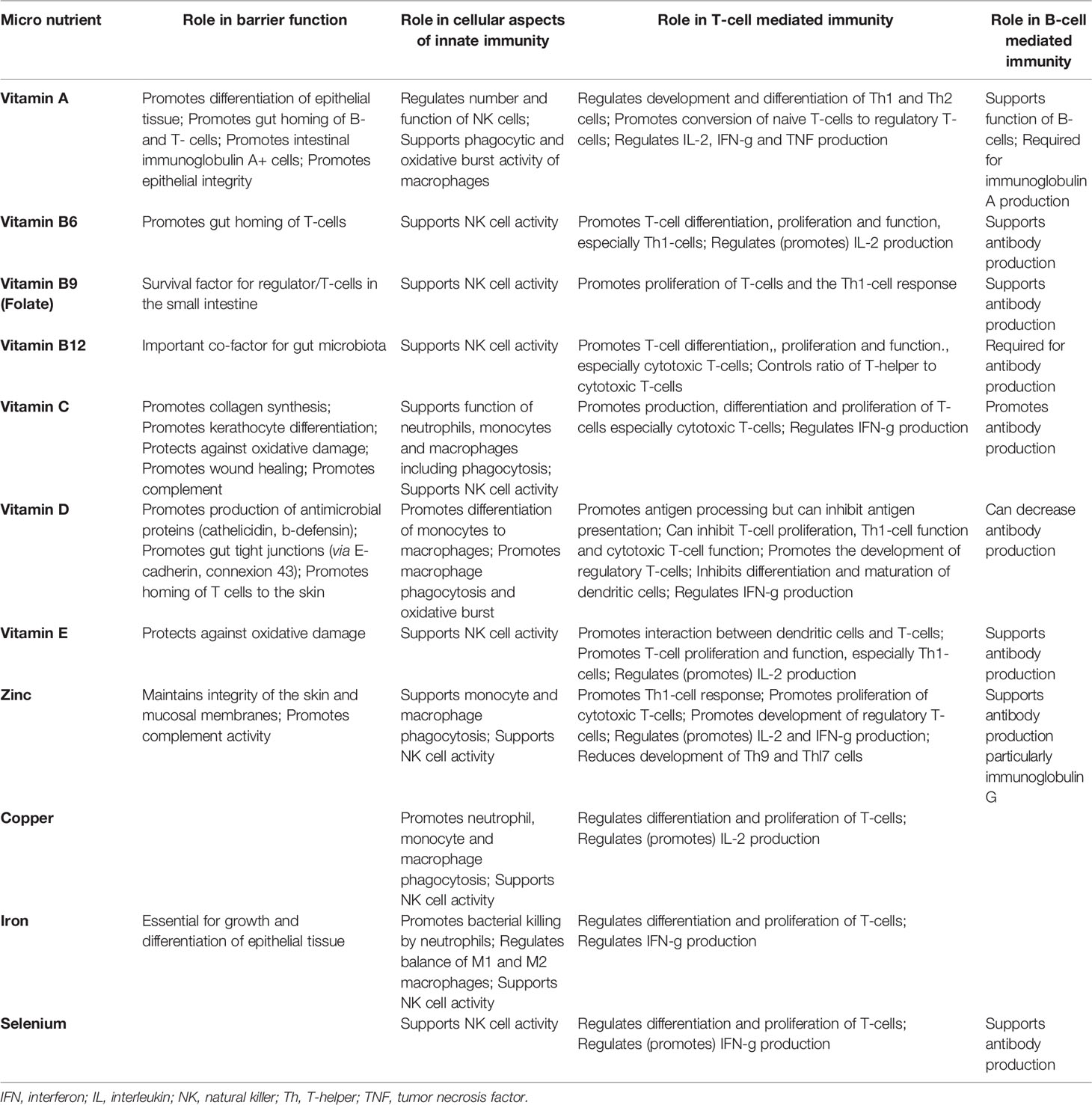
Table 1 Taken from (58). Summary of the effects of various micronutrients on different aspects of immunity.
Vitamin A and its metabolites (e.g. retinoic acid) are important for normal differentiation of epithelial tissue and for immune cell maturation and function (62–69). Vitamin A is key to an effective barrier function and it regulates many aspects of innate immunity including neutrophil maturation and function and NK cell activity, thus contributing to defense against viruses including respiratory viruses (Figure 2). With regard to acquired immunity, vitamin A controls DC and CD4+ T lymphocyte maturation and promotes Th1 type responses that are involved in antibacterial and anti-viral defenses. The vitamin A metabolite retinoic acid promotes homing of T lymphocytes to the gut-associated lymphoid tissue by upregulating surface homing molecules. In this context it is interesting to note that some gut-associated immune cells are able to synthesise retinoic acid. Retinoic acid is required for CD8+ T lymphocyte survival and proliferation and the normal functioning of B lymphocytes including antibody generation, including IgA. Thus vitamin A deficiency is associated with increased susceptibility to many infections, especially in children, including respiratory infections, diarrhea and severe measles (70, 71). Vitamin A supplementation can improve symptoms in patients with acute pneumonia (69). A meta-analysis of 15 randomized controlled trials in children with pneumonia identified that vitamin A decreased pneumonia morbidity, increased the clinical response rate, shortened clearance time of signs and shortened length of hospital stay, although it did not affect mortality (72).
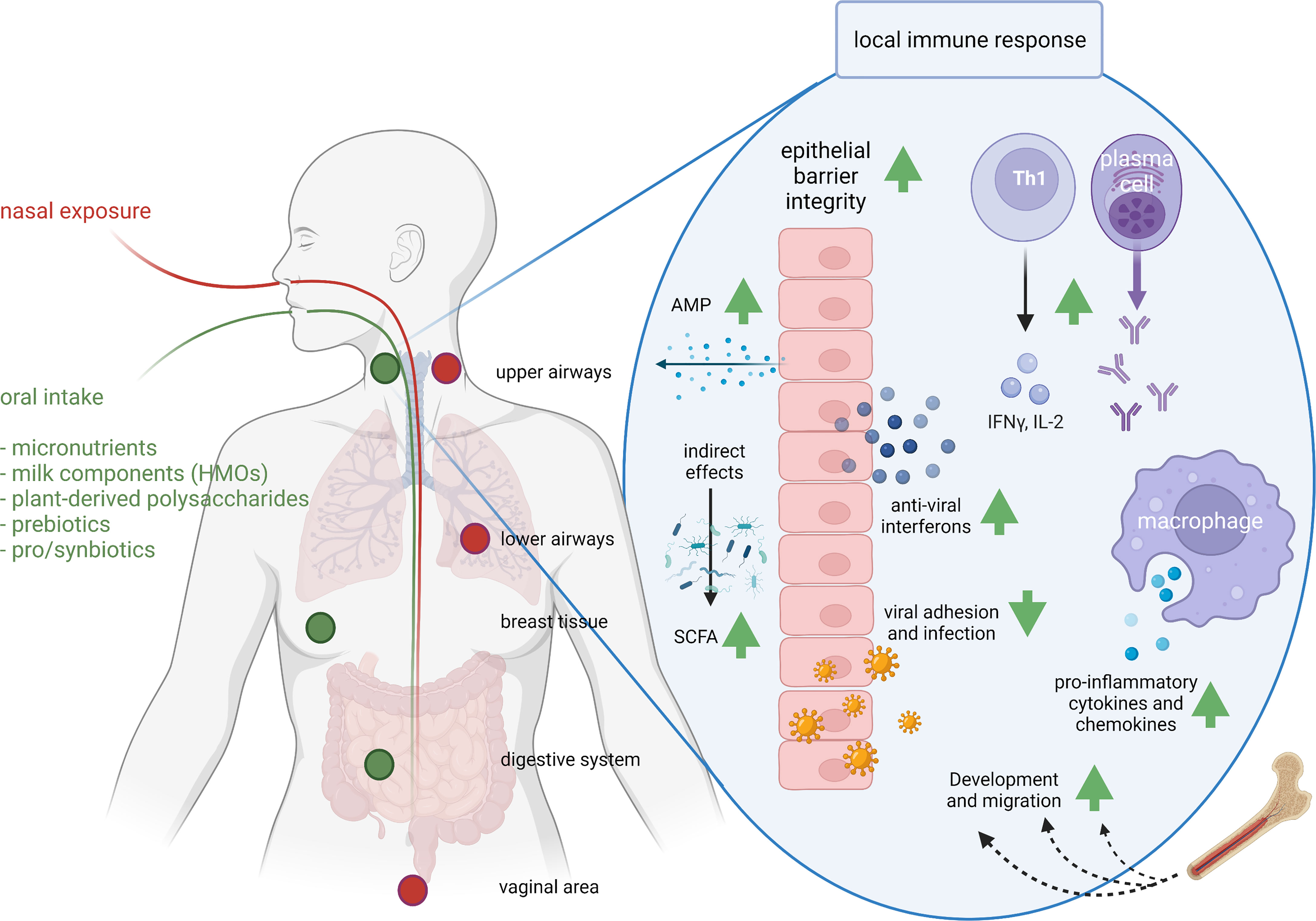
Figure 2 Exposure routes and potential concerted immunomodulatory effects of food components. The route of administration determines the sites of immunomodulation. As a result of nasal exposure (the route that respiratory pathogens follow) pathogens or intranasal vaccines lead to IgA production in upper and lower airways and the vaginal area, and oral exposure leads to IgA production in the upper airways, breast and digestive system. Clinical studies have demonstrated support of respiratory immunity by many food components, whereas preclinical, ex vivo and in vitro studies identified possible mechanisms. For instance, milk-derived HMOs, pre- and probiotics modify the microbiome and SCFA production; vitamins A, C and D improve epithelial barrier integrity; vitamin D induces anti-microbial protein (AMP) secretion and acetate anti-viral interferon secretion in epithelial cells; viral adhesion and infection is reduced by zinc, bIgG, lactoferrin or HMOs; iron supports immune cell development and migration; bIgG and NDPs induce innate immune training and as a results increased cytokine and chemokine production; zinc and vitamin C support Th1 activity and IFN-γ and IL-2 release; and selenium and vitamin C support antibody production. AMP, anti-microbial peptide; HMO, human milk oligosaccharides; IFN-γ, interferon gamma; IL-2, interleukin-2; SCFA, short chain fatty acid. This figure was created with BioRender.com.
Vitamin C is required for collagen biosynthesis and is vital for maintaining epithelial integrity (73, 74) (Figure 2). It also has roles in several aspects of immunity, including supporting leucocyte migration to sites of infection, phagocytosis and bacterial killing, scavenging reactive oxygen species (ROS), NK cell activity, T lymphocyte function and IFN-γ production (especially by CD8+ cytotoxic T lymphocytes) and antibody production (73, 74) (Figure 2). People that are deficient in vitamin C are susceptible to severe viral respiratory infections such as pneumonia and acute respiratory disease syndrome (ARDS) (73, 74). Trials of vitamin C supplementation report reduced risk of pneumonia, with some trials reporting decreased severity and mortality (75). A meta-analysis of trials of vitamin C and common cold infections found that it decreased the incidence in those individuals under physical stress and decreased the duration and severity (76).
Vitamin D receptors have been identified in most immune cells and, besides the occurrence of genetic polymorphisms in the vitamin D receptor gene, vitamin D has pleiotropic actions within the immune system supporting the activity of several cell types (77, 78). Furthermore, some immune cells (DCs, macrophages) can produce the active form of vitamin D suggesting it is important to immunity. Vitamin D enhances epithelial integrity and induces synthesis of antimicrobial peptides (e.g., cathelicidin) in epithelial cells and macrophages (79, 80) (Figure 2). Vitamin D promotes homing of immune cells to the respiratory tract (81–84). On a functional level vitamin D promotes differentiation of monocytes to macrophages and increases phagocytosis, superoxide production and bacterial killing by innate immune cells. It also promotes antigen processing by DCs although antigen presentation may be impaired. Thus, vitamin D has many actions which would support immunity against respiratory infections. Nevertheless, the effects of vitamin D on the cellular components of immunity are rather complex and paradoxical in nature as reviewed recently (85). Berry et al. described an inverse linear relationship between vitamin D levels and respiratory tract infections in a cross-sectional study of 6,789 British adults (86). In agreement with this, data from the US Third National Health and Nutrition Examination Survey which included 18,883 adults showed an independent inverse association between serum 25(OH)-vitamin D and recent upper respiratory tract infection (87). A meta-analysis of such observational studies identified that serum vitamin D is inversely associated with risk and severity of acute respiratory tract infections (88). There have been a large number of randomized controlled trials investigating the effect of vitamin D to reduce incidence or severity of respiratory tract infections and these have been subject to meta-analysis. A meta-analysis of 25 RCTs involving over 11,000 adults and children identified that vitamin D decreased the risk of acute respiratory tract infections, with greater effects being observed in those with low starting status (89).
Zinc supports the activity of many cells of the immune system, helps to control oxidative stress and inflammation and has specific anti-viral actions including inhibiting the replication of RNA viruses (90–92). Zinc-binding metallothioneins seem to play an important role in antiviral defense (93). Zinc is important in early immune cell development in the bone marrow and is important in maintaining T and B lymphocyte numbers. Zinc also supports the release of neutrophil extracellular traps that capture microbes. Zinc promotes CD4+ T lymphocyte numbers and function (e.g., IL-2 and IFN-γ production) and supports the proliferation of CD8+ cytotoxic T lymphocytes, key cells in antiviral defense (Figure 2). Zinc can disrupt the replication and infectivity of some respiratory viruses and prevents ARDS and lung damage in COVID-19 (94). Zinc supplementation has been reported to improve some markers of immunity especially in older people or those with low zinc intake (95). Recent systematic reviews and meta-analyses of trials with zinc report shorter duration of common cold in adults, reduced incidence and prevalence of pneumonia in children and reduced mortality when given to adults with severe pneumonia (96–98).
Iron is another micronutrient that is required by the immune system for proper functioning. Iron is important to maintain thymus function and the output of naive T lymphocytes and also to support neutrophil respiratory burst and bacterial killing, NK cell activity, T lymphocyte proliferation and production of T helper 1 cytokines (99–101) (Figure 2). These observations would suggest a clear case for iron deficiency increasing susceptibility to infection, which it does (102). However, many pathogens also have a high demand for iron and in an acute infection, host-driven iron removal inhibits the growth of pathogens (103–105). Chronic immune activation due to persistent infection, however, sequesters iron not only from infectious agents but also from erythroid progenitors, thereby causing anemia associated with chronic inflammation (106). Therefore, the relationship between iron availability, including deficiency, and susceptibility to infection remains complex (107–109). Evidence suggests that infections caused by organisms that spend part of their life-cycle intracellularly may actually be enhanced by iron, and in such situations providing iron may be harmful. Perhaps because of this harm from iron, several host immune mechanisms have developed for withholding iron from a pathogen (104, 105, 110).
Selenium has important roles in supporting the immune system in general (111, 112) and in promoting anti-viral immunity in particular (113–115). Selenium supports the activity of many cells of the immune system and helps to control oxidative stress and inflammation. Cellular entry of viruses, including SARS-CoV-2, into lung cells causes cellular oxidative stress resulting in the formation of free radicals (ROS) and subsequent disruption of the cellular membrane and lysis because of the budding process. Selenium could protect against such stress. Extensive research in mice has shown that selenium deficiency adversely affects several components of both innate and acquired immunity, including NK cell, T and B lymphocyte function and antibody production (Figure 2), increases susceptibility to viral infection, permits viruses (including coxsackievirus, polio virus and influenza viruses) to mutate, and allows normally weak viruses to become more virulent (116–118). A study in South Korea showed that selenium deficiency in peripheral blood was associated with a higher mortality in COVID-19 patients (119). Selenium supplementation improves some markers of immunity especially in older people or those with low selenium intake; for example a supplementation study conducted in UK adults with marginal selenium status showed that selenium improved ex vivo anti-viral immune responses, promoted polio virus clearance and decreased polio virus mutation (120). It seems likely that selenium is important in reducing risk and severity of viral respiratory infections.
Taken together, it is evident that multiple micronutrients play several roles in the immune system. Individuals with a deficiency in either one of these vitamins or minerals show immune impairments and increased susceptibility to infection. These impairments can be reversed by repletion of the deficient micronutrient. Each micronutrient has its own biochemical actions that result in its effect on the immune system; although the immunologic effect (e.g. improved Th1 cell function) can be shared by many micronutrients, the mechanism underpinning that effect is, generally speaking, unique to each particular micronutrient. In other words, the immune impairments that result from deficiencies in multiple micronutrients are unlikely to be reversed by repletion of only one of those micronutrients due to limited redundancy in function. In such a scenario a multiple micronutrient mixture is likely to be more effective in supporting (impaired) immune function than any single micronutrient.
Milk Components, Immune Function, and Respiratory Infection
Milk production has evolved in mammals to support growth and development in early life, as well as to provide protection against infections. Therefore, there are many components in breast milk that have direct anti-infective and immune modulatory actions or are important for supporting the immune system (121–131).
Breastfeeding
The first 1000 days of a child’s life are important for maturing the immune system, partly under the influence of the development of the gut microbiota. Breast milk contains many bioactive components that support the development and can regulate the immune system, as well as components that can have direct antibacterial and antiviral effects. It is therefore not surprising that breast-fed children have fewer infections than bottle-fed children (125, 132, 133). This has been reported for gastrointestinal infections, but also relates to (viral) respiratory tract infections, pneumonia and mortality (134–136).
