- 1Department of Microbiology, Immunology, and Cell Biology, West Virginia University, Morgantown, WV, United States
- 2Vaccine Development Center, West Virginia University Health Sciences Center, Morgantown, WV, United States
Over two decades ago acellular pertussis vaccines (aP) replaced whole cell pertussis vaccines (wP) in several countries. Since then, a resurgence in pertussis has been observed, which is hypothesized to be linked, in part, to waning immunity. To better understand why waning immunity occurs, we developed a long-term outbred CD1 mouse model to conduct the longest murine pertussis vaccine studies to date, spanning out to 532 days post primary immunization. Vaccine-induced memory results from follicular responses and germinal center formation; therefore, cell populations and cytokines involved with memory were measured alongside protection from challenge. Both aP and wP immunization elicit protection from intranasal challenge by decreasing bacterial burden in both the upper and lower airways, and by generation of pertussis specific antibody responses in mice. Responses to wP vaccination were characterized by a significant increase in T follicular helper cells in the draining lymph nodes and CXCL13 levels in sera compared to aP mice. In addition, a population of B. pertussis+ memory B cells was found to be unique to wP vaccinated mice. This population peaked post-boost, and was measurable out to day 365 post-vaccination. Anti-B. pertussis and anti-pertussis toxoid antibody secreting cells increased one day after boost and remained high at day 532. The data suggest that follicular responses, and in particular CXCL13 levels in sera, could be monitored in pre-clinical and clinical studies for the development of the next-generation pertussis vaccines.
Introduction
Pertussis (whooping cough) is a vaccine-preventable respiratory disease caused by the Gram-negative bacterium Bordetella pertussis (1). Whole cell pertussis vaccines (DTP/wP) were first developed and implemented in 1914, but did not become widely available for distribution until the 1940s (1, 2). After their implementation in the United States, DTP vaccines dramatically reduced pertussis disease from ~200,000 cases a year in the 1930s to ~2,000 in the 1970s (1). However, safety concerns arose that led to the development of acellular pertussis vaccines (aP) in the United States and Europe in the late 1990’s (3, 4). Unlike wPs, which are composed of inactivated B. pertussis, aPs contain 1-5 pertussis antigens adsorbed to aluminum hydroxide (1, 5). While aP vaccines protect against symptomatic disease, they do not prevent asymptomatic carriage or transmission of pertussis (6). Pertussis outbreaks have been increasing at an alarming rate despite high vaccine coverage since wP vaccines were replaced with aP vaccines (7–9). During the 2012 pertussis outbreak in the US, there were ~50,000 cases and 20 deaths attributed to this disease which is the worst incidence of pertussis observed since the 1950s (10). Fluctuation of pertussis incidence is hypothesized to be in part due to waning in immunity elicited by the current aP vaccines. This is illustrated by an increase in incidence rates and risk ratios for pertussis in between boosters during adolescence in aP vaccinated individuals (11–13). The pertussis field has several hypotheses for the resurgence of pertussis including (in no particular order): 1) more sensitive PCR-based testing, 2) increased reporting, 3) bacterial evolution, 4) differences in the type of TH immune response induced by aP vaccination compared to wP or natural infection, and 5) lack of duration of the aP vaccine-induced immune response (3, 14–18).
Pre-clinical models of pertussis are instrumental to understand vaccine protection prior to human studies. Mice have been the primary model used for both experimental vaccine development and to test vaccine lot potency. For example, the Kendrick’s test was utilized to standardize the potency of wP vaccine lots by measuring immunogenicity and protection against intracranial challenge (19). Intranasal mouse models were later developed to better recapitulate the natural route of infection. Overall, the murine model has been extremely useful for the study of pertussis, illuminating many aspects of pertussis pathogenesis, immune responses to infection, and vaccine development (20–23). Furthermore, the mouse model has allowed researchers to better understand mucosal responses and neonatal responses to pertussis (24, 25). Recent work has identified several new immune factors associated with pertussis vaccination and intranasal challenge using the mouse model including: T resident memory cells, secretory IgAs, and interleukin-6, to name a few (23, 26–29). Despite some caveats, such as lack of audible coughing, this model recapitulates disease manifestation, continues to provide novel findings, and remains a relevant model for pertussis vaccine development.
In addition to mice, immunogenicity and protection provided by pertussis vaccines have been studied in the green olive baboon model, which is currently the gold standard pre-clinical model of pertussis (30). The baboon is ideal for studying pertussis vaccines using the human immunization schedule (2, 4, 6, 8 months) with subsequent challenge at 9 to 12 months (6, 31). At this age, baboons cough and show similar disease manifestation as humans. The baboon model demonstrated that aP immunized hosts are protected from symptomatic disease, yet transmit B. pertussis, which recapitulates the current pertussis problems observed in humans (30, 32). Unfortunately, baboons are not suitable for longevity studies because after 15 months of age they become resistant to infection [Tod Merkel, personal communication]. Other models of pertussis have been developed, including the rat model that allows for measurement of the hallmark cough of pertussis, and mini pigs that were recently used to study longevity of pertussis vaccines (33, 34). While highly useful to study pertussis and vaccine-mediated responses, these models are still under-developed and suffer from the lack of broadly available tools and reagents. In the murine model, immunological memory responses are well characterized and there are a variety of established tools available to study vaccine-induced memory. Therefore, in this study, we focused on adapting the mouse model of pertussis to study the longevity of the pertussis vaccine-mediated humoral memory response.
Immunological memory is the ability of the immune system to rapidly recognize and respond to an antigen after prior exposure (35). In order to induce immunological memory, B cell affinity maturation reactions, known as follicular immune responses, occur in microenvironments called germinal centers (GCs) (12). GCs are located within the draining lymph node near the site of vaccination, and in the spleen (36). T follicular helper cells (TFH cells) are crucial for the formation of GCs, B cell affinity maturation, development of memory B cells (MBCs), and high affinity antibody secreting cells (37–40). TFH cells and follicular dendritic cells in the GC express a signaling molecule known as C-X-C motif chemokine ligand 13 (CXCL13) which binds to C-X-C motif chemokine receptor 5 (CXCR5). CXCR5 is a G-protein coupled receptor expressed by TFH cells and B cells (41). CXCL13:CXCR5 interactions play a role in recruitment of B cells to the follicle and in the organization of the GC (42, 43). Once B cells have migrated to the follicle in response to CXCL13, high affinity B cells are selected by TFH cells to migrate from the light zone to the dark zone where they undergo proliferation and somatic hypermutation. The products of the GC reaction are MBCs and antibody secreting plasma cells that function to persist over time and prevent subsequent infection by producing antibodies.
In humans, both exposure to live B. pertussis and pertussis vaccines can induce memory B and T cells that result from germinal center reactions (44–46). In vaccinated individuals, B. pertussis specific memory B cells expand following antigen exposure, decay rapidly, but can be detected years post-immunization (44). Furthermore, it has been suggested in mice that B. pertussis specific memory B cells have protective roles even in the absence of circulating antibodies at the time of challenge (44, 47). Overall, the relevance of germinal center CD4+ TFH cells and B. pertussis memory B cells in pertussis immunity remains unclear.
Therefore, the objective of this study was to examine the follicular responses induced by vaccination against pertussis in mice to gain insights into the duration of vaccine-induced memory. To do so, we developed a long-term murine model of pertussis vaccine prime, boost, and intranasal challenge. We used this model to compare aP and wP protection and follicular responses beginning at day 20 and concluding at day 532 post-prime. Given that administration of wP leads to longer-lasting protection than aP in humans, we hypothesized that wP would induce more robust follicular responses than aP immunization in mice (48). Responses to vaccination were measured by quantification of pertussis-specific antibody titers, antibody secreting cells, and follicular responses. Protection provided by vaccination was measured by quantifying bacterial burden in the lungs, trachea, and nasal wash 3 days post-challenge. In this work, we describe immunological memory markers that are significantly increased in wP and not aP immunization, such as CXCL13 and antigen-specific memory B cell production. Our data identify that B memory responses are an underappreciated aspect of pertussis immunity that could guide future pertussis vaccine development.
Materials and Methods
B. pertussis Strains and Growth Conditions
Bordetella pertussis strain UT25Sm1 was kindly provided by Dr. Sandra Armstrong (University of Minnesota) (49, 50). UT25Sm1 strain has been fully genome sequenced (NCBI Reference Sequence: NZ_CP015771.1). UT25 was originally isolated in 1977 from a child diagnosed with pertussis. UT25Sm1 was grown on Remel Bordet Gengou (BG) agar (Thermo Scientific, Cat. #R452432) supplemented with 15% defibrillated sheep blood (Hemostat Laboratories, Cat. #DSB500) and streptomycin 100 μg/mL (Gibco™, Cat. #11860038) at 36°C for 48 hours. For each infection point, the number of viable bacteria and the Bvg+ (hemolytic and characteristic colony morphology) phenotype were confirmed to ensure consistency between each challenge. Bacteria were then collected using polyester swabs and resuspended in Stainer Scholte media (51) (SSM) supplemented with L-proline and SSM supplement. SSM liquid culture was incubated for 24 hours at 36°C with constant shaking at 180 rpm until reaching mid-log phase OD600nm 0.5 with 1 cm path width (Beckman Coulter™ DU 530 UV Vis spectrophotometer). The UT25Sm1 B. pertussis culture was diluted in supplemented SSM to OD600nm = 0.24 - 0.245 (equivalent to 109 CFU/mL) to be used for challenge or serological analysis by ELISA.
Vaccine Preparation and Immunization, Bacterial Challenge, and Euthanasia
The World Health Organization (WHO) standard whole cell B. pertussis vaccine (wP) was obtained from the National Institute for Biological Standards and Control (NIBSC, Cat. #94/532, batch 41S) and compared to the acellular B. pertussis vaccine DTaP (Infranrix®, GlaxoSmithKline). The NIBSC wP is not a DTP alum adjuvanted human vaccine and was not supplemented with alum in this work. All vaccines were intramuscularly administered at 1/10th the human dose in 50 μL. The wP vaccine was diluted using endotoxin free phosphate buffered saline (PBS) (Millipore Sigma™, Cat. #TMS012A). DTaP was administered without dilution as 50 μL is 1/10th the human dose. PBS was administered as a vehicle control. In all experimental groups, 6-week-old outbred female CD1 mice (Charles River, Strain code 022) were used. Mice were primed at day 0, followed by a booster of the same vaccine at day 21. Mice were euthanized at days 20, 22, 35, 60, 90, 152, 365, and 532 post-vaccination. For challenged animals, mice were anesthetized three days before euthanasia by intraperitoneal injection (IP) ketamine (7.7 mg/kg) (Patterson Veterinary, Cat. #07-803-6637) and xylazine (0.77 mg/kg) (Patterson Veterinary, Cat. #07-808-1939) in sterile 0.9% NaCl (Baxter, Cat. #2F7124) and challenged intranasally with ~2x107 CFU/dose of live B. pertussis (10 µL per nostril) (25, 52). At day three post-challenge mice were euthanized by IP injection of Euthasol (390 mg pentobarbital/kg) (Patterson Veterinary, Cat. #07-805-9296) in sterile 0.9% w/v NaCl.
Quantification of Bacterial Burden
Lung and trachea homogenates as well as nasal lavage (nasal wash) were collected post mortem and used to enumerate bacterial burden per tissue. Mice were challenged at days 38, 63, 93, 155, 368, and 535 post-prime. The nasal cavity was flushed with 1 mL sterile PBS for nasal lavage. The lung and trachea were homogenized separately in 1 mL sterile PBS using a Polytron PT 2500 E homogenizer (Kinematica). Samples were serially diluted in ten-fold dilutions in PBS and plated on BG agar to quantify viable bacterial burden. Plates were incubated at 36°C for 48-72 hours to determine colony forming units (CFUs) per mL.
Serological Analysis of Immunized Mice
Enzyme linked immunosorbent assay (ELISA) was utilized to measure antigen-specific antibodies in the serum of immunized mice (52–54). After euthanasia, blood was collected in BD Microtainer serum separator tubes (BD, Cat. #365967) via cardiac puncture at days 20, 22, 35, 60, 90, 152, 365, and 532 post primary immunization. Blood was centrifuged at 14,000 x g for 2 minutes and sera were stored at -80°C. Pierce™ high-binding 96 well plates (Thermo Scientific™, Cat. #15041) were coated with 5x107 CFU/well viable B. pertussis, 6.25 ng/well of diphtheria toxoid (List Biological Laboratories, Cat. #151), 6.25 ng/well tetanus toxoid (List Biological Laboratories, Cat. #191), 50 ng/well pertussis toxin (List Biological Laboratories, Cat. #180) or 50 ng/well of filamentous hemagglutinin (Life Sciences, Inc., Cat. #ALX-630-123-0100 Enzo) overnight at 4°C. Plates were washed three times with PBS-Tween®20 (Fisher Scientific, Cat. #BP337-500), then blocked with 5% w/v non-fat dry milk (Nestle Carnation, Cat. #000500002292840) in PBS-Tween®20. Serum samples were serially diluted from 1:50 to 1:819,200 using 5% w/v milk in PBS-Tween®20 for anti- B. pertussis and anti-FHA ELISAs. Serum samples were diluted 1:200 using 5% w/v milk in PBS-Tween®20 and serially diluted to 1:3,276,800 for anti-pertussis toxin, anti-diphtheria toxoid, and anti-tetanus toxoid ELISAs. Plates were incubated at 37°C for 2 hours and washed four times with PBS-Tween®20. Secondary goat anti-mouse IgG antibody 1:2000 (Southern Biotech, Cat. #1030-04) conjugated to alkaline phosphatase was added and incubated for 1 hour at 37°C. Wells were washed five times with PBS-Tween®20 and Pierce p-Nitrophenyl Phosphate (PNPP) (Thermo Scientific, Cat. #37620) was added to each well to develop plates for 30 minutes in the dark at room temperature. The absorbance at 405 nm was read utilizing a SpectraMax® i3 plate reader (Molecular Devices). The lower limit of detection for serum titers was 1:50, and for statistical analysis, all values below the limit of detection are represented with the arbitrary value of one. Endpoint titers were determined by selecting the dilution at which the absorbance was greater than or equal to twice that of the negative control.