Bovine Milk and Its Components
Even though human breastmilk contains many immune supporting components (125, 130), studies on breastfeeding cannot show exactly which components confer resistance to respiratory infections. Cow’s milk contains similar components, often in comparable concentrations (131), and apparently with comparable activity on the human immune system (61, 121, 123, 131). Epidemiological studies have seen an inverse association between the consumption of raw cow’s milk and asthma and respiratory infections (137–140) and have shown that it is heat-sensitive components of raw cow’s milk, likely proteins or peptides, that play an important role (141, 142). In addition, it is known that, like human breast milk, raw cow’s milk contains components that may have antiviral activity like IgG, lactoferrin and lactadherin (131, 143). These are not included in infant formulas in their active form due to structural alterations as a result of heating during processing. Thus, infants that are not breastfed or switch to formula at an early age are at increased risk of infection, in particular when introduced to new foods or an altered environmental exposure to microbes.
Epidemiological research has shown that the consumption of raw cow’s milk by young children is associated with a lower chance of developing asthma and hay fever (137–139, 141, 142, 144–146) and respiratory infections (140). These studies show that heat-sensitive components in cow’s milk - the proteins and peptides - play an important role in protecting against infections and allergies. In vitro research has established that milk proteins like IgG, lactoferrin, IL-10 and others can have effects on the human immune system (123, 124, 126, 127, 147–149). These components may contribute to antiviral immunity, but they are not well researched in this context. However, on a cautionary note, it should be stated that raw milk consumption also brings about risks of gastrointestinal infections and therefore consumption of raw milk is not recommended. Heating is applied to kill potential pathogens but also destroys some of the bioactive components of raw milk. Application of these components to support immune function is thus dependent on milk processing that ensures microbiological safety without disrupting their functionality.
Bovine IgG
Gamma immunoglobulin (IgG) antibodies from cow’s milk can bind to respiratory pathogens such as RSV and influenza virus (148), and also to many bacterial pathogens and to allergens (128). Moreover, cows can be infected by the bovine RSV and thereby induce an anti-RSV response; the IgG antibodies produced can prevent infection of human cells with human RSV in vitro (127), and prevent RSV infection in an animal model (147) (Figure 2).
The possible role of IgG in relation to other respiratory viruses is not well known. In addition to RSV, cows encounter respiratory coronaviruses (150–152). Cows therefore have coronavirus-specific antibodies, which may also be able to bind cross-reactively to SARS-CoV-2, as can IgA against SARS-CoV-2 from breast milk of infected (153) as well as vaccinated (154) women.
Bovine IgG, like raw cow’s milk, can induce trained immunity (122, 155), a concept that is discussed earlier (Figure 2). Through this mechanism, milk IgG antibodies may also provide protection against other pathogens. It is not yet known whether this also works against respiratory infections, but it is known that bovine IgG-induced trained immunity, as well as lactoferrin supplementation in elderly women, enhances subsequent innate immune responses via TLR7, a key receptor in immunity against single stranded RNA viruses (122, 155, 156).
Lactoferrin
Lactoferrin is an iron-scavenging immunomodulatory protein found in milk, but also in serum and other bodily fluids. It has anti-infective effects, including antibacterial and antiviral effects (110, 157, 158) (Figure 2). The antiviral properties of lactoferrin present in human breast milk and also in cow’s milk make it a natural supplement with a potential to be used to help protect against respiratory viruses. Lactoferrin’s benefits as a contributor to innate defense, are well documented as supported by a recent meta-analysis (158). Based on the observation that lactoferrin can inhibit infection of cells with the original SARS virus (159), there has recently been a lot of interest in whether lactoferrin can do the same for SARS-CoV-2, but data on this are not yet available (160, 161). It is also known that lactoferrin can induce IFN-λ as an antiviral cytokine in intestinal epithelial cells (40), and can partially restore the deficient response of pDCs of elderly women to TLR7 stimulation (156).
Other Milk Proteins
Cow’s milk also contains other proteins that may have an effect on the immune system, such as IL-10 (mostly in colostrum), transforming growth factor (TGF)-ß, osteopontin, MFGM8 (lactadherin) and lactoperoxidase. TGF-ß, IL-10 and osteopontin are anti-inflammatory components and do not play a direct role in viral infection, but can help prevent tissue damage arising from infection. Lactoperoxidase and MFGE8 have antimicrobial and possibly antiviral effects (143, 162, 163). These components have not been studied in relation to respiratory tract infection.
Human Milk Oligosaccharides (HMOs) and Prebiotic Oligosaccharides
As mentioned above, breastfeeding is known to protect against gastrointestinal and respiratory tract infections in infants (132, 164). The concentrations of human milk oligosaccharides (HMOs) in breastmilk are inversely associated with prevalence of gastrointestinal and respiratory tract infections (165), and HMOs and prebiotic oligosaccharides have been shown to enhance immune responses to vaccination or infection (166–171). HMOs play a role in the maturation of the immune system in early life, partly because of their influence on the microbiota composition and the production of microbial metabolites such as short-chain fatty acids (SCFAs) (129, 172, 173). The composition of the intestinal microbiota has been linked to the prevalence of respiratory infections (174–177). The effect of HMOs may in part be indirect via an effect on Bifidobacteria (178).
Breastfeeding and early life nutrition may also influence respiratory infections through interaction with the microbiota in the nasopharynx. The composition of the microbiota in the nasopharynx of infants is associated with the occurrence of respiratory tract infections (179, 180) and asthma (181). Furthermore, in breastfed children microbial communities in the nasopharynx are significantly different from those of bottle-fed infants (182), and the early nasopharyngeal microbial composition is linked to reduced risk for respiratory infection (183). These data suggest that breastmilk, and possibly HMOs, may affect the local microbiota composition in the nasopharynx of infants as well as that of the intestinal tract (Figures 2, 3).
Although most of the oligosaccharides in breastmilk are fermented by bifidobacteria and other bacteria in the intestine or are excreted, a small fraction of these HMOs can enter the circulation (184–186), as do the SCFAs that are produced after fermentation - especially acetate and to a lesser extent propionate and butyrate (186, 187). These SCFAs are also induced after the fermentation of prebiotic oligosaccharides like galactooligosaccharrides (GOS), fructooligosaccharrides (FOS) and inulin, as previously reviewed in (188).
SCFAs have been shown to protect against the development of asthma (189–191), suggesting that they might also influence immune responses to respiratory pathogens. Indeed, Trompette et al. showed in a murine influenza model that a high fiber diet and resulting increased intestinal SCFA levels led to decreased neutrophil recruitment to the airways preventing tissue damage in acute infection, as well as enhancing CD8+ T cell responses (192). Recent evidence suggests that SCFAs play a role in the control of respiratory infections through direct action both on microbiota and on host immune signaling (193). Influenza infection itself can also alter the microbiota composition (194), which leads to decreased SCFA production enabling bacterial superinfection by a reduction in the bactericidal activity of alveolar macrophages (195).
In addition to these indirect effects of HMOs via the microbiota, sialyllactose (SL) that is also present in bovine milk, has long been known to inhibit the binding of human influenza haemagglutinins to target cells (43, 44, 196, 197) (Figure 2). Likewise, HMOs that prevent bacterial adhesion to the respiratory epithelium may play a role in preventing bacterial pneumonia, as was shown for the HMO Lacto-N-Neotetraose (LNnT) in an animal model of pneumococcal pneumonia (198).
At the level of the respiratory epithelium, the HMO, 2’fucosyllactose (2’FL) could decrease the epithelial viral load and cytokine production after RSV exposure, and LNnT and 6’SL reduced viral load and cytokine production of epithelial cells after exposure to influenza virus (45), suggesting that these HMOs prevent infection of epithelial cells by these viruses directly by preventing adhesion. Interestingly HMOs can also enter the amniotic fluid (199), and may, after ‘inhalation’ of the fluid by the fetus, protect against respiratory infections like RSV and influenza in the first days or weeks after birth, as was also proposed for amniotic fluid antibodies by Jacobino et al. (200).
To date several studies have shown a reduction in respiratory infections by inclusion of prebiotics in infant formulas (201–205), and GOS supplementation was reported to reduce the duration of the common cold in healthy students (206). As HMOs have only recently been introduced into infant formulas, so far only a single study has addressed their effect on respiratory infections. In an infant study with formula supplemented with 2’FL and LNnT, parents reported reduced bronchitis, lower respiratory tract infection and less antibiotic use (207). In a follow-up study, infants without bronchitis or lower respiratory tract infections had increased levels of acetate and B. longum subsp. infantis in their stools, which suggests a causal relationship between HMOs, SCFAs and protection against bronchitis (178).
Plant-Derived Non-Digestible Polysaccharides, Microbiota, Immune Function and Respiratory Infection
Non-digestible polysaccharides (NDPs) constitute a large group of molecules that are found in many different foods and have been demonstrated to broadly and effectively support health and immune activity (208). NDPs include ß-glucans, pectins, resistant starch, arabinoxylans and many other types of NDPs extracted from plants (e.g. ginseng, carrot, oat), fungi (e.g. Saccharomyces cerevisiae, Lentinula edodes) or bacteria (e.g., Alcaligenes faecalis). Many in vitro and preclinical studies have demonstrated the immunomodulatory potency of NDPs [expertly reviewed by Ferreira and colleagues (209) and Jin and colleagues (210)], and clinical studies confirmed some of these findings. Double-blind placebo controlled clinical studies have demonstrated that oral intake of some NDPs (e.g. β-glucans) reduces incidence and duration of upper respiratory tract infections (URTIs) in elderly subjects or athletes (211–214).
Athletes typically demonstrate enhanced frequencies of symptoms of URTIs following intensive exercise (215). Several studies were performed to investigate whether NDP administration could lower incidence of URTIs in athletes. In a first study these subjects were provided with an insoluble ß-glucan derived from the mushroom Pleurotus ostreatus (211). A daily intake of 200 mg ß-glucan mixed with 200 mg vitamin C was compared to the placebo intake of only 200 mg vitamin C. During three months of supplementation, subjects in the test group reported a total of 65 episodes of URTI, which was significantly less than the 117 episodes reported by subjects in the placebo group. These results might have been related to the reduced drop in phagocytic potential of PBMCs and increased frequency of NK cells in the PBMCs. In a similar setting, healthy marathon runners were provided a dairy drink as placebo or a dairy drink containing 250 mg per serving per day of insoluble or soluble ß-glucan from yeast for 91 days (213). URTI symptoms were scored according to a validated questionnaire and confirmed by a physician. The total severity of URTI symptoms over the test period was significantly lower for the subjects provided with the insoluble ß-glucan. A final study involving athletes also investigated the administration of insoluble ß-glucan from yeast (216). The ß-glucan was provided in capsules, as was the rice flour placebo, at a dose of 250 or 500 mg per day for four weeks; a significantly reduced number of subjects reported URTI symptoms after two and four weeks of β-glucan when compared to the control group.
In other study population (with older people) similar effects were observed, demonstrating that the beneficial effects are not related to this specific metabolism or physical state of athletes. Older people with an approximate age of 59 years (212) or women selected according to experiencing moderate levels of stress (217) where provided with 250 mg per day of an insoluble yeast ß-glucan for 90 days or rice flour as placebo in capsules. In the older people, the intake of ß-glucan resulted in a trend towards fewer illness episodes and fewer days of illness and in women with above average stress levels β-glucan resulted in a significant reduction in days of symptoms and fewer subjects with URTI symptoms during the test period. Similarly, ß-glucan supplementation in healthy adults reduced severity and duration of URTI symptoms (218, 219).
With ginseng extracts enriched for NDPs, it has been shown in a number of clinical trials that addition to food resulted in support of immune function (220–222) and reduced incidence and severity of viral respiratory infections (223, 224). Research has shown that so-called RG-I fibers (Rhamnogalacturonan-I, referring to specific domains of pectins) are responsible for this effect of ginseng. It has now become clear that RG-I fibers from various plant sources have a modulating effect on immune function and on the microbiota (225, 226). A recent randomized controlled trial explored a low or a higher dose of cRG-I from carrot as a dietary supplement in heathy volunteers (227). After 8 weeks, the volunteers were infected with a low dose of rhinovirus in their nasal cavity and then the immune response and the development of cold symptoms were monitored. Such studies in which the effect of a nutritional intervention on the course after a (viral) challenge is measured in humans are seen as particularly relevant by experts and regulators (228, 229). Consumption of cRG-I was found to greatly accelerate the anti-viral IFN response in infected nasal epithelium and the local innate immune response, resulting in a significantly milder clinical course with less severe and shorter cold symptoms (227).
Fluorescently labelled NDP particles, being either ß-glucan capsules or particulate ß-glucan, were demonstrated to reach the ileum, colon, liver and lung and macrophages in spleen, lymph nodes and bone marrow upon oral administration in mice (230–233). In a different study, radiolabeled NDPs were shown to reach blood, intestine, and spleen and accumulated in tumors in mice (234). These studies confirm that NDPs interact with immune cells of the intestine and that they do not only move to the colon for fermentation. Moreover, these studies show that NDPs can become available in distant peripheral tissues where they can interact with local APCs and exert their immunomodulatory effect. There is clear evidence that molecular patterns on NDPs are recognized by PRRs of the immune system like Dectin-1 while actively “sensing” the contents of the gut (235, 236). This recognition and the subsequent innate immune training or direct immune activation are manners through which NPDs could modulate peripheral macrophage activity (Figure 2). Human trials to investigate induction of innate immune training so far were limited to i.v. introduction of NDPs rather than oral intake. To date only a single clinical trial has been performed to investigate orally mediated innate immune training by NDPs. Unfortunately, in this study ß-glucan was barely detectable in blood and blood-derived immune cells did not demonstrate signs of innate immune training (237). Over the past decade many NDPs, including arabinoxylans, ß-glucans and pectins, have been tested for their potency to activate innate immune cells. NDP exposure to peripheral blood monocyte-derived macrophages, either anti- or proinflammatory, or pro-tumorogenic (i.e. TAMs) induced a distinct transcriptional profile (238) and a secretome rich in chemokines and increased co-stimulatory receptor expression (239, 240). Of note, studies have demonstrated that NDP characteristics of solubility, size, monosaccharide composition and side chains (226, 239, 241) were crucial to induce immunomodulation in vitro or ex vivo.
Taken together, NDPs have been demonstrated to modulate immune responsiveness beyond the GI tract and to support APCs in expressing co-stimulatory receptors and releasing pro-inflammatory cytokines through training and in addition improve recruitment of additional immune cells.
Pro and Synbiotics
The gut and airway microbiota are linked to respiratory tract health and immunity (‘gut-lung axis’) and modulation by pharmacological agents and pro- and synbiotics has in some studies been shown to manage RTIs (242). One implication of the dynamic bidirectional relationship between gut microbes and the immune system is that changes in the composition and metabolic activity of the gut microbiota can alter immune function, potentially altering host susceptibility to infection including within the pulmonary system.
Probiotics are defined as live microorganisms that, when administered in adequate amounts, confer a health benefit on the host (243). The most commonly used probiotics are Lactobacillus and Bifidobacterium species, followed by the genera Streptococcus, Enterococcus, Propionibacterium, Bacillus, and Escherichia coli (244, 245). In addition, some yeast species are used as probiotics, for example, Saccharomyces boulardii and Saccharomyces cerevisiae to treat gastrointestinal disorders (246, 247). Generally, probiotics do not extensively divide and therefore do not permanently colonize the gut. In the gut of children, probiotics can persist for up to 4 weeks (248, 249) as they have a relatively stable ecology, while in the gut of adults probiotics persist for only around a week after consumption (250).
Besides their well-described effects in the gastrointestinal tract and in allergic diseases, probiotics also display clinical effectiveness in respiratory tract infections and support lung immunity and inflammation in both children and adults (251). Clinical studies have shown that certain probiotic strains help to prevent bacterial and viral infections, including gastroenteritis, sepsis, and RTIs (252) although others did not find such effects (253). This dynamic bidirectional relationship between gut microbiota and the immune system is sensitive to changes in the composition and metabolic activity of the gut microbiota. This also influences the pulmonary system and alters general host immune competence and thereby susceptibility to infection.
The potential for the use of probiotics has been shown in different studies including a double-blind placebo-controlled trial, in which milk was supplemented with the probiotic Lactobacillus reuteri, but not Lactobacillus casei, which showed that acute infectious diarrhoea could be reduced in Indonesian children with a low nutritional status (254). Probiotics such as Lactobacillus and Bifidobacterium have shown direct potential in respiratory infections as illustrated in a meta-analysis in which they were able to increase the efficacy of influenza vaccines in adults (255). These probiotics enhanced the rate of seroconversion in older individuals and thereby have potential to be used as preventive treatment before seasonal influenza vaccination. In a systematic review it was shown that the use of selective probiotics and synbiotics in optimized formulations can provide prophylactic and complementary treatment benefits in patients with RTIs, including COVID-19 and influenza infections as they reduce the severity of the infection symptoms and the duration of disease (256).
Probiotics, including Lactobacillus plantarum and Lactobacillus reuteri, are mainly used to prevent infections and for recovery from gastrointestinal infections and are not yet widely used to prevent or treat URTIs, although some studies have shown them to alleviate symptoms of URTIs by strengthening the respiratory mucosa (257, 258). In recent meta-analyses of double-blind, randomized, and placebo-controlled trials it was shown that probiotics significantly reduced rates of acute URTI and the associated antibiotic use, but they did not decrease the duration of each single infection episode (259, 260). Children that were supplemented with probiotics had fewer days with URTIs and therefore had fewer days absent from day care or school compared with children receiving placebo (234, 245).