CXCL13 Assay
CXCL13 chemokine levels were measured in sera from mice using the CXCL13/BLC/BCA-1 DuoSet ELISA kit (R&D Systems, Cat. #DY470) and the DuoSet Ancillary Reagent kit (R&D Systems, Cat. #DY008). Samples were diluted 4-fold and the assay was performed according to the manufacturer’s protocol.
Tissue Isolation, Preparation, Staining, and Flow Cytometry
Flow cytometry was used to characterize cell populations from the spleen and inguinal lymph nodes. Organs were harvested at days 20, 22, 35, 60, 90, 152, 365, and 532 post-prime. Spleen and lymph nodes were homogenized using disposable pestles (USA Scientific, Cat. #1405-4390) in Dulbecco’s Modified Eagle Media (DMEM) (Corning Incorporated, Cat. #10-013-CV) with 10% v/v fetal bovine serum (FBS) (Gemini Bio, Cat. #100-500). Homogenized samples were strained for separation using 70 μM pore nylon mesh (Elko Filtering Co, Cat. #03-70/33) and centrifuged for 5 minutes at 1,000 x g. Splenocytes were resuspended in 1 mL red blood cell lysis buffer BD Pharm Lyse (BD Biosciences, Cat. #555899) and incubated at 37°C for 2 minutes. After red blood cell lysis, samples were centrifuged 1000 x g for 5 minutes and resuspended in PBS with 5mM ethylenediaminetetraacetic acid (EDTA) (Fisher Scientific, Cat. #50-103-5745) and 1% v/v FBS. Single cell suspensions were incubated with 5 μg/mL anti-mouse CD16/CD32 Fc block (clone 2.4G2, Thermo Fisher Scientific, Cat. #553142) for 15 minutes at 4°C per the manufacturer’s instructions. Cells from tissues were stained with antibodies against cell surface markers (Table S2). Each single cell suspension was incubated with the antibody cocktail for 1 hour at 4°C in the dark. Samples were washed by resuspending in PBS, centrifuging, removing the supernatant, and washing in PBS with 5mM EDTA and 1% v/v FBS and fixed with 0.4% w/v paraformaldehyde (Santa Cruz Biotechnology, Cat. #sc-281692) overnight. After fixation, samples were centrifuged and washed before resuspension in PBS with 5mM EDTA and 1% v/v FBS. The samples were processed using an LSR Fortessa flow cytometer (BD Biosciences) and analyzed using FlowJo (FlowJo™ Software Version v10). Cells were counted using Sphero AccuCount 5-5.9 μm beads according to the manufacturer’s protocol (Spherotech, Cat. #ACBP-50-10).
Bacterial Preparation for Antigen-Specific Memory B Cell Purification
B. pertussis was grown as described above in section 3.1. Liquid culture was diluted to 6x107 CFU/mL using PBS and inactivated in 1 mL aliquots by heating at 65°C for 1.5 hours with constant shaking. Heat-killed bacteria were then stained with BacLight Red (Invitrogen™, Cat. #B35001) overnight per the manufacturer’s instructions. Fluorescently labeled B. pertussis cells were centrifuged at 15,000 x g for 15 minutes, supernatant was removed, and the labeled B. pertussis cells were resuspended in DMEM with 10% v/v FBS for incubation with splenocytes from immunized mice.
Antigen-Specific Memory B Cell Purification
Spleens were extracted into DMEM + 10% v/v FBS and homogenized with a small pestle and centrifuged at 1000 x g for 5 minutes. Cells were resuspended in DMEM with 10% v/v FBS and filtered through 70 μM pore nylon mesh. Filtrate was centrifuged at 1000 x g for 5 minutes. Cell pellets were resuspended in 1 mL BD Pharm Lyse (BD Biosciences, Cat. #555899) at 37°C for 3 minutes to lyse red blood cells. The remaining cells were centrifuged at 1000 x g for 5 minutes at 4°C. The supernatant was discarded, and pellets were resuspended in DMEM with 10% v/v FBS. The remaining cells were centrifuged at 1000 x g for 5 minutes at room temperature and resuspended at a 1:5 splenocytes to B. pertussis ratio in 6x107 CFU/mL heat-killed fluorescently labeled B. pertussis reconstituted in DMEM with v/v 10% FBS. Splenocytes and fluorescently labeled B. pertussis were mixed end over end for 1 hour at 4°C.
After incubation, the splenocytes were centrifuged at 1000 x g for 5 minutes and resuspended in the Miltenyi Memory B Cell Biotin-Antibody Cocktail (Miltenyi Memory B Cell Isolation Kit, Cat. #130-095-838), Miltenyi anti-IgG1-APC and Miltenyi anti-IgG2-APC antibodies for a total volume of 500 µL per sample. After incubation, anti-biotin magnetic beads (Miltenyi Memory B Cell Isolation Kit) were added to the cocktail. The samples were mixed end over end in the dark for an additional 10 minutes at 4°C. Samples were then transferred to 15 mL conical tubes and non-memory B cells were depleted using the AutoMACS Magnetic Sorter (Miltenyi Biotec) “deplete” proprietary program. The negative fraction was retained and centrifuged at 1000 x g for 5 minutes. Samples were resuspended with anti-APC magnetic beads (Miltenyi Memory B Cell Isolation Kit), and mixed end over end in the dark for 15 minutes at 4°C. PBS with 1% v/v FBS and 5 mM EDTA was added to the samples before centrifuging at 1000 x g for 5 minutes. Supernatant was discarded and samples were resuspended in PBS + 1% FBS + 5 mM EDTA. Memory B cells were enriched using the AutoMACS “possel_s” program. The positive fraction was retained, and the cells were centrifuged at 1000 x g for 5 minutes. Supernatant was discarded and memory B cells were resuspended in 1 mL PBS with 1% v/v FBS and 5 mM EDTA. Memory B cells were diluted to 106 cells/mL and resuspended in 100 µL of antibody cocktail (Table S2B) for further memory B cell analysis by flow cytometry.
ELISpot Sample Preparation and Analysis
The Mouse IgG ELISpot (ImmunoSpot®, Cat. #mIgGIgA-DCE-1M/10) was utilized to quantify antibody secreting cells specific for B. pertussis. UT25Sm1 was cultured as described above. PVDF membrane 96-well plates were coated with 5x107 CFU/well B. pertussis and incubated overnight at 4°C. To measure pertussis toxoid specific antibody secreting cells, wells were coated with 50 ng/well heat inactivated pertussis toxin. Bone marrow samples were isolated by centrifuging femurs at 400 x g for 5 minutes in 200 µL PCR tubes with holes in the bottom that were placed into 2 mL Eppendorf tubes. The bone marrow was resuspended in heat-inactivated filter-sterilized FBS and filtered through 70 µm mesh with FBS with 10% v/v dimethyl sulfoxide (DMSO) (Sigma-Aldrich, Cat. #D8418-100ML) and stored at -80°C. Bone marrow cells were thawed in a 37°C water bath and placed in DMEM with 10% v/v FBS. Cells were centrifuged at 400 x g for 5 minutes, resuspended in CTL Test B Culture medium (ImmunoSpot), diluted 1:10 with PBS and 1:1 with trypan blue stain (Invitrogen™, Cat. #T10282), and counted on the Countess II Automated Cell Counter (Invitrogen). Plates were washed with PBS and bone marrow cells were added to the first row then serially diluted two-fold down the plate. Cells were incubated at 36°C overnight and then imaged and counted using the ImmunoSpot® S6 ENTRY Analyzer and CTL Software. Dilutions with spots ranging from ~10-100 per well were selected to enumerate the number of anti-B. pertussis antibody-producing cells per sample. Cell counts were normalized to spots per 106 cells using the cell and spot counts.
Statistics
Statistical analysis was performed using GraphPad Prism version 8 (GraphPad). When comparing three or more groups of parametric data a one-way ANOVA (analysis of variance) with Tukey’s multiple comparison test was used unless otherwise noted. For non-parametric data a Kruskal-Wallis test with Dunnet’s post-hoc test was used.
Animal Care and Use
All mouse experiments were approved by the West Virginia University Institutional Animal Care and Use Committees (WVU-AUCU protocol 1901021039) and completed in strict accordance of the National Institutes of Health Guide for the care and use of laboratory animals. All work was done using universal precautions at BSL2 under the IBC protocol # 17-11-01.
Results
Use of a Long-Term Vaccine Memory Model Using Outbred CD1 Mice
Immune memory against pertussis varies greatly depending on the vaccine used. It is estimated that the duration of protection conferred by wP vaccines lasts 4-12 years (48). For aP vaccines, immunity wanes much more quickly, as observed and underscored by the outbreaks in California and other locations (55). Surprisingly, the mechanisms underlying the differences in the longevity of protection provided by aP and wP vaccines remain unknown (45). In this study, we set out to establish a long-term pertussis immunization and challenge model to evaluate the duration of immunity and identify additional factors that contribute to either wP protection or the inadequate responses of aPs. To model the human outbred population, we selected outbred CD1 mice that are known to have a long lifespan. We aimed to compare the DTaP vaccine (Infanrix; GSK) to a prototype whole cell pertussis vaccine. Licensed whole cell pertussis vaccines are no longer available in the US; therefore, we used the standard NIBSC whole cell pertussis antigen vaccine for comparison. Unlike some whole cell pertussis vaccines, the NIBSC whole cell pertussis vaccine is not adjuvanted with alum nor combined with diphtheria and tetanus toxins. Female CD1 mice were mock-immunized (PBS), immunized with 1/10th human dose DTaP, or 1/10th human dose of NIBSC wP vaccine at six weeks of age by intramuscular administration and subsequently boosted three weeks later. We performed our analysis on mice at day 20 post prime (1 day pre-boost), day 22 (1 day post-boost), day 35 (2 weeks post-boost; Bp challenge), day 60 (~5.5 weeks post-boost; Bp challenge), day 90 (~10 weeks post-boost; Bp challenge), day 152 (~19 weeks post-boost; Bp challenge), day 365 (~49 weeks post-boost; Bp challenge), and at day 532 (~73 weeks post-boost; Bp challenge) (Figure 1A).
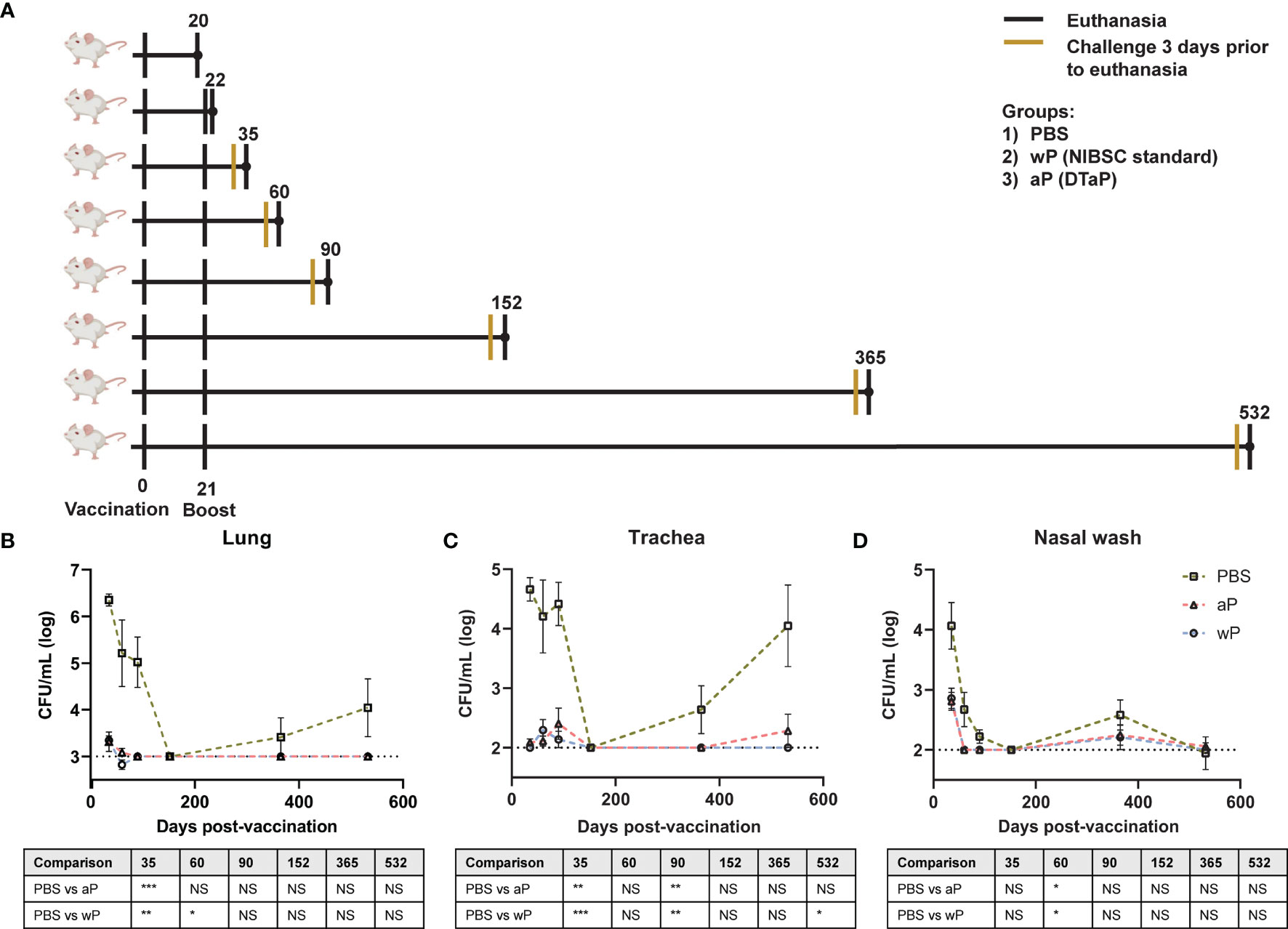
Figure 1 Susceptibility to B. pertussis in CD1 mice changes depending on age. (A) Experimental design and vaccination timeline. Mice were vaccinated at day 0 and boosted at day 21. Vertical black lines indicate the day that non-challenged mice were processed post-vaccination. Mustard colored lines represent groups of mice that were challenged three days prior to processing. (B–D) Bacterial burden in mice challenged with B. pertussis. Mice were vaccinated on day 0 with PBS, aP, or wP at 1/10th the human dose and boosted with the same vaccine at day 21. Mice were challenged with 2x107 CFU/dose by intranasal instillation 3 days prior to euthanasia. Bacterial burden of PBS vaccinated mice in the lung (B), trachea (C), and nasal wash (D). PBS was used as a vehicle control. Days 35, 60, and 90 are from two independent experiments (n=8). Days 152 (n=4), 365 (n=8), and 532 (n=4) were from one independent experiment each. Data were log10-transformed. The p-values were calculated for each time point using ANOVA followed by a Tukey’s multiple-comparison test, *p < 0.05, **p < 0.01, and ***p < 0.001. NS indicates no significance. Error bars are mean ± SEM values.
wP and aP Vaccines Provide Long-Term Protection in CD1 Mice Against Respiratory Challenge With B. pertussis
To design the next generation of pertussis vaccines, it is important to understand the underlying immunological cause of the relatively short-term immunity provided by aP vaccines. aP vaccines were originally developed and tested in mouse models that provided limited information regarding the longevity of protection. Furthermore, it remains to be determined if this model can mimic the waning immunity observed in humans. We hypothesized that mice immunized with 1/10th the human dose of wP and aP would be protected from challenge early on, but that protection would decrease over time in aP immunized mice. Mice were intranasally challenged with 2x107 CFU/dose of B. pertussis at multiple time points between day 35 and day 532 post-vaccination (25, 52). Mice were then euthanized three days post-infection to study vaccine-mediated protection. The bacterial burdens in the lung (Figure 1B), trachea (Figure 1C), and nasal wash (Figure 1D) were determined by plating serial dilutions and colony counting. Mice vaccinated with vehicle control (PBS) and intranasally challenged at days 35, 60, and 90 post-vaccination had high bacterial burden in the lung, trachea, and nasal wash. Surprisingly, vehicle control immunized mice challenged at day 152 post-vaccination had undetectable bacterial burden in the airways. By days 365 and 532 post-vaccination, bacterial burden was again detectable in the vehicle control mice. When comparing the protection of aP and wP vaccines over time, we observed that bacterial burden in the lung (Figure 1B), trachea (Figure 1C), and nasal wash (Figure 1D) was significantly decreased in immunized mice, regardless of the vaccine administered, compared to the vehicle control group at days 35, 60, and 90. Protection remained significant at day 532 in the trachea of wP immunized animals compared to mock-vaccinated animals (Figure 1C). At this dose, both aP and wP vaccines protected mice from intranasal challenge. The data illustrate different susceptibility patterns of CD1 mice to B. pertussis over time and the importance of longitudinal studies to identify the optimal timeframe to study vaccine-mediated protection.
Pertussis Specific Antibody Responses to aP and wP Immunization Persist as Far as Day 532 Post-Prime
To gain insights into the differences between aP and wP immunological responses, the model described above was applied to study pre- and post-boost responses, and their evolution over time. The subsequent studies were performed in non-challenged animals to clearly separate response to vaccination from response to challenge.
Antibodies play a major role in vaccine-mediated protection against numerous pathogens and are a common correlate of protection used to evaluate or predict vaccine efficacy (3, 56). DTP/wP and DTaP vaccines induce opsonizing antibodies that contribute to bacterial clearance. However, the value of some of these antibodies in protection against pertussis disease or B. pertussis infection is highly debated (57). Here, we hypothesized that antibody responses to B. pertussis antigens in aP immunized mice would decrease over time compared to wP immunized mice. We first measured anti-B. pertussis and anti-FHA IgG antibodies in the serum of immunized mice over time to determine the levels of surface-binding antibodies (Figures 2A, B). Both aP and wP immunization elicited significant production of anti-B. pertussis and anti-FHA IgG antibodies compared to vehicle-control immunized mice (Table S1). Anti-B. pertussis antibody levels were not statistically different between aP and wP vaccinated groups, and they peaked after boost and remained elevated out to day 532 post-prime (Figure 2A and Table S1). Anti-FHA antibodies also increased after boost and remained elevated at day 532 post-prime (Figure 2B).
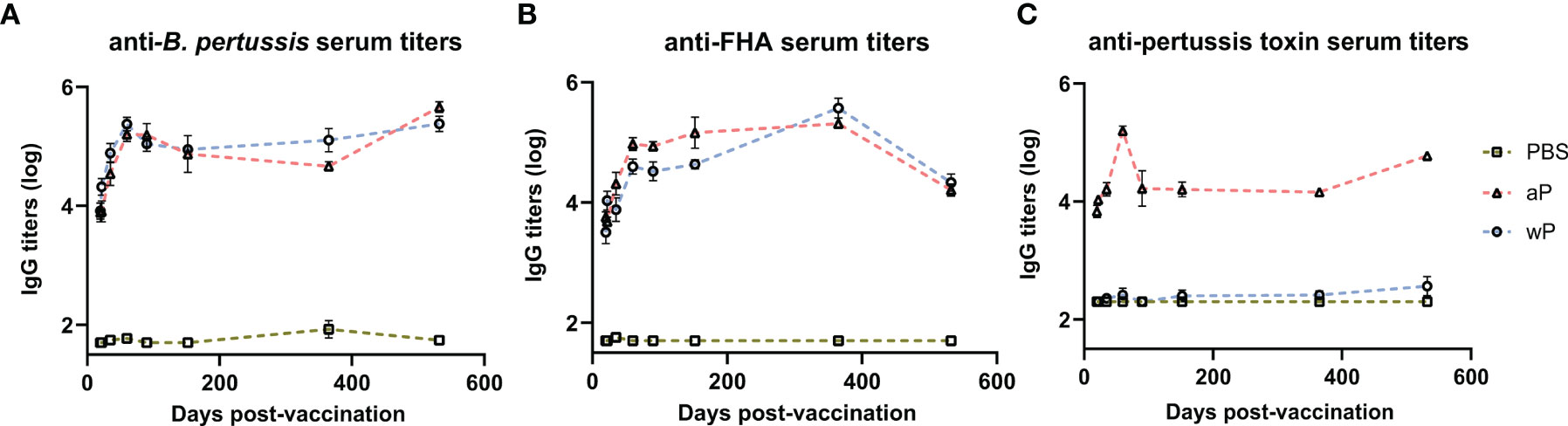
Figure 2 Pertussis specific antibody responses peak after boost and persist over time in immunized CD1 mice. Measured in serum of non-challenged mice collected at day 20, day 22, day 35, day 60, day 90, day 152, day 365, and day 532 post-vaccination. (A) IgG anti-B. pertussis antibodies in vaccinated mice (B) IgG anti-FHA antibodies in vaccinated mice (C) IgG anti-PT antibodies in vaccinated mice. Antibody responses were at or below the limit of detection in PBS vaccinated mice. To note, there is a lack of PT in the NIBSC wP formulation due to manufacturing practices. Days 20 and 22 were from three independent experiments (n=12). Day 35 is from four independent experiments (n=16). Days 60 and 90 were from two independent experiments (n=8). Days 152 (n=4), 365 (n=8), and 532 (n=4) are from one independent experiment. Data were log10-transformed. Statistical analysis and p values are shown in Table S1.
In addition to opsonizing antibodies, acellular pertussis immunization stimulates the production of PT-neutralizing antibodies that can prevent symptoms, disease manifestation, and in the case of infants, death (58–60). Anti-PT antibodies have been proposed as a correlate of protection against pertussis; however, this is highly debated (57, 61). PT is an AB5 exotoxin that plays a key role in the pathogenesis of pertussis by triggering ADP-ribosylation which inhibits G protein-coupled signaling (24, 62–66). PT activity leads to a decrease in airway macrophages, induction of leukocytosis, and modulation of adaptive immune responses to B. pertussis. Significant anti-PT IgG antibody responses were only observed in aP vaccinated animals compared to both vehicle control and wP immunized mice (Figure 2C and Table S1). The lack of anti-PT IgG antibody responses in wP immunized animals is not surprising as the bacteria used to produce wPs are washed during the manufacturing process removing the majority of PT since it is secreted from the cell. Overall, we observed that anti-PT antibodies in the serum of aP vaccinated mice peaked at day 60 post vaccination and remained elevated until day 532.
DTaP is a combination vaccine also containing diphtheria toxoid and tetanus toxoid. Unlike the immunity to pertussis conferred by DTaP and Tdap immunization, immunity against diphtheria and tetanus does not wane overtime in humans (67). We observed that aP immunization elicits significant anti-diphtheria toxoid (Figure S1A) and anti-tetanus toxoid (Figure S1B) IgG antibody production compared to vehicle control mice at all time points. The anti-diphtheria toxoid and anti-tetanus toxoid IgG responses were similar to anti-PT responses as they increased significantly after prime and boost and remained high out to day 532 post-vaccination. Overall, the data indicate that antibodies against the whole bacterium, FHA, PT, diphtheria toxoid, and tetanus toxoid peaked post-boost and remained stable throughout the course of the study in aP immunized mice.
wP But Not aP Immunization Induces Significant TFH Cell and CXCL13 Responses
The presence of antibodies over a long period of time indicates that B. pertussis antigen-specific plasmablasts were produced in response to vaccination, were alive, and likely being repopulated (68). However, antibody titers themselves do not directly predict vaccine recall capacity (69). Conversely, cells associated with GC activity, such as TFH cells and MBCs, are critical populations that dictate recall capacity. GC reactions take place in secondary lymphoid organs such as the spleen and lymph nodes (70). Initiation of GC formation and the development of immunological memory relies on TFH cells. TFH cells are crucial for the survival and proliferation of B cells within the GC, and ultimately affinity maturation of B cells (40, 71). Our objective was to quantify TFH cells in the secondary lymphoid organs of immunized mice to better understand how wP versus aP immunization influences GC activity.
First, we measured the absolute count of TFH cells (CD4+CD3ϵ+CXCR5+PD-1+) (72–74) per sample in the draining lymph node and spleen (data not shown) of immunized mice at time points between day 20 and day 532 post-vaccination (Table S2). TFH cells play a crucial role in germinal center formation, and therefore are important early after vaccination. In the right inguinal lymph node (Figure 3A) immunization with wP induced significant TFH responses compared to PBS immunized mice 35 days post-vaccination. Although no significant differences were observed between immunized animals at other time points, TFH cells in the draining lymph node at days 20, 22, 35, and 90 were more numerous in the wP group (Figure 3B). Consistent with previous studies with other vaccines, a greater number of TFH cells were detected at day 35, an early time point, in wP vaccinated animals (Figure 3A) (75–77).