Probiotics can modulate the plasma levels of cytokines and exert differential modulation of innate and the adaptive arms of the immune response based on host (epi)genetic differences and the particular microbial strain (251, 261). Dietary supplementation with probiotics like Bifidobacterium longum, can stimulate trained immunity in alveolar macrophages, putatively by the NOD2 receptor and the action of histone 3 lysine 4 trimethylation (H3K4me3) to induce epigenetic changes at the level of histones. These macrophages respond more efficiently with increased IFN-γ production to respiratory pathogens like influenza as observed in mice that received these beneficial microorganisms. In these mice, NK cell activity was significantly increased, resulting in enhanced levels of IFN-γ, IL-2, IL-12, and IL-18 in both spleen and lungs resulting in decreased virus proliferation and suppression of inflammation (262). At the same time, the probiotic Bifidobacterium longum significantly reduced influenza-infection induced production of pro-inflammatory cytokines, including IL-6 and TNF-α.
Probiotics therefore may promote resistance against pathogens (261). Probiotics increase leukocyte, neutrophil, and NK cell counts and activity while increasing the production of IL-10 and decreasing production of pro-inflammatory TNF-α, IL-1ß, and IL-8 (263). It has been shown that probiotics inhibit Th17 differentiation and the production of IL-17F, IL-23 and TNF-α, while promoting the IL-10 secreting Treg subset (246). Thereby, probiotics inhibit inflammatory responses and regulate immune cell homeostasis. In addition, probiotics increase the frequency of IgA-positive cells in Peyer’s patches in the lamina propria and thereby inhibit bacterial adherence to epithelial cells, maintain the epithelial barrier function, and facilitate the neutralization of bacterial toxins (264). Because IgA-positive B-cells were shown to be able to migrate to other mucosal tissues in the body, their potential to provide protection in the respiratory tract should be investigated further.
Likely candidates responsible for the functional impact of the microbiota and probiotics are SCFAs. Through their activity as histone deacetylase (HDAC) inhibitors, SCFAs might also induce innate immune memory. Mainly butyrate and propionate, and to a lesser extend acetate, demonstrate this inhibitory activity. SCFAs have been demonstrated to affect the immune system in the respiratory tract, for example with influenza infection (192), but also RSV infection (265). In turn, this also means that fibers and complex sugars that lead to the growth of healthy bifidobacteria and lactobacilli, as well as the production of SCFAs, can be important for antiviral immunity. Moreover, production of SCFAs also induced enhanced expression of respiratory IFN-β thereby increasing the expression of interferon-stimulated genes (ISGs) in the lung and thereby protected against RSV-induced disease involving activation of the membrane receptor GPR43 (265).
A profound imbalance in gut microbiota can drive the progression of COVID-19 symptoms towards the acute respiratory distress syndrome (ARDS), and this development might be reinforced by the use of antiviral medication (266). Respiratory infectious diseases might thus be affected by gut dysbiosis characterized by a decline in beneficial commensals and enrichment of opportunistic pathogens (242, 267). Administration of a Lactobacillus plantarum 06CC2 in mice with influenza-like disease, decreased expression of pro-inflammatory cytokines and increased the mRNA level of IFN-β, IFN-γ, OAS1a, and ISG15 in the lungs underscoring the antiviral activities of this probiotic (268). Bradley et al. showed that the intestinal microbiota influences IFN-α/β receptor expression on the surface of respiratory epithelial cells, which in the case of respiratory virus infection are able to respond more efficiently to type I IFNs stimulation with enhanced ISG levels and suppressed early virus replication (269). Some studies suggested there is transfer of bacterial strains like Lactobacillus plantarum from the gut to the lungs upon development of COVID-19 (270). Next to these demonstrated effects, probiotics are also anticipated to dampen the cytokine storm observed in COVID-19 patients, especially in severe stages of the disease, which was associated with increased levels of cytokines and chemokines like IP-10, MCP-1, MIP-1α, TNF-α, IL-1β, IFN-γ, IL-4 and IL-10 (268).
Probiotic supplementation could inhibit the COVID-19 cytokine storm by simultaneously boosting innate immunity and avoiding the exaggeration of adaptive immunity by the suppression of the inflammatory cytokine response. This way, probiotics can prevent the severity and the occurrence of ARDS. Taken together, modulation of gut microbiota by probiotics is suggested as a possible strategy to improve the clinical manifestations of respiratory tract infections and crucially also of COVID-19 (271).
Probiotics and prebiotics, as described above, individually have roles in improving immune health and in combination (synbiotics) might even synergize. The use of synbiotics, often a combination of Bifidobacterium breve, Lactobacillus casei, Streptococcus and galacto-oligosaccharides (GOS) or fructo-oligosaccharides (FOS) and inulin, is primarily based on the assumption that the included prebiotics enhance the survival of the probiotics present in the supplement in the gut as well as stimulating indigenous anaerobic bacteria. Gut microbial components and metabolites (postbiotics) including SCFAs, for instance, are involved in gut-lung immune communication (272).
As indicated earlier, the elderly population is particularly affected by a decline in immune function including the antibody response to influenza vaccination although in this population no beneficial effects in reversal of immunosenescence by synbiotics were found (273). In contrast, a synbiotic (Lactocare©) consisting of a mixture of Lactobacillus rhamnosus, Lactobacillus casei, Lactobacillus acidophilus, Bifidobacterium breve, Lactobacillus bulgaricus, Bifidobacterium longum, Streptococcus thermophilus (each at 10⁹CFU) plus fructooligosacharide FOS was shown to reduce episodes of viral infection in asthmatic children (274).
A recent systematic review and meta-analysis identified 16 studies including 10,443 participants examining the effects of synbiotics on RTI incidence, duration, and/or severity. Results demonstrated that synbiotic interventions reduced the incidence rate of RTIs and the proportion of participants who experienced a RTI by 16% (275). Three separate large randomized controlled trials compared similar synbiotic supplements (containing 3 to 5 strains of Lactobacillus plantarum, Lactobacillus rhamnosus, and Bifidobacterium lactis, lactoferrin and prebiotics such as either FOS (short-chain fructooligosaccharides) or GOS (galactooligosaccharides)) to placebo, and all three of these trials showed reductions in the incidence, duration, and severity of RTI with the synbiotic (276). It was also proposed that this synbiotic could reduce SARS-Cov2 infection in high-risk medical staff working in COVID-19 hospital wards (277).
Such synbiotic interventions are suggested to be most effective in the prevention phase of a respiratory infection while they are mostly unable to influence the course of the infection once the pathogen has started to replicate in the host. They are therefore more a preventive than a therapeutic option. Nevertheless, a review describing mechanisms whereby probiotic and prebiotic interventions may link the gut microbiota to the outcome of COVID-19 infection provided arguments that synbiotics may help patients after infection with SARS-COV-2 (278) and this was based on clinical evidence indicating that modulation of the gut microbiota can positively influence COVID-19 progression. The consumption of synbiotic products may thus reduce incidence, duration and severity of respiratory tract infections (279). It would be very interesting to see if the consumption of pre- pro- or synbiotics – or other components discussed in this review - can partially reverse the loss of smell, that is associated with a loss of appetite, and hinders recovery after SARS-CoV-2 infection.
Anatomical and Physiological Aspects of Interaction of Nutrition With the Immune System in the Respiratory Tract
Micronutrients, milk-derived components, plant derived NDPs and pre-, pro- and synbiotics have all been demonstrated to support anti-viral immunity in in vitro and in vivo animal models as well as in some clinical studies.
Likewise, oral vaccines can lead to immune responses in the airways, suggesting that the oral route of administration is interconnected with the upper airways. Oral immunization with cholera toxin results in specific IgA being produced in the upper airways, the lymphoid tissues in the head/neck area, the digestive system, as well as in breast tissue [(280) and Figure 2]. These data indicate that oral intake of cholera toxin can induce immune responses that also target the upper respiratory tract. Several studies have since then confirmed that oral administration of antigens result in IgA production in the upper respiratory tract (281–283).
However, there appears to be a knowledge gap on how nutritional components in the gastrointestinal tract can affect (viral) infections in the respiratory tract. Here, we introduce a hypothetical model of how many of these food components can have an effect on respiratory tract infections, keeping in mind the anatomy of the GI and respiratory tracts, interaction sites of food and food components with the immune system, and the interplay with the microbiota.
Nutritional components that are absorbed in the GI tract can exert their effects on the local immune system, but via systemic circulation also at distant immune sites. Mostly non-absorbed nutrients can have local effects, or can have distinct effects through instructing migratory cells of the immune system, or induction of microbial metabolites, that can become available in the circulation and can reach distant tissues. These local effects can occur before the food reaches the stomach and is digested, and can, in addition to immune effects, have anti-adhesive effects on swallowed respiratory pathogens.
The nasopharyngeal mucosa is an environment that is heavily populated with commensal organisms, some of which are associated with increased or decreased risk for respiratory infections (179, 180, 183). As discussed in the oligosaccharide section, breastmilk-derived HMOs can influence the microbiota in the nasopharynx, thus reducing the risk for respiratory infections via a local effect (182).
Because of their anatomical location, the immune tissues of Waldeyer’s ring could have a crucial role in developing potent immune responses to respiratory pathogens (Figure 3). The immunological tissues in the head/neck area are mainly structured in tonsils, lymph nodes and lymph follicles. The largest area of contact between food and nasal contents and the immune system occurs in the area where nose and mouth come together, the nasopharynx. The pharynx is surrounded by a number of structured lymphoid nodules (tonsils), comprising the lingual tonsil, the nasopharyngeal tonsils (adenoids), the palatine tonsils and tubal tonsils. The tonsils contain large numbers of cells (1 x 109 lymphoid cells) mainly consisting of B-cells and CD4+ T-cells (284). Together these are named Waldeyer’s ring, also referred to as the nasal mucosa associated lymphoid tissue (NALT).
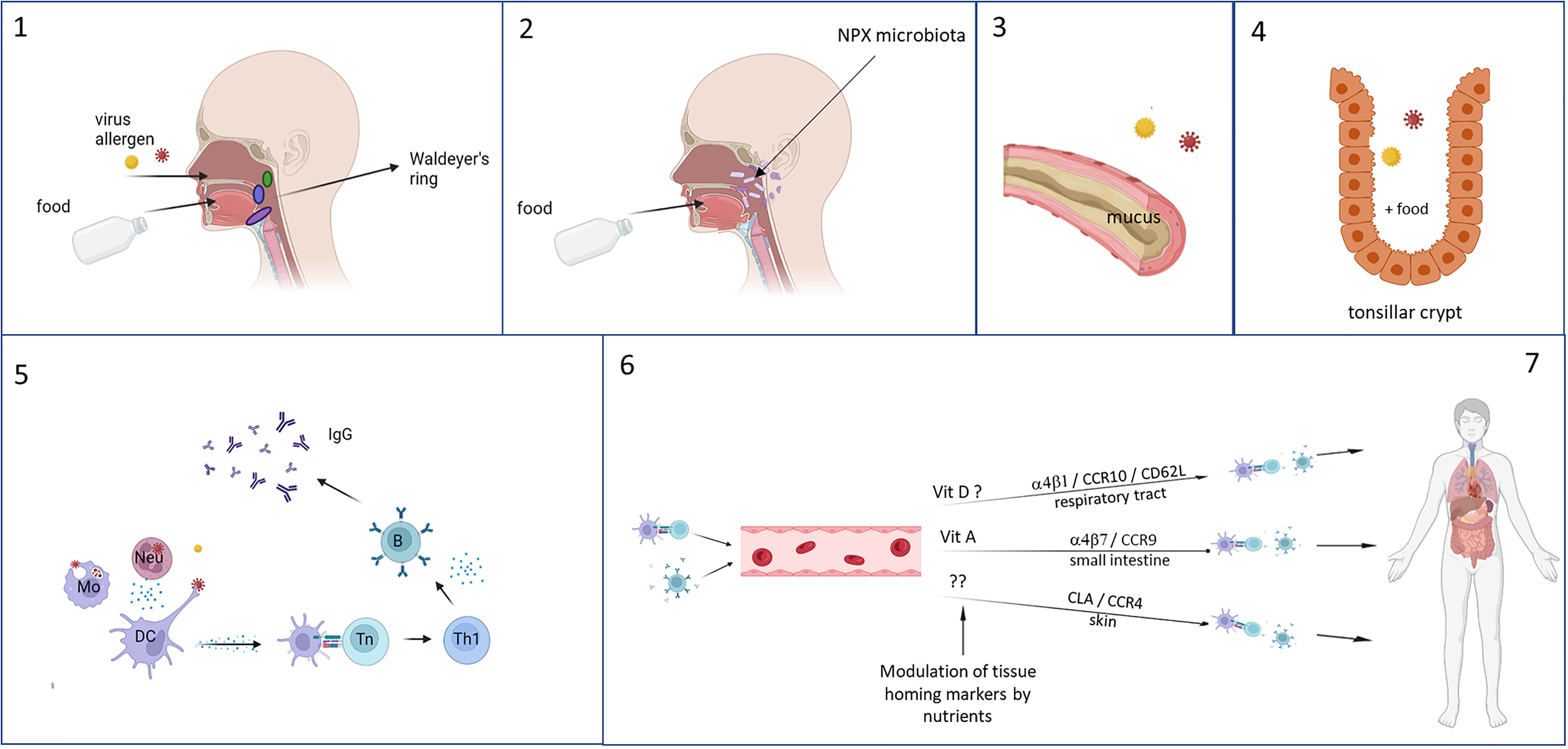
Figure 3 Foods are ingested and enter the gastrointestinal tract via the mouth and respiratory pathogens via the nasal cavity, both ending up in the tonsils of Waldeyer’s ring (1). Especially in infants, reflux can cause a direct interaction of the foods with the nasopharyngeal microbiota and influences their composition (2). Mixing of the food with saliva and mucus (based on charge and polarity) enhances the residence time in the nasopharynx (3), and as a result the still undigested food and respiratory pathogens can interact with each other and the immune tissues in the tonsil, where stasis occurs in the crypts which further lengthening the duration of interaction (4). Local immune responses can occur, during which can be modulated by the food components (5). Immune cell recirculate and home to different tissues – especially to the upper respiratory tract because of instruction by stromal cells in the tonsils – which is determined by the expression of homing receptors. This can also be influenced by food components, selectively homing, for example by vitamins, resulting in increased memory responses in the local tissues of the upper respiratory tract (6). This figure was created with BioRender.com.
The tonsils and adenoids have multiple antigen-retaining crypts, that greatly increase the surface area of contact between food components and the underlying cells. The reticulated epithelium lining the tonsillar crypts is probably an antigen entry portal, specialized in transporting antigens to the subepithelial tissue within on average about 10 minutes after food consumption (284–286). The adenoids, but to a much lesser extent the palatine tonsils, have epithelia that have well developed tight junctions, and that also contain M-like cells and DCs that contribute to antigen sampling (287, 288). Because of the anatomical structure and location of Waldeyer’s ring, it functions as a gatekeeper for respiratory, swallowed pathogens from nasal secretions. Likewise, it is also exposed to nutritional components that locally can modulate the immune responses to respiratory pathogens sampled in Waldeyer’s ring (Figure 3).
As stromal factors determine tissue homing properties of locally activated lymphocytes, it has been suggested that antigens taken up in the tonsils of Waldeyer’s ring will induce the formation of especially IgG-antibody producing memory B cells with homing capacity to lungs, upper airways and peripheral blood (289).
Selective homing of immune cells to the respiratory tract and the intestine is tightly regulated by the expression of chemokine receptors and adhesion molecules (281, 289–292). Of note, expression of homing receptors can be influenced by interactions with food components, which may also influence recirculation and homing properties of immune cells. For example, the vitamin A metabolite retinoic acid induces expression of CCR9 and thus promotes homing to the intestine (293, 294), and vitamin D3 induces CCR10 that is associated with homing to the respiratory tract (81–84). Interestingly, vitamin D3 has also been shown to block the upregulation of retinoic acid-induced gut homing markers (82, 295) and may thus promote homing to the respiratory tract.
A further aspect to consider is the food matrix. The food matrix may affect whether the nutritional components in the food can mix with saliva and the mucus overlaying the nasopharyngeal epithelium and Waldeyer’s ring. This mixing will increase the duration of retention of foods in the oral and nasopharyngeal mucosa. A longer retention time will increase interaction of these food components with the underlying epithelium and cells of the immune system in Waldeyer’s ring.
Two known examples that can promote residence time and mixing with mucus are charge and water in oil emulsions. Mucins have sialic acid and sulfate groups located on terminal side chains on the glycoprotein molecules, as a result of which they are anionic (negatively charged) at neutral pH (296, 297). Positively charged chitosan microparticles strongly enhance systemic as well as local immune responses to diphteria toxin when applied orally, and significantly enhance IgG production after nasal administration (298). Similar results were obtained with ovalbumin bound to cationic (i.e. positively charged) maltodextrin nanoparticles (299). In addition, water in oil emulsions and gels are other options to increase mouth and GI tract presence of compounds of interest (300). Thus, positively charged components – and ideally a matrix (particulate or viscous) that surrounds ingredients by a positive charge - can enhance the mixing behavior with mucus and saliva, and are expected to result in enhanced interaction between the food product and the mucosal immune system of Waldeyer’s ring.
The above rationale suggests that foods or food components may exert their immunomodulatory function locally in Waldeyer’s ring, thus explaining their effects on respiratory infections.
Concluding Remarks
Nutritional components can support immune function against respiratory pathogens via several mechanisms, and at several levels as described in this review. Nutritional approaches to prevent infections can thus be relevant to help prevent infections in vulnerable people like infants and the elderly, and can help to reduce the pressure on healthcare systems, especially in the winter months when respiratory infections occur frequently.