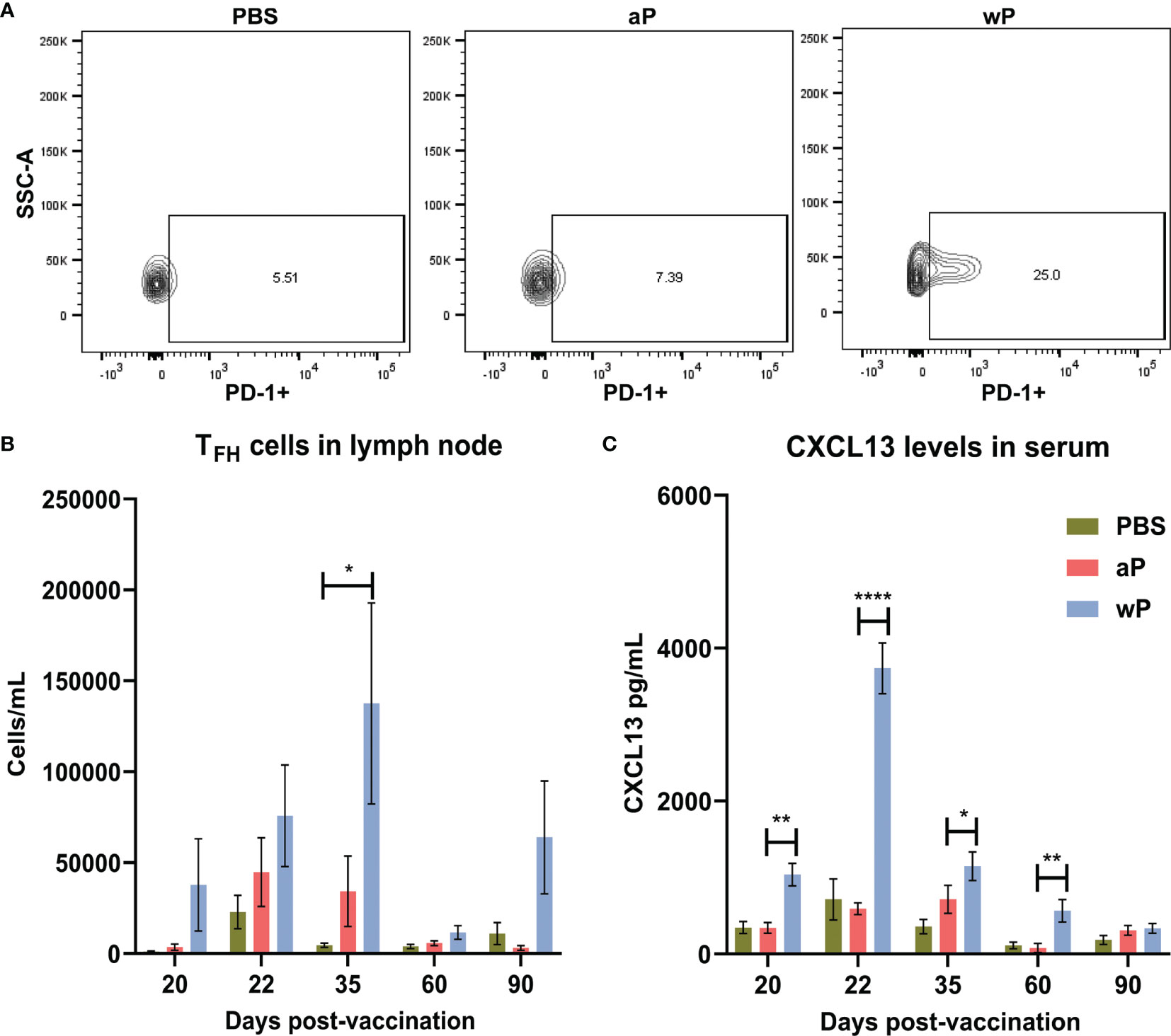
Figure 3 T follicular helper cell and CXCL13 responses in the draining lymph node are significantly increased in wP immunized CD1 mice compared to mock immunized animals. Flow cytometry was performed using single cell suspensions from the draining lymph node to identify TFH cells. (A) Representative contour plots of PBS, aP, and wP groups showing TFH cells gated on CD4+CD3ϵ+CXCR5+PD-1+. (B) Group comparisons of TFH cells in the draining lymph node of non-challenged, immunized mice. (C) CXCL13 levels (pg/mL) were measured in the blood serum of non-challenged, immunized mice. Day 20 is from one independent experiment (n=4). Day 22, 60, and 90 are from two independent experiments (n=8). Day 35 is from four independent experiments (n=16). The p-values were calculated for each time point using ANOVA followed by a Tukey’s multiple-comparison test, *p < 0.05, **p < 0.01, and ****p < 0.0001. Error bars are mean ± SEM values.
To facilitate their function in the GCs, TFH cells express the chemokine CXCL13 which is a signaling molecule that plays a crucial role in B cell recruitment and GC organization through binding to CXCR5 (40). While CXCL13 can be found locally in GCs it can also be measured in the serum as a biomarker of GC activity (42). Germinal center reactions are not indefinite, as they occur roughly three weeks after antigen exposure, and can form upon subsequent exposure (70). Therefore, we measured CXCL13 levels in the serum of immunized mice during the course of this experiment as an indirect measurement of germinal center formation and activity (Figure 3C). Levels of CXCL13 in the serum of vehicle control and aP-immunized mice were low (Figure 3C). In contrast, we observed that only wP immunization elicits significant production of CXCL13 compared to both aP and mock-immunized mice. CXCL13 levels in wP vaccinated animals peaked at day 22 post-vaccination and were significantly higher than aP mice at days 20 and 60 post-vaccination. Overall, the data suggest that TFH cells and CXCL13 responses are differentially regulated in response to aP and wP early after vaccination, and that GC responses are greater in wP-vaccinated animals.
wP Immunization Induces B. pertussis Specific Memory B Cells in CD1 Mice
MBCs are a vital component of the host’s immune system involved in protection against invading pathogens (78). This population of cells is a product of the GC reaction and can be found in the spleen, lymph nodes, and in circulation. MBCs are quiescent until recognition of antigen occurs (78). These cells can then rapidly respond by differentiating into plasma cells and mounting an antibody response (78). As MBCs are an important product of the GC reaction and a key component of immunological memory, we sought to measure these cells over the course of this study in response to vaccination against B. pertussis.
To study B. pertussis specific MBCs, we incubated splenocytes with fluorescently labeled B. pertussis. We then separated MBCs from the rest of the splenocytes using a proprietary kit (Miltenyi), followed by labeling with CD3ε, CD45R, IgG, CD38, and CD80. We analyzed MBC populations based on labeling with B. pertussis (antigen-specific) and further defined the B cell populations based on CD38 and CD80 surface expression (79–81) (Figure S2). CD38 is an ectoenzyme with various functions and a transmembrane receptor in immune cells such as B cells (82). CD38 is involved in B cell regulation and CD38 knockout mice exhibit deficiencies in antibody responses that result from T-cell-dependent antigens (82–84). CD80 is a costimulatory molecule that plays a role in B and T cell interactions, and is expressed by both human and murine MBCs (85).
We observed significant expansion of B. pertussis+ MBCs in wP immunized mice compared to both mock and aP immunized mice (Figure 4A; Table S2; Figure S2). In the wP group, this population peaked at day 35 post-boost, and although not statistically different from the mock vaccinated control group, B. pertussis+ MBCs were measurable at days 152 and 365 post-prime in wP vaccinated mice (Figures 4A, B). We observed that in vaccinated animals, B. pertussis+ MBCs tend to be double positive for CD38+CD80+ out to day 152 post-prime, while B. pertussis- MBCs are mainly CD38+ (Figures 4C–F). This is likely important as IgG+ CD80+ MBCs have been shown to differentiate into antibody secreting cells with the capacity to seed GCs (78, 80). Interestingly, single-labeled CD80+ MBCs were low in both B. pertussis+ and B. pertussis- populations. Overall, the data show that significant production of B. pertussis+ MBCs is most prevalent in wP immunized mice, and that B. pertussis+ MBCs are characterized by a unique combination of cell surface marker profiles.
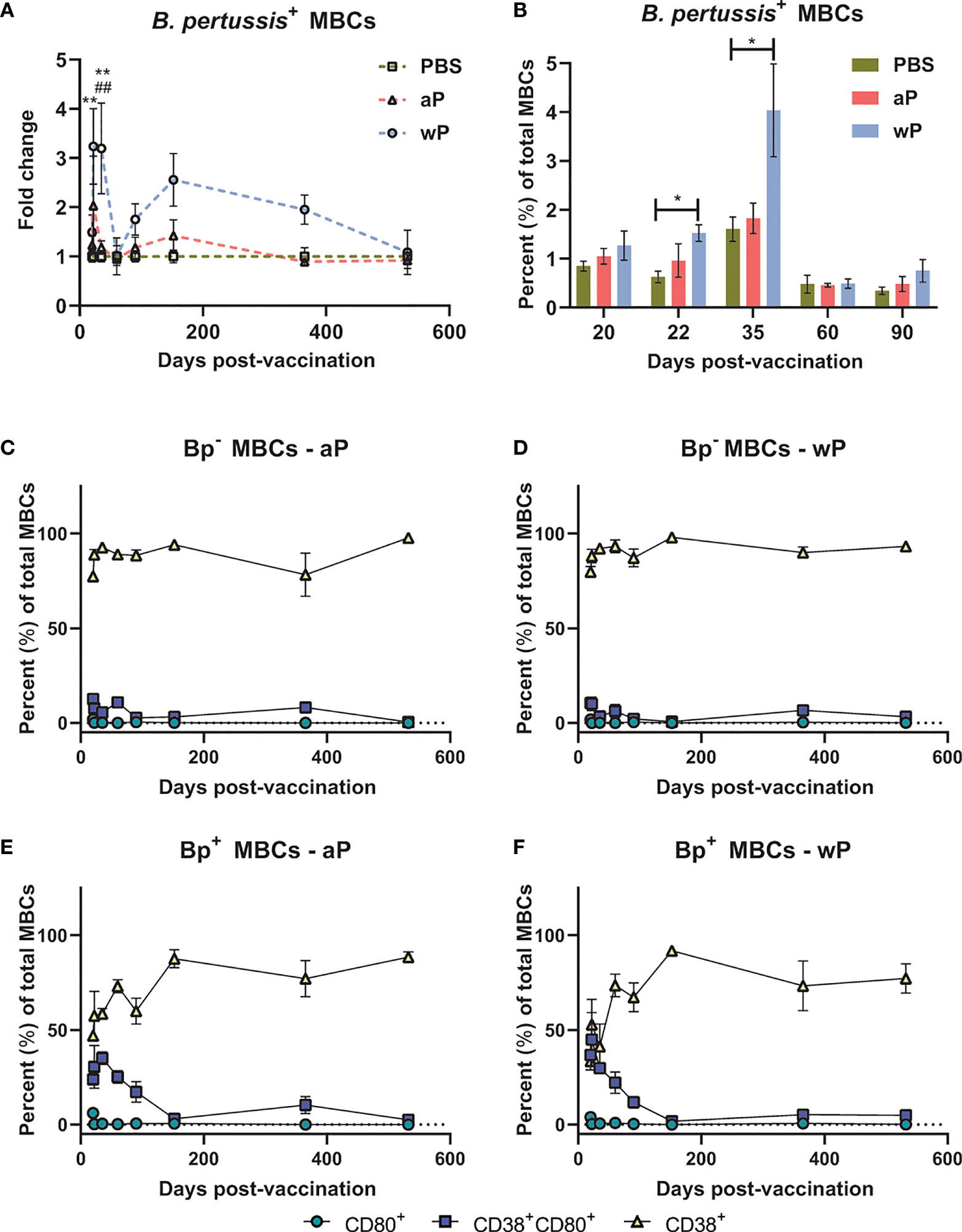
Figure 4 B. pertussis+ memory B cells are elevated in wP immunized CD1 mice. Flow cytometry was performed using single cell suspensions from the spleen of immunized non-challenged mice. B. pertussis+ MBCs were identified following the protocol outlined in the methods. Extracellular markers were used to label B. pertussis+ MBCs. (A) Fold change of B. pertussis+ MBCs in aP and wP groups compared to PBS-vaccinated mice. (B) Percent total B. pertussis+ MBCs. (C–F) show the percent of CD80+, CD38+CD80+, and CD38+ MBCs in immunized mice. (C) B. pertussis- MBCs in aP immunized animals. (D) B. pertussis- MBCs in wP immunized animals. (E) B. pertussis+ MBCs in aP immunized animals. (F) B. pertussis+ MBCs in wP immunized animals. Days 20, 60, 152, 365 (n=8), and 532 are from one independent experiment each (n=4). Days 22, 35, and 90 are from two independent experiments (n=8). The p-values were calculated for each time point using mixed-effects analysis with a Tukey’s multiple comparison test, *p < 0.05 and **p < 0.01 denote comparison to PBS. ##p < 0.01 denotes comparison of wP to aP.
Immunization Against B. pertussis Elicits Production of Antibody Secreting Cells in Mice
In the GC, TFH cells provide signals that recruit naïve B cells and trigger affinity maturation. B cells that undergo this process can differentiate into plasma cells that migrate to the bone marrow and function to secrete antibodies and protect from infection. Long-lived plasma cells are terminally differentiated cells that can survive and continue to secrete antibodies for years, which has been demonstrated in both humans and mice (86). We hypothesized that wP immunization would induce greater production of pertussis-specific long-lived plasma cells compared to aP immunization, mimicking the waning immunity observed in the human population. Therefore, we isolated bone marrow cells and quantified the number of total long-lived plasma cells and the number of antigen-specific antibody secreting cells.
Using flow cytometry, we first observed that there was no difference in the number of total long-lived plasma cells (CD19-CD45R+CD138+) (87) in the bone marrow in any of the groups at any of the time points studied (data not shown). To determine the number of antigen-specific antibody secreting cells, we performed B cell ELISPOT on bone marrow samples (Figures 5A–F). We only observed a significant increase in the number of anti-B. pertussis antibody secreting cells in wP immunized mice one day post-boost compared to both mock and aP vaccinated animals (Figure 5B). Although there were no significant differences in antibody secreting cells at days 20 and 532 post-prime (Figures 5A, C), wP immunized animals had higher numbers of spots overall (Figures 5A–C). This contrasts with what was observed in the serological studies in which anti-B. pertussis titers remained high during the course of the study (Figure 2A).