Author Contributions
All authors contributed to the writing, preparation and finalization of the manuscript.
Funding
As this is a review paper, no research was funded. The authors work affiliations covered the hours spent on writing the review.
Conflict of Interest
PC has research funding from Bayer Consumer Care and acts as an advisor/consultant to DSM, BASF AS, Cargill, Smartfish, Fresenius-Kabi, Bayer Consumer Care, GSK Consumer Healthcare, Danone/Nutricia, Nutrileads and Kemin. RN is employed by FrieslandCampina. RA is employed by Nutrileads.
The remaining authors declare that the research was conducted in the absence of any commercial or financial relationships that could be construed as a potential conflict of interest.
Publisher’s Note
All claims expressed in this article are solely those of the authors and do not necessarily represent those of their affiliated organizations, or those of the publisher, the editors and the reviewers. Any product that may be evaluated in this article, or claim that may be made by its manufacturer, is not guaranteed or endorsed by the publisher.
References
1. Dhariwal J, Edwards MR, Johnston SL. Anti-Viral Agents: Potential Utility in Exacerbations of Asthma. Curr Opin Pharmacol (2013) 13:331–6. doi: 10.1016/j.coph.2013.04.010
2. Farne HA, Johnston SL. Immune Mechanisms of Respiratory Viral Infections in Asthma. Curr Opin Immunol (2017) 48:31–7. doi: 10.1016/j.coi.2017.07.017
3. Jartti T, Gern JE. Clinical Reviews in Allergy and Immunology Role of Viral Infections in the Development and Exacerbation of Asthma in Children. J Allergy Clin Immunol (2018) 140:895–906. doi: 10.1016/j.jaci.2017.08.003
4. Lopez-Leon S, Wegman-Ostrosky T, Perelman C, Sepulveda R, Rebolledo PA, Cuapio A, et al. More Than 50 Long-Term Effects of COVID-19: A Systematic Review and Meta-Analysis. Sci Rep (2021) 11:16144. doi: 10.1038/s41598-021-95565-8
5. Michelen M, Manoharan L, Elkheir N, Cheng V, Dagens A, Hastie C, et al. Characterising Long COVID: A Living Systematic Review. BMJ Glob Heal (2021) 6(9):e005427. doi: 10.1136/bmjgh-2021-005427
6. Munblit D, Simpson F, Mabbitt J, Dunn-Galvin A, Semple C, Warner JO. Legacy of COVID-19 Infection in Children: Long-COVID Will Have a Lifelong Health/Economic Impact. Arch Dis Child (2021). doi: 10.1136/archdischild-2021-321882
7. Spagnolo P, Balestro E, Aliberti S, Cocconcelli E, Biondini D, Della CG, et al. Pulmonary Fibrosis Secondary to COVID-19: A Call to Arms? Lancet Respir Med (2020) 8:750–2. doi: 10.1016/S2213-2600(20)30222-8
8. Linden D, Guo-Parke H, Coyle PV, Fairley D, McAuley DF, Taggart CC, et al. Respiratory Viral Infection: A Potential “Missing Link” in the Pathogenesis of COPD. Eur Respir Rev (2019) 28(151):180063. doi: 10.1183/16000617.0063-2018
9. Taubenberger JK, Morens DM. 1918 Influenza: The Mother of All Pandemics. Emerg Infect Dis (2006) 12:15–22. doi: 10.3201/eid1201.050979
10. Flasche S, Slack M ME. Long Term Trends Introduce a Potential Bias When Evaluating the Impact of the Pneumococcal Conjugate Vaccination Programme in England and Wales. Euro Surveill (2011) 16(20):19868. doi: 10.2807/ese.16.20.19868-en
11. Kollmann TR, Levy O, Montgomery RR, Goriely S. Innate Immune Function by Toll-Like Receptors: Distinct Responses in Newborns and the Elderly. Immunity (2012) 37:771–83. doi: 10.1016/j.immuni.2012.10.014
12. Simon AK, Hollander GA, McMichael A. Evolution of the Immune System in Humans From Infancy to Old Age. Proc Biol Sci (2015) 282:20143085. doi: 10.1098/rspb.2014.3085
13. Lewis ED, Richard C, Larsen BM, Field CJ. The Importance of Human Milk for Immunity in Preterm Infants. Clin Perinatol (2017) 44:23–47. doi: 10.1016/j.clp.2016.11.008
14. Andreas NJ, Kampmann B, Mehring Le-Doare K. Human Breast Milk: A Review on Its Composition and Bioactivity. Early Hum Dev (2015) 91:629–35. doi: 10.1016/j.earlhumdev.2015.08.013
15. Ogra PL. Immunology of Human Milk and Lactation: Historical Overview. Nestle Nutr Inst Workshop Ser (2020) 94:11–26. doi: 10.1159/000505211
16. Gopalakrishna KP, Hand TW. Influence of Maternal Milk on the Neonatal Intestinal Microbiome. Nutrients (2020) 12:823. doi: 10.3390/nu12030823
17. Goldsmith F, O’Sullivan A, Smilowitz JT, Freeman SL. Lactation and Intestinal Microbiota: How Early Diet Shapes the Infant Gut. J Mammary Gland Biol Neoplasia (2015) 20:149–58. doi: 10.1007/s10911-015-9335-2
18. Agarwal S, Busse PJ. Innate and Adaptive Immunosenescence. Ann Allergy, Asthma. Immunol Off Publ Am Coll Allergy Asthma Immunol (2010) 104:182–3. doi: 10.1016/j.anai.2009.11.009 210.
19. Bektas A, Schurman SH, Sen R, Ferrucci L. Human T Cell Immunosenescence and Inflammation in Aging. J Leukoc Biol (2017) 102:977–88. doi: 10.1189/jlb.3RI0716-335R
20. Castelo-Branco C, Soveral I. The Immune System and Aging: A Review. Gynecol Endocrinol Off J Int Soc Gynecol Endocrinol (2014) 30:16–22. doi: 10.3109/09513590.2013.852531
21. De Martinis M, Modesti M, Ginaldi L. Phenotypic and Functional Changes of Circulating Monocytes and Polymorphonuclear Leucocytes From Elderly Persons. Immunol Cell Biol (2004) 82:415–20. doi: 10.1111/j.0818-9641.2004.01242.x
22. Pawelec G, Larbi A, Derhovanessian E. Senescence of the Human Immune System. J Comp Pathol (2010) 142 Suppl:S39–44. doi: 10.1016/j.jcpa.2009.09.005
23. Pera A, Campos C, López N, Hassouneh F, Alonso C, Tarazona R, et al. Immunosenescence: Implications for Response to Infection and Vaccination in Older People. Maturitas (2015) 82:50–5. doi: 10.1016/j.maturitas.2015.05.004
24. Yoshikawa TT. Epidemiology and Unique Aspects of Aging and Infectious Diseases. Clin Infect Dis (2000) 30:931–3. doi: 10.1086/313792
25. Canaday DH, Amponsah NA, Jones L, Tisch DJ, T.R H, Ramachandra L. Influenza-Induced Production of Interferon-Alpha Is Defective in Geriatric Individuals. JClin Immunol (2010) 30:373–83. doi: 10.1007/s10875-010-9374-9.Influenza-Induced
26. van Splunter M, Perdijk O, Fick-Brinkhof H, Floris-Vollenbroek EG, Meijer B, Brugman S, et al. Plasmacytoid Dendritic Cell and Myeloid Dendritic Cell Function in Ageing: A Comparison Between Elderly and Young Adult Women. PloS One (2019) 14:e0225825. doi: 10.1371/journal.pone.0225825
27. van der Made CI, Simons A, Schuurs-Hoeijmakers J, van den Heuvel G, Mantere T, Kersten S, et al. Presence of Genetic Variants Among Young Men With Severe COVID-19. JAMA (2020) 324:663–73. doi: 10.1001/jama.2020.13719
28. Kotenko SV, Durbin JE. Contribution of Type III Interferons to Antiviral Immunity: Location, Location, Location. J Biol Chem (2017) 292:7295–303. doi: 10.1074/jbc.R117.777102
29. Sykes A, Edwards MR, MacIntyre J, Del Rosario A, Bakhsoliani E, Trujillo-Torralbo MB, et al. Rhinovirus 16-Induced IFN-α and IFN-β Are Deficient in Bronchoalveolar Lavage Cells in Asthmatic Patients. J Allergy Clin Immunol (2012) 129:1506–14. doi: 10.1016/j.jaci.2012.03.044
30. Kalinowski A, Galen BT, Ueki IF, Sun Y, Mulenos A, Osafo-Addo A, et al. Respiratory Syncytial Virus Activates Epidermal Growth Factor Receptor to Suppress Interferon Regulatory Factor 1-Dependent Interferon-Lambda and Antiviral Defense in Airway Epithelium. Mucosal Immunol (2018) 11:958–67. doi: 10.1038/mi.2017.120
31. Juno JA, Keynan Y, Fowke KR. Invariant NKT Cells: Regulation and Function During Viral Infection. PloS Pathog (2012) 8:e1002838. doi: 10.1371/journal.ppat.1002838
32. Kulkarni RR, Haeryfar SM, Sharif S. The Invariant NKT Cell Subset in Anti-Viral Defenses: A Dark Horse in Anti-Influenza Immunity? J Leukoc Biol (2010) 88:635–43. doi: 10.1189/jlb.0410191
33. Diana J, Lehuen A. NKT Cells: Friend or Foe During Viral Infections? Eur J Immunol (2009) 39:3283–91. doi: 10.1002/eji.200939800
34. De Santo C, Salio M, Masri SH, Lee LY-H, Dong T, Speak AO, et al. Invariant NKT Cells Reduce the Immunosuppressive Activity of Influenza A Virus-Induced Myeloid-Derived Suppressor Cells in Mice and Humans. J Clin Invest (2008) 118:4036–48. doi: 10.1172/JCI36264
35. Ho L-P, Denney L, Luhn K, Teoh D, Clelland C, McMichael AJ. Activation of Invariant NKT Cells Enhances the Innate Immune Response and Improves the Disease Course in Influenza A Virus Infection. Eur J Immunol (2008) 38:1913–22. doi: 10.1002/eji.200738017
36. Brennan PJ, Cheng T-Y, Pellicci DG, Watts GFM, Veerapen N, Young DC, et al. Structural Determination of Lipid Antigens Captured at the CD1d–T-Cell Receptor Interface. Proc Natl Acad Sci (2017) 114:8348–53. doi: 10.1073/pnas.1705882114
37. Nieuwenhuis EES, Matsumoto T, Lindenbergh D, Willemsen R, Kaser A, Simons-Oosterhuis Y, et al. Cd1d-Dependent Regulation of Bacterial Colonization in the Intestine of Mice. J Clin Invest (2009) 119:1241–50. doi: 10.1172/JCI36509
38. Middendorp S, Nieuwenhuis EES. NKT Cells in Mucosal Immunity. Mucosal Immunol (2009) 2:393–402. doi: 10.1038/mi.2009.99
39. Abos Gracia B, López Relaño J, Revilla A, Castro L, Villalba M, Martín Adrados B, et al. Human Invariant Natural Killer T Cells Respond to Antigen-Presenting Cells Exposed to Lipids From Olea Europaea Pollen. Int Arch Allergy Immunol (2017) 173:12–22. doi: 10.1159/000467394
40. Shin K, Oda H, Wakabayashi H, Yamauchi K, Abe F. Effects of Lactoferrin on the Production of Interferon-λ by the Human Intestinal Epithelial Cell Line HT-29. Biochem Cell Biol (2017) 95:53–6. doi: 10.1139/bcb-2016-0031
41. Ioannidis I, Ye F, McNally B, Willette M, Flaño E. Toll-Like Receptor Expression and Induction of Type I and Type III Interferons in Primary Airway Epithelial Cells. J Virol (2013) 87:3261–70. doi: 10.1128/JVI.01956-12
42. Tengroth L, Millrud CR, Kvarnhammar AM, Kumlien Georén S, Latif L, Cardell L-O. Functional Effects of Toll-Like Receptor (TLR)3, 7, 9, RIG-I and MDA-5 Stimulation in Nasal Epithelial Cells. PloS One (2014) 9:e98239. doi: 10.1371/journal.pone.0098239
43. Günther SC, Maier JD, Vetter J, Podvalnyy N, Khanzhin N, Hennet T, et al. Antiviral Potential of 3’-Sialyllactose- and 6’-Sialyllactose-Conjugated Dendritic Polymers Against Human and Avian Influenza Viruses. Sci Rep (2020) 10:768. doi: 10.1038/s41598-020-57608-4
44. Pandey RP, Kim DH, Woo J, Song J, Jang SH, Kim JB, et al. Broad-Spectrum Neutralization of Avian Influenza Viruses by Sialylated Human Milk Oligosaccharides: In Vivo Assessment of 3’-Sialyllactose Against H9N2 in Chickens. Sci Rep (2018) 8:2563. doi: 10.1038/s41598-018-20955-4
45. Duska-mcewen G, Senft AP, Ruetschilling TL, Barrett EG, Buck RH. Human Milk Oligosaccharides Enhance Innate Immunity to Respiratory Syncytial Virus and Influenza In Vitro. Food Nutr Sci (2014) 5:1387–98. doi: 10.4236/fns.2014.514151
46. Terabayashi T, Morita M, Ueno M, Nakamura T, Urashima T. Inhibition of Influenza-Virus-Induced Cytopathy by Sialylglycoconjugates. Carbohydr Res (2006) 341:2246–53. doi: 10.1016/j.carres.2006.06.017
47. Aaby P, Roth A, Ravn H, Napirna BM, Rodrigues A, Lisse IM, et al. Randomized Trial of BCG Vaccination at Birth to Low-Birth-Weight Children: Beneficial Nonspecific Effects in the Neonatal Period? J Infect Dis (2011) 204:245–52. doi: 10.1093/infdis/jir240
48. Benn CS, Netea MG, Selin LK, Aaby P. A Small Jab - a Big Effect: Nonspecific Immunomodulation by Vaccines. Trends Immunol (2013) 34:431–9. doi: 10.1016/j.it.2013.04.004
49. Netea MG, Quintin J, Van Der Meer JWM. Perspective Trained Immunity : A Memory for Innate Host Defense. Cell Host Microbe (2011) 9:355–61. doi: 10.1016/j.chom.2011.04.006
50. Kleinnijenhuis J, Quintin J, Preijers F, Joosten LA, Ifrim DC, Saeed S, et al. Bacille Calmette-Guerin Induces NOD2-Dependent Nonspecific Protection From Reinfection via Epigenetic Reprogramming of Monocytes. Proc Natl Acad Sci USA (2012) 109:17537–42. doi: 10.1073/pnas.1202870109
51. Saeed S, Quintin J, Kerstens HHD, Rao NA, Aghajanirefah A, Matarese F, et al. Epigenetic Programming of Monocyte-to-Macrophage Differentiation and Trained Innate Immunity. Science (2014) 345:1251086. doi: 10.1126/science.1251086
52. Quintin J, Saeed S, Martens JH, Giamarellos-Bourboulis EJ, Ifrim DC, Logie C, et al. Candida Albicans Infection Affords Protection Against Reinfection via Functional Reprogramming of Monocytes. Cell HostMicrobe (2012) 12:223–32. doi: 10.1016/j.chom.2012.06.006
53. O’Neill LAJ, Netea MG. BCG-Induced Trained Immunity: Can It Offer Protection Against COVID-19? Nat Rev Immunol (2020) 20:335–7. doi: 10.1038/s41577-020-0337-y
54. Mantovani A, Netea MG. Trained Innate Immunity, Epigenetics, and Covid-19. N Engl J Med (2020) 383:1078–80. doi: 10.1056/NEJMcibr2011679
55. van Daal MT, Folkerts G, Garssen J, Braber S. Pharmacological Modulation of Immune Responses by Nutritional Components. Pharmacol Rev (2021) 73:198–232. doi: 10.1124/pharmrev.120.000063
56. Gombart AF, Pierre A, Maggini S. A Review of Micronutrients and the Immune System–Working in Harmony to Reduce the Risk of Infection. Nutrients (2020) 12:236. doi: 10.3390/nu12010236
57. Calder PC. Nutrition, Immunity and COVID-19. BMJ Nutr Prev Health (2020) 3:74–92. doi: 10.1136/bmjnph-2020-000085
58. Calder PC. Nutrition and Immunity: Lessons for COVID-19. Eur J Clin Nutr (2021) 75:1309–18. doi: 10.1038/s41430-021-00949-8
59. Calder PC. Feeding the Immune System. Proc Nutr Soc (2013) 72:299–309. doi: 10.1017/S0029665113001286
60. Calder PC, Carr AC, Gombart AF, Eggersdorfer M. Optimal Nutritional Status for a Well-Functioning Immune System Is an Important Factor to Protect Against Viral Infections. Nutrients (2020) 12:1181. doi: 10.3390/nu12041181
61. van Neerven RJJ, Albers R, Wichers HJ, Garssen J, Savelkoul HFJ. Voeding En Immuniteit: Kan Voeding Bijdragen Aan Het Voorkomen Van Allergie, Infectie En Ontsteking? Ned Tijdschr voor Allerg en Astma (2017) 17:21–8.
62. Ross AC. Vitamin A. And Retinoic Acid in T Cell-Related Immunity. Am J Clin Nutr (2012) 96:1166S–72S. doi: 10.3945/ajcn.112.034637
63. Raverdeau M, Mills KHG. Modulation of T Cell and Innate Immune Responses by Retinoic Acid. J Immunol (2014) 192:2953–8. doi: 10.4049/jimmunol.1303245
64. Brown CC, Noelle RJ. Seeing Through the Dark: New Insights Into the Immune Regulatory Functions of Vitamin a. Eur J Immunol (2015) 45:1287–95. doi: 10.1002/eji.201344398
65. Larange A, Cheroutre H. Retinoic Acid and Retinoic Acid Receptors as Pleiotropic Modulators of the Immune System. Annu Rev Immunol (2016) 34:369–94. doi: 10.1146/annurev-immunol-041015-055427
66. Erkelens MN, Mebius RE. Retinoic Acid and Immune Homeostasis: A Balancing Act. Trends Immunol (2017) 38:168–80. doi: 10.1016/j.it.2016.12.006
67. Huang Z, Liu Y, Qi G, Brand D, Zheng SG. Role of Vitamin A in the Immune System. J Clin Med (2018) 7:258. doi: 10.3390/jcm7090258
68. Oliveira L de M, Teixeira FME, Sato MN. Impact of Retinoic Acid on Immune Cells and Inflammatory Diseases. Mediators Inflammation (2018) 2018:3067126. doi: 10.1155/2018/3067126
69. Villamor E, Fawzi WW. Effects of Vitamin A Supplementation on Immune Responses and Correlation With Clinical Outcomes. Clin Microbiol Rev (2005) 18:446–64. doi: 10.1128/CMR.18.3.446
70. Sommer A, Katz J, Tarwotjo I. Increased Risk of Respiratory Disease and Diarrhea in Children With Preexisting Mild Vitamin A Deficiency. Am J Clin Nutr (1984) 40:1090–5. doi: 10.1093/ajcn/40.5.1090
71. Butler JC, Havens PL, Sowell AL, Huff DL, Peterson DE, Day SE, et al. Measles Severity and Serum Retinol (Vitamin A) Concentration Among Children in the United States. Pediatrics (1993) 91:1176–81. doi: 10.1542/peds.91.6.1176
72. Hu N, Li Q-B, Zou S-Y. [Effect of Vitamin A as an Adjuvant Therapy for Pneumonia in Children: A Meta Analysis]. Zhongguo Dang Dai Er Ke Za Zhi (2018) 20:146–53. doi: 10.7499/j.issn.1008-8830.2018.02.013
73. Abobaker A, Alzwi A, Alraied AHA. Overview of the Possible Role of Vitamin C in Management of COVID-19. Pharmacol Rep (2020) 72:1517–28. doi: 10.1007/s43440-020-00176-1
74. Carr AC, Maggini S. Vitamin C and Immune Function. Nutrients (2017) 9(11):1211. doi: 10.3390/nu9111211
75. Hemilä H, Louhiala P. Vitamin C for Preventing and Treating Pneumonia. Cochrane Database Syst Rev (2013). doi: 10.1002/14651858.CD005532.pub3
76. Hemilä H, Chalker E. Vitamin C for Preventing and Treating the Common Cold. Cochrane Database Syst Rev (2013) 2013:CD000980. doi: 10.1002/14651858.CD000980.pub4
77. Pludowski P, Grant WB, Konstantynowicz J, Holick MF. Editorial: Classic and Pleiotropic Actions of Vitamin D. Front Endocrinol (Lausanne) (2019) 10:341. doi: 10.3389/fendo.2019.00341
78. Sassi F, Tamone C, D’Amelio P. Vitamin D: Nutrient, Hormone, and Immunomodulator. Nutrients (2018) 10:1656. doi: 10.3390/nu10111656
79. Gombart AF. The Vitamin D-Antimicrobial Peptide Pathway and Its Role in Protection Against Infection. Future Microbiol (2009) 4:1151–65. doi: 10.2217/fmb.09.87
80. Wang T-T, Nestel FP, Bourdeau V, Nagai Y, Wang Q, Liao J, et al. Cutting Edge: 1,25-Dihydroxyvitamin D3 Is a Direct Inducer of Antimicrobial Peptide Gene Expression. J Immunol (2004) 173:2909–12. doi: 10.4049/jimmunol.173.5.2909
81. Shirakawa A-K, Nagakubo D, Hieshima K, Nakayama T, Jin Z, Yoshie O. 1,25-Dihydroxyvitamin D3 Induces CCR10 Expression in Terminally Differentiating Human B Cells. J Immunol (2008) 180:2786–95. doi: 10.4049/jimmunol.180.5.2786
82. Sigmundsdottir H, Pan J, Debes GF, Alt C, Habtezion A, Soler D, et al. DCs Metabolize Sunlight-Induced Vitamin D3 to “Program” T Cell Attraction to the Epidermal Chemokine CCL27. Nat Immunol (2007) 8:285–93. doi: 10.1038/ni1433
83. Baeke F, Korf H, Overbergh L, Verstuyf A, Thorrez L, Van Lommel L, et al. The Vitamin D Analog, TX527, Promotes a Human CD4+CD25highCD127low Regulatory T Cell Profile and Induces a Migratory Signature Specific for Homing to Sites of Inflammation. J Immunol (2011) 186:132–42. doi: 10.4049/jimmunol.1000695
84. Khoo A-L, Koenen HJPM, Michels M, Ooms S, Bosch M, Netea MG, et al. High-Dose Vitamin D3 Supplementation Is a Requisite for Modulation of Skin-Homing Markers on Regulatory T Cells in HIV-Infected Patients. AIDS Res Hum Retroviruses (2013) 29:299–306. doi: 10.1089/AID.2012.0051
85. Briceno Noriega D, Savelkoul HFJ. Vitamin D and Allergy Susceptibility During Gestation and Early Life. Nutrients (2021) 13:1015. doi: 10.3390/nu13031015
86. Berry DJ, Hesketh K, Power C, Hyppönen E. Vitamin D Status has a Linear Association With Seasonal Infections and Lung Function in British Adults. Br J Nutr (2011) 106:1433–40. doi: 10.1017/S0007114511001991
87. Ginde AA, Mansbach JM, Camargo CAJ. Association Between Serum 25-Hydroxyvitamin D Level and Upper Respiratory Tract Infection in the Third National Health and Nutrition Examination Survey. Arch Intern Med (2009) 169:384–90. doi: 10.1001/archinternmed.2008.560
88. Pham H, Rahman A, Majidi A, Waterhouse M, Neale RE. Acute Respiratory Tract Infection and 25-Hydroxyvitamin D Concentration: A Systematic Review and Meta-Analysis. Int J Environ Res Public Health (2019) 16:3020. doi: 10.3390/ijerph16173020
89. Martineau AR, Jolliffe DA, Hooper RL, Greenberg L, Aloia JF, Bergman P, et al. Vitamin D Supplementation to Prevent Acute Respiratory Tract Infections: Systematic Review and Meta-Analysis of Individual Participant Data. BMJ (2017) 356. doi: 10.1136/bmj.i6583
90. Suara RO, Crowe JEJ. Effect of Zinc Salts on Respiratory Syncytial Virus Replication. Antimicrob Agents Chemother (2004) 48:783–90. doi: 10.1128/AAC.48.3.783-790.2004
91. Uchide N, Ohyama K, Bessho T, Yuan B, Yamakawa T. Effect of Antioxidants on Apoptosis Induced by Influenza Virus Infection: Inhibition of Viral Gene Replication and Transcription With Pyrrolidine Dithiocarbamate. Antiviral Res (2002) 56:207–17. doi: 10.1016/s0166-3542(02)00109-2
92. Read SA, Obeid S, Ahlenstiel C, Ahlenstiel G. The Role of Zinc in Antiviral Immunity. Adv Nutr (2019) 10:696–710. doi: 10.1093/advances/nmz013
93. Subramanian Vignesh K, Deepe GSJ. Metallothioneins: Emerging Modulators in Immunity and Infection. Int J Mol Sci (2017) 18:2197. doi: 10.3390/ijms18102197
94. Sadeghsoltani F, Mohammadzadeh I, Safari M-M, Hassanpour P, Izadpanah M, Qujeq D, et al. Zinc and Respiratory Viral Infections: Important Trace Element in Anti-Viral Response and Immune Regulation. Biol Trace Elem Res (2021) 9:1–16. doi: 10.1007/s12011-021-02859-z
95. Barnett JB, Dao MC, Hamer DH, Kandel R, Brandeis G, Wu D, et al. Effect of Zinc Supplementation on Serum Zinc Concentration and T Cell Proliferation in Nursing Home Elderly: A Randomized, Double-Blind, Placebo-Controlled Trial. Am J Clin Nutr (2016) 103:942–51. doi: 10.3945/ajcn.115.115188
96. Wang MX, Win SS, Pang J. Zinc Supplementation Reduces Common Cold Duration Among Healthy Adults: A Systematic Review of Randomized Controlled Trials With Micronutrients Supplementation. Am J Trop Med Hyg (2020) 103:86–99. doi: 10.4269/ajtmh.19-0718
97. Rouhani P, Rezaei Kelishadi M, Saneei P. Effect of Zinc Supplementation on Mortality in Under 5-Year Children: A Systematic Review and Meta-Analysis of Randomized Clinical Trials. Eur J Nutr (2022) 61:37–54 doi: 10.1007/s00394-021-02604-1
98. Hunter J, Arentz S, Goldenberg J, Yang G, Beardsley J, Myers SP, et al. Zinc for the Prevention or Treatment of Acute Viral Respiratory Tract Infections in Adults: A Rapid Systematic Review and Meta-Analysis of Randomised Controlled Trials. BMJ Open (2021) 11:e047474. doi: 10.1136/bmjopen-2020-047474
99. Pfeifhofer-Obermair C, Tymoszuk P, Nairz M, Schroll A, Klais G, Demetz E, et al. Regulation of Th1 T Cell Differentiation by Iron via Upregulation of T Cell Immunoglobulin and Mucin Containing Protein-3 (TIM-3). Front Immunol (2021) 12:637809:637809. doi: 10.3389/fimmu.2021.637809
100. Oppenheimer SJ. Iron and its Relation to Immunity and Infectious Disease. J Nutr (2001) 131:616S–35S. doi: 10.1093/jn/131.2.616S
101. Weiss G. Iron and Immunity: A Double-Edged Sword. Eur J Clin Invest (2002) 32:70–8. doi: 10.1046/j.1365-2362.2002.0320s1070.x
102. Ahluwalia N, Sun J, Krause D, Mastro A, Handte G. Immune Function Is Impaired in Iron-Deficient, Homebound, Older Women. Am J Clin Nutr (2004) 79:516–21. doi: 10.1093/ajcn/79.3.516
103. Nairz M, Dichtl S, Schroll A, Haschka D, Tymoszuk P, Theurl I, et al. Iron and Innate Antimicrobial Immunity-Depriving the Pathogen, Defending the Host. J Trace Elem Med Biol Organ Soc Miner Trace Elem (2018) 48:118–33. doi: 10.1016/j.jtemb.2018.03.007
104. Drakesmith H, Prentice AM. Hepcidin and the Iron-Infection Axis. Science (2012) 338:768–72. doi: 10.1126/science.1224577
105. Nairz M, Theurl I, Swirski FK, Weiss G. “Pumping Iron”-How Macrophages Handle Iron at the Systemic, Microenvironmental, and Cellular Levels. Pflugers Arch (2017) 469:397–418. doi: 10.1007/s00424-017-1944-8
106. Ganz T, Nemeth E. Iron Homeostasis in Host Defence and Inflammation. Nat Rev Immunol (2015) 15:500–10. doi: 10.1038/nri3863
107. Schaible UE, Kaufmann SHE. Iron and Microbial Infection. Nat Rev Microbiol (2004) 2:946–53. doi: 10.1038/nrmicro1046
108. Cherayil BJ. Iron and Immunity: Immunological Consequences of Iron Deficiency and Overload. Arch Immunol Ther Exp (Warsz) (2010) 58:407–15. doi: 10.1007/s00005-010-0095-9
109. Kumar V, Choudhry VP. Iron Deficiency and Infection. Indian J Pediatr (2010) 77:789–93. doi: 10.1007/s12098-010-0120-3
110. Weinberg ED. Antibiotic Properties and Applications of Lactoferrin. Curr Pharm Des (2007) 13:801–11. doi: 10.2174/138161207780363095
111. Huang Z, Rose AH, Hoffmann PR. The Role of Selenium in Inflammation and Immunity: From Molecular Mechanisms to Therapeutic Opportunities. Antioxidants Redox Signal (2012) 16:705–43. doi: 10.1089/ars.2011.4145
112. Avery JC, Hoffmann PR. Selenium, Selenoproteins, and Immunity. Nutrients (2018) 10:1203. doi: 10.3390/nu10091203
113. Guillin OM, Vindry C, Ohlmann T, Chavatte L. Selenium, Selenoproteins and Viral Infection. Nutrients (2019) 11:2101. doi: 10.3390/nu11092101
114. Zhang J, Saad R, Taylor EW, Rayman MP. Selenium and Selenoproteins in Viral Infection With Potential Relevance to COVID-19. Redox Biol (2020) 37:101715. doi: 10.1016/j.redox.2020.101715
115. Hiffler L, Rakotoambinina B. Selenium and RNA Virus Interactions: Potential Implications for SARS-CoV-2 Infection (COVID-19). Front Nutr (2020) 7:164. doi: 10.3389/fnut.2020.00164
116. Beck MA, Handy J, Levander OA. Host Nutritional Status: The Neglected Virulence Factor. Trends Microbiol (2004) 12:417–23. doi: 10.1016/j.tim.2004.07.007
117. Beck MA, Nelson HK, Shi Q, Van Dael P, Schiffrin EJ, Blum S, et al. Selenium Deficiency Increases the Pathology of an Influenza Virus Infection. FASEB J (2001) 15:1481–3. doi: 10.1096/fj.00-0721fje
118. Nelson HK, Shi Q, Van Dael P, Schiffrin EJ, Blum S, Barclay D, et al. Host Nutritional Selenium Status as a Driving Force for Influenza Virus Mutations. FASEB J (2001) 15:1727–38. doi: 10.1096/fj.01-0108com
119. Khatiwada S, Subedi A. A Mechanistic Link Between Selenium and Coronavirus Disease 2019 (COVID-19). Curr Nutr Rep (2021) 10:125–36. doi: 10.1007/s13668-021-00354-4
120. Broome CS, McArdle F, Kyle JAM, Andrews F, Lowe NM, Hart CA, et al. An Increase in Selenium Intake Improves Immune Function and Poliovirus Handling in Adults With Marginal Selenium Status. Am J Clin Nutr (2004) 80:154–62. doi: 10.1093/ajcn/80.1.154
121. van Esch BCAM, Porbahaie M, Abbring S, Garssen J, Potaczek DP, Savelkoul HFJ, et al. The Impact of Milk and Its Components on Epigenetic Programming of Immune Function in Early Life and Beyond: Implications for Allergy and Asthma. Front Immunol (2020) 11:2141. doi: 10.3389/fimmu.2020.02141
122. van Splunter M, van Osch TLJ, Brugman S, Savelkoul HFJ, Joosten LAB, Netea MG, et al. Induction of Trained Innate Immunity in Human Monocytes by Bovine Milk and Milk-Derived Immunoglobulin G. Nutrients (2018) 10:1378. doi: 10.3390/nu10101378
123. Perdijk O, van Splunter M, Savelkoul HFJ, Brugman S, van Neerven RJJ. Cow’s Milk and Immune Function in the Respiratory Tract: Potential Mechanisms. Front Immunol (2018) 9:143. doi: 10.3389/fimmu.2018.00143
124. Chatterton DEW, Nguyen DN, Bering SB, Sangild PT. Anti-Inflammatory Mechanisms of Bioactive Milk Proteins in the Intestine of Newborns. Int J Biochem Cell Biol (2013) 45:1730–47. doi: 10.1016/j.biocel.2013.04.028
125. Verhasselt V. Neonatal Tolerance Under Breastfeeding Influence. Curr Opin Immunol (2010) 22:623–30. doi: 10.1016/j.coi.2010.08.008
126. Abbring S, Hols G, Garssen J, van Esch BCAM. Raw Cow’s Milk Consumption and Allergic Diseases - The Potential Role of Bioactive Whey Proteins. Eur J Pharmacol (2019) 843:55–65. doi: 10.1016/j.ejphar.2018.11.013
127. Den Hartog G, Jacobino S, Bont L, Cox L, Ulfman LH, Leusen JHW, et al. Specificity and Effector Functions of Human RSV-Specific IgG From Bovine Milk. PloS One (2014) 9:1–8. doi: 10.1371/journal.pone.0112047
128. Ulfman LH, Leusen JHW, Savelkoul HFJ, Warner JO, van Neerven RJJ. Effects of Bovine Immunoglobulins on Immune Function, Allergy, and Infection. Front Nutr (2018) 5:52. doi: 10.3389/fnut.2018.00052
129. Triantis V, Bode L, van Neerven RJJ. Immunological Effects of Human Milk Oligosaccharides. Front Pediatr (2018) 6:190. doi: 10.3389/fped.2018.00190
130. Dawod B, Marshall JS, Azad MB. Breastfeeding and the Developmental Origins of Mucosal Immunity: How Human Milk Shapes the Innate and Adaptive Mucosal Immune Systems. Curr Opin Gastroenterol (2021) 37:547–56. doi: 10.1097/MOG.0000000000000778
131. van Neerven RJJ, Knol EF, Heck JML, Savelkoul HFJ. Which Factors in Raw Cow’s Milk Contribute to Protection Against Allergies? J Allergy Clin Immunol (2012) 130:853–8. doi: 10.1016/j.jaci.2012.06.050