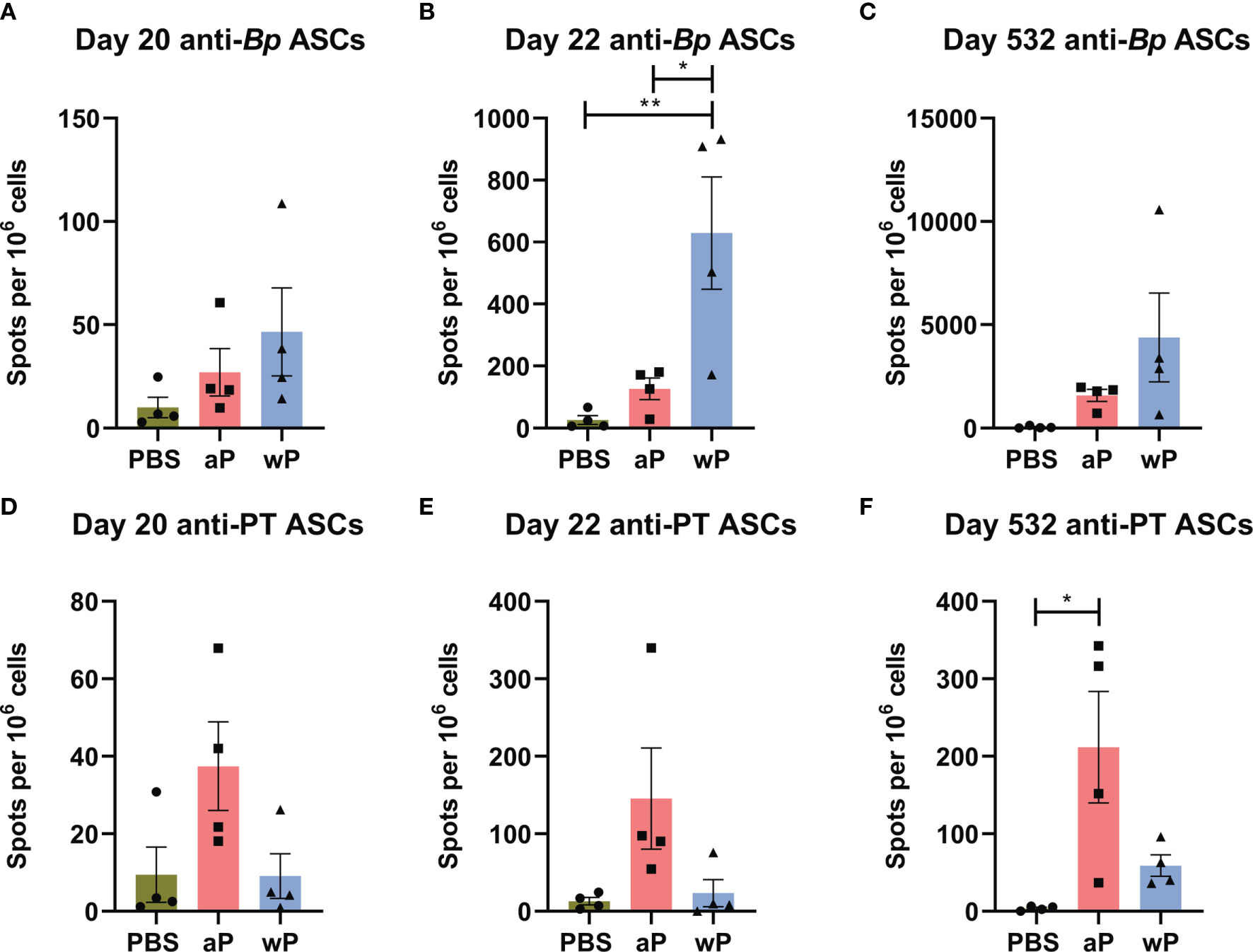
Figure 5 Anti-B. pertussis and anti-pertussis toxoid antibody secreting cells (ASCs) in immunized CD1 mice persist as far as day 532 post-prime. ASCs were analyzed at day 20, 22, and 532 post-vaccination in non-challenged, immunized mice. Anti-B. pertussis ASCs are shown for day 20 (A), day 22 (B), and day 532 (C). Anti- pertussis toxoid ASCs are shown for day 20 (D), day 22 (E), and day 532 (F). To note, there is a lack of PT in the NIBSC wP formulation due to manufacturing practices. All time points are from one independent experiment (n=4) and day 365 (n=8). The p-values were calculated using ANOVA followed by a Tukey's multiple-comparison test, *p < 0.05 and **p < 0.01. Error bars are mean ± SEM values (n=4), aP (n=4), and wP (n=4).
In this study, we also observed that anti-pertussis toxoid antibody secreting cells were present in greater numbers in aP mice compared to vehicle or wP mice, and persisted out to day 532 post-vaccination (Figure 5F). Notably, the number of anti-PT antibody secreting cells was significantly greater at day 532 post-prime in aP immunized animals (Figure 5F). This observation is consistent with the anti-PT titers detected in the serological study (Figure 2C) and with the fact that the wP vaccine used in this study contains minimal pertussis toxin. The data highlight important differences between serological detection of B. pertussis antibodies and quantification of antibody-producing cells in the bone marrow.
Altogether, the data described in this study highlight important differences in the immunological signatures triggered by aP and wP vaccination in mice (Figure 6). wP vaccination was characterized by stronger TFH cell responses, CXCL13 production, B. pertussis+ MBCs, and anti-B. pertussis antibody secreting cells (Figures 6, 5), compared to aP vaccination. These markers correlate with the stimulation of, or result from GC reactions, suggesting that wP vaccination triggers stronger follicular responses and vaccine-induced memory (Figure 6).
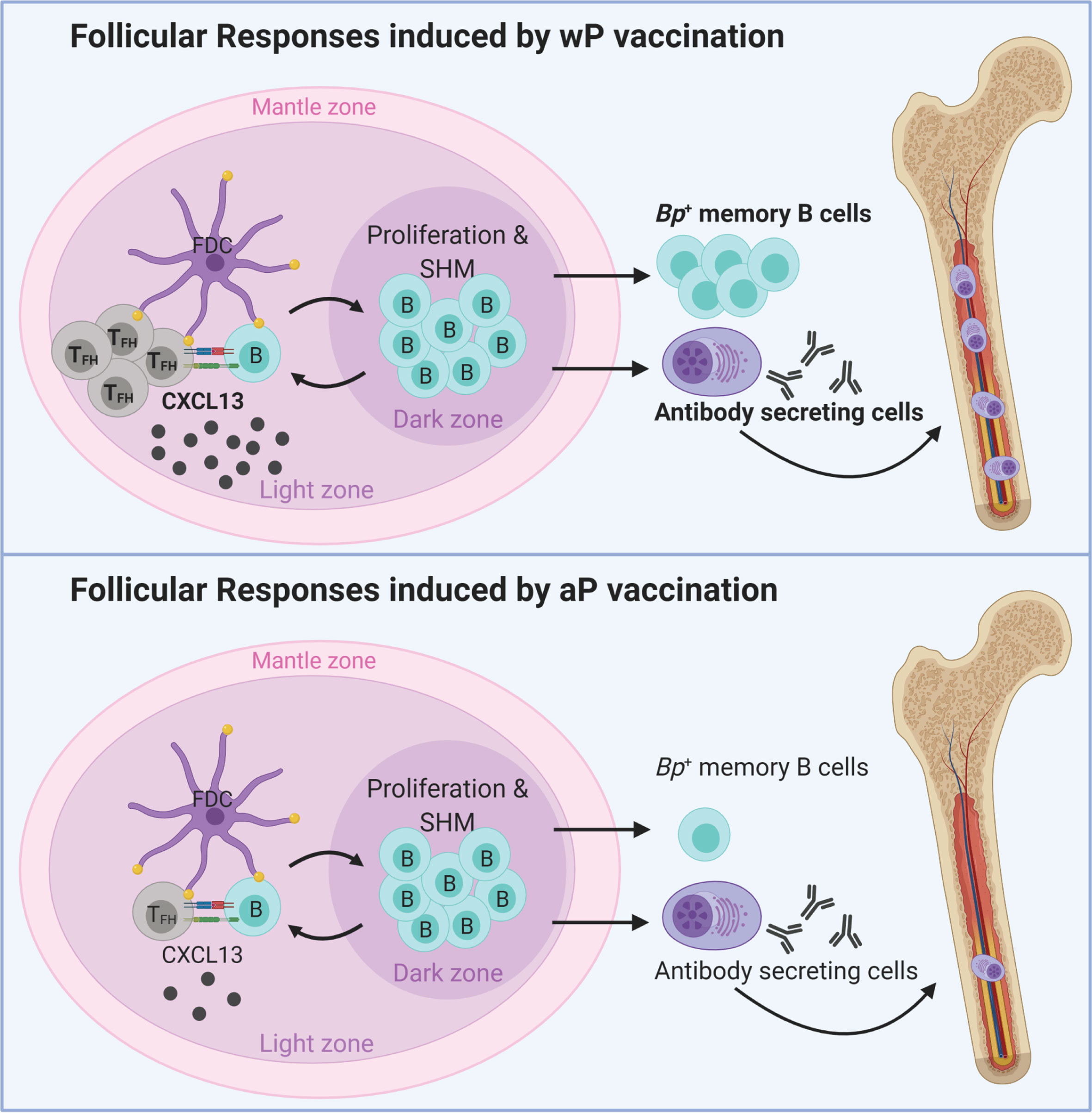
Figure 6 Model of vaccination and follicular responses highlighting differences in aP versus wP immunization. Created with Biorender.com.
Discussion
Pre-clinical animal models of vaccination and challenge have provided important insights into pertussis immunity. However, the majority of the recent conversations around pertussis vaccine immunity are focused on the pertussis T helper and T memory cell responses (27, 57, 88). Furthermore, “longevity of protection” markers have not truly been identified in neither animals nor humans. Identifying biomarkers associated with vaccine-induced immunity that predict longevity of protection could bridge animal models and human vaccine trials, and help develop the next generation of pertussis vaccines. In the past, pertussis vaccine potency and immunogenicity were measured in pre-clinical models utilizing the Kendrick test, a lethal intracranial pertussis challenge model (19). To replace the intracranial challenge model, lethal and sub-lethal aerosol and intranasal murine challenge models were utilized to measure pertussis vaccine efficacy (89–91). The sub-lethal intranasal challenge, lung infection, model demonstrated statistical and reproducible differences in protection conferred by vaccination in short term experiments. These studies were helpful for determining that both cellular and humoral responses are involved in pertussis vaccine-mediated protection (89, 92). However, to this date, no absolute correlate of protection has been identified in the mouse model that can predict longevity of protection in humans.
During the development of acellular pertussis vaccines, immunogenicity of candidate vaccines was assessed in animals and humans (93–96). Antibodies to PT, FHA, fimbriae, pertactin, DT, and TT were measured in immunized infants, along with toxin neutralization assays to determine levels of agglutinating antibodies (97). In humans, immunoglobulin levels are currently measured to provide an approximation of vaccine efficacy; however, these metrics do not predict the duration of immunological memory and protection. Unfortunately, rarely in pertussis studies is the timing and longevity of protection considered due to the obvious challenges. This study addresses this gap in knowledge by determining long-term aP and wP vaccine-mediated protection out to day 532 post-vaccination, which we suspect is the longest-lasting pertussis vaccine study in mice performed to date. In this study we used numerous approaches to characterize the follicular response to pertussis vaccination including antibody titers to vaccine antigens, CXCL13 levels in sera, TFH cells, B. pertussis specific MBCs, and B. pertussis/PT specific bone marrow antibody secreting cells (Figure 6). We also identified serum levels of CXCL13 and B. pertussis-specific MBCs as potential biomarkers of pertussis vaccine-induced immune memory. Additional studies are needed to determine their exact function in pertussis immunity in humans, and their potential as biomarker of vaccine-mediated memory against B. pertussis.
One of the challenges associated with monitoring the longevity of vaccine-mediated protection is that the model used must remain susceptible to infection over time. This was illustrated with the infant baboon model, which allows vaccine schedules to be studied and recapitulates human disease, but in which adults are not susceptible to pertussis infection (~15 months of age). Therefore, we first studied the susceptibility of mice to B. pertussis over time. We assessed bacterial burden in the respiratory system of mice (lungs, trachea, and nasal wash) and found that susceptibility appears to change overtime, as seen in humans (Figures 1B–D). Neonates and unvaccinated infants are highly susceptible to pertussis infection; however, susceptibility decreases as they age toward adulthood. Furthermore, adults over 65 years of age are more likely to be hospitalized for pertussis than younger adults (98, 99). We observed a similar pattern of susceptibility in our vehicle control mice, in which mice between days 35 and 90 post-vaccination were susceptible to infection, but bacterial burden was below the limit of detection by day 152 post-vaccination. Bacterial burden was again detectable by day 365 post-vaccination.
One potential explanation behind the fluctuation in susceptibility to B. pertussis in mice, is that we suspect that like pigs, mice have differential expression over time of some inhibitory factors such as beta-defensin 1 that may render mice no longer susceptible to B. pertussis (22, 100). Alternatively, specific microbiota in the airway might out compete the challenge dose (101). These data highlight the importance of conducting these types of studies over a long period of time, as intermediate lengths of studies may not allow measurement of vaccine-mediated protection due to low susceptibility. This is also important as susceptibility to B. pertussis in humans and in particular, death associated with pertussis, varies over time. Combinations of neonatal models with long-term models may be able to better evaluate pertussis vaccine-induced memory. A caveat to using the long-term mouse model is that the lifespan of laboratory mice is about 24 months (102). Additionally, inbred mice may have different windows of susceptibility that should be considered and studied further. Mahon et al. used BALB/c mice in long-term studies and control animals were susceptible to challenge at both 6 and 44 weeks after primary immunization (103). Wolf et al. also conducted short-term and long-term studies using BALB/c mice and found that mock-vaccinated mice were susceptible to infection at both day 35 and 7 months, 3 days after challenge (25). In a long-term study with the attenuated live vaccine BPZE1, BALB/c mice were susceptible to infection with B. pertussis at 3, 6, 9, and 12 months after vaccination (104). It is unclear why susceptibility changes over time in CD1 mice, and additional studies are needed to determine the cause, and if this phenomenon is strain or gender-specific.