132. Duijts L, Ramadhani MK, Moll HA. Breastfeeding Protects Against Infectious Diseases During Infancy in Industrialized Countries. A Systematic Review. MaternChildNutr (2009) 5:199–210. doi: 10.1111/j.1740-8709.2008.00176.x
133. Sankar MJ, Sinha B, Chowdhury R, Bhandari N, Taneja S, Martines J, et al. Optimal Breastfeeding Practices and Infant and Child Mortality: A Systematic Review and Meta-Analysis. Acta Paediatr (2015) 104:3–13. doi: 10.1111/apa.13147
134. Lamberti LM, Zakarija-Grković I, Fischer Walker CL, Theodoratou E, Nair H, Campbell H, et al. Breastfeeding for Reducing the Risk of Pneumonia Morbidity and Mortality in Children Under Two: A Systematic Literature Review and Meta-Analysis. BMC Public Health (2013) 13:S18. doi: 10.1186/1471-2458-13-S3-S18
135. Vassilopoulou E, Feketea G, Koumbi L, Mesiari C, Berghea EC, Konstantinou GN. Breastfeeding and COVID-19: From Nutrition to Immunity. Front Immunol (2021) 12:661806:661806. doi: 10.3389/fimmu.2021.661806
136. Harvey SM, Murphy VE, Whalen OM, Gibson PG, Jensen ME. Breastfeeding and Wheeze-Related Outcomes in High-Risk Infants: A Systematic Review and Meta-Analysis. Am J Clin Nutr (2021) 113:1609–18. doi: 10.1093/ajcn/nqaa442
137. Riedler J, Braun-Fahrl+ñnder C, Eder W, Schreuer M, Waser M, Maisch S, et al. Exposure to Farming in Early Life and Development of Asthma and Allergy: A Cross-Sectional Survey. Lancet (2001) 358:1129–33. doi: 10.1016/S0140-6736(01)06252-3
138. Waser M, Michels KB, Bieli C, Flöistrup H, Pershagen G, von Mutius E, et al. Inverse Association of Farm Milk Consumption With Asthma and Allergy in Rural and Suburban Populations Across Europe. Clin Exp Allergy (2007) 37:661–70. doi: 10.1111/j.1365-2222.2006.02640.x
139. Brick T, Hettinga K, Kirchner B, Pfaffl MW, Ege MJ. The Beneficial Effect of Farm Milk Consumption on Asthma, Allergies, and Infections: From Meta-Analysis of Evidence to Clinical Trial. J Allergy Clin Immunol Pract (2020) 8:878–889.e3. doi: 10.1016/j.jaip.2019.11.017
140. Loss G, Depner M, Ulfman LH, Van Neerven RJJ, Hose AJ, Genuneit J, et al. Consumption of Unprocessed Cow’s Milk Protects Infants From Common Respiratory Infections. J Allergy Clin Immunol (2015) 135:56–62. doi: 10.1016/j.jaci.2014.08.044
141. Loss G, Apprich S, Waser M, Kneifel W, Genuneit J, Büchele G, et al. The Protective Effect of Farm Milk Consumption on Childhood Asthma and Atopy: The GABRIELA Study. J Allergy Clin Immunol (2011) 128:766–773.e4. doi: 10.1016/j.jaci.2011.07.048
142. Sozańska B, Pearce N, Dudek K, Cullinan P. Consumption of Unpasteurized Milk and Its Effects on Atopy and Asthma in Children and Adult Inhabitants in Rural Poland. Allergy (2013) 68:644–50. doi: 10.1111/all.12147
143. Ng TB, Chi R, Cheung F, Wong JH, Wang Y, Tsz D, et al. Antiviral Activities of Whey Proteins. Appl Microbiol Biotechnol (2015) 99:6997–7008. doi: 10.1007/s00253-015-6818-4
144. Perkin MR, Strachan DP. Original Articles Which Aspects of the Farming Lifestyle Explain the Inverse Association With Childhood Allergy? J Allergy Clin Immunol (2006) 117:1374–81. doi: 10.1016/j.jaci.2006.03.008
145. Von Mutius E, Vercelli D. Farm Living: Effects on Childhood Asthma and Allergy. Nat Publ Gr (2010) 10:861–8. doi: 10.1038/nri2871
146. Sozańska B. Raw Cow’s Milk and Its Protective Effect on Allergies and Asthma. Nutrients (2019) 11:469. doi: 10.3390/nu11020469
147. Nederend M, van Stigt AH, Jansen JHM, Jacobino SR, Brugman S, de Haan CAM, et al. Bovine IgG Prevents Experimental Infection With RSV and Facilitates Human T Cell Responses to RSV. Front Immunol (2020) 11:1701. doi: 10.3389/fimmu.2020.01701
148. den Hartog G, Savelkoul HF, Schoemaker R, Tijhaar E, Westphal AH, de Ruiter T, et al. Modulation of Human Immune Responses by Bovine Interleukin-10. PloS One (2011) 6:e18188. doi: 10.1371/journal.pone.0018188
149. Perdijk O, van Neerven RJJ, van den Brink E, Savelkoul HFJ, Brugman S. Bovine Lactoferrin Modulates Dendritic Cell Differentiation and Function. Nutrients (2018) 10:848. doi: 10.3390/nu10070848
150. Lin X, O’Reilly KL, Burrell ML, Storz J. Infectivity-Neutralizing and Hemagglutinin-Inhibiting Antibody Responses to Respiratory Coronavirus Infections of Cattle in Pathogenesis of Shipping Fever Pneumonia. Clin Diagn Lab Immunol (2001) 8:357–62. doi: 10.1128/CDLI.8.2.357-362.2001
151. Toftaker I, Sanchez J, Stokstad M, Nødtvedt A. Bovine Respiratory Syncytial Virus and Bovine Coronavirus Antibodies in Bulk Tank Milk - Risk Factors and Spatial Analysis. Prev Vet Med (2016) 133:73–83. doi: 10.1016/j.prevetmed.2016.09.003
152. Toftaker I, Toft N, Stokstad M, Sølverød L, Harkiss G, Watt N, et al. Evaluation of a Multiplex Immunoassay for Bovine Respiratory Syncytial Virus and Bovine Coronavirus Antibodies in Bulk Tank Milk Against Two Indirect ELISAs Using Latent Class Analysis. Prev Vet Med (2018) 154:1–8. doi: 10.1016/j.prevetmed.2018.03.008
153. Pullen KM, Atyeo C, Collier A-RY, Gray KJ, Belfort MB, Lauffenburger DA, et al. Selective Functional Antibody Transfer Into the Breastmilk After SARS-CoV-2 Infection. Cell Rep (2021) 37:109959. doi: 10.1016/j.celrep.2021.109959
154. Gonçalves J, Juliano AM, Charepe N, Alenquer M, Athayde D, Ferreira F, et al. Secretory IgA and T Cells Targeting SARS-CoV-2 Spike Protein are Transferred to the Breastmilk Upon mRNA Vaccination. Cell Rep Med (2021) 2:100468. doi: 10.1016/j.xcrm.2021.100468
155. Hellinga AH, Tsallis T, Eshuis T, Triantis V, Ulfman LH, van Neerven RJJ. In Vitro Induction of Trained Innate Immunity by Bigg and Whey Protein Extracts. Int J Mol Sci (2020) 21:9077. doi: 10.3390/ijms21239077
156. van Splunter M, Perdijk O, Fick-Brinkhof H, Feitsma AL, Floris-Vollenbroek EG, Meijer B, et al. Bovine Lactoferrin Enhances TLR7-Mediated Responses in Plasmacytoid Dendritic Cells in Elderly Women: Results From a Nutritional Intervention Study With Bovine Lactoferrin, GOS and Vitamin D. Front Immunol (2018) 9:2677. doi: 10.3389/fimmu.2018.02677
157. Valenti P, Antonini G. Lactoferrin: An Important Host Defence Against Microbial and Viral Attack. Cell Mol Life Sci (2005) 62:2576–87. doi: 10.1007/s00018-005-5372-0
158. Ali AS, Hasan SS, Kow CS, Merchant HA. Lactoferrin Reduces the Risk of Respiratory Tract Infections: A Meta-Analysis of Randomized Controlled Trials. Clin Nutr ESPEN (2021) 45:26–32. doi: 10.1016/j.clnesp.2021.08.019
159. Lang J, Yang N, Deng J, Liu K, Yang P, Zhang G, et al. Inhibition of SARS Pseudovirus Cell Entry by Lactoferrin Binding to Heparan Sulfate Proteoglycans. PloS One (2011) 6:e23710. doi: 10.1371/journal.pone.0023710
160. AlKhazindar M, Elnagdy SM. Can Lactoferrin Boost Human Immunity Against COVID-19? Pathog Glob Health (2020) 114:234–5. doi: 10.1080/20477724.2020.1779514
161. Campione E, Cosio T, Rosa L, Lanna C, Di Girolamo S, Gaziano R, et al. Lactoferrin as Protective Natural Barrier of Respiratory and Intestinal Mucosa Against Coronavirus Infection and Inflammation. Int J Mol Sci (2020) 21:4903. doi: 10.3390/ijms21144903
162. Sugita C, Shin K, Wakabayashi H, Tsuhako R, Yoshida H, Watanabe W, et al. Antiviral Activity of Hypothiocyanite Produced by Lactoperoxidase Against Influenza A and B Viruses and Mode of its Antiviral Action. Acta Virol (2018) 62:401–8. doi: 10.4149/av_2018_408
163. Sabha BH, Alzahrani F, Almehdar HA, Uversky VN, Redwan EM. Disorder in Milk Proteins: Lactadherin Multifunctionality and Structure. Curr Protein Pept Sci (2018) 19:983–97. doi: 10.2174/1389203719666180608091849
164. Duijts L, Jaddoe VWV, Hofman A, Moll HA. Prolonged and Exclusive Breastfeeding Reduces the Risk of Infectious Diseases in Infancy. Pediatrics (2010) 126:e18–25. doi: 10.1542/peds.2008-3256
165. Stepans MBF, Hertzog M, Kim Callahan Rodehorst T, Blaney S, Clemens B, Polak JJ, et al. Early Consumption of Human Milk Oligosaccharides Is Inversely Related to Subsequent Risk of Respiratory and Enteric Disease in Infants. Breastfeed Med (2006) 1:207–15. doi: 10.1089/bfm.2006.1.207
166. Lei W-T, Shih P-C, Liu S-J, Lin C-Y, Yeh T-L. Effect of Probiotics and Prebiotics on Immune Response to Influenza Vaccination in Adults: A Systematic Review and Meta-Analysis of Randomized Controlled Trials. Nutrients (2017) 9:1175. doi: 10.3390/nu9111175
167. Nagafuchi S, Yamaji T, Kawashima A, Saito Y, Takahashi T, Yamamoto T, et al. Effects of a Formula Containing Two Types of Prebiotics, Bifidogenic Growth Stimulator and Galacto-Oligosaccharide, and Fermented Milk Products on Intestinal Microbiota and Antibody Response to Influenza Vaccine in Elderly Patients: A Randomized Controll. Pharmaceuticals (Basel) (2015) 8:351–65. doi: 10.3390/ph8020351
168. Schijf MA, Kruijsen D, Bastiaans J, Coenjaerts FE, Garssen J, van Bleek GM, et al. Specific Dietary Oligosaccharides Increase Th1 Responses in a Mouse Respiratory Syncytial Virus Infection Model. J Virol (2012) 86:11472–82. doi: 10.1128/JVI.06708-11
169. van den Elsen LWJ, Tims S, Jones AM, Stewart A, Stahl B, Garssen J, et al. Prebiotic Oligosaccharides in Early Life Alter Gut Microbiome Development in Male Mice While Supporting Influenza Vaccination Responses. Benef Microbes (2019) 10:279–91. doi: 10.3920/BM2018.0098
170. Xiao L, Leusink-Muis T, Kettelarij N, van Ark I, Blijenberg B, Hesen NA, et al. Human Milk Oligosaccharide 2’-Fucosyllactose Improves Innate and Adaptive Immunity in an Influenza-Specific Murine Vaccination Model. Front Immunol (2018) 9:452:452. doi: 10.3389/fimmu.2018.00452
171. Yeh T-L, Shih P-C, Liu S-J, Lin C-H, Liu J-M, Lei W-T, et al. The Influence of Prebiotic or Probiotic Supplementation on Antibody Titers After Influenza Vaccination: A Systematic Review and Meta-Analysis of Randomized Controlled Trials. Drug Des Devel Ther (2018) 12:217–30. doi: 10.2147/DDDT.S155110
172. Perdijk O, van Baarlen P, Fernandez-Gutierrez MM, van den Brink E, Schuren FHJ, Brugman S, et al. Sialyllactose and Galactooligosaccharides Promote Epithelial Barrier Functioning and Distinctly Modulate Microbiota Composition and Short Chain Fatty Acid Production In Vitro. Front Immunol (2019) 10:94. doi: 10.3389/fimmu.2019.00094
173. Bode L. Human Milk Oligosaccharides: Every Baby Needs a Sugar Mama. Glycobiology (2012) 22:1147–62. doi: 10.1093/glycob/cws074
174. Zhu W, Wu Y, Liu H, Jiang C, Huo L. Gut-Lung Axis: Microbial Crosstalk in Pediatric Respiratory Tract Infections. Front Immunol (2021) 12:741233:741233. doi: 10.3389/fimmu.2021.741233
175. Eribo OA, du Plessis N, Chegou NN. The Intestinal Commensal, Bacteroides Fragilis, Modulates Host Responses to Viral Infection and Therapy: Lessons for Exploration During Mycobacterium Tuberculosis Infection. Infect Immun (2021) 90:IAI0032121. doi: 10.1128/IAI.00321-21
176. Ichinohe T, Pang IK, Kumamoto Y, Peaper DR, Ho JH, Murray TS, et al. Microbiota Regulates Immune Defense Against Respiratory Tract Influenza A Virus Infection. Proc Natl Acad Sci USA (2011) 108:5354–9. doi: 10.1073/pnas.1019378108
177. Yitbarek A, Alkie T, Taha-Abdelaziz K, Astill J, Rodriguez-Lecompte JC, Parkinson J, et al. Gut Microbiota Modulates Type I Interferon and Antibody-Mediated Immune Responses in Chickens Infected With Influenza Virus Subtype H9N2. Benef Microbes (2018) 9:417–27. doi: 10.3920/BM2017.0088
178. Dogra SK, Martin F-P, Donnicola D, Julita M, Berger B, Sprenger N. Human Milk Oligosaccharide-Stimulated Bifidobacterium Species Contribute to Prevent Later Respiratory Tract Infections. Microorganisms (2021) 9:1939. doi: 10.3390/microorganisms9091939
179. de Steenhuijsen Piters WAA, Binkowska J, Bogaert D. Early Life Microbiota and Respiratory Tract Infections. Cell Host Microbe (2020) 28:223–32. doi: 10.1016/j.chom.2020.07.004
180. Unger SA, Bogaert D. The Respiratory Microbiome and Respiratory Infections. J Infect (2017) 74:S84–8. doi: 10.1016/S0163-4453(17)30196-2
181. Bisgaard H, Hermansen MN, Buchvald F, Loland L, Halkjaer LB, Bonnelykke K, et al. Childhood Asthma After Bacterial Colonization of the Airway in Neonates. N Engl J Med (2007) 357:1487–95. doi: 10.1056/NEJMoa052632
182. Biesbroek G, Bosch AATM, Wang X, Keijser BJF, Veenhoven RH, Sanders EAM, et al. The Impact of Breastfeeding on Nasopharyngeal Microbial Communities in Infants. Am J Respir Crit Care Med (2014) 190:298–308. doi: 10.1164/rccm.201401-0073OC
183. Biesbroek G, Tsivtsivadze E, Sanders EAM, Montijn R, Veenhoven RH, Keijser BJF, et al. Early Respiratory Microbiota Composition Determines Bacterial Succession Patterns and Respiratory Health in Children. Am J Respir Crit Care Med (2014) 190:1283–92. doi: 10.1164/rccm.201407-1240OC
184. Goehring KC, Kennedy AD, Prieto PA, Buck RH. Direct Evidence for the Presence of Human Milk Oligosaccharides in the Circulation of Breastfed Infants. PloS One (2014) 9:e101692. doi: 10.1371/journal.pone.0101692
185. Vazquez E, Santos-Fandila A, Buck R, Rueda R, Ramirez M. Major Human Milk Oligosaccharides Are Absorbed Into the Systemic Circulation After Oral Administration in Rats. Br J Nutr (2017) 117:237–47. doi: 10.1017/S0007114516004554
186. Bloemen JG, Venema K, van de Poll MC, Olde Damink SW, Buurman WA. Dejong CH. Short Chain Fatty Acids Exchange Across the Gut and Liver in Humans Measured at Surgery. Clin Nutr (2009) 28:657–61. doi: 10.1016/j.clnu.2009.05.011
187. Boets E, Gomand SV, Deroover L, Preston T, Vermeulen K, De Preter V, et al. Systemic Availability and Metabolism of Colonic-Derived Short-Chain Fatty Acids in Healthy Subjects : A Stable Isotope Study. J Physiol (2017) 2:541–55. doi: 10.1113/JP272613
188. Lomax AR, Calder PC. Prebiotics, Immune Function, Infection and Inflammation: A Review of the Evidence. Br J Nutr (2009) 101:633–58. doi: 10.1017/S0007114508055608
189. Goverse G, Molenaar R, Macia L, Tan J, Erkelens MN, Konijn T, et al. Diet-Derived Short Chain Fatty Acids Stimulate Intestinal Epithelial Cells To Induce Mucosal Tolerogenic Dendritic Cells. J Immunol (2017) 198:2172–81. doi: 10.4049/jimmunol.1600165