In this study, we observed that aP and wP protected mice overtime by decreasing bacterial burden in both the upper and lower airways. These findings contrast with other studies that used different bacterial challenge doses, vaccine doses, and mouse strains in which they observed that both aP and wP protect against lung infection, but only wP protects against nasal colonization (105, 106). Interestingly, Wolf et al. reported that aP protects mice from upper and lower respiratory infection using the same challenge dose and volume than this study, and a relatively high vaccine dose (1/20th) in a different mouse strain (25). This suggests that factors such as challenge dose, volume, vaccine dose, and strain have an impact on the establishment of respiratory infection in mice and that these factors need to be considered in study design.
In humans, studies clearly show that antibody titers against pertussis decay over time (11, 107). For example, human serum titers against PT decay as quickly as 6-12 months after vaccination whereas anti-tetanus serum titers last up to 19 years (108, 109). Similar to humans, BALB/c mice immunized with a low dose of wP or aP elicited high serum IgG antibody titers that increased rapidly after vaccination, but were undetectable by 6-9 months (92, 103). However, in our model and at 1/10th the human dose of the vaccines, we did not observe antibody decay over time. In fact, antibody levels peaked after boost and remained elevated out to day 532 post-vaccination (Figure 2 and Figure S1). These observations could be due, in part, to the relatively high dose of vaccine used here, and correlate with the sustained protection provided by both aP and wP vaccines over time. To note, no anti-PT IgG antibodies were detected in wP immunized animals, consistent with the lack of PT in the NIBSC wP formulation due to manufacturing practices (Figure 2C). This data also recapitulates what is observed in humans in which wP vaccinated individuals have low anti-PT antibody responses in comparison to aP vaccinated individuals (110, 111).
To further investigate the humoral immune response to B. pertussis vaccination, we measured B. pertussis+ MBCs in the spleen of immunized mice (Figure 4 and Figure S2) and the presence of antibody-secreting cells in the bone marrow. We observed that wP immunization elicited significant B. pertussis-specific MBC responses compared to both PBS and aP immunization (Figures 4A, B). Additionally, CD38+CD80+ cells were associated with B. pertussis+ MBCs but not B. pertussis- MBCs (Figures 4C–F). The unique memory profile associated with B. pertussis+ MBCs support the hypothesis that B memory exists on a spectrum and could influence vaccine-induced memory. Further studies are needed to elucidate the importance of the notable cell marker profile associated with B. pertussis+ MBCs.
Detection of antigen-specific MBCs from the spleen and antibody secreting cells in the bone marrow provides insight into the differences between wP and aP vaccine-induced immunity. Unfortunately, the protocols described here to detect antigen-specific MBCs are time consuming and technically challenging due to the rarity of these populations. Therefore, the implementation of antigen specific MBC analysis for clinical evaluation of pertussis vaccines in humans is unlikely. In addition, detection of antibody secreting cells requires invasive procedures to obtain samples for analysis, making its implementation unfavorable at the clinical level.
To identify additional markers of vaccine-induced memory, we measured CXCL13 in immunized mice as it has previously been measured in sera from humans and would be more feasible to monitor in clinical studies (112). CXCL13 levels in the serum of immunized (non-challenged) mice peaked one day post-boost in wP-, but not in aP-immunized mice (Figure 3C), again highlighting another difference between both vaccines (Figure 6). CXCL13 levels were significantly increased in wP compared to aP immunized animals both pre-boost and post-boost as far as day 60 post-vaccination (Figure 3C). Additional studies are needed to monitor the production of CXCL13 earlier on after vaccine priming. There appears to be a narrow window of CXCL13 production in mice, likely consistent with GC formation in response to vaccination. CXCL13 is a reliable plasma biomarker of GC activity in both humans and nonhuman primates (42). Therefore, measuring CXCL13 levels post-vaccination using a minimally invasive blood draw could be utilized during clinical studies when testing candidate pertussis vaccines in humans.
There are obvious caveats to using CXCL13 as a biomarker for the longevity of pertussis vaccine-induced memory that need to be considered when designing clinical trials. The first is that CXCL13 is not antigen-specific. Another caveat is that CXCL13 expression is altered in response to infection, in cancer, systemic lupus erythematosus, rheumatoid arthritis, and other diseases involving germinal center, or ectopic lymphoid structure formation (113, 114). This should be taken into account when establishing exclusion criteria for clinical trials.
From this work, we propose that CXCL13, circulating B. pertussis+ MBCs, and pertussis specific antibody titers should be evaluated further to determine their potential as biomarkers for pertussis vaccine-induced immunity (Figure 6). These parameters could be measured together in the blood of patients enrolled in clinical trial vaccine trials to inform the longevity of vaccine-mediated protection. Future studies need to address if follicular responses induced by aP vaccination can be improved to levels similar to that induced by wP vaccination. Addition of adjuvants known to stimulate GC formation could enhance the longevity of aP vaccines and prevent waning immunity. This work strongly suggests that GC quantification, size, and composition should be evaluated in response to vaccination to guide formulations of the next generation pertussis vaccine that provide long-term protection.
Data Availability Statement
The original contributions presented in the study are included in the article/Supplementary Material. Further inquiries can be directed to the corresponding author.
Ethics Statement
The animal study was reviewed and approved by West Virginia University Institutional Animal Care and Use Committees (WVU-AUCU protocol 1901021039).
Author Contributions
FHD, MB, and KLW were responsible for conceptualization of the study. MB, KLW, and FHD prepared the initial draft and all other authors participated in manuscript editing and revision. KLW and AMH were involved in protocol development, data analysis and validation. KLW, MB, CBB, GMP, ES-K, EG, ABH, WTW, MAD, and MAW assisted with experimentation and data collection. All authors contributed to the article and approved the submitted version.
Funding
The study was supported by a 1R01AI14167101A1 to MB. KLW received funding from the Cell and Molecular Biology and Biomedical Engineering Training Program funded by NIGMS grant T32 GM133369 (2019-2021), as well as the NASA West Virginia Space Grant Consortium Graduate Research Fellowship Program, Grant #80NSSC20M0055 (2021-2022). FHD is supported by NIH through grants 1R01AI137155-01A1 and 1R01AI153250-01A1. The WVU Vaccine Development Center, MB and FHD are supported by a Research Challenge Grant No. HEPC.dsr.18.6 from the Division of Science and Research, WV Higher Education Policy Commission. Flow Cytometry experiments were performed in the West Virginia University Flow Cytometry & Single Cell Core Facility, which is supported by the National Institutes of Health equipment grant number S10OD016165 and the Institutional Development Awards (IDeA) from the National Institute of General Medical Sciences of the National Institutes of Health under grant numbers P30GM121322 (TME CoBRE) and P20GM103434 (INBRE).
Conflict of Interest
The authors declare that the research was conducted in the absence of any commercial or financial relationships that could be construed as a potential conflict of interest.
Publisher’s Note
All claims expressed in this article are solely those of the authors and do not necessarily represent those of their affiliated organizations, or those of the publisher, the editors and the reviewers. Any product that may be evaluated in this article, or claim that may be made by its manufacturer, is not guaranteed or endorsed by the publisher.
Acknowledgments
This article was submitted as a preprint to BioRxiv doi: https://doi.org/10.1101/2021.10.01.462695 (115). The authors would like to thank Dr. Kathleen Brundage, director of the WVU Flow Cytometry and Single Cell Core Facility for her support. The Authors would like to thank the reviewers for their thoughtful comments that improved the quality of the manuscript.
Supplementary Material
The Supplementary Material for this article can be found online at: https://www.frontiersin.org/articles/10.3389/fimmu.2022.838504/full#supplementary-material
References
1. Centers for Disease Control and Prevention. Pertussis. In: Hamborsky J, Kroger A, Wolfe C, editors. Epidemiology and Prevention of Vaccine-Preventable Diseases, 13th ed. Washington, D.C: Public Health Foundation (2015). p. 261–77. Available at: https://www.cdc.gov/vaccines/pubs/pinkbook/pert.html.
2. Klein NP. Licensed Pertussis Vaccines in the United States: History and Current State. Hum Vaccines Immunother (2014) 10(9):2684–90. doi: 10.4161/hv.29576
4. Mattoo S, Cherry JD. Molecular Pathogenesis, Epidemiology, and Clinical Manifestations of Respiratory Infections Due to Bordetella Pertussis and Other Bordetella Subspecies. Clin Microbiol Rev (2005) 18(2):326. doi: 10.1128/CMR.18.2.326-382.2005
5. Chen Z, He Q. Immune Persistence After Pertussis Vaccination. Hum Vaccines Immunother (2017) 13:744–56. Taylor and Francis Inc. doi: 10.1080/21645515.2016.1259780
6. Warfel JM, Edwards KM. Pertussis Vaccines and the Challenge of Inducing Durable Immunity. Curr Opin Immunol (2015) 35:48–54. doi: 10.1016/j.coi.2015.05.008
7. Misegades LK, Winter K, Harriman K, Talarico J, Messonnier NE, Clark TA, et al. Association of Childhood Pertussis With Receipt of 5 Doses of Pertussis Vaccine by Time Since Last Vaccine Dose, California, 2010. JAMA (2012) 308(20):2126–32. doi: 10.1001/jama.2012.14939
8. Witt MA, Katz PH, Witt DJ. Unexpectedly Limited Durability of Immunity Following Acellular Pertussis Vaccination in Preadolescents in a North American Outbreak. Clin Infect Dis (2012) 54(12):1730–5. doi: 10.1093/cid/cis287
9. Hill HA, Elam-Evans LD, Yankey D, Singleton JA, Kang Y. Vaccination Coverage Among Children Aged 19–35 Months — United States, 2016. MMWR Morb Mortal Wkly Rep (2017) 66(43):1171. doi: 10.15585/mmwr.mm6643a3
10. Pertussis. Whooping Cough | Outbreaks. Atlanta, GA:CDC (2019). Available at: https://www.cdc.gov/pertussis/outbreaks.html.
11. Klein NP, Bartlett J, Rowhani-Rahbar A, Fireman B, Baxter R. Waning Protection After Fifth Dose of Acellular Pertussis Vaccine in Children. N Engl J Med (2012) 367:1012–21. doi: 10.1056/NEJMoa1200850
12. Zerbo O, Bartlett J, Goddard K, Fireman B, Lewis E, Klein NP. Acellular Pertussis Vaccine Effectiveness Over Time. Pediatrics (2019) 144(1):2018–3466. doi: 10.1542/peds.2018-3466
13. Tartof SY, Lewis M, Kenyon C, White K, Osborn A, Liko J, et al. Waning Immunity to Pertussis Following 5 Doses of DTaP. Pediatrics (2013) 131(4):e1047–52. doi: 10.1542/peds.2012-1928
14. Brummelman J, Wilk MM, Han WGH, van Els CACM, Mills KHG. Roads to the Development of Improved Pertussis Vaccines Paved by Immunology. Pathog Dis (2015) 73(8):ftv067. doi: 10.1093/femspd/ftv067
15. Bart MJ, Harris SR, Advani A, Arakawa Y, Bottero D, Bouchez V, et al. Global Population Structure and Evolution of Bordetella Pertussis and Their Relationship With Vaccination. MBio (2014) 5(2):e01074–14. doi: 10.1128/mBio.01074-14
16. Pawloski LC, Queenan AM, Cassiday PK, Lynch AS, Harrison MJ, Shang W, et al. Prevalence and Molecular Characterization of Pertactin-Deficient Bordetella Pertussis in the United States. Clin Vaccine Immunol (2014) 21(2):119. doi: 10.1128/CVI.00717-13
17. Williams MM, Sen KA, Weigand MR, Skoff TH, Cunningham VA, Halse TA, et al. Bordetella Pertussis Strain Lacking Pertactin and Pertussis Toxin. Emerg Infect Dis (2016) 22(2):319. doi: 10.3201/eid2202.151332
18. Leber AL. Pertussis Relevant Species and Diagnostic Update. Clin Lab Med (2014) 34:237–55. doi: 10.1016/j.cll.2014.02.003
19. Xing D, Markey K, Gaines Das R, Feavers I. Whole-Cell Pertussis Vaccine Potency Assays: The Kendrick Test and Alternative Assays. Expert Rev Vaccines (2014) 13(10):1175–82. doi: 10.1586/14760584.2014.939636
20. Sato Y, Izumiya K, Sato H, Cowell JL, Manclarkw CR. Aerosol Infection of Mice With Bordetella Pertussis. Infect Immun (1980) 29:261–66. doi: 10.1128/iai.29.1.261-266.1980
21. Sato H, Sato Y. Protective Antigens of Bordetella Pertussis Mouse-Protection Test Against Intracerebral and Aerosol Challenge of B. Pertussis. Dev Biol Stand (1985) 61:461–7.
22. Mills KHG, Gerdts V. Mouse and Pig Models for Studies of Natural and Vaccine-Induced Immunity to Bordetella Pertussis. J Infect Dis (2014) 209(suppl_1):S16–9. doi: 10.1093/infdis/jit488
23. Solans L, Locht C. The Role of Mucosal Immunity in Pertussis. Front Immunol (2019) 10:3068. Frontiers Media S.A. doi: 10.3389/fimmu.2018.03068
24. Scanlon KM, Snyder YG, Skerry C, Carbonetti NH. Fatal Pertussis in the Neonatal Mouse Model Is Associated With Pertussis Toxin-Mediated Pathology Beyond the Airways. Infect Immun (2017) 85(11):e00355–17. McCormick B, editor. doi: 10.1128/IAI.00355-17
25. Wolf MA, Boehm DT, DeJong MA, Wong TY, Sen-Kilic E, Hall JM, et al. Intranasal Immunization With Acellular Pertussis Vaccines Results in Long-Term Immunity to Bordetella Pertussis in Mice. Infect Immun (2021) 89(3):e00355–17. doi: 10.1128/IAI.00607-20
26. Boehm DT, Varney ME, Wong TY, Nowak ES, Sen-Kilic E, Hall J, et al. Characterizing the Innate and Adaptive Responses of Immunized Mice to Bordetella Pertussis Infection Using In Vivo Imaging and Transcriptomic Analysis. bioRxiv (2019):674408. doi: 10.1101/674408
27. Wilk MM, Misiak A, McManus RM, Allen AC, Lynch MA, Mills KHG. Lung CD4 Tissue-Resident Memory T Cells Mediate Adaptive Immunity Induced by Previous Infection of Mice With Bordetella Pertussis. J Immunol (2017) 199(1):233–43. doi: 10.4049/jimmunol.1602051
28. Wilk MM, Borkner L, Misiak A, Curham L, Allen AC, Mills KHG. Immunization With Whole Cell But Not Acellular Pertussis Vaccines Primes CD4 TRM Cells That Sustain Protective Immunity Against Nasal Colonization With Bordetella Pertussis. Emerg Microbes Infect (2019) 8(1):169. doi: 10.1080/22221751.2018.1564630
29. Allen AC, Wilk MM, Misiak A, Borkner L, Murphy D, Mills KHG. Sustained Protective Immunity Against Bordetella Pertussis Nasal Colonization by Intranasal Immunization With a Vaccine-Adjuvant Combination That Induces IL-17-Secreting T RM Cells. Mucosal Immunol (2018) 11(6):1763–76. doi: 10.1038/s41385-018-0080-x
30. Warfel JM, Merkel TJ. The Baboon Model of Pertussis: Effective Use and Lessons for Pertussis Vaccines. Expert Rev Vaccines (2014) 13(10):1241–52. doi: 10.1586/14760584.2014.946016
31. Pertussis Vaccination: Use of Acellular Pertussis Vaccines Among Infants and Young Children Recommendations of the Advisory Committee on Immunization Practices (ACIP) . Available at: https://www.cdc.gov/mmwr/preview/mmwrhtml/00048610.htm.
32. Merkel TJ, Halperin SA. Nonhuman Primate and Human Challenge Models of Pertussis. J Infect Dis (2014) 209(SUPPL. 1):S20–3. doi: 10.1093/infdis/jit493
33. Vaure C, Grégoire-Barou V, Courtois V, Chautard E, Dégletagne C, Liu Y. Göttingen Minipigs as a Model to Evaluate Longevity, Functionality, and Memory of Immune Response Induced by Pertussis Vaccines. Front Immunol (2021) 12:613810. doi: 10.3389/fimmu.2021.613810
34. Hall JM, Kang J, Kenney SM, Wong TY, Bitzer GJ, Kelly CO, et al. Re-Investigating the Coughing Rat Model of Pertussis to Understand Bordetella Pertussis Pathogenesis. Infect Immun (2021) 89:e00304–21. doi: 10.1101/2021.04.02.438291
35. Janeway CA Jr, Travers P, Walport M, Shlomchik MJ. Immunological Memory - Immunobiology - NCBI Bookshelf. In: Immunobiology: The Immune System in Health and Disease, 5th ed. New York: Garland Science (2001). Available at: https://www.ncbi.nlm.nih.gov/books/NBK27158/.
36. Stebegg M, Kumar SD, Silva-Cayetano A, Fonseca VR, Linterman MA, Graca L. Regulation of the Germinal Center Response. Front Immunol (2018) 9:2469. doi: 10.3389/fimmu.2018.02469
37. Victora GD, Nussenzweig MC. Germinal Centers. Annu Rev Immunol (2012) 30:429–57. doi: 10.1146/annurev-immunol-020711-075032
38. Schwickert TA, Victora GD, Fooksman DR, Kamphorst AO, Mugnier MR, Gitlin AD, et al. A Dynamic T Cell-Limited Checkpoint Regulates Affinity-Dependent B Cell Entry Into the Germinal Center. J Exp Med (2011) 208(6):1243–52. doi: 10.1084/jem.20102477
39. Gitlin AD, Shulman Z, Nussenzweig MC. Clonal Selection in the Germinal Centre by Regulated Proliferation and Hypermutation. Nature (2014) 509(7502):637–40. doi: 10.1038/nature13300
40. Crotty S. T Follicular Helper Cell Differentiation, Function, and Roles in Disease. Immunity (2014) 41:529–42. doi: 10.1016/j.immuni.2014.10.004
41. Kazanietz MG, Durando M, Cooke M. CXCL13 and its Receptor CXCR5 in Cancer: Inflammation, Immune Response, and Beyond. Front Endocrinol (2019) 10:471. doi: 10.3389/fendo.2019.00471
42. Havenar-Daughton C, Lindqvist M, Heit A, Wu JE, Reiss SM, Kendric K, et al. CXCL13 is a Plasma Biomarker of Germinal Center Activity. Proc Natl Acad Sci USA (2016) 113(10):2702–7. doi: 10.1073/pnas.1520112113
43. Ansel KM, Ngo VN, Hyman PL, Luther SA, Förster R, Sedgwlck JD, et al. A Chemokine-Driven Positive Feedback Loop Organizes Lymphoid Follicles. Nature (2000) 406(6793):309–14. doi: 10.1038/35018581
44. van Twillert I, Han WGH, van Els CACM. Waning and Aging of Cellular Immunity to Bordetella Pertussis. Pathog Dis (2015) 73(8):ftv071. doi: 10.1093/femspd/ftv071
45. Diavatopoulos DA, Edwards KM. What Is Wrong With Pertussis Vaccine Immunity? Cold Spring Harb Perspect Biol (2017) 9(12):a029553. doi: 10.1101/cshperspect.a029553
46. Buisman AM, De Rond CGH, Öztürk K, Ten Hulscher HI, Van Binnendijk RS. Long-Term Presence of Memory B-Cells Specific for Different Vaccine Components. Vaccine (2010) 28:179–86. doi: 10.1016/j.vaccine.2009.09.102
47. Leef M, Elkins KL, Barbic J, Shahin RD. Protective Immunity to Bordetella Pertussis Requires Both B Cells and Cd4+ T Cells for Key Functions Other Than Specific Antibody Production. J Exp Med (2000) 191(11):1841. doi: 10.1084/jem.191.11.1841
48. Wendelboe AM, Van Rie A, Salmaso S, Englund JA. Duration of Immunity Against Pertussis After Natural Infection or Vaccination. Pediatr Infect Dis J (2005) 24:S58–61. doi: 10.1097/01.inf.0000160914.59160.41
49. Brickman TJ, Armstrong SK. The Ornithine Decarboxylase Gene Odc is Required for Alcaligin Siderophore Biosynthesis in Bordetella Spp.: Putrescine Is a Precursor of Alcaligin. J Bacteriol (1996) 178(1):54. doi: 10.1128/jb.178.1.54-60.1996
50. Parker CD, Doyle S, Field LH, Hewlett E. Variability in Derivative Strains of Bordetella Pertussis. Developments in Biological Standardization (1980) 45:119–27.
51. Stainer DW, Scholte MJ. A Simple Chemically Defined Medium for the Production of Phase I Bordetella Pertussis. Microbiology (1970) 63(2):211–20. doi: 10.1099/00221287-63-2-211
52. Boehm DT, Hall JM, Wong TY, Divenere AM, Sen-Kilic E, Bevere JR, et al. Evaluation of Adenylate Cyclase Toxoid Antigen in Acellular Pertussis Vaccines by Using a Bordetella Pertussis Challenge Model in Mice. Infect Immun (2018) 86(10):e00857–17. doi: 10.1128/IAI.00857-17
53. Sen-Kilic E, Blackwood CB, Huckaby AB, Horspool AM, Weaver KL, Malkowski AC, et al. Defining the Mechanistic Correlates of Protection Conferred by Whole-Cell Vaccination Against Pseudomonas Aeruginosa Acute Murine Pneumonia. Infect Immun (2021) 89(2):e00451–20. Palmer GH, editor. doi: 10.1128/IAI.00451-20
54. Blackwood CB, Sen-Kilic E, Boehm DT, Hall JM, Varney ME, Wong TY, et al. Innate and Adaptive Immune Responses Against Bordetella Pertussis and Pseudomonas Aeruginosa in a Murine Model of Mucosal Vaccination Against Respiratory Infection. Vaccines (2020) 8(4):1–21. doi: 10.3390/vaccines8040647
55. Winter K, Zipprich J, Harriman K. Pertussis in California: A Tale of 2 Epidemics. Pediatr Infect Dis J (2018) 37(4):324–8. doi: 10.1097/INF.0000000000001761
56. Plotkin SA. Correlates of Protection Induced by Vaccination. Clin Vaccine Immunol (2010) 17(7):1055–65. doi: 10.1128/CVI.00131-10
57. Damron FH, Barbier M, Dubey P, Edwards KM, Gu X-X, Klein NP, et al. Overcoming Waning Immunity in Pertussis Vaccines: Workshop of the National Institute of Allergy and Infectious Diseases. J Immunol (2020) 205(4):877–82. doi: 10.4049/jimmunol.2000676
58. Scanlon K, Skerry C, Carbonetti N. Association of Pertussis Toxin With Severe Pertussis Disease. Toxins (2019) 11:373. doi: 10.3390/toxins11070373
59. Spensieri F, Siena E, Borgogni E, Zedda L, Cantisani R, Chiappini N, et al. Early Rise of Blood T Follicular Helper Cell Subsets and Baseline Immunity as Predictors of Persisting Late Functional Antibody Responses to Vaccination in Humans. PloS One (2016) 11(6):e0157066. doi: 10.1371/journal.pone.0157066
60. Taranger J, Trollfors B, Lagergård T, Sundh V, Bryla DA, Schneerson R, et al. Correlation Between Pertussis Toxin IgG Antibodies in Postvaccination Sera and Subsequent Protection Against Pertussis. J Infect Dis (2000) 181:1010–3. doi: 10.1086/315318
61. Gregg KA, Merkel TJ. Pertussis Toxin: A Key Component in Pertussis Vaccines? Toxins (Basel) (2019) 11(10):557. doi: 10.3390/toxins11100557
62. Katada T, Tamura M, Ui M. The A Protomer of Islet-Activating Protein, Pertussis Toxin, as an Active Peptide Catalyzing ADP-Ribosylation of a Membrane Protein. Arch Biochem Biophys (1983) 224(1):290–8. doi: 10.1016/0003-9861(83)90212-6
63. Plaut RD, Carbonetti NH. Retrograde Transport of Pertussis Toxin in the Mammalian Cell. Cell Microbiol (2008) 10(5):1130–9. doi: 10.1111/j.1462-5822.2007.01115.x
64. Witvliet MH, Burns DL, Brennan MJ, Poolman JT, Manclark CR. Binding of Pertussis Toxin to Eucaryotic Cells and Glycoproteins. Infect Immun (1989) 57(11):3324. doi: 10.1128/iai.57.11.3324-3330.1989
65. Tamura M, Nogimori K, Murai S, Yajima M, Ito K, Katada T, et al. Subunit Structure of Islet-Activating Protein, Pertussis Toxin, in Conformity With the A-B Model. Biochemistry (1982) 21(22):5516–22. doi: 10.1021/bi00265a021
66. Carbonetti NH. Pertussis Toxin and Adenylate Cyclase Toxin: Key Virulence Factors of Bordetella Pertussis and Cell Biology Tools. Future Microbiol (2010) 5(3):455. doi: 10.2217/fmb.09.133
67. Hammarlund E, Thomas A, Poore EA, Amanna IJ, Rynko AE, Mori M, et al. Durability of Vaccine-Induced Immunity Against Tetanus and Diphtheria Toxins: A Cross-Sectional Analysis. Clin Infect Dis (2016) 62(9):1111. doi: 10.1093/cid/ciw066
68. Kallies A, Hasbold J, Tarlinton DM, Dietrich W, Corcoran LM, Hodgkin PD, et al. Plasma Cell Ontogeny Defined by Quantitative Changes in Blimp-1 Expression. J Exp Med (2004) 200(8):967. doi: 10.1084/jem.20040973
69. Budroni S, Buricchi F, Cavallone A, Bourguignon P, Caubet M, Dewar V, et al. Antibody Avidity, Persistence, and Response to Antigen Recall: Comparison of Vaccine Adjuvants. NPJ Vaccines (2021) 6(1):1–11. doi: 10.1038/s41541-021-00337-0
70. MacLennan ICM. Germinal Centers. Annu Rev Immunol (1994) 12(1):117–39. doi: 10.1146/annurev.iy.12.040194.001001
71. Crotty S. T Follicular Helper Cell Biology: A Decade of Discovery and Diseases. Immunity (2019) 50(5):1132. doi: 10.1016/j.immuni.2019.04.011
72. Crotty S. Follicular Helper CD4 T Cells (TFH). Annu Rev Immunol (2011) 29:621–63. doi: 10.1146/annurev-immunol-031210-101400
73. Schaerli P, Willimann K, Lang AB, Lipp M, Loetscher P, Moser B. Cxc Chemokine Receptor 5 Expression Defines Follicular Homing T Cells With B Cell Helper Function. J Exp Med (2000) 192(11):1553. doi: 10.1084/jem.192.11.1553