190. Tan J, McKenzie C, Vuillermin PJ, Goverse G, Vinuesa CG, Mebius RE, et al. Dietary Fiber and Bacterial SCFA Enhance Oral Tolerance and Protect Against Food Allergy Through Diverse Cellular Pathways. Cell Rep (2016) 15:2809–24. doi: 10.1016/j.celrep.2016.05.047
191. Trompette A, Gollwitzer ES, Yadava K, Sichelstiel AK, Sprenger N, Ngom-Bru C, et al. Gut Microbiota Metabolism of Dietary Fiber Influences Allergic Airway Disease and Hematopoiesis. Nat Med (2014) 20:159–66. doi: 10.1038/nm.3444
192. Trompette A, Gollwitzer ES, Pattaroni C, Lopez-Mejia IC, Riva E, Pernot J, et al. Dietary Fiber Confers Protection Against Flu by Shaping Ly6c(-) Patrolling Monocyte Hematopoiesis and CD8(+) T Cell Metabolism. Immunity (2018) 48:992–1005.e8. doi: 10.1016/j.immuni.2018.04.022
193. Machado MG, Sencio V, Trottein F. Short-Chain Fatty Acids as a Potential Treatment for Infections: A Closer Look at the Lungs. Infect Immun (2021) 89:e0018821. doi: 10.1128/IAI.00188-21
194. Groves HT, Higham SL, Moffatt MF, Cox MJ, Tregoning JS. Respiratory Viral Infection Alters the Gut Microbiota by Inducing Inappetence. MBio (2020) 11:e03236–19. doi: 10.1128/mBio.03236-19
195. Sencio V, Barthelemy A, Tavares LP, Machado MG, Soulard D, Cuinat C, et al. Gut Dysbiosis During Influenza Contributes to Pulmonary Pneumococcal Superinfection Through Altered Short-Chain Fatty Acid Production. Cell Rep (2020) 30:2934–47.e6. doi: 10.1016/j.celrep.2020.02.013
196. Zevgiti S, Zabala JG, Darji A, Dietrich U, Panou-Pomonis E, Sakarellos-Daitsiotis M. Sialic Acid and Sialyl-Lactose Glyco-Conjugates: Design, Synthesis and Binding Assays to Lectins and Swine Influenza H1N1 Virus. J Pept Sci (2012) 18:52–8. doi: 10.1002/psc.1415
197. Kwon SJ, Na DH, Kwak JH, Douaisi M, Zhang F, Park EJ, et al. Nanostructured Glycan Architecture Is Important in the Inhibition of Influenza A Virus Infection. Nat Nanotechnol (2017) 12:48–54. doi: 10.1038/nnano.2016.181
198. Idänpään-Heikkilä I, Simon PM, Zopf D, Vullo T, Cahill P, Sokol K, et al. Oligosaccharides Interfere With the Establishment and Progression of Experimental Pneumococcal Pneumonia. J Infect Dis (1997) 176:704–12. doi: 10.1086/514094
199. Wise A, Robertson B, Choudhury B, Rautava S, Isolauri E. Infants Are Exposed to Human Milk Oligosaccharides Already In Utero. Front Pediatr (2018) 6:1–4. doi: 10.3389/fped.2018.00270
200. Jacobino S, Nederendm M, Hennus M, Houben ML, Ngwuta JO, Viveen M, et al. Human Amniotic Fluid Antibodies Protect the Neonate Against Respiratory Syncytial Virus Infection. J Allergy Clin Immunol (2016) 138:1477–480.e5. doi: 10.1016/j.jaci.2016.06.001
201. Arslanoglu S, Moro GE, Boehm G. Early Supplementation of Prebiotic Oligosaccharides Protects Formula-Fed Infants Against Infections During the First 6 Months of Life. J Nutr (2007) 137:2420–4. doi: 10.1093/jn/137.11.2420
202. Weizman Z. The Role of Probiotics and Prebiotics in the Prevention of Infections in Child Day-Care Centres. Benef Microbes (2015) 6:181–3. doi: 10.3920/BM2014.0101
203. Ranucci G, Buccigrossi V, Borgia E, Piacentini D, Visentin F, Cantarutti L, et al. Galacto-Oligosaccharide/Polidextrose Enriched Formula Protects Against Respiratory Infections in Infants at High Risk of Atopy: A Randomized Clinical Trial. Nutrients (2018) 10:286. doi: 10.3390/nu10030286
204. Bruzzese E, Volpicelli M, Squeglia V, Bruzzese D, Salvini F, Bisceglia M, et al. A Formula Containing Galacto- and Fructo-Oligosaccharides Prevents Intestinal and Extra-Intestinal Infections: An Observational Study. Clin Nutr (2009) 28:156–61. doi: 10.1016/j.clnu.2009.01.008
205. Luoto R, Ruuskanen O, Waris M, Kalliomäki M, Salminen S, Isolauri E. Prebiotic and Probiotic Supplementation Prevents Rhinovirus Infections in Preterm Infants: A Randomized, Placebo-Controlled Trial. J Allergy Clin Immunol (2014) 133:405–13. doi: 10.1016/j.jaci.2013.08.020
206. Hughes C, Davoodi-Semiromi Y, Colee JC, Culpepper T, Dahl WJ, Mai V, et al. Galactooligosaccharide Supplementation Reduces Stress-Induced Gastrointestinal Dysfunction and Days of Cold or Flu: A Randomized, Double-Blind, Controlled Trial in Healthy University Students. Am J Clin Nutr (2011) 93:1305–11. doi: 10.3945/ajcn.111.014126.INTRODUCTION
207. Puccio G, Alliet P, Cajozzo C, Janssens E, Corsello G, Sprenger N, et al. Effects of Infant Formula With Human Milk Oligosaccharides on Growth and Morbidity: A Randomized Multicenter Trial. J Pediatr Gastroenterol Nutr (2017) 64:624–31. doi: 10.1097/MPG.0000000000001520
208. van Steenwijk HP, Bast A, de Boer A. Immunomodulating Effects of Fungal Beta-Glucans: From Traditional Use to Medicine. Nutrients (2021) 13:1333. doi: 10.3390/nu13041333
209. Ferreira SS, Passos CP, Madureira P, Vilanova M, Coimbra MA. Structure-Function Relationships of Immunostimulatory Polysaccharides: A Review. Carbohydr Polym (2015) 132:378–96. doi: 10.1016/j.carbpol.2015.05.079
210. Jin Y, Li P, Wang F. Beta-Glucans as Potential Immunoadjuvants: A Review on the Adjuvanticity, Structure-Activity Relationship and Receptor Recognition Properties. Vaccine (2018) 36:5235–44. doi: 10.1016/j.vaccine.2018.07.038
211. Bergendiova K, Tibenska E, Majtan J. Pleuran (Beta-Glucan From Pleurotus Ostreatus) Supplementation, Cellular Immune Response and Respiratory Tract Infections in Athletes. Eur J Appl Physiol (2011) 111:2033–40. doi: 10.1007/s00421-011-1837-z
212. Fuller R, Moore MV, Lewith G, Stuart BL, Ormiston RV, Fisk HL, et al. Yeast-Derived Beta-1,3/1,6 Glucan, Upper Respiratory Tract Infection and Innate Immunity in Older Adults. Nutrition (2017) 39–40:30–5. doi: 10.1016/j.nut.2017.03.003
213. Mah E, Kaden VN, Kelley KM, Liska DJ. Soluble and Insoluble Yeast Beta-Glucan Differentially Affect Upper Respiratory Tract Infection in Marathon Runners: A Double-Blind, Randomized Placebo-Controlled Trial. J Med Food (2020) 23:416–9. doi: 10.1089/jmf.2019.0076
214. Laue C, Stevens Y, van Erp M, Papazova E, Soeth E, Pannenbeckers A, et al. Adjuvant Effect of Orally Applied Preparations Containing Non-Digestible Polysaccharides on Influenza Vaccination in Healthy Seniors: A Double-Blind, Randomised, Controlled Pilot Trial. Nutrients (2021) 13:2683. doi: 10.3390/nu13082683
215. Nieman DC. Risk of Upper Respiratory Tract Infection in Athletes: An Epidemiologic and Immunologic Perspective. J Athl Train (1997) 32:344–9.
216. Talbott S, Talbott J. Effect of BETA 1, 3/1, 6 GLUCAN on Upper Respiratory Tract Infection Symptoms and Mood State in Marathon Athletes. J Sports Sci Med (2009) 8:509–15.
217. Talbott SM, Talbott JA. Baker’s Yeast Beta-Glucan Supplement Reduces Upper Respiratory Symptoms and Improves Mood State in Stressed Women. J Am Coll Nutr (2012) 31:295–300. doi: 10.1080/07315724.2012.10720441
218. Dharsono T, Rudnicka K, Wilhelm M, Schoen C. Effects of Yeast (1,3)-(1,6)-Beta-Glucan on Severity of Upper Respiratory Tract Infections: A Double-Blind, Randomized, Placebo-Controlled Study in Healthy Subjects. J Am Coll Nutr (2019) 38:40–50. doi: 10.1080/07315724.2018.1478339
219. Fuller R, Butt H, Noakes PS, Kenyon J, Yam TS, Calder PC. Influence of Yeast-Derived 1,3/1,6 Glucopolysaccharide on Circulating Cytokines and Chemokines With Respect to Upper Respiratory Tract Infections. Nutrition (2012) 28:665–9. doi: 10.1016/j.nut.2011.11.012
220. Scaglione F, Ferrara F, Dugnani S, Falchi M, Santoro G, Fraschini F. Immunomodulatory Effects of Two Extracts of Panax Ginseng C.A. Meyer. Drugs Exp Clin Res (1990) 16:537–42.
221. Predy GN, Goel V, Lovlin RE, Basu T. Immune Modulating Effects of Daily Supplementation of COLD-fX (a Proprietary Extract of North American Ginseng) in Healthy Adults. J Clin Biochem Nutr (2006) 39:162–7. doi: 10.3164/jcbn.39.162
222. Scaglione F, Cogo R CMC, Beretta A. Immunomodulatory Effects of Panax Ginseng C.A. Meyer (G115) on Alveolar Macrophages From Patients Suffering With Chronic Bronchitis. Int J Immunother (1994) 10:21–4.
223. Predy GN, Goel V, Lovlin R, Donner A, Stitt L, Basu TK. Efficacy of an Extract of North American Ginseng Containing Poly-Furanosyl-Pyranosyl-Saccharides for Preventing Upper Respiratory Tract Infections: A Randomized Controlled Trial. CMAJ (2005) 173:1043–8. doi: 10.1503/cmaj.1041470
224. McElhaney JE, Gravenstein S, Cole SK, Davidson E, O’neill D, Petitjean S, et al. A Placebo-Controlled Trial of a Proprietary Extract of North American Ginseng (CVT-E002) to Prevent Acute Respiratory Illness in Institutionalized Older Adults. J Am Geriatr Soc (2004) 52:13–9. doi: 10.1111/j.1532-5415.2004.52004.x
225. Wu D, Zheng J, Mao G, Hu W, Ye X, Linhardt RJ, et al. Rethinking the Impact of RG-I Mainly From Fruits and Vegetables on Dietary Health. Crit Rev Food Sci Nutr (2020) 60:2938–60. doi: 10.1080/10408398.2019.1672037
226. McKay S, Oranje P, Helin J, Koek JH, Kreijveld E, van den Abbeele P, et al. Development of an Affordable, Sustainable and Efficacious Plant-Based Immunomodulatory Food Ingredient Based on Bell Pepper or Carrot RG-I Pectic Polysaccharides. Nutrients (2021) 13:963. doi: 10.3390/nu13030963
227. Lutter R, Teitsma-Jansen A, Floris E, Lone-Latif S, Ravi A, Pineros YS, et al. The Dietary Intake of Carrot-Derived Rhamnogalacturonan-I Accelerates and Augments the Innate Immune and Anti-Viral Interferon Response to Rhinovirus Infection and Reduces Duration and Severity of Symptoms in Humans in a Randomized Trial. Nutrients (2021) 13:4395. doi: 10.3390/nu13124395
228. Albers R, Michel Antoine J-M, Bourdet-Sicard RR, Calder PC, Gleeson M, Lesourd B, et al. Markers to Measure Immunomodulation in Human Nutrition Intervention Studies. Br J Nutr (2005) 94:452. doi: 10.1079/BJN20051469
229. Albers R, Bourdet-Sicard R, Braun D, Calder PC, Herz U, Lambert C, et al. Monitoring Immune Modulation by Nutrition in the General Population: Identifying and Substantiating Effects on Human Health. Br J Nutr (2013) 110:S1–30. doi: 10.1017/S0007114513001505
230. Aouadi M, Tesz GJ, Nicoloro SM, Wang M, Chouinard M, Soto E, et al. Orally Delivered siRNA Targeting Macrophage Map4k4 Suppresses Systemic Inflammation. Nature (2009) 458:1180–4. doi: 10.1038/nature07774
231. Li X, Zhao Z, Yang Y, Liu Z, Wang J, Xu Y, et al. Novel Beta-1,3-D-Glucan Porous Microcapsule Enveloped Folate-Functionalized Liposomes as a Trojan Horse for Facilitated Oral Tumor-Targeted Co-Delivery of Chemotherapeutic Drugs and Quantum Dots. J Mater Chem B (2020) 8:2307–20. doi: 10.1039/c9tb02674f
232. Hong F, Yan J, Baran JT, Allendorf DJ, Hansen RD, Ostroff GR, et al. Mechanism by Which Orally Administered Beta-1,3-Glucans Enhance the Tumoricidal Activity of Antitumor Monoclonal Antibodies in Murine Tumor Models. J Immunol (2004) 173:797–806. doi: 10.4049/jimmunol.173.2.797
233. Li B, Cai Y, Qi C, Hansen R, Ding C, Mitchell TC, et al. Orally Administered Particulate Beta-Glucan Modulates Tumor-Capturing Dendritic Cells and Improves Antitumor T-Cell Responses in Cancer. Clin Cancer Res (2010) 16:5153–64. doi: 10.1158/1078-0432.CCR-10-0820
234. Zheng Z, Pan X, Wang H, Wu Z, Sullivan MA, Liu Y, et al. Mechanism of Lentinan Intestinal Absorption: Clathrin-Mediated Endocytosis and Macropinocytosis. J Agric Food Chem (2021) 69:7344–52. doi: 10.1021/acs.jafc.1c00349
235. Wang C-L, Lu C-Y, Pi C-C, Zhuang Y-J, Chu C-L, Liu W-H, et al. Extracellular Polysaccharides Produced by Ganoderma Formosanum Stimulate Macrophage Activation via Multiple Pattern-Recognition Receptors. BMC Complement Altern Med (2012) 12:119. doi: 10.1186/1472-6882-12-119
236. Brown GD, Herre J, Williams DL, Willment JA, Marshall ASJ, Gordon S. Dectin-1 Mediates the Biological Effects of Beta-Glucans. J Exp Med (2003) 197:1119–24. doi: 10.1084/jem.20021890
237. Leentjens J, Quintin J, Gerretsen J, Kox M, Pickkers P, Netea MG. The Effects of Orally Administered Beta-Glucan on Innate Immune Responses in Humans, a Randomized Open-Label Intervention Pilot-Study. PloS One (2014) 9:e108794. doi: 10.1371/journal.pone.0108794
238. Tang Y, Govers C, Wichers HJ, Mes JJ. Macrophages Treated With Non-Digestible Polysaccharides Reveal a Transcriptionally Unique Phenotype. J Funct Foods (2017) 36:280–9. doi: 10.1016/j.jff.2017.07.003
239. Govers C, Tang Y, Stolte EH, Wichers HJ, Mes JJ. Wheat-Derived Arabinoxylans Reduced M2-Macrophage Functional Activity, But Enhanced Monocyte-Recruitment Capacity. Food Funct (2020) 11:7073–83. doi: 10.1039/d0fo00316f
240. de Graaff P, Berrevoets C, Rsch C, Schols HA, Verhoef K, Wichers HJ, et al. Curdlan, Zymosan and a Yeast-Derived Beta-Glucan Reshape Tumor-Associated Macrophages Into Producers of Inflammatory Chemo-Attractants. Cancer Immunol Immunother (2021) 70:547–61. doi: 10.1007/s00262-020-02707-4
241. Moerings BGJ, de Graaff P, Furber M, Witkamp RF, Debets R, Mes JJ, et al. Continuous Exposure to Non-Soluble $β$-Glucans Induces Trained Immunity in M-CSF-Differentiated Macrophages. Front Immunol (2021) 12:672796. doi: 10.3389/fimmu.2021.672796
242. Cruz CS, Ricci MF, Vieira AT. Gut Microbiota Modulation as a Potential Target for the Treatment of Lung Infections. Front Pharmacol (2021) 12:724033. doi: 10.3389/fphar.2021.724033
243. Hill C, Guarner F, Reid G, Gibson GR, Merenstein DJ, Pot B, et al. Expert Consensus Document. The International Scientific Association for Probiotics and Prebiotics Consensus Statement on the Scope and Appropriate Use of the Term Probiotic. Nat Rev Gastroenterol Hepatol (2014) 11:506–14. doi: 10.1038/nrgastro.2014.66
244. Ishizuka T, Kanmani P, Kobayashi H, Miyazaki A, Soma J, Suda Y, et al. Immunobiotic Bifidobacteria Strains Modulate Rotavirus Immune Response in Porcine Intestinal Epitheliocytes via Pattern Recognition Receptor Signaling. PloS One (2016) 11:e0152416. doi: 10.1371/journal.pone.0152416