74. Iyer SS, Latner DR, Zilliox MJ, Mccausland M, Akondy RS, Penaloza-Macmaster P, et al. Identification of Novel Markers for Mouse CD4 T Follicular Helper Cells. Eur J Immunol (2013) 43(12):3219. doi: 10.1002/eji.201343469
75. Ueno H. Tfh Cell Response in Influenza Vaccines in Humans: What is Visible and What Is Invisible. Curr Opin Immunol (2019) 59:9. doi: 10.1016/j.coi.2019.02.007
76. Yin M, Xiong Y, Liang D, Tang H, Hong Q, Liu G, et al. Circulating Tfh Cell and Subsets Distribution Are Associated With Low-Responsiveness to Hepatitis B Vaccination. Mol Med (2021) 27(1):1–12. doi: 10.1186/s10020-021-00290-7
77. Helmold Hait S, Hogge CJ, Rahman MA, Hunegnaw R, Mushtaq Z, Hoang T, et al. TFH Cells Induced by Vaccination and Following SIV Challenge Support Env-Specific Humoral Immunity in the Rectal-Genital Tract and Circulation of Female Rhesus Macaques. Front Immunol (2021) 11:3593. doi: 10.3389/fimmu.2020.608003
78. Palm AKE, Henry C. Remembrance of Things Past: Long-Term B Cell Memory After Infection and Vaccination. Front Immunol (2019) 10:1787. NLM (Medline). doi: 10.3389/fimmu.2019.01787
79. Shlomchik Mary M Tomayko MJ, Steinel NC. Murine Memory B Cell Subsets Cutting Edge: Hierarchy of Maturity of Downloaded From. J Immun (2021) 185:7146–50. doi: 10.4049/jimmunol.10021163
80. Zuccarino-Catania GV, Sadanand S, Weisel FJ, Tomayko MM, Meng H, Kleinstein SH, et al. CD80 and PD-L2 Define Functionally Distinct Memory B Cell Subsets That Are Independent of Antibody Isotype. Nat Immunol (2014) 15(7):631–7. doi: 10.1038/ni.2914
81. Riedel R, Addo R, Ferreira-Gomes M, Heinz GA, Heinrich F, Kummer J, et al. Discrete Populations of Isotype-Switched Memory B Lymphocytes Are Maintained in Murine Spleen and Bone Marrow. Nat Commun (2020) 11(1):1–14. doi: 10.1038/s41467-020-16464-6
82. Vences-Catalán F, Santos-Argumedo L. CD38 Through the Life of a Murine B Lymphocyte. IUBMB Life (2011) 63(10):840–6. doi: 10.1002/iub.549
83. Cockayne DA, Muchamuel T, Grimaldi JC, Muller-Steffner H, Randall TD, Lund FE, et al. Mice Deficient for the Ecto-Nicotinamide Adenine Dinucleotide Glycohydrolase CD38 Exhibit Altered Humoral Immune Responses. Blood (1998) 92(4):1324–33. doi: 10.1182/blood.V92.4.1324
84. Lund FE, Muller-Steffner H, Romero-Ramirez H, Moreno-García ME, Partida-Sánchez S, Makris M, et al. CD38 Induces Apoptosis of a Murine Pro-B Leukemic Cell Line by a Tyrosine Kinase-Dependent But ADP-Ribosyl Cyclase- and NAD Glycohydrolase-Independent Mechanism. Int Immunol (2006) 18(7):1029–42. doi: 10.1093/intimm/dxl037
85. Good-Jacobson KL, Song E, Anderson S, Sharpe AH, Shlomchik MJ. CD80 Expression on B Cells Regulates Murine T Follicular Helper Development, Germinal Center B Cell Survival and Plasma Cell Generation. J Immunol (2012) 188(9):4217. doi: 10.4049/jimmunol.1102885
86. Nguyen DC, Joyner CJ, Sanz I, Lee FEH. Factors Affecting Early Antibody Secreting Cell Maturation Into Long-Lived Plasma Cells. Front Immunol (2019) 10:2138. doi: 10.3389/fimmu.2019.02138
87. Mårtensson I-L, Rimkute I, Brynjolfsson SF, Olsen Ekerhult T, Persson Berg L, Wick M-J, et al. Long-Lived Plasma Cells in Mice and Men. Front Immunol (2018) 9(November):1–7. doi: 10.3389/fimmu.2018.02673
88. Higgs R, Higgins SC, Ross PJ, Mills KHG. Immunity to the Respiratory Pathogen Bordetella Pertussis. Mucosal Immunol (2012) 5(5):485–500. doi: 10.1038/mi.2012.54
89. Guiso N, Capiau C, Carletti G, Poolman J, Hauser P. Intranasal Murine Model of Bordetella Pertussis Infection. I. Prediction of Protection in Human Infants by Acellular Vaccines. Vaccine (1999) 17(19):2366–76. doi: 10.1016/s0264-410x(99)00037-7
90. Boursaux-Eude C, Thiberge S, Carletti G, Guiso N. Intranasal Murine Model of Bordetella Pertussis Infection: II. Sequence Variation and Protection Induced by a Tricomponent Acellular Vaccine. Vaccine (1999) 17(20–21):2651–60. doi: 10.1016/s0264-410x(99)00037-9
91. Denoël P, Godfroid F, Guiso N, Hallander H, Poolman J. Comparison of Acellular Pertussis Vaccines-Induced Immunity Against Infection Due to Bordetella Pertussis Variant Isolates in a Mouse Model. Vaccine (2005) 23(46–47):5333–41. doi: 10.1016/j.vaccine.2005.06.021
92. Mills KHG, Ryan M, Ryan E, Mahon BP. A Murine Model in Which Protection Correlates With Pertussis Vaccine Efficacy in Children Reveals Complementary Roles for Humoral and Cell- Mediated Immunity in Protection Against Bordetella Pertussis. Infect Immun (1998) 66(2):594–602. doi: 10.1128/IAI.66.2.594-602.1998
93. Decker MD, Edwards K, Steinhoff MC, Rennels M, Pichichero M, Englund J, et al. Comparison of 13 Acellular Pertussis Vaccines. Pediatrics (1995) 96(3):557–6. doi: 10.1542/peds.96.3.557
94. Sato Y, Kimura M, Fukumi H. Development of a Pertussis Component Vaccine in Japan. Lancet (1984) 1:122–6. doi: 10.1016/S0140-6736(84)90061-8
95. WHO. Report of the Meeting on the Results of the WHO Collaborative Study of the Acellular DTP Vaccine. (1984). Unpublished WHO document WHO/BVI/PERT/84.1.
96. De Wilt D, DeJong Y, Nikjkamp F, Kreeftenberg J. Pharmacological Evaluation of Purified Component and Whole Cell Pertussis Vaccine in the Cardiovascular System of Rats. J Pharmacol Exp Ther (1985) 232:541–4.
97. Edwards KM, Meade BD, Decker MD, Reed GF, Rennels MB, Steinhoff MC, et al. Comparison of 13 Acellular Pertussis Vaccines: Overview and Serologic Response. Pediatrics (1995) 96(3):548–57. doi: 10.1542/peds.96.3.548
98. Liu BC, McIntyre P, Kaldor JM, Quinn HE, Ridda I, Banks E. Pertussis in Older Adults: Prospective Study of Risk Factors and Morbidity. Clin Infect Dis (2012) 55(11):1450–6. doi: 10.1093/cid/cis627
99. McGuiness CB, Hill J, Fonseca E, Hess G, Hitchcock W, Krishnarajah G. The Disease Burden of Pertussis in Adults 50 Years Old and Older in the United States: A Retrospective Study. BMC Infect Dis (2013) 13(1):32. doi: 10.1186/1471-2334-13-32
100. Elahi S, Buchanan RM, Attah-Poku S, Townsend HGG, Babiuk LA, Gerdts V. The Host Defense Peptide Beta-Defensin 1 Confers Protection Against Bordetella Pertussis in Newborn Piglets. Infect Immun (2006) 74(4):2338. doi: 10.1128/IAI.74.4.2338-2352.2006
101. Weyrich LS, Feaga HA, Park J, Muse SJ, Safi CY, Rolin OY, et al. Resident Microbiota Affect Bordetella Pertussis Infectious Dose and Host Specificity. J Infect Dis (2014) 209(6):913. doi: 10.1093/infdis/jit597
102. Dutta S, Sengupta P. Men and Mice: Relating Their Ages. Life Sci (2015) 152:244–8. doi: 10.1016/j.lfs.2015.10.025
103. Mahon BP, Brady MT, Mills KHG. Protection Against Bovdetella Pertussis in Mice in the Absence of Detectable Circulating Antibody: Implications for Long-Term Immunity in Children. J Infect Dis (2000) 181(6):2087–91. doi: 10.1086/315527
104. Feunou PF, Kammoun H, Debrie A-S, Mielcarek N, Locht C. Long-Term Immunity Against Pertussis Induced by a Single Nasal Administration of Live Attenuated B. Pertussis BPZE1. Vaccine (2010) 28:7047–53. doi: 10.1016/j.vaccine.2010.08.017
105. Dubois V, Chatagnon J, Thiriard A, Bauderlique-Le Roy H, Debrie AS, Coutte L, et al. Suppression of Mucosal Th17 Memory Responses by Acellular Pertussis Vaccines Enhances Nasal Bordetella Pertussis Carriage. NPJ Vaccines (2021) 6(1):1–10. doi: 10.1038/s41541-020-00270-8
106. Holubová J, Staněk O, Brázdilová L, Mašín J, Bumba L, Gorringe AR, et al. Acellular Pertussis Vaccine Inhibits Bordetella Pertussis Clearance From the Nasal Mucosa of Mice. Vaccines (2020) 8(4):1–20. doi: 10.3390/vaccines8040695
107. Le T, Cherry JD, Chang S-J, Knoll MD, Lee ML, Barenkamp S, et al. Immune Responses and Antibody Decay After Immunization of Adolescents and Adults With an Acellular Pertussis Vaccine: The APERT Study. J Infect Dis (2004) 190(3):535–44. doi: 10.1086/422035
108. Acquaye-Seedah E, Reczek EE, Russell HH, DiVenere AM, Sandman SO, Collins JH, et al. Characterization of Individual Human Antibodies That Bind Pertussis Toxin Stimulated by Acellular Immunization. Infect Immun (2018) 86(6):e00004–18. doi: 10.1128/IAI.00004-18
109. Amanna IJ, Carlson NE, Slifka MK. Duration of Humoral Immunity to Common Viral and Vaccine Antigens. N Engl J Med (2009) 357(19):1903–15. doi: 10.1056/NEJMoa066092
110. Podda A, Carapella De Luca E, Contu B, Furlan R, Maida A, Moiraghi A, et al. Comparative Study of a Whole-Cell Pertussis Vaccine and a Recombinant Acellular Pertussis Vaccine. J Pediatr (1994) 124(6):921–6. doi: 10.1016/S0022-3476(05)83181-6
111. Greco D, Salmaso S, Mastrantonio P, Giuliano M, Tozzi AE, Anemona A, et al. A Controlled Trial of Two Acellular Vaccines and One Whole-Cell Vaccine Against Pertussis. N Engl J Med (2009) 334(6):341–9. doi: 10.1056/NEJM199602083340601
112. Marcellini V, Mortari EP, Fedele G, Gesualdo F, Pandolfi E, Midulla F, et al. Protection Against Pertussis in Humans Correlates to Elevated Serum Antibodies and Memory B Cells. Front Immunol (2017) 8(SEP). doi: 10.3389/fimmu.2017.01158
113. Corsiero E, Nerviani A, Bombardieri M, Pitzalis C. Ectopic Lymphoid Structures: Powerhouse of Autoimmunity. Front Immunol (2016) 7(OCT):17. doi: 10.3389/fimmu.2016.00430
114. Finch DK, Ettinger R, Karnell JL, Herbst R, Sleeman MA. Effects of CXCL13 Inhibition on Lymphoid Follicles in Models of Autoimmune Disease. Eur J Clin Invest (2013) 43(5):501–9. doi: 10.1111/eci.12063
115. Weaver KL, Blackwood CB, Horspool AM, Pyles GM, Sen-Kilic E, Grayson EM, et al. Long-Term Analysis of Pertussis Vaccine Immunity Uncovers a Memory B Cell Response to Whole Cell Pertussis Immunization That Is Absent From Acellular Immunized Mice. bioRxiv (2021), 2021.10.01.462695. doi: 10.1101/2021.10.01.462695v1
Keywords: Bordetella pertussis, vaccine development, TFH cells, CXCL13, germinal centers, humoral immunity, immunological memory, B memory cells
Citation: Weaver KL, Blackwood CB, Horspool AM, Pyles GM, Sen-Kilic E, Grayson EM, Huckaby AB, Witt WT, DeJong MA, Wolf MA, Damron FH and Barbier M (2022) Long-Term Analysis of Pertussis Vaccine Immunity to Identify Potential Markers of Vaccine-Induced Memory Associated With Whole Cell But Not Acellular Pertussis Immunization in Mice. Front. Immunol. 13:838504. doi: 10.3389/fimmu.2022.838504
Received: 17 December 2021; Accepted: 19 January 2022;
Published: 08 February 2022.
Edited by:
Daniela F. Hozbor, Universidad Nacional de La Plata, ArgentinaReviewed by:
Karl O. A. Yu, University at Buffalo, United StatesAndrew Gorringe, Public Health England, United Kingdom
Bahaa Abu Raya, Children’s & Women’s Health Centre of British Columbia, Canada
Copyright © 2022 Weaver, Blackwood, Horspool, Pyles, Sen-Kilic, Grayson, Huckaby, Witt, DeJong, Wolf, Damron and Barbier. This is an open-access article distributed under the terms of the Creative Commons Attribution License (CC BY). The use, distribution or reproduction in other forums is permitted, provided the original author(s) and the copyright owner(s) are credited and that the original publication in this journal is cited, in accordance with accepted academic practice. No use, distribution or reproduction is permitted which does not comply with these terms.
*Correspondence: Mariette Barbier, mabarbier@hsc.wvu.edu
†These authors have contributed equally to this work