245. Li K-L, Wang B-Z, Li Z-P, Li Y-L, Liang J-J. Alterations of Intestinal Flora and the Effects of Probiotics in Children With Recurrent Respiratory Tract Infection. World J Pediatr (2019) 15:255–61. doi: 10.1007/s12519-019-00248-0
246. Sanders ME, Merenstein DJ, Reid G, Gibson GR, Rastall RA. Probiotics and Prebiotics in Intestinal Health and Disease: From Biology to the Clinic. Nat Rev Gastroenterol Hepatol (2019) 16:605–16. doi: 10.1038/s41575-019-0173-3
247. Lehtoranta L, Pitkäranta A, Korpela R. Probiotics in Respiratory Virus Infections. Eur J Clin Microbiol Infect Dis Off Publ Eur Soc Clin Microbiol (2014) 33:1289–302. doi: 10.1007/s10096-014-2086-y
248. Frese SA, Hutton AA, Contreras LN, Shaw CA, Palumbo MC, Casaburi G, et al. Persistence of Supplemented Bifidobacterium Longum Subsp. Infantis EVC001 in Breastfed Infants. mSphere (2017) 2:e00501–17. doi: 10.1128/mSphere.00501-17
249. Reddel S, Del Chierico F, Quagliariello A, Giancristoforo S, Vernocchi P, Russo A, et al. Gut Microbiota Profile in Children Affected by Atopic Dermatitis and Evaluation of Intestinal Persistence of a Probiotic Mixture. Sci Rep (2019) 9:4996. doi: 10.1038/s41598-019-41149-6
250. Taverniti V, Koirala R, Dalla Via A, Gargari G, Leonardis E, Arioli S, et al. Effect of Cell Concentration on the Persistence in the Human Intestine of Four Probiotic Strains Administered Through a Multispecies Formulation. Nutrients (2019) 11:285. doi: 10.3390/nu11020285
251. Karl JP. Gut Microbiota-Targeted Interventions for Reducing the Incidence, Duration, and Severity of Respiratory Tract Infections in Healthy Non-Elderly Adults. Mil Med (2021) 186:e310–8. doi: 10.1093/milmed/usaa261
252. Shimizu K, Yamada T, Ogura H, Mohri T, Kiguchi T, Fujimi S, et al. Synbiotics Modulate Gut Microbiota and Reduce Enteritis and Ventilator-Associated Pneumonia in Patients With Sepsis: A Randomized Controlled Trial. Crit Care (2018) 22:239. doi: 10.1186/s13054-018-2167-x
253. Enani S, Przemska-Kosicka A, Childs CE, Maidens C, Dong H, Conterno L, et al. Impact of Ageing and a Synbiotic on the Immune Response to Seasonal Influenza Vaccination; a Randomised Controlled Trial. Clin Nutr (2018) 37:443–51. doi: 10.1016/j.clnu.2017.01.011
254. Agustina R, Bovee-Oudenhoven IM, Lukito W, Fahmida U, van de RO, Zimmermann MB, et al. Probiotics Lactobacillus Reuteri DSM 17938 and Lactobacillus Casei CRL 431 Modestly Increase Growth, But Not Iron and Zinc Status, Among Indonesian Children Aged 1-6 Years. J Nutr (2013) 143:1184–93. doi: 10.3945/jn.112.166397
255. Agustina R, Kok FJ, van de Rest O, Fahmida U, Firmansyah A, Lukito W, et al. Randomized Trial of Probiotics and Calcium on Diarrhea and Respiratory Tract Infections in Indonesian Children. Pediatrics (2012) 129:e1155–64. doi: 10.1542/peds.2011-1379
256. Darbandi A, Asadi A, Ghanavati R, Afifirad R, Darb Emamie A, Kakanj M, et al. The Effect of Probiotics on Respiratory Tract Infection With Special Emphasis on COVID-19: Systemic Review 2010-20. Int J Infect Dis IJID Off Publ Int Soc Infect Dis (2021) 105:91–104. doi: 10.1016/j.ijid.2021.02.011
257. Esposito S, Rigante D, Principi N. Do Children’s Upper Respiratory Tract Infections Benefit From Probiotics? BMC Infect Dis (2014) 14:194. doi: 10.1186/1471-2334-14-194
258. Percopo CM, Rice TA, Brenner TA, Dyer KD, Luo JL, Kanakabandi K, et al. Immunobiotic Lactobacillus Administered Post-Exposure Averts the Lethal Sequelae of Respiratory Virus Infection. Antiviral Res (2015) 121:109–19. doi: 10.1016/j.antiviral.2015.07.001
259. Amaral MA, Guedes GHBF, Epifanio M, Wagner MB, Jones MH, Mattiello R. Network Meta-Analysis of Probiotics to Prevent Respiratory Infections in Children and Adolescents. Pediatr Pulmonol (2017) 52:833–43. doi: 10.1002/ppul.23643
260. Wang Y, Li X, Ge T, Xiao Y, Liao Y, Cui Y, et al. Probiotics for Prevention and Treatment of Respiratory Tract Infections in Children: A Systematic Review and Meta-Analysis of Randomized Controlled Trials. Med (Baltimore) (2016) 95:e4509. doi: 10.1097/MD.0000000000004509
261. West CE. Probiotics for Allergy Prevention. Benef Microbes (2016) 7:171–9. doi: 10.3920/BM2015.0073
262. Kawahara T, Takahashi T, Oishi K, Tanaka H, Masuda M, Takahashi S, et al. Consecutive Oral Administration of Bifidobacterium Longum MM-2 Improves the Defense System Against Influenza Virus Infection by Enhancing Natural Killer Cell Activity in a Murine Model. Microbiol Immunol (2015) 59:1–12. doi: 10.1111/1348-0421.12210
263. Hardy H, Harris J, Lyon E, Beal J, Foey AD. Probiotics, Prebiotics and Immunomodulation of Gut Mucosal Defences: Homeostasis and Immunopathology. Nutrients (2013) 5:1869–912. doi: 10.3390/nu5061869
264. Bron PA, Kleerebezem M, Brummer R-J, Cani PD, Mercenier A, MacDonald TT, et al. Can Probiotics Modulate Human Disease by Impacting Intestinal Barrier Function? Br J Nutr (2017) 117:93–107. doi: 10.1017/S0007114516004037
265. Antunes KH, Fachi JL, de Paula R, da Silva EF, Pral LP, Dos Santos AÁ, et al. Microbiota-Derived Acetate Protects Against Respiratory Syncytial Virus Infection Through a GPR43-Type 1 Interferon Response. Nat Commun (2019) 10:3273. doi: 10.1038/s41467-019-11152-6
266. Ceccarelli G, Scagnolari C, Pugliese F, Mastroianni CM, d’Ettorre G. Probiotics and COVID-19. Lancet Gastroenterol Hepatol (2020) 5:721–2. doi: 10.1016/S2468-1253(20)30196-5
267. Shahbazi R, Yasavoli-Sharahi H, Alsadi N, Ismail N, Matar C. Probiotics in Treatment of Viral Respiratory Infections and Neuroinflammatory Disorders. Molecules (2020) 25:4891. doi: 10.3390/molecules25214891
268. Mahooti M, Miri SM, Abdolalipour E, Ghaemi A. The Immunomodulatory Effects of Probiotics on Respiratory Viral Infections: A Hint for COVID-19 Treatment? Microb Pathog (2020) 148:104452. doi: 10.1016/j.micpath.2020.104452
269. Bradley KC, Finsterbusch K, Schnepf D, Crotta S, Llorian M, Davidson S, et al. Microbiota-Driven Tonic Interferon Signals in Lung Stromal Cells Protect From Influenza Virus Infection. Cell Rep (2019) 28:245–256.e4. doi: 10.1016/j.celrep.2019.05.105
270. Sundararaman A, Ray M, Ravindra PV, Halami PM. Role of Probiotics to Combat Viral Infections With Emphasis on COVID-19. Appl Microbiol Biotechnol (2020) 104:8089–104. doi: 10.1007/s00253-020-10832-4
271. d’Ettorre G, Ceccarelli G, Marazzato M, Campagna G, Pinacchio C, Alessandri F, et al. Challenges in the Management of SARS-CoV2 Infection: The Role of Oral Bacteriotherapy as Complementary Therapeutic Strategy to Avoid the Progression of COVID-19. Front Med (2020) 7:389. doi: 10.3389/fmed.2020.00389
272. Liu Q, Tian X, Maruyama D, Arjomandi M, Prakash A. Lung Immune Tone via Gut-Lung Axis: Gut-Derived LPS and Short-Chain Fatty Acids’ Immunometabolic Regulation of Lung IL-1β, FFAR2, and FFAR3 Expression. Am J Physiol Lung Cell Mol Physiol (2021) 321:L65–78. doi: 10.1152/ajplung.00421.2020
273. Przemska-Kosicka A, Childs CE, Enani S, Maidens C, Dong H, Bin Dayel I, et al. Effect of a Synbiotic on the Response to Seasonal Influenza Vaccination Is Strongly Influenced by Degree of Immunosenescence. Immun Ageing (2016) 13:6. doi: 10.1186/s12979-016-0061-4
274. Ahanchian H, Jafari SA, Ansari E, Ganji T, Kiani MA, Khalesi M, et al. A Multi-Strain Synbiotic May Reduce Viral Respiratory Infections in Asthmatic Children: A Randomized Controlled Trial. Electron Physician (2016) 8:2833–9. doi: 10.19082/2833
275. Chan CKY, Tao J, Chan OS, Li H-B, Pang H. Preventing Respiratory Tract Infections by Synbiotic Interventions: A Systematic Review and Meta-Analysis of Randomized Controlled Trials. Adv Nutr (2020) 11:979–88. doi: 10.1093/advances/nmaa003
276. Pregliasco F, Anselmi G, Fonte L, Giussani F, Schieppati S, Soletti L. A New Chance of Preventing Winter Diseases by the Administration of Synbiotic Formulations. J Clin Gastroenterol (2008) 42:S224–33. doi: 10.1097/MCG.0b013e31817e1c91
277. Ahanchian H, Ranjbar A, Reihani H, Yazdi AP, Jafari SA, Kiani MA, et al. Synbiotic for Prevention of SARS-Cov2 Infection in High Risk Hospital Staffs: A Randomized Controlled Trial. Open J Nurs (2021) 11:281–90. doi: 10.4236/ojn.2021.115025
278. Walton GE, Gibson GR, Hunter KA. Mechanisms Linking the Human Gut Microbiome to Prophylactic and Treatment Strategies for COVID-19. Br J Nutr (2021) 126:219–27. doi: 10.1017/S0007114520003980
279. Bustamante M, Oomah BD, Oliveira WP, Burgos-Díaz C, Rubilar M, Shene C. Probiotics and Prebiotics Potential for the Care of Skin, Female Urogenital Tract, and Respiratory Tract. Folia Microbiol (Praha) (2020) 65:245–64. doi: 10.1007/s12223-019-00759-3
280. Holmgren J, Czerkinsky C. Mucosal Immunity and Vaccines. Nat Med (2005) 11:S45–53. doi: 10.1038/nm1213
281. van Splunter M, van Hoffen E, Floris-Vollenbroek EG, Timmerman H, de Bos EL-V, Meijer B, et al. Oral Cholera Vaccination Promotes Homing of IgA+memory B Cells to the Large Intestine and the Respiratory Tract. Mucosal Immunol (2018) 11:1254–64. doi: 10.1038/s41385-018-0006-7
282. eirik Johansen F-E, Baekkevold ES, Carlsen HS, Farstad IN, Soler D, Brandtzaeg P. Regional Induction of Adhesion Molecules and Chemokine Receptors Explains Disparate Homing of Human B Cells to Systemic and Mucosal Effector Sites: Dispersion From Tonsils. Blood (2005) 106:593–600. doi: 10.1182/blood-2004-12-4630
283. Shikina T, Hiroi T, Iwatani K, Jang MH, Fukuyama S, Tamura M, et al. IgA Class Switch Occurs in the Organized Nasopharynx- and Gut-Associated Lymphoid Tissue, But Not in the Diffuse Lamina Propria of Airways and Gut. J Immunol (2004) 172:6259–64. doi: 10.4049/jimmunol.172.10.6259
284. Perry M, Whyte A. Immunology of the Tonsils. Immunol Today (1998) 5699:414–21. doi: 10.1016/s0167-5699(98)01307-3
285. Perry ME. The Specialised Structure of Crypt Epithelium in the Human Palatine Tonsil and Its Functional Significance. J Anat (1994) 185(Pt 1):111–27.
286. Lange MJ, Lasiter JC, Misfeldt ML. Toll-Like Receptors in Tonsillar Epithelial Cells. Int J Pediatr Otorhinolaryngol (2009) 73:613–21. doi: 10.1016/j.ijporl.2008.12.013
287. Ogasawara N, Kojima T, Go M, ichi Takano K, Kamekura R, Ohkuni T, et al. Epithelial Barrier and Antigen Uptake in Lymphoepithelium of Human Adenoids. Acta Otolaryngol (2010) 131:116–23. doi: 10.3109/00016489.2010.520022
288. Menard S, Cerf-Bensussan N, Heyman M. Multiple Facets of Intestinal Permeability and Epithelial Handling of Dietary Antigens. Mucosal Immunol (2010) 3:247–59. doi: 10.1038/mi.2010.5
289. Brandtzaeg P. Potential of Nasopharynx-Associated Lymphoid Tissue for Vaccine Responses in the Airways. Am J Respir Crit Care Med (2011) 183:1595–604. doi: 10.1164/rccm.201011-1783OC
290. Agace WW. Tissue-Tropic Effector T Cells: Generation and Targeting Opportunities. Nat Rev Immunol (2006) 6:682–92. doi: 10.1038/nri1869
291. Johansson-Lindbom B, Agace WW. Generation of Gut-Homing T Cells and Their Localization to the Small Intestinal Mucosa. Immunol Rev (2007) 215:226–42. doi: 10.1111/j.1600-065X.2006.00482.x
292. Holt PG, Strickland DH, Wikström ME, Jahnsen FL. Regulation of Immunological Homeostasis in the Respiratory Tract. Nat Rev Immunol (2008) 8:142–52. doi: 10.1038/nri2236
293. Hammerschmidt SI, Ahrendt M, Bode U, Wahl B, Kremmer E, Forster R, et al. Stromal Mesenteric Lymph Node Cells Are Essential for the Generation of Gut-Homing T Cells In Vivo. J Exp Med (2008) 205:2483–90. doi: 10.1084/jem.20080039
294. Hammerschmidt SI, Friedrichsen M, Boelter J, Lyszkiewicz M, Kremmer E, Pabst O, et al. Retinoic Acid Induces Homing of Protective T and B Cells to the Gut After Subcutaneous Immunization in Mice. J Clin Invest (2011) 121:3051–61. doi: 10.1172/JCI44262DS1
295. Yamanaka T, Helgeland L, Farstad IN, Fukushima H, Midtvedt T, Brandtzaeg P. Microbial Colonization Drives Lymphocyte Accumulation and Differentiation in the Follicle-Associated Epithelium of Peyer’s Patches. J Immunol (2003) 170:816–22. doi: 10.4049/jimmunol.170.2.816
296. Andrews GP, Laverty TP, Jones DS. Mucoadhesive Polymeric Platforms for Controlled Drug Delivery. Eur J Pharm Biopharm (2009) 71:505–18. doi: 10.1016/j.ejpb.2008.09.028
297. King M, Rubin BK. Pharmacological Approaches to Discovery and Development of New Mucolytic Agents. Adv Drug Delivery Rev (2002) 54:1475–90. doi: 10.1016/s0169-409x(02)00156-4
298. Van Der Lubben IM, Kersten G, Fretz MM, Beuvery C, Verhoef JCC, Junginger HE, et al. Chitosan Microparticles for Mucosal Vaccination Against Diphtheria : Oral and Nasal Efficacy Studies in Mice. Vaccine (2003) 21:1400–8. doi: 10.1016/s0264-410x(02)00686-2
299. Razafindratsita A, Saint-lu N, Mascarell L, Berjont N, Bardon T, Betbeder D. Improvement of Sublingual Immunotherapy Efficacy With a Mucoadhesive Allergen Formulation. J Allergy Clin Immunol (2007) 120:278–85. doi: 10.1016/j.jaci.2007.04.009
Keywords: infection, immunity, nutrition, infant, elderly, respiratory virus
Citation: Govers C, Calder PC, Savelkoul HFJ, Albers R and van Neerven RJJ (2022) Ingestion, Immunity, and Infection: Nutrition and Viral Respiratory Tract Infections. Front. Immunol. 13:841532. doi: 10.3389/fimmu.2022.841532
Received: 22 December 2021; Accepted: 02 February 2022;
Published: 28 February 2022.
Edited by:
Xiuyang Li, Zhejiang University, ChinaReviewed by:
Manfred Eggersdorfer, University Medical Center Groningen, NetherlandsLinda Saif, The Ohio State University, United States
Copyright © 2022 Govers, Calder, Savelkoul, Albers and van Neerven. This is an open-access article distributed under the terms of the Creative Commons Attribution License (CC BY). The use, distribution or reproduction in other forums is permitted, provided the original author(s) and the copyright owner(s) are credited and that the original publication in this journal is cited, in accordance with accepted academic practice. No use, distribution or reproduction is permitted which does not comply with these terms.
*Correspondence: R. J. Joost van Neerven, am9vc3QudmFubmVlcnZlbkB3dXIubmw